- Department of Neurosurgery, The First Hospital of Jilin University, Changchun, China
Although the treatment modalities of cancers are developing rapidly, chemotherapy is still the primary treatment strategy for most solid cancers. The progress in nanotechnology provides an opportunity to upregulate the tumor suppression efficacy and decreases the systemic toxicities. As a promising nanoplatform, the polymer micelles are fascinating nanocarriers for the encapsulation and delivery of chemotherapeutic agents. The chemical and physical properties of amphiphilic co-polymers could significantly regulate the performances of the micellar self-assembly and affect the behaviors of controlled release of drugs. Herein, two amphiphilic Y-shaped polypeptides are prepared by the ring-opening polymerization of cyclic monomer l-leucine N-carboxyanhydride (l-Leu NCA) initiated by a dual-amino-ended macroinitiator poly(ethylene glycol) [mPEG-(NH2)2]. The block co-polypeptides with PLeu8 and PLeu16 segments could form spontaneously into micelles in an aqueous solution with hydrodynamic radii of 80.0 ± 6.0 and 69.1 ± 4.8 nm, respectively. The developed doxorubicin (DOX)-loaded micelles could release the payload in a sustained pattern and inhibit the growth of xenografted human HepG2 hepatocellular carcinoma with decreased systemic toxicity. The results demonstrated the great potential of polypeptide micellar formulations in cancer therapy clinically.
Introduction
Chemotherapy is still an essential modality for the treatments of most solid cancers, although a variety of emerging strategies have been developed in the past few decades (Neoptolemos et al., 2018; Bukowski et al., 2020; Lu et al., 2020; Zheng et al., 2020; Zhao et al., 2021). However, the low water solubility, instability, short circulation period, and poor selectivity to tumor tissue of the mainstream small-molecule chemotherapeutic drugs in the clinic restrict the applicable diseases, reduce the anticancer efficacy, and even induce severe side effects (Steinbrueck et al., 2020; Zheng et al., 2021). The development of nanotechnology and the preparation of various organic and inorganic nanoparticles provide a robust tool for the controlled delivery of small-molecule chemotherapeutic drugs (Jiang et al., 2019; Sun et al., 2019; Jiang et al., 2020; Kim et al., 2020; Thomas et al., 2020; Zhang et al., 2020). Among them, the micelles self-assembled from the amphiphilic polymers are attracting increasing attention in the controlled delivery of chemotherapeutic drugs because of their controlled sizes, morphologies, stability, stimulus responsiveness, high drug loading efficiency, and targeted drug delivery behaviors (Zeinali et al., 2020; Bai et al., 2021; Rajes et al., 2021; Wei et al., 2021).
The chemical structures and physical properties of amphiphilic polymers could always significantly regulate the behavior of self-assembly and the performances of obtained micelles (Danafar et al., 2018; Saravanakumar et al., 2018; Brega et al., 2019). The length of hydrophilic and hydrophobic blocks and their ratio are the main factors regulating the sizes, shape, and stability of the polymer micelles and even significantly influence their drug loading properties (Li et al., 2013; Zhang et al., 2013). Typically, longer hydrophobic polymer moieties correspond to smaller sizes, more compact cores, upregulated drug loading capability, and more constant drug release (Hussein and Youssry, 2018).
In addition, the topology of polymers is another critical factor for changing the behavior of self-assembly of polymers and properties of micelles (Li et al., 2013; Yang et al., 2017). The nonlinear amphiphilic polymers, including the graft, Y-shaped, dumbbell-shaped, and even star ones, always induce more stable self-assembled micellar nanoparticles compared with the linear amphiphilic polymers with the same components (Ding et al., 2011; Li et al., 2012; Li et al., 2013; Yang et al., 2021). Therefore, the micelles based on the nonlinear amphiphilic polymers exhibited more promising applications in controlled drug delivery for cancer therapy (Shi et al., 2018; Perin et al., 2021).
In this study, a kind of Y-shaped amphiphilic block co-polymers of methoxy poly(ethylene glycol) (mPEG) and poly(l-leucine) (PLeu) was developed to form spontaneously into micelles and deliver chemotherapeutic agent doxorubicin (DOX) in a controlled manner. PLeu was used as a typical polypeptide segment in this study, and other hydrophobic polypeptides, such as polyglycine, polyvaline, and polyphenylalanine, could also be used as components of amphiphilic block co-polymers for effective drug loading and controlled release. The block co-polypeptides were prepared by the ring-opening polymerization (ROP) of l-leucine N-carboxyanhydride (l-Leu NCA) initiated by a dual-amino-ended macroinitiator poly(ethylene glycol) [mPEG-(NH2)2] (Wang et al., 2019b; Song et al., 2019; Liu et al., 2020). The co-polymer could form into spherical micellar nanoparticles, which exhibited excellent drug encapsulation and release behaviors. The loaded polypeptide micelles showed fascinating tumor growth inhibition efficacy, indicating their great potential for anticancer application clinically (Deng et al., 2020; Zheng et al., 2021).
