- 1School of Dental Medicine, University of Connecticut Health Center, Farmington, CT, United States
- 2School of Medicine, Connecticut Convergence Institute for Translation in Regenerative Engineering, University of Connecticut Health Center, Farmington, CT, United States
- 3Department of Medicine, Division of Endocrinology, School of Medicine, University of Connecticut Health Center, Farmington, CT, United States
- 4Department of Biomedical Engineering, School of Engineering, University of Connecticut, Storrs, CT, United States
- 5School of Engineering, Institute of Materials Science (IMS), University of Connecticut, Storrs, CT, United States
The comprehensive reconstruction of extensive craniofacial and dentoalveolar defects remains a major clinical challenge to this day, especially in complex medical cases involving cancer, cranioplasty, and traumatic injury. Currently, osteogenic small molecule-based compounds have been explored extensively to repair and regenerate bone tissue because of their unique advantages. Over the past few years, a number of small molecules with the potential of craniofacial and periodontal bone tissue regeneration have been reported in literature. In this review, we discuss current progress using small molecules to regulate cranial and periodontal bone regeneration. Future directions of craniofacial bone regenerative engineering using the small molecule-based compounds will be discussed as well.
Background
The multi-faceted complex of craniofacial anatomy and dentoalveolar tissues (referring to the teeth and neighboring bone) contrives a plethora of intricate functions that are undeniably integral to the maintenance of systems throughout the body. The craniofacial bones composing the upper skull are known to provide immediate protection to the brain and other sections of the central nervous system while also shielding numerous sensory fibers responsible for major perceptive capabilities like olfaction, vision, auditory processing, and gustation. Craniofacial and dentoalveolar bones of the lower skull provide a rigid and fortuitous framework for soft tissues regularly used in the mastication and swallowing of food, production of speech, respiration, and formation of facial expressions (Anderson et al., 2021). Defects or lesions of these regions are often characterized by extensive trauma, malformation, or pathology affecting bony structures comprising the skull; as such, they are known to exist as especially debilitating hindrances that ascribe lasting functional, esthetic, and social barriers to patients who unwillingly incur a diminished quality of life because of them (Manlove et al., 2020). Examples of blatant and insidious impediments commonly braced by these patients include restricted communication, dysphagia, entropion, and ectropion, hindered temporomandibular joint mobility, reduced interpersonal relations, pronounced sentiments of ostracism, etc. Ubiquitous across many global populations, craniofacial and dentoalveolar anomalies may be observed in child and adult demographics alike—some such anomalies include congenital and idiopathic forms of orofacial clefting which affects 1 in every 700 live births, Treacher-Collins syndrome that leads to underdevelopment of facial bones and tissues responsible for proper maturation of the zygomatic bone and jawbones, Apert syndrome that yields an underdeveloped maxilla and misalignment of the teeth on account of premature suture closure, among several other oppressive disorders and acute traumatic injuries that result in a distinct loss of bone structure. In many cases, natural processes of bone healing are sufficient in restoring smaller lesions. Specifically, incumbent mesenchymal stem cells can undergo differentiation into regulatory osteoclasts and osteoblasts imperative for bone remodeling, complimented by increased activity of numerous cytokine-releasing lymphoid cells and growth factors that promote bone formation. While these types of subtle, confined, or localized defects are typically amenable through intrinsic pathways of biological bone repair, particularly large craniofacial and dentoalveolar defects often exhibit insufficiency of innate regeneration as the extent of involvement exceeds the body’s natural capabilities of osteogenic repair. With this in mind, translational applications of tissue rehabilitation have been established in the form of a limited number of clinical interventions that have provided defect patients with more viable solutions for meeting their needs. Despite this, many patients burdened with conditions of large craniofacial and dentoalveolar defects involving lesion-prone structures like the calvaria, mandible, temporomandibular joint, palate, cranial sensory organs, cranial muscles, periodontium, or dentition of the skull are still confronted with enduring challenges regarding available treatment options and their associated drawbacks.
A few prominent modalities of treatment currently being used to address large craniofacial and dentoalveolar defects include reconstructive procedures based in the use of techniques like prosthetic obturation which invokes upon the integrative and ostensibly seamless attributes of compatible biomaterials in the reformation of lesions, as well as in the resolution of many functional impairments displayed by patients (e.g., 3D-printing technologies for fabrication of tissue-specific prostheses). However, methods of prosthetic obturation remain an imperfect solution with their own constraints. For example, patients with material-based prosthetic obturators must often have their prostheses removed for regular maintenance and cleaning. Such prostheses also do not change color in response to UV exposure or aging as would be apparent in adjacent tissues of the patient’s skin. Conversely, reconstructive practices embedded in principles of tissue obturation and grafting (e.g., tissue transfer, allogeneic grafting, and autogenetic grafting) are more aptly used in the correction of sizeable anatomical deformities without requisites for regular maintenance. They also appeal to concerns of functional esthetics by avoiding jarring discrepancies in coloring that juxtapose the appearance of surrounding tissues. In fact, the current gold standard of treatment for segmental craniofacial and dentoalveolar defects utilizes methods of tissue obturation, particularly autologous bone grafting techniques, oriented around the replacement of endogenous tissues and the preservation of functional capacity. It’s estimated that over two million bone grafting procedures are carried out annually across the globe, approximately 500,000 of those procedures occurring within the United Stated. Touting high success rates of site infusion, along with a list of profound biomechanical, osteoconductive, osteoinductive, and osteogenetic properties, autologous bone grafts have proven to be advantageous in more ways than one for effective reconstruction. The benefits afforded by autologous grafts have made them a favorable mode of treatment for both surgeons and patients, especially when considering the adaptive curtailments of more synthetic bone substitutes. Though still, even these grafting methods are not without their own caveats. Auspicious as this technique may seem, autologous bone grafts are continuously associated with various restrictions that bring a degree of ambiguity to their unadulterated incorporation into a patient’s treatment plan; for instance, these methods may suffer from a possible lack of adequate bone structure for graft sampling, increased donor site morbidity related to bone sample procurement, patient pain or discomfort, and even progressive bone resorption in some cases (Schmidt et al., 2020). Minor tangential complications such as impaired wound healing, temporary sensory loss, prolonged wound drainage, and superficial infection have also been reported post-op by patients treated with autologous bone grafts. The osteodinductive growth factor, bone morphogenetic protein-2 (BMP-2), as another source of treatment has been applied clinically as a substitute for bone grafts in procedures amending sumptuous bone lesions. In fact, evidence from previous clinical trials has shown that BMP-2 use decreases total operatory time and is even more effective in treating certain lesions than autologous grafting materials (James et al., 2016). The likelihood of secondary, post-op interventions also sees a considerable drop with a conversion from grafting to BMP-2. However, the clinical profile of BMP-2 is not devoid of its own blemishes. Potentially life-threatening complications associated with BMP-2 are commonly observed, effecting as high as 20%–70% of cases; side effects include ectopic bone formation, osteolysis and subsidence, unregulated inflammation, bone cyst formation, adipogenesis, etc., which have all been reported in patients treated with BMP-2. There also remains the concern of high structural integrity requirements for adjacent tissues and the implementation costs tied to clinical BMP-2 use.
In order to nullify some of the indelible pitfalls coupled with techniques of modern obturation and BMP-2, novel approaches centered around the use of regenerative engineering are being evaluated as less constrained alternatives, or supplements, to treatment. Fervent research into potent stem cell populations, biomimetic scaffolding, and more comprehensive interventions for large-scale bone regeneration, has helped us realize new innovative means for optimizing the care of patients presenting with such substantial and complex defects (Fan et al., 2017a; Shi et al., 2019; Zhang et al., 2020; Gregory et al., 2022; Shah et al., 2022; Zhang et al., 2022; Zhu et al., 2022). Namely, within the recent decade, advancements in the clinical aptitude of osteogenic small molecules and the delivery systems used to facilitate molecular targeting of specialized tissues have substantially bolstered prospects for new therapeutic treatments (Lo et al., 2012; Carbone et al., 2014; Laurencin et al., 2014; Lo et al., 2014; O’Neill et al., 2019; Lo, 2022).
