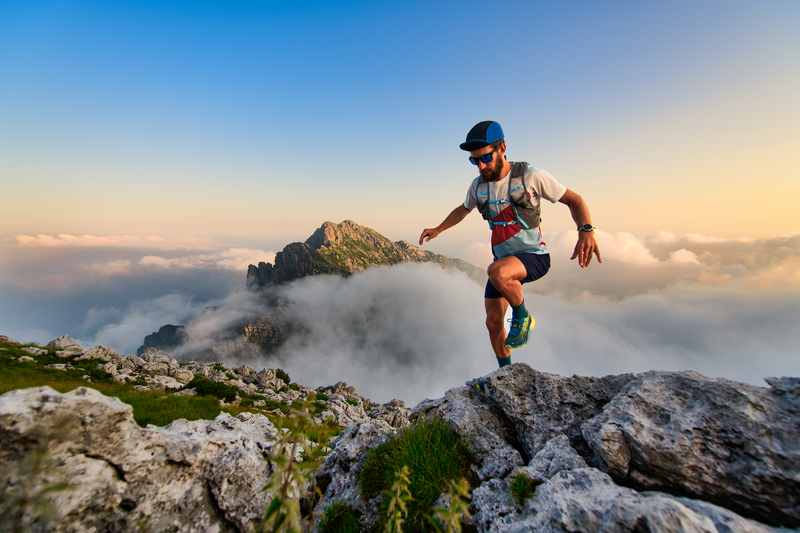
95% of researchers rate our articles as excellent or good
Learn more about the work of our research integrity team to safeguard the quality of each article we publish.
Find out more
REVIEW article
Front. Bioeng. Biotechnol. , 13 January 2022
Sec. Tissue Engineering and Regenerative Medicine
Volume 9 - 2021 | https://doi.org/10.3389/fbioe.2021.837464
This article is part of the Research Topic Insights in Tissue Engineering and Regenerative Medicine 2021: Novel Developments, Current Challenges, and Future Perspectives View all 14 articles
Over the last decade, stem cell-based regenerative medicine has progressed to clinical testing and therapeutic applications. The applications range from infusions of autologous and allogeneic stem cells to stem cell-derived products. Adult stem cells from adipose tissue (ASCs) show significant promise in treating autoimmune and neurodegenerative diseases, vascular and metabolic diseases, bone and cartilage regeneration and wound defects. The regenerative capabilities of ASCs in vivo are primarily orchestrated by their secretome of paracrine factors and cell-matrix interactions. More recent developments are focused on creating more complex structures such as 3D organoids, tissue elements and eventually fully functional tissues and organs to replace or repair diseased or damaged tissues. The current and future applications for ASCs in regenerative medicine are discussed here.
ASCs and ASC-derived extracellular vesicles (ASC-EVs) and ASC conditioned media (ASCs-CM) have been extensively studied and widely used in regenerative medicine. Studies have shown the effectiveness of ASCs and ASCs secretome (Zuk et al., 2001; Giannasi et al., 2020; Trzyna and Banaś-Ząbczyk, 2021) therapy in numerous diseases such as cardiovascular, bone regeneration, osteoarthritis graft versus host disease (GvHD) and autoimmune disorders such Crohn’s diseases (Galindo et al., 2017), systemic lupus erythematosus (SLE) and multiple sclerosis (Maria et al., 2017; Al-Ghadban and Bunnell, 2020). In addition, researchers have recently been designing new 3D biomaterials by combining ASCs with biomimetic scaffolds composed of either natural or synthetic materials. These 3D biomaterials have proven effective in tissue repair and organ regeneration (Storti et al., 2019; Gibler et al., 2021). These scaffolds have biological and physical properties that imitate the native ECM niche, which is crucial for stem cell adhesion, growth, proliferation and differentiation along particular lineages. Researchers are also incorporating ASCs into novel microfluidic systems, also known as organ-on-a-chip models, to model diseases in systems that function as intact organs, as substitutes for living organisms for testing of new therapeutic interventions (Zhang et al., 2017; Mofazzal Jahromi et al., 2019; O’Donnell et al., 2020). The content of this review is focused on the current and future applications of ASCs in regenerative medicine. The review also presents strategies and challenges in this field and explores the potential advancement of tissue engineering for clinical applications.
Adipose tissue is distributed throughout the body in tissues including the bone marrow, beneath the skin (subcutaneous), within joints (intra-articular) and around internal organs (visceral adipose tissue). Adipose tissue is also located in ectopic sites, including the liver (intra-hepatic) and muscle (intra-muscular). Adipose tissue was viewed as an inactive organ that functioned primarily as an energy reservoir for the longest time (Trayhurn and Wood, 2005; Cawthorn et al., 2012). However, the initial identification of leptin, a cytokine produced by adipose tissue, and subsequently numerous other adipokines, led to the re-classification of adipose tissue as an endocrine organ (Zhang et al., 1994). Adipose tissue can also produce several other cytokines, including pro-inflammatory mediators such as IL-6, TNF-a, IL-1b, IL-8, and MCP-1, that drive inflammation (Coppack, 2001; Caer et al., 2017).
Three distinct types of adipose tissue have been described in humans. White adipose tissue (WAT), localized subcutaneously or in depots within the abdomen, comprises adipocytes and functions as an energy storage depot. Brown adipose tissue (BAT) is distributed throughout the body in interscapular regions, supraclavicular, suprarenal, pericardial, para-aortic and around the pancreas, kidney and trachea. BAT has thermogenic activity induced by shivering and non-shivering mechanisms. The thermogenic activity of BAT is driven by the expression of the mitochondrial membrane protein Uncoupling Protein 1 (UCP1).
In comparison, beige (“brite” or “brown/white”) adipose tissue typically localizes with WAT. It serves as an energy storage depot that can express UCP1 and have thermogenic activity. The most common source of adipose tissue used to isolate ASCs is subcutaneous WAT collected from the abdomen, thigh, hips, or buttocks, typically during plastic surgical procedures.
Mature adipocytes are the primary cellular component of adipose tissue; however, adipose tissue represents a heterogeneous population of cells. Adipose tissue is comprised of preadipocytes, pericytes, fibroblasts, smooth muscle cells, endothelial cells, hematopoietic cells, mature immune cells such as B and T-cells, macrophages, myeloid cells and adipose tissue-derived mesenchymal stem cells (ASC).
ASCs are fibroblast-like cells isolated from the stromal vascular fraction (SVF) isolated by processing the adipose tissue (AT) by either enzymatic or mechanical methods. ASCs, comprise roughly 1–10% of the SVF. ASCs adhere to tissue culture plastic, proliferate in cell culture, undergo self-renewal, and effectively differentiate into multiple lineages, in vitro and in vivo. ASCs have been reported to differentiate into adipocytes, chondrocytes, osteoblasts, cardiomyocytes, skeletal muscle cells, neurons, hepatocytes and tenocytes, at least in vitro (Aurich et al., 2009; Vallée et al., 2009; Choi et al., 2010; Harris et al., 2011; Gimble et al., 2017; George et al., 2018; Mohiuddin O. A. et al., 2019; Lee et al., 2020; Harrison et al., 2022).
Flow cytometric analysis of the cell surface cluster of differentiation (CD) antigens is used to characterize ASCs. The majority of CD antigens screened are canonical markers of mesenchymal lineage cells. To date, an ASC-specific CD marker has not been identified that explicitly identifies ASCs. The analysis typically includes screening for both positive and negative CD antigens. Human ASCs are positive for known mesenchymal stem cell surface markers, including the cell adhesion molecules CD29, CD44, CD146, and CD166; the receptor molecules CD90 and CD105; and the GPI anchored enzyme CD73. In addition, ASC should be negative (< 2%) for the hematopoietic cell surface antigens, including CD11b, CD13, CD14, CD19, and CD45. They do not express the endothelial markers (CD31) and the human leukocyte antigen (HLA)-DR (Tucker and Bunnell, 2011; Al-Ghadban and Bunnell, 2020; Harrison et al., 2022). ASCs are unique in their expression of CD36 (fatty acid translocase) and the absence of vascular cell adhesion molecule (VCAM-1/CD106) (Rajashekhar et al., 2008; Baer et al., 2013). It is worth noting that ASCs isolated from two different subcutaneous adipose tissue depots (abdomen and thigh) express the same CD markers and demonstrated a similar multi-differentiation potential (Choudhery et al., 2015). However, ASCs isolated from subcutaneous adipose tissue showed functional differences such as gene expression, growth factor secretion, proliferation rates and differentiation potential compared to ASCs isolated from visceral AT, suggesting that ASCs isolated from different depots may have unique properties (Hocking et al., 2010; Baglioni et al., 2012; Ong et al., 2014). Despite the differences in their characteristics, ASCs isolated from different depots have been used successfully in both clinical research and tissue engineering applications.