Materials and Methods
The materials, synthesis of methoxy poly(ethylene glycol)-(poly (l-leucine))2; characterizations, preparation, and characterization of DOX-loaded co-polypeptide micelles; and DOX release in vitro are described in the Supplementary Material in detail.
In Vitro Biocompatibility Toward L929 Cells and Cytotoxicity Toward HepG2 Cells
The biocompatibility of mPEG-(PLeu)2 against mouse fibroblast L929 cells and the cytotoxicity of mPEG-(PLeu)2/DOX toward human hepatocellular carcinoma HepG2 cells were assessed by a typical methyl thiazolyl tetrazolium (MTT) technique in vitro. The selected cells were inoculated into tissue culture plates (TCPs) with 96 wells, with 7,000 cells in a well and dispersed in 200.0 μl of Dulbecco’s modified Eagle’s medium (DMEM) with fetal bovine serum (FBS) and antibiotics. At 24 h post-inoculation, the original incubation DMEM was removed, and the solutions of mPEG-(PLeu)2 micelles at a concentration from 1.6 to 100.0 μg ml−1 or mPEG-(PLeu)2/DOX at a concentration from 0.16 to 10.0 μg ml−1 in DMEM was supplemented. In 72 h post-co-incubation, the cell viability was determined by an MTT technique. The absorbances of the MTT co-incubated cell solutions in dimethyl sulfoxide (DMSO) were tested at 490 nm on a microplate reader (Bio-Rad 680, Bio-Rad Laboratories, Hercules, CA, USA). The cell viability is assessed in Eq. 1.
In Eq. 1, ASample and AControl represent the absorbances of the corresponding groups.
In Vivo Tumor Suppression
The BALB/c nude mice (female, 6 weeks old) were bought from Beijing Vital River Laboratory Animal Technology Co., Ltd. (Beijing, China). The suppression efficacy of tumor growth by various DOX formulations was evaluated toward the human HepG2 hepatocellular carcinoma-xenografted BALB/c node mice (Zhao et al., 2013; Wang et al., 2019a). The tumor-bearing mouse model was prepared by injecting subcutaneously 1.0 × 106 HepG2 cells dispersed with 100.0 μl of 0.01 M of phosphate-buffered saline (PBS) to the right anterior limb’s armpit in BALB/c nude mice (5 weeks old, ∼25 g). When the tumor volume reached about 72 mm3, the mice were stochastically separated into four groups (n = 6). The control of PBS or various formulations of model chemotherapeutic agent DOX, including free DOX, mPEG-(PLeu8)2/DOX, or mPEG-(PLeu16)2/DOX, were administrated by the tail-vein injection to treat the tumor-bearing mice. The equivalent DOX dosage was set as 5.0 mg for every kg body weight [mg (kg BW)−1]. The chemotherapy was given every 4 days. The tumor volume was assessed every 2 days, and the body weight was detected at the same frequency.
The tumor volume could be assessed according to Eq. 2.
The tumor growth rate was calculated according to Eq. 3.
Results and Discussion
Synthesis and Characterizations of Methoxy Poly(ethylene glycol)–(Poly(l-leucine))2
Polypeptides could be prepared by the ROP of amino acid NCA monomers in a controlled manner (Liu et al., 2020; Lv et al., 2020; Vrijsen et al., 2020). In this study, the Y-shaped co-polypeptide mPEG-(PLeu)2 was prepared by the ROP of l-Leu NCA with the dual-amino-ended mPEG-(NH2)2 as a macroinitiator, as shown in Schemes 1 and 2. The successful synthesis of macroinitiator mPEG-(NH2)2 and the co-polypeptide mPEG-(PLeu)2 was demonstrated through the spectrum results of 1H NMR and Fourier transform IR (FT-IR). As demonstrated by the results of Figure 1, the appearance of the peaks at 4.45 ppm is attributed to the backbone proton (d, –NHCHC(O)–(CH2–)), 1.41 ppm is attributed to the methylene protons in the side segment (e, –CH2CH(CH3)2), and 0.71 ppm belonged to the protons of side methyl groups (f, –CH2CH(CH3)2), which proved the successful preparation of mPEG-(PLeu)2. The degrees of polymerization (DPs) of PLeu segments were detected to be 8 and 16 based on the area ratios between the resonance peak at 1.41 ppm and that at 3.64 ppm assigned to the protons in methylene segment in the backbone of mPEG (b, –CH2CH2O–). Moreover, the successful preparation of mPEG-(PLeu)2 was further confirmed by the results of FT-IR, as depicted in Figure 2. The wavenumbers at 1,543 and 1,663 cm−1 should be attributed to the stretching vibration of C(O)–NH (νC(O)–NH) and C═O (νC═O), respectively, revealing the formation of the amide bond and the synthesis of PLeu. Moreover, the stretching vibration signal of C–O–C (νC–O–C) demonstrated the appearance of mPEG in the co-polypeptides. Therefore, the results proved the successful synthesis of mPEG-(PLeu8)2 and mPEG-(PLeu16)2.