Small molecules are defined as organic compounds of low molecular weight, expressly ≤1,000 Da, which include certain metabolites, drugs, monosaccharides, secondary messengers, and xenobiotics. Small molecules have largely seen use as favorable starting points in the discovery and development of new therapeutics for many chronic and acute conditions. Additionally, they have been utilized by some researchers as resourceful probes into the intricacies of less understood biological pathways. The regenerative properties demonstrated by some small molecules have shown promise in retaining the functional and physical traits of natural tissues observed at regions of craniofacial and dentoalveolar trauma or congenital defect while concomitantly ameliorating the risk of secondary infections, chronic pain, and other adverse effects that muddle the current index of treatments (Birjandi et al., 2020; Zhang et al., 2020; Shang et al., 2021). With the intent of enhancing osteoblast proliferation and effectively regulating osteoclast activity, a growing number of studies assessing the use of small molecules on large defects of the mandibular, maxillofacial, periodontal, and dental tissues have succeeded in prompting the osteogenic repair through various models. As a form of intervention, small molecules have also been shown to supplement angiogenesis and the differentiation of mesenchymal stem cells into osteoprogenitor cells (Segar et al., 2013; Ottensmeyer et al., 2018). At this time, there are many small molecule drugs that have already been awarded FDA approval following subjection to numerous trials for safety and efficacy assurance (De la Torre and Albericio, 2020). Notably, a vast majority of these small molecular agents also incur relatively low preparation costs, are easier to control, and can be conveniently synthesized in a lab or sourced from natural origins like plants and fruits (Awale et al., 2017). Through extensive in vitro and in vivo surveying of multiple biofactors and miniscule healing agents, the utility of small molecules in the regeneration of craniofacial and dentoalveolar tissues has become abundantly apparent and helped spur newfound hope for a better standard of care (Rodríguez-Méndez et al., 2018; Aghali, 2021; Emara and Shah, 2021) (Figures 1, 2). However, it must be noted that even though bioactive small molecules may potentially offer clinicians and their patients an array of propitious advantages, there still arises the question of proper dosing and delivery systems—specifically in regard to short-term and long-term drug administration (Song and Leeuwenburgh, 2014; Sarigol-Calamak and Hascicek, 2018; Liu F et al., 2021; Lo, 2022; Murugan et al., 2022). While these limitations require their own attention, the gradual expansion of small molecule therapeutics has still acted to further elucidate a multitude of burgeoning avenues through which clinically applicable tissue regeneration may be achieved in the near future for significant craniofacial and dentoalveolar defects (Ding et al., 2017; Mozaffari et al., 2019; Terauchi et al., 2021; Yi et al., 2020). Here, we discuss some of the recent strides made in using small molecules as a mode of rehabilitation for craniofacial and dentoalveolar tissues.
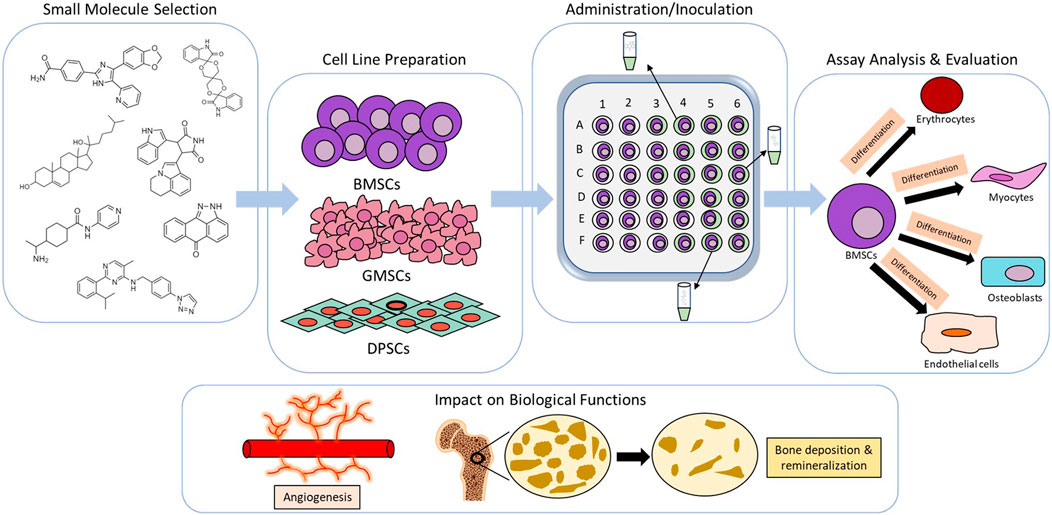
FIGURE 1. Example methodology of in vitro analysis for craniofacial and dentoalveolar tissue engineering. Meticulous selection of small molecules with favorable osteogenic properties and stem cell lineages from craniofacial or dentoalveolar tissues. Media imbued with small molecules are typically inoulated with stem cells and later assessed for expression of osteogenic markers and cellular differentiation. Cumulative change in biological functions (e.g.,angiogenesis and bone mineralization) are then evaluated.
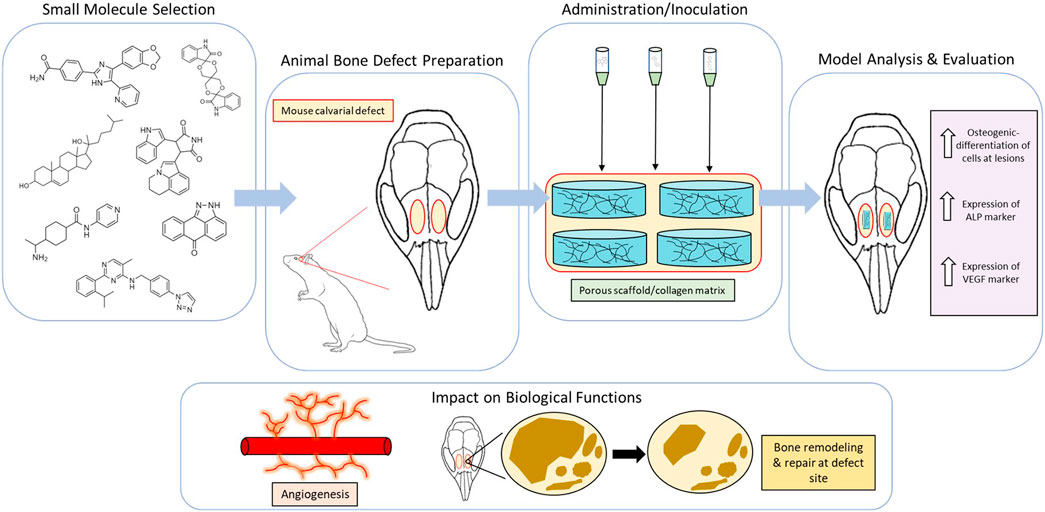
FIGURE 2. Example methodology of in vivo analysis for craniofacial and dentoalveolar tissue engineering. Meticulous selection of small molecules with favorable osteogenic properties and animal models involving defects of the craniofacial or dentoalveolar tissues. Scaffolds incorporated with small molecules are typically implanted at defect sites and later measured for expression of osteogenic markers. Cumulative change in biological functions (e.g.,angiogenesis and bone remodeling) are then evaluated.
