- 1Laboratorio de Genómica Funcional, Facultad de Ciencias, Instituto de Bioquímica y Microbiología, Universidad Austral de Chile, Valdivia, Chile
- 2ANID–Millennium Science Initiative–Millennium Institute for Integrative Biology (iBIO), Santiago, Chile
In the budding yeast Saccharomyces cerevisiae, the FUN-LOV (FUNgal Light Oxygen and Voltage) optogenetic switch enables high levels of light-activated gene expression in a reversible and tunable fashion. The FUN-LOV components, under identical promoter and terminator sequences, are encoded in two different plasmids, which limits its future applications in wild and industrial yeast strains. In this work, we aim to expand the molecular versatility of the FUN-LOV switch to increase its biotechnological applications. Initially, we generated new variants of this system by replacing the promoter and terminator sequences and by cloning the system in a single plasmid (FUN-LOVSP). In a second step, we included the nourseothricin (Nat) or hygromycin (Hph) antibiotic resistances genes in the new FUN-LOVSP plasmid, generating two new variants (FUN-LOVSP-Nat and FUN-LOVSP-Hph), to allow selection after genome integration. Then, we compared the levels of light-activated expression for each FUN-LOV variants using the luciferase reporter gene in the BY4741 yeast strain. The results indicate that FUN-LOVSP-Nat and FUN-LOVSP-Hph, either episomally or genome integrated, reached higher levels of luciferase expression upon blue-light stimulation compared the original FUN-LOV system. Finally, we demonstrated the functionality of FUN-LOVSP-Hph in the 59A-EC1118 wine yeast strain, showing similar levels of reporter gene induction under blue-light respect to the laboratory strain, and with lower luciferase expression background in darkness condition. Altogether, the new FUN-LOV variants described here are functional in different yeast strains, expanding the biotechnological applications of this optogenetic tool.
1 Introduction
Optogenetics technology began in neurobiology, where light-activated membrane transporters (bacterial channelrhodopsin-2) were used to control neurons upon light stimulation (Boyden et al., 2005; Deisseroth, 2011). After this pioneering experiment, the use of light as an activator of biological processes has grown considerably, largely due to its numerous advantages, including precise spatiotemporal resolution, moderated toxic effects, and the ability to replace the use of toxic and expensive chemical inducers (Shimizu-Sato et al., 2002; Wang et al., 2012). Optogenetics is based on photosensitive proteins known as photoreceptors, which are capable of light sensing at different wavelengths (Losi et al., 2018; Tang et al., 2021). Currently, optogenetic tools include a large set of different photoreceptors, which have been implemented in a variety of biological platforms (Kolar et al., 2018), including microorganisms such as the budding yeast Saccharomyces cerevisiae (Salinas et al., 2017; Figueroa et al., 2021).
Yeast is a prominent chassis for optogenetics due to the absence of photoreceptors encoded in its genome (Goffeau et al., 1996), enabling the use of light to orthogonally control biological processes. Furthermore, yeast is a well-known model organism for cellular and molecular biology studies, and a cell factory for the production of valuable proteins and metabolites (Nielsen, 2019). In yeast, optogenetics has proved its utility in the control of multiple cellular processes and biotechnologically relevant phenotypes, such as: gene expression, subcellular protein localization, protein-protein interaction, cell cycle, cell spatial organization, metabolic rewiring, and heterologous protein production (Shimizu-Sato et al., 2002; Tyszkiewicz and Muir, 2008; Witte et al., 2017; Xu et al., 2018; Figueroa et al., 2021; Moreno Morales et al., 2021; Hoffman et al., 2022).
Among the multiplicity of optogenetic tools developed in yeast, optogenetic switches enable light-controlled gene expression of any target gene (Salinas et al., 2017; Figueroa et al., 2021). Optogenetic switches are typically based on the Yeast Two-Hybrid system (Y2H) (Fields and Song, 1989), where light triggers photoreceptor self-dimerization, protein-protein interaction between different photoreceptors (i.e heterodimerization), or interaction between the photoreceptor and its interacting partner, which then result in activation of target gene expression (Salinas et al., 2017; Figueroa et al., 2021). For instance, the yLightOn optogenetic switch is based on self-dimerization, through the LOV (Light-Oxygen and Voltage) domain, of the blue-light photoreceptor Vivid (VVD) from Neurospora crassa, which has been used to enable light-mediated activation of the yeast cell cycle (Xu et al., 2018). Similarly, the OptoEXP, OptoINVT, and OptoAMP systems are based on self-dimerization of the EL222, a LOV-containing blue-light photoreceptor from Erythrobacter litoralis, which has been used to control the yeast metabolism and production of valuable metabolites upon light stimulation (Zhao et al., 2018; Zhao et al., 2020; Zhao et al., 2021).
Heterodimerization of blue-light photoreceptors has been also used in yeast optogenetics (Salinas et al., 2018). For instance, the FUN-LOV (FUNgal Light-Oxygen-Voltage) optogenetic switch is based on Y2H and the light-mediated interaction of LOV domains from the N. crassa blue-light photoreceptors WC-1 and VVD (Salinas et al., 2018). This switch has been used for light-dependent transcriptional activation of different genes, including the luciferase reporter gene (Luc), flocculation-associated genes (FLO1 and FLO11), and the heterologous protein limonene synthase (Salinas et al., 2018). Recently, we have updated the FUN-LOV switch to place its components under the control of a strong promoter (TDH3) in low copy number plasmids (pRS313 and pRS315) (Romero et al., 2021). This optimization led to a FUN-LOV variant termed FUN-LOVLS (Low copy number and Strong promoter), which resulted in a 10-fold increase in the levels of light-mediated target gene activation (Romero et al., 2021). One limitation of these systems, however, is that the components are encoded in two different plasmids, both of which require auxotrophic selection in the laboratory strain BY4741 (Salinas et al., 2018; Romero et al., 2021). Furthermore, the plasmids encoding the FUN-LOV system contain the same promoter and terminator sequences, restricting its genome integration by homologous recombination, and compromising the genetic stability of the system in the absence of auxotrophic selection (Salinas et al., 2018). Therefore, while the FUN-LOV system represents a powerful tool, various elements may limit its potential use for new applications in wild or industrial yeast strains.
In this work, we report a molecular redesign of FUN-LOV, encoding its components in a single plasmid (FUN-LOVSP), using different promoter and terminator sequences, and including the nourseothricin (NatMx) and hygromycin (HphMx) antibiotic resistances as selectable markers. These FUN-LOV variants (FUN-LOVSP-Nat and FUN-LOVSP-Hph) showed similar levels of light-activated gene expression of the luciferase reporter compared to the FUN-LOVLS and higher than the original FUN-LOV system. Furthermore, we integrate the FUN-LOVSP-Nat and FUN-LOVSP-Hph variants into the HO locus of the BY4741 strain, confirming the feasibility of stable and functional integration in the yeast genome. Finally, as proof of concept, we evaluate the functionality of the FUN-LOVSP-Hph variant in the 59A-EC1118 wine yeast strain, showing similar levels of reporter gene induction upon blue-light stimulation compared to the laboratory strain, and with a lower background expression in darkness condition. Overall, we have reduced the molecular biology limitations of FUN-LOV, expanding its potential applications to wild and industrial yeast strains.
