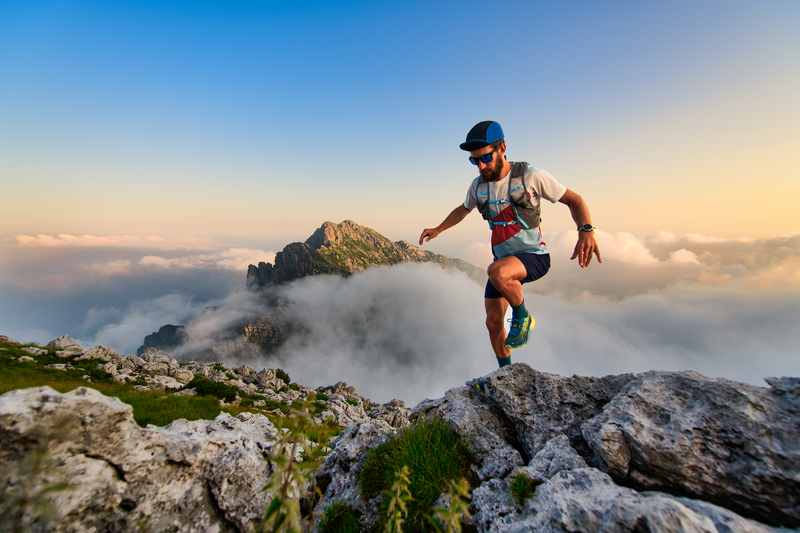
95% of researchers rate our articles as excellent or good
Learn more about the work of our research integrity team to safeguard the quality of each article we publish.
Find out more
ORIGINAL RESEARCH article
Front. Bioeng. Biotechnol. , 31 October 2022
Sec. Industrial Biotechnology
Volume 10 - 2022 | https://doi.org/10.3389/fbioe.2022.1007004
Several Levilactobacillus brevis strains have the potential to be used as probiotics since they provide health benefits due to the interaction of live cells, and of their secreted products, with the host (tissues). Therefore, the development of simple fermentation processes that improve cell viability to reduce industrial production costs, and at the same time the characterization and biological evaluation of cell-free postbiotics that can further promote application, are of great interest. In the present study, small scale batch fermentations on semi defined media, deprived of animal derived raw materials, were used to optimize growth of L. brevis SP48, reaching 1.2 ± 0.4 × 1010 CFU/ml of viable cells after 16 h of growth. Displacement, competition, and inhibition assays compared the effect, on Helicobacter pylori, of L. brevis cells to that of its partially purified potentially postbiotic fraction rich in exopolysaccharides and proteins. The expression of pro and anti-inflammatory biochemical markers indicated that both samples activated antimicrobial defenses and innate immunity in a gastric model. Moreover, these compounds also acted as modulators of the inflammatory response in a gut in vitro model. These data demonstrate that the high molecular weight compounds secreted by L. brevis SP48 can contrast H. pylori and reduce inflammation related to intestinal bowel disease, potentially overcoming issues related to the preservation of probiotic viability.
Besides targeting gastrointestinal disorders probiotics such as some lactobacilli have been reported to improve immunomodulation and protection of the host against infections caused by viral and bacterial pathogens (Di Cerbo et al., 2016). Interestingly, also inactivated microbial (probiotic) cells and their metabolic products, indicated as parabiotics and postbiotics (Aguilar-Toala et al., 2018), respectively, are crucial and act as mediators in the modulation of the host’s immune function (Teame et al., 2020). Moreover, the use of cell structural components and metabolites reduces safety concerns regarding potential theoretical risks that have been described in specific conditions in relation to live probiotic administrations (e.g. experimental models, systemic infections, deleterious metabolic activities, excessive immune stimulation in susceptible individuals, gene transfer and gastrointestinal side effects) (Doron and Snydman, 2015). Therefore overall, health preservation can be improved on the one side by the direct competition of probiotics with pathogens through nutrient subtraction and production of antimicrobial and immunostimulatory molecules (postbiotics), and on the other hand by the interaction of specific parabiotics with human cells via mucosa-associated lymphoid tissue, resulting in the activation of the immune system (Taverniti and Guglielmetti, 2011).
Levilactobacillus brevis (previously identified as Lactobacillus brevis) is an obligate heterofermentative LAB isolated from plants, food, and human microbiota. In addition to common and demonstrated probiotic properties (Kishi et al., 1996; Suzuki et al., 2013; Abdelazez et al., 2018) several L. brevis strains also possess other features. L. brevis NCL912 and L. brevis CGMCC1306, for instance, have the natural ability to produce γ-aminobutyric acid (GABA), a neurotransmitter that reduces the threat of type 1 diabetes (Wu and Shah, 2017; Lyu et al., 2018; Wang et al., 2018; Wu and Shah, 2018). L. brevis SP48 is present in commercial products (e.g. Florap lady) designed for women to promote the balance of the intestinal flora and the functionality of the urinary tract. It was also evaluated as a tool to target the dysbiosis associated with periodontal diseases (Giannini et al., 2022).
Among the array of molecules produced by LAB, polysaccharides attached on the cell surface or secreted in the medium from numerous probiotic strains showed a wide range of properties as antitumor, immunostimulatory, cholesterol-lowering, and promoting adhesion to intestinal epithelial cells (Živković et al., 2016; Wei et al., 2019; Butorac et al., 2021). Those produced by L. brevis KB290 and L. brevis CD2 demonstrated to have a critical role in increasing cell-mediated cytotoxic activity in mouse spleen (Sasaki et al., 2015) and enhancing inhibition of the gut pathogen Salmonella typhimurium, respectively (Alfano et al., 2020). The composition of the exopolysaccharides (EPS) produced by few L. brevis strains has been previously described suggesting the polysaccharide to be either a glucose homotype or a glucose and N-acetylglucosamine heterotype polymer (Suzuki et al., 2013; Fraunhofer et al., 2018; Fukao et al., 2019).
To enhance the production of viable biomass and/or the synthesis of potentially bioactive compounds, such as EPS, efficient fermentation processes are necessary. Batch and fed-batch experiments on semidefined media previously investigated the final concentration of biomass, in terms of viable cells, and the production of related products focusing on the pH, the aeration, and on the feeding strategy (Martesson et al., 2003; Brooijmans et al., 2009; Alfano et al., 2020). Also, the simplification of the cultivation medium and the reduction of cultivation time are crucial aspects, especially considering potential industrial development. Another issue regards the identification of simple and industrially suitable purification processes to obtain bioactive postbiotic fractions applicable in the biomedical field.
In this regard the aim of the present study was to investigate growth of L. brevis SP48 in 2l-batch processes to favor the production of biomass and live cells and to assess whether a simple and industrially applicable downstream strategy could provide potentially bioactive secreted fractions.
Recent literature reports that extracellular polysaccharides can modulate intestinal immune responses by regulating Toll-like receptors (e.g TLR-2 and 4) signaling pathways, which are responsible for the induction of cytokines and chemokines in response (Wachi et al., 2014).
We here investigated the anti-inflammatory effects of secreted potential postbiotic fractions, mainly composed of EPS and secreted proteins, recovered from the L. brevis fermentation broth, on human enterocytes obtained by the differentiation of Caco-2 cells. The potentially bioactive compounds were also evaluated on a gut inflammation model (using LPS from Salmonella minnesota) exploiting both enterocytes and NF-κB reporter THP-1 cells.
L. brevis CD2 was previously shown to reduce the intragastric load of Helicobacter pylori (Linsalata et al., 2004), and to inhibit oral pathogens causing mucositis and periodontal inflammation (Sharma et al., 2017). Therefore, in the present work a comparison of the antimicrobial effect of live cells and of the secreted potential postbiotics on H. pylori (ATCC) was investigated as well as the composition of the polysaccharides present in the partially purified supernatant by nuclear magnetic resonance (NMR), gas chromatography-mass spectrometry (GC-MS) and high-performance anion exchange chromatography (HPAE-PAD).
L. brevis SP48 (DSM deposit number 16806) was provided lyophilized by the “Centro Sperimentale del latte S.r.l.-” and stored at 4°C. Stocks were prepared from exponentially growing cells in Man, Rogosa and Sharpe (MRS) broth and conserved at −80°C in 20% v/v glycerol.
Human gastric adenocarcinoma cell-line AGS cells ATCC® CRL-1739™ (American Type Culture Collection, Rockville, MD, United States), were routinely cultured in Ham’s F-12K medium (Gibco, Waltham, Massachusetts,United States) supplemented with 1% Penstrep, 1% glutamine and 10% fetal calf serum (Gibco) at 37°C at 5% CO2.
Helicobacter pylori ATCC® 43629™ was cultured on Trypticase Soy Agar (Oxoid; Unipath, Basingstoke, United Kingdom) at 37°C under microaerophilic conditions for 2–4 days (as described on the ATCC website). L. brevis before use was cultured in MRS anaerobically at 37°C for 24–48 h, centrifuged at 4,000 × g, for 10 min, at 4°C, washed twice with saline, resuspended at the concentration of 0.5 OD in DMEM without antibiotics and added to cell cultures.
All medium components and salts were supplied by Sigma-Aldrich (St. Louis, MO, United States). Yeast extract was furnished by Organotechnie (La Corneuve, France), while sulphuric acid was purchased by Biochem s.r.l. (Turin, Italy). The semi-defined media used for growth experiments contained per liter: yeast extract 10 g, soy peptone 10 g, MgSO₄* 7 H₂O, 0.25 g, MnSO₄*H₂O 0.05 g, Na3C6H5O7 2 g, Tween 80 0.45 ml, L-ascorbic acid 0.5 g and NaCl 0.2 g. Glucose and fructose (Sigma-Aldrich, St. Louis, MO, United States) used as carbon sources, were filter sterilized and added to all semi-defined media after autoclaving.
