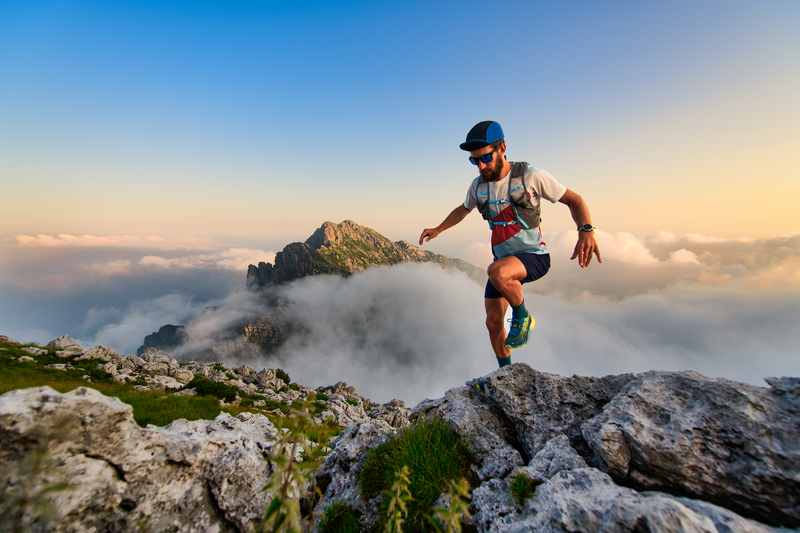
95% of researchers rate our articles as excellent or good
Learn more about the work of our research integrity team to safeguard the quality of each article we publish.
Find out more
REVIEW article
Front. Bioeng. Biotechnol. , 11 October 2022
Sec. Nanobiotechnology
Volume 10 - 2022 | https://doi.org/10.3389/fbioe.2022.1027468
This article is part of the Research Topic Advances in the Development of Functional Biomaterial Nanosystem in Tumor Therapy and Tissue Regeneration View all 9 articles
With the continuous development of nanobiotechnology in recent years, combining photothermal materials with nanotechnology for tumor photothermal therapy (PTT) has drawn many attentions nanomedicine research. Although nanomaterial-mediated PTT is more specific and targeted than traditional treatment modalities, hyperthermia can also damage normal cells. Therefore, researchers have proposed the concept of low-temperature PTT, in which the expression of heat shock proteins (HSPs) is inhibited. In this article, the research strategies proposed in recent years based on the inhibition of HSPs expression to achieve low-temperature PTT was reviewed. Folowing this, the synthesis, properties, and applications of these nanomaterials were introduced. In addition, we also summarized the problems of nanomaterial-mediated low-temperature PTT at this stage and provided an outlook on future research directions.
Traditional treatments for tumors mainly include surgery, radiotherapy (RT), and chemotherapy. However, these treatments, may have problems such as incomplete treatment and many associated side effects. Researchers have been seeking more targeted and effective treatment methods due to high morbidity and mortality. In recent years, with the rapid development of synthetic technology, the new therapeutic modality of photothermal therapy (PTT) has been recognized as one of the most promising treatments to attract widespread attention in the biomedical field (Pinto and Pocard, 2018; Liu et al., 2019; Li X. et al., 2020; Lu et al., 2021; Lv et al., 2021; Peng et al., 2021; Zafar et al., 2021). The main treatment principle of PTT is to use photosensitizers (such as indocyanine green (ICG), etc.) that absorb in the near-infrared (NIR) to produce thermal therapeutic effects for tumor elimination. Compared with traditional anti-tumor therapies (such as RT and chemotherapy), photosensitizers are non-toxic substances in the dark, which makes NIR-triggered PTT more significantly reduce toxic side effects; in addition, the light radiation parameters have great specificity (such as duration, power intensity and location), can be precisely controlled, which further increases its therapeutic effect. For sustainable efficient photothermal systems, researchers adopted black nanomaterials as light absorber to boost the absorption and conversion efficiency of photothermal materials (Liu et al., 2017; Wang L. et al., 2021). Moreover, many materials exhibit high absorption peaks in the NIR band and can be used as effective photothermal sensors (Pelaz et al., 2017; Fernandes et al., 2020; Lee and Gaharwar, 2020; Zhang et al., 2020; Wang Y. et al., 2021; Yu et al., 2021). Furthermore, changes in synthesis methods of materials can modulate the light absorption peaks to a narrow wavelength range, improving the specificity of light absorption;
For the conventional PTT treatment, photothermal agent with strong photothermal conversion efficiency was mainly synthesized through various assembly modifications. After injecting into the body, the photothermal agent accumulates at the tumor site, and transform the light to heat conversion can kill tumor cells under external irradiation (e.g., NIR laser) (Melancon et al., 2011; Zhou et al., 2016; De Melo-Diogo et al., 2017). However, PTT has some disadvantages, which mainly disadvantages were heat-shock response and limited light penetration. As previous studies shown, the temperature >45°C is usually required to achieve the photothermal damage effect on tumor cells. Based on this, a higher temperature (>50°C) is usually required in the centre of the tumor site and then gradually a temperature gradient difference forms outward so that the marginal parts of the tumor also reach the treatment temperature (Tang, 2011; Liu et al., 2019; Sun Q. et al., 2021). Beside this, the limited light penetration is also the focus issue for PTT clinical application.
Fueled by the improving nanotechnology, the application of nanomaterials in PTT is one of the hotspots in nanomedicine research. Nanomaterials are one-dimensional materials with sizes between 1 and 100 nm and have a variety of unique properties that depend on their chemical structure, synthesis methods and modifications. Nanomaterial-based PTT has many advantages in tumor therapy. Many nanomaterials can be attached to various surface modification molecules (e.g. polymers or antibodies), which can alter the biological properties of the nanomaterials and attenuate or eliminate the toxicity of some nanomaterials (Guo and Wang, 2011; Doughty et al., 2019; Obuobi et al., 2019; Andraos and Gulumian, 2021; Subjakova et al., 2021). Firstly, the highly specific and target to tumor site which could reduce damage to normal tissue cells. Furthermore, adjustable effects on the tumor site can be produced via adjusting the NIR laser irradiation parameters (e.g., time, power, and site). In addition, Nanomaterial-based PTT can promote photothermal agents selective accumulation in tumors via enhancing permeability and retention (EPR) effects, which significantly improved both the selectivity and efficacy of PTT and have been used to treat many types of tumors. However, under the stimulation of high temperature, although PTT will have a better killing effect on tumor cells, it may also produce certain damage to the surrounding healthy tissues and cells (Zhu et al., 2016); For example, the cell damage caused by high temperature will release some intracellular factors, which may aggravate local tissue inflammation (Bonfil et al., 1988). Meanwhile, tumor cells stimulated by high temperature easily acquire tolerance to hyperthermia, which will further enhance the viability of tumor cells, a phenomenon known as thermotolerance (Kampinga, 1993; Wu, 1995). In response to the many problems associated with high-temperature PTT, the research strategy of low-temperature PTT has been proposed in recent years (Hu et al., 2021; Yi et al., 2021).
Unlike high power laser irradiation in high temperature PTT, low-temperature PTT mainly refers to the induction of tumor cell apoptosis under low power laser irradiation, i.e. low temperature (≤45°C) photothermal conditions (Jung et al., 2016; Xia et al., 2021). Although low-temperature PTT reduces the damage to normal tissue cells, it may not be able to achieve a satisfactory anti-tumor therapeutic effect due to the existence of molecular chaperones and heat shock proteins (HSPs) that are associated with heat resistance in living organisms. HSPs are highly conserved and present in almost all cells and perform various concomitant functions, which involved in nascent polypeptide chain formation, protein folding, protein transport and antigen delivery (Morimoto, 1998). HSPs are located inside and outside normal cells but also in tumor cells and virus/bacterial-infected cells and facilitate resistance to adverse environmental factors (e.g. high temperature or toxic environment stimulation) (Chen et al., 1999; Sherman and Multhoff, 2007; Liang et al., 2015). HSPs are widely expressed in tumor cells and play an important role in mediating intrinsic tumor cell characteristics, such as regulating non-programmed cell division, evading programmed cell death and senescence, promoting neovascularization and increasing metastasis and invasion (Li et al., 1995). Moreover, HSPs take part in the interaction between tumor cells and the TME, which can promote the release of HSPs from tumor cells to influence the behaviour of neighboring cells and infiltrate normal cell (Lang et al., 2019; Marchan, 2019; Wang et al., 2020; Seclì et al., 2021; Fu and Jia, 2022).
Therefore, in the application of low-temperature PTT, it is crucial to reduce the expression of HSPs, not only to inhibit the protective effect of HSPs on tumor cells and improve tumor cells sensitivity to thermal stimulation, thus improving the efficacy of low-temperature PTT, but also to facilitate the apoptosis of tumor cells. Therefore, researchers have searched for a series of methods to inhibit HSPs, and below we review several strategies to inhibit the production and expression of HSPs and thus achieve low-temperature PTT. Current therapies targeting HSPs utilize four strategies: 1) HSPs (HSP 90 and 70) small molecule inhibitor, 2) 3. Small interfering RNA for HSPs, 3) Starvation therapy for HSPs inhibition, 4) Cytokines inhibition.