In vitro evidence of small molecules on craniofacial and dentoalveolar bone regeneration
Techniques for small molecule screening such as cell panel screening are widely used for investigating in vitro biological mechanism and drug discovery (Figure 1). A number of groups have investigated the role of various glycogen synthase kinase-3 (GSK3)-inhibiting small molecules in the regeneration and differentiation of odontogenic stem cells, primarily endogenous to tissues of the dental pulp and dentin (Neves et al., 2017; Neves & Sharpe, 2018; Birjandi et al., 2020; Masuda et al., 2020). GSK3 has been identified as a key enzyme in the modulation of many signaling pathways responsible for the growth and maturation of bone, including those common to the formation of craniofacial and dentoalveolar tissues e.g., the Wnt/β-catenin pathway which has been demarcated as a pertinent cascade in stem cell differentiation and the regulation of dentin repair. One such study has shown that five unique small molecule drugs known to work through different mechanisms of action (Famotidine, Olanzapine, Naproxen, Cromolyn, and Tivantinib) are capable of acting as inhibitory agents against GSK3 (Birjandi et al., 2020). Of these drugs, Tivantinib—a non-ATP competitive small molecule mainly used in the treatment of hepatocellular carcinoma—was observed to incite Axin2 expression and Wnt/β-catenin pathway signaling at a low concentration (50 nM) while simultaneously maintaining dental pulp cell viability through inconsequential levels of toxicity, thus potentially making it a suitable candidate for in vivo studies on the natural regeneration of odontoblasts necessary for reversing profound dentinal lesions and enhancing dentin preservation. In Masuda et al., in vitro cell culture treatments using the small molecule Tideglusib, another GSK3 antagonist, were seen to yield odontoblast-like cells with alkaline phosphatase (ALP)-positive calcified nodules in rat dental pulp cells after periods of intermittent and continuous exposure (Masuda et al., 2020). At a concentration of 50 nM, Tiderglusib significantly increased the number of calcified nodules observed among cultured pulp cells of the intermittent 6-h experimental group when compared with the control group. Though interestingly, a decline in the number of calcified nodules was reported among pulp cells of the continuous 48-h experimental group in comparison to the 6-h experimental and control groups. Additionally, the 6-h experimental group exhibited greater expression of the odontogenic biomarkers dentin sialophosphoprotein (Dspp) and osteocalcin (OCN) mRNA, while also promoting β-catenin aggregation and less β-catenin phosphorylation (i.e., minimal suppressor activity) within the cytoplasm of cultured pulp cells. The 48-h experimental group displayed less Dspp and OCN mRNA expression, along with more β-catenin phosphorylation. Purmorphamine, another osteoinductive small molecule with some conflicting findings across studies, has been shown to incite expression of osteoblastic phenotype markers in neural crest-derived dental pulp stem cells (DPSCs) (Rezia Rad et al., 2016). Elevated levels of DNA were examined among stem cells cultured on β-TCP granules with 2 µM Purmorphamine compared to cells cultured without 2 µM Purmorphamine, suggesting it plays a promotional role in the proliferation of DPSCs. In addition to promoting adhesion, proliferation, and differentiation of DPSCs cultured on β-TCP granules, Purmorphamine has been seen to increase the prominence of ALP, osteopontin (OPN), and OCN at both 7 and 14 days of induction. The small molecule ML323, a USP1 inhibitor that prompts ubiquitination and degradation of the anti-osteogenic gene ID1, was also found to encourage osteogenesis of DPSCs during osteodifferentiation. ML323 increased ALP activity on day 14 of DPSC osteodifferentiation, complimented by calcium accumulation on day 21 (Kim and Choung, 2020). Similarly, the GSK3 inhibiting small molecule 6-Bromoindirubin-3′-Oxime (BIO) initiated osteodifferentiation of DPSCs in another study as evidenced by elevated ALP activity, mineral deposition, and osteogenic gene expression. BIO was seen to increase β-catenin translocation which upregulated the Wnt signaling pathway in the DPSCs. BIO also increased expression of osteogenic genes ANKH, ENPP1, RUNX2, OSX, OCN, DSPP, DMP1, and OPG paired with downregulation of osteoclastogenic RANKL (Kornsuthisopon et al., 2022).
It should also be noted that the stimulated differentiation of neural crest-derived DPSCs retains a unique advantage through the shared origin of DPSCs and other neural crest-derived craniofacial tissues, therein emboldening their candidacy in the development of regenerative therapies not limited solely to dental repair. The neurogenic aptitude of a specially tailored mosaic of small molecules was verified in Heng et al. (2019) through analyses on small molecule-induced differentiation of DPSCs, stem cells from apical papilla (SCAPs), and gingival mesenchymal stem cells (GMSCs) into neural cell lineages at 8 and 14 day checkpoints. A noteworthy increase in the presence of mature neural markers NeuN, NFM, NSE, and MAP2 was observed in DPSCs and SCAPs, while other immature neural markers Musashi1, βIII-Tubulin, NGN2, and MASH1 were seen in GMSCs. The small molecule cocktail used in this study (consisting of Valproic acid, Forskolin, SP600125, GO6983, Y-27632, Repsox, CHIR99021, Dorsomorphin) also showed ample apoptosis of initial stem cells consistent with the natural death of immature neural cells that precedes neuronal differentiation. Therapeutic applications hinging on the use of such neurogenic molecules suggest a feasible approach for the regeneration of nervous tissues incumbent to craniofacial and dentoalveolar bone. In another study by Shang et al. (2021), the prolyl hydroxylases (PHDs) inhibiting small molecule, Dimethyloxalylglycine (DMOG), paired with bioactive nanosilicate (nSi) in a polylactic acid/polyglycolic acid (PLGA) scaffold was also seen to be beneficial in the regeneration of periodontal tissues and remodeling of periodontal bone (Liu Z. Q et al., 2021). From the in vitro studies on this combination, an increase in ALP activity and other osteogenic markers like Runx2, OPN, OCN, and bone sialo-protein (BSP) were observed in the differentiation of periodontal ligament stem cells (PDLSCs) using DMOG/nSi scaffolds. With the expression of these markers, they noted an apparent formation of functional cementum-periodontal ligament alveolar bone complexes that further supported the occurrence of adequate differentiation. To complement these findings, sustained release of DMOG and profound degrees of angiogenic revascularization essential to periodontal healing were also apparent from these trials. Another integral approach to craniofacial bone regeneration and tooth repair explores the mechanisms of collagen biomineralization used in the preservation of innate functional and mechanical properties seen in many of the largely collagenous tissues of the cranium. Two other small molecules that have been shown to foster intrafibrillar mineralization include citrate and fluoride. The small molecule citrate, abundant across many craniofacial and dentoalveolar tissues, has been tokened as a purported osteopromotive factor that orchestrates the process of citrate metabolism for facilitating downstream effects on the differentiation of mesenchymal stem cells (MSCs) into osteoblasts and other osteogenic factors. In Ma et al., in vitro studies found that 200 µM citrate increased osteophenotype progression to amplify the expression of several osteogenic factors such as ALP, OPN, Runx2, Col1a1, and SPP1 while also producing higher calcium/protein ratios (Ma et al., 2018). Other studies have also shown that citrate can prompt intrafibrillar mineralization by diminishing interfacial energy observed between collagen matrices and ACP precursors. Similarly, the inductive effects of fluoride in the synthesis and extrafibrillar deposition of collagen fibrils are seen to enhance extents of crystallization and rod-like mineralization (Saxena et al., 2018). Fluoride has already been evidenced to promote the remineralization of hard dental tissues (namely enamel and dentin with large compositions of inorganic material) through fluorapatite formation. Though it should be mentioned, that while fluoride does display components of collagen mineralization, it has largely been cited in dental cavity prophylaxis and dental repair only due to its lower solubility threshold and heightened hardness in relation to hydroxyapatite. Drug delivery techniques in craniofacial and dentoalveolar engineering have also been tested using small molecules. For instance, small molecule-incorporated scaffolds have been assessed for their use in the controlled release of agents intended to accelerate the proliferation and osteodifferentiation of stem cells. In Song et al. (2021), a small molecule TGF-β inhibitor, SB431542, was consorted with an injectable calcium phosphate cement (CPC) scaffold for in vitro analyses. These scaffolds were then evaluated for their utility in promoting the proliferation, differentiation, and viability of human-induced pluripotent stem cells (hiPSCs). Of the scaffolds tested in this study, the CPC scaffolds with integrated 100 µM SB431542 showed the highest prevalence of viable hiPSC-MSCs as well as the greatest effects on osteogenic differentiation in comparison to the CPC control scaffolds without SB431542. All scaffolds, including those embedded with the small molecule, displayed continuous release over the 14-day trial period as required of a satisfactory delivery system. In the absence of scaffolding, SB431542 was also observed to encourage osteogenic differentiation in mesoderm-derived parietal bone osteoblasts (PObs) and dura mater (DM) cells through TGF-β inhibition. Heightened BMP signaling paired with increased OCN and Runx2 expression at days 12 and day 18 in SB431542-treated cell cultures, were demonstrated by PObs and DM cells of the experimental group during differentiation and time course assays. A reduction in activity of the crucial apoptotic protease Caspase 3 was also observed. As a point of interest, it was noted that SB431542 unexpectedly upregulated Smad6 in both of the surveyed cell lines instead of downregulating its expression—refuting the prospect of reduced Smad6 being the principal mechanism behind increased BMP signaling seen during SM431542-mediated inhibition of TGF-β (Senarath-Yapa et al., 2016). In Zhang et al. (2020), potential methods of advanced craniofacial reconstruction and regeneration using multi-material 3D printing techniques were explored. They developed polycaprolactone/β-tricalcium phosphate (PCL/TCP) and hydrogel-based bioink composite scaffolds embedded with two osteogenic small molecules, resveratrol (RSV) and strontium ranelate (SrRn), seen to promote bone formation and reduce bone resorption. Through in vitro analysis, it was demonstrated that RSV and SrRn scaffolds were effective in increasing ALP activity through BMP-2, inciting osteoblastic differentiation, upregulating Wnt/β-catenin signaling, spurring promotion of mineralization in mouse MSCs, among a slew of other osteogenic properties. Scaffolded combination of RSV and SrRn was also shown to exhibit a synergistic coalition in promoting angiogenesis while concurrently inhibiting degenerative osteoclast activity. In characterizing the release of these two small molecules, an initial burst of release followed by more sustained release for SrRn but consistent sustained release for RSV over the 3-week release period. Primary outcomes for in vitro trials are outlined in Table 1.
