- 1Faculty of Business and Economics, Mendel University in Brno, Brno, Czechia
- 2Smart Society Research Team, Faculty of Business and Economics, Mendel University in Brno, Brno, Czechia
- 3Department of Production Engineering, Warsaw University Of Life Sciences, Warsaw, Poland
Nanocellulose is a broader term used for nano-scaled cellulosic crystal and/or fibrils of plant or animal origin. Where bacterial nanocellulose was immediately accepted in biomedicine due to its “cleaner” nature, the plant-based nanocellulose has seen several roadblocks. This manuscript assesses the technological aspects (chemistry of cellulose, nanocellulose producing methods, its purity, and biological properties including toxicity and suggested applications in final drug formulation) along with legal aspects in REACH (Registration, Evaluation, Authorization, and Restriction of Chemicals) regulation by the European Union, EMA (European Medicine Agency). The botanical biomass processing methods leading to the nanoscale impurity (lignin and others) on nanocellulose surface, along with surface modification with harsh acid treatments are found to be two major sources of “impurity” in botanical biomass derived nanocellulose. The status of nanocellulose under the light of REACH regulation along with EMA has been covered. The provided information can be directly used by material and biomedical scientists while developing new nanocellulose production strategies as well as formulation design for European markets.
Introduction
Nanocellulose or nano-structured cellulose is general term used for cellulose fibers with diameters around 5–20 nm and varying degree of length (depending on source and processing parameters). Generally, nanocellulose includes three categories of cellulose, which are 1) Cellulose Nano Fibrils (CNF)/Nano Fibrillated Cellulose (NFC), 2) Cellulose Nano Crystals (CNC)/Cellulose Nano Whiskers (CNWs), and 3) Bacterial Nano Cellulose (nano structured cellulose of bacterial origin, BNC) (Klemm et al., 2011).
The classical approach to synthesize CNC is by acid hydrolysis (mostly sulfuric acid; phosphoric acid and hydrochloric acid are also reported) (Abitbol et al., 2016). Recently, two new methods have also been patented by the companies American Process Inc. (Nelson et al., 2014) and Blue Goose Biorefineries Inc. (Olkowski and Laarveld, 2013). These two methods were based on acid and solvent-based pretreatment to minimize mechanical energy consumption and transition metal-based nano-catalyst for biorefinery, respectively. CNF is mainly produced by mechanical processing assisted by enzymatic or chemical pretreatments. Mechanical methods to produce CNF include high pressure homogenization (HPH), microfluidization, grinding, cryocrushing, and high intensity ultrasonication (Abdul Khalil et al., 2014). HPH involves passing of cellulose slurry through a fine nozzle into a vessel. Size reduction takes place mainly due to shear forces generated at high pressure, velocity, and impact forces (Figure 1). First reports of the HPH process are as old as 1983 (Herrick et al., 1983). Microfluidizer is similar to HPH with an additional pump to generate high pressure streams. Interaction chamber here collides the streams and walls to create the fibrillating forces (Ferrer et al., 2012). Grinding involves passage of cellulose slurry between the static and mobile grinding stones, and motion of the stone provides the fibrillating force to get nanoscale fibers (Wang et al., 2012). In cryocrushing, water swollen cellulosic fibers frozen by liquid nitrogen are crushed using a mortar and pestle. The crushing impact forces and force exerted by ice crystals during the process create the liberating force for cellulose fibers from the cell wall of plant materials (Siró and Plackett, 2010). During high intensity ultrasonication, cavitation leads to powerful mechanical oscillating power involving formation, expansion, and implosion of microscopic gas bubbles due to absorption of ultrasonic energy by molecules (Chen et al., 2013).
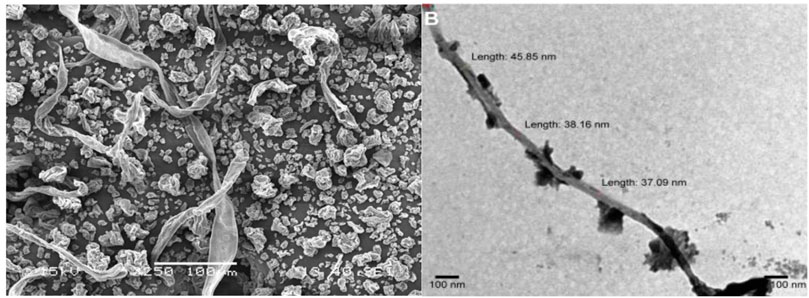
FIGURE 1. Electron microscopic structure of nanocellulose (Bhandari et al., 2017; Mishra et al., 2017, 2019).