Fermentation experiments were performed in a Biostat CT plus (Sartorius Stedim, Gottingen, Germany) bioreactor with a working volume of about 2.2 l. Temperature was controlled at 32°C, pH at 6.1 and agitation was fixed at 150 rpm. Batch experiments were conducted on a semidefined medium described in the previous paragraph. Fructose (30 ± 3 g/l) and glucose (32 ± 4 and 43 ± 2 g/l) were used as carbon sources. For batch experiments with 58 ± 1 g/l of glucose, the concentration of complex nitrogen sources was increased to 15 g/l of soya peptone and 20 g/l of yeast extract in the medium. Before each experiment, a concentrated stock solution (20 OD600, 2% v/v) was inoculated in 0.45 l medium and incubated at 32°C and 150 rpm for 8 h. A peristaltic pump (model 313 U, Watson-Marlow, England) was used to transfer the pre-culture inside the vessel reaching up to 10% (v/v) of the working volume inside the fermenter. The airflow was kept constant at 0.44 vvm, and stirring was set to 150 rpm. Experiments lasted from 16 to 19 h. Each fermentation experiment was performed at least in triplicate.
Dry cell weight was evaluated by centrifuging 50 ml of broth in a falcon tube at 5,000 × g at 4°C for 30 min. After discarding the supernatant, the pellet was washed with 0.5 volumes of saline solution and then transferred in a pre-weighted Eppendorf tube. The pellet was dried o/n at 70°C before measuring the dry weight. Viability was evaluated by serially diluting the samples and plating on MRS-agar medium. Plates were incubated at 37°C for 36 h before counting viable cells. Each sample was analyzed in triplicate.
Broth samples of about 10 ml were withdrawn during the experiments (at the time zero and after 16, 17, 18, and 19 h for fermentations on media 1 and 2, and only at time zero and after 16 h for fermentations on medium 3) for HPLC analyses of substrate consumption and acid production. The broth was centrifuged for 15 min at 10,000 ×g to separate the biomass and recover the supernatant. One ml was then UF/DF on 3 kDa centrifugal filter devices (Centricon, Amicon, and Sigma-Aldrich) at 10,000 ×g and the permeate was analysed for the determination of the concentrations of fructose, glucose, lactic and acetic acids and ethanol by HPLC (UHPLC Dionex Ultimate 3,000; Thermofisher) on a Alltech IOA-2000 column (500 mm × 6.5 mm ID) as previously reported (D’ambrosio et al., 2021).
The broth collected at the end of the batch processes was centrifuged at 5,000 ×g for 30 min at 4°C to separate biomass and supernatant. The supernatant was ultrafiltrated on 10 kDa cut-off polyether sulfone membranes (GE Healthcare, Illinois, United States) with a filtering area of 0.1 m2. Tangential flow filtration was performed on a Sartoflow alpha (Sartorius Stedim, Gottingen, and Germany) system connected with a thermostatic bath that kept a temperature of about 20–25°C. The retentate was concentrated about 6 fold and washed with 3 volumes of milliQ water to remove low molecular weight molecules still attached to the membrane, and salts. The retentate was then precipitated with 2 volumes of a 1:1 ethanol:acetone solution, after adjusting the conductivity to 20 mS/cm, and dried in a vacuum oven at 40°C over-night. The obtained powder was then suspended at 30 g/l in milliQ water and treated with 1% (w/v) of activated charcoal (Supelco Analytical, Sigma-Aldrich, Missouri, United States) in batch for 1 h at room temperature. The suspension was filtered on Velapad 60 filtration system (PALL Corporation, Milan, Italy) with nitrocellulose filters (BECO—PR Steril S80) purchased from Fluxa Filtri (Milan, Italy). The solution was precipitated, and the pellet was dried as described above. The recovered powder was used for assays described in the following paragraphs. Protein concentration was determined by Bradford assays (Bradford 1976).
By slightly modifying the protocol reported by Tallon and collaborators (2003) the cell-bound portion of the exopolysaccharide was extracted from L. brevis SP48 biomass obtained from fermentation processes. Briefly, 25 ml of broth were centrifuged at 6,500 rpm for 10 min at 4°C, the pellet was washed twice with sterile saline, and suspended it in 5 ml of EDTA 5 mg/ml (Sigma-Aldrich, MO, United States) and stirred gently for 4 h at 4°C. After incubation the suspension was centrifuged as described above, and the pellet was suspended in 10 ml of Dulbecco’s Phosphate-Buffered Saline (PBS, Gibco, California, United States). Two volumes of chilled ethanol (Sigma-Aldrich, MO, United States) were added and incubated at 4°C overnight. The suspension was centrifuged (6,500 rpm, 10 min, 4°C) and the precipitate was suspended in 10 ml of distilled water and dialyzed against 3 l of distilled water using a 3.5 kDa dialysis membrane (Delchimica Scientific Glassware, Napoli, Italy) for 2 days with three water changes per day. The recovered solution was lyophilized in a Beta 2-8 LD Plus Freeze Dryer (Christ, Osterode am Harz, Germany). The powder was suspended in 50 mmol/l Tris–HCl buffer at pH 8.6 (Sigma-Aldrich, MO, United States) and treated with 2 μg/ml DNase (Sigma-Aldrich, MO, United States) and 0.2 μg/ml RNase (Sigma-Aldrich, MO, United States) at 37°C for 6 h and following it was treated with 20 μg/ml proteinase K (Sigma-Aldrich, MO, United States) at 37°C for 16 h. The reaction was stopped by heating the solution at 95°C for 10 min. Ethanol precipitation and dialysis were repeated as described above. The obtained sample was dried in a vacuum oven (OV-11, Thermo Fisher Scientific, MA, United States) at 40°C overnight. This sample was hydrolysed and analysed by HPAE-PAD as described in paragraph 2.6.
Quantification of polysaccharides was performed by phenolsulfuric acid test (Dubois et al., 1956), a colorimetric assay used for the determination of total sugars in a sample. During hydrolysis pentoses are dehydrated to furfural, and hexoses to hydroxymethylfurfural producing a yellow-gold color in presence of phenol. The calibration curve was obtained with standard solutions of D (+) glucose at concentrations ranging from 0.01 to 0.1 mg/ml. Briefly, 200 μl of standards/sample were placed in a reaction tube with 200 μl of aqueous solution of phenol 5% w/v. Then, 1 ml of concentrated sulfuric acid (98% w/w) was added and the reaction tube was quickly closed and after vigorous stirring, the reaction was carried out for 30 min at 30°C. Sample absorbance was read at 490 nm. The blank was obtained by adding water to the reaction mixture.
Mono- and two-dimensional NMR spectra were recorded at 298 K, dissolved in D2O (sample concentration 2 mg/ml), by using a Bruker Avance-600 (1H: 600 MHz, and 13C: 150 MHz) spectrometer as reported previously (Fresno et al., 2007).
To determine the monosaccharide composition 1 mg of the sample deriving from the partial purification of the fermentation supernatant (containing potential postbiotics) was subjected to a methanolysis reaction with 1.25 M MeOH/HCl (80°C, 16 h), to obtain the O-methyl glycosides (Casillo et al., 2021). The methanol layer was extracted three times with hexane to remove the fatty acids eventually present, and dried. Then the O-methyl glycosides were acetylated with 25 μl of acetic anhydride and 25 μl of pyridine (100°C for 30 min). The acetylated methyl glycosides were dissolved in acetone and analysed by using an Agilent Technologies gas chromatograph 7820A equipped with a mass selective detector 5977B and a HP-5ms capillary column (Agilent, Italy 30 m × 0.25 mm i. d., flow rate 1 ml/min, He as carrier gas). The analysis was performed by using the following temperature program: 140°C for 3 min, then 140 → 240°C at 3 C/min.
The linkage pattern of the EPS was obtained by analyzing the partially methylated and acetylated alditol derivatives of the monosaccharide units. The linkages of the monosaccharides were recognized by their mass spectra fragmentation and by comparison of their retention times with those of authentic standards. The methylation reaction was carried out by using Ciucanu and Kerek procedure (Ciucanu and Kerek, 1984). The methylated EPS was hydrolyzed with trifluoroacetic acid, reduced with NaBD4, and acetylated with 25 μl of acetic anhydride and 25 μl of pyridine (100°C for 30 min). The partially methylated acetylated alditols dissolved in acetone were analyzed by GC–MS using the following temperature program: 90°C for 1 min, 90°C → 140°C at 25 C/min, 140°C → 200°C at 5 C/min, 200 °C → 280°C at 10 C/min, 280°C for 10 min. HPAEC-PAD (model ICS- 3,000, Dionex, CA, United States) was used to analyse the monosaccharides obtained after hydrolysis of the other partially purified fermentation supernatant containing the high molecular weight potentially bioactive compounds, on the treated unspent medium, and on the cell-bound polysaccharide sample obtained as described in paragraph 2.4. Before the analyses, samples (20 mg/ml powder) were hydrolysed in HCl 5 M for 6 h at 100°C. Diluted samples were analysed on a CarboPac PA10 column (4 × 250 mm) equipped with a guard column (4 × 50 mm) (Dionex, CA, United States) at a flow rate of 1 ml/min. The solutions used for the gradient are the following: 1) 1 mM NaOH and 1 M NaAcOH, 2) 1 mM NaOH. Analytes were detected by a pulsed amperometric detector (reference electrode Ag–AgCl; working electrode Au) and quantified using external standard calibration curves. Standard concentrations were linear from 0.008 to 0.0005 mg/ml for Glucose, Galactose and Mannose.