HSP 90 is a highly conserved ATP-dependent heat stress protein with increased expression during cellular stress and can correct protein misfolding to maintain its activity and reduce cellular damage (Hong et al., 2013; Hoter et al., 2018). Therefore, the inhibition of HSP 90 expression with small-molecule inhibitors which is an important therapeutic idea for low-temperature PTT. Unlike the side effects of nontargeted therapies such as chemotherapeutic drugs, small molecule inhibitors particularly inhibit tumor cell growth by inhibiting certain nucleic acids and proteins associated with tumor growth and metastasis with minimal adverse effects. Moreover, combining HSP 90 small molecule inhibitors with nanomaterials can not only solve the problem of poor targeting but also reduce the adverse effects on other organs and tissues, further improving the therapeutic effect (Zhao et al., 2017).
Geldanamycin, a small molecule inhibitor of ATP-competitive HSPs, which binds to HSP90 and alters the construction of HSP90, inhibiting ATPase activity required for HSP90, thereby preventing HSP90 from binding properly to its client proteins and ultimately leading to the degradation of its client proteins by proteases (Stebbins et al., 1997). 17-Allylamino-17-demethoxygeldanamycin (17-AAG) is a derivative obtained by substituting the 17th methoxy position of geldanamycin with N,N-dimethylethylamine, which has substantially increased biological activity and improved toxicity compared to geldanamycin (Yun et al., 2015; Todurga Seven et al., 2022). Hollow mesoporous organosilica nanocapsules (HMONs) designed by Wu et al., which were loaded with indocyanine green (ICG) and 17-AAG onto HMONs using gemcitabine (Gem) molecules as capping ends, and after correction with polyethylene glycol (NH2-PEG), the assembled ICG-17AAG@HMONS-Gem-PEG exhibited pH responsive molecular release properties and glutathione (GSH)-dependent degradation properties, which can precisely control the release of ICG and 17AAG to accomplish low-temperature PTT (Wu et al., 2018). Using a similar application of 17-AAG, Fu et al. designed and synthesized a boron (B)-based multifunctional nanoplatform with NH2-PEG-NH2 and cRGD (targeting αvβ3 integrins overexpressed in tumor cells), respectively, by “electrostatic adsorption” and “amide reaction” surface modification of B nanosheets (B-PEG-cRGD), which both prolongs the half-life of the platform in vivo and enables the platform to specifically target tumor sites and then coloads adriamycin (DOX) and 17-AAG onto B-PEG-cRGD nanosheets to form a multifunctional nanoplatform (DOX-17AAG@B-PEG-cRGD). The NIR laser and an acidic pH environment will accelerate the release of DOX and 17-AAG from the above nanoplatform to achieve low temperature PTT to kill tumor cells (Fu et al., 2020).
Another natural small molecule inhibitor of HSPs, gambogic acid (GA), suppressed cell proliferation and induced degradation of client proteins of HSPs in cells (Davenport et al., 2011). Bi@ZIF-8 (BZ) nanoparticles were prepared by Li et al. Then, GA was effectively loaded onto BZ nanomaterials by a physical mixing method. Under acidic conditions, the drug release rate increased significantly with increasing temperature (Li J. et al., 2020). Sun et al. synthesized non-toxic, high photothermal conversion hollow mesoporous carbon spheres (HMCS) to form the HMCS-PEG-GA system with GA loading. HMCS-PEG-GA showed good stability in biological media and good photothermal imaging and photoacoustic imaging (PAI) capability. Guided by PAI signals, HMCS-PEG-GA shows great promise for accurate tumor diagnosis and low-temperature PTT (Sun et al., 2020). In addition, Yang et al. combining metal ions Mn2+ and ICG together via poly-l-histidine-polyethylene glycol (pHis-PEG) to form one-dimensional nanoscale coordination polymers (1D-NCPs).After that, GA was loaded in 1D-NCPs to form finally nanoplatform (Mn-ICG@pHis-PEG/GA), which exhibited effective pH responsiveness after systemic administration, and in low-temperature photothermal conditions, they can then effectively target tumor cells and induce apoptosis to achieve low-temperature PTT (Yang et al., 2017). They not only developed a facile method to prepare 1D-NCPs that can bind to different metal ions, but the nanoplatform is pH-responsive and can improve tumor targeting efficiency and specificity in a more direct manner. Similarly, inhibiting the expression of HSP90 and regulating nanoparticle release and degradation depends on the pH in the TME, thus improving the effectiveness of cryogenic antitumor therapy. Gao et al. self-assembled GA with human serum albumin (HSA) and DC-IR825 (an anabolic dye and photothermal agent) in aqueous solution through simple mixing to form stable HSA/DC-IR825/GA, which then escapes into the cytoplasm by mitochondrial breakage under NIR laser irradiation, releasing GA and blocking high temperature-induced HSP90 overexpression. In addition, HSA/DC-IR825/GA nanoparticles exhibited pH-responsive charge reversal properties, effective tumor site aggregation properties and minor liver deposition effects, which ultimately promoted the low-temperature PTT effect (Gao et al., 2019). Unlike simple material mixing or self-assembly, Song et al. prepared Bi2Se3 hollow nanocubes (HNCs) via moderate cation exchange and Kirkendall effect, and then revised it with hyaluronic acid (HA) based on disulfide bonds (-s-s-), which enabling HNC to target CD44 high expression tumor cells. Finally, GA, as a heat-shock protein inhibitor, was loaded into Bi2Se3-HNC to form HNC-s-s -HA/GA, which could induce effective tumor cell apoptosis under NIR laser irradiation. In addition, based on the radiosensitizer Bi2Se3-HNC, the nanoparticles can also be used for depth-independent RT (Figure 1) (Song et al., 2019). This study designed a method to synthesize nanoparticles by chemical bonding linkage to control the effective release of GA for more targeted action on tumor cells, while combining low-temperature PTT with RT to achieve more powerful antitumor therapeutic effects.
FIGURE 1. Schematic diagram of (A) the construction of Bi2Se3 HNC-s-s-HA/GA (HNC-s-s-HA/GA), (B) HNC-s-s-HA/GA provoked low-temperature PTT and boosted RT (Song et al., 2019).
Mitochondria-targeted low-temperature PTT is a agreeing therapeutic strategy which maximize antitumor effects and reduce adverse effects. Zhang. et al. synthesized a mitochondria-targeted nanomicelles based on IR780 and BIIB021 (a small molecule inhibitor of HSP90) (Yan et al., 2017). PEG-IR780-BIIB021 nano-micelles have good biocompatibility and biostability, have a high encapsulation rate of BIIB021, can be selectively enriched in mitochondria, and can be released after NIR irradiation. The release of BIIB021 after NIR light irradiation not only reduces cellular tolerance to heat but also decreases mitochondrial membrane potential, which resulting in key endogenous apoptotic factors affection (Cyt-C, Caspase-9, Bcl-2 and Bax), synergistically exerting the effect of low-temperature PTT to strike tumor cells down (Zhang et al., 2021). Deng et al. combined low-temperature PTT with the induction of cellular autophagy to exert a more complete tumor cell killing effect. The tumor-targeting ligand folic acid (FA) was introduced into SNX-2112 (HSP90 small molecule inhibitor) (Liu et al., 2012) loaded graphene oxide (GO) to assemble GO-FA-SNX-2112 nanoparticles. GO has photothermal properties, and with heating from NIR irradiation, the non-covalent interaction between GO and other materials was attenuated, promoting the release of SNX-2112, while GO-FA-SNX-2112 could alter the level of tumors autophagy within 90 s after NIR light irradiation. Thus, combining low-temperature PTT with autophagy resulted in significant antitumor activity (Deng et al., 2020). Epigallocatechin gallate (EGCG) is a extracted from green tea and a natural small molecule inhibitor for HSP90. EGCG has low cytotoxicity, good stability, which can selectively interfere HSP90 activity through multiple cellular signaling pathways (Palermo et al., 2005; Bhat et al., 2014). For real-time diagnosis, Jiang et al. proposed a novel diagnostic method by synthesizing NaLuF4:Nd nanoparticles (Lu:Nd)-coated mesoporous SiO2 (mSiO2) to form a core-shell structure (Lu:Nd@mSiO2). After alkaline conditions, the flowerlike Lu:Nd@NiSiO3 was obtained via continuously reacted with the nickel-ammonia complex under high temperature. Then, loading EGCG by “electrostatic adsorption” to form finally product of Lu:Nd@NiS2-EGCG for NIR imaging and MRI (Jiang et al., 2019). The above examples of integrating various HSPs small molecule inhibition into the PTT nanoplatform not only provide more insights into the working mechanism of low-temperature PTT but also provide important strategies and ideas for achieving safe and effective low-temperature PTT-induced tumor apoptosis.
Heat shock protein 70 (HSP 70) is a potent negative regulator of apoptosis and an important factor in promoting tumor cell growth and survival (Elmallah et al., 2020; Kabakov and Gabai, 2021; Lei et al., 2021; Sharapova et al., 2021); In addition, HSP70 level elevation in tumors was correlated with poor patient prognosis, and HSP70 deficiency speeds up tumor cell death and often selectively sensitizes malignant tumor cells to some chemotherapeutic agents (Ciocca and Calderwood, 2005). Similarly, many researchers have also reduced heat resistance to achieved apoptosis of tumor cells at lower temperatures by inhibiting the expression of HSP70. Therefore, the development of small molecule inhibitors or nanocarriers for inhibiting HSP70 expression will provide another strategy to improve low-temperature PTT.