Bacterial nanocellulose or biocellulose is the cellulose synthesized by the action of microorganisms. It is synthesized mainly by the bacterium Gluconacetobacter xylinus (also named as Acetobacter xylinus). Some other microorganisms also exhibit the ability to synthesize the cellulose, such as other species of Gluconacetobacter, Agrobacterium tumefaciens, Rhizobium spp., and Gram-positive Sarcina ventriculli (Tanskul et al., 2013; Mohammadkazemi et al., 2015; Jozala et al., 2016). G. xylinus is the primary microbe producing the biocellulose and commonly studied as a model system for the study of the biosynthetic mechanisms of cellulose synthesis. For the production of cellulose, G. xylinus secretes a nanofibrillar film with a denser lateral surface and a gelatinous layer on the opposite side (Kurosumi et al., 2009). The process of cellulose synthesis by G. xylinus can be explained in three steps: 1) polymerization of glucose residues, 2) extracellular secretion of linear chains, and 3) organization and crystallization of glucan chains (from Step 1) in a hierarchy into fibrils/strips (Klemm et al., 2011).
In regard of the established applications of the above three varieties of nanocelluloses, bacterial nanocellulose based biomedical products (wound care and wound healing) are already being marketed. Botanical-biomass based celluloses are mainly used in reinforcement and composite applications. There are several reasons for easy acceptability of bacterial nanocellulose in biomedical applications. First is its inherent purity. It is free of lignin, hemicelluloses, pectin, and other plant-based phenolic compounds (Chawla et al., 2009), and therefore it is simpler to purify as compared to botanical-NC. Second, bacterial-NC possesses a highly porous structure with high water absorption capacity that can help in absorbing exudates from wounds (Ullah et al., 2016). Finally, the lack of impurity provides a higher number of hydroxyl groups for surface modification, harmless degradation products (only glucose), negligible amount of endotoxin (approved by FDA for surgical sheets and tissue reinforcements), higher complement activation parameters as compared to alternates, slower blood-coagulation in comparison to clinically available materials, and lack of skin irritation potential (Fink et al., 2011; Petersen and Gatenholm, 2011; Almeida et al., 2014) and hence improved compatibility properties. On the other hand, the botanical-biomass based nanocellulose carry remnants of chemicals from processing steps, which can be explained as a main reason for their less acceptability in applications requiring a cleaner surface. Although new reports of botanical-biomass based nanocellulose drug delivery and wound care system are showing promising results, this area needs more reports and tests to reach a reasonably strong conclusion.
Chemistry, Crystallinity, and Biological Properties of Cellulose
Cellulose is a linear polymer of D-glucopyranose units linked by β-1,4-glycosidic bonds. At molecular levels, it is composed of carbon (44.44%), hydrogen (6.17%), and oxygen (49.39%). Cellulose can also be represented by a basic chemical formula of (C6H10O5)n; where n is called as the degree of polymerization (DP), attributed to the number of glucose groups, ranging from hundreds to thousands, varying from source to source and also affected by the method of processing for obtaining the cellulose. Traditionally, β-cellulose and γ-cellulose (Table 1) constitute industrial hemicellulose, and holocellulose refers to all the carbohydrates (cellulose and hemicellulose).

TABLE 1. Classification of cellulose based on DP values (Staudinger, 1933).