The ability of L. brevis SP48 to reduce the inflammation in AGS (human gastric adenocarcinoma) cells after infection with H. pylori, was investigated in three different experimental set ups: 1) competitive assay, in which AGS cells (105) were incubated simultaneously with L. brevis (108 CFU/ml) and H. pylori (108 CFU/ml) for 2 h; 2) inhibition assay, in which AGS cells (105 cells) were preincubated with (108 CFU/ml) for 1.5 h and then H. pylori (108 CFU/ml) was added and incubated for 2 h; 3) displacement assay in which AGS were pre-incubated with H. pylori (108 CFU/ml) for 2 h and then L. brevis (108 CFU/ml) was added and further incubated for 1.5 h (Kui et al., 2018). Semi confluent monolayers of AGS cells, cultured as previously described, were infected with H. pylori (108 CFU/ml) in the presence of postbiotics produced from L. brevis, purified as described in paragraph 2.4, at 200 μg/ml and 600 μg/ml of powder dissolved in PBS for 4 h. At the end of the experiments, in order to evaluate the expression of pro- and anti-inflammatory cytokines, the cells were washed three times with sterile PBS, and the total RNA was extracted using High Pure RNA Isolation Kit (Roche Diagnostics, Monza, and Italy). Two hundred nanograms of total cellular RNA were reverse-transcribed (Expand Reverse Transcriptase, Roche Diagnostics) into complementary DNA (cDNA) using random hexamer primers (Random hexamers, Roche Diagnostics) at 42°C for 45 min, according to the manufacturer’s instructions [19]. Real time PCR for IL-6, IL-8, TNF-α, IL-1α, TGF-β, and HBD-2 was carried out with the LC Fast Start DNA Master SYBR Green kit using 2 µl of cDNA, corresponding to 10 ng of total RNA in a 20 ml final volume, 3 mM MgCl2 and 0.5 mM sense and antisense primers (Table 1). After amplification, melting curve analysis was performed by heating to 95°C for 15 s with a temperature transition rate of 20°C/s, cooling to 60°C for 15 s with a temperature transition rate of 20°C/s, and then heating the sample at 0.1°C/s to 95°C. The results were then analyzed using LightCycler software (Roche Diagnostics). The standard curve of each primer pair was established with serial dilutions of cDNA. The relative gene expression was measured by adding a sample at known concentration of the standard curve in each test carried out. All PCR reactions were run in triplicate. The specificity of the amplification products was verified by electrophoresis on a 2% (w/v) agarose gel and visualization by ethidium bromide staining.
TABLE 1. Primer sequences and amplification programs used in the studies on AGS and Caco-2 cell cultures.
Supernatants of AGS cells infected as described above at the end of the experiment, were harvested and the presence of cytokines IL-6, IL-8, IL-1α, TNF-α, TGF-β, and HBD-2 was analyzed by enzyme-linked immunosorbent assay (ELISA; ThermoFischer Scientific Inc., Waltham, Massachusetts,United States; Phoenix Pharmaceuticals, Burlingame, United States).
The in vitro model presented was used in a previous study from our group, (Di giuda et al., 2021) and here slightly modified. Specifically, Caco-2 cells (Human Caucasian colon adenocarcinoma cells, ATCC® HTB-37™) were cultivated in Dulbecco’s modified eagle medium (DMEM) containing glucose and glutamine and supplemented with 10% (v/v) fetal bovine serum (FBS) heat inactivated (56°C for 30 min). The cells were grown in a sterile 25 cm2 flask at a concentration of 3 × 105 at confluence for 21 days to reach differentiation in normal enterocytes (Fusco et al., 2021). THP1-Blue™ NF-κB reporter cells were purchased by In Vivo Gen (Toulouse, France) and maintained in Roswell Park Memorial Institute (RPMI) 1,640 medium containing glucose (2 g/l) and glutamine (0.3 g/l), supplemented with 10% (v/v) FBS, 100 μg/ml, Normocin™, and Pen-Strep (100 U/ml). Cells were incubated at 37°C in a humidified atmosphere of air/CO2 (95:5, v/v). Total RNA was isolated by cells using TRIzol RNA Isolation Reagents (Thermo Fisher Scientific, Waltham, MA, United States) and subsequently the cDNA was reverse transcribed by Reverse Transcription System Kit (Promega, Milan, and Italy) according to the manufacturer’s instructions. Quantitative real-time polymerase chain reactions (qRT-PCR) were performed in duplicate for all genes of interest using IQ ™ SYBR® Green Supermix (Bio-Rad Laboratories, Milan, Italy) and as internal control the glyceraldehyde-3-phosphate dehydrogenase (GAPDH) housekeeping gene. The primers of the target genes are reported in Table 1. The data are expressed as a fold change (2−∆∆Ct method), (Livak and Schmittgen, 2001) in treated cells vs. untreated cells (the control) and normalized to transcript levels of housekeeping gene (Stellavato et al., 2020). To analyze the anti-inflammatory properties of L. brevis SP48 alone and of the secreted high molecular weight fraction (potential postbiotics), human enterocytes treated with LPS were used as cellular model of inflammation. Specifically, different experimental set ups were exploited in order to compare: 1) LPS treated (20 μg/ml) and untreated cells (CTR), 2) L. brevis alone (1 × 106 CFU/ml), 3) the combination LPS + L. brevis, iiii) postbiotics produced by L. brevis alone, and LPS + postbiotics at the final concentration of 200 μg/ml.
Western blotting analyses were performed after 48 h of treatment. Ripa buffer (Cell Signaling Technology) was used to lyse cells. The intracellular protein concentration was quantified using the Bradford method. In particular, 30 µg of proteins for each sample were resolved on a 10% SDS–PAGE gel and transferred onto nitrocellulose membrane (GE, Amersham, United Kingdom). Following, the membrane was blocked by 5% w/v non-fat milk in Tris-buffered saline and 0.05% v/v Tween-20 (TTBS) for 30 min and incubated overnight at 4°C with primary antibodies against TLR-4 (Abcam, Cambridge, United Kingdom) and NF-κB (Santa Cruz, Dallas, TX, United States) diluted 1:500. The membrane was then incubated with secondary antibodies, horseradish peroxidase-conjugated donkey anti-rabbit and goat anti-mouse, which was diluted 1:5,000 for 2 h at room temperature. Anti-β-tubulin antibody diluted 1:1,000 was used as the loading control. The signal was detected using the ECL system (Chemicon-Millipore, Milano, Italy), and the semi-quantitative analyses of protein expression were carried out with the ImageJ program.
Caco-2 monolayers were cultured to confluence (triplicates for each condition) for 21 days to reach full differentiation according to previously reported protocols (Fusco et al., 2021). In order to obtain a gut inflammation model, these monolayers were incubated with L. brevis EPS alone (200 μg/ml), and in the presence of commercial LPS (LPS from Salmonella minnesota S-form, Enzo Life Sciences, Farmingdale, NY, United States) at 20 μg/ml for 24 h. Successively, THP1-Blue™ (5 × 104 cells/ml) were added to all the treated monolayers according to a recently reported co-culture model (Calatayud et al., 2021). Supernatants were collected after 6 h incubation for NF-κB quantification (QUANTI-Blue reagent, InvivoGen, Toulouse, France). The assay was performed following the manufacturer’s instructions, and luminescence intensity was quantified at 655 nm using a Microplate Reader (Biorad Laboratories, Milan, Italy) to determine SEAP (secreted embryonic alkaline phosphatase) levels.
Data generated during the fermentation processes were analyzed by means one way ANOVA (JASP) with post-hoc comparisons (Tukey, Boferroni, Scheffe, Holm, and Sidak). p < 0.05 was considered as statistically significant. For cultivations of AGS cells with H. pylori and L. brevis, significant differences among groups were assessed by two-way ANOVA using GraphPad Prism 6.0, and the comparison between the means was calculated by t-student test. Data are expressed as means ± standard deviation (SD) of three independent experiments.
Batch processes were performed initially on a medium containing high concentrations of glucose (58 ± 2 g/l) and complex nitrogen (yeast extract and soy peptone) to support the production of viable L. brevis cells and postbiotics. In this condition, glucose was almost completely consumed and 6.3 ± 0.2 gcdw/l of dry biomass and 9.1 ± 0.4 × 109 CFU/ml were obtained, accompanied by about 41.0 ± 5.0 g/l of lactic acid (Table 2). A reduction of the glucose concentration, by 25 and 40%, respectively, combined with only half of the complex nitrogen sources lowered significantly the titer of dry biomass (p < 0.001) and of LA (p < 0.01), although it did not decrease the concentration of viable cells (Table 2) and of EPS, that was not significantly different in the three media. Growth of L. brevis on medium 3 with fructose as main carbon source reduced the production of viable cells and the concentration of dry biomass (p < 0.001) that was in fact 2.2 and 1.7-fold higher on the same medium containing glucose as carbon source (Table 2). Growth on fructose was accompanied by a high residue of unconsumed carbon (14.0 ± 0.2 g/l) which also led to a significantly higher Yx/s (Table 2, p < 0.001). Overall ANOVA data analysis highlighted a significative increase of the production of viable cells in the presence of glucose, whereas similar results were obtained by changing the concentration of glucose itself and of complex nitrogen sources, with an average production of 1.02 ± 0.35 × 1010 CFU/ml. Interestingly fructose produced very similar C moles of lactic and acetic acid, whereas in the presence of glucose the spectrum of by-products changed in favor of lactic acid and ethanol, increasing the YLA/X yield up to 3.5 fold. Moreover, EPS values were below the sensitivity threshold on the medium containing fructose.
TABLE 2. Batch experiments performed on a Biostat CT plus reactor with a working volume of 2.2 l at 32°C, on semidefined medium with different carbon sources and amounts of complex nitrogen sources. n.d. not detected, below assay threshold. Mean and standard deviation were calculated from three biological replicates for each condition.
