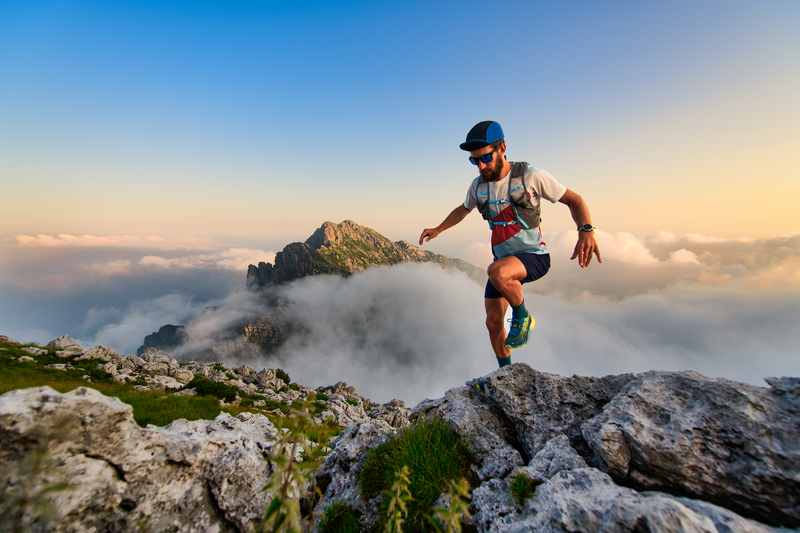
95% of researchers rate our articles as excellent or good
Learn more about the work of our research integrity team to safeguard the quality of each article we publish.
Find out more
ORIGINAL RESEARCH article
Front. Bioeng. Biotechnol. , 06 December 2021
Sec. Nanobiotechnology
Volume 9 - 2021 | https://doi.org/10.3389/fbioe.2021.799325
This article is part of the Research Topic Micro/Nano Optical Devices for Biosensing and Cellular Analysis View all 9 articles
A retraction of this article was approved in:
Retraction: Disposable Polymeric Nanostructured Plasmonic Biosensors for Cell Culture Adhesion Monitoring
Citation: Vila JC, Castro-Aguirre N, López-Muñoz GA, Ferret-Miñana A, De Chiara F and Ramón-Azcón J (2021) Disposable Polymeric Nanostructured Plasmonic Biosensors for Cell Culture Adhesion Monitoring. Front. Bioeng. Biotechnol. 9:799325. doi: 10.3389/fbioe.2021.799325
Received: 21 October 2021; Accepted: 15 November 2021;
Published: 06 December 2021; Retracted: 27 February 2025.
Edited by:
Tuhin Subhra Santra, Indian Institute of Technology Madras, IndiaReviewed by:
Arif Engin Cetin, Dokuz Eylul University, TurkeyDisclaimer: All claims expressed in this article are solely those of the authors and do not necessarily represent those of their affiliated organizations, or those of the publisher, the editors and the reviewers. Any product that may be evaluated in this article or claim that may be made by its manufacturer is not guaranteed or endorsed by the publisher.
Research integrity at Frontiers
Learn more about the work of our research integrity team to safeguard the quality of each article we publish.