In the chemical structure of cellulose (Figure 2), the primary structure (linear chain and stereo-chemical structure) shows a cellobiose unit, reducing end (open ring structure), and non-reducing end (close ring structure). Within the linear chain, every glucose unit can be seen rotated by 180° and new glucose units are added at non-reducing ends deciding the direction of chain growth. The intramolecular hydrogen bonding can be seen in hydroxyl groups attached to the 2nd and 6th carbons, hydroxyl groups attached to the 3rd carbon, and adjacent oxygen from adjacent molecules. The intramolecular hydrogen bonds and van der Waal forces come into play during stacking of cellulose chains one above the other, between hydroxyl groups attached to the 3rd and 6th carbons of stacking chains. Surface characteristics of cellulose are most important for surface modifications, which in turn play a pivotal role in final product applications. Starting from the molecular level, each anhydro-glucopyranose unit does not lie in the plane of their structure but they assume a chair form (lowest energy conformational isomer) and each next molecule is rotated by 180° about the molecular axis with hydroxyl group at the equatorial position (Habibi et al., 2010). Each cellulose unit has three hydroxyl groups in cellulose, at the 2nd, 3rd, and 6th positions (Figure 2). At the 6th position, the hydroxyl group behaves as a primary carbon but at the 2nd and 3rd positions it acts as a secondary alcohol. Comparing the reactivity, the hydroxyl group at the 6th position shows 10 times more reactivity than the other two and the hydroxyl group at the 2nd position is twice as reactive as that at the 3rd position.
However, in the case of surface characteristics of nanocellulose, the chemical nature of the reactive agents and solvents also plays a major role in determination of reactivity. The reactivity of the hydroxyl group toward the nucleophilic attack was reported as almost the same for the 6th and 2nd carbon, which is more than reactivity of the hydroxyl group at the 3rd carbon (de la Motte et al., 2011; Lin and Dufresne, 2014).
In the specific case of CNC, it is produced by hydrolysis of an amorphous region of cellulose using sulfuric acid, leaving behind the crystalline part. This process results in negatively charged sulfate esters (condensation esterification/sulfation) on the CNC surface, which determines most of the surface characteristics of resultant material. As the surface charge is mainly due to sulphate esters, it is affected by duration and temperature of sulfation reaction (hydrolytic reaction).
Several methods based on XRD and 13C NMR spectra have been reported to study the crystallinity of cellulose. Based on XRD data, there can be a peak height method (Segal et al., 1959), peak deconvolution method (Hult et al., 2003; Garvey et al., 2005; He et al., 2008), and amorphous subtraction method (Ruland, 1961). In the NMR-based method used is C4 peak separation, the peak height method was based on a ratio of heights of 002 peak and minimum between 002 and 101 peak. This method worked well for comparative studies but had several limitations, too. Limitations include underestimation of a minimum due to its non-alignment with amorphous peak (Park et al., 2010); due to choice of only peak one out of at least four peaks, one specific orientation gets more attention than others; peaks in the cellulose spectrum are very wide and peak height cannot be used as an exact measure (Garvey et al., 2005). The amorphous subtraction method involves subtracting the amorphous spectra from diffraction pattern and crystallinity index (CI) is calculated as the ratio of crystalline area and total area. In this case, the challenge was to choose the right amorphous standard. Peak deconvolution method requires a software to separate different peaks. Four to five peaks have been separated in different cases. This method assumes an amorphous component as the main contributor to peak broadening, but other factors like crystalline size and non-uniform strain with the sample can also lead to the same results. Another aspect is that cellulose peaks are very broad and are resolved only with peak overlaps.