In vivo evidence of osteogenic small molecules on craniofacial and dentoalveolar bone regeneration
In pursuit of more pragmatic modes of treatment and therapy for patients suffering from large craniofacial and dentoalveolar bone lesions, it is commonplace that inquiries into the assimilative aptitude of these small molecular agents be brought into question. Currently, a number of studies have proven that many of the osteogenic attributes provided by small molecular agents are steadfast in animal models seen to effectively mimic the microenvironment and stromal interactions seen in settings of significant bone loss and defect (Figure 2). One such study has assessed the osteogenic properties of SB431542 in minipigs with craniofacial bone lesions. Shi et al. (2019) postulates that SB431542 downregulates canonical TGF-β signaling by competitively binding to TGF-β type I receptors in order to prevent Smad3 gene phosphorylation and induce activation of BMP signaling. They also found that this small TGF-β inhibitor acts as a catalyst in the differentiation of iPSCs to human gingival mesenchymal stem cells (hGMSCs) while also substantially amplifying BMP-2 and BMP-4 expression in immature osteoblasts for enhanced regeneration of large maxillofacial defects. In fact, in vivo and in vitro trials utilizing 1 µM SB431542 were observed to elicit a number of osteogenic effects; reduced hGMSC apoptosis rates, extensive osteogenic differentiation of hGMSCs, increased subcutaneous osteogenesis of hGMSCs in nude mice, and elevated bone regeneration of autologous GMSCs in the presence of defects. In other trials with SB431542, an increased rate of bone healing was established in mice with parietal bone defects that were a part of the experimental group as opposed to those of the control group. This was confirmed using immunohistochemistry analysis of the dural membrane extending these calvarial defects which showed a greater reduction in pSmad2/3 staining for the SB431542-treated group (Senarath-Yapa et al., 2016). In a study using rats with alveolar cleft defects branching from the zygomatic arch to the ipsilateral maxillary incisor, collagen sponges treated with a hydroxycholesterol mixture comprised of the small molecules 20(S)-hydroxycholesterol and 22(R)-hydroxycholesterol was the most effective in promoting osteogenic bone formation among all other treatment and control groups. Specifically, the hydroxycholesterol mixture exhibited the highest trabecular number and thickness in morphometric analysis while also retaining the lowest trabecular separation. The histological presence of pseudostratified columnar epithelium on the side of the nasal cavity in hydroxycholesterol-treated defect models indicated the lack of any adverse inflammatory reactions spurred by this mixture of small molecules (Bakshi et al., 2019). The small molecule phenamil, an amiloride derivative, is shown to prompt osteogenic differentiation of mesenchymal progenitors to encourage bone regeneration in animal models with large craniofacial defects. It is believed that phenamil accomplishes this through tribbles homolog 3 (Trib3)-incited inhibition of Smad ubiquitin regulatory factor 1 (Smurf1) that negatively regulates BMP-receptor related Smads. In Fan et al., trials administered on large rat mandibular defects using 300 µM phenamil were seen to yield almost complete formation of trabeculae and bone volume at lesion sites, even when paired with low doses of BMP-2 (Fan et al., 2017b). Fascinatingly, doses of BMP-2 seen to produce hollow cysts containing fatty marrow and peroxisome proliferator activated receptor gamma (PPARγ)—both being markers of BMP-induced adipogenesis and inflammation—were not observed to produce such cysts when introduced with phenamil. Thus, phenamil was not only seen to promote BMP-induced osteogenesis but also circumvent some of the adverse manifestations of BMP-2 based therapies (i.e., adipogenesis and inflammation). Also, when delivered alongside noggin siRNA in sterosomes containing stearylamine and cholesterol, phenamil benefits from a synergistic increase in MSC osteogenesis and bone formation (Cui et al., 2017). In the aforementioned Zhang et al. (2020) study employing RSV-SrRn scaffolds, in vivo implantation trials on rat models with critical-sized mandibular defects showed increased bone formation following RSV-SrRn scaffold introduction. From baseline, comparatively higher bone volume to total volume (BV/TV) ratios were observed in scaffolds integrated with the RSV-SrRn combination as opposed to those control scaffolds without small molecule incorporation—therein supporting the in vivo efficacy of this small molecule combination. Also noted from their study, peripheral and central coverage of bone regeneration in large mandibular defects was evident using the RSV-SrRn dual small molecule scaffold. Dexamethasone (DEX), a small osteogenic hydroxysteroid, has also been shown to promote bone healing in rats with skull defects when delivered using chitosan-modified mesoporous silica nanoparticles (chi-MSNs). At 4 weeks, rat models treated with DEX@chi-MSNs demonstrated a significant increase in BV/TV ratios which was not mimicked in those models treated with the chi-MSN control vacant of DEX. After 8 weeks, elevated bone formation was observed in all groups, including the control; however, those incorporating DEX were still seen to entice the most growth (Sun et al., 2019) (Figure 3). In a similar manner, in vivo studies conducted on the ROCK (Rho-associated coiled-coil kinase) inhibitors HA-1077 (Fasudil) and Y-27632 showed that these small molecular agents were capable of accelerating the induction of bone healing in rats with parietal bone defects. Using atelocollagen sponges that were injected with PBS, 0.5 µM HA-1077, or 0.5 µM Y-27632 and implanted at defect sites, it was determined that HA-1077 and Y-27632 both promoted a high degree of osteoregeneration over the span of 4 weeks. The expression of VEGF-A mRNA, an important marker of the osteogenic protein VEGF that is imperative for proper bone defect repair and formation, was detected in osteoblast progenitor cells derived from injured rat bone marrow treated with 5 µM Y-27632 (Nakata et al., 2020).