2 Materials and methods
2.1 Yeast strains and culture conditions
Two S. cerevisiae strains derived from the BY4741 genetic background (MATa; his3∆1; leu2∆0; met15∆0; ura3∆0, gal3∆::KanMxRv-PGAL1-Luc or P5XGAL1-Luc) were used in the experiments with FUN-LOV, FUN-LOVLS, FUN-LOVSP, FUN-LOVSP-Nat, and FUN-LOVSP-Hph. These strains carry the firefly luciferase reporter (Luc) gene controlled by either GAL1 promoter or a 5XGAL1 synthetic promoter (five repetitions of the Gal4 Upstream Activating Sequence) integrated into the GAL3 locus (Salinas et al., 2018). The 59A-EC1118 wine yeast strain was used for transformation and genome integration of the FUN-LOVSP-Hph variant into the HO locus. Furthermore, the Luc reporter regulated by the 5XGAL1 promoter was integrated into the GAL3 locus of the 59A-EC1118 strain. This strain is a haploid derivative from the EC1118 commercial wine strain previously described (Ambroset et al., 2011). The strains were maintained in YPDA medium (2% glucose, 2% peptone, 1% yeast extract, and 2% agar) at 30°C. For yeast genome integration of FUN-LOVSP-Nat and FUN-LOVSP-Hph, the YPDA medium was supplemented with 300 μg/ml of nourseothricin and 100 μg/ml of hygromycin, respectively. The yeast strains used and developed in this work are listed in the Supplementary Table S1.
2.2 Design and generation of genetic constructs
The FUN-LOVSP variant was designed in silico using the Geneious Prime software version 1.1 (Biomatters, New Zealand) and the online molecular biology platform Benchling (https://www.benchling.com/). The FUN-LOVSP (3927 bp) variant was then synthetized using the Genewiz synthesis service (Genewiz, United States ). The synthetic FUN-LOVSP was PCR amplified and cloned into the pRS316 and pRS426 plasmids using Yeast Recombinational Cloning (YRC) according to (Oldenburg et al., 1997). The FUN-LOVSP-Nat and FUN-LOVSP-Hph were constructed by PCR amplification of the FUN-LOVSP and the nourseothricin (NatMx) or hygromycin (HphMx) antibiotic resistances, respectively, cloning both PCR fragments into the pRS316 plasmid through YRC. Briefly, the PCR products were amplified with Phusion Flash High-Fidelity PCR Master Mix (ThermoFisher Scientific, United States ), using primers with 50 bp of overhangs between adjacent PCR fragments (Oldenburg et al., 1997). These PCR products were co-transformed with the linearized target plasmid into the BY4741 strain using the standard Lithium acetate transformation protocol (Gietz and Schiestl, 2007). The assembled plasmids were then transferred to E. coli and confirmed by standard colony PCR. Finally, the genetic constructs were confirmed by Sanger sequencing (Macrogen Inc., Republic of Korea). Integration of the FUN-LOVSP-Nat and FUN-LOVSP-Hph variants into the genome of the BY4741 strain was carried out by direct PCR amplification, transformation, and recombination with the HO locus, using primers with 50 bp of overhang to the target locus. A similar procedure was carried out for genome integration of FUN-LOVSP-Hph into the HO locus of the 59A-EC1118 wine yeast strain. Correct integration was confirmed by standard colony PCR using primers upstream and downstream the target locus. Plasmids and primers used and generated in this work are listed in Supplementary Tables S2, S3.
2.3 Luciferase expression and growth curves
Luciferase (Luc) was used as the reporter gene for light-activated gene expression (Salinas et al., 2018; Romero et al., 2021). Briefly, we used the destabilized version of the firefly luciferase, which has been optimized for real-time measurements of gene expression in yeast (Rienzo et al., 2012). The yeast strains carrying the Luc reporter and the different FUN-LOV variants were grown overnight in a 96-well plate format containing 200 µL of SC medium at 30°C. The next day, 20 µL of the overnight cultures were transferred to a 96-well plate with optical bottom (Nunc, ThermoFisher Scientific, United States ), containing 280 µL of SC media supplemented with 1 mM of luciferin. This plate was incubated at 25°C in a Synergy H1 microplate reader (Biotek, United States ) for simultaneous acquisition of Optical Density at 600 nm (OD600) and Luminescence (Lum) from each well every 10 min for 24 h (Romero et al., 2021). The Luc experiments were performed in three conditions: constant darkness (DD), constant blue-light illumination (BL), and a single blue-light pulse (BLP) of 2 h (h) duration (Romero et al., 2021; Rojas et al., 2022). In the DD assays, the 96-well plate was incubated inside the plate reader (dark) at 25°C with data acquisition of Lum and OD600 every 10 min. In the BL and BLP assays, the plate reader was programmed in a discontinuous kinetics protocol using the Gene5 software version 3.11 (Biotek, United States ). In the BL assays, the 96-well plate was incubated outside the plate reader (room temperature, 25°C) during 24-h for blue light illumination, inserting the 96-well plate automatically inside the equipment every 10 min for data acquisition of Lum and OD600. In the BLP assays, the 96-well plate was incubated inside the plate reader during 7-h (dark) at 25°C, then the 96-well plate was incubated outside of the equipment for blue light illumination during 2-h at room temperature (25°C), after the illumination, the 96-well plate was incubated again inside the equipment for 15-h. Data acquisition of Lum and OD600 in the BLP assays was performed every 10 min. The BL and BLP assays were performed using a LED blue-light illumination system developed by our research group (Supplementary Figure S1), which provide blue light at 466 nm with 24 μmol m−2 s−1 of light intensity, as was previously described (Romero et al., 2021). All the Luc expression assays were carried out in six biological replicates. The raw data of Luc expression measured as Lum in arbitrary units (a.u.) and the OD600 of the yeast cultures were graphed and used to generate the Supplementary Figures S2–S6. Based on these data sets, we normalized the luciferase expression dividing the Lum by the OD600 of the yeast cell cultures (Lum/OD600 nm), as described (Salinas et al., 2018).
2.4 Data analysis
The normalized Luc expression levels were used to compare the different FUN-LOV variants under a single BLP of 2-h duration (Romero et al., 2021). Furthermore, the average normalized Luc expression under DD condition and the fold-induction of Luc expression were also compared among FUN-LOV variants. The fold induction for each FUN-LOV variant was calculated according to the following equation:
Three parameters were statistically compared for each FUN-LOV variant respect to the original FUN-LOV system: 1) the maximal peak of Luc expression under BLP condition, 2) the average normalized Luc expression under DD condition, and 3) fold-induction of Luc expression for the GAL1 and 5XGAL1 promoters. Statistical comparisons were carried out using GraphPad Prism version 9.4.0, performing one-way ANOVA with Kruskal-Wallis multiple comparisons test.