In the 13C NMR method, the ratio of the area under C4 peaks (assigned to crystalline cellulose at 89 ppm) and total areas of C4 peaks (assigned to amorphous cellulose at 84 ppm and crystalline peak both) are considered as CI values. Based on 13C CP/MASS NMR spectra reports in which C (4) and C (6) of cellulose show different signals (Horii et al., 1982), cellulose polymorphs can be divided into two groups: Cellulose (I, IIII, and IVI) and Cellulose (II, IIIII, and IVII). Cellulose I (the native cellulose) has cellulose chains arranged in such a way that glucopyranose rings are parallel to the “bc” plane of crystal (Gardner and Blackwell, 1974), hence exposing the glucopyranose ring from one crystal to another one. This property has been supported by evidence of binding enzymes on glucopyranose structures. The native cellulose (cellulose I) also shows two polymorphs called Iα and Iβ. The former is dominant in cellulose produced by primitive organisms whereas the latter dominates in higher plants. Iα has triclinic one chain unit cell in which chains are stacked by van der Waal forces and Iβ has monoclinic two chain unit cells stacking with alternating shear. The Iα gets converted into Iβ upon hydrothermal treatment and some solvents. Cellulose II is the result of alkali treatment of cellulose I. Cellulose II has cellulose chains rotated by 30° from the parallel to “ab” crystal phase (most applicable for 2 chain model but some cellulose requires 8 chain models). Additionally, intermolecular hydrogen bonding is significantly complex in crystalline parts of cellulose II as compared to cellulose I. The main difference lies in the configuration of the 6th carbon. For cellulose I this arrangement is trans-gauche (tg), whereas for cellulose II it is gauche-trans (gt) (Gardiner and Sarko, 1985). The additional feature of hydrogen bonding in cellulose II over cellulose I is due to intersheet bonding of C (2) hydroxyl group of corner chain and oxygen of the central chain, which is absent in native cellulose. Cellulose II has two chain monoclinic unit cells stacked by opposite polarity (antiparallel structure). Cellulose IIII and IIIII can be reversibly transformed into cellulose I and II, respectively, using ammonia. Cellulose IIII is a monoclinic one chain unit cell where parallel cellulose chains are stacked with no stagger along the chain axis. Cellulose IVI and IVII are possible transformed products of cellulose I and II, respectively. Although some reports suggest that direct transformation of cellulose I and II to IVI and IVII, respectively, does not happen in a step, there is possible formation of IIII and IIIII (Wada et al., 2004). In the diffraction study of microfibrils, alternate light and dark regions have been found, which are explained as crystalline and amorphous cellulose, respectively. Amorphous cellulose has been explained by various postulates, including isotropically distributed straight chains (Fink et al., 1987), and bent and twisted chains (Paakkari et al., 1989). Furthermore, it was also postulated that light and dark bands could be because of a slight curvature which makes in and out of Bragg’s diffraction conditions (Gautam et al., 2010). More details on cellulose crystallinity are beyond the scope of this article. Authors recommend an excellent work on this topic by Park et al. (2010).
Cellulose does not get digested in the human gut due to the absence of β-1-4 glycosidic bond cleaving enzymes, which is present in ruminating animals. This property of cellulose is used for its utilization as excipient in drug delivery formulations. In the environment, microorganism degrading cellulose (called cellulases) produce two types of cellulases, namely, endoglucanases and cellobiohydrolases (CBH). Endoglucanases hydrolyze internal bonds (preferably the amorphous regions) releasing new terminal ends. CBH (exo-1,4-b-glucanases) act on the existing or endoglucanase-generated chain ends. Amorphous cellulose can be degraded by both types of enzymes, but crystalline cellulose is efficiently degraded only by CBH. Both cellulases release cellobiose molecules as the result of hydrolytic cleavage. Also, breakdown of cellobiose requires β-glucosidases, which converts it into two molecules of glucose. Further hydrolysis of glucose results in carbon and energy sources for cellulolytic microorganisms (Pérez et al., 2002).
Cellulose is relatively stable to UV absorption and decomposition as compared to lignin (Mishra and Wimmer, 2017). For the toxicity studies, each type of cellulose needs to be considered individually as the method of production affects the surface characteristics significantly, which in turn plays a significant role in toxicity. For purified cellulose, the production steps result in trace organochlorine contamination originating from the chemical reactions in the purification process. However, the chronic ingestion did not show any increase in spontaneous disease or neoplasia. Additionally, any promotional activity in the mammary gland, colon, or bladder of the rats was not reported and any negative impact on the absorption or the metabolism of dietary components was not concluded. Therefore, no adverse health effects in humans were suggested from exposure to purified cellulose (Anderson et al., 1992). For bacterial cellulose produced by Gluconacetobacter xylinus, Jeong et al. reported a study of toxicity of bacterial cellulose nanofibers in human umbilical vein endothelial cells (HUVECs) using viability and flow cytometric assays, and in C57/Bl6 mice. The absence of toxicity in vitro and in vivo supported the view that bacterial cellulose may be used as a tissue engineering biomaterial (Jeong et al., 2010). In a study done on respirable cellulose fibers, short-term inhalation of cellulose caused an inflammatory lung response which resolved despite continuing exposure. Intraperitoneal injection of cellulose fibers induced sarcomas rather than mesotheliomas at the highest dose (109 fibers), while the two middle doses (107 and 108 fibers) each produced a mesothelioma (Cullen et al., 2002). In a review published on toxicity of CNC, it was mentioned that oral and dermal toxicity assessment of CNCs have shown a lack of adverse health effects, whereas studies on the pulmonary and cytotoxicity have yielded discordant results. Authors suggested the need for additional studies to support the general conclusion that CNCs are nontoxic on ingestion or contact with the skin and to determine whether CNCs have adverse health effects on inhalation or elicit inflammatory or oxidative stress responses at the cellular level.