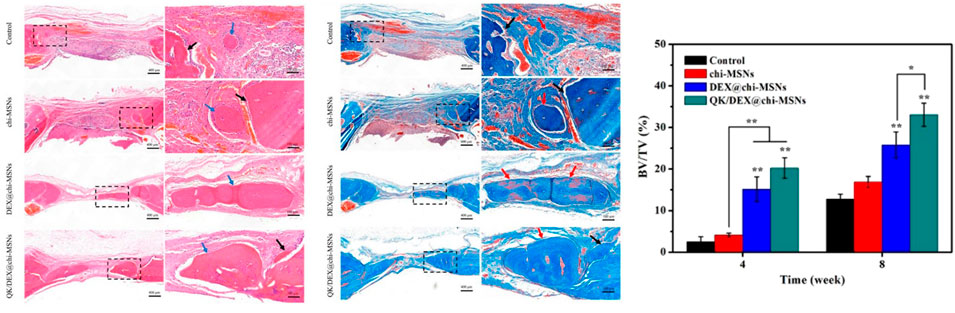
FIGURE 3. Comparative H&E and Masson’s trichrome stains characterizing new bone formation in rat cranial bone defects treated with small molecule dexamethasone (DEX)-incorporating gelfoams (DEX/@chi-MSNs; QK/DEX@chi-MSNs) in addition to DEX-devoid gelfoams (Control; Chi-MSNs), 8 weeks after placement within defect. Blue arrow represent new bone, while black arrows represent incumbent host bone. Using CT-Analyser Software, elevated bone volume/total volume ratios were measured in treatment groups incorporating DEX. Reproduced from (Sun et al., 2019)
Furthermore, viable applications in the regeneration of large orofacial defects, like those of the alveolar bone, have also been explored using the small molecule drug simvastatin (SV) which is generally indicated in the treatment of cholesterol-mediated cardiovascular diseases. Along with its cholesterol reducing properties, simvastatin has been established as a prominent osteogenic agent capable of inducing BMP-2 gene expression to potentiate bone regeneration while also displaying some anti-resorption elements to impede osteoclast proliferation. In Wu et al. (2008), PLGA scaffolds incorporated with SV were administered to sites of residual alveolar ridge resorption following tooth extraction in rat models. Over a release period of 12 weeks, SV/PLGA scaffolds were seen to be much more successful at stimulating bone formation than non-treated control PLGA scaffolds. Particularly, SV/PLGA scaffolds exhibited higher residual alveolar ridge heights and bone mineral densities at all reference points for release. These findings have provided in vivo evidence of simvastatin’s distinct osteogenic propensities that may work to accelerate healing and bone deposition in cases of large orofacial bone loss. DMOG/nSi scaffolds were also seen to promote revascularization and bone regeneration in rats with periodontal and mandibular defects through stimulation of PDLSC osteogenic differentiation and modulation of deleterious inflammatory responses during tissue repair (Liu F. et al., 2021; Shang et al., 2021). In studies conducted by Neves et al. (2017), Tideglusib has also been explored in the incitement of tertiary dentin formation in deeply lesioned in vivo mouse molars due to the drug’s promotion of Wnt/β-catenin pathways in odontoblastic pulp cells (e.g., DSPCs) for elevated deposition of dentin matrix (Neves & Sharpe, 2018). Preliminary trials showed that Tideglusib, along with the small molecule GSK3 antagonists CHIR99021 and BIO, elicited increased formation of reparative dentin in injured molars with pulp exposure at both 4 and 6 weeks. Reconstruction of dentin tissues using these molecules was also paired with notable preservation of pulp vitality, an important distinction to be made in considering probable clinic applications (Neves & Sharpe, 2018). In a later study, impressive extents of reactionary dentin remineralization emulating thickness levels seen in healthy non-damaged molars, were observed at carious lesion sites bereft of pulp exposure in mouse molars treated with Tideglusib, compared to the minimal mineralization recorded in control molars treated with collagen sponges devoid of the GSK3 antagonist (Neves et al., 2017). Evidence from these studies support the intrinsic ability of small-molecule drugs like Tideglusib to infiltrate regions of damaged dentin and act on the naturally restorative capacities of odontoblasts and pulp cells for advanced healing in large dental lesions exhibiting dentin and/or pulp exposure. The Wang laboratory showed that another small molecule GSK3-inhibitor, CHIR99021, prompted upregulation of Wnt/β-catenin signaling to induce vasculogenic differentiation of DSPCs in immunodeficient mice, as evidenced by a marked increase in the expression of endothelial components VEGFR2, VE-Cadherin, CD31, and Tie-2 (Zhang et al., 2016). Mesenchymal DPSCs were employed as fundamental precursor cells in the de novo generation of primordial endothelium imperative for initial proliferation of new vascular networks. This type of vasculogenesis using DPSCs, or even bone marrow mesenchymal stem cells (BMSCs) (Xu et al., 2008), has elucidated a plausible method for the secondary neovascularization of dental tissues following events of expansive trauma or defect. In this same study, it was seen that use of the small molecule inhibitor JW67, specifically in the repression of Wnt/β-catenin signaling, enhanced odontoblast and osteoblast differentiation of DPSCs. These findings raised interest around the implications of Wnt/β-catenin acting as an amenable pathway for the osteogenic and/or vasculogenic differentiation of mesenchymal stem cells, deriving potential therapeutic benefit as a mechanism for facilitating craniofacial or dentoalveolar regeneration. In regard to the misconfiguration and underdevelopment of the palatal bones seen in cleft palate, some small molecular Wnt agonists have been indicated in the disruption of congenital clefting during embryonic formation of the palate. Examples of such small molecules include the DKK1 inhibitors WAY-262611 and IIIc3a which effectively preserved the cleft phenotype in Pax9−/−Dkk1f/+; Wnt1Cre mouse embryos by encouraging complete and partial fusion between the primary and secondary palates. Controlled intravenous delivery of these two Wnt agonist small molecules into pregnant Pax9+/− mice amends a majority of cleft palate defects in mutant offspring. Treatment with WAY-262611 from embryonic days 10.5–13.5 rescued the cleft phenotype, though to a lesser extent than observed during regimented dosing from embryonic days 10.5–14.5. Of those mutant progenies studied, ∼60% (11/18) displayed complete fusion between the primary and secondary palates while the other 7/18 showed small residual defects at the zone of fusion for the primary and secondary palates near the 3rd palatal rugae. Administration of IIIc3ac to pregnant Pax9+/− mice from embryonic days 10.5–14.5 showed successful closure of the secondary palate defects in 80% (12/15) of mutant embryos, of which six embryos retained residual fusion defects. Although the corrective properties of both DKK1 inhibtors were highlighted in this study, it was also observed that Wnt agonist therapies using WAY-262611 did not prevent defects in tooth organs and other structures affected in modified mouse embryos (Jia et al., 2017). Primary outcomes for in vivo trials are outlined in Table 2.
Concluding remarks and future directions
Craniofacial and dentoalveolar defects are major bone diseases affecting millions of individuals worldwide, yet the efficacy and safety of current treatment methods are limited and present significant complications that prevent their unmarred integration into patient care. Small molecule mediated-bone regeneration has been proposed as a promising strategy due to the inherent characteristics of small molecules that can minimize or even bypass the limitations observed in both protein-based therapeutics and conventional means of obturation such as high manufacturing cost, protein instability and degradation, risk of contamination, and unwanted immune response (Lu and Atala, 2014). We provide a review for the prospects of small molecules mediated craniofacial and dentoalveolar bone regenerative engineering. We also review the preclinical study of small molecules associated with craniofacial and dentoalveolar bone regeneration. In fact, over the past decade, a number of small molecules with bone regenerative potential have been reported in the literature, these small molecule compounds include known pharmaceutical bioactive compounds and unknown novel small molecule compounds (Figure 4). Although many of these efforts have focused on identifying small molecules that augment BMP-2 mediated osteoblast function (Fan et al., 2015; Fan et al., 2017a; Moon et al., 2017; Qi et al., 2017) there has been less emphasis on identifying small molecules alone that are able to stimulate primitive and undifferentiated cells to develop into the osteogenic cell lineage with the capacity to form new bone. Therefore, it is important to identify small molecules with potent inherent osteoinductivity without the need of exogenous BMP recombinant protein supplementation.
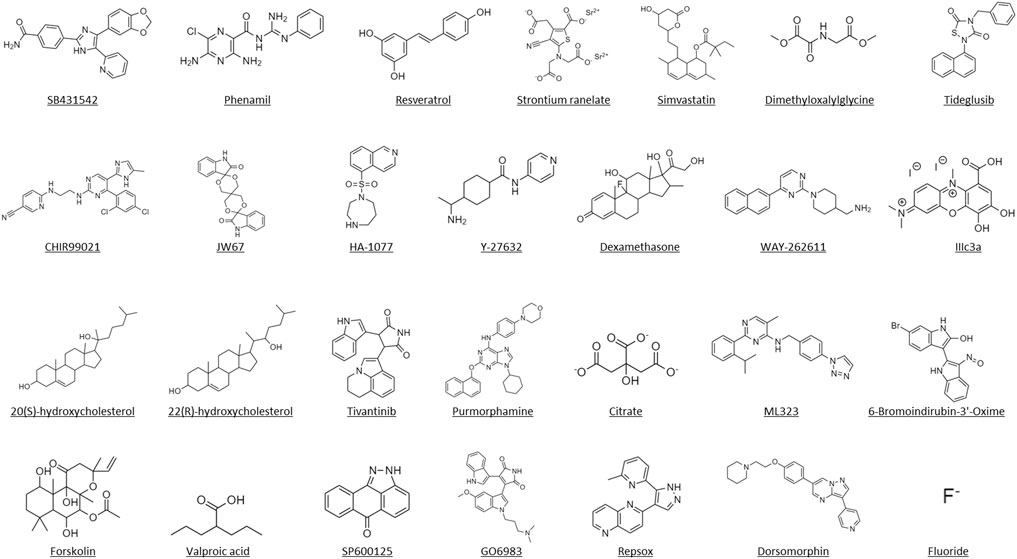
FIGURE 4. Molecular structures of the identified small molecules that have been investigated for in vitro and in vivo craniofacial and dentoalveolar tissue engineering.