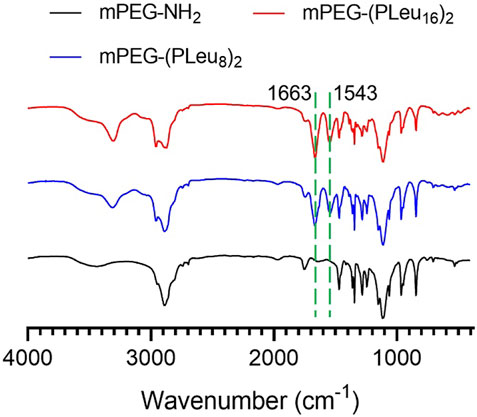
FIGURE 2. FT-IR spectra of mPEG-(NH2)2, mPEG-(PLeu8)2, and mPEG-(PLeu16)2. FT-IR, Fourier transform IR.
Preparation and Detection of Doxorubicin-Loaded Co-Polypeptide Micelles In Vitro
The micelles from various amphiphilic polymers or co-polymers could be effective nanocarriers for the controlled delivery of anticancer drugs (Ray et al., 2018; Pinyakit et al., 2020). In this work, the chemotherapeutic agent DOX was encapsulated to the cores of micelles of mPEG-(PLeu8)2 and mPEG-(PLeu16)2 through nanoprecipitation. The contents of DOX in the micelles were tested by high-performance liquid chromatography (HPLC), and the drug-loading content (DLC) of mPEG-(PLeu8)2, and mPEG-(PLeu16)2 micelles were calculated to be 8.75 and 12.94 wt%. The drug-loading efficiency (DLE) of the above micelles were assessed to be 47.95 and 74.32 wt%, respectively. The higher DLC and DLE of mPEG-(PLeu16)2 micelle resulted from the more hydrophobic property of a longer PLeu block (Chen et al., 2018; Lübtow et al., 2019). As shown in Figure 3, the hydrophobic radii (Rhs) of DOX-loaded co-polypeptide micelles mPEG-(PLeu8)2/DOX and mPEG-(PLeu16)2/DOX were detected to be 80.0 ± 6.0 and 69.1 ± 4.8 nm, respectively. The smaller size of mPEG-(PLeu16)2/DOX also should be assigned to the more hydrophobic performance of PLeu16 (Hussein and Youssry, 2018).
The release behaviors of micelles always affected the therapeutic efficacy of loaded micelles (Wang et al., 2019c). The sustained drug release profiles of mPEG-(PLeu8)2/DOX and mPEG-(PLeu16)2/DOX were detected. As depicted in Figure 4, mPEG-(PLeu8)2/DOX released 58.88% of loaded DOX in the detected 60 h, while mPEG-(PLeu16)2/DOX released 35.89%. The more compact and hydrophobic core of the micelle always induces a higher drug loading capability and more sustained drug release behavior.
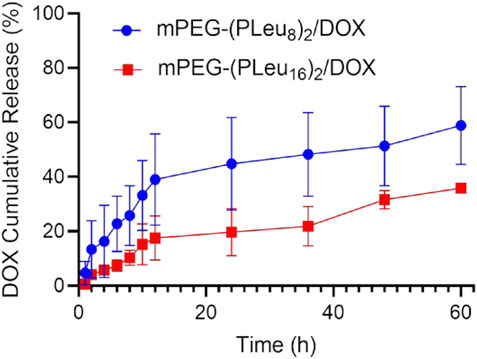
FIGURE 4. Cumulative DOX release from mPEG-(PLeu8)2/DOX and mPEG-(PLeu16)2/DOX in release medium of PBS at pH 7.4. DOX, doxorubicin; PBS, phosphate-buffered saline.
The biocompatibility of materials is a critical factor for biomedical applications (Ding et al., 2013; Abbina et al., 2017; Reina et al., 2017). This study detected the biocompatibility of the prepared co-polypeptide micelles of mPEG-(PLeu8)2 and mPEG-(PLeu16)2 by an MTT protocol toward L929 cells. As depicted in Figure 5, both the micelles showed excellent biocompatibility at the concentration of 100.0 μg ml−1, which was revealed by the high cell viability at above 80%.