The purification strategy applied in this work was performed on about 2 l of broth recovered from batch fermentations on medium 3 containing glucose. During the ultrafiltration of the supernatant the TMP stayed constant at 0.2 bar and on average the flux decreased from about 14 to 12 l/m2 h resulting in a process time of about 60 min. After ultrafiltration, carbon treatment and precipitation, the obtained powder was characterized to quantify the amount of polysaccharides, proteins and water. The same strategy was applied to the sterile medium, as control, to estimate the amount of carbohydrates present in the complex nitrogen sources that could contaminate supernatant samples. Results are reported in Table 2. The solids recovered from the purifications of the fermentation supernatant contained about 40% carbohydrates and 20% proteins. The solids recovered by applying the same purification protocol to the unspent sterile medium accounted for less than 10% of those obtained from L. brevis supernatants; this fraction was composed by 40% of carbohydrates, 1.4% proteins and 1.3% water (Table 3).
TABLE 3. Quantification of exopolysaccharides, proteins and water in the partially purified supernatant obtained from L. brevis SP48 fermentations on medium 3 with glucose as main carbon source. Data recorded from the characterization of powders obtained from two downstream processes of two different batch processes were used to calculate average values.
The polysaccharides were subjected to methanolysis which resulted in the formation of O-methyl glycosides. After the acetylation, the mixture was injected into the GC-MS. The chromatogram indicated the presence of mannose (Man), galactose (Gal), and glucose (Glc) as the main components. Furthermore, traces of xylose (Xyl) and arabinose (Ara) were found (Figure 1). The monosaccharides were identified based on their mass spectra and the retention times on the GC column as described in the Materials and Methods. The methylation analysis of EPS indicated the attachment points of the monosaccharides and revealed the occurrence of terminal glucose, terminal galactose, terminal arabinose, terminal xylose, 6-substituted glucose, 6-substituted mannose, 2,6-disubstituted mannose, and 3,6-disubstituted galactose. In agreement with the different abundance of the monosaccharides, the study of NMR spectra, revealed that the EPS is constituted by a mixture of polysaccharides (Figure 2A). Compatible with the linkage types, in the 1H,13C DEPT-HSQC spectrum (Figure 2B) signals of anomeric protons attributable to mannose units of an α-mannan were identifiable. The 13C chemical shifts were identified by comparing them with those of the unsubstituted residue (Bock and Pedersen 1983). Indeed, the anomeric signals of the residues of the mannan were found at δ 5.19/101.8 (2-Man), 5.04/103.3 (t-Man), 5.01 and 4.99/99.5 (2,6-Man), 4.93/103.4 (3-Man), 4.79/100.7 (6-Man), and 4.37/103.2 (t-Glc) (Casillo et al., 2021). In addition, signals indicating a α-(1–6)-dextran (Mclntyre and Vogel, 1991) were present (Figure 2B and Table 4). Finally, the remaining signals can be attributed to an heteropolysaccharide containing galactose, arabinose, and xylose.
FIGURE 2. (A) 1H NMR and (B) 1H, 13C DEPT-HSQC spectra of EPS from L. brevis. Letters “M” and “G” refer to mannan and glucan signals, respectively.
The solid fraction recovered after the downstream process based on UF/DF and ethanol/acetone precipitation, and containing the exopolysaccharide released in the culture medium during cultivation was also hydrolyzed using acidic conditions and analyzed by HPAE-PAD to obtain the main monosaccharides compositions. To better evaluate differences with the polysaccharides eventually present in the medium components, the medium itself was also treated and analyzed using the same protocols. In particular, the three most abundant monosaccharides revealed by the previous GC-MS/NMR experiments were quantified indicating that the broth supernatant derived sample, despite showing the same sugar components of the medium itself, presented a major abundance of galactose and especially glucose in respect to mannose (Cell-free treated supernatant: Galactose2.6: Glucose:Mannose; Medium: Galactose1.8:Glucose0.6:Mannose). These data, although indicating that the polysaccharide fraction also contains some residues of the medium components, ensures a de novo synthesis of exopolysaccharides from the strain. To further investigate this, small aliquots of biomass were extracted using previously reported protocols (Tallon et al., 2003), and the polysaccharides derived analyzed as previously described, reported a molar percentage of glucose of 84% and 12% of galactose with residual mannose.
The infection to the AGS cells with H. pylori was carried out in three different ways to better understand in which condition L. brevis best expresses its anti-inflammatory properties. The data obtained show that L. brevis added before, simultaneously or after H. pylori, significantly reduces the inflammatory state induced in AGS cells. In fact, the genes encoding proinflammatory cytokines IL-1α, IL-6, TNF-α, and IL-8 are strongly downregulated compared to infection with H. pylori alone (Figure 3A). The expression of related proteins was evaluated by ELISA assay and confirmed these data (Figure 3B). Only anti-inflammatory cytokine TGF-β is unmodulated. Therefore, L. brevis, which alone can reduce the basal expression of proinflammatory molecules, is also able to increase anti-microbial defenses by inducing the expression of HBD-2 (Figure 3C), thereby improving the conditions of the gastric mucosa damaged by H. pylori.
FIGURE 3. Real-Time PCR (A) and ELISA (B,C) show the expression levels of pro-inflammatory cytokines and HBD-2 in AGS cells infected with H. pylori and/or L. brevis SP48. Data are expressed as relative mRNAs expression (A) and protein concentration (B,C) in each group and are representative of three different PCR and ELISA experiments ±SD. RNA was extracted from 3 different AGS cell samples treated with the same batch of H. pylori and L. brevis. Significant differences are indicated by *p < 0.05, **p < 0.01, and ***p < 0.001.
The same model (Figure 4A–C) showed that postbiotics of L. brevis found in the supernatant after 16 h of growth can also significantly downregulate at both tested concentrations the expression of pro-inflammatory cytokines IL-1α, IL-6, TNF-α, and IL-8 and upregulate the expression of anti inflammatory cytokine TGF-β after infection with H. pylori. In addition about 200 μg/ml of the fraction are already sufficient, to induce the expression of HBD-2.
FIGURE 4. Real-Time PCR (A) and ELISA (B,C) show the expression levels of proinflammatory cytokines and HBD-2 in AGS cells infected with H. pylori and/or potential postbiotics of L. brevis SP48. Data are expressed as relative mRNAs expression (A) and protein concentration (B,C) in each group and are representative of three different PCR and ELISA experiments ±SD. RNA was extracted from 3 different AGS cell samples treated with the same batch of H. pylori and postbiotics. Significant differences are indicated by *p < 0.05, **p < 0.01, and ***p < 0.001.
In order to evaluate the anti-inflammatory efficacy of L. brevis alone and in combination with LPS, the transcriptional level of TLR-4 and IL-6 gene expression was analyzed in vitro on a cell based model (Figure 5A). Both TLR-4 and IL-6 were upregulated, as expected, in the presence of LPS from S. minnesota. TLR-4 expression was significantly reduced, respect to the CTR, when enterocytes were treated with the sole L. brevis, when the probiotic strain was added to LPS treated cells TLR-4 was strongly reduced (about 9 and 5 fold less). Also, the expression level of IL-6 with the sole L. brevis and after the insult with LPS, determined a significant reduction (12 and 20 fold less) versus LPS treatment (p < 0.01). In fact, both biomarkers showed a similar, or even diminished expression when compared to untreated cells. As shown in Figure 5, TLR-4 expression was significantly reduced even in respect to the CTR, when enterocytes were treated with the sole postbiotics, and the reduction was very high when compared to LPS treatment (about 75 fold less). When postbiotics were added in combination to LPS, they proved to reduce the detrimental effects of the LPS that caused upregulation of the inflammation biomarkers tested. In fact, with LPS treatment, IL-6 increased its expression of about 12 fold vs. CTR. The expression level of IL-6 with the sole postbiotics from L. brevis was similar to CTR; the combination of postbiotics and LPS, caused a marked reduction of the expressions of the 2 markers in respect to LPS insulted cells corresponding to a 1.8 and a 3 fold reduction for IL-6 and TLR-4, respectively, with respect to the LPS treatment (p < 0.01).
FIGURE 5. Gene expression analyses: results are expressed as fold change of (A) LPS, L. brevis alone and LPS + L. brevis and (B) LPS, postbiotic, and LPS + postbiotic treated cells in respect to untreated cells (CTR) for TLR-4 and IL-6 in human enterocytes. RNA was extracted from two different wells treated with the same batch of samples. Data are shown as average ±SD. Student t-test was used for the evaluation of statistical differences and significance was indicated as *p < 0.01 vs CTR, **p < 0.01 vs LPS.
A recently proposed colorimetric assay based on the detection of NF-kB activation in monocytes was also used in the framework of this study. The results further highlighted the activation of the inflammation pathway (through THP1-Blue™ cells) when enterocytes were challenged with LPS. In fact, the co-culture of differentiated Caco-2/THP1-Blue™ NF-κB reporter cells allowed to evaluate the inflammatory modulation via the NF-κB pathway comparing the response of enterocytes to the addition of L. brevis live cells and postbiotics alone, or upon their addition after the challenging of cells with LPS to induce inflammation. As reported in Figure 6 the LPS treatment upregulates NF-kB with respect to the CTR. The treatment with L. brevis and postbiotic fraction (Figure 6A) was similar to the control. The addition of either L. brevis or postbiotics to LPS treated cells on average slightly reduced the NF-kB level (Figure 6A). These data indicated an anti-inflammatory response of both L. brevis SP48 cells and secreted compounds (potential postbiotics). However, since results were not statistically significant, to identify and confirm the underlying signaling pathways, TLR-4 and NF-κB activation were evaluated by western blotting experiments in protein extracts derived from treated enterocytes (Figure 7). TLR-4 expression slightly increased with respect to the control, in LPS treated cells. However, addition of L. brevis to LPS challenged cells significantly decreased TLR-4 expression to levels similar to those of untreated cell. LPS markedly increased NF-kB expression of about 2 fold with respect to untreated cells. Enterocytes treated with LPS showed, upon addition of L. brevis, a 2.8 fold lower NF-kB level compared to the LPS treated cells (Figure 7A).