Drug repositioning is the application of approved small molecule drugs for new indications (Jourdan et al., 2020). An advantage of drug repositioning over traditional drug development is that since the repositioned drugs have already passed a significant number of toxicity and other tests, their safety is known and the risk of failure for reasons of adverse toxicology are significantly reduced (O’Neill et al., 2019). Herein, a new drug repositioning method should be performed that is able to identify approved small molecule-based drug(s) with potent osteoinductivity inherent without the need of exogenous BMP recombinant protein supplementation. In addition, artificial intelligence (AI) tools are starting to enable pharmaceutical researchers to search chemistry from expansive small molecule databases for developing drugs at a much faster rate than ever before (Sharma and Sharma, 2018).
It is also worth mentioning that stem cells hold great promise for regenerative therapies for a wide spectrum of diseases or injuries by virtue of their ability to regenerate tissues and contribute to their homeostasis (Bacakova et al., 2018). Interestingly, deciduous teeth contain a rich supply of stem cells in their dental pulp. These cells called stem cells from human exfoliated deciduous teeth (SHEDs) are highly proliferative, clonogenic and capable of differentiating into a variety of cell types including osteoblasts and odontoblasts (Miura et al., 2003; Yang et al., 2019). It is believed that the great potential of SHEDs for repairing and regenerating bone tissue would certainly bring basic science concept closer to clinical studies. In addition, it is possible to investigate the potential of osteogenic small molecule in conjunction with SHEDs for engineering bone tissue for dental as well as orthopaedic applications. An effective delivery approach for small molecules is another objective of small molecule-based bone regenerative engineering. Given that small molecule compounds can be formulated into numerous forms of biomaterials (Laurencin et al., 2014), thus it is vital to develop an engineered scaffold delivery system that provides adequate doses of the small molecule and/or acts as a structural support for infiltrating cells (Lo, 2022).
Author contributions
JM and KL wrote the mini-review manuscript. Both contributed to revisions of the manuscript. JM prepared all tables and figures.
Funding
The work was supported by funding from the NSF/EFRI fund (#1332329) and the seed fund from the Institute at UConn Health.
Conflict of interest
The authors declare that the research was conducted in the absence of any commercial or financial relationships that could be construed as a potential conflict of interest.
Publisher’s note
All claims expressed in this article are solely those of the authors and do not necessarily represent those of their affiliated organizations, or those of the publisher, the editors and the reviewers. Any product that may be evaluated in this article, or claim that may be made by its manufacturer, is not guaranteed or endorsed by the publisher.
Abbreviations
GSK3, Glycogen synthase kinase-3; Dspp, Dentin sialophosphoprotein; OCN, Osteocalcin; DPSC, Dental pulp stem cell; OPN, Osteopontin; SCAP, Stem cells from apical papilla; PHD, Prolyl hydroxylases; GMSC, Gingival mesenchymal stem cells; DMOG, Dimethyloxalylglycine; nSi, Nanosilicate; BSP, Bone sialo-protein; PLGA, Polylactic acid/polyglycolic acid; RSV, Resveratrol; PDLSC, Periodontal ligament stem cells; SrRn, Strontium ranelate; MSC, Mesenchymal stem cell; Trib3, Tribbles homolog 3; CPC, Calcium phosphate cement; SV, Simvastatin; BMP-2, Bone morphogenetic protein-2; PCL/TCP, Polycaprolactone/β-tricalcium phosphate; hiPSCs, Human-induced pluripotent stem cells; BMSC, Bone marrow mesenchymal stem cells; Smurf1, Smad ubiquitin regulatory factor 1; PPARγ, Peroxisome proliferator activated receptor gamma; SHED, Stem cells from human exfoliated deciduous teeth; BIO, 6-Bromoindirubin-3′-Oxime.
References
Aghali, A. (2021). Craniofacial bone tissue engineering: Current approaches and potential therapy. Cells (Basel, Switz. 10 (11), 2993. doi:10.3390/cells10112993
Anderson, B. W., Kortz, M. W., and Al Kharazi, K. A. (2021). “Anatomy, head and neck, skull,” in StatPearls [Internet] (Treasure Island (FL): StatPearls Publishing).
Awale, G., Wong, E., Rajpura, K., and W-H Lo, K. (2017). Engineered bone tissue with naturally-derived small molecules. Curr. Pharm. Des. 23 (24), 3585–3594. doi:10.2174/1381612823666170516145800
Bacakova, L., Zarubova, J., Travnickova, M., Musilkova, J., Pajorova, J., Slepicka, P., et al. (2018). Stem cells: Their source, potency and use in regenerative therapies with focus on adipose-derived stem cells - a review. Biotechnol. Adv. 36 (4), 1111–1126. doi:10.1016/j.biotechadv.2018.03.011
Bakshi, R., Hokugo, A., Zhou, S., Zhang, Z., Wang, L., Rezzadeh, K., et al. (2019). Application of hydroxycholesterols for alveolar cleft osteoplasty in a rodent model. Plastic Reconstr. Surg. 143 (5), 1385–1395. doi:10.1097/prs.0000000000005528
Birjandi, A. A., Suzano, F. R., and Sharpe, P. T. (2020). Drug repurposing in dentistry; towards application of small molecules in dentin repair. Int. J. Mol. Sci. 21 (17), 6394. doi:10.3390/ijms21176394
Carbone, E. J., Jiang, T., Nelson, C., Henry, N., and Lo, K. W.-H. (2014). Small molecule delivery through nanofibrous scaffolds for musculoskeletal regenerative engineering. Nanomedicine Nanotechnol. Biol. Med. 10 (8), 1691–1699. doi:10.1016/j.nano.2014.05.013
Cui, Z.-K., Sun, J. A., Baljon, J. J., Fan, J., Kim, S., Wu, B. M., et al. (2017). Simultaneous delivery of hydrophobic small molecules and siRNA using Sterosomes to direct mesenchymal stem cell differentiation for bone repair. Acta Biomater. 58, 214–224. doi:10.1016/j.actbio.2017.05.057
De la Torre, B. G., and Albericio, F. (2020). The pharmaceutical industry in 2019. An analysis of FDA drug approvals from the perspective of molecules. Mol. (Basel, Switz. 25 (3), 745. doi:10.3390/molecules25030745
Ding, C., Chen, Z., and Li, J. (2017). From molecules to macrostructures: Recent development of bioinspired hard tissue repair. Biomater. Sci. 5 (8), 1435–1449. doi:10.1039/c7bm00247e
Emara, A., and Shah, R. (2021). Recent update on craniofacial tissue engineering. J. Tissue Eng. 12, 204173142110037. doi:10.1177/20417314211003735
Fan, J., Guo, M., Im, C. S., Pi-Anfruns, J., Cui, Z.-K., Kim, S., et al. (2017b). Enhanced mandibular bone repair by combined treatment of bone morphogenetic protein 2 and small-molecule phenamil. Tissue Eng. Part A 23 (5–6), 195–207. doi:10.1089/ten.tea.2016.0308