An MTT technique detected the antitumor efficacy of various DOX formulations toward HepG2 cells in vitro. As demonstrated by Figure 6, free DOX showed the best inhibition efficiency of cell proliferation as a benefit of its fastest cell entry speed through dispersion. The slightly weaker anticancer effect of DOX-loaded co-polypeptide micelles could be assigned to the slower endocytosis speed by HepG2 cells. In addition, the better anticancer effect of mPEG-(PLeu16)2 should be attributed to the more sustained release profile of the chemotherapeutic agent in the cells.
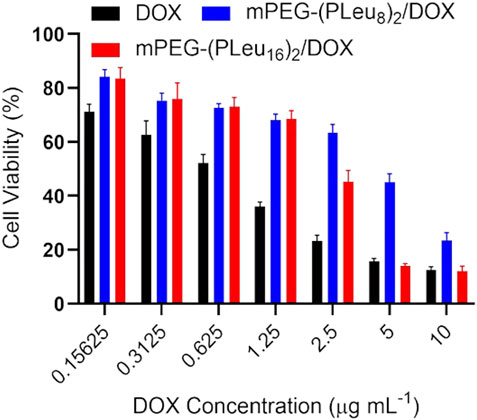
FIGURE 6. Cell proliferation inhibition efficacy of free DOX, mPEG-(PLeu8)2/DOX, and mPEG-(PLeu16)2/DOX toward HepG2 cells. DOX, doxorubicin.
Anticancer Efficacy of Doxorubicin-Loaded Co-Polypeptide Micelles In Vivo
The excellent anticancer efficacy of nanoformulations of chemotherapeutic drugs is one of the essential properties for potential clinical application (Ghosh et al., 2021; Zheng et al., 2021). Herein, the anticancer efficacy of PBS as control, free DOX, mPEG-(PLeu8)2/DOX, and mPEG-(PLeu16)2/DOX were detected toward human HepG2 hepatocellular carcinoma-xenografted BALB/c node murine model.
The tumor-bearing mice were constructed by inoculating 1.0 × 106 HepG2 cells dispersed in 100.0 μl of PBS into the right anterior limb’s armpit in a BALB/c nude mouse. As the tumor grew to ∼72 mm3 in volume, the model animals were divided into four groups (n = 6). On days 1, 4, and 8, the human HepG2 hepatocellular carcinoma-xenografted mice were treated with PBS as control, free DOX, mPEG-(PLeu8)2/DOX, or mPEG-(PLeu16)2/DOX three times. The dosage of DOX was set at 5.0 mg (kg BW)−1. The tumor volume was monitored every other day. As shown in Figure 7, the tumor growth rates calculated based on the data of tumor volumes decreased to some extent in the groups of mPEG-(PLeu8)2/DOX and mPEG-(PLeu16)2/DOX, while it increased to about 3.96 and 1.72 in the PBS as control and free DOX groups, respectively. The best anticancer efficacy should be assigned to the target release of DOX by the micelles of Y-shaped co-polypeptides.
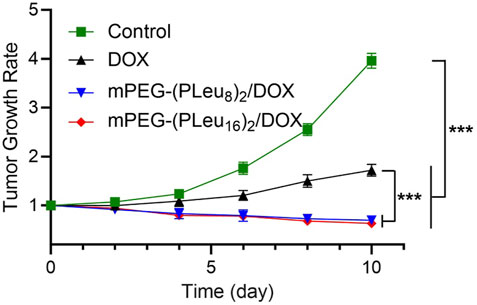
FIGURE 7. Tumor growth rate of human HepG2 hepatocellular carcinoma-xenografted BALB/c node mice during treatment with PBS as control, free DOX, mPEG-(PLeu8)2/DOX, or mPEG-(PLeu16)2/DOX. The data are represented as mean ± SD (n = 6; ***p < 0.001). PBS, phosphate-buffered saline; DOX, doxorubicin.
The safety of nanoformulations of chemotherapeutic drugs is another critical factor influencing their potential application in a clinic (Zheng et al., 2021). In this work, the safety of free DOX and DOX-loaded Y-shaped co-polypeptide micelles was revealed by the change of body weight of human HepG2 hepatocellular carcinoma-xenografted mice in the process of treatment. As shown in Figure 8, the body weight decreased significantly after the treatment with free DOX, indicating the toxicity of free DOX in vivo. Fortunately, the body weight of mPEG-(PLeu8)2/DOX and mPEG-(PLeu16)2/DOX groups was similar to the body weight of the control group, demonstrating their excellent safety in vivo. Therefore, the findings confirmed that the Y-shaped polypeptide micelles exhibited great potential for controlled delivery of chemotherapeutic drugs in vivo, which benefited the improved anticancer efficacy and reduced systemic toxicity.