FIGURE 6. Spectrophotometric quantification of NF-κB by QUANTI-Blue™ assay in untreated cells (CTR) or cells exposed to commercial LPS from Salmonella, L. brevis, and potential postbiotics secreted from L. brevis and partially purified as described in the text. (A) Cells treated with LPS, LPS + L. brevis, and L. brevis alone (B) Cells treated with LPS, LPS + postbiotics and postbiotics alone (B). The assay was performed in duplicate, cells were treated with the same batch of postbiotics. The results are shown as average ± SD and normalized with respect to CTR (%). Student t-test was used for the evaluation of statistical differences and indicated as *p < 0.01 and #p < 0.05 vs. LPS.
FIGURE 7. TLR-4 and NF-kB protein expression analyzed by western blotting in differentiated Caco-2 cells treated with (A) Cells insulted with LPS were treated with L. brevis (B) Cells insulted with LPS were treated with postbiotics secreted by L. brevis (and partially purified as described in the text). The intensity of the bands was normalized in respect to tubulin in the densitometry reported as average and SD. Student t-test was used for the evaluation of statistical differences and indicated as *p < 0.01 vs. CTR, §p < 0.01 vs. LPS and §§p < 0.05 vs. LPS.
The same experiment was performed to evaluate the potential postbiotic fraction secreted by L. brevis SP48. Unexpectedly, addition of the sole postbiotics resulted in slightly higher NF-kB expression levels. However, addition of the postbiotics to LPS challenged cells significantly reduced the expression of NF-kB. TLR-4 expression, on the contrary, was not significantly reduced in LPS challegend cells treated with the postbiotic fraction due to high data variability (Figure 7B).
Probiotic strains have been demonstrated to provide several health benefits that are due to their specific interaction with the host and/or with eventual pathogens, or to the production of a variety of molecules with critical functions/roles. Several L. brevis strains have been recognized to modulate the immune system and affect human health (Fang et al., 2018; Prado Acosta et al., 2021). Therefore, not only the production of live cells but also the identification of potentially bioactive molecules, such as exopolysaccharides, produced from L. brevis strains during growth, is of great interest. To this end, this work focused on the identification of a fermentation medium that could support production of biomass and bioactive metabolites from L. brevis, and at the same time allow the application of a simple downstream process to obtain a potentially bioactive fraction and characterize it from a biological (and structural) point of view.
The use of De Man, Rogosa and Sharpe (MRS) medium is still quite common for the growth of Lactobacillus also in bioreactor experiments (Yue et al., 2013; Song et al., 2021). In previous research studies aeration combined with an exponential feeding profile on MRS medium resulted in the production of about 9 x109 CFU/ml of L. brevis CD2 in 42 h of growth (Alfano et al., 2020).
On the other hand, renewable waste materials were conveniently used as substrates for the development of processes targeting the recovery of products secreted by different L. brevis strains (e.g. antimicrobial molecules, mannitol, GABA etc) (Li et al., 2010; Yue et al., 2013; Xie et al., 2019; Gubelt et al., 2020).
Obtaining high cell densities during fermentation and further processing, is crucial to ensure the delivery of an adequate number of viable cells in the target area, for potential industrial purposes. Only few reports describe the optimization of L. brevis fermentations, and titers of viable cells are often not reported (Haixing et al., 2010; Mårtensson et al., 2003). In this study a semi defined medium deprived of components of animal origin allowed to obtain the highest concentration of viable cells (1010) described up to date and about 200 mg/L of exopolysaccharides in a simple 16 h batch experiment. A cheap medium with a high concentration of glucose, yeast extract and soy peptone was investigated to achieve suitable biomass yields and productivities, thereby shortening process time. However, the presence of polysaccharide contaminants in complex nitrogen sources complicates the purification of potentially bioactive compounds, such as exopolysaccharides produced by lactobacilli, and the characterization of their structure (Ferrari et al., 2022). To define a simpler medium and an industrially feasible purification strategy, the effect of lower concentrations of complex nitrogen sources (and glucose) was evaluated in the same experimental set-up demonstrating, unexpectedly, that the reduction did not affect the titer of viable cells and of EPS produced. Although higher glucose and complex nitrogen concentrations corresponded to improved dry biomass production, the Yx/s and the concentrations of viable cells did not change significantly. This might partly be due to the toxicity of lactic acid that doubled on the richer medium reaching a titer of about 40 g/l. L. brevis was previously demonstrated to possess the complete PTS system and the enzymes that carry out the first steps of glycolysis; these enzymes are fructose inducible and oxygen repressible (Saier et al., 1996; Guo et al., 2017). Growth on glucose in anaerobic conditions, confirmed previous results indicating the production of lactate and ethanol, mainly, with trace amounts of acetate (Guo et al., 2017). Higher glucose concentrations favored ethanol production to the detriment of LA (medium 2 vs. medium 3), whereas the concurrent increase of glucose and complex nitrogen sources in the medium (medium 1, Table 2) re-established a higher LA:Ethanol molar ratio. Growth on fructose in the same conditions yielded equimolar amounts of lactic acid and acetic acid, as expected, probably due to oxidation of fructose to carbon dioxide and ribulose-5P via the heterolactic fermentation, and the formation of acetate from acetyl phosphate catalyzed by acetokinase that produces additional ATP (Guo et al., 2017). Differently from other strains (Neveling et al., 2012; Alfano et al., 2020), compared to glucose, growth of L. brevis SP 48 on fructose was less efficient resulting in lower biomass and lactic acid titers, and interestingly no exopolysaccharide was detected in the fermentation broth in the assayed conditions, probably indicating an overall lower concentration of polymer produced. The use of D-fructose as carbon source for L. brevis TMW 1.2112, although enabling fastest and strongest growth compared to other sugars, resulted in the lowest slime formation (Fraunhofer et al., 2018). This strain is known to produce a β-glucan, and the conversion of D-fructose to UDP-glucose, the polysaccharide precursor, requires three metabolic steps that consume 2 ATP molecules, in contrast to consumption of 1 ATP and two metabolic steps for the conversion of Glc-1-P from glucose. The authors suggested that instead of producing high quantities of β-glucan-cell networks, D-fructose was probably rather directed to the glycolysis for generating energy (Fraunhofer et al., 2018). Therefore, for L. brevis TMW 1.2112 fructose does not induce/is not preferred for β-glucan biosynthesis; it seems that similarly here fructose does not promote EPS production. Fraunhofer and colleagues (2018) demonstrated that the type of sugar (maltose, glucose, fructose, galactose, ribose, arabinose, xylose, melibiose) used as carbon source strongly impacts the concentration of polysaccharide produced, whereas it has no effect on the composition of the EPS. Also for other LAB species a strong correlation between the concentration of EPS produced and the carbon source was previously described (Padmanabhan et al., 2018).
EPS titres here obtained on the simpler medium (0.25 g/ll) were in the range with those observed in other studies that report the production of about 0.16 g/l and 0.13 g/l from L. brevis MSR 104 (Riaz Rajoka et al., 2020) and L. brevis CD2 (Alfano et al., 2020), in batch process on MRS medium.
The compounds secreted by probiotics (e.g. polysaccharides, proteins, fatty acids, peptides, and organic acids) are biologically active molecules that often optimize physiological and immune functions of the host and provide numerous benefits. Cell-free supernatants of lactobacilli have frequently been shown to possess antimicrobial properties due to the presence of organic acids and bacteriocins (Yang et al., 2021; Moon et al., 2022). The supernatants obtained from the growth of L. brevis SP 48 were here investigated to study the effects of a polysaccharide- and protein-enriched secreted fraction, deprived of organic acids and bacteriocins removed by ultrafiltration.
The NMR and GC/MS analyses highlighted the presence of different polysaccharides, mannan prevalently coming from the medium (possibly from yeast extracts), galactan and glucan, in this last case an α-glycosidic linkage was found. Further structural features were difficult to assess due to the presence of multiple species and of neutral polymers (no amines and no uronic acid residues were found). However, from the monomers quantification it was possible to highlight that the biosynthesis of the strain studied regarded principally glucans and possibly a lower amount of galactose based polymers (or glucose/gal) ones. Overall, these data agree with the scientific literature dealing with EPS from diverse L. brevis strains.
Since yeast extract was present in the growth medium, the sterile initial fermentation medium underwent the same purification protocol to demonstrate that only a portion of the bioactive fraction may derive from medium components. The two solid powders obtained from the downstream protocol, the one recovered from the sterilized medium and the one here considered as potential postbiotic, were also hydrolysed, and analysed by HPAE-PAD to quantify the three main sugar components (glucose, mannose and galactose) found by NMR/GC-MS analysis. Data indicated a prevalence of galactose in both samples and of glucose over mannose in the cell-free supernatant. This suggests the presence of one or more polysaccharides in the samples, besides those deriving from yeast extract, with glucose and galactose as main components. Extraction of the cell-bound EPS from the biomass of L. brevis SP48 indicated the presence of a glucose rich polysaccharide confirming de novo biosynthesis of EPS from the strain.