Fan, J., Im, C. S., Cui, Z.-K., Guo, M., Bezouglaia, O., Fartash, A., et al. (2015). Delivery of phenamil enhances BMP-2-induced osteogenic differentiation of adipose-derived stem cells and bone formation in calvarial defects. Tissue Eng. Part A 21 (13–14), 2053–2065. doi:10.1089/ten.tea.2014.0489
Fan, J., Pi-Anfruns, J., Guo, M., Im, D. C. S., Cui, Z.-K., Kim, S., et al. (2017a). Small molecule-mediated tribbles homolog 3 promotes bone formation induced by bone morphogenetic protein-2. Sci. Rep. 7 (1), 7518. doi:10.1038/s41598-017-07932-z
Gregory, C. A., Lee, R. H., Liu, F., and Alge, D. (2022). Editorial: Approaches that foster a pro-regenerative environment. Front. Bioeng. Biotechnol. 10, 873375. doi:10.3389/fbioe.2022.873375
Heng, B. C., Jiang, S., Yi, B., Gong, T., Lim, L. W., and Zhang, C. (2019). Small molecules enhance neurogenic differentiation of dental-derived adult stem cells. Archives Oral Biol. 102, 26–38. doi:10.1016/j.archoralbio.2019.03.024
James, A. W., LaChaud, G., Shen, J., Asatrian, G., Nguyen, V., Zhang, X., et al. (2016). A review of the clinical side effects of bone morphogenetic protein-2. Tissue Eng. Part B Rev. 22 (4), 284–297. doi:10.1089/ten.teb.2015.0357
Jia, S., Zhou, J., Fanelli, C., Wee, Y., Bonds, J., Schneider, P., et al. (2017). Small-molecule Wnt agonists correct cleft palates in Pax9 mutant mice in utero. Dev. Camb. Engl. 144 (20), 3819–3828. doi:10.1242/dev.157750
Jourdan, J.-P., Bureau, R., Rochais, C., and Dallemagne, P. (2020). Drug repositioning: A brief overview. J. Pharm. Pharmacol. 72 (9), 1145–1151. doi:10.1111/jphp.13273
Kim, J.-Y., and Choung, P.-H. (2020). USP1 inhibitor ML323 enhances osteogenic potential of human dental pulp stem cells. Biochem. Biophysical Res. Commun. 530 (2), 418–424. doi:10.1016/j.bbrc.2020.05.095
Kornsuthisopon, C., Rochanavibhata, S., Nowwarote, N., Tompkins, K. A., Sukarawan, W., and Osathanon, T. (2022). 6-bromoindirubin-3’-oxime regulates colony formation, apoptosis, and odonto/osteogenic differentiation in human dental pulp stem cells. Int. J. Mol. Sci. 23 (15), 8676. doi:10.3390/ijms23158676
Laurencin, C. T., Ashe, K. M., Henry, N., Kan, H. M., and Lo, K. W.-H. (2014). Delivery of small molecules for bone regenerative engineering: Preclinical studies and potential clinical applications. Drug Discov. Today 19 (6), 794–800. doi:10.1016/j.drudis.2014.01.012
Liu, F., Cheng, X., Xiao, L., Wang, Q., Yan, K., Su, Z., et al. (2021). Inside-outside Ag nanoparticles-loaded polylactic acid electrospun fiber for long-term antibacterial and bone regeneration. Int. J. Biol. Macromol. 167, 1338–1348. doi:10.1016/j.ijbiomac.2020.11.088
Liu, Z.-Q., Shang, L.-L., and Ge, S.-H. (2021). Immunomodulatory effect of dimethyloxallyl glycine/nanosilicates-loaded fibrous structure on periodontal bone remodeling. J. Dent. Sci. 16 (3), 937–947. doi:10.1016/j.jds.2020.10.008
Lo, K. W.-H., Ashe, K. M., Kan, H. M., and Laurencin, C. T. (2012). The role of small molecules in musculoskeletal regeneration. Regen. Med. 7 (4), 535–549. doi:10.2217/rme.12.33
Lo, K. W.-H. (2022). Effects on bone regeneration of single-dose treatment with osteogenic small molecules. Drug Discov. Today 27 (6), 1538–1544. doi:10.1016/j.drudis.2022.02.020
Lo, K. W.-H., Jiang, T., Gagnon, K. A., Nelson, C., and Laurencin, C. T. (2014). Small-molecule based musculoskeletal regenerative engineering. Trends Biotechnol. 32 (2), 74–81. doi:10.1016/j.tibtech.2013.12.002
Lu, B., and Atala, A. (2014). Small molecules and small molecule drugs in regenerative medicine. Drug Discov. Today 19 (6), 801–808. doi:10.1016/j.drudis.2013.11.011
Ma, C., Tian, X., Kim, J. P., Xie, D., Ao, X., Shan, D., et al. (2018). Citrate-based materials fuel human stem cells by metabonegenic regulation. Proc. Natl. Acad. Sci. U. S. A. 115 (50), E11741–E11750. doi:10.1073/pnas.1813000115
Manlove, A. E., Romeo, G., and Venugopalan, S. R. (2020). Craniofacial growth: Current theories and influence on management. Oral Maxillofac. Surg. Clin. N. Am. 32 (2), 167–175. doi:10.1016/j.coms.2020.01.007
Masuda, Y., Sakagami, H., Yokose, S., and Udagawa, N. (2020). Effect of small-molecule GSK3 antagonist on differentiation of rat dental pulp cells into odontoblasts. Vivo 34 (3), 1071–1075. doi:10.21873/invivo.11877
Miura, M., Gronthos, S., Zhao, M., Lu, B., Fisher, L. W., Robey, P. G., et al. (2003). Shed: Stem cells from human exfoliated deciduous teeth. Proc. Natl. Acad. Sci. U. S. A. 100 (10), 5807–5812. doi:10.1073/pnas.0937635100
Moon, S.-H., Kim, I., and Kim, S. H. (2017). Mollugin enhances the osteogenic action of BMP-2 via the p38-Smad signaling pathway. Arch. Pharm. Res. 40 (11), 1328–1335. doi:10.1007/s12272-017-0964-4
Mozaffari, M. S., Emami, G., Khodadadi, H., and Baban, B. (2019). Stem cells and tooth regeneration: Prospects for personalized dentistry. EPMA J. 10 (1), 31–42. doi:10.1007/s13167-018-0156-4
Murugan, N. J., Vigran, H. J., Miller, K. A., Golding, A., Pham, Q. L., Sperry, M. M., et al. (2022). Acute multidrug delivery via a wearable bioreactor facilitates long-term limb regeneration and functional recovery in adult Xenopus laevis. Sci. Adv. 8 (4), eabj2164. doi:10.1126/sciadv.abj2164
Nakata, J., Akiba, Y., Nihara, J., Thant, L., Eguchi, K., Kato, H., et al. (2020). ROCK inhibitors enhance bone healing by promoting osteoclastic and osteoblastic differentiation. Biochem. Biophysical Res. Commun. 526 (3), 547–552. doi:10.1016/j.bbrc.2020.03.033
Neves, V. C. M., Babb, R., Chandrasekaran, D., and Sharpe, P. T. (2017). Promotion of natural tooth repair by small molecule GSK3 antagonists. Sci. Rep. 7, 39654. doi:10.1038/srep39654
Neves, V. C. M., and Sharpe, P. T. (2018). Regulation of reactionary dentine formation. J. Dent. Res. 97 (4), 416–422. doi:10.1177/0022034517743431
O’Neill, E., Rajpura, K., Carbone, E. J., Awale, G., Kan, H.-M., and Lo, K. W.-H. (2019). Repositioning tacrolimus: Evaluation of the effect of short-term tacrolimus treatment on osteoprogenitor cells and primary cells for bone regenerative engineering. Assay Drug Dev. Technol. 17 (2), 77–88. doi:10.1089/adt.2018.876
Ottensmeyer, P. F., Witzler, M., Schulze, M., and Tobiasch, E. (2018). Small molecules enhance scaffold-based bone grafts via purinergic receptor signaling in stem cells. Int. J. Mol. Sci. 19 (11), 3601. doi:10.3390/ijms19113601
Qi, X., Liu, Y., Ding, Z.-Y., Cao, J.-Q., Huang, J.-H., Zhang, J.-Y., et al. (2017). Synergistic effects of dimethyloxallyl glycine and recombinant human bone morphogenetic protein-2 on repair of critical-sized bone defects in rats. Sci. Rep. 7, 42820. doi:10.1038/srep42820