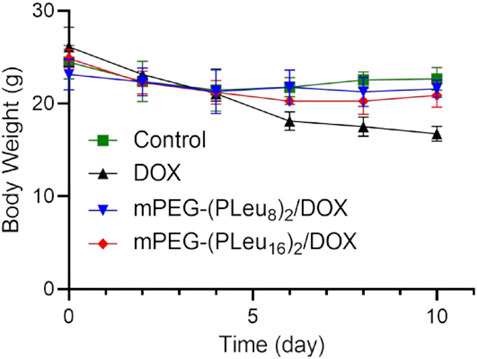
FIGURE 8. Body weight change of human HepG2 hepatocellular carcinoma-xenografted BALB/c node mouse model during treatment with PBS as control, free DOX, mPEG-(PLeu8)2/DOX, or mPEG-(PLeu16)2/DOX. PBS, phosphate-buffered saline; DOX, doxorubicin.
Conclusion
Chemotherapy is still the primary treatment modality for most solid tumors. To overcome the disadvantages of small-molecule drugs, including low water solubility, short circulation time, and low targeting in vivo, a variety of nanocarriers were developed. In this study, two Y-shaped co-polypeptides were prepared by the ROP of l-Leu NCA. mPEG-(PLeu)2 could self-assemble into micelle and effectively encapsulate and release the model chemotherapeutic drug DOX in a sustained manner. The DOX-loaded Y-shaped co-polypeptide micelles could significantly suppress the growth of HepG2 hepatocellular carcinoma in vitro and in vivo with reduced systemic toxicity. The results indicated the great potential of the micelles from nonlinear amphiphilic polymers or co-polymers in cancer therapy clinically.
In this study, the human HepG2 hepatocellular carcinoma and DOX are just models to characterize and confirm the advantages of micelles from nonlinear amphiphilic polymers or co-polymers. The developed advanced micelles could be used for the controlled delivery of other therapeutic agents to treat different kinds of cancers and even other diseases.
Data Availability Statement
The original contributions presented in the study are included in the article/Supplementary Material. Further inquiries can be directed to the corresponding author.
Ethics Statement
The animal study was reviewed and approved by the Institutional Animal Care and Use Committee of Jilin University.
Author Contributions
CH provided the idea of this study and supersized the experiment implementation and data analysis. CH, YZ, and YL designed and performed all the experiments and analyzed all the obtained results. CH and YZ prepared the draft article, and all authors carefully revised it. All authors have read and agreed to the published version of the article.
Conflict of Interest
The authors declare that the research was conducted in the absence of any commercial or financial relationships that could be construed as a potential conflict of interest.
Publisher’s Note
All claims expressed in this article are solely those of the authors and do not necessarily represent those of their affiliated organizations, or those of the publisher, the editors, and the reviewers. Any product that may be evaluated in this article, or claim that may be made by its manufacturer, is not guaranteed or endorsed by the publisher.
Acknowledgments
The authors appreciated the assistance from Changchun Institute of Applied Chemistry, Chinese Academy of Sciences, Changchun, P. R. China, for the polymer synthesis and characterizations.
Supplementary Material
The Supplementary Material for this article can be found online at: https://www.frontiersin.org/articles/10.3389/fbioe.2021.817143/full#supplementary-material
References
Abbina, S., Vappala, S., Kumar, P., Siren, E. M. J., La, C. C., Abbasi, U., et al. (2017). Hyperbranched Polyglycerols: Recent Advances in Synthesis, Biocompatibility and Biomedical Applications. J. Mater. Chem. B 5, 9249–9277. doi:10.1039/c7tb02515g
Bai, S., Jia, D., Ma, X., Liang, M., Xue, P., Kang, Y., et al. (2021). Cylindrical Polymer Brushes-Anisotropic Unimolecular Micelle Drug Delivery System for Enhancing the Effectiveness of Chemotherapy. Bioactive Mater. 6, 2894–2904. doi:10.1016/j.bioactmat.2021.02.011