Cell-free supernatants of L. brevis (strain MK757902) have previously been shown to exhibit anti-cancer properties (Park et al., 2015). In particular, the partially purified proteins from the spent culture supernatant of L. brevis MK05 induced apoptosis of MCF-7 cancerous cells (Pourbaferani et al., 2021). Sasaki and collaborators demonstrated that heat-killed L. brevis KB290 and crude EPS significantly enhanced cytotoxic activity of mouse splenocytes, and, that the EPS demonstrated to have a critical role in enhancing cell-mediated cytotoxic activity in mouse spleen (Sasaki et al., 2015). Since L. brevis CD2 was previously shown to reduce the intragastric load of H. pylori (Linsalata et al., 2004) the effect of L. brevis SP48 viable cells and of the potentially bioactive compounds on the inflammatory state of the intestinal epithelium during H. pylori infection was here evaluated. Competition, inhibition, and displacement assays on AGS cells treated with L. brevis/potential postbiotics alone or following H. pylori infection were performed. The experiment was carried out in 3 different ways to better understand the conditions in which L. brevis best exerts its inhibitory activity against H. pylori. First, by adding the L. brevis before H. pylori, its production of bacteriocins and other antimicrobial factors could inhibit the growth of Helicobacter by decreasing its concentration and therefore its power to adhere to the cells; the simultaneous addition of the two species, on the other hand, could lead to a competition both for the intake of nutritional factors (which would necessarily cause one species to prevail over the other) and for the binding sites to the receptors present on the surface of the epithelial cells. Finally, the addition of L. brevis after infection with H. pylori could cause a displacement of the latter from the binding sites, again owing to the production of antimicrobial factors capable of causing its killing. On the other hand, it is know that L. brevis and other lactobacillus strains are able to modulate immune responses of the host by regulating the expression of proinflammatory cytokines, soluble mediators of natural immunity (Kanmani and Kim, 2020), and of Human β-defensin-2 (HBD-2), inducible antimicrobial peptide active against Gram-positive and Gram-negative bacteria, fungi, and the envelope of some viruses, and involved in the innate immune response (Kanmani and Kim, 2020; Donnarumma et al., 2016). Our results showed that L. brevis alone and the secreted compounds at both concentrations increased the production of HBD-2 by gastric epithelial cells indicating the stimulation/activation of antimicrobial defenses and of innate immunity. Concurrently both live cells and postbiotics by themselves strongly downregulated the expression of proinflammatory cytokines caused by H. pylori infection, demonstrating an anti-inflammatory effect. These data demonstrated that the high molecular weight potentially postbiotic fraction (mainly containing exopolysaccharide and proteins) secreted by L. brevis is by itself sufficient to contrast H. pylori, thereby overcoming issues related to the preservation of probiotic viability.
The fact that substantial differences in the results obtained in the 3 different tests are not appreciated, could mean that at the basis of the inhibition of H. pylori mediated by L. brevis, different mechanisms are involved simultaneously, including those previously hypothesized, but it is also interesting to underline how this behavior is species-specific, in fact, as observed in our previous work (D’Ambrosio et al., 2022), using viable cells of L. fermentum the inhibition of H. pylori proved efficient only in the displacement assay.
L. brevis SP48 and its secreted high molecular weight fraction was also tested in vitro in a gut model. Recent literature reports that extracellular polysaccharides modulate intestinal immune responses by regulating Toll-like receptors (e.g TLR-2 and 4) signaling pathways, which are responsible for the induction of cytokines and chemokines in response (Wachi et al., 2014).
In vitro cell models play an important role in understanding cellular mechanisms related to the function of specific molecules. Lactic acid bacteria showed anti-inflammatory properties activating a non-specific response of the immune system (El-Deeb et al., 2018). They are also known to play a significant role in activating NF-kB, which regulates the production of pro-inflammatory cytokines in LPS-stimulated cells (Lee et al., 2008; El-Deeb et al., 2018).
In the present study cells challenged with LPS from S. minnesota showed upregulation of all the inflammatory biomarkers tested. The treatment with L. brevis SP48 reduced the mRNA expression of TLR-4 and IL-6 like pro-inflammatory targets, as well as the protein expressions of TLR-4 and NF-kB. These data suggested that L. brevis SP48 exerts a direct action on the human enterocytes stimulating the immune response and inactivating the NF-κB inflammatory pathway. Interestingly, also the bioactive compounds isolated from L. brevis supernatants acted as modulators of the inflammatory pathway by reducing the expression of inflammatory mediators in the presence of LPS. In fact, upon LPS addition the NF-κB pathway was activated through TLR-4 binding and prompted cytokines production as already described in the recent literature (Nyati et al., 2017; Di Guida et al., 2021). The potential postbiotics isolated in this work reduced pro-inflammatory cytokines (e.g. IL-6), by decreasing expression/activation of NF-kB and TLR-4, thereby indicating that they can modulate the enterocytes’immune response (Di Guida et al., 2021). The THP-1 blue NF-κB reporter assay indicated an inhibitory effect of L. brevis SP48 secreted compounds on inflammation in response to LPS binding. Western blotting experiments confirmed the downregulation of NF-kB upon treatment of LPS challenged cells with the postbiotic fraction. Also other exopolysaccharides reduced the secretion of cytokines and downregulated the NF-κB pathway (El Deeb et al., 2018; Chen et al., 2019; Liu et al., 2017) demonstrating appealing anti-inflammatory properties, for the potential treatment of the intestinal bowel disease (e.g. IBD) without the need of live cells.
The optimization of fermentation processes and the development of economically viable probiotic production processes is necessary to reduce costs of commercial products. On the other hand, several probiotic strains secrete a plethora of biologically active molecules that by themselves exert beneficial effects and can be useful in the medical and food fields. Results presented in this study demonstrate the production of high titers of viable L. brevis SP48 cells on a cheap medium deprived of animal derived raw materials, and at the same time the obtainment of a biologically active cell-free fraction. Live cells and secreted compounds reduced inflammation of gastric epithelial cells following H. pylori infections. The high molecular weight compounds present in the fermentation supernatant also modulated the expression of inflammatory mediators in an in vitro gut model. These effects seem to be due, at least in part, to EPS and/or secreted proteins other than low molecular weight compounds (e.g. organic acids) that were removed during the ultrafiltration and precipitation processes. These preliminary results indicate that further investigations on L. brevis SP48 and on its secreted high molecular weight fraction (potential postbiotic) as potential tools to counteract stomach pathogens and inflammation also in intestinal diseases, are of great interest.
The original contributions presented in the study are included in the article/Supplementary Material, further inquiries can be directed to the corresponding authors.
Conceptualization, CS, DC, and GD; Fermentation experiments, SD and DC; Downstream processing, SD; Biological assays, AF, AS; Viability determination, SD, AD; NMR and GC-MS, MC, AC. Writing—Original Draft Preparation, DC, and other authors’ specific contributions; Funding Acquisition, CS. AMG, provided the strain.
This research was funded by the project Incube-MISE Sportello, number F/200035/01/X45.
We kindly thank Luca Falco for the help provided during EPS quantification assays.
AMG is employed by the company R&D—IBSA Farmaceutici Italia.
The remaining authors declare that the research was conducted in the absence of any commercial or financial relationships that could be construed as a potential conflict of interest.
All claims expressed in this article are solely those of the authors and do not necessarily represent those of their affiliated organizations, or those of the publisher, the editors and the reviewers. Any product that may be evaluated in this article, or claim that may be made by its manufacturer, is not guaranteed or endorsed by the publisher.
Abdelazez, A., Abdelmotaal, H., Evivie, S. E., Melak, S., Jia, F. F., Khoso, M. H., et al. (2018). Screening potential probiotic characteristics of Lactobacillus brevis strains in vitro and intervention effect on type I diabetes in vivo. Biomed. Res. Int. 2018, 1–20. doi:10.1155/2018/7356173
Aguilar-Toalá, J. E., García-Varela, R., García, H. S., Mata-Haro, V., González-Córdova, A. F., Vallejo‐Cordoba, B., et al. (2018). Postbiotics: An evolving term within the functional foods field. Trends Food Sci. Technol. 75, 105–114. doi:10.1016/j.tifs.2018.03.009
Alfano, A., Perillo, F., Fusco, A., Savio, V., Corsaro, M. M., Donnarumma, G., et al. (2020). Lactobacillus brevis CD2: Fermentation strategies and extracellular metabolites characterization. Probiotics Antimicrob. Proteins 12, 1542–1554. doi:10.1007/s12602-020-09651-w
Bock, K., and Pedersen, C. J. (1983). Carbon-13 nuclear magnetic resonance spectroscopy of monosaccharides. Adv. Carbohydr. Chem. Biochem. 41, 27–66.
Bradford, M. M. (1976). A rapid and sensitive method for the quantitation of microgram quantities of protein utilizing the principle of protein-dye binding. Anal. Biochem. 72, 248–254. doi:10.1016/0003-2697(76)90527-3
Brooijmans, R., Smit, B., Santos, F., van Riel, J., de Vos, W. M., and Hugenholtz, J. (2009). Heme and menaquinone induced electron transport in lactic acid bacteria. Microb. Cell Fact. 8, 28–38. doi:10.1186/1475-2859-8-28
Butorac, K., Novak, J., Bellich, B., Terán, L. C., Banić, M., Leboš Pavunc, A., et al. (2021). Lyophilized alginate-based microspheres containing Lactobacillus fermentum D12, an exopolysaccharides producer, contribute to the strain's functionality in vitro. Microb. Cell Fact. 20 (1), 85. doi:10.1186/s12934-021-01575-6
Calatayud, M., Verstrepen, L., Ghyselinck, J., Van den Abbeele, P., Marzorati, M., Modica, S., et al. (2021). Chitin glucan shifts luminal and mucosal microbial communities, improve epithelial barrier and modulates cytokine production in vitro. Nutrients 13, 3249–3264. doi:10.3390/nu13093249
Casillo, A., Fabozzi, A., Fabozzi, I., Parrilli, E., Biggs, C. I., Gibson, M. I., et al. (2021). Physicochemical approach to understanding the structure, conformation, and activity of mannan polysaccharides. Biomacromolecules 22, 1445–1457.