Over the last decade, ASCs have been broadly applied in regenerative medicine applications. ASCs secrete numerous soluble mediators, inflammatory cytokines, angiogenic, trophic and growth factors such as vascular endothelial growth factor (VEGF), hepatocyte growth factor (HGF), brain-derived neurotrophic factor (BDNF), insulin-like growth factor (IGF), basic fibroblast growth factor (bFGF), transforming growth factor beta 1 (TGF-β1), stromal cell-derived factor (SDF)-1α, and interleukins. The paracrine factors secreted by ASCs contribute to tissue repair, wound healing and organ regeneration (Kapur and Katz, 2013; Suga et al., 2014; Brini et al., 2017; Li et al., 2019; Trzyna and Banaś-Ząbczyk, 2021). Studies have also shown that ASCs-EVs stimulate the regeneration of damaged tissue similar to the ASCs (Hu et al., 2016; Qiu et al., 2018). Basalova et al., have shown that ASCs-EV reduced fibrosis by stimulating myofibroblast dedifferentiation (Basalova et al., 2020). Other studies have also demonstrated the immunomodulatory effect of ASCs conditioned media in tissue repair and therapy (Yuan et al., 2018). In addition to the 2D monolayer cell cultures and 3D scaffolds extensively used to study the ASCs, an “organ-on-a-chip” technology is emerging as an advanced technique in stem cell-based therapy and will be discussed in this paper.
Fat grafting is a procedure that was initially attempted in 1889. Since then, many attempts have been made to create a standard protocol that will provide ideal outcomes. Cell-assisted lipotransfer (CAL), SVF and ASCs have demonstrated promise in adipose tissue regeneration and augmentation (Zhu et al., 2015; Li et al., 2017; Hong, 2020). The administration of ASCs enhances the outcomes of fat grafting in humans, particularly breast reconstruction, facial reconstruction and cosmetic surgery applications (Shukla et al., 2020b; Fang et al., 2021). This is mainly due to the ability of ASCs to differentiate into endothelial and epithelial cells as well as secrete cytokines and growth factors that promote angiogenesis through paracrine mechanisms and cell-cell interactions in a co-culture system, thus enhancing neovascularization and accelerating wound healing (Nie et al., 2011; Haubner et al., 2013). A study conducted by Kim et al. showed that ASCs intravenously injected in mice migrate to the wound area and promote tissue repair by differentiating into epithelial cells, thus inducing cutaneous regeneration by re-epithelialization of the dermal layer (Kim et al., 2019). Another study by Yu et al. and others demonstrated that transplanted ASC sheets enhance wound healing and reduce scar formation in a nude mice model. In this study, ASCs decreased inflammation and increased cellular viability in damaged tissue (Yu et al., 2018). In addition, fat grafting has shown to be an alternative for patients with wounds that do not respond to traditional therapy. A case report conducted by Vyas et al. demonstrated the ability of ASCs to promote wound healing in a patient suffering from a radiation-induced wound (Vyas et al., 2021). Furthermore, the use of lipoaspirate transplants containing ASCs has been shown to reverse skin necrosis after irradiation-induced degenerative chronic lesions in tissues exposed to oncologic radiotherapy (Rigotti et al., 2007).
Currently, cosmetic surgery represents the highest demand for ASCs, primarily for autologous transfers, with breast reconstruction and facial rejuvenation being the most popular applications (Bora and Majumdar, 2017). Fat reconstruction is typically used in facial soft-tissue repairs and to rebuild tissues subjected to surgical oncological treatments (Li et al., 2017). Nevertheless, the success rate of fat transplants varies between patients, which might be due to several factors, including the methods of ASCs isolation, surgical procedure, and the site of fat transplantation. One of the challenges of autologous fat grafts is fat reabsorption, decreasing the total volume of transplanted fat grafts by 20–70% (Bellini, 2017). However, the use of platelet-rich plasma (PRP), b-FGF, VEGF and estradiol as supplements for ASCs culture has demonstrated the ability to decrease the rate of fat reabsorption and enhanced fat transplant (Yuksel et al., 2000; Pires Fraga et al., 2010; Luo et al., 2013; Li et al., 2017).
ASCs have been demonstrated to mediate improvements after myocardial infarction via their inherent anti-apoptotic, anti-inflammatory, and pro-angiogenic effects. ASCs also contribute to inhibiting fibrosis and cardiac remodeling through the recruitment of endogenous stem cells and influence their re-entry into the cardiovascular lineage cell cycle (Li et al., 2019a). In a rat model of chronic ischemic cardiomyopathy (ICM), a group of researchers sought to identify early changes in cardiac cellular subpopulations and transcription after treatments with a well-characterized and pure cryopreserved allogeneic ASCs isolated from male Lewis (Follin et al., 2021). Treatment with ASCs resulted in altered inflammatory monocyte/macrophage subpopulations, increased CD4/CD8 ratio, and an increased uncharacterized CD31−CD45−CD90− population. Also, colony-stimulating factor 2, VEGFA and VEGFB were upregulated in the treated animals. These changes are associated with positive chemotaxis, monocyte and macrophage differentiation, and angiogenesis. These results indicate that some of the immediate effects of ASCs were related primarily to monocyte/macrophage regulation (Follin et al., 2021). The first human clinical trial report on the use of cryopreserved off-the-shelf ASCs in ischemic heart disease and heart failure treatment was published in 2017 (Kastrup et al., 2017). The study included ten patients ranging in ages from 30 to 80 years old. The follow-up was done at 1, 2, 3, and 6 months. ASCs were obtained from lipoaspirates from three healthy female donors of ages between 28 and 33 years old. After the 6-months follow-up, overall cardiac function showed a tendency to improve, with left ventricle pump function and a reduction in dilatation of the left ventricle. Interestingly enough, while two patients had donor-specific HLA antibodies at baseline and four patients developed donor-specific de novo, this did not affect the efficacy of the treatment, nor were there any significant adverse events post-treatment, suggesting that even with allogeneic ASCs transplantation, immunosuppressant drugs may not be required.
Bone tissue has an inherent ability to repair and regenerate; however, the “critical-size bone defect” concept limits the extent of this regeneration potential. This concept simply refers to the point at which the extension of the lesion is too great for the effective signal transduction and delivery of growth factors to drive the regeneration. At this point, scaffolds combined with stem cells such as ASCs are required (Mohiuddin O. A. et al., 2019; Storti et al., 2019). Nonetheless, bone-derived grafts are the second most transplanted material, next to blood. Only 28% of patients with open fractures can make a full recovery (Alonso-Goulart et al., 2021) on their own. Bone tissue is composed of three types of cells that maintain its integrity: osteoblast, osteoclast, and osteocyte cells. Bone remodeling and regeneration require the interaction between osteoclasts and osteoblasts (Baddour et al., 2012). Several studies have shown that ASCs can be differentiated into osteoblasts. Adding bone morphogenetic protein 2 (BMP-2), growth factors, PRP, extracellular calcium enhances their differentiation and bone regeneration (Zhang et al., 2014; Yanai et al., 2019). A case study by Mesimäki et al. demonstrated that autologous transplanted ASCs with BMP2 and beta-tricalcium phosphate reconstituted the defect area of the maxillary (Mesimäki et al., 2009). A similar study conducted by Wolff et al. and others showed successful reconstitution of the mandibular defects using tissue engineered constructs of autologous ASCs, beta-tricalcium phosphate (β-TCP) granules, recombinant human BMP-2 in a cohort of three patients (Wolff et al., 2013).
In addition to ASCs therapeutic potential, studies have shown that ASCs-Exo can stimulate bone regeneration through a paracrine mechanism (Zhu et al., 2021). Furthermore, in mouse models, decellularized adipose tissue hydrogels have shown promise, especially when combined with ASCs. The decellularized adipose tissue scaffolds promote bone regeneration and a higher volume of partially mineralized tissue and higher levels of collagen and osteopontin when the scaffolds are treated with either ASCs or osteogenic lineage induced ASCs and hydroxyapatite (Mohiuddin O. A. et al., 2019).
In general, cartilage has a narrow potential for regeneration (Tiku and Sabaawy, 2015). Of the different types of cartilage, articular cartilage is the most common therapy target, with joint injury and osteoarthritis (OA) being the main targets of regenerative medicine (Hulme et al., 2021). Clinical trials using autologous and allogeneic ASCs injections, ASCs-Exo, ASCs-CM to treat knee osteoarthritis have been reported to be well-tolerated and yield positive results in pain amelioration, decreased stiffness, and increased physical function with no severe side effects (Chen et al., 2021). A study conducted by Spasovski et al. showed that treating patients with autologous ASCs enhances their clinical scores and reduces pain levels (Spasovski et al., 2018). Another study by Pers and others demonstrated that patients who received autologous ASCs injections in the knee showed improvement due to the paracrine factors released from the cells inducing an anti-inflammatory response (Pers et al., 2018). A recent study conducted by Li and others described the use of ECM/SVF-gel fraction in cartilage defect repair as ASCs migrate from the gel and differentiate in a natural microenvironment, thus increasing the therapeutic potential of ASCs (Li et al., 2020). Furthermore, engineered cartilage is another area that is gaining interest, as ASCs and Poly ε-Caprolactone Scaffolds have successfully been used to regenerate cartilage in vitro for later transplantation into a mouse model (Nguyen and Vu, 2021).