Brega, V., Scaletti, F., Zhang, X., Wang, L.-S., Li, P., Xu, Q., et al. (2019). Polymer Amphiphiles for Photoregulated Anticancer Drug Delivery. ACS Appl. Mater. Inter. 11, 2814–2820. doi:10.1021/acsami.8b18099
Bukowski, K., Kciuk, M., and Kontek, R. (2020). Mechanisms of Multidrug Resistance in Cancer Chemotherapy. Ijms 21, 3233. doi:10.3390/ijms21093233
Chen, W., Zhou, S., Ge, L., Wu, W., and Jiang, X. (2018). Translatable High Drug Loading Drug Delivery Systems Based on Biocompatible Polymer Nanocarriers. Biomacromolecules 19, 1732–1745. doi:10.1021/acs.biomac.8b00218
Danafar, H., Rostamizadeh, K., and Hamidi, M. (2018). Polylactide/poly(ethylene Glycol)/polylactide Triblock Copolymer Micelles as Carrier for Delivery of Hydrophilic and Hydrophobic Drugs: a Comparison Study. J. Pharm. Investig. 48, 381–391. doi:10.1007/s40005-017-0334-8
Deng, C., Zhang, Q., Guo, J., Zhao, X., and Zhong, Z. (2020). Robust and Smart Polypeptide-Based Nanomedicines for Targeted Tumor Therapy. Adv. Drug Deliv. Rev. 160, 199–211. doi:10.1016/j.addr.2020.10.019
Ding, J., Chen, J., Li, D., Xiao, C., Zhang, J., He, C., et al. (2013). Biocompatible Reduction-Responsive Polypeptide Micelles as Nanocarriers for Enhanced Chemotherapy Efficacy In Vitro. J. Mater. Chem. B 1, 69–81. doi:10.1039/c2tb00063f
Ding, J., Shi, F., Xiao, C., Lin, L., Chen, L., He, C., et al. (2011). One-step Preparation of Reduction-Responsive Poly(ethylene Glycol)-Poly(amino Acid)s Nanogels as Efficient Intracellular Drug Delivery Platforms. Polym. Chem. 2, 2857–2864. doi:10.1039/c1py00360g
Ghosh, S., Javia, A., Shetty, S., Bardoliwala, D., Maiti, K., Banerjee, S., et al. (2021). Triple Negative Breast Cancer and Non-small Cell Lung Cancer: Clinical Challenges and Nano-Formulation Approaches. J. Controlled Release 337, 27–58. doi:10.1016/j.jconrel.2021.07.014
Hussein, Y. H. A., and Youssry, M. (2018). Polymeric Micelles of Biodegradable Diblock Copolymers: Enhanced Encapsulation of Hydrophobic Drugs. Materials (Basel) 11, 688. doi:10.3390/ma11050688
Jiang, Z.-Y., Feng, X.-R., Xu, W.-G., Zhuang, X.-L., Ding, J.-X., and Chen, X.-S. (2020). Calcium Phosphate-Cured Nanocluster of Poly(L-Glutamic Acid)-Cisplatin and Arsenic Trioxide for Synergistic Chemotherapy of Peritoneal Metastasis of Ovarian Cancer. Acta Polymerica Sinica 51, 901–910. doi:10.11777/j.issn1000-3304.2020.20053
Jiang, Z., Liu, Y., Feng, X., and Ding, J. (2019). Functional Polypeptide Nanogels. J. Funct. Polym. 32, 13–27. doi:10.14133/j.cnki.1008-9357.20180612001
Kim, S., Im, S., Park, E.-Y., Lee, J., Kim, C., Kim, T.-I., et al. (2020). Biology, and MedicineDrug-Loaded Titanium Dioxide Nanoparticle Coated with Tumor Targeting Polymer as a Sonodynamic Chemotherapeutic Agent for Anti-cancer Therapy. Nanomedicine: Nanotechnology, Biol. Med. 24, 102110. doi:10.1016/j.nano.2019.102110
Li, D., Ding, J. X., Tang, Z. H., Sun, H., Zhuang, X. L., Xu, J. Z., et al. (2012). In Vitro evaluation of Anticancer Nanomedicines Based on Doxorubicin and Amphiphilic Y-Shaped Copolymers. Int. J. Nanomedicine 7, 2687–2697. doi:10.2147/IJN.S30687
Li, D., Sun, H., Ding, J., Tang, Z., Zhang, Y., Xu, W., et al. (2013). Polymeric Topology and Composition Constrained Polyether-Polyester Micelles for Directional Antitumor Drug Delivery. Acta Biomater. 9, 8875–8884. doi:10.1016/j.actbio.2013.06.041
Liu, Y., Li, D., Ding, J., and Chen, X. (2020). Controlled Synthesis of Polypeptides. Chin. Chem. Lett. 31, 3001–3014. doi:10.1016/j.cclet.2020.04.029
Lu, Z., Peng, Z., Liu, C., Wang, Z., Wang, Y., Jiao, X., et al. (2020). Current Status and Future Perspective of Immunotherapy in Gastrointestinal Cancers. The Innovation 1, 100041. doi:10.1016/j.xinn.2020.100041