Chen, Y. C., Wu, Y. J., and Hu, C. Y. (2019). Monosaccharide composition influence and immunomodulatory effects of probiotic exopolysaccharides. Int. J. Biol. Macromol. 133, 575–582. doi:10.1016/j.ijbiomac.2019.04.109
Ciucanu, I., and Kerek, F. (1984). A simple and rapid method for the permethylation of carbohydrates. Carbohydr. Res. 131, 209–217. doi:10.1016/0008-6215(84)85242-8
D’ambrosio, S., Ventrone, M., Alfano, A., Schiraldi, C., and Cimini, D. (2021). Microbioreactor (micro-Matrix) potential in aerobic and anaerobic conditions with different strains. Biotechnol. Progr. 37, e3184. doi:10.1002/btpr.3184
D’Ambrosio, S., Ventrone, M., Fusco, A., Casillo, A., Dabous, A., Cammarota, M., et al. (2022). Limolactobacillus fermentum from buffalomilk is suitable for potential biotechnological process development and inhibits Helicobacter pylori in a gastric epithelial cell model. Biotechnol. Rep. 34, e00732. doi:10.1016/j.btre.2022.e00732
Di Cerbo, A., Palmieri, B., Aponte, M., Morales-Medina, J. C., and Iannitti, T. (2016). Mechanisms and therapeutic effectiveness of lactobacilli. J. Clin. Pathol. 69 (3), 187–203. doi:10.1136/jclinpath-2015-202976
Di Guida, R., Casillo, A., Stellavato, A., Di Meo, C., Kawai, S., Kawamoto, J., et al. (2021). Complete lipooligosaccharide structure from Pseudoalteromonas nigrifaciens sq02-rifr and study of its immunomodulatory activity. Mar. Drugs 19, 646–660. doi:10.3390/md19110646
Donnarumma, G., Paoletti, I., Fusco, A., Perfetto, B., Buommino, E., de Gregorio, V., et al. (2016). β-Defensins: Work in progress. Adv. Exp. Med. Biol. 901, 59–76. doi:10.1007/5584_2015_5016
Doron, S., and Snydman, D. R. (2015). Risk and safety of probiotics. Clin. Infect. Dis. 60, 129–134. doi:10.1093/cid/civ085
Dubois, M., Gilles, K. A., Hamilton, J. K., Rebers, P. A., and Smith, F. (1956). Colorimetric method for determination of sugars related substances. Anal. Chem. 3, 350–356. doi:10.1021/ac60111a017
El-Deeb, N. M., Yassin, A. M., Al-Madboly, L. A., and El-Hawiet, A. (2018). A novel purified Lactobacillus acidophilus 20079 exopolysaccharide, LA-EPS-20079, molecularly regulates both apoptotic and NF-κB inflammatory pathways in human colon cancer. Microb. Cell Fact. 17 (1), 29–44. doi:10.1186/s12934-018-0877-z
Fang, F., Xu, J., Li, Q., Xia, X., and Du, G. (2018). Characterization of a Lactobacillus brevis strain with potential oral probiotic properties. BMC Microbiol. 18, 221–230. doi:10.1186/s12866-018-1369-3
Ferrari, M., Hameleers, L., Stuart, M. C. A., Oerlemans, M. M. P., de Vos, P., Jurak, E., et al. (2022). Efficient isolation of membrane-associated exopolysaccharides of four commercial bifidobacterial strains. Carbohydr. Polym. 278, 118913–118924. doi:10.1016/j.carbpol.2021.118913
Fraunhofer, M. E., Geissler, A. J., Wefers, D., Bunzel, M., Jakob, F., and Vogel, R. F. (2018). Characterization of β-glucan formation by Lactobacillus brevis TMW 1.2112 isolated from slimy spoiled beer. Int. J. Biol. Macromol. 107, 874–881. doi:10.1016/j.ijbiomac.2017.09.063
Fresno, S., Jiménez, N., Canals, R., Merino, S., Corsaro, M. M., Lanzetta, R., et al. (2007). A second galacturonic acid transferase is required for core lipopolysaccharide biosynthesis and complete capsule association with the cell surface in Klebsiella pneumoniae. J. Bacteriol. 189 (3), 1128–1137. doi:10.1128/JB.01489-06
Fukao, M., Zendo, T., Inoue, T., Nakayama, J., Suzuki, S., Fukaya, T., et al. (2019). Plasmid-encoded glycosyltransferase operon is responsible for exopolysaccharide production, cell aggregation, and bile resistance in a probiotic strain, Lactobacillus brevis KB290. J. Biosci. Bioeng. (19), 391–397. doi:10.1016/j.jbiosc.2019.04.008
Fusco, A., Savio, V., Donniacuo, M., Perfetto, B., and Donnarumma, G. (2021). Antimicrobial peptides human beta-defensin-2 and -3 protect the gut during Candida albicans infections enhancing the intestinal barrier integrity: In vitro study. Front. Cell. Infect. Microbiol. 11, 666900–666910. doi:10.3389/fcimb.2021.666900
Giannini, G., Ragusa, I., Nardone, G. N., Soldi, S., Elli, M., Valenti, P., et al. (2022). Probiotics-containing mucoadhesive gel for targeting the dysbiosis associated with periodontal diseases. Int. J. Dent. 2022, 1–16. doi:10.1155/2022/5007930
Gubelt, A., Blaschke, L., Hahn, T., Rupp, S., Hirth, T., and Zibek, S. (2020). Comparison of different lactobacilli regarding substrate utilization and their tolerance towards lignocellulose degradation products. Curr. Microbiol. 77, 3136–3146. doi:10.1007/s00284-020-02131-y
Guo, T., Zhang, L., Xin, Y., Xu, Z., He, H., and Kong, J. (2017). Oxygen-inducible conversion of lactate to acetate in heterofermentative Lactobacillus brevis ATCC 367. Appl. Environ. Microbiol. 83 (21), 016599–e1717. doi:10.1128/AEM.01659-17
Haixing, L., Ting, Q., Guidong, H., and Yu-sheng, C. (2010). Production of gamma-aminobutyric acid by Lactobacillus brevis NCL912 using fed-batch fermentation. Micro. Cell Fact. 9, 85–91.
Kanmani, P., and Kim, H. (2020). Beneficial effect of immunobiotic strains on attenuation of Salmonella induced inflammatory response in human intestinal epithelial cells. PLoS One. 15, e0229647. doi:10.1371/journal.pone.0229647
Kishi, A., Uno, K., Matsubara, Y., Okuda, C., and Kishida, T. (1996). Effect of the oral administration of Lactobacillus brevis subsp. coagulans on interferon-alpha producing capacity in humans. J. Am. Coll. Nutr. 15, 408–412. doi:10.1080/07315724.1996.10718617
Kui, Z., Qiong, X., Di, X., Yilin, G., Xueying, T., Hua, W., et al. (2018). Antagonistics of Lactobacillus plantarum ZDY2013 against Helicobacter pylori SS1 and its infection in vitro in human gastric epithelial AGS cells. J. Biosci. Bioeng. 126 (4), 458–463. doi:10.1016/j.jbiosc.2018.04.003
Li, H., Qiu, T., Huang, G., and Cao, Y. (2010). Production of gamma-aminobutyric acid by Lactobacillus brevis NCL912 using fed-batch fermentation. Microb. Cell Fact. 9, 85–91. doi:10.1186/1475-2859-9-85
Linsalata, M., Russo, F., Berloco, P., Caruso, M. L., Matteo, G. D., Cifone, M. G., et al. (2004). The influence of Lactobacillus brevis on ornithine decarboxylase activity and polyamine profiles in Helicobacter pylori-infected gastric mucosa. Helicobacter 9, 165–172. doi:10.1111/j.1083-4389.2004.00214.x
Liu, J., Wang, X., Pu, H., Liu, S., Kan, J., and Jin, C. (2017). Recent advances in endophytic exopolysaccharides: Production, structural characterization, physiological role and biological activity. Carbohydr. Polym. 157, 1113–1124. doi:10.1016/j.carbpol.2016.10.084
Livak, K. J., and Schmittgen, T. D. (2001). Analysis of relative gene expression data using real-time quantitative PCR and the 2−ΔΔCT method. Methods 25 (4), 402–408. doi:10.1006/meth.2001.1262
Lyu, C., Zhao, W., Peng, C., Hu, S., Fang, H., Hua, Y., et al. (2018). Exploring the contributions of two glutamate decarboxylase isozymes in Lactobacillus brevis to acid resistance and γ-aminobutyric acid production. Microb. Cell Fact. 17, 180–193. doi:10.1186/s12934-018-1029-1
Mårtensson, O., Dueñas, M., Irastorza, A. R. O., and Holst, O. (2003). Comparison of growth characteristics and exopolysaccharide formation of two lactic acid bacteria strains, Pediococcus damnosus 2.6 and Lactobacillus brevis G-77, in an oat-based, nondairy medium. LWT - Food Sci. Technol. 36, 353–357. doi:10.1016/S0023-6438(03)00020-3
Mclntyre, D. D., and Vogel, H. J. (1991). Nuclear magnetic resonance studies of homopolysaccharides related to starch. Starch/Starke. 43, 69–76. doi:10.1002/star.19910430209
Moon, E. C., Park, M. S., Lim, T., Kim, R. H., Ji, G. E., Kim, S. Y., et al. (2022). Antibacterial effect of cell-free supernatant fraction from Lactobacillus paracasei CH88 against Gardnerella vaginalis. Sci. Rep. 12, 4763–4772. doi:10.1038/s41598-022-08808-7
Neveling, D. P., Endo, A., and Dicks, L. M. (2012). Fructophilic Lactobacillus kunkeei and Lactobacillus brevis isolated from fresh flowers, bees and bee-hives. Curr. Microbiol. 65 (5), 507–515. doi:10.1007/s00284-012-0186-4
Nyati, K. K., Masuda, K., Zaman, M. M., Dubey, P. K., Millrine, D., Chalise, J. P., et al. (2017). TLR4-induced NF-κB and MAPK signaling regulate the IL-6 mRNA stabilizing protein Arid5a. Nucleic Acids Res. 45, 2687–2703. doi:10.1093/nar/gkx064