Rezia Rad, M., Khojaste, M., Hasan Shahriari, M., Asgary, S., and Khojasteh, A. (2016). Purmorphamine increased adhesion, proliferation and expression of osteoblast phenotype markers of human dental pulp stem cells cultured on beta-tricalcium phosphate. Biomed. Pharmacother. 82, 432–438. doi:10.1016/j.biopha.2016.05.016
Rodríguez-Méndez, I., Fernández-Gutiérrez, M., Rodríguez-Navarrete, A., Rosales-Ibáñez, R., Benito-Garzón, L., Vázquez-Lasa, B., et al. (2018). Bioactive Sr(II)/chitosan/poly(ε-caprolactone) scaffolds for craniofacial tissue regeneration. in vitro and in vivo behavior. Polymers 10 (3), 279. doi:10.3390/polym10030279
Sarigol-Calamak, E., and Hascicek, C. (2018). Tissue scaffolds as a local drug delivery system for bone regeneration. Adv. Exp. Med. Biol. 1078, 475–493. doi:10.1007/978-981-13-0950-2_25
Saxena, N., Cremer, M. A., Dolling, E. S., Nurrohman, H., Habelitz, S., Marshall, G. W., et al. (2018). Influence of fluoride on the mineralization of collagen via the polymer-induced liquid-precursor (PILP) process. Dent. Mat. 34 (9), 1378–1390. doi:10.1016/j.dental.2018.06.020
Schmidt, C. D., Patel, S. Y., Woerner, J. E., and Ghali, G. E. (2020). Obturation and tissue transfer for large craniofacial defects. Oral Maxillofac. Surg. Clin. N. Am. 32 (2), 219–232. doi:10.1016/j.coms.2020.01.009
Segar, C. E., Ogle, M. E., and Botchwey, E. A. (2013). Regulation of angiogenesis and bone regeneration with natural and synthetic small molecules. Curr. Pharm. Des. 19 (19), 3403–3419. doi:10.2174/1381612811319190007
Senarath-Yapa, K., Li, S., Walmsley, G. G., Zielins, E., Paik, K., Britto, J. A., et al. (2016). Small molecule inhibition of transforming growth factor beta signaling enables the endogenous regenerative potential of the mammalian calvarium. Tissue Eng. Part A 22 (9–10), 707–720. doi:10.1089/ten.tea.2015.0527
Shah, S., Esdaille, C. J., Bhattacharjee, M., Kan, H.-M., and Laurencin, C. T. (2022). The synthetic artificial stem cell (SASC): Shifting the paradigm of cell therapy in regenerative engineering. Proc. Natl. Acad. Sci. U. S. A. 119 (2), e2116865118. doi:10.1073/pnas.2116865118
Shang, L., Liu, Z., Ma, B., Shao, J., Wang, B., Ma, C., et al. (2021). Dimethyloxallyl glycine/nanosilicates-loaded osteogenic/angiogenic difunctional fibrous structure for functional periodontal tissue regeneration. Bioact. Mater. 6 (4), 1175–1188. doi:10.1016/j.bioactmat.2020.10.010
Sharma, S., and Sharma, D. (2018). Intelligently applying artificial intelligence in chemoinformatics. Curr. Top. Med. Chem. 18 (20), 1804–1826. doi:10.2174/1568026619666181120150938
Shi, A., Heinayati, A., Bao, D., Liu, H., Ding, X., Tong, X., et al. (2019). Small molecule inhibitor of TGF-β signaling enables robust osteogenesis of autologous GMSCs to successfully repair minipig severe maxillofacial bone defects. Stem Cell Res. Ther. 10 (1), 172. doi:10.1186/s13287-019-1281-2
Song, B., Fu, H., Liu, J., Ren, K., Weir, M. D., Schneider, A., et al. (2021). Bioactive small molecules in calcium phosphate scaffold enhanced osteogenic differentiation of human induced pluripotent stem cells. Dent. Mat. J. 40 (3), 615–624. doi:10.4012/dmj.2019-263
Song, J., and Leeuwenburgh, S. C. (2014). Sustained delivery of biomolecules from gelatin carriers for applications in bone regeneration. Ther. Deliv. 5 (8), 943–958. doi:10.4155/tde.14.42
Sun, P., Zhang, Q., Nie, W., Zhou, X., Chen, L., Du, H., et al. (2019). Biodegradable mesoporous silica nanocarrier bearing angiogenic QK peptide and dexamethasone for accelerating angiogenesis in bone regeneration. ACS Biomater. Sci. Eng. 5 (12), 6766–6778. doi:10.1021/acsbiomaterials.9b01521
Terauchi, M., Tamura, A., Arisaka, Y., Masuda, H., Yoda, T., and Yui, N. (2021). Cyclodextrin-based supramolecular complexes of osteoinductive agents for dental tissue regeneration. Pharmaceutics 13 (2), 136. doi:10.3390/pharmaceutics13020136
Wu, Z., Liu, C., Zang, G., and Sun, H. (2008). The effect of simvastatin on remodelling of the alveolar bone following tooth extraction. Int. J. Oral Maxillofac. Surg. 37 (2), 170–176. doi:10.1016/j.ijom.2007.06.018
Xu, J., Liu, X., Jiang, Y., Chu, L., Hao, H., Liua, Z., et al. (2008). MAPK/ERK signalling mediates VEGF-induced bone marrow stem cell differentiation into endothelial cell. J. Cell. Mol. Med. 12 (6A), 2395–2406. doi:10.1111/j.1582-4934.2008.00266.x
Yang, X., Ma, Y., Guo, W., Yang, B., and Tian, W. (2019). Stem cells from human exfoliated deciduous teeth as an alternative cell source in bio-root regeneration. Theranostics 9 (9), 2694–2711. doi:10.7150/thno.31801
Yi, B., Dissanayaka, W. L., and Zhang, C. (2020). Growth factors and small-molecule compounds in derivation of endothelial lineages from dental stem cells. J. Endod. 46 (9S), S63–S70. doi:10.1016/j.joen.2020.06.024
Zhang, J., Tong, D., Song, H., Ruan, R., Sun, Y., Lin, Y., et al. (2022). Osteoimmunity-regulating biomimetically hierarchical scaffold for augmented bone regeneration. Adv. Mater. Deerf. Beach, Fla.) 34 (36), 2202044. doi:10.1002/adma.202202044
Zhang, W., Shi, W., Wu, S., Kuss, M., Jiang, X., Untrauer, J. B., et al. (2020). 3D printed composite scaffolds with dual small molecule delivery for mandibular bone regeneration. Biofabrication 12 (3), 035020. doi:10.1088/1758-5090/ab906e
Zhang, Z., Nör, F., Oh, M., Cucco, C., Shi, S., and Nör, J. E. (2016). Wnt/β-catenin signaling determines the vasculogenic fate of postnatal mesenchymal stem cells. Stem Cells Dayt. Ohio) 34 (6), 1576–1587. doi:10.1002/stem.2334
Keywords: small molecules, regenerative engineering, drug discovery and delivery, craniofacial bone, dental materials, dentoalveolar
Citation: Mitchell J and Lo KWH (2022) Small molecule-mediated regenerative engineering for craniofacial and dentoalveolar bone. Front. Bioeng. Biotechnol. 10:1003936. doi: 10.3389/fbioe.2022.1003936
Received: 26 July 2022; Accepted: 18 October 2022;
Published: 02 November 2022.
Edited by:
Liqiang Wang, Shanghai Jiao Tong University, ChinaReviewed by:
Jianhua Li, Shandong University, ChinaJianxun Ding, Changchun Institute of Applied Chemistry (CAS), China
Copyright © 2022 Mitchell and Lo. This is an open-access article distributed under the terms of the Creative Commons Attribution License (CC BY). The use, distribution or reproduction in other forums is permitted, provided the original author(s) and the copyright owner(s) are credited and that the original publication in this journal is cited, in accordance with accepted academic practice. No use, distribution or reproduction is permitted which does not comply with these terms.
*Correspondence: Kevin W. H. Lo, d2xvQHVjaGMuZWR1