LY294002 is a small molecule inhibitor that inhibits phosphatidylinositol 3-kinase (PI3K)/Akt activity, glycogen synthase kinase-3 (GSK-3) activity, and then reduces HSP70 expression via the above (PI3K/Akt/GSK-3/HSP) signaling pathway (Wigmore et al., 2007). PI3K/Akt is a pivotal kinase which involves in the intracellular antiapoptotic pathways by regulating relevant downstream proteins. Accordingly, PI3K/Akt inhibition can promote apoptosis and inhibit cell growth, proliferation, invasion, and tumor metastasis. However, the inhibitor itself is insoluble in water and exhibits severe toxicity in therapeutic indications, which widely limits its wide application. Therefore, a multifunctional Bi2S3-Tween 20 nanoplatform loaded with LY294002 was formed by Song et al. The Bi2S3-Tween 20@LY294002 had good biocompatibility and not only exhibited excellent photothermal efficiency under the action of an 808 nm laser but also rapidly removed the ultrasmall Bi2S3 nanodots from the body and could be used as a safe drug delivery platform in vivo (Song et al., 2018). Similarly, to address a series of side effects of conventional PTT, Ni et al. prepared C6TI/Apo-Tat nanoparticles by cowrapping C6TI (photothermal agent) and Apo (heat shock protein 70 inhibitor) and then coupling them with TAT peptide to improve cell penetration. The results demonstrate that C6TI/Apo-Tat NPs can achieve low temperature PTT by inhibiting HSP70 expression (Ni et al., 2020).
A NIR corresponding nanoplatform combining low-temperature PTT with heat-triggered NO donors (RSNO) was designed by You et al. Specifically, a layer of mesoporous silica (SiO2) was coating on high-performance gold nanorods (Au) to obtain multifunctional Au@SiO2, which were further coupled with S-nitrosothiols on their surface, and then, low-toxicity, stable 2-phenylethynylsulfonamide (PES) (HSP70 inhibitor) was wrapped into Au@SiO2 to reduce the tumor cell heat resistance. Subsequently, polyethylene glycol (PEG) was modified onto Au@SiO2 to provide better stability and biocompatibility. When PES/Au@SiO2 entered tumor cells, the generated hypothermia under NIR irradiation not only caused apoptosis or necrosis under low temperature with the aid of an HSP70 inhibitor but also promoted NO releasing, which achieving the synergistic effect of low-temperature PTT and gas therapy. In vitro and in vivo experiments showed that this method has high synergistic efficiency and can effectively inhibit tumor growth (You et al., 2020).
Quercetin is a natural product with excellent antioxidant capacity against free radicals; it also inhibits tumors and exerts anticancer effects; in addition, quercetin is considered to be one of the most potent small molecule HSPs inhibitors, inhibiting the expression of HSP70 by interfering with the HSPs transcription factors phosphorylation. The ketone and hydroxyl groups of quercetin presented three possible metal chelating sites and can bind to various metal ions (De Souza and De Giovani, 2004). In this regard, Yang et al. developed multifunctional Qu-FeIIP nanospheres based on the coordination interactions between quercetin, iron (Fe) and polyvinylpyrrolidone (PVP) (Yang et al., 2019). The prepared Qu-FeIIP package carries quercetin as the backbone component of the nanodrug, thus avoiding problems of drug loading and release; Meanwhile, the hydroxyl group of quercetin is transformed to ketone during free radical scavenge (Valko et al., 2006). This weakens chelating ability of quercetin with metals and facilitate the decomposition and scavenging of Qu-FeIIP nanodrug in vivo; meanwhile, ROS scavenging can alleviate the ROS-related inflammatory response. After, free quercetin can continue to exert its inherent anticancer and anti-inflammatory effects (Middleton et al., 2000). This provides us with a widely used nanotherapeutic platform that can combine multiple metal ions while encapsulating multiple small molecule inhibitors of HSPs to construct various nanosystems for low-temperature PTT.
To inhibit both HSP90 and HSP70 and exert a better effect of inhibiting HSPs, thus further improving the therapeutic effect of low-temperature PTT, researchers applied both HSP90 and HSP70 small molecule inhibitors. Miyagawa, T. et al. Investigated 17-DMAG (HSP90 inhibitor) and quercetin (HSP70 inhibitor) with ferromagnetic particle (FMP)-mediated antitumor effects of low-temperature PTT and found that tumor growth was significantly inhibited (Miyagawa et al., 2014). Instead of applying two small molecule inhibitors of HSPs simultaneously, Tang et al. applied VER-155008, a small molecule inhibitor of both HSP90 and HSP70, to nanomaterials and established a therapeutic system consisting of methoxy-polyethylene-glycol-coated-gold-nanorods (MPEG-AuNR) and VER-155008 micelles (VER-M), which improved the biocompatibility and boosted permeability and retention (EPR) effects of the nanomaterials and reduced toxicity (Tang et al., 2018; Brünnert et al., 2020; Sakai et al., 2021).
SiRNA regulate the expression of HSPs at the gene level through gene silencing. Many problems are encountered during RNA interference processing. First, during blood transport, siRNA cannot be delivered alone due to its negative charge. Secondly, therapeutic siRNA suffers from low cellular delivery efficiency as well as nontargeting. Finally, after siRNA is taken into the cell and then escapes from the nuclear endosome into the cytoplasm, many factors that interfere with RNA are also encountered in the cytoplasm. Finally, when siRNA is taken into the cell and then escapes from the nucleosome to the cytoplasm, many factors that interfere with RNA are encountered in the cytoplasm, such as cleavage by nucleic acid endonucleases and RNA-induced silencing complex (RISC). To overcome these delivery barriers, researchers have designed various nanocarriers to enhance the blood stability of siRNA, cellular uptake of siRNA and escape from the nucleosome (Lee et al., 2012).
Wang et al. developed a multifunctional photothermal platform consisting of a gold nanoshell core wrapped around siRNA targeting HSP70. siRNA can specifically detach from the gold surface and achieve in vivo lysosomal escape under NIR light irradiation, thereby inhibiting the expression of HSP70(Wang et al., 2017). This strategy cleverly combines local PTT and controlled HSP70 silencing with a simple and easily controlled structure, which has considerable potential for clinical applications. Similarly, inhibiting the expression of HSP70, Ding et al. constructed a HSP70-siRNA worked as crosslinkers to direct the DNA-grafted polycaprolactone (DNA-g-PCL) assemble into nanosized hydrogel (dopamine (PDA)-coated nucleic acid nanogel) through nucleic acid hybridization. After that, the siRNA-embedded nanogels was coated by a thin layer of polydopamine. The assembled siRNA delivery complex had good blood stability, enhanced accumulation at the tumor site, and ablated tumors effectively under low temperature light conditions (Figure 2) (Ding et al., 2020). In response to the lack of specific surface markers to distinguish triplenegative breast cancer (TNBC) from normal cells, Wang. et al. used a layer-by-layer preparation to synthesize a gold nanostar (GNS)/siRNA72/hyaluronic acid (HA) nanosystem to selectively target and sensitize tumor cells to PTT. Despite CD44 is a good marker for triple-negative breast cancer, it is also highly expressed in other normal tissues, such as human breast epithelium (Jordan et al., 2015). Therefore, HA alone cannot completely distinguish triple-negative breast cancer from adjacent normal breast tissue. The experimental design of GNS/siHSP72/HA, which specifically introduces siHSP72 into CD44-positive cells, particularly sensitizes triple-negative breast cancer cells by downregulating HSP72 overexpression in CD44-positive cells and improves the effect of PTT on TNBC with lowest damage to healthy cells. By targeting CD44 and subsequently removing of HSP72, the therapeutic window of PTT was successfully narrowed from irradiating all cells to only CD44-positive cells and TNBC cells (Wang S. et al., 2016). This undoubtedly reduced damage to normal cells and improved targeting of abnormal cells.
FIGURE 2. Schematic diagram of (A) PDA-encapsulated nucleic acid nanogels and (B) mediated low-temperature PTT (Ding et al., 2020).
BAG3 protein is belong to BAG cochaperone protein family, which is the only member of the family induced by stressful stimuli, mainly through activation of heat shock factor 1 (HSF1) on the promoter of the BAG3 gene, and plays a cytoprotective role in preventing cell death and an important role in heat resistance (Rosati et al., 2011; De Marco et al., 2018; Marzullo et al., 2020). Wang et al. modified gold nanorods (GNRs) with positive dots on the surface and then bound to negative ionized siRNA targeting BAG3 by “electrostatic adsorption” to form GNRs-siRNA nanocomplexes. The nanocomplex could inhibit high temperature-induced BAG3 expression at the mRNA and protein levels and produced stable NIR-activated photothermal properties (Wang B. et al., 2016).