3 Results
3.1 Molecular redesign of the FUN-LOV system
The components of the original FUN-LOV system and its variant FUN-LOVLS are encoded on two different plasmids, where both plasmids include the same promoter and terminator sequences (Figure 1A). This, makes genome integration of the systems difficult, limiting its application in wild and industrial yeast strains. Thus, we performed a rational molecular redesign and simplification of the original FUN-LOV system considering three elements: 1), the system must be encoded in a single plasmid to reduce plasmid burden (Karim et al., 2013); 2), the system should be devoid of repetitive elements such as the same promoter and terminator sequences for all components, facilitating its genome integration by homologous recombination; and 3), it must include an antibiotic resistance gene for selection after genome integration. The redesigned system, termed FUN-LOVSP (Single Plasmid) and described here, was synthesized, and cloned into the pRS316 (low copy number) or into pRS426 plasmid (high copy number) (Figure 1B). The FUN-LOVSP variant include two strong promoters, PGK1 (PPGK1) and PTDH3, and two terminators, ADH1 (ADH1ter) and CYC1 (CYC1ter) for its different components (Figure 1B). We also developed two versions that includes the nourseothricin (NatMx) and hygromycin (HphMx) antibiotic resistance cassettes in reverse direction to avoid polymerase collisions with PPGK1 (Figure 1B). These variants were named FUN-LOVSP-Nat and FUN-LOVSP-Hph, and considering that, we previously demonstrated that combining strong promoters with low copy number plasmids improves FUN-LOV performance (Romero et al., 2021), we cloned the FUN-LOVSP-Nat and FUN-LOVSP-Hph into the pRS316 plasmid (Figure 1B). Therefore, we generated three new FUN-LOV variants that reduce the molecular biology limitations of the original system.
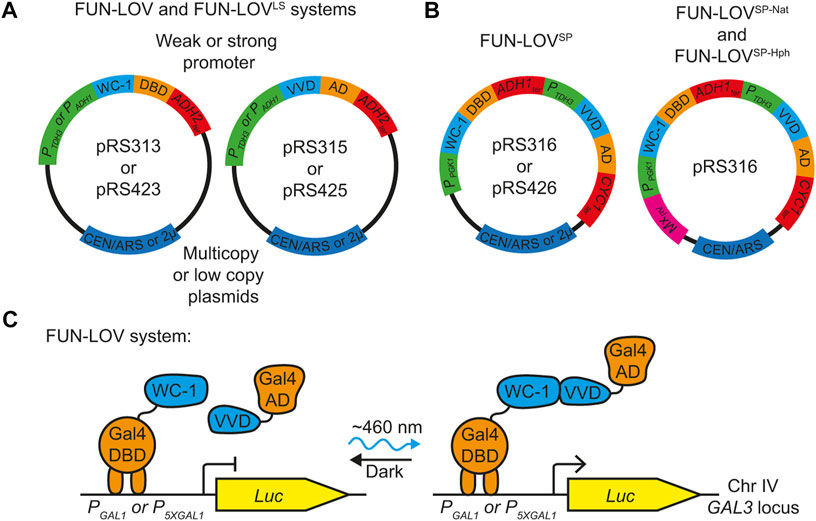
FIGURE 1. Molecular configuration of the FUN-LOV variants. (A) Plasmids encoding the original FUN-LOV switch. This system is encoded in two multicopy plasmids (pRS423 and pRS425), using the ADH1 promoter (PADH1) and ADH2 terminator (ADH2ter) for all the components. The FUN-LOVLS variant is encoded in two low-copy number plasmids (pRS313 and pRS315), and PADH1 was replaced by the TDH3 promoter (PTDH3). (B) The new FUN-LOV variants. The FUN-LOVSP system is encoded in a single multicopy plasmid (pRS426) or a single low-copy plasmid (pRS316). In addition, the FUN-LOVSP-Nat and FUN-LOVSP-Hph variants are carrying the nourseothricin and hygromycin antibiotic resistances in the reverse orientation (MxRv), respectively. (C) The original FUN-LOV system architecture. The Gal4 DNA-Binding Domain (DBD) is linked to the LOV domain of WC-1, and the Gal4-activation domain (AD) is tethered to the LOV domain of VVD. Under blue light, the interaction between the LOV domains of both proteins reconstructs the Gal4 transcription factor, resulting in the expression of luciferase (Luc). The Luc gene is integrated into the yeast genome (Chr IV) and is controlled by GAL1 (PGAL1) or 5XGAL1 (P5XGAL1) promoter.
The different FUN-LOV variants do not affect the Y2H-like architecture of the system, which is based on the light-mediated interaction of WC-1 and VVD (Figure 1C). Therefore, we expected the FUN-LOVSP, FUN-LOVSP-Nat, and FUN-LOVSP-Hph to show similar levels of light-dependent reporter gene expression to the FUN-LOV and FUN-LOVLS systems. To evaluate this, we compared the levels of light-controlled gene expression that can be achieved with each of the different FUN-LOV variants, using the luciferase (Luc) reporter regulated by the GAL1 promoter (PGAL1) or the 5XGAL1 (P5XGAL1) synthetic promoter, and integrated into the GAL3 locus (Salinas et al., 2018). Importantly, we selected a genome-integrated reporter gene to avoid a copy number variation effect on Luc expression. Each FUN-LOV variant was transformed episomally in the yeast strains carrying the Luc reporter (Figure 2A), and Luc expression was assayed under three experimental conditions: constant darkness (DD), constant blue-light (BL), and a single blue-light pulse (BLP) of 2-h duration. As expected, we observed that all the FUN-LOV variants resulted in low background levels of Luc expression in DD (Figures 2B,E). In contrast, under BL (Figures 2C,F) or BLP conditions (Figures 2D,G), high levels of Luc expression were observed. The background Luc expression in DD was higher for the P5XGAL1 promoter (compare Figures 2B,E), a phenomenon previously reported for this synthetic promoter (Salinas et al., 2018). Similarly, the Luc expression levels under BL and BLP were higher when the FUN-LOV variants targeted the P5XGAL1 promoter compared to the PGAL1 promoter (Figure 2). Remarkably, the FUN-LOVSP, FUN-LOVSP-Nat, FUN-LOVSP-Hph variants, in the context of a low copy number plasmid (pRS316), showed equivalent or higher levels of Luc expression under BL and BLP conditions than FUN-LOV and FUN-LOVLS systems (Figure 2). Interestingly, the FUN-LOVSP in the context of a high copy number plasmid (pRS426) exhibited growth defects (Supplementary Figures S2, S3), confirming our previous observation that expression of FUN-LOV using strong promoters in a high copy number plasmid impairs yeast growth (Romero et al., 2021). In addition, we observed differences in the 24-h kinetics of normalized Luc expression among FUN-LOV variants under BL condition (Figures 2C,F), which are explained by differences in the yeast growth curves (Supplementary Figures S2, S3). In conclusion, the molecular redesign of FUN-LOV described here (FUN-LOVSP, FUN-LOVSP-Nat, and FUN-LOVSP-Hph variants) increases Luc expression upon light stimulation.