While most reports on the use of ASCs to treat spinal cord injuries have been performed in animal models, human clinical trials are underway. Takahashi et al. investigated the outcomes of treating SCIs with either BM-MSCs or ASCs in a murine model (Takahashi et al., 2018). Their data revealed comparable levels of motor function improvement in moderate SCI models. They also observed a higher survival rate after transplantation in the severe SCIs model and improved neuronal and vascular protection in the ASC-treated group (Takahashi et al., 2018). Another group explored using hASCs combined with low-level laser in neuropathic pain in experimental SCI models in rats. Their results demonstrated that combining low-level laser treatment with ASCs improved the level of the motor function recovery and had superior outcomes in alleviating SCI-induced allodynia and hyperalgesia; compared to groups treated with ASCs alone (Sarveazad et al., 2019). The therapeutic potential of mesenchymal stem cells isolated from either bone marrow, adipose tissue, or dental pulp was explored by another group in both a small (rats) and a large (pigs) animal model in spinal cord injury during the spinal contusion subacute period (Mukhamedshina et al., 2019). Applying ASCs in combination with fibrin matrix in the rat model provided significantly higher post-traumatic regeneration results than other MSCs. However, while the application of ASCs embedded in the fibrin matrix at the SCI site in pigs restored neural tissue integrity, no significant functional improvements were noted. A few case studies utilizing ASCs in human spinal cord injury patients have positive outcomes. The first human trial was performed in South Korea in 14 patients. It employed intrathecal transplantation of autologous ASCs with an 8-months follow-up. While the injury site and degree of impairments were diverse, none of the patients developed severe adverse effects from the transplantation procedure. While there were no differences in the areas of spinal damage as observed in the MRIs before and after the procedure, five patients showed an improved ASIA motor score, ten patients showed improved ASIA sensory score, and two patients that had no control over anal sphincters recovered it after 1- and 4-months post-treatment, respectively (Hur et al., 2016). Finally, the first report from a patient from the CELLTOP study, an ongoing multidisciplinary phase 1 clinical trial conducted at Mayo Clinic, has been released (Bydon et al., 2020). A 53-year-old male patient underwent an autologous intrathecal injection 11 months after a surfing injury., there was a progressive improvement in motor and sensory ASIA scores and the overall quality of life. There were no reports of adverse events within the 18-months follow-up period. Overall, these results highlight that, in general, the potential benefits of using ASCs for spinal cord injury.
ASCs are known for their potent immunomodulatory potential, which permits the cells to sense their microenvironment and respond according to the circumstances. Additionally, mesenchymal stem cells from diverse sources have proven effective in impairing viral replication and reducing the viral load (Rogers et al., 2020). Based on that, researchers are currently investigating the possibility of utilizing ASCs to treat COVID-19 infected patients. The primary complications from COVID-19 infection include damage to the lung tissue, excessive inflammation, and progression of lung fibrosis. Shi and others summarized early clinical testing of ASC injections, which highlights the ability of MSCs to reduce infiltration by immune cells and help repair the damaged tissue (Shi et al., 2021). A study conducted by Leng et al. showed that intravenous injection of stem cells was very effective in treating COVID-19 patients with pneumonia by decreasing pro-inflammatory cytokines and increasing the anti-inflammatory cytokines such as IL-10, thus promoting lung repair (Leng et al., 2020). A study by Gentile and Sterodimas investigated the use of autologous or allogeneic ASCs to treat severe COVID-19 cases (Gentile and Sterodimas, 2020). ASC infusions were shown to inhibit the over-activation of the immune system, promoting endogenous repair by improving the lung microenvironment. Although ASCs have shown promising outcomes, the type of stem cells, the dose administered, the interval of time, and the delivery mechanism should be optimized for future clinical applications. Some of these issues may be addressed as results of ongoing COVID-19 clinical trials are published.
Nanotechnology has opened a new opportunities for novel applications of stem cells for numerous diseases, such as cardiovascular, neurological, vascular diseases, diabetes and inflammation (Masoudi Asil et al., 2020; Dong et al., 2021; Sarathkumar et al., 2021). It is worth noting that NPs are naturally occurring products from ASCs and are secreted as EVs (Wang et al., 2009; Dong et al., 2021). ASC-derived NPs offer novel, non-invasive methods and provide much information about tissue repair and develop a precise method for targeted therapy (Qiu et al., 2018; Dong et al., 2021). Beyond that combining stem cells, with scaffolds is driving the creation of organoids, organ-on-a-chip systems and lab grown organs and tissues.
Scaffolds, synthetic or natural “biologically-derived”, to be used in tissue engineering applications, must recapitulate the extracellular matrix (ECM) and imitate an in vivo like microenvironment favorable for stem cell attachment and proliferation. Synthetic scaffolds are made up of polyesters, polyethers, polyethylene glycol and polylactic acid (PLLA) (Gibler et al., 2021b; Reddy et al., 2021), while natural scaffolds “commonly used with ASCs” are comprised of collagen, fibrin, gelatin, vitronectin, laminin, alginate, hyaluronic acid, or decellularized materials (DAT) (Sadeghi-Ataabadi et al., 2017; Vinson et al., 2017; Kook et al., 2018; Mohiuddin O. A. et al., 2019; Colle et al., 2020; O’Donnell et al., 2020). The central characteristics of the scaffolds include the composition and porosity necessary to promote cell adhesion, proliferation and differentiation; fibrosity and stiffness; biocompatibility with the tissue and biodegradability with a negligible amount of toxicity or inflammation in vivo (Dhandayuthapani et al., 2011; Reddy et al., 2021). Several studies have incorporated ASCs into 3D scaffolds and demonstrated their ability to adhere, migrate and differentiate into specific cell lineages. The choice of nanomaterial used in generating ASC scaffolds should be carefully considered as it might influence the differentiation ability of stem cells. In addition to the ECM-ASC interactions in a scaffold, growth factors (such as VEGF, bFGF and TGF-β) can effectively be integrated to enhance the therapeutic potential by enhancing the proliferation and differentiation of stem cells (Howard et al., 2008; Sell et al., 2011; Sadeghi-Ataabadi et al., 2017). Similarly, studies have demonstrated the function of ASCs seeded on PRP fibrin enriched scaffolds in cartilage repair and tendon regeneration (Drengk et al., 2009; Barbon et al., 2019), as well as skin graft transplantation by inducing tissue angiogenesis (Wang et al., 2019; Gao et al., 2020). Nair and others showed that MSCs incorporated into graphene oxide (GO) scaffolds enhanced osteogenic differentiation. Thus, they may be used for bone regeneration in orthopedic applications (Nair et al., 2015). A similar test of GO scaffolds demonstrated their ability to induce neuronal differentiation of mesenchymal stem cells (Kim et al., 2015). In another study, PLLA and poly 3-hydroxybutyrate scaffolds combined with dental pulp-derived MSCs are a potential treatment of cardiovascular diseases (Castellano et al., 2014). Yin and others demonstrated ASC differentiation into chondrocytes in the context of PLGA gelatin scaffolds supplemented with TGF-β1 (Yin et al., 2015).
Decellularized adipose tissue hydrogels (hDAT) impregnated with stem cells have been widely tested as treatments for spinal cord and peripheral nerve injury, wound healing, myocardial infarction, cartilage repair, and bone tissue engineering. Mohiuddin and others have shown that DAT hydrogels support ASC proliferation and multilineage differentiation capabilities. Additionally, the ASCs remodeled the microstructure of the hydrogels, making them more compatible for in vivo-like adipose tissue regeneration (Mohiuddin O. A. et al., 2019). The use of decellularized scaffolds and ASCs in regenerative medicine is promising. However, more research into the method of preparation, “decellularization and sterilization,” should be optimized for each use, as it will affect the quality of stem cells attachment and differentiation. Yang et al. described the different decellularized and sterilization techniques used in the preparation of DAT hydrogels as well as the problems that needs to be resolved before their application in clinicals trials (Yang et al., 2020). The decellularization methods involve a combination of biological (enzymatic digestion DNase/RNase) chemical (isopropanol, Triton X and sodium chloride), and physical (freeze and thaw cycles, homogenization) treatments. The sterilization techniques mainly included 70% ethanol, penicillin and streptomycin, ethylene oxide and UV light (Song et al., 2018; Thomas-Porch et al., 2018; Yang et al., 2020). Although these methods have been proven to be effective in decellularization, the use of certain detergents and enzymes cause the loss of the main ECM components such as collagen, laminin and glycosaminoglycans (GAGs) and destruction of protein-protein interactions. Yang et al. also mentioned that residues of chemical and enzymatic substances or cell debris might affect stem cell adhesion differentiation of stem cells as well as induce an immune response. Thus, optimizing protocols for adipose tissue decellularization will be important to develop 3D biomaterial scaffolds that serve as effective in vitro models of the pathophysiology of various diseases and can be used to screen novel therapeutics and reduce the use of experimental animal models.