Despite the considerable research potential of siRNA drugs, they have not been strongly promoted in scientific experiments and clinical drug applications due to problems such as poor blood stability and nontargeted effects. To solve these critical problems, not only the structure of siRNA needs to be optimized, but specialized carrier transport systems also need to be developed. At present, researchers have designed many strategies to optimize the structure and transport carriers of siRNA.For example, siRNA can be assembled by simple chemical ligation, complementary base pairing and interaction forces with nanocarriers to form stable complexes, which can not only compensate for the defects of easy degradation of a single siRNA but also improve the intracellular delivery. New siRNA transport carriers were designed with better siRNA delivery properties to avoid other undesirable reactions while maintaining the inherent biological activity. Furthermore, by introducing a cleavable chemical bond between siRNA and the carrier, the siRNA and the nanocarrier can be easily dissociated from each other and the original siRNA can be released to function in a specific environment. Of course, we still need to perform rigorous biological characterization and experimental validation of the newly fabricated siRNA-based structures and carriers to identify and resolve their possible adverse reactions.
Although there are numerous small molecule inhibitors of HSPs, their effect is delayed because they only act on the stimulated HSPs that are already presentand do not prevent their production in advance, so they do not inhibit the effect before treatment. Moreover, tumor cells can overexpress multiple HSPs, but one siRNA and small molecule inhibitors of HSPs may have a single effect and can only impair the expression of one heat shock protein. Therefore, some researchers have considered further inhibiting the production of HSPs at the source. Tumor “starvation therapy” is an emerging approach to inhibit tumor growth by cutting off nutrient supply, which is usually achieved through vascular embolization (Jing et al., 2018). In addition, rapid tumor cells proliferation requires a large amount of vitality to survive and grow, causing tumor cells to absorb more glucose than normal cells and become vulnerable to changes in glucose concentration.
Glucose oxidase (GOx) is the most commonly used biocatalyst for regulating tumor glucose metabolism; Gox converts glucose to gluconate and toxic H2O2 and inhibits the production of ATP by promoting glucose consumption, which is the main energy donor of the body and is important for both cell growth and protein synthesis. In addition, tumor hypoxia is a great challenge in tumor therapy due to negative feedback of various antitumor treatments. Zhou. et al. designed a composite nanosystem that targets tumor cells and has redox-responsive properties. The nanosystem loads GOx onto porous hollow Prussian blue nanoparticles (PHPBNs) and coats hyaluronic acid (HA) on its surface via disulfide bonds (-s-s-). The PHPBN can catalyze the breakdown of H2O2 to O2 in tumor cells to enhance hypoxia-inhibited GOx-mediated therapy. Furthermore, PHPBNs and GOx enhance intracellular glucose consumption and reduce ATP supply, which further reduce the resistance to PHPBN-mediated low-temperature PTT (Zhou et al., 2018). Using the same application of GOx, Song. et al. designed a nanoparticle using PDA as the core and hollow silica (PDA@hm) as the shell to carry the autophagy inhibitor drug chloroquine (CQ) and GOx to form PDA@hm@CQ@GOx. PDA@hm@CQ@GOx can effectively mediates low-temperature PTT of tumors through starvation effect. Moreover, Gao. et al. rationally designed a multifunctional temperature-sensitive liposome, and constructed temperature-sensitive phase transition liposomes through lipid molecules DPPC and DSPE-PEG2000 to compress GOx, ICG, and GA. The formed GOIGLs could achieve thermally responsive drug delivery at tumor sites and hypothermic hyperthermia (Gao et al., 2020).
Moreover, the combined catabolism of glucose to inhibit ATP production and inhibition of autophagy showed the good effect of PDA@hm@CQ@GOx for boosting low-temperature PTT (Figure 3). Firstly, PDA@hm was formed via a four-step process. Then, the autophagy inhibitor CQ was loaded in PDA@hm, which further combined with glucose consumer GOx to form PDA@hm@CQ@GOx ( corona-like structure) (Shao et al., 2020). Cao. et al. designed a nanoreactor Fe-PDAP/GOx/ICG for enhancing low-temperature PTT using a combination of mechanisms. Glucose is broken down by GOx to gluconic acid and H2O2, which is converted to O2 to promote oxygenation, thereby enhancing O2-dependent photodynamic therapy (PDT). Results showed that the nanoreactor not only reduced tumor cell heat resistance, but also enhanced intracellular ROS production and cytotoxicity in tumor cells, successfully achieving a multimechanism combination to induce low-temperature PTT (Cao et al., 2020). Unlike GOx, which promotes glucose consumption, lonidamine (LND) is an antitumor drug that reduces ATP production indirectly by regulating the secretion of hexokinase, a key biochemical process in energy production such as glycolysis and mitochondrial respiration (Nath et al., 2016). From this, Wang. et al. designed tumor cell membrane-encapsulated nanoparticles targeting tumor cell mitochondria. ZnGlu-PB nanoparticles (PG NPs) were synthesized by encapsulating the photothermal agent Prussian blue nanoparticles (PB NPs) with zinc glutamate (ZnGlu) and then coupling the LND molecule to the mitochondrial targeting group TPP (denoted TLND) (PGTL NPs) to increase the inhibitory effect on ATP production. Finally, HepG2 cell membranes were wrapped around PGTL to form HmPGT NPs, which considerably prolonged the in vivo circulation time and interfered with ATP production to improve treatment effect of low-temperature PTT to tumor cells (Wang P. et al., 2021). The production of ATP is dependent on glucose enzymes, which to a large extent requires the help of glucose transporters (Gluts) to transfer glucose from extracellular to intracellular. The typical representative is Glut1, which is widely express in tumor cells (Macheda et al., 2005). Chen et al. developed a GNR/HA-DC nanosystem by coupling diclofenac (DC), a highly selective inhibitor of Glut1, and hyaluronic acid (HA) and further modifying them on gold nanorods (GNRs). Upon reaching the tumor site, tumor cells overexpressing hyaluronidase (HAase) can cleave the nanosystem and trigger DC release, and the released DCs induce downregulation of Glut1, inhibiting glucose transport, metabolism and ATP-dependent HSPs synthesis for the purpose of low temperature PTT (Chen et al., 2017).
FIGURE 3. Schematic diagram of (A) the construction of PDA@Hm@CQ@GOx and (B) the enhancement of low-temperature PTT through inhibition of autophagy and energy metabolism (Shao et al., 2020).
Cytokines secreted by immune cells (such as TNF-α, IL-2, IFN-γ, etc.) play a key role in antitumor immune effect. On the one hand, cytokines can promote tumor apoptosis through enhancing the expression of apoptosis-related proteins (Bax, Caspase 3 and Caspase8), reduce the expression of apoptosis-inhibiting proteins (Bcl-2, Bcl-xL), and promote tumor apoptosis. TNF-αbinds to two receptors, TNF-R1 and TNF-R2, leading to the recruitment of signal transducers and the activation of at least three distinct effectors through complex signalling cascades and networks. These factors then lead to the activation of caspases and proinflammatory transcription factors, AP-1 and NF-κB, trigger apoptosis in target cells. According to Ravichandran et al., apoptotic cell metabolic secretions positively regulate neighbouring cells within tissues. The gene expression of HSPs plays an anti-inflammatory role in the inflammatory environment in vivo to stabilize the stress environment (Baud and Karin, 2001; Green, 2020), thereby reducing the defensive overexpression of HSPs in tumor cells. On the other hand, cytokines can inhibit the expression of HSPs families (such as HSP27, HSP70, HSP90, HSP105, and HSF1, etc.) in tumor cells and enhance the sensitivity of tumor cells to heat stimulation (Schett et al., 2003; Sun Z. et al., 2021). For example, TNF-α can reduce amount of HSP27 Ser78 phosphorylation through the activation of PKC, thereby reducing the level of HSP27 in PBMCs (peripheral blood mononuclear cells) (Niwa et al., 2006) and attenuating the tolerance of target cells to thermal stimulation.
During tumorigenesis, the expression of tumor necrosis factor receptor-related factor 1 antibody (TNF-R1) on the cell membrane of tumor vascular endothelial cells and tumor cells is upregulated (Sun Z. et al., 2021). In the study of Schett. et al., after antibody (TNF-R1) and protein phosphatase-mediated inhibition of heat shock transcription factor 1 (HSF1) phosphorylation, TNF-a transiently downregulates the HSP70 stress response, resulting in increased cell susceptibility to apoptosis (Sun Z. et al., 2021). However, the immunosuppressive tumor microenvironment (TME) promotes tolerogenic dendritic cells (DCs) generation, the stimulation of CD4+ T-cell and CD8+ T-cell proliferation and activation is attenuated. Therefore, the design of engineered DCs has considerable potential to promote T-cell killing of tumor cells. Sun et al. designed an artificial DC (iDC) that consists of a nanoparticle core loaded with a photothermal agent (IR-797) and an outer coating of a mature DC film. NIRdots were firstly formulated through a nanoprecipitation procedure. Then, iDCs were further formed by coating mature DC cell membranes on NIRdots using an extruding method. The ability of DC cells to present antigens and activate T cells was retained by the iDCs on the surface of DC cell membrane (Figure 4). T cells activated by iDCs can produce cytokines which inhibit the expression of HSPs (HSP27, HSP70, HSP90, HSP105 and HSF1), as well as promoting tumor apoptosis and synergistically amplifying the antitumor effect of low temperature photothermal treatment (42–45°C). Immune cells and apoptotic tumor cells can induce immunogenic cell death, initiating a cycle of tumor immunity (Sun Z. et al., 2021).
FIGURE 4. The schematic of iDCs activating T cells and mediating hypothermic PTT. (A) The construction of iDCs. (B) iDCs injected intratumorally to primary tumor. (C) iDCs enhanced low temperature PTT. (D) iDCs induced synergistic antitumor effect (Sun Z. et al., 2021).