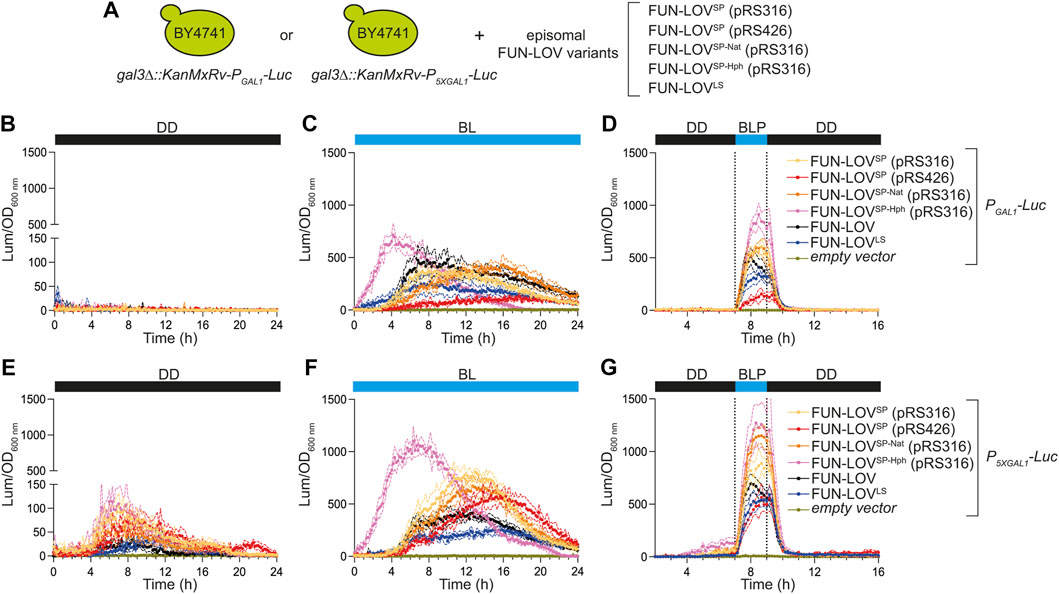
FIGURE 2. Luciferase expression in the BY4741 strains carrying different FUN-LOV variants episomally. (A) BY4741 yeast strains carrying the luciferase reporter gene (Luc) integrated into the genome and controlled by either the GAL1 (PGAL1) or the 5XGAL1 (P5XGAL1) promoters. These strains were transformed with different FUN-LOV variants episomally to evaluate the impact of these systems in light-regulated reporter gene expression. (B–G) The luciferase expression was measured as luminescence (Lum) and normalized by the Optical Density at 600 nm (OD600 nm) of the corresponding yeast cell culture. Three experimental conditions were assayed: (B,E) constant darkness (DD), (C,F) constant blue-light (BL), and (D, G) a single blue-light pulse (BLP) of 2-h duration (dotted lines). In all panels, the average of six biological replicates is shown, with the standard deviation represented by dashed lines of the corresponding color. (B–D) assays with the strain expressing Luc under PGAL1. (E–G) assays with strains expressing Luc under P5XGAL1. The BY4741 yeast strain with the pRS316 plasmid not encoding the optogenetic system was used as basal luminescence level (empty vector).
3.2 FUN-LOV variants show expanded functionality
Potential applications of FUN-LOVSP-Nat and FUN-LOVSP-Hph in wild or industrial yeast isolates, where auxotrophic selection is not available, depend on the capacity to integrate these variants into the yeast genome. Initially, we used the yeast strains carrying the Luc reporter to integrate the FUN-LOVSP-Nat and FUN-LOVSP-Hph into the HO locus (Figure 3A). We selected the HO locus for genome integration since its deletion do not affect the yeast growth (Baganz et al., 1997). In the reporter strains, we measured Luc expression levels under DD, BL, and BLP conditions, confirming that genome integrated copy of the FUN-LOVSP-Nat and FUN-LOVSP-Hph variants are functional (Figure 3; and Supplementary Figures S4, S5). The genome-integrated copy of FUN-LOVSP-Nat and FUN-LOVSP-Hph resulted in comparable levels of Luc expression to those observed with FUN-LOVSP-Nat and FUN-LOVSP-Hph cloned into a low-copy plasmid (pRS316) under BL and BLP conditions (compare Figures 2, 3). Interestingly, the genome integrated FUN-LOVSP-Hph variant resulted in higher Luc expression levels compared to the FUN-LOVSP-Nat variant in the BL and BLP conditions (Figure 3). The HphMx and NatMx cassettes included in the FUN-LOVSP-Hph and FUN-LOVSP-Nat variants contain the same promoter and terminator sequences (TEF); where the open reading frames of hph and nat1 have a high difference in the GC content, 58.7% and 70.7%, respectively (Goldstein and McCusker, 1999). This may explain the different levels of light-mediated gene expression of the Luc reporter obtained with the FUN-LOVSP-Hph and FUN-LOVSP-Nat variants (Figure 3). In addition, the FUN-LOVSP-Nat and FUN-LOVSP-Hph variants showed a different 24-h kinetics of Luc expression in BL condition (Figures 3C,F), which is due to differences in the growth curves between yeast strains (Supplementary Figures S4, S5). Therefore, the FUN-LOVSP-Nat and FUN-LOVSP-Hph variants can be integrated into the yeast genome and result in similar levels of target gene expression compared to episomally maintained copies, a finding that can simplify the implementation of this switch in multiple applications.