Biomedical Applications of Botanical Nanocellulose
Nanocellulose has gained great popularity as a drug carrier in the past few years. Owing to its several favorable characteristics, including nanosize, high surface area, bioavailability, biocompatibility, and surface tunable chemistry, different drug delivery systems could be explored. There have been hydrogels, gels, microparticles, membranes, scaffolds, and films. Not only the diversity in drug delivery systems, but also nanocellulose possesses diversity in route of administration. There are oral, transdermal, mucoadhesive, and even injectable formulations too. Further, nanocellulose has been used to deliver drugs locally, as well as systemically (Salimi et al., 2019).
One of the main applications of nanocellulose in drug delivery is the controlled and sustained release of the active pharmaceutical ingredient. Reportedly, nanocellulose sustains the drug release by forming a tight fiber network around the incorporated drug entities (Kolakovic et al., 2012). Also, the hydrogen bonding that nanocellulose forms with various drugs improves the stability and cohesion of the biopolymer matrix leading to a sustained release (Guo et al., 2017). Apart from controlled release of drugs, nanocellulose has served other purposes too in drug delivery, such as targeting, improved stability, better bioavailability, and increased solubility (Table 2). Nanocellulose can even be made to carry an imaging agent inside the body and thus aid in diagnostics (Colombo et al., 2015; Zhou et al., 2015).
Another very common biomedical application of nanocellulose is in tissue engineering. The unique three-dimensional network formed by cellulose makes it an ideal candidate for a variety of tissue engineering applications. Other properties of nanocellulose, such as its mechanical strength and biocompatibility also add to its suitability for tissue engineering (Jorfi and Foster, 2015).
Tissue engineering is a novel field where cells, biomaterials, and growth factors are combined to produce engineered-organs and tissues for replacement of damaged tissues in the human body (Moroni et al., 2014). The required properties of scaffolds to be used for tissue engineering include good mechanical properties to sustain cell proliferation for more than 4 weeks, ability to support its differentiation into specialized structured tissues, and porous gel structure to allow gases transport and promote vascularization. The growth tissue, after sufficient development, is to be incorporated into the organism, expecting a minimal inflammatory response. Further, the scaffold should ideally degrade naturally into the body, without the need of any invasive procedure for its removal (Drury and Mooney, 2003). Nanocellulose fulfills all these requirements and thus is a suitable tissue engineering material (Curvello et al., 2019).
The most common type of tissue engineering application that nanocellulose is used for is wound healing. Chronic wounds pose an increasingly significant worldwide economic burden (over £1 billion per annum in the United Kingdom alone). With the escalation in global obesity and diabetes, chronic wounds will increasingly be a significant cause of morbidity and mortality (Jack et al., 2017). Wound dressing materials should have a porous network, ability to swell, a specific elasticity, and the ability to retain moisture and pH over time (Zhong et al., 2010). Since there always are high chances of infections, it is desired for dressings to also have antibacterial properties to minimize the bacterial growth during the healing process (Curvello et al., 2019). Nanocellulose has been extensively explored for scaffold and wound dressing applications. Nanocellulose is highly versatile and can be tailored with specific physical properties to produce an assortment of three-dimensional structures (hydrogels, aerogels, or films), for subsequent utilization as wound dressing material. It can also be loaded with antimicrobial agents to prevent infection in the wound (Kupnik et al., 2020).
After wound healing, bioprinting is another innovative tissue engineering strategy which nanocellulose is commonly used for. 3D bioprinting is emerging as a powerful tool for the construction of highly structured tissue engineering scaffolds. Through this technique, a 3D bioprinter is able to precisely dispense materials in three dimensions while moving in X, Y, and Z directions, enabling the production of complex structures from the bottom up (Markstedt et al., 2015). Cells are encapsulated within the printed material gel in homogeneous density and quantity. This feature makes 3D bioprinting better than the traditional two-step process where cells are inoculated into pre-made scaffolds, leading to heterogeneous cell distribution (Mandrycky et al., 2016).