In summary, we have reviewed several ways that researchers have accomplished low temperature PTT by inhibiting HSPs expression in recent years, as summarized in Table 1.
PTT is a promising tool for using lasers to treat early-stage tumors or other types of disease. A good photothermal conversion agent can improve the selective heating of the target area. Molecular motion inside matter can generate heat. After decades of development, exciting progress has been made in all aspects. Several models of biological heat transfer which relied on Fourier and non-Fourier effects have been explored. Due to special structures and properties of biological tissues, and many other factors, the effects of metabolic heat production, blood flow, local thermal imbalances, time delays have been considered in different models. In addition, numerical models of radiative transport in biological tissues consider the complex optical organization and photothermal agent interactions (Ren et al., 2022). As an emerging treatment method, PTT has the advantages of strong controllability, remarkable curative effect, and small toxic/side effects, and which has been widely used in cancer research. Generally, most researches focus on PTT at high temperature (>50°C). Although high temperature PTT has good effect, which will also inevitably cause irreversible damage to normal tissues around the tumor. Therefore, low-temperature PTT has developed very rapidly, especially in the application of nanomaterials mediated low-temperature PTT.
In recent years, low-temperature PTT with nanomaterials has attracted increasing attention as an efficient, noninvasive and highly targeted therapeutic modality and has shown considerable potential in solving some problems in the biomedical field; For example, the size and physicochemical properties of nanomaterials make it easier to penetrate biological membranes and enable nanoparticles to specifically target tumor cells by integrating some tumor-targeting molecules or wrapping homologous cell membranes. In addition, nanomaterials have some remarkable properties such as pH responsiveness, organelle targeting, etc., which enable tumor cells to accumulate at the tumor site through the EPR effect. In addition, nanomaterials have some remarkable properties, such as pH responsiveness and organelle targeting, which can enhance the anti-tumor therapeutic effect by significantly increasing the ability of tumor cells to take up and accumulate drugs through low temperature PTT.
In this paper, we review the progress of nanomaterial-mediated low-temperature PTT mainly in terms of inhibiting the expression of HSPs. First, given that HSPs are the main factor in tumor cell heat resistance and play an important role in assisting tumor cells escape apoptosis-mediated death, the use of small molecule inhibitors of heat shock proteins (such as 17-AAG, GA, BIIB021, SNX-2112, EGCG, LY294002, Apo, 17-DMAG, quercetin, VER- 155008, etc.) and siRNAs can not only make tumor cells more susceptible to low-temperature PTT but also promote apoptosis; In addition, because HSPs synthesis and biological functions require energy, fundamental inhibition of HSPs production can be achieved by reducing or depleting nutrients or blocking nutrient supply. Second, the combination with autophagy activation/inhibition therapies can further improve the effectiveness of tumor clearance. Third, as many organelles play integral role in maintaining intracellular homeostasis, are susceptible to high temperatures, and combining organelle-targeting strategies with low-temperature PTT, which can also further improve the effectiveness of killing tumor cells. Finally, synergistic treatment combining low-temperature PTT with other therapeutic modalities (e.g., chemotherapy, immunotherapy, PDT, RT, etc.) will further improve the efficacy of low-temperature PTT: high temperature can increase blood flow, which can enhance the transport and accumulation of chemotherapeutic drugs and radiosensitizers in tumor cells; Other therapeutic can make cancer cells more susceptible to low-temperature PTT and exert better therapeutic effects; In addition, studies have also confirmed that high temperature can improve the hypoxic environment at the tumor site, providing the basic conditions for oxygen-dependent therapeutic modalities such as PDT. Therefore, nanotechnology is crucial for achieving synergistic therapeutic effects, and using diverse modification and synthesis methods, various drugs, materials and technological approaches with different therapeutic effects can be assembled and combined to exert better anti-tumor effects while achieving higher efficacy and fewer side effects.
Although there have been many innovative studies on low-temperature PTT, there are still some issues that we must address. First, although numerous nanocarriers have been developed for low-temperature PTT and have shown satisfactory results, such as efficient drug encapsulation rate and loading capacity, low toxicity to normal cells, and fine biocompatibility, more effective nanocarriers needs to be discovered. First, to improve the delivery capacity, enhance biodegradability, and prolong blood circulation time and stability, more studies should carry out in order to achieve low-temperature PTT. For example, a nanoplatform to target organelles other than mitochondria and lysosomes could be designed, and the effect of acting on different organelles to optimize the targeting of organelles for low-temperature PTT should be compared. In addition, novel therapeutic modalities including iron death, transgenic technology, and gene editing technology, can also be further explored in relation to low-temperature PTT. More importantly, we should identify more detailed mechanisms of action of these synergistic treatments develop more effective combinations and sequences of applications. Finally, there are some reports on low-temperature PTT for other diseases, such as endocrine system diseases, neurological diseases and cardiovascular system diseases, which can further expand the research. Overall, the translation of nanomedicine carriers into real clinical applications still requires a lot of study and the joint efforts of more researchers.
Conceptualization, ZS (2nd author) and GD; investigation, YX, and ZS; resources, WL; writing-original draft preparation, ZS (2nd author), GD, YX and ZS; writing-review and editing, ZS (7th author), JL, MW and YC; visualization, GD; supervision, JL, MW and YC; funding acquisition, JL and GD All authors have read and agreed to the published version of the manuscript.
This work was supported by the Yantai Science and Technology Plan Project (no: 2022MSGY074 for JL), The National Natural Science Foundation of China (no. 32000982 for GD), and Guangdong Province Basic and Applied Basic Research Fund Project (no. 2021A1515220108 for GD).
The authors declare that the research was conducted in the absence of any commercial or financial relationships that could be construed as a potential conflict of interest.
All claims expressed in this article are solely those of the authors and do not necessarily represent those of their affiliated organizations, or those of the publisher, the editors and the reviewers. Any product that may be evaluated in this article, or claim that may be made by its manufacturer, is not guaranteed or endorsed by the publisher.
Andraos, C., and Gulumian, M. (2021). Intracellular and extracellular targets as mechanisms of cancer therapy by nanomaterials in relation to their physicochemical properties. Wiley Interdiscip. Rev. Nanomed. Nanobiotechnol. 13, e1680. Wiley interdisciplinary reviews. doi:10.1002/wnan.1680
Baud, V., and Karin, M. (2001). Signal transduction by tumor necrosis factor and its relatives. Trends Cell Biol. 11, 372–377. doi:10.1016/s0962-8924(01)02064-5
Bhat, R., Adam, A., Lee, J., Gasiewicz, T., Henry, E., and Rotella, D. (2014). Towards the discovery of drug-like epigallocatechin gallate analogs as Hsp90 inhibitors. Bioorg. Med. Chem. Lett. 24, 2263–2266. doi:10.1016/j.bmcl.2014.03.088