Lübtow, M. M., Haider, M. S., Kirsch, M., Klisch, S., and Luxenhofer, R. (2019). Like Dissolves like? A Comprehensive Evaluation of Partial Solubility Parameters to Predict Polymer-Drug Compatibility in Ultrahigh Drug-Loaded Polymer Micelles. Biomacromolecules 20, 3041–3056. doi:10.1021/acs.biomac.9b00618
Lv, S., Kim, H., Song, Z., Feng, L., Yang, Y., Baumgartner, R., et al. (2020). Unimolecular Polypeptide Micelles via Ultrafast Polymerization of N-Carboxyanhydrides. J. Am. Chem. Soc. 142, 8570–8574. doi:10.1021/jacs.0c01173
Neoptolemos, J. P., Kleeff, J., Michl, P., Costello, E., Greenhalf, W., and Palmer, D. H. (2018). Therapeutic Developments in Pancreatic Cancer: Current and Future Perspectives. Nat. Rev. Gastroenterol. Hepatol. 15, 333–348. doi:10.1038/s41575-018-0005-x
Perin, F., Motta, A., and Maniglio, D. (2021). Amphiphilic Copolymers in Biomedical Applications: Synthesis Routes and Property Control. Mater. Sci. Eng. C 123, 111952. doi:10.1016/j.msec.2021.111952
Pinyakit, Y., Palaga, T., Kiatkamjornwong, S., and Hoven, V. P. (2020). Sequential post-polymerization Modification of a Pentafluorophenyl Ester-Containing Homopolymer: a Convenient Route to Effective pH-Responsive Nanocarriers for Anticancer Drugs. J. Mater. Chem. B 8, 454–464. doi:10.1039/c9tb01533g
Rajes, K., Walker, K. A., Hadam, S., Zabihi, F., Ibrahim-Bacha, J., Germer, G., et al. (2021). Oxidation-Sensitive Core-Multishell Nanocarriers for the Controlled Delivery of Hydrophobic Drugs. ACS Biomater. Sci. Eng. 7, 2485–2495. doi:10.1021/acsbiomaterials.0c01771
Ray, S., Li, Z., Hsu, C.-H., Hwang, L.-P., Lin, Y.-C., Chou, P.-T., et al. (2018). Dendrimer- and Copolymer-Based Nanoparticles for Magnetic Resonance Cancer Theranostics. Theranostics 8, 6322–6349. doi:10.7150/thno.27828
Reina, G., González-Domínguez, J. M., Criado, A., Vázquez, E., Bianco, A., and Prato, M. (2017). Promises, Facts and Challenges for Graphene in Biomedical Applications. Chem. Soc. Rev. 46, 4400–4416. doi:10.1039/c7cs00363c
Saravanakumar, G., Park, H., Kim, J., Park, D., Pramanick, S., Kim, D. H., et al. (2018). Miktoarm Amphiphilic Block Copolymer with Singlet Oxygen-Labile Stereospecific β-Aminoacrylate Junction: Synthesis, Self-Assembly, and Photodynamically Triggered Drug Release. Biomacromolecules 19, 2202–2213. doi:10.1021/acs.biomac.8b00290
Shi, X., Bai, S., Yang, C., Ma, X., Hou, M., Chen, J., et al. (2018). Improving the Carrier Stability and Drug Loading of Unimolecular Micelle-Based Nanotherapeutics for Acid-Activated Drug Delivery and Enhanced Antitumor Therapy. J. Mater. Chem. B 6, 5549–5561. doi:10.1039/c8tb01384e
Song, Z., Tan, Z., and Cheng, J. (2019). Recent Advances and Future Perspectives of Synthetic Polypeptides from N-Carboxyanhydrides. Macromolecules 52, 8521–8539. doi:10.1021/acs.macromol.9b01450
Steinbrueck, A., Sedgwick, A. C., Brewster, J. T., Yan, K.-C., Shang, Y., Knoll, D. M., et al. (2020). Transition Metal Chelators, Pro-chelators, and Ionophores as Small Molecule Cancer Chemotherapeutic Agents. Chem. Soc. Rev. 49, 3726–3747. doi:10.1039/c9cs00373h
Sun, Y., Shan, H., Wang, J., Wang, X., Yang, X., and Ding, J. (2019). Laden Nanofiber Capsules for Local Malignancy Chemotherapy. J Biomed. Nanotechnol 15, 939–950. doi:10.1166/jbn.2019.2745
Thomas, R. G., Surendran, S. P., and Jeong, Y. Y. (2020). Tumor Microenvironment-Stimuli Responsive Nanoparticles for Anticancer Therapy. Front. Mol. Biosciences 7, 414. doi:10.3389/fmolb.2020.610533
Vrijsen, J. H., Rasines Mazo, A., Junkers, T., and Qiao, G. G. (2020). Accelerated Polypeptide Synthesis via N ‐Carboxyanhydride Ring Opening Polymerization in Continuous Flow. Macromol. Rapid Commun. 41, 2000071. doi:10.1002/marc.202000071