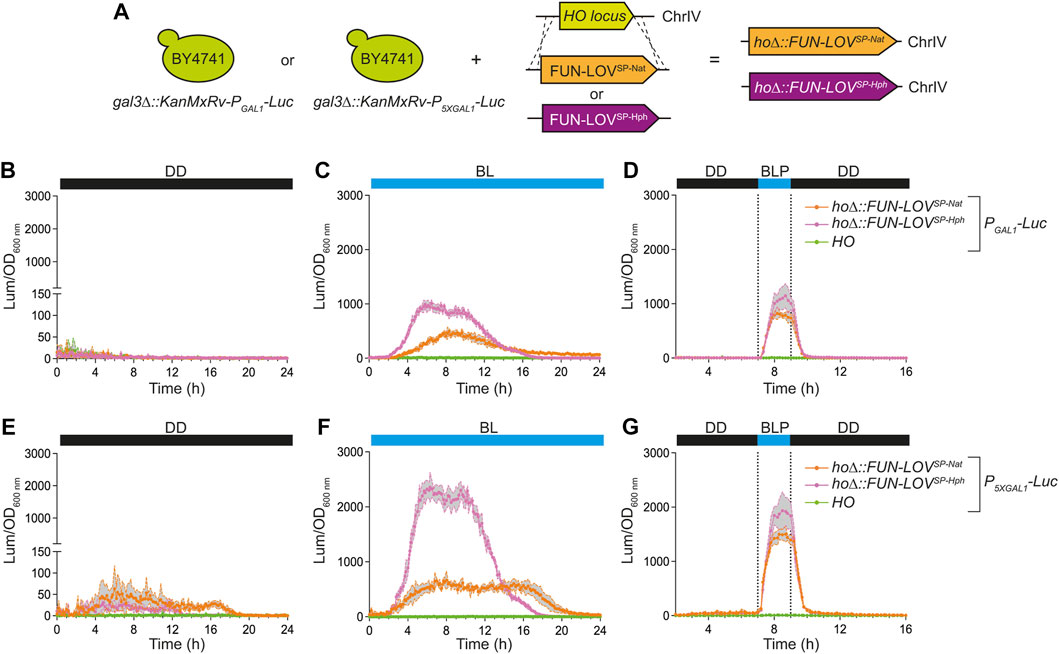
FIGURE 3. Luciferase expression in the BY4741 yeast strains carrying the FUN-LOVSP-Nat and FUN-LOVSP-Hph variants integrated into the genome. (A) BY4741 yeast strains carrying the luciferase reporter gene (Luc) integrated into the genome and controlled by GAL1 (PGAL1) or 5XGAL1 (P5XGAL1) promoters. In these strains the FUN-LOVSP-Nat and FUN-LOVSP-Hph variants were integrated into the HO locus. (B–G) The luciferase expression was measured as luminescence (Lum) and normalized by the Optical Density at 600 nm (OD600 nm) of the corresponding yeast cell culture. Three illumination conditions were assayed: (B,E) constant darkness (DD), (C,F) constant blue-light (BL), and (D,G) a single 2-h blue-light pulse (BLP) (dotted lines). In all panels, the average of six biological replicates is shown, with the standard deviation represented by a shaded region. (B–D) assays with the strain expressing Luc under PGAL1. (E–G) assays with strains expressing Luc under P5XGAL1.
As a proof of concept to demonstrate the applicability of the new FUN-LOV variants in a different genetic background, we demonstrated the FUN-LOVSP-Hph functionality in the 59A-EC1118 wine yeast strain. Initially, we inserted the Luc reporter controlled by the P5XGAL1 into the GAL3 locus as target gene to assess the FUN-LOVSP-Hph functionality. Then, we integrated the FUN-LOVSP-Hph into the HO locus of the 59A-EC1118 strain (Figure 4A). The 59A-EC1118 strain carrying the integrated copy of FUN-LOVSP-Hph was assayed under DD, BL, and BLP conditions (Figure 4 and Supplementary Figures S6). The results demonstrated that FUN-LOVSP-Hph is functional in the 59A-EC1118 wine yeast strain, showing high levels of Luc expression in BL and BLP conditions, with lower Luc expression in DD (Figure 4). Under BL and BLP conditions the Luc expression levels in the 59A-EC1118 strain resulted in comparable levels to those observed in the BY4741 strain (compare Figures 3, 4). Interestingly, under DD condition, 59A-EC1118 strain showed a lower Luc expression background respect to the BY4741 strain with the P5XGAL1 (Figures 3E, 4B), suggesting differences in the galactose genes regulation between these yeast strains. In conclusion, the FUN-LOVSP-Hph variant is functional in a wine yeast strain, confirming that we reduced the molecular biology limitations of the original system, and expanding the potential application of this optogenetic tool beyond the laboratory strain.
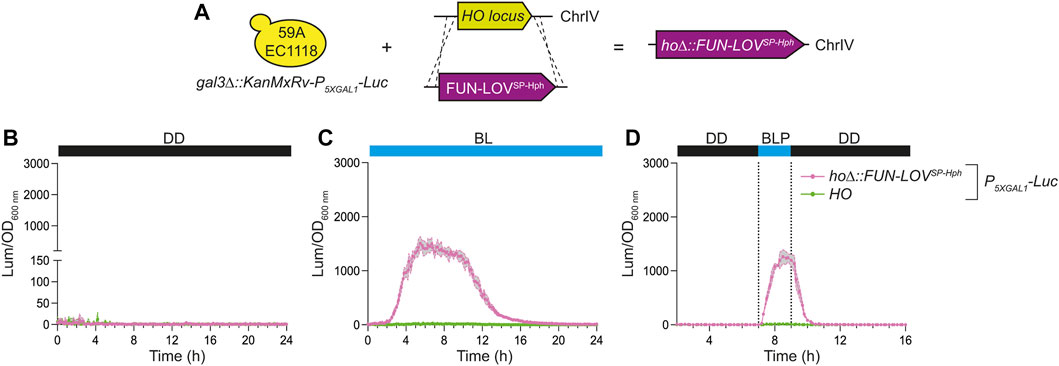
FIGURE 4. Luciferase expression in the 59A-EC1118 wine yeast strains carrying the FUN-LOVSP-Hph variant integrated into the genome. (A) The 59A-EC1118 wine yeast strain is carrying the luciferase reporter gene (Luc) integrated into the genome and controlled by the 5XGAL1 (P5XGAL1) promoter. In this strain, the FUN-LOVSP-Hph variant was integrated into the HO locus. (B,C) The luciferase expression was measured as luminescence (Lum) and normalized by the Optical Density at 600 nm (OD600 nm) of the corresponding yeast cell culture. Three illumination conditions were assayed: (B) constant darkness (DD), (C) constant blue-light (BL), and (D) a single 2-h blue-light pulse (BLP) (dotted lines). In all panels, the average of six biological replicates is shown, with the standard deviation represented by a shaded region.
3.3 Comparing transcriptional activation capacity among FUN-LOV variants
We compared the maximal level of Luc expression for each FUN-LOV variant respect to the original FUN-LOV system using the same illumination conditions: a single BLP of 2-h duration (see methods for details). Interestingly, the low-copy versions of FUN-LOVSP, FUN-LOVSP-Nat, and FUN-LOVSP-Hph variants showed higher levels of maximal Luc expression upon a single BLP compared to FUN-LOV and FUN-LOVSP (Figure 5A,B). Importantly, the copy of FUN-LOVSP-Nat and FUN-LOVSP-Hph integrated into the HO locus did not decrease the maximal luciferase expression, showing a similar behavior compared to the FUN-LOVSP-Nat and FUN-LOVSP-Hph encoded in the pRS316 plasmid (Figures 5A,B). In the BY4741 strain with the Luc reporter controlled by the P5XGAL1, the FUN-LOVSP-Nat and FUN-LOVSP-Hph variants resulted in higher Luc expression levels under the BLP condition compared to the BY4741 strain with Luc reporter regulated by PGAL1 (Figures 5A,B), but also showed a higher background of Luc expression in DD (Figure 5B). Interestingly, in the 59A-EC1118 strain with the Luc reporter controlled by the P5XGAL1, the Luc expression levels in DD and BLP conditions for FUN-LOVSP-Hph are comparable to those observed in the BY4741 strain with the Luc reporter regulated by the PGAL1 (Figures 5A,C). Thus, the results indicate that in the 59A-EC1118 genetic background the P5XGAL1 behaves as the canonical PGAL1.