Microphysiological systems (MPS) are an advanced technology that permits researchers to study cell-cell, cell-ECM and cell-tissue interactions in an in the vivo-like dynamic microenvironment. MPS uses either 3D organoids “assembled from cell-cell interactions in a scaffold-free manner” or on scaffolds to develop “organ-on-a-chip” systems applicable for drug screening and assessment of novel treatments (Zhang et al., 2017; Ronaldson-Bouchard and Vunjak-Novakovic, 2018; Wu et al., 2020; Marrazzo et al., 2021). In MPS, stem cells can be seeded in layers or interconnected cell culture chambers linked by microchannels filled with media (particular to the cell type). They can also incorporate mechanical and biochemical stimuli to replicate human tissues’ physiology and biology in vivo. The microchannels included in MPS are designed to control the flow of the media between the chambers, and they can be sealed or perfused depending on the type of planned experiments. Although MPS has been widely investigated, only a few published articles presently report using stem cells, including ASCs (Zhang et al., 2017; Paek et al., 2019; O’Donnell et al., 2020; Marrazzo et al., 2021). Kefallinou and others demonstrated the generation of bone marrow-on-a-chip (BMoC), which may be useful as a system to study the stromal niche in diseases such as SLE (Kefallinoua et al., 2020). Another article published by Lavrentieva described a microfluidic gradient system using hASCs and human umbilical cord vein endothelial cells (HUVECs) were embedded in a methacrylated gelatin (GelMA) hydrogel to study the morphological changes and cellular interaction in a stem cell niche and how the stiffness of the gels plays a significant role in developing scaffolds used for clinical applications (Lavrentieva et al., 2020). An MPS combining human ASCs and human adipose microvascular endothelial cells (hAMECs) in a collagen and fibrin hydrogel scaffold to create a 3D microvascular-like network that recapitulates the interaction between the cell types and facilitates the study of vascular inflammation in a dynamic fluid system has been reported (Paek et al., 2019). The data collected using the MPS will provide novel research tools for developing preclinical models representing the physiological niche and potential treatment of vascular and metabolic diseases. However, more research is needed on how mechanical stimulus affects stem cell niche development under physiological conditions. One study has shown that interstitial shear stress in a fluidic system might decrease the ASCs’ ability to undergo adipogenesis (Bender et al., 2020).
Human pre-clinical and clinical trials are underway to provide treatment for multiple diseases, including chronic conditions such as systemic sclerosis, neurological degenerative diseases, non-healing wounds as in diabetic ulcers, or the treatment of fistulas in patients with Chron’s Disease, neurodegenerative diseases, atherothrombotic diseases, chronic kidney disease, degenerative osteoarthritis, and enhance recovery after ligament and tendon injuries, and promote the field of reconstructive plastic surgery in breast reconstruction and skin rejuvenation (Shukla et al., 2020). Nevertheless, there are still many hoops to jump ahead and a long way to get these therapies to move from clinical trials to FDA-approved treatments. One of the limitations for the usage of ASCs in regenerative medicine is the donor’s age, body mass index (BMI) and health conditions (underlying disease or comorbidities), which might result in reduced immunomodulatory abilities of main regulatory factors (Strong et al., 2013; Barwinska et al., 2018). Thus, ASCs should be fully characterizing and thoroughly screened for in vitro aging and inflammatory markers that might affect their regenerative abilities and hinder their usage in clinical application. Further, in-depth research is needed to study the effect of nanomaterial, pore size, stiffness, biodegradability on stem cell proliferation, migration and differentiation while developing scaffolds as a novel therapeutic tool that imitates the in vivo microenvironment. One of the limitations for using ASCs scaffolds in an MPS is their proliferation, migration and capability to multi-differentiate into particular lineages in a co-culture system for an extended period.
ASCs have therapeutic potential in regenerative medicine and applications in tissue engineering. While the biologic properties of ASCs are not yet fully delineated, the cells are under clinical investigation in human trials for an array of diseases. ASCs have been widely studied for their immunomodulatory effects, anti-fibrotic, anti-apoptotic, and anti-oxidative capabilities in preclinical and human clinical trials. Additionally, the ASC secretome (conditioned media or exosomes) has demonstrated similar effectiveness in regenerative medicine applications. However, the field must be cognizant of the inherent donor-to-donor variation, which can significantly impact the therapeutic potential of ASCs and ASC-derived products regarding their growth and differentiation efficiency, which will directly impact their therapeutic potential as cell therapies their effectiveness in more complex 3D systems.
Furthermore, the emerging technology combining stem cells with scaffolds or microfluidic chip technologies provides novel opportunities to develop more sophisticated and effective treatments with minimal risks and side effects. Therefore, ASC-based scaffolds and “organ-on-a-chip” models represent an advanced technology in regenerative medicine that will provide researchers with new tools to treat numerous diseases that conventional medicine could not effectively cure. However, investigators must address several considerations as the more complex 3D systems develop including, cell types required for their creation, development of standardized methodologies for their generation, criteria for characterization of how effectively it recapitulates an intact organ, and readouts for biologic and efficacy assessments.
Conceptualization and content design by BB; BB, SA-G and MA performed writing, review and editing. All authors have read and agreed to the published version of the manuscript.
This work was supported by NIH Grants UG3TR002136 and UG3TR003090.
The authors declare that the research was conducted in the absence of any commercial or financial relationships that could be construed as a potential conflict of interest.
All claims expressed in this article are solely those of the authors and do not necessarily represent those of their affiliated organizations, or those of the publisher, the editors, and the reviewers. Any product that may be evaluated in this article, or claim that may be made by its manufacturer, is not guaranteed or endorsed by the publisher.
Al-Ghadban, S., and Bunnell, B. A. (2020). Adipose Tissue-Derived Stem Cells: Immunomodulatory Effects and Therapeutic Potential. Physiology 35, 125–133. doi:10.1152/physiol.00021.2019
Alonso-Goulart, V., Carvalho, L. N., Marinho, A. L. G., De Oliveira Souza, B. L., De Aquino Pinto Palis, G., Lage, H. G. D., et al. (2021). Biomaterials and Adipose-Derived Mesenchymal Stem Cells for Regenerative Medicine: a Systematic Review. Materials 14, 4641. doi:10.3390/ma14164641
Aurich, H., Sgodda, M., Kaltwasser, P., Vetter, M., Weise, A., Liehr, T., et al. (2009). Hepatocyte Differentiation of Mesenchymal Stem Cells from Human Adipose Tissue In Vitro Promotes Hepatic Integration In Vivo. Gut 58, 570–581. doi:10.1136/gut.2008.154880
Baddour, J. A., Sousounis, K., and Tsonis, P. A. (2012). Organ Repair and Regeneration: an Overview. Birth Defects Res. C: Embryo Today Rev. 96, 1–29. doi:10.1002/bdrc.21006
Baer, P. C., Kuçi, S., Krause, M., Kuçi, Z., Zielen, S., Geiger, H., et al. (2013). Comprehensive Phenotypic Characterization of Human Adipose-Derived Stromal/stem Cells and Their Subsets by a High Throughput Technology. Stem Cells Development 22, 330–339. doi:10.1089/scd.2012.0346
Baglioni, S., Cantini, G., Poli, G., Francalanci, M., Squecco, R., Di Franco, A., et al. (2012). Functional Differences in Visceral and Subcutaneous Fat Pads Originate from Differences in the Adipose Stem Cell. PLoS One 7, e36569. doi:10.1371/journal.pone.0036569
Barbon, S., Stocco, E., Macchi, V., Contran, M., Grandi, F., Borean, A., et al. (2019). Platelet-rich Fibrin Scaffolds for Cartilage and Tendon Regenerative Medicine: from Bench to Bedside. Ijms 20, 1701. doi:10.3390/ijms20071701
Barwinska, D., Traktuev, D. O., Merfeld-Clauss, S., Cook, T. G., Lu, H., Petrache, I., et al. (2018). Cigarette Smoking Impairs Adipose Stromal Cell Vasculogenic Activity and Abrogates Potency to Ameliorate Ischemia. Stem Cells 36, 856–867. doi:10.1002/stem.2813
Basalova, N., Sagaradze, G., Arbatskiy, M., Evtushenko, E., Kulebyakin, K., Grigorieva, O., et al. (2020). Secretome of Mesenchymal Stromal Cells Prevents Myofibroblasts Differentiation by Transferring Fibrosis-Associated microRNAs within Extracellular Vesicles. Cells 9, 1272. doi:10.3390/cells9051272
Bender, R., Mccarthy, M., Brown, T., Bukowska, J., Smith, S., Abbott, R. D., et al. (2020). Human Adipose Derived Cells in Two- and Three-Dimensional Cultures: Functional Validation of an In Vitro Fat Construct. Stem Cells Int 2020, 4242130. doi:10.1155/2020/4242130
Bora, P., and Majumdar, A. S. (2017). Adipose Tissue-Derived Stromal Vascular Fraction in Regenerative Medicine: a Brief Review on Biology and Translation. Stem Cell Res Ther 8, 145. doi:10.1186/s13287-017-0598-y
Brini, A. T., Amodeo, G., Ferreira, L. M., Milani, A., Niada, S., Moschetti, G., et al. (2017). Therapeutic Effect of Human Adipose-Derived Stem Cells and Their Secretome in Experimental Diabetic Pain. Sci. Rep. 7, 9904. doi:10.1038/s41598-017-09487-5
Bydon, M., Dietz, A. B., Goncalves, S., Moinuddin, F. M., Alvi, M. A., Goyal, A., et al. (2020). CELLTOP Clinical Trial: First Report from a Phase 1 Trial of Autologous Adipose Tissue-Derived Mesenchymal Stem Cells in the Treatment of Paralysis Due to Traumatic Spinal Cord Injury. Mayo Clinic Proc. 95, 406–414. doi:10.1016/j.mayocp.2019.10.008
Caer, C., Christine Rouault, C., Le Roy, T., Christine Poitou, C., Aron-Wisnewsky, J., Adriana Torcivia, A., et al. (2017). Immune Cell-Derived Cytokines Contribute to Obesity-Related Inflammation, Fibrogenesis and Metabolic Deregulation in Human Adipose Tissue. Sci. Rep. 7, 3000.