Wang, Q., Zhang, P., Li, Z., Feng, X., Lv, C., Zhang, H., et al. (2019a). Evaluation of Polymer Nanoformulations in Hepatoma Therapy by Established Rodent Models. Theranostics 9, 1426–1452. doi:10.7150/thno.31683
Wang, Y., Jiang, Z., Xu, W., Yang, Y., Zhuang, X., Ding, J., et al. (2019b). Chiral Polypeptide Thermogels Induce Controlled Inflammatory Response as Potential Immunoadjuvants. ACS Appl. Mater. Inter. 11, 8725–8730. doi:10.1021/acsami.9b01872
Wang, Y., Wang, X., Zhang, J., Wang, L., Ou, C., Shu, Y., et al. (2019c). Gambogic Acid-Encapsulated Polymeric Micelles Improved Therapeutic Effects on Pancreatic Cancer. Chin. Chem. Lett. 30, 885–888. doi:10.1016/j.cclet.2019.02.018
Wei, L., Chen, J., and Ding, J. (2021). Sequentially Stimuli-Responsive Anticancer Nanomedicines. Nanomedicine 16, 261–264. doi:10.2217/nnm-2021-0019
Yang, D.-P., Oo, M. N. N. L., Deen, G. R., Li, Z., and Loh, X. J. (2017). Nano-Star-Shaped Polymers for Drug Delivery Applications. Macromol. Rapid Commun. 38, 1700410. doi:10.1002/marc.201700410
Yang, J.-Z., Zou, H.-Y., Ding, J.-X., and Chen, X.-S. (2021). Controlled Synthesis and Biomedical Applications of Cystine-Based Polypeptide Nanomaterials. Acta Polymerica Sinica 52, 960–977. doi:10.11777/j.issn1000-3304.2021.21115
Zeinali, M., Abbaspour-Ravasjani, S., Ghorbani, M., Babazadeh, A., Soltanfam, T., Santos, A. C., et al. (2020). Nanovehicles for Co-delivery of Anticancer Agents. Drug Discov. Today 25 (8), 1416–1430. doi:10.1016/j.drudis.2020.06.027
Zhang, H., Dong, S., Li, Z., Feng, X., Xu, W., Tulinao, C. M. S., et al. (2020). Biointerface Engineering Nanoplatforms for Cancer-Targeted Drug Delivery. Asian J. Pharm. Sci. 15, 397–415. doi:10.1016/j.ajps.2019.11.004
Zhang, Z., Qu, Q., Li, J., and Zhou, S. (2013). The Effect of the Hydrophilic/Hydrophobic Ratio of Polymeric Micelles on Their Endocytosis Pathways into Cells. Macromol. Biosci. 13, 789–798. doi:10.1002/mabi.201300037
Zhao, D., Huang, X., Zhang, Z., Ding, J., Cui, Y., and Chen, X. (2021). Engineered Nanomedicines for Tumor Vasculature Blockade Therapy. Wiley Interdiscip. Rev. Nanomed Nanobiotechnol 13, e1691. doi:10.1002/wnan.1691
Zhao, L., Ding, J., Xiao, C., He, P., Tang, Z., and Chen, X. (2013). Phenylboronic Acid Functionalized Polypeptide Micelles for Glucose-Responsive Insulin Release at Physiological pH. J. Controlled Release 172, E110. doi:10.1016/j.jconrel.2013.08.267
Zheng, C., Li, M., and Ding, J. (2021). Challenges and Opportunities of Nanomedicines in Clinical Translation. BIO Integration 2, 57–60. doi:10.15212/bioi-2021-0016
Keywords: polypeptide, polymer topology, micelle, controlled drug delivery, cancer therapy
Citation: Hua C, Zhang Y and Liu Y (2021) Enhanced Anticancer Efficacy of Chemotherapy by Amphiphilic Y-Shaped Polypeptide Micelles. Front. Bioeng. Biotechnol. 9:817143. doi: 10.3389/fbioe.2021.817143
Received: 17 November 2021; Accepted: 30 November 2021;
Published: 31 December 2021.
Edited by:
Yuce Li, Sungkyunkwan University, South KoreaReviewed by:
Jun Yue, Sun Yat-sen University, ChinaRuogu Qi, Nanjing University of Chinese Medicine, China
Kewa Gao, University of California, Davis, United States
Chao Zhao, University of Alabama, United States
Copyright © 2021 Hua, Zhang and Liu. This is an open-access article distributed under the terms of the Creative Commons Attribution License (CC BY). The use, distribution or reproduction in other forums is permitted, provided the original author(s) and the copyright owner(s) are credited and that the original publication in this journal is cited, in accordance with accepted academic practice. No use, distribution or reproduction is permitted which does not comply with these terms.
*Correspondence: Cong Hua, aHVhY29uZ0BqbHUuZWR1LmNu