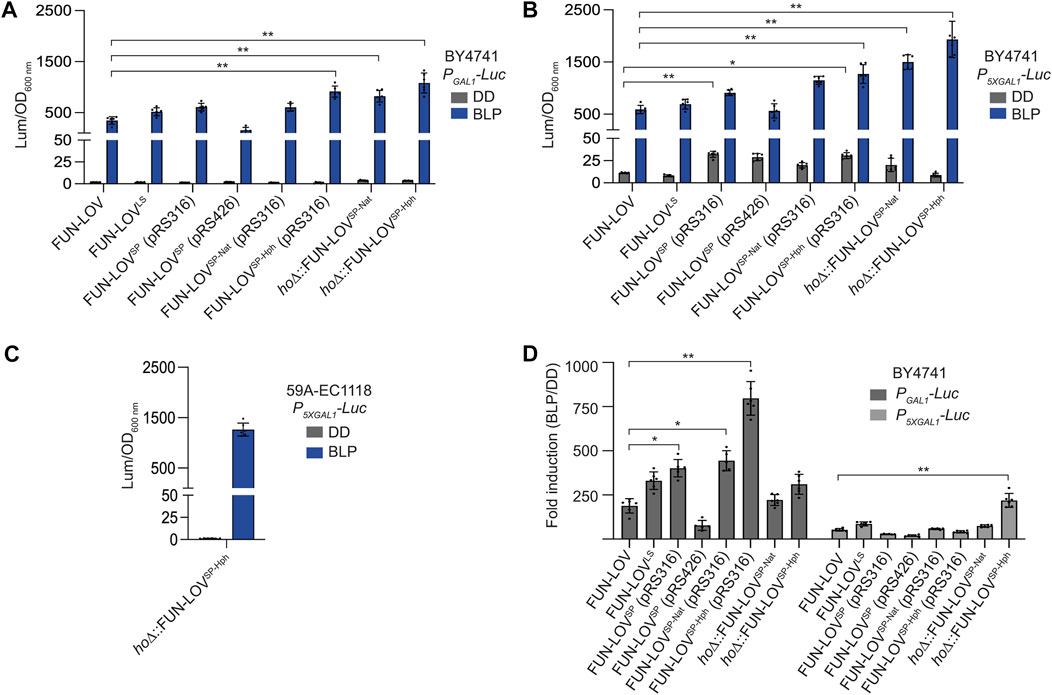
FIGURE 5. Dynamic range for different FUN-LOV variants. (A,B) Maximal normalized luciferase expression for each FUN-LOV variant upon a single 2-h blue-light pulse (BLP) and its average background expression in constant darkness condition (DD). Results for the BY4741 yeast strains carrying the luciferase reporter (Luc) gene controlled by PGAL1 (A) or P5XGAL1 (B) promoters are shown. (C) Maximal normalized luciferase expression for the FUN-LOVSP-Hph variant upon a single 2-h blue-light pulse (BLP) and its average background expression in constant darkness condition (DD). Results for the 59A-EC1118 wine yeast strains carrying the Luc reporter gene controlled by 5XGAL1 promoter (P5XGAL1) promoter is shown. (D) Fold induction of luciferase expression (BLP/DD) controlled by PGAL1 and P5XGAL1 promoters in the BY4741 strains carrying different FUN-LOV variants. The asterisk (*p < 0.05) and double asterisk (** p < 0.01) represents statistically significant differences using One-way ANOVA. In all panels, the average of six biological replicates with the standard deviation is shown.
We then calculated the fold induction of each system, dividing the maximal Luc expression in BLP condition by the average background of Luc expression in DD (see methods). As a result, we observed that FUN-LOVSP-Hph encoded in pRS316 plasmid is the system with higher fold-induction of Luc expression when PGAL1 is used (Figure 5D). Importantly, in all the FUN-LOV variants, the Luc fold-induction strongly decreased in the BY4741 yeast strain carrying the luciferase reporter controlled by the P5XGAL1 (Figure 5D). This is due to the higher Luc expression background observed in DD for the P5XGAL1 compared to PGAL1 (Figures 5A,B), a phenomenon previously reported for P5XGAL1 (Salinas et al., 2018). Interestingly, the Luc fold-induction calculated in the 59A-EC1118 wine yeast strain (FUN-LOVSP-Hph + P5XGAL1-Luc) is 982-fold, which is comparable to the fold-induction observed in the BY4741 strain with the FUN-LOVSP-Hph variant and Luc reporter regulated by the PGAL1 (Figure 5D). Altogether, the FUN-LOV variants developed in this work reached higher levels of Luc expression upon a single BLP and higher fold-induction of Luc expression compared to the previously described versions, increasing the dynamic range of this optogenetic system.
4 Discussion
The original FUN-LOV optogenetic switch is encoded in two different multicopy plasmids (Salinas et al., 2018). Recently, the FUN-LOV switch was subjected to a molecular optimization, whereby the system is encoded in two low copy number plasmids and its expression is controlled by the TDH3 promoter, in a variant known as FUN-LOVLS (Romero et al., 2021). This variant results in a 10-fold increase in the levels of luciferase expression upon blue-light stimulation. These experiments, however, were carried out using the FUN-LOVLS and the luciferase reporter encoded in multicopy plasmids (Romero et al., 2021), which can result in copy number variation and genetic instability, and thus, in high variability. In this work, we aimed to avoid copy number variation by using two strains where the luciferase reporter has been integrated into the yeast genome (Salinas et al., 2018). Importantly, in the FUN-LOV variants developed here (FUN-LOVSP, FUN-LOVSP-Nat, and FUN-LOVSP-Hph), the complete optogenetic system is encoded in a single plasmid (SP) of low copy number (pRS316). In addition, we integrated the FUN-LOVSP-Nat and FUN-LOVSP-Hph variants into the yeast genome and demonstrated its functionality and showing that can result in higher levels of luciferase expression than to the original system (Figure 5). Interestingly, the FUN-LOV switch and its variants showed a response to blue-light during the exponential growth phase, which begins at 4 h of yeast growth approximatively (Supplementary Figures S2–S6). This observation is related to the transcriptional activity of the promoters (ADH1, TDH3, and PGK1) used to control the FUN-LOV components expression. These promoters are active during the glucose consuming phase, reducing its activity in stationary phase or when glucose has been depleted (Partow et al., 2010; Salinas et al., 2018). This suggest that the chimeric proteins of the FUN-LOV system are available for light response only during the exponential growth phase. In conclusion, our results of light-dependent Luc expression are consistent with the growth kinetics of each yeast strain.