Padmanabhan, A., Tong, Y., Wu, Q., Zhang, J., and Shah, N. P. (2018). Transcriptomic insights into the growth phase-and sugar-associated changes in the exopolysaccharide production mechanism of a high EPS-producing Streptococcus thermophilus ASCC 1275. Front. Microbiol. 9, 1919–1936. doi:10.3389/fmicb.2018.01919
Park, K. B., Oh, C. H., and Oh, S. H. (2015). Anti-proliferative and apoptosis inducing activity of Lactobacillus brevis OPK-3 isolated from kimchi on leukemia cell lines. Curr. Top. Lact. Acid. Bact. Probiotics 3 (1), 1–7. doi:10.35732/ctlabp.2015.3.1.1
Pourbaferani, M., Modiri, S., Norouzy, A., Maleki, H., Heidari, M., Alidoust, L., et al. (2021). A newly characterized potentially probiotic strain, Lactobacillus brevis MK05, and the toxicity effects of its secretory proteins against MCF-7 breast cancer cells. Probiotics Antimicrob. Proteins 13 (4), 982–992. doi:10.1007/s12602-021-09766-8
Prado Acosta, M., Goyette-Desjardins, G., Scheffel, J., Dudeck, A., Ruland, J., and Lepenies, B. (2021). S-layer from Lactobacillus brevis modulates antigen-presenting cell functions via the mincle-syk-card9 Axis. Front. Immunol. 12, 602067. doi:10.3389/fimmu.2021.602067
Riaz Rajoka, M. S., Mehwish, H. M., Zhang, H., Ashraf, M., Fang, H., Zeng, X., et al. (2020). Antibacterial and antioxidant activity of exopolysaccharide mediated silver nanoparticle synthesized by Lactobacillus brevis isolated from Chinese koumiss. Colloids Surfaces B Biointerfaces 186, 110734–110745. doi:10.1016/j.colsurfb.2019.110734
Saier, M. H., Ye, J. J., Klinke, S., and Nino, E. (1996). Identification of an anaerobically induced phosphoenolpyruvate-dependent fructose-specific phosphotransferase system and evidence for the Embden-Meyerhof glycolytic pathway in the heterofermentative bacterium Lactobacillus brevis. J. Bacteriol. 178 (1), 314–316. doi:10.1128/jb.178.1.314-316.1996
Sasaki, E., Suzuki, Y., Fukui, Y., and Yajima, N. (2015). Cell-bound exopolysaccharides of Lactobacillus brevis KB290 enhance cytotoxic activity of mouse splenocytes. J. Appl. Microbiol. 118 (2), 506–514. doi:10.1111/jam.12686
Sharma, A., Tilak, T., Bakhshi, S., Raina, V., Kumar, L., Chaudhary, S. P., et al. (2017). Lactobacillus brevis CD2 lozenges prevent oral mucositis in patients undergoing high dose chemotherapy followed by haematopoietic stem cell transplantation. ESMO Open 1 (6), e000138. doi:10.1136/esmoopen-2016-000138
Song, J., Peng, S., Yang, J., Zhou, F., and Suo, H. (2021). Isolation and identification of novel antibacterial peptides produced by Lactobacillus fermentum SHY10 in Chinese pickles. Food Chem. x. 348, 129097–97. doi:10.1016/j.foodchem.2021.129097
Stellavato, A., Abate, L., Vassallo, V., Donniacuo, M., Rinaldi, B., and Schiraldi, C. (2020). An in vitro study to assess the effect of hyaluronan based gels on muscle-derived cells: Highlighting a new perspective in regenerative medicine. PLoS ONE 15, e0236164. doi:10.1371/journal.pone.0236164
Suzuki, S., Yakabe, T., Suganuma, H., Fukao, M., Saito, T., and Yajima, N. (2013). Cell-bound exopolysaccharides of Lactobacillus brevis KB290: Protective role and monosaccharide composition. Can. J. Microbiol. 59, 549–555. doi:10.1139/cjm-2013-0115
Tallon, R., Bressollier, P., and Urdaci, M. C. (2003). Isolation and characterization of two exopolysaccharides produced by Lactobacillus plantarum EP56. Res. Microbiol. 154 (10), 705–712. doi:10.1016/j.resmic.2003.09.006
Taverniti, V., and Guglielmetti, S. (2011). The immunomodulatory properties of probiotic microorganisms beyond their viability (ghost probiotics: Proposal of paraprobiotic concept). Genes Nutr. 6 (3), 261–274. doi:10.1007/s12263-011-0218-x
Teame, T., Wang, A., Xie, M., Zhang, Z., Yang, Y., Ding, Q., et al. (2020). Paraprobiotics and postbiotics of probiotic lactobacilli, their positive effects on the host and action mechanisms: A review. Front. Nutr. 7, 570344–570359. doi:10.3389/fnut.2020.570344
Wachi, S., Kanmani, P., Tomosada, Y., Kobayashi, H., Yuri, T., Egusa, S., et al. (2014). Lactobacillus delbrueckii TUA4408L and its extracellular polysaccharides attenuate enterotoxigenic Escherichia coli-induced inflammatory response in porcine intestinal epitheliocytes via Toll-like receptor-2 and 4. Mol. Nutr. Food Res. 58 (10), 2080–2093. doi:10.1002/mnfr.201400218
Wang, Q., Liu, X., Fu, J., Wang, S., Chen, Y., Chang, K., et al. (2018). Substrate sustained release-based high efficacy biosynthesis of GABA by Lactobacillus brevis NCL912. Microb. Cell Fact. 17 (1), 80–87. doi:10.1186/s12934-018-0919-6
Wei, Y., Li, F., Li, L., Huang, L., and Li, Q. (2019). Genetic and biochemical characterization of an exopolysaccharide with in vitro antitumoral activity produced by Lactobacillus fermentum YL-11. Front. Microbiol. 10, 2898–2908. doi:10.3389/fmicb.2019.02898
Wu, Q., and Shah, N. P. (2017). High γ-aminobutyric acid production from lactic acid bacteria: Emphasis on Lactobacillus brevis as a functional dairy starter. Crit. Rev. Food Sci. Nutr. 57, 3661–3672. doi:10.1080/10408398.2016.1147418
Wu, Q., and Shap, N. P. (2018). Restoration of GABA production machinery in Lactobacillus brevis by accessible carbohydrates, anaerobiosis and early acidification. Food Microbiol. 69, 151–158. doi:10.1016/j.fm.2017.08.006
Xie, C., Coda, R., Chamlagain, B., Varmanen, P., Piironen, V., and Katina, K. (2019). Co-Fermentation of Propionibacterium freudenreichii and Lactobacillus brevis in wheat bran for in situ production of vitamin B12. Front. Microbiol. 10, 1541–1550. doi:10.3389/fmicb.2019.01541
Yang, K. M., Kim, J. S., Kim, H. S., Kim, Y. Y., Oh, J. K., Jung, H. W., et al. (2021). Lactobacillus reuteri AN417 cell-free culture supernatant as a novel antibacterial agent targeting oral pathogenic bacteria. Sci. Rep. 11, 1631–1646. doi:10.1038/s41598-020-80921-x
Yue, M., Cao, H., Zhang, J., Li, S., Meng, Y., Chen, W., et al. (2013). Improvement of mannitol production by Lactobacillus brevis mutant 3-A5 based on dual-stage pH control and fed-batch fermentations. World J. Microbiol. Biotechnol. 29 (10), 1923–1930. doi:10.1007/s11274-013-1357-6
Živković, M., Miljković, M. S., Ruas-Madiedo, P., Markelić, M. B., Veljović, K., Tolinački, M., et al. (2016). EPS-SJ exopolisaccharide produced by the strain Lactobacillus paracasei subsp. paracasei BGSJ2-8 is involved in adhesion to epithelial intestinal cells and decrease on E. coli association to caco-2 cells. Front. Microbiol. 7, 286–299. doi:10.3389/fmicb.2016.00286
Keywords: Levilactobacillus brevis, probiotic, postbiotic, exopolysaccharide, Helicobacter pylori, gut model
Citation: Cimini D, D’ambrosio S, Stellavato A, Fusco A, Corsaro MM, Dabous A, Casillo A, Donnarumma G, Giori AM and Schiraldi C (2022) Optimization of growth of Levilactobacillus brevis SP 48 and in vitro evaluation of the effect of viable cells and high molecular weight potential postbiotics on Helicobacter pylori. Front. Bioeng. Biotechnol. 10:1007004. doi: 10.3389/fbioe.2022.1007004
Received: 29 July 2022; Accepted: 14 October 2022;
Published: 31 October 2022.
Edited by:
Haifeng Zhao, South China University of Technology, ChinaReviewed by:
Jasna Novak (maiden Beganovic), University of Zagreb, CroatiaCopyright © 2022 Cimini, D’ambrosio, Stellavato, Fusco, Corsaro, Dabous, Casillo, Donnarumma, Giori and Schiraldi. This is an open-access article distributed under the terms of the Creative Commons Attribution License (CC BY). The use, distribution or reproduction in other forums is permitted, provided the original author(s) and the copyright owner(s) are credited and that the original publication in this journal is cited, in accordance with accepted academic practice. No use, distribution or reproduction is permitted which does not comply with these terms.
*Correspondence: Donatella Cimini, ZG9uYXRlbGxhLmNpbWluaUB1bmljYW1wYW5pYS5pdA==; Chiara Schiraldi, Y2hpYXJhLnNjaGlyYWxkaUB1bmljYW1wYW5pYS5pdA==
Disclaimer: All claims expressed in this article are solely those of the authors and do not necessarily represent those of their affiliated organizations, or those of the publisher, the editors and the reviewers. Any product that may be evaluated in this article or claim that may be made by its manufacturer is not guaranteed or endorsed by the publisher.
Research integrity at Frontiers
Learn more about the work of our research integrity team to safeguard the quality of each article we publish.