Bonfil, R., Bustuoabad, O., Ruggiero, R., Meiss, R., and Pasqualini, C. (1988). Tumor necrosis can facilitate the appearance of metastases. Clin. Exp. Metastasis 6, 121–129. doi:10.1007/bf01784843
Brünnert, D., Langer, C., Zimmermann, L., Bargou, R., Burchardt, M., Chatterjee, M., et al. (2020). The heat shock protein 70 inhibitor VER155008 suppresses the expression of HSP27, HOP and HSP90β and the androgen receptor, induces apoptosis, and attenuates prostate cancer cell growth. J. Cell. Biochem. 121, 407–417. doi:10.1002/jcb.29195
Cao, J., Qiao, B., Luo, Y., Cheng, C., Yang, A., Wang, M., et al. (2020). A multimodal imaging-guided nanoreactor for cooperative combination of tumor starvation and multiple mechanism-enhanced mild temperature phototherapy. Biomater. Sci. 8, 6561–6578. doi:10.1039/d0bm01350a
Chen, H., Guh, J., Tsai, J., and Lai, Y. (1999). Induction of heat shock protein 70 protects mesangial cells against oxidative injury. Kidney Int. 56, 1270–1273. doi:10.1046/j.1523-1755.1999.00693.x
Chen, W., Luo, G., Lei, Q., Hong, S., Qiu, W., Liu, L., et al. (2017). Overcoming the heat endurance of tumor cells by interfering with the anaerobic glycolysis metabolism for improved photothermal therapy. ACS Nano 11, 1419–1431. doi:10.1021/acsnano.6b06658
Ciocca, D., and Calderwood, S. (2005). Heat shock proteins in cancer: Diagnostic, prognostic, predictive, and treatment implications. Cell Stress Chaperones 10, 86–103. doi:10.1379/csc-99r.1
Davenport, J., Manjarrez, J., Peterson, L., Krumm, B., Blagg, B., and Matts, R. (2011). Gambogic acid, a natural product inhibitor of Hsp90. J. Nat. Prod. (Gorakhpur). 74, 1085–1092. doi:10.1021/np200029q
De Marco, M., Basile, A., Iorio, V., Festa, M., Falco, A., Ranieri, B., et al. (2018). Role of BAG3 in cancer progression: A therapeutic opportunity. Seminars Cell & Dev. Biol. 78, 85–92. doi:10.1016/j.semcdb.2017.08.049
De Melo-Diogo, D., Pais-Silva, C., Dias, D., Moreira, A., and Correia, I. (2017). Strategies to improve cancer photothermal therapy mediated by nanomaterials. Adv. Healthc. Mat. 6, 1700073. doi:10.1002/adhm.201700073
De Souza, R., and De Giovani, W. (2004). Antioxidant properties of complexes of flavonoids with metal ions. Redox Rep. 9, 97–104. doi:10.1179/135100004225003897
Deng, X., Guan, W., Qing, X., Yang, W., Que, Y., Tan, L., et al. (2020). Ultrafast low-temperature photothermal therapy activates autophagy and recovers immunity for efficient antitumor treatment. ACS Appl. Mat. Interfaces 12, 4265–4275. doi:10.1021/acsami.9b19148
Ding, F., Gao, X., Huang, X., Ge, H., Xie, M., Qian, J., et al. (2020). Polydopamine-coated nucleic acid nanogel for siRNA-mediated low-temperature photothermal therapy. Biomaterials 245, 119976. doi:10.1016/j.biomaterials.2020.119976
Doughty, A., Hoover, A., Layton, E., Murray, C., Howard, E., and Chen, W. (2019). Nanomaterial applications in photothermal therapy for cancer. Materials 12, 779. doi:10.3390/ma12050779
Elmallah, M., Cordonnier, M., Vautrot, V., Chanteloup, G., Garrido, C., and Gobbo, J. (2020). Membrane-anchored heat-shock protein 70 (Hsp70) in cancer. Cancer Lett. 469, 134–141. doi:10.1016/j.canlet.2019.10.037
Fernandes, N., Rodrigues, C., Moreira, A., and Correia, I. (2020). Overview of the application of inorganic nanomaterials in cancer photothermal therapy. Biomater. Sci. 8, 2990–3020. doi:10.1039/d0bm00222d
Fu, Z., and Jia, B. (2022). Advances in the role of heat shock protein 90 in prostate cancer. Andrologia 54, e14376. doi:10.1111/and.14376
Fu, Z., Williams, G., Niu, S., Wu, J., Gao, F., Zhang, X., et al. (2020). Functionalized boron nanosheets as an intelligent nanoplatform for synergistic low-temperature photothermal therapy and chemotherapy. Nanoscale 12, 14739–14750. doi:10.1039/d0nr02291h
Gao, G., Jiang, Y., Sun, W., Guo, Y., Jia, H., Yu, X., et al. (2019). Molecular targeting-mediated mild-temperature photothermal therapy with a smart albumin-based nanodrug. Small (Weinheim der Bergstrasse, Ger. 15, e1900501. doi:10.1002/smll.201900501
Gao, G., Jiang, Y., Guo, Y., Jia, H., Cheng, X., Deng, Y., et al. (2020). Enzyme-mediated tumor starvation and phototherapy enhance mild-temperature photothermal therapy. Adv. Funct. Mat. 30, 1909391. doi:10.1002/adfm.201909391
Green, D. (2020). Ghostly metabolic messages from dying cells. Nature 580, 36–37. doi:10.1038/d41586-020-00641-0
Guo, S., and Wang, E. (2011). Functional micro/nanostructures: Simple synthesis and application in sensors, fuel cells, and gene delivery. Acc. Chem. Res. 44, 491–500. doi:10.1021/ar200001m
Hong, D. S., Banerji, U., Tavana, B., George, G. C., Aaron, J., and Kurzrock, R. (2013). Targeting the molecular chaperone heat shock protein 90 (HSP90): Lessons learned and future directions. Cancer Treat. Rev. 39, 375–387. doi:10.1016/j.ctrv.2012.10.001
Hoter, A., El-Sabban, M., and Naim, H. (2018). The HSP90 family: Structure, regulation, function, and implications in health and disease. Int. J. Mol. Sci. 19, 2560. doi:10.3390/ijms19092560
Hu, H., Deng, X., Song, Q., Yang, W., Zhang, Y., Liu, W., et al. (2021). Mitochondria-targeted accumulation of oxygen-irrelevant free radicals for enhanced synergistic low-temperature photothermal and thermodynamic therapy. J. Nanobiotechnology 19, 390. doi:10.1186/s12951-021-01142-6
Jiang, A., Liu, Y., Ma, L., Mao, F., Liu, L., Zhai, X., et al. (2019). Biocompatible heat-shock protein inhibitor-delivered flowerlike short-wave infrared nanoprobe for mild temperature-driven highly efficient tumor ablation. ACS Appl. Mat. Interfaces 11, 6820–6828. doi:10.1021/acsami.8b21483
Jing, L., Qu, H., Wu, D., Zhu, C., Yang, Y., Jin, X., et al. (2018). Platelet-camouflaged nanococktail: Simultaneous inhibition of drug-resistant tumor growth and metastasis via a cancer cells and tumor vasculature dual-targeting strategy. Theranostics 8, 2683–2695. doi:10.7150/thno.23654
Jordan, A., Racine, R., Hennig, M., and Lokeshwar, V. (2015). The role of CD44 in disease pathophysiology and targeted treatment. Front. Immunol. 6, 182. doi:10.3389/fimmu.2015.00182
Jung, B., Lee, Y., Hong, J., Ghandehari, H., and Yun, C. (2016). Mild hyperthermia induced by gold nanorod-mediated plasmonic photothermal therapy enhances transduction and replication of oncolytic adenoviral gene delivery. ACS Nano 10, 10533–10543. doi:10.1021/acsnano.6b06530
Kabakov, A., and Gabai, V. (2021). HSP70s in breast cancer: Promoters of tumorigenesis and potential targets/tools for therapy. Cells 10, 3446. doi:10.3390/cells10123446
Kampinga, H. (1993). Thermotolerance in mammalian cells. Protein denaturation and aggregation, and stress proteins. J. Cell Sci. 104, 11–17. doi:10.1242/jcs.104.1.11
Lang, B. J., Guerrero-Gimenez, M. E., Prince, T. L., Ackerman, A., Bonorino, C., and Calderwood, S. K. (2019). Heat shock proteins are essential components in transformation and tumor progression: Cancer cell intrinsic pathways and beyond. Int. J. Mol. Sci. 20, 4507. doi:10.3390/ijms20184507
Lee, H., and Gaharwar, A. (2020). Light-responsive inorganic biomaterials for biomedical applications. Adv. Sci. (Weinh). 7, 2000863. doi:10.1002/advs.202000863
Lee, S., Chung, B., Park, T., Nam, Y., and Mok, H. (2012). Small-interfering RNA (siRNA)-based functional micro- and nanostructures for efficient and selective gene silencing. Acc. Chem. Res. 45, 1014–1025. doi:10.1021/ar2002254
Lei, Z., Xia, X., He, Q., Luo, J., Xiong, Y., Wang, J., et al. (2021). HSP70 promotes tumor progression by stabilizing Skp2 expression in gastric cancer cells. Mol. Carcinog. 60, 826–839. doi:10.1002/mc.23346
Li, G., Mivechi, N., and Weitzel, G. (1995). Heat shock proteins, thermotolerance, and their relevance to clinical hyperthermia. Int. J. Hyperth. 11, 459–488. doi:10.3109/02656739509022483
Li, J., Zhu, D., Ma, W., Yang, Y., Wang, G., Wu, X., et al. (2020a). Rapid synthesis of a Bi@ZIF-8 composite nanomaterial as a near-infrared-II (NIR-II) photothermal agent for the low-temperature photothermal therapy of hepatocellular carcinoma. Nanoscale 12, 17064–17073. doi:10.1039/d0nr03907a
Li, X., Lovell, J., Yoon, J., and Chen, X. (2020b). Clinical development and potential of photothermal and photodynamic therapies for cancer. Nat. Rev. Clin. Oncol. 17, 657–674. doi:10.1038/s41571-020-0410-2