Other optogenetic systems based on blue-light photoreceptors have been integrated into the yeast genome, reporting similar levels of a reporter gene expression compared to the genome integrated FUN-LOVSP-Nat and FUN-LOVSP-Hph variants (Zhao et al., 2018; Zhao et al., 2020). For instance, the OptoEXP and OptoINVRT optogenetic systems have been integrated into the yeast genome, using the HIS3 locus or ∂-sites (Zhao et al., 2018; Zhao et al., 2020). Similarly, the optogenetic system based on CRY2-CIB1 interaction has been integrated into the URA3 locus (An-Adirekkun et al., 2020). Importantly, the fold-induction of luciferase expression obtained by FUN-LOVSP-Nat and FUN-LOVSP-Hph variants are comparable to the yLightOn system (573-fold), an optogenetic switch described in yeast and based on VVD self-dimerization (Xu et al., 2018). Beyond blue-light optogenetic systems, the fold-induction for luciferase expression achieved by the FUN-LOV variants is comparable to those previously reported for optogenetic systems based on red-light photoreceptors in yeast. For instance, the optogenetic system based on the red-light photoreceptor Phytochrome B (PhyB) and its interacting protein PIF3, both from Arabidopsis thaliana, achieved a 1000-fold of induction for the lacZ reporter (Shimizu-Sato et al., 2002). Similarly, the optogenetic system based on the red light-dependent interaction of the Phytochrome A (PhyA) photoreceptor and FHY1 protein, also both from A. thaliana, permitted a 300-fold of induction for a luciferase reporter (Sorokina et al., 2009). Therefore, the levels of gene expression achieved by the FUN-LOV variants developed in this work are higher than the original FUN-LOV system, and similar to other optogenetic systems described in yeast.
As proof of concept to demonstrate the applicability of our FUN-LOV variants, we implemented the FUN-LOVSP-Hph variant in the 59A-EC1118 wine yeast strain, showing to be fully functional in this genetic background. This is the first demonstration of a functional optogenetic switch in a wine yeast strain, opening the possibility for optogenetic control of oenological phenotypes such as glycerol, acetate, and ethanol production. Furthermore, the FUN-LOV variants described here could be used to control the microbial interactions of the yeast community present at the beginning of the fermentation process (Walker and Pretorius, 2022). In addition, the FUN-LOV variants developed in this work could be also used in wine strains for functional characterization of horizontally acquired genes, which have been described as key players in yeast adaptation to different environmental conditions (Devia et al., 2020). Therefore, the FUN-LOV variants reported in this work have multiple applications in wine yeast research.
In conclusion, we have expanded the FUN-LOV variants, encoding the system in a single plasmid (FUN-LOVSP), and including antibiotic resistances (FUN-LOVSP-Nat and FUN-LOVSP-Hph) that enables its genome integration in two different genetic backgrounds (BY4741 and 59A-EC1118). These variants reduced the molecular biology limitations of FUN-LOV, promoting its future applications in wild and industrial strains to control biotechnologically relevant phenotypes by light.
Data availability statement
The original contributions presented in the study are included in the article/Supplementary Material, further inquiries can be directed to the corresponding author.
Author contributions
DF, CB, DR, CI, AR, and RT generated the plasmids, developed the yeast strains, performed the experiments, and analyzed the data. DF, CB, DR, AR, and FS performed the in silico design of FUN-LOVSP, FUN-LOVSP-Nat, and FUN-LOVSP-Hph. DF, CB, AR, and FS conceptualized the research project. FS wrote the manuscript with insight from all the authors. The final version of the manuscript was approved by all the authors.
Funding
This work was funded by the ANID-Millennium Science Initiative Program-ICN17_022. FS was supported by ANID-FONDECYT grant number 1210955. DF, CB, and AR were supported by ANID PhD scholarship number 21200745, 21200066, and 21210525, respectively.
Acknowledgments
We thank Vicente Rojas and Luis F. Larrondo for sending us the BY4741 strain; and Camila Bastias and Claudio Martinez for sending us the 59A-EC1118 strain. We also thank the generous donation of reagents from two Chilean researchers. Lastly, we thank Alejandro Montenegro-Montero for language and editorial support.
Conflict of interest
The authors declare that the research was conducted in the absence of any commercial or financial relationships that could be construed as a potential conflict of interest.
Publisher’s note
All claims expressed in this article are solely those of the authors and do not necessarily represent those of their affiliated organizations, or those of the publisher, the editors and the reviewers. Any product that may be evaluated in this article, or claim that may be made by its manufacturer, is not guaranteed or endorsed by the publisher.
Supplementary material
The Supplementary Material for this article can be found online at: https://www.frontiersin.org/articles/10.3389/fbioe.2022.1029217/full#supplementary-material
References
Ambroset, C., Petit, M., Brion, C., Sanchez, I., Delobel, P., Guérin, C., et al. (2011). Deciphering the molecular basis of wine yeast fermentation traits using a combined genetic and genomic approach. G3 Genes|Genomes|Genetics 1, 263–281. doi:10.1534/g3.111.000422
An-Adirekkun, J., Stewart, C. J., Geller, S. H., Patel, M. T., Melendez, J., Oakes, B. L., et al. (2020). A yeast optogenetic toolkit (yOTK) for gene expression control in Saccharomyces cerevisiae. Biotechnol. Bioeng. 117, 886–893. doi:10.1002/bit.27234
Baganz, F., Hayes, A., Marren, D., Gardner, D. C. J., and Oliver, S. G. (1997). Suitability of replacement markers for functional analysis studies in Saccharomyces cerevisiae. Yeast 13, 1563–1573. doi:10.1002/(sici)1097-0061(199712)13:16<1563::aid-yea240>3.0.co;2-6
Boyden, E. S., Zhang, F., Bamberg, E., Nagel, G., and Deisseroth, K. (2005). Millisecond-timescale, genetically targeted optical control of neural activity. Nat. Neurosci. 8, 1263–1268. doi:10.1038/nn1525
Devia, J., Bastías, C., Kessi-Pérez, E. I., Villarroel, C. A., De Chiara, M., Cubillos, F. A., et al. (2020). Transcriptional activity and protein levels of horizontally acquired genes in yeast reveal hallmarks of adaptation to fermentative environments. Front. Genet. 11, 293. doi:10.3389/fgene.2020.00293
Fields, S., and Song, O.-K. (1989). A novel genetic system to detect protein–protein interactions. Nature 340, 245–246. doi:10.1038/340245a0