Castellano, D., Blanes, M., Marco, B., Cerrada, I., Ruiz-Saurí, A., Pelacho, B., et al. (2014). A Comparison of Electrospun Polymers Reveals Poly(3-Hydroxybutyrate) Fiber as a superior Scaffold for Cardiac Repair. Stem Cells Development 23, 1479–1490. doi:10.1089/scd.2013.0578
Cawthorn, W. P., Scheller, E. L., and MacDougald, O. A. (2012). Adipose Tissue Stem Cells Meet Preadipocyte Commitment: Going Back to the Future. J. Lipid Res. 53, 227–246. doi:10.1194/jlr.r021089
Chen, C.-F., Hu, C.-C., Wu, C.-T., Wu, H.-T. H., Chang, C.-S., Hung, Y.-P., et al. (2021). Treatment of Knee Osteoarthritis with Intra-articular Injection of Allogeneic Adipose-Derived Stem Cells (ADSCs) ELIXCYTE®: a Phase I/II, Randomized, Active-Control, Single-Blind, Multiple-center Clinical Trial. Stem Cell Res. Ther. 12. doi:10.1186/s13287-021-02631-Z
Choi, Y. S., Dusting, G. J., Stubbs, S., Arunothayaraj, S., Han, X. L., Collas, P., et al. (2010). Differentiation of Human Adipose-Derived Stem Cells into Beating Cardiomyocytes. J. Cell Mol Med 14, 878–889. doi:10.1111/j.1582-4934.2010.01009.x
Choudhery, M. S., Badowski, M., Muise, A., Pierce, J., and Harris, D. T. (2015). Subcutaneous Adipose Tissue-Derived Stem Cell Utility Is Independent of Anatomical Harvest Site. BioResearch Open Access 4, 131–145. doi:10.1089/biores.2014.0059
Colle, J., Blondeel, P., De Bruyne, A., Bochar, S., Tytgat, L., Vercruysse, C., et al. (2020). Bioprinting Predifferentiated Adipose-Derived Mesenchymal Stem Cell Spheroids with Methacrylated Gelatin Ink for Adipose Tissue Engineering. J. Mater. Sci. Mater. Med. 31, 36. doi:10.1007/s10856-020-06374-w
Coppack, S. W. (2001). Pro-inflammatory Cytokines and Adipose Tissue. Proc. Nutr. Soc. 60, 349–356. doi:10.1079/pns2001110
Dhandayuthapani, B., Yoshida, Y., Maekawa, T., and Kumar, D. S. (2011). Polymeric Scaffolds in Tissue Engineering Application: a Review. Int. J. Polym. Sci 2011, 290602. doi:10.1155/2011/290602
Dong, Y., Wu, X., Chen, X., Zhou, P., Xu, F., and Liang, W. (2021). Nanotechnology Shaping Stem Cell Therapy: Recent Advances, Application, Challenges, and Future Outlook. Biomed. Pharmacother. 137, 111236. doi:10.1016/j.biopha.2021.111236
Drengk, A., Zapf, A., Stürmer, E. K., Stürmer, K. M., and Frosch, K.-H. (2009). Influence of Platelet-Rich Plasma on Chondrogenic Differentiation and Proliferation of Chondrocytes and Mesenchymal Stem Cells. Cells Tissues Organs 189, 317–326. doi:10.1159/000151290
Fang, J., Chen, F., Liu, D., Gu, F., and Wang, Y. (2021). Adipose Tissue-Derived Stem Cells in Breast Reconstruction: a Brief Review on Biology and Translation. Stem Cell Res Ther 12, 8. doi:10.1186/s13287-020-01955-6
Follin, B., Hoeeg, C., Højgaard, L. D., Juhl, M., Lund, K. B., Døssing, K. B. V., et al. (2021). The Initial Cardiac Tissue Response to Cryopreserved Allogeneic Adipose Tissue-Derived Mesenchymal Stromal Cells in Rats with Chronic Ischemic Cardiomyopathy. Int. J. Mol. Sci. 22. doi:10.3390/ijms222111758
Galindo, S., Herreras, J. M., López-Paniagua, M., Rey, E., De La Mata, A., Plata-Cordero, M., et al. (2017). Therapeutic Effect of Human Adipose Tissue-Derived Mesenchymal Stem Cells in Experimental Corneal Failure Due to Limbal Stem Cell Niche Damage. Stem Cells 35, 2160–2174. doi:10.1002/stem.2672
Gao, Y., Gao, B., Zhu, H., Yu, Q., Xie, F., Chen, C., et al. (2020). Adipose-derived Stem Cells Embedded in Platelet-Rich Plasma Scaffolds Improve the Texture of Skin Grafts in a Rat Full-Thickness Wound Model. Burns 46, 377–385. doi:10.1016/j.burns.2019.07.041
Gentile, P., and Sterodimas, A. (2020). Adipose-derived Stromal Stem Cells (ASCs) as a New Regenerative Immediate Therapy Combating Coronavirus (COVID-19)-Induced Pneumonia. Expert Opin. Biol. Ther. 20, 711–716. doi:10.1080/14712598.2020.1761322
George, S., Hamblin, M. R., and Abrahamse, H. (2018). Current and Future Trends in Adipose Stem Cell Differentiation into Neuroglia. Photomed. Laser Surg. 36, 230–240. doi:10.1089/pho.2017.4411
Giannasi, C., Niada, S., Magagnotti, C., Ragni, E., Andolfo, A., and Brini, A. T. (2020). Comparison of Two ASC-Derived Therapeutics in an In Vitro OA Model: Secretome versus Extracellular Vesicles. Stem Cell Res Ther 11, 521. doi:10.1186/s13287-020-02035-5
Gibler, P., Gimble, J., Hamel, K., Rogers, E., Henderson, M., Wu, X., et al. (2021). Human Adipose-Derived Stromal/stem Cell Culture and Analysis Methods for Adipose Tissue Modeling In Vitro: a Systematic Review. Cells 10, 1378. doi:10.3390/cells10061378
Gimble, J. M., Ray, S. P., Zanata, F., Wu, X., Wade, J., Khoobehi, K., et al. (2017). Adipose Derived Cells and Tissues for Regenerative Medicine. ACS Biomater. Sci. Eng. 3, 1477–1482. doi:10.1021/acsbiomaterials.6b00261
Harris, L. J., Abdollahi, H., Zhang, P., Mcilhenny, S., Tulenko, T. N., and Dimuzio, P. J. (2011). Differentiation of Adult Stem Cells into Smooth Muscle for Vascular Tissue Engineering. J. Surg. Res. 168, 306–314. doi:10.1016/j.jss.2009.08.001
Harrison, M. A. A., Al-Ghadban, S. I., O’Donnell, B. T., Mohiuddin, O. A., Wise, R. M., Sullivan, B. N., et al. (2022). Establishing the Adipose Stem Cell Identity: Characterization Assays and Functional Properties. Scientific Principles of Adipose Stem Cells, 23–56. doi:10.1016/b978-0-12-819376-1.00002-0
Haubner, F., Leyh, M., Ohmann, E., Pohl, F., Prantl, L., and Gassner, H. G. (2013). Effects of External Radiation in a Co-culture Model of Endothelial Cells and Adipose-Derived Stem Cells. Radiat. Oncol. 8, 66. doi:10.1186/1748-717x-8-66