Liang, H., Wang, Z., Lei, Q., Huang, R., Deng, Y., Wang, Q., et al. (2015). Molecular cloning and expression analysis of a pearl oyster (Pinctada martensii) heat shock protein 90 (HSP90). Genet. Mol. Res. 14, 18778–18791. doi:10.4238/2015.december.28.27
Liu, K., Liu, H., Qi, J., Liu, Q., Liu, Z., Xia, M., et al. (2012). SNX-2112, an Hsp90 inhibitor, induces apoptosis and autophagy via degradation of Hsp90 client proteins in human melanoma A-375 cells. Cancer Lett. 318, 180–188. doi:10.1016/j.canlet.2011.12.015
Liu, G., Xu, J., and Wang, K. (2017). Solar water evaporation by black photothermal sheets. Nano Energy 41, 269–284. doi:10.1016/j.nanoen.2017.09.005
Liu, Y., Bhattarai, P., Dai, Z., and Chen, X. (2019). Photothermal therapy and photoacoustic imaging via nanotheranostics in fighting cancer. Chem. Soc. Rev. 48, 2053–2108. doi:10.1039/c8cs00618k
Lu, W., Yao, J., Zhu, X., and Qi, Y. (2021). Nanomedicines: Redefining traditional medicine. Biomed. Pharmacother. 134, 111103. doi:10.1016/j.biopha.2020.111103
Lv, Z., He, S., Wang, Y., and Zhu, X. (2021). Noble metal nanomaterials for NIR-triggered photothermal therapy in cancer. Adv. Healthc. Mat. 10, e2001806. doi:10.1002/adhm.202001806
Macheda, M., Rogers, S., and Best, J. (2005). Molecular and cellular regulation of glucose transporter (GLUT) proteins in cancer. J. Cell. Physiol. 202, 654–662. doi:10.1002/jcp.20166
Marchan, J. (2019). In silico identification of epitopes present in human heat shock proteins (HSPs) overexpressed by tumour cells. J. Immunol. methods 471, 34–45. doi:10.1016/j.jim.2019.05.005
Marzullo, L., Turco, M., and De Marco, M. (2020). The multiple activities of BAG3 protein: Mechanisms. Biochimica Biophysica Acta - General Subj. 1864, 129628. doi:10.1016/j.bbagen.2020.129628
Melancon, M., Zhou, M., and Li, C. (2011). Cancer theranostics with near-infrared light-activatable multimodal nanoparticles. Acc. Chem. Res. 44, 947–956. doi:10.1021/ar200022e
Middleton, E., Kandaswami, C., and Theoharides, T. (2000). The effects of plant flavonoids on mammalian cells: Implications for inflammation, heart disease, and cancer. Pharmacol. Rev. 52, 673–751. doi:10.0000/PMID11121513
Miyagawa, T., Saito, H., Minamiya, Y., Mitobe, K., Takashima, S., Takahashi, N., et al. (2014). Inhibition of Hsp90 and 70 sensitizes melanoma cells to hyperthermia using ferromagnetic particles with a low Curie temperature. Int. J. Clin. Oncol. 19, 722–730. doi:10.1007/s10147-013-0606-x
Morimoto, R. (1998). Regulation of the heat shock transcriptional response: Cross talk between a family of heat shock factors, molecular chaperones, and negative regulators. Genes Dev. 12, 3788–3796. doi:10.1101/gad.12.24.3788
Nath, K., Guo, L., Nancolas, B., Nelson, D., Shestov, A., Lee, S., et al. (2016). Mechanism of antineoplastic activity of lonidamine. Biochimica Biophysica Acta - Rev. Cancer 1866, 151–162. doi:10.1016/j.bbcan.2016.08.001
Ni, J., Zhang, X., Yang, G., Kang, T., Lin, X., Zha, M., et al. (2020). A photoinduced nonadiabatic decay-guided molecular motor triggers effective photothermal conversion for cancer therapy. Angew. Chem. Int. Ed. Engl. 59, 11394–11398. doi:10.1002/ange.202002516
Niwa, M., Hotta, K., Hara, A., Hirade, K., Ito, H., Kato, K., et al. (2006). TNF-Alpha decreases hsp 27 in human blood mononuclear cells: Involvement of protein kinase c. Life Sci. 80, 181–186. doi:10.1016/j.lfs.2006.08.035
Obuobi, S., Tay, H., Tram, N., Selvarajan, V., Khara, J., Wang, Y., et al. (2019). Facile and efficient encapsulation of antimicrobial peptides via crosslinked DNA nanostructures and their application in wound therapy. J. Control. Release 313, 120–130. doi:10.1016/j.jconrel.2019.10.013
Palermo, C., Westlake, C., and Gasiewicz, T. (2005). Epigallocatechin gallate inhibits aryl hydrocarbon receptor gene transcription through an indirect mechanism involving binding to a 90 kDa heat shock protein. Biochemistry 44, 5041–5052. doi:10.1021/bi047433p
Pelaz, B., Alexiou, C., Alvarez-Puebla, R., Alves, F., Andrews, A., Ashraf, S., et al. (2017). Diverse applications of nanomedicine. ACS Nano 11, 2313–2381. doi:10.1021/acsnano.6b06040
Peng, Z., Yuan, L., Xuhong, J., Tian, H., Zhang, Y., Deng, J., et al. (2021). Chiral nanomaterials for tumor therapy: Autophagy, apoptosis, and photothermal ablation. J. Nanobiotechnology 19, 220. doi:10.1186/s12951-021-00965-7
Pinto, A., and Pocard, M. (2018). Photodynamic therapy and photothermal therapy for the treatment of peritoneal metastasis: A systematic review. Pleura Perit. 3, 20180124. doi:10.1515/pp-2018-0124
Ren, Y., Yan, Y., and Qi, H. (2022). Photothermal conversion and transfer in photothermal therapy: From macroscale to nanoscale. Adv. Colloid Interface Sci. 308, 102753. doi:10.1016/j.cis.2022.102753
Rosati, A., Graziano, V., De Laurenzi, V., Pascale, M., and Turco, M. (2011). BAG3: A multifaceted protein that regulates major cell pathways. Cell Death Dis. 2, e141. doi:10.1038/cddis.2011.24
Sakai, K., Inoue, M., Mikami, S., Nishimura, H., Kuwabara, Y., Kojima, A., et al. (2021). Functional inhibition of heat shock protein 70 by VER-155008 suppresses pleural mesothelioma cell proliferation via an autophagy mechanism. Thorac. Cancer 12, 491–503. doi:10.1111/1759-7714.13784
Schett, G., Steiner, C., Xu, Q., Smolen, J., and Steiner, G. (2003). TNFα mediates susceptibility to heat-induced apoptosis by protein phosphatase-mediated inhibition of the HSF1/hsp70 stress response. Cell Death Differ. 10, 1126–1136. doi:10.1038/sj.cdd.4401276
Seclì, L., Fusella, F., Avalle, L., and Brancaccio, M. (2021). The dark-side of the outside: How extracellular heat shock proteins promote cancer. Cell. Mol. Life Sci. 78, 4069–4083. doi:10.1007/s00018-021-03764-3
Shao, L., Li, Y., Huang, F., Wang, X., Lu, J., Jia, F., et al. (2020). Complementary autophagy inhibition and glucose metabolism with rattle-structured polydopamine@mesoporous silica nanoparticles for augmented low-temperature photothermal therapy and in vivo photoacoustic imaging. Theranostics 10, 7273–7286. doi:10.7150/thno.44668
Sharapova, T., Romanova, E., Ivanova, O., Yashin, D., and Sashchenko, L. (2021). Hsp70 interacts with the TREM-1 receptor expressed on monocytes and thereby stimulates generation of cytotoxic lymphocytes active against MHC-negative tumor cells. Int. J. Mol. Sci. 22, 6889. doi:10.3390/ijms22136889
Sherman, M., and Multhoff, G. (2007). Heat shock proteins in cancer. Ann. N. Y. Acad. Sci. 1113, 192–201. doi:10.1196/annals.1391.030
Song, L., Dong, X., Zhu, S., Zhang, C., Yin, W., Zhang, X., et al. (2018). Bi2S3-Tween 20 nanodots loading PI3K inhibitor, LY294002, for mild photothermal therapy of LoVo cells in vitro and in vivo. Adv. Healthc. Mat. 7, e1800830. doi:10.1002/adhm.201800830
Song, Y., Wang, Y., Zhu, Y., Cheng, Y., Wang, Y., Wang, S., et al. (2019). Biomodal tumor‐targeted and redox‐responsive Bi 2 Se 3 hollow nanocubes for MSOT/CT imaging guided synergistic low‐temperature photothermal radiotherapy. Adv. Healthc. Mat. 8, e1900250. doi:10.1002/adhm.201900250
Stebbins, C., Russo, A., Schneider, C., Rosen, N., Hartl, F., and Pavletich, N. (1997). Crystal structure of an hsp90-geldanamycin complex: Targeting of a protein chaperone by an antitumor agent. Cell 89, 239–250. doi:10.1016/s0092-8674(00)80203-2
Subjakova, V., Oravczova, V., and Hianik, T. (2021). Polymer nanoparticles and nanomotors modified by DNA/RNA aptamers and antibodies in targeted therapy of cancer. Polymers 13, 341. doi:10.3390/polym13030341
Sun, J., Li, Y., Teng, Y., Wang, S., Guo, J., and Wang, C. (2020). NIR-controlled HSP90 inhibitor release from hollow mesoporous nanocarbon for synergistic tumor photothermal therapy guided by photoacoustic imaging. Nanoscale 12, 14775–14787. doi:10.1039/d0nr02896g
Sun, Q., Tang, K., Song, L., Li, Y., Pan, W., Li, N., et al. (2021a). Covalent organic framework based nanoagent for enhanced mild-temperature photothermal therapy. Biomater. Sci. 9, 7977–7983. doi:10.1039/d1bm01245b
Sun, Z., Deng, G., Peng, X., Xu, X., Liu, L., Peng, J., et al. (2021b). Intelligent photothermal dendritic cells restart the cancer immunity cycle through enhanced immunogenic cell death. Biomaterials 279, 121228. doi:10.1016/j.biomaterials.2021.121228