Figueroa, D., Rojas, V., Romero, A., Larrondo, L. F., and Salinas, F. (2021). The rise and shine of yeast optogenetics. Yeast 38, 131–146. doi:10.1002/yea.3529
Gietz, R. D., and Schiestl, R. H. (2007). High-efficiency yeast transformation using the LiAc/SS carrier DNA/PEG method. Nat. Protoc. 2, 31–34. doi:10.1038/nprot.2007.13
Goffeau, A., Barrell, B. G., Bussey, H., Davis, R. W., Dujon, B., Feldmann, H., et al. (1996). Life with 6000 genes. Science 274 (546), 546–567. doi:10.1126/science.274.5287.546
Goldstein, A. L., and Mccusker, J. H. (1999). Three new dominant drug resistance cassettes for gene disruption in Saccharomyces cerevisiae. Yeast 15, 1541–1553. doi:10.1002/(sici)1097-0061(199910)15:14<1541::aid-yea476>3.0.co;2-k
Hoffman, S. M., Tang, A. Y., and Avalos, J. L. (2022). Optogenetics illuminates applications in microbial engineering. Annu. Rev. Chem. Biomol. Eng. 13, 373–403. doi:10.1146/annurev-chembioeng-092120-092340
Karim, A. S., Curran, K. A., and Alper, H. S. (2013). Characterization of plasmid burden and copy number in Saccharomyces cerevisiae for optimization of metabolic engineering applications. FEMS Yeast Res. 13, 107–116. doi:10.1111/1567-1364.12016
Kolar, K., Knobloch, C., Stork, H., Žnidarič, M., and Weber, W. (2018). OptoBase: A web platform for molecular optogenetics. ACS Synth. Biol. 7, 1825–1828. doi:10.1021/acssynbio.8b00120
Losi, A., Gardner, K. H., and Möglich, A. (2018). Blue-light receptors for optogenetics. Chem. Rev. 118, 10659–10709. doi:10.1021/acs.chemrev.8b00163
Moreno Morales, N., Patel, M. T., Stewart, C. J., Sweeney, K., and Mcclean, M. N. (2021). Optogenetic tools for control of public goods in Saccharomyces cerevisiae. mSphere 6, e0058121. doi:10.1128/msphere.00581-21
Nielsen, J. (2019). Yeast systems biology: Model organism and cell factory. Biotechnol. J. 14, e1800421. doi:10.1002/biot.201800421
Oldenburg, K. R., Vo, K. T., Michaelis, S., and Paddon, C. (1997). Recombination-mediated PCR-directed plasmid construction in vivo in yeast. Nucleic Acids Res. 25, 451–452. doi:10.1093/nar/25.2.451
Partow, S., Siewers, V., Bjørn, S., Nielsen, J., and Maury, J. (2010). Characterization of different promoters for designing a new expression vector in Saccharomyces cerevisiae. Yeast 27, 955–964. doi:10.1002/yea.1806
Rienzo, A., Pascual-Ahuir, A., and Proft, M. (2012). The use of a real-time luciferase assay to quantify gene expression dynamics in the living yeast cell. Yeast 29, 219–231. doi:10.1002/yea.2905
Rojas, V., Salinas, F., Romero, A., Larrondo, L. F., and Canessa, P. (2022). Interactions between core elements of the Botrytis cinerea circadian clock are modulated by light and different protein domains. J. Fungi (Basel). 8, 486. doi:10.3390/jof8050486
Romero, A., Rojas, V., Delgado, V., Salinas, F., and Larrondo, L. F. (2021). Modular and molecular optimization of a LOV (Light–Oxygen–Voltage)-based optogenetic switch in yeast. Int. J. Mol. Sci. 22, 8538. doi:10.3390/ijms22168538
Salinas, F., Rojas, V., Delgado, V., Agosin, E., and Larrondo, L. F. (2017). Optogenetic switches for light-controlled gene expression in yeast. Appl. Microbiol. Biotechnol. 101, 2629–2640. doi:10.1007/s00253-017-8178-8
Salinas, F., Rojas, V., Delgado, V., Lopez, J., Agosin, E., and Larrondo, L. F. (2018). Fungal light-oxygen-voltage domains for optogenetic control of gene expression and flocculation in yeast. MBio 9, e00626–e00618. doi:10.1128/mbio.00626-18
Shimizu-Sato, S., Huq, E., Tepperman, J. M., and Quail, P. H. (2002). A light-switchable gene promoter system. Nat. Biotechnol. 20, 1041–1044. doi:10.1038/nbt734
Sorokina, O., Kapus, A., Terecskei, K., Dixon, L. E., Kozma-Bognar, L., Nagy, F., et al. (2009). A switchable light-input, light-output system modelled and constructed in yeast. J. Biol. Eng. 3, 15. doi:10.1186/1754-1611-3-15
Tang, K., Beyer, H. M., Zurbriggen, M. D., and Gärtner, W. (2021). The red edge: Bilin-binding photoreceptors as optogenetic tools and fluorescence reporters. Chem. Rev. 121, 14906–14956. doi:10.1021/acs.chemrev.1c00194
Tyszkiewicz, A. B., and Muir, T. W. (2008). Activation of protein splicing with light in yeast. Nat. Methods 5, 303–305. doi:10.1038/nmeth.1189
Walker, R. S. K., and Pretorius, I. S. (2022). Synthetic biology for the engineering of complex wine yeast communities. Nat. Food 3, 249–254. doi:10.1038/s43016-022-00487-x
Wang, X., Chen, X., and Yang, Y. (2012). Spatiotemporal control of gene expression by a light-switchable transgene system. Nat. Methods 9, 266–269. doi:10.1038/nmeth.1892
Witte, K., Strickland, D., and Glotzer, M. (2017). Cell cycle entry triggers a switch between two modes of Cdc42 activation during yeast polarization. eLife 6, e26722. doi:10.7554/elife.26722
Xu, X., Du, Z., Liu, R., Li, T., Zhao, Y., Chen, X., et al. (2018). A single-component optogenetic system allows stringent switch of gene expression in yeast cells. ACS Synth. Biol. 7, 2045–2053. doi:10.1021/acssynbio.8b00180
Zhao, E. M., Lalwani, M. A., Chen, J.-M., Orillac, P., Toettcher, J. E., and Avalos, J. L. (2021). Optogenetic amplification circuits for light-Induced metabolic control. ACS Synth. Biol. 10, 1143–1154. doi:10.1021/acssynbio.0c00642
Zhao, E. M., Lalwani, M. A., Lovelett, R. J., García-Echauri, S. A., Hoffman, S. M., Gonzalez, C. L., et al. (2020). Design and characterization of rapid optogenetic circuits for dynamic control in yeast metabolic engineering. ACS Synth. Biol. 9, 3254–3266. doi:10.1021/acssynbio.0c00305
Keywords: yeast, optogenetics, transcription, blue light, wine yeast
Citation: Figueroa D, Baeza C, Ruiz D, Inzunza C, Romero A, Toro R and Salinas F (2022) Expanding the molecular versatility of an optogenetic switch in yeast. Front. Bioeng. Biotechnol. 10:1029217. doi: 10.3389/fbioe.2022.1029217
Received: 26 August 2022; Accepted: 02 November 2022;
Published: 15 November 2022.
Edited by:
Sonja Billerbeck, University of Groningen, NetherlandsReviewed by:
David T. Stuart, University of Alberta, CanadaBeatriz Sabater-Munoz, Polytechnic University of Valencia, Spain
Copyright © 2022 Figueroa, Baeza, Ruiz, Inzunza, Romero, Toro and Salinas. This is an open-access article distributed under the terms of the Creative Commons Attribution License (CC BY). The use, distribution or reproduction in other forums is permitted, provided the original author(s) and the copyright owner(s) are credited and that the original publication in this journal is cited, in accordance with accepted academic practice. No use, distribution or reproduction is permitted which does not comply with these terms.
*Correspondence: Francisco Salinas, ZnJhbmNpc2NvLnNhbGluYXNAdWFjaC5jbA==
†These authors have contributed equally to this work