Hocking, S. L., Wu, L. E., Guilhaus, M., Chisholm, D. J., and James, D. E. (2010). Intrinsic Depot-specific Differences in the Secretome of Adipose Tissue, Preadipocytes, and Adipose Tissue-Derived Microvascular Endothelial Cells. Diabetes 59, 3008–3016. doi:10.2337/db10-0483
Hong, K. Y. (2020). Fat Grafts Enriched with Adipose-Derived Stem Cells. Arch. Craniofac. Surg. 21, 211–218. doi:10.7181/acfs.2020.00325
Howard, D., Buttery, L. D., Shakesheff, K. M., and Roberts, S. J. (2008). Tissue Engineering: Strategies, Stem Cells and Scaffolds. J. Anat. 213, 66–72. doi:10.1111/j.1469-7580.2008.00878.x
Hu, L., Wang, J., Zhou, X., Xiong, Z., Zhao, J., Yu, R., et al. (2016). Exosomes Derived from Human Adipose Mensenchymal Stem Cells Accelerates Cutaneous Wound Healing via Optimizing the Characteristics of Fibroblasts. Sci. Rep. 6, 32993. doi:10.1038/srep32993
Hulme, C. H., Perry, J., Mccarthy, H. S., Wright, K. T., Snow, M., Mennan, C., et al. (2021). Cell Therapy for Cartilage Repair. Emerging Top. Life Sci. 5, 575–589. doi:10.1042/etls20210015
Hur, J. W., Cho, T.-H., Park, D.-H., Lee, J.-B., Park, J.-Y., and Chung, Y.-G. (2016). Intrathecal Transplantation of Autologous Adipose-Derived Mesenchymal Stem Cells for Treating Spinal Cord Injury: A Human Trial. J. Spinal Cord Med. 39, 655–664. doi:10.1179/2045772315y.0000000048
Kapur, S. K., and Katz, A. J. (2013). Review of the Adipose Derived Stem Cell Secretome. Biochimie 95, 2222–2228. doi:10.1016/j.biochi.2013.06.001
Kastrup, J., Haack-Sørensen, M., Juhl, M., Harary Søndergaard, R., Follin, B., Drozd Lund, L., et al. (2017). Cryopreserved Off-The-Shelf Allogeneic Adipose-Derived Stromal Cells for Therapy in Patients with Ischemic Heart Disease and Heart Failure-A Safety Study. Stem Cells Transl Med 6, 1963–1971. doi:10.1002/sctm.17-0040
Kefallinou, D., Grigoriou, M., Boumpas, D. T., Gogolides, E., and Tserepi, A. (2020). Fabrication of a 3D Microfluidic Cell Culture Device for Bone Marrow-On-A-Chip. Micro Nano Eng. 9, 100075. doi:10.1016/j.mne.2020.100075
Kim, H., Hyun, M. R., and Kim, S. W. (2019). The Effect of Adipose-Derived Stem Cells on Wound Healing: Comparison of Methods of Application. Stem Cells Int 2019, 2745640. doi:10.1155/2019/2745640
Kim, J., Park, S., Kim, Y. J., Jeon, C. S., Lim, K. T., Seonwoo, H., et al. (2015). Monolayer Graphene-Directed Growth and Neuronal Differentiation of Mesenchymal Stem Cells. J. Biomed. Nanotechnol 11, 2024–2033. doi:10.1166/jbn.2015.2137
Kook, Y.-M., Kim, H., Kim, S., Heo, C. Y., Park, M. H., Lee, K., et al. (2018). Promotion of Vascular Morphogenesis of Endothelial Cells Co-cultured with Human Adipose-Derived Mesenchymal Stem Cells Using Polycaprolactone/gelatin Nanofibrous Scaffolds. Nanomaterials 8, 117. doi:10.3390/nano8020117
Lavrentieva, A., Fleischhammer, T., Enders, A., Pirmahboub, H., Bahnemann, J., and Pepelanova, I. (2020). Fabrication of Stiffness Gradients of GelMA Hydrogels Using a 3D Printed Micromixer. Macromol Biosci. 20, e2000107. doi:10.1002/mabi.202000107
Lee, J., Lee, C. Y., Park, J.-H., Seo, H.-H., Shin, S., Song, B.-W., et al. (2020). Differentiation of Adipose-Derived Stem Cells into Functional Chondrocytes by a Small Molecule that Induces Sox9. Exp. Mol. Med. 52, 672–681. doi:10.1038/s12276-020-0424-y
Leng, Z., Zhu, R., Hou, W., Feng, Y., Yang, Y., Han, Q., et al. (2020). Transplantation of ACE2- Mesenchymal Stem Cells Improves the Outcome of Patients with COVID-19 Pneumonia. Aging Dis. 11, 216–228. doi:10.14336/ad.2020.0228
Li, K., Li, F., Li, J., Wang, H., Zheng, X., Long, J., et al. (2017). Increased Survival of Human Free Fat Grafts with Varying Densities of Human Adipose-Derived Stem Cells and Platelet-Rich Plasma. J. Tissue Eng. Regen. Med. 11, 209–219. doi:10.1002/term.1903
Li, Q., Zhao, F., Li, Z., Duan, X., Cheng, J., Zhang, J., et al. (2020). Autologous Fractionated Adipose Tissue as a Natural Biomaterial and Novel One-Step Stem Cell Therapy for Repairing Articular Cartilage Defects. Front Cell Dev Biol 8, 694. doi:10.3389/fcell.2020.00694
Li, X., Ma, T., Sun, J., Shen, M., Xue, X., Chen, Y., et al. (2019). Harnessing the Secretome of Adipose-Derived Stem Cells in the Treatment of Ischemic Heart Diseases. Stem Cell Res Ther 10, 196. doi:10.1186/s13287-019-1289-7
Luo, S., Hao, L., Li, X., Yu, D., Diao, Z., Ren, L., et al. (2013). Adipose Tissue-Derived Stem Cells Treated with Estradiol Enhance Survival of Autologous Fat Transplants. Tohoku J. Exp. Med. 231, 101–110. doi:10.1620/tjem.231.101
Maria, A. T. J., Maumus, M., Le Quellec, A., Jorgensen, C., Noël, D., and Guilpain, P. (2017). Adipose-derived Mesenchymal Stem Cells in Autoimmune Disorders: State of the Art and Perspectives for Systemic Sclerosis. Clinic Rev. Allerg Immunol. 52, 234–259. doi:10.1007/s12016-016-8552-9
Marrazzo, P., Pizzuti, V., Zia, S., Sargenti, A., Gazzola, D., Roda, B., et al. (2021). Microfluidic Tools for Enhanced Characterization of Therapeutic Stem Cells and Prediction of Their Potential Antimicrobial Secretome. Antibiotics (Basel) 10. doi:10.3390/antibiotics10070750
Masoudi Asil, S., Ahlawat, J., Guillama Barroso, G., and Narayan, M. (2020). Application of Nanotechnology in Stem-Cell-Based Therapy of Neurodegenerative Diseases. Appl. Sci. 10, 4852. doi:10.3390/app10144852
Mesimäki, K., Lindroos, B., Törnwall, J., Mauno, J., Lindqvist, C., Kontio, R., et al. (2009). Novel Maxillary Reconstruction with Ectopic Bone Formation by GMP Adipose Stem Cells. Int. J. Oral Maxillofac. Surg. 38, 201–209.