Tang, J., Shao, X., Gao, F., Jin, H., Zhou, J., Du, L., et al. (2011). Effect of hyperthermia on invasion ability and TGF-β1 expression of breast carcinoma MCF-7 cells. Oncol. Rep. 25, 1573–1579. doi:10.3892/or.2011.1240
Tang, X., Tan, L., Shi, K., Peng, J., Xiao, Y., Li, W., et al. (2018). Gold nanorods together with HSP inhibitor-VER-155008 micelles for colon cancer mild-temperature photothermal therapy. Acta Pharm. Sin. B 8, 587–601. doi:10.1016/j.apsb.2018.05.011
Todurga Seven, Z., Tombulturk, F., Gokdemir, S., and Ozyazgan, S. (2022). The effects of the heat shock protein 90 inhibitor 17-allylamino-17-demethoxygeldanamycin, cannabinoid agonist WIN 55, 212-2, and nitric oxide synthase inhibitor nω-nitro-L-arginine methyl ester hydrochloride on the serotonin and dry skin-induced itch. Int. Arch. Allergy Immunol. 183, 443–452. doi:10.1159/000520509
Valko, M., Rhodes, C., Moncol, J., Izakovic, M., and Mazur, M. (2006). Free radicals, metals and antioxidants in oxidative stress-induced cancer. Chemico-biological Interact. 160, 1–40. doi:10.1016/j.cbi.2005.12.009
Wang, B., Yu, X., Wang, J., Li, Z., Li, P., Wang, H., et al. (2016a). Gold-nanorods-siRNA nanoplex for improved photothermal therapy by gene silencing. Biomaterials 78, 27–39. doi:10.1016/j.biomaterials.2015.11.025
Wang, S., Tian, Y., Tian, W., Sun, J., Zhao, S., Liu, Y., et al. (2016b). Selectively sensitizing malignant cells to photothermal therapy using a CD44-targeting heat shock protein 72 depletion nanosystem. ACS Nano 10, 8578–8590. doi:10.1021/acsnano.6b03874
Wang, Z., Li, S., Zhang, M., Ma, Y., Liu, Y., Gao, W., et al. (2017). Laser-triggered small interfering RNA releasing gold nanoshells against heat shock protein for sensitized photothermal therapy. Adv. Sci. (Weinh). 4, 1600327. doi:10.1002/advs.201600327
Wang, S., Zhang, X., Wang, H., Wang, Y., Chen, P., and Wang, L. (2020). Heat shock protein 27 enhances SUMOylation of heat shock protein B8 to accelerate the progression of breast cancer. Am. J. pathology 190, 2464–2477. doi:10.1016/j.ajpath.2020.04.012
Wang, L., Feng, Y., Wang, K., and Liu, G. (2021a). Solar water sterilization enabled by photothermal nanomaterials. Nano Energy 87, 106158. doi:10.1016/j.nanoen.2021.106158
Wang, P., Kankala, R., Chen, B., Zhang, Y., Zhu, M., Li, X., et al. (2021b). Cancer cytomembrane-cloaked prussian blue nanoparticles enhance the efficacy of mild-temperature photothermal therapy by disrupting mitochondrial functions of cancer cells. ACS Appl. Mat. Interfaces 13, 37563–37577. doi:10.1021/acsami.1c11138
Wang, Y., Meng, H., and Li, Z. (2021c). Near-infrared inorganic nanomaterial-based nanosystems for photothermal therapy. Nanoscale 13, 8751–8772. doi:10.1039/d1nr00323b
Wigmore, S., Sangster, K., Mcnally, S., Harrison, E., Ross, J., Fearon, K., et al. (2007). De-repression of heat shock transcription factor-1 in interleukin-6- treated hepatocytes is mediated by downregulation of glycogen synthase kinase 3beta and MAPK/ERK-1. Int. J. Mol. Med. 19, 413–420.
Wu, C. (1995). Heat shock transcription factors: Structure and regulation. Annu. Rev. Cell Dev. Biol. 11, 441–469. doi:10.1146/annurev.cb.11.110195.002301
Wu, J., Bremner, D., Niu, S., Shi, M., Wang, H., Tang, R., et al. (2018). Chemodrug-gated biodegradable hollow mesoporous organosilica nanotheranostics for multimodal imaging-guided low-temperature photothermal therapy/chemotherapy of cancer. ACS Appl. Mat. Interfaces 10, 42115–42126. doi:10.1021/acsami.8b16448
Xia, J., Qing, X., Shen, J., Ding, M., Wang, Y., Yu, N., et al. (2021). Enzyme-loaded pH-sensitive photothermal hydrogels for mild-temperature-mediated combinational cancer therapy. Front. Chem. 9, 736468. doi:10.3389/fchem.2021.736468
Yan, L., Zhang, W., Zhang, B., Xuan, C., and Wang, D. (2017). BIIB021: A novel inhibitor to heat shock protein 90-addicted oncology. Tumour. Biol. 39, 101042831769835. doi:10.1177/1010428317698355
Yang, Y., Zhu, W., Dong, Z., Chao, Y., Xu, L., Chen, M., et al. (2017). 1D coordination polymer nanofibers for low-temperature photothermal therapy. Adv. Mat. 29, 3588. doi:10.1002/adma.201703588
Yang, G., Zhou, D., Pan, Z., Yang, J., Zhang, D., Cao, Q., et al. (2019). Multifunctional low-temperature photothermal nanodrug with in vivo clearance, ROS-Scavenging and anti-inflammatory abilities. Biomaterials 216, 119280. doi:10.1016/j.biomaterials.2019.119280
Yi, X., Duan, Q., and Wu, F. (2021). Low-temperature photothermal therapy: Strategies and applications. Res. Wash. D.C.) 2021, 9816594. doi:10.34133/2021/9816594
You, C., Li, Y., Dong, Y., Ning, L., Zhang, Y., Yao, L., et al. (2020). Low-temperature trigger nitric oxide nanogenerators for enhanced mild photothermal therapy. ACS Biomater. Sci. Eng. 6, 1535–1542. doi:10.1021/acsbiomaterials.9b01771
Yu, Z., Chan, W., Zhang, Y., and Tan, T. (2021). Near-infrared-II activated inorganic photothermal nanomedicines. Biomaterials 269, 120459. doi:10.1016/j.biomaterials.2020.120459
Yun, I., Lee, M., Rah, D., Lew, D., Park, J., and Lee, W. (2015). Heat shock protein 90 inhibitor (17-AAG) induces apoptosis and decreases cell migration/motility of keloid fibroblasts. Plastic Reconstr. Surg. 136, 44e–53e. doi:10.1097/prs.0000000000001362
Zafar, H., Raza, F., Ma, S., Wei, Y., Zhang, J., and Shen, Q. (2021). Recent progress on nanomedicine-induced ferroptosis for cancer therapy. Biomater. Sci. 9, 5092–5115. doi:10.1039/d1bm00721a
Zhang, F., Wu, Q., and Liu, H. (2020). NIR light-triggered nanomaterials-based prodrug activation towards cancer therapy. Wiley Interdiscip. Rev. Nanomed. Nanobiotechnol. 12, e1643. doi:10.1002/wnan.1643
Zhang, T., Wu, B., Akakuru, O., Yao, C., Sun, S., Chen, L., et al. (2021). Hsp90 inhibitor-loaded IR780 micelles for mitochondria-targeted mild-temperature photothermal therapy in xenograft models of human breast cancer. Cancer Lett. 500, 41–50. doi:10.1016/j.canlet.2020.12.028
Zhao, W., Qiu, Y., and Kong, D. (2017). Class I phosphatidylinositol 3-kinase inhibitors for cancer therapy. Acta Pharm. Sin. B 7, 27–37. doi:10.1016/j.apsb.2016.07.006
Zhou, F., Wang, H., and Chang, J. (2016). Progress in the field of constructing near-infrared light-responsive drug delivery platforms. J. Nanosci. Nanotechnol. 16, 2111–2125. doi:10.1166/jnn.2016.10945
Zhou, J., Li, M., Hou, Y., Luo, Z., Chen, Q., Cao, H., et al. (2018). Engineering of a nanosized biocatalyst for combined tumor starvation and low-temperature photothermal therapy. ACS Nano 12, 2858–2872. doi:10.1021/acsnano.8b00309
Keywords: low-temperature, photothermal therapy, heat shock proteins, nanotechnology, nanomaterial
Citation: Xin Y, Sun Z, Liu J, Li W, Wang M, Chu Y, Sun Z and Deng G (2022) Nanomaterial-mediated low-temperature photothermal therapy via heat shock protein inhibition. Front. Bioeng. Biotechnol. 10:1027468. doi: 10.3389/fbioe.2022.1027468
Received: 25 August 2022; Accepted: 16 September 2022;
Published: 11 October 2022.
Edited by:
Liangliang Dai, Northwestern Polytechnical University, ChinaReviewed by:
Kaiying Wang, University of South-Eastern Norway, NorwayCopyright © 2022 Xin, Sun, Liu, Li, Wang, Chu, Sun and Deng. This is an open-access article distributed under the terms of the Creative Commons Attribution License (CC BY). The use, distribution or reproduction in other forums is permitted, provided the original author(s) and the copyright owner(s) are credited and that the original publication in this journal is cited, in accordance with accepted academic practice. No use, distribution or reproduction is permitted which does not comply with these terms.
*Correspondence: Zhihong Sun, aG9uZ3poaXN1bjQ5MEAxNjMuY29t; Guanjun Deng, ZGVuZ2dqM0BtYWlsLnN5c3UuZWR1LmNu
†These authors have contributed equally to this work
Disclaimer: All claims expressed in this article are solely those of the authors and do not necessarily represent those of their affiliated organizations, or those of the publisher, the editors and the reviewers. Any product that may be evaluated in this article or claim that may be made by its manufacturer is not guaranteed or endorsed by the publisher.
Research integrity at Frontiers
Learn more about the work of our research integrity team to safeguard the quality of each article we publish.