Mofazzal Jahromi, M. A., Abdoli, A., Rahmanian, M., Bardania, H., Bayandori, M., Moosavi Basri, S. M., et al. (2019). Microfluidic Brain-On-A-Chip: Perspectives for Mimicking Neural System Disorders. Mol. Neurobiol. 56, 8489–8512. doi:10.1007/s12035-019-01653-2
Mohiuddin, O. A., O'Donnell, B. T., Poche, J. N., Iftikhar, R., Wise, R. M., Motherwell, J. M., et al. (2019b). Human Adipose-Derived Hydrogel Characterization Based on In Vitro ASC Biocompatibility and Differentiation. Stem Cells Int 2019, 9276398. doi:10.1155/2019/9276398
Mohiuddin, O. A., Campbell, B., Poche, J. N., Ma, M., Rogers, E., Gaupp, D., et al. (2019a). Decellularized Adipose Tissue Hydrogel Promotes Bone Regeneration in Critical-Sized Mouse Femoral Defect Model. Front. Bioeng. Biotechnol. 7, 211. doi:10.3389/fbioe.2019.00211
Mukhamedshina, Y., Shulman, I., Ogurcov, S., Kostennikov, A., Zakirova, E., Akhmetzyanova, E., et al. (2019). Mesenchymal Stem Cell Therapy for Spinal Cord Contusion: A Comparative Study on Small and Large Animal Models. Biomolecules 9, 811. doi:10.3390/biom9120811
Nair, M., Nancy, D., Krishnan, A. G., Anjusree, G. S., Vadukumpully, S., and Nair, S. V. (2015). Graphene Oxide Nanoflakes Incorporated Gelatin-Hydroxyapatite Scaffolds Enhance Osteogenic Differentiation of Human Mesenchymal Stem Cells. Nanotechnology 26, 161001. doi:10.1088/0957-4484/26/16/161001
Nguyen, H. T., and Vu, N. B. (2021). A Simple Method to Produce Engineered Cartilage from Human Adipose-Derived Mesenchymal Stem Cells and Poly ε-Caprolactone Scaffolds. Adv. Exp. Med. Biol. doi:10.1007/5584_2021_669
Nie, C., Yang, D., Xu, J., Si, Z., Jin, X., and Zhang, J. (2011). Locally Administered Adipose-Derived Stem Cells Accelerate Wound Healing through Differentiation and Vasculogenesis. Cell Transpl. 20, 205–216. doi:10.3727/096368910x520065
O’Donnell, B. T., Al-Ghadban, S., Ives, C. J., L’ecuyer, M. P., Monjure, T. A., Romero-Lopez, M., et al. (2020). Adipose Tissue-Derived Stem Cells Retain Their Adipocyte Differentiation Potential in Three-Dimensional Hydrogels and Bioreactors (†). Biomolecules 10 (7), 1–16. doi:10.3390/biom10071070
Ong, W. K., Tan, C. S., Chan, K. L., Goesantoso, G. G., Chan, X. H. D., Chan, E., et al. (2014). Identification of Specific Cell-Surface Markers of Adipose-Derived Stem Cells from Subcutaneous and Visceral Fat Depots. Stem Cell Rep. 2, 171–179. doi:10.1016/j.stemcr.2014.01.002
Paek, J., Park, S. E., Lu, Q., Park, K.-T., Cho, M., Oh, J. M., et al. (2019). Microphysiological Engineering of Self-Assembled and Perfusable Microvascular Beds for the Production of Vascularized Three-Dimensional Human Microtissues. ACS Nano 13, 7627–7643. doi:10.1021/acsnano.9b00686
Pers, Y.-M., Quentin, J., Feirreira, R., Espinoza, F., Abdellaoui, N., Erkilic, N., et al. (2018). Injection of Adipose-Derived Stromal Cells in the Knee of Patients with Severe Osteoarthritis Has a Systemic Effect and Promotes an Anti-inflammatory Phenotype of Circulating Immune Cells. Theranostics 8, 5519–5528. doi:10.7150/thno.27674
Pires Fraga, M. F., Nishio, R. T., Ishikawa, R. S., Perin, L. F., Helene, A., and Malheiros, C. A. (2010). Increased Survival of Free Fat Grafts with Platelet-Rich Plasma in Rabbits. J. Plast. Reconstr. Aesthet. Surg. 63, e818–e822. doi:10.1016/j.bjps.2010.07.003
Qiu, G., Zheng, G., Ge, M., Wang, J., Huang, R., Shu, Q., et al. (2018). Mesenchymal Stem Cell-Derived Extracellular Vesicles Affect Disease Outcomes via Transfer of microRNAs. Stem Cell Res Ther 9, 320. doi:10.1186/s13287-018-1069-9
Rajashekhar, G., Traktuev, D. O., Roell, W. C., Johnstone, B. H., Merfeld‐Clauss, S., Van Natta, B., et al. (2008). IFATS Collection: Adipose Stromal Cell Differentiation Is Reduced by Endothelial Cell Contact and Paracrine Communication: Role of Canonical Wnt Signaling. Stem Cells 26, 2674–2681. doi:10.1634/stemcells.2008-0277
Reddy, M. S. B., Ponnamma, D., Choudhary, R., and Sadasivuni, K. K. (2021). A Comparative Review of Natural and Synthetic Biopolymer Composite Scaffolds. Polymers 13, 1105. doi:10.3390/polym13071105
Rigotti, G., Marchi, A., Galie, M., Baroni, G., Benati, D., Krampera, M., et al. (2007). Clinical Treatment of Radiotherapy Tissue Damage by Lipoaspirate Transplant: a Healing Process Mediated by Adipose-Derived Adult Stem Cells. Plast. Reconstr. Surg. 119, 1409–1422. doi:10.1097/01.prs.0000256047.47909.71
Rogers, C. J., Harman, R. J., Bunnell, B. A., Schreiber, M. A., Xiang, C., Wang, F.-S., et al. (2020). Rationale for the Clinical Use of Adipose-Derived Mesenchymal Stem Cells for COVID-19 Patients. J. Transl Med. 18, 203. doi:10.1186/s12967-020-02380-2
Ronaldson-Bouchard, K., and Vunjak-Novakovic, G. (2018). Organs-on-a-Chip: A Fast Track for Engineered Human Tissues in Drug Development. Cell Stem Cell 22, 310–324. doi:10.1016/j.stem.2018.02.011
Sadeghi-Ataabadi, M., Mostafavi-Pour, Z., Vojdani, Z., Sani, M., Latifi, M., and Talaei-Khozani, T. (2017). Fabrication and Characterization of Platelet-Rich Plasma Scaffolds for Tissue Engineering Applications. Mater. Sci. Eng. C 71, 372–380. doi:10.1016/j.msec.2016.10.001
Sarathkumar, E., Victor, M., Menon, J. A., Jibin, K., Padmini, S., and Jayasree, R. S. (2021). Nanotechnology in Cardiac Stem Cell Therapy: Cell Modulation, Imaging and Gene Delivery. RSC Adv. 11, 34572–34588. doi:10.1039/d1ra06404e
Sarveazad, A., Janzadeh, A., Taheripak, G., Dameni, S., Yousefifard, M., and Nasirinezhad, F. (2019). Co-administration of Human Adipose-Derived Stem Cells and Low-Level Laser to Alleviate Neuropathic Pain after Experimental Spinal Cord Injury. Stem Cell Res Ther 10, 183. doi:10.1186/s13287-019-1269-y
Sell, S. A., Wolfe, P. S., Ericksen, J. J., Simpson, D. G., and Bowlin, G. L. (2011). Incorporating Platelet-Rich Plasma into Electrospun Scaffolds for Tissue Engineering Applications. Tissue Eng. A 17, 2723–2737. doi:10.1089/ten.tea.2010.0663
Shi, L., Wang, L., Xu, R., Zhang, C., Xie, Y., Liu, K., et al. (2021). Mesenchymal Stem Cell Therapy for Severe COVID-19. Sig Transduct Target. Ther. 6, 339. doi:10.1038/s41392-021-00754-6
Shukla, L., Yuan, Y., Shayan, R., Greening, D. W., and Karnezis, T. (2020). Fat Therapeutics: the Clinical Capacity of Adipose-Derived Stem Cells and Exosomes for Human Disease and Tissue Regeneration. Front. Pharmacol. 11, 158. doi:10.3389/fphar.2020.00158
Song, M., Liu, Y., and Hui, L. (2018). Preparation and Characterization of Acellular Adipose Tissue Matrix Using a Combination of Physical and Chemical Treatments. Mol. Med. Rep. 17, 138–146. doi:10.3892/mmr.2017.7857
Spasovski, D., Spasovski, V., Baščarević, Z., Stojiljković, M., Vreća, M., Anđelković, M., et al. (2018). Intra-articular Injection of Autologous Adipose-Derived Mesenchymal Stem Cells in the Treatment of Knee Osteoarthritis. J. Gene Med. 20. doi:10.1002/jgm.3002
Storti, G., Scioli, M. G., Kim, B. S., Orlandi, A., and Cervelli, V. (2019). Adipose-derived Stem Cells in Bone Tissue Engineering: Useful Tools with New Applications. Stem Cells Int 2019, 3673857. doi:10.1155/2019/3673857
Strong, A. L., Strong, T. A., Rhodes, L. V., Semon, J. A., Zhang, X., Shi, Z., et al. (2013). Obesity Associated Alterations in the Biology of Adipose Stem Cells Mediate Enhanced Tumorigenesis by Estrogen Dependent Pathways. Breast Cancer Res. 15, R102. doi:10.1186/bcr3569
Suga, H., Glotzbach, J. P., Sorkin, M., Longaker, M. T., and Gurtner, G. C. (2014). Paracrine Mechanism of Angiogenesis in Adipose-Derived Stem Cell Transplantation. Ann. Plast. Surg. 72, 234–241. doi:10.1097/sap.0b013e318264fd6a
Takahashi, A., Nakajima, H., Uchida, K., Takeura, N., Honjoh, K., Watanabe, S., et al. (2018). Comparison of Mesenchymal Stromal Cells Isolated from Murine Adipose Tissue and Bone Marrow in the Treatment of Spinal Cord Injury. Cell Transpl. 27, 1126–1139. doi:10.1177/0963689718780309
Thomas-Porch, C., Li, J., Zanata, F., Martin, E. C., Pashos, N., Genemaras, K., et al. (2018). Comparative Proteomic Analyses of Human Adipose Extracellular Matrices Decellularized Using Alternative Procedures. J. Biomed. Mater. Res. 106, 2481–2493. doi:10.1002/jbm.a.36444
Tiku, M. L., and Sabaawy, H. E. (2015). Cartilage Regeneration for Treatment of Osteoarthritis: a Paradigm for Nonsurgical Intervention. Ther. Adv. Musculoskelet. 7, 76–87. doi:10.1177/1759720x15576866
Trayhurn, P., and Wood, I. S. (2005). Signalling Role of Adipose Tissue: Adipokines and Inflammation in Obesity. Biochem. Soc. Trans. 33, 1078–1081. doi:10.1042/bst0331078
Trzyna, A., and Banaś-Ząbczyk, A. (2021). Adipose-Derived Stem Cells Secretome and its Potential Application in "Stem Cell-Free Therapy". Biomolecules 11. doi:10.3390/biom11060878
Tucker, H. A., and Bunnell, B. A. (2011). Characterization of Human Adipose-Derived Stem Cells Using Flow Cytometry. Methods Mol. Biol. 702, 121–131. doi:10.1007/978-1-61737-960-4_10
Vallée, M., Côté, J. F., and Fradette, J. (2009). Adipose-tissue Engineering: Taking Advantage of the Properties of Human Adipose-Derived Stem/stromal Cells. Pathol. Biol. (Paris) 57, 309–317.
Vinson, B. T., Phamduy, T. B., Shipman, J., Riggs, B., Strong, A. L., Sklare, S. C., et al. (2017). Laser Direct-Write Based Fabrication of a Spatially-Defined, Biomimetic Construct as a Potential Model for Breast Cancer Cell Invasion into Adipose Tissue. Biofabrication 9, 025013. doi:10.1088/1758-5090/aa6bad
Vyas, K. S., Saba, E. S., and Tran, N. (2021). Regenerative Properties of Autologous Fat Grafting in a Complicated Radiation-Induced Wound. Wounds 33, E20–E23.
Wang, M., Wang, C., Chen, M., Xi, Y., Cheng, W., Mao, C., et al. (2019). Efficient Angiogenesis-Based Diabetic Wound Healing/Skin Reconstruction through Bioactive Antibacterial Adhesive Ultraviolet Shielding Nanodressing with Exosome Release. ACS Nano 13, 10279–10293. doi:10.1021/acsnano.9b03656
Wang, Z., Ruan, J., and Cui, D. (2009). Advances and prospect of Nanotechnology in Stem Cells. Nanoscale Res. Lett. 4, 593–605. doi:10.1007/s11671-009-9292-z
Wolff, J., Sándor, G. K., Miettinen, A., Tuovinen, V. J., Mannerström, B., Patrikoski, M., et al. (2013). GMP-level Adipose Stem Cells Combined with Computer-Aided Manufacturing to Reconstruct Mandibular Ameloblastoma Resection Defects: Experience with Three Cases. Ann. Maxillofac. Surg. 3, 114–125. doi:10.4103/2231-0746.119216
Wu, Q., Liu, J., Wang, X., Feng, L., Wu, J., Zhu, X., et al. (2020). Organ-on-a-chip: Recent Breakthroughs and Future Prospects. Biomed. Eng. Online 19, 9. doi:10.1186/s12938-020-0752-0
Yanai, R., Tetsuo, F., Ito, S., Itsumi, M., Yoshizumi, J., Maki, T., et al. (2019). Extracellular Calcium Stimulates Osteogenic Differentiation of Human Adipose-Derived Stem Cells by Enhancing Bone Morphogenetic Protein-2 Expression. Cell Calcium 83, 102058. doi:10.1016/j.ceca.2019.102058
Yang, J.-Z., Qiu, L.-H., Xiong, S.-H., Dang, J.-L., Rong, X.-K., Hou, M.-M., et al. (2020). Decellularized Adipose Matrix Provides an Inductive Microenvironment for Stem Cells in Tissue Regeneration. Wjsc 12, 585–603. doi:10.4252/wjsc.v12.i7.585
Yin, F., Cai, J., Zen, W., Wei, Y., Zhou, W., Yuan, F., et al. (2015). Cartilage Regeneration of Adipose-Derived Stem Cells in the TGF-β1-Immobilized PLGA-Gelatin Scaffold. Stem Cell Rev Rep 11, 453–459. doi:10.1007/s12015-014-9561-9
Yu, J., Wang, M.-Y., Tai, H.-C., and Cheng, N.-C. (2018). Cell Sheet Composed of Adipose-Derived Stem Cells Demonstrates Enhanced Skin Wound Healing with Reduced Scar Formation. Acta Biomater. 77, 191–200. doi:10.1016/j.actbio.2018.07.022
Yuan, B., Broadbent, J. A., Huan, J., and Yang, H. (2018). The Effects of Adipose Stem Cell-Conditioned Media on Fibrogenesis of Dermal Fibroblasts Stimulated by Transforming Growth Factor-β1. J. Burn Care Res. 39, 129–140. doi:10.1097/BCR.0000000000000558
Yuksel, E., Weinfeld, A. B., Cleek, R., Wamsley, S., Jensen, J., Boutros, S., et al. (2000). Increased Free Fat-Graft Survival with the Long-Term, Local Delivery of Insulin, Insulin-like Growth Factor-I, and Basic Fibroblast Growth Factor by PLGA/PEG Microspheres. Plast. Reconstr. Surg. 105, 1712–1720. doi:10.1097/00006534-200004050-00017
Zhang, J., Wei, X., Zeng, R., Xu, F., and Li, X. (2017). Stem Cell Culture and Differentiation in Microfluidic Devices toward Organ-On-A-Chip. Future Sci. OA 3, Fso187. doi:10.4155/fsoa-2016-0091
Zhang, X., Guo, J., Zhou, Y., and Wu, G. (2014). The Roles of Bone Morphogenetic Proteins and Their Signaling in the Osteogenesis of Adipose-Derived Stem Cells. Tissue Eng. B: Rev. 20, 84–92. doi:10.1089/ten.teb.2013.0204
Zhang, Y., Proenca, R., Maffei, M., Barone, M., Leopold, L., and Friedman, J. M. (1994). Positional Cloning of the Mouse Obese Gene and its Human Homologue. Nature 372, 425–432. doi:10.1038/372425a0
Zhu, M., Dong, Z., Gao, J., Liao, Y., Xue, J., Yuan, Y., et al. (2015). Adipocyte Regeneration after Free Fat Transplantation: Promotion by Stromal Vascular Fraction Cells. Cell Transpl. 24, 49–62. doi:10.3727/096368913x675133
Zhu, M., Liu, Y., Qin, H., Tong, S., Sun, Q., Wang, T., et al. (2021). Osteogenically-induced Exosomes Stimulate Osteogenesis of Human Adipose-Derived Stem Cells. Cell Tissue Bank 22, 77–91. doi:10.1007/s10561-020-09867-8
Keywords: adipose-derived stem cells (ASCs), regenerative medicine, tissue engineering, scaffolds, microfluidic systems
Citation: Al-Ghadban S, Artiles M and Bunnell BA (2022) Adipose Stem Cells in Regenerative Medicine: Looking Forward. Front. Bioeng. Biotechnol. 9:837464. doi: 10.3389/fbioe.2021.837464
Received: 16 December 2021; Accepted: 27 December 2021;
Published: 13 January 2022.
Edited by:
Ranieri Cancedda, Independent researcher, Genova, ItalyReviewed by:
Roberta Tasso, University of Genoa, ItalyCopyright © 2022 Al-Ghadban, Artiles and Bunnell. This is an open-access article distributed under the terms of the Creative Commons Attribution License (CC BY). The use, distribution or reproduction in other forums is permitted, provided the original author(s) and the copyright owner(s) are credited and that the original publication in this journal is cited, in accordance with accepted academic practice. No use, distribution or reproduction is permitted which does not comply with these terms.
*Correspondence: Bruce A. Bunnell, YnJ1Y2UuYnVubmVsbEB1bnRoc2MuZWR1
Disclaimer: All claims expressed in this article are solely those of the authors and do not necessarily represent those of their affiliated organizations, or those of the publisher, the editors and the reviewers. Any product that may be evaluated in this article or claim that may be made by its manufacturer is not guaranteed or endorsed by the publisher.
Research integrity at Frontiers
Learn more about the work of our research integrity team to safeguard the quality of each article we publish.