It is often challenging to identify a bioink that supports cell growth, tissue maturation, and the successful formation of functional grafts to be used in regenerative medicine. In important research, a mitogenic hydrogel system based on alginate sulfate was identified as a bioink as it supports chondrocyte phenotype, but it was not printable due to its unfavorable rheological properties. To convert alginate sulfate into a printable bioink, the researchers combined it with nanocellulose, which has been shown to possess very good printability (Müller et al., 2017). The ability to spatially control the placement of cells, biomaterials, and biological molecules makes the nanocellulose a suitable tool for bioprinting (Martínez Ávila et al., 2016).
The important studies performed on biomedical applications of nanocellulose are summarized in Table 2.
Legal Aspects of Botanical Nanocellulose
EU Legislation
The free movement of goods, persons, services, and capital is part of the EU’s internal market. Free movement of chemicals is also related to the free movement of goods. Nanotechnologies and issues related to nanocellulose are of great importance and relevant legislation is also gaining importance. This gives rise to an obvious question, ‘how is the production and distribution of nanocellulose regulated and specifically what conditions must be satisfied in the case of nanocellulose from plants?’ The EU internal market could not function without regulation of chemical requirements. EU legislation in this area aims to ensure a high level of protection of human health and the environment (Rauscher et al., 2017). The protection of health and the environment is one of the prior areas of EU law, and EU legislation seeks to protect them.
EU legislation regulates registration of chemical substances. However, currently there is no specific EU legislation regulating nanocellulose, and therefore the general legislation of Regulation (EC) No 1907/2006 of the European Parliament and of the Council of 18 December 2006 concerning the Registration, Evaluation, Authorisation and Restriction of Chemicals (“REACH”) must be applied (Foth and Hayes, 2008; Kautto and Valve, 2019). It is therefore necessary to apply REACH to any handling of nanocellulose from plants. For REACH, the European Commission has repeatedly defined nanomaterials as a natural, incidental or manufactured material containing particles, in an unbound state or as an aggregate or as an agglomerate and where, for 50% or more of the particles in the number size distribution, one or more external dimensions is in the size range 1–100 nm. In specific cases and where warranted by concerns for the environment, health, safety, or competitiveness the number size distribution threshold of 50% may be replaced by a threshold between 1 and 50% (European Commission (2012)). However, the current legislative setting in EU law is not entirely perfect. The European Parliament has repeatedly called on the European Commission to revise REACH to better adapt to nanotechnologies, including nanocellulose from plants (European Commission (2012)).
EU Legislation on the Registration of Chemicals
The basic legislation in the field of chemicals is REACH, establishing a European Chemicals Agency and Regulation (EC) No 1272/2008 of the European Parliament and of the Council of 16 December 2008 on classification, labelling, and packaging of substances and mixtures. Another regulation governing nanocellulose is Regulation (EU) No 649/2012 of the European Parliament and of the Council of 4 July 2012 concerning the export and import of hazardous chemicals Text with EEA relevance. Commission Regulation (EC) No. Regulation (EC) No 440/2008 laying down test methods pursuant to Regulation (EC) No 1907/2006 of the European Parliament and of the Council on the Registration, Evaluation, Authorization and Restriction of Chemicals (REACH). There is currently no legislation that targets nanotechnologies, including nanocellulose. Therefore, REACH can only be applied very generally.
REACH defines a substance within the meaning of Article 3 1) as follows: substance means a chemical element and its compounds in the natural state or obtained by any manufacturing process, including any additive necessary to preserve its stability and any impurity deriving from the process used, but excluding any solvent which may be separated without affecting the stability of the substance or changing its composition. Nanocellulose is a substance within the meaning of this article (Foth and Hayes, 2008). Under Article 6 1) of REACH, an application for registration of a substance is submitted to the Agency by any manufacturer or importer of a substance on its own or in one or more preparations in quantities of 1 tonne or more per year; the application is subject to a fee, the amount of which is set under REACH. The application for registration has the prescribed requirements which apply to the substance itself as well as to the manufacturer. The substance is then identified according to the sections in Annex VI of REACH (Article 7) and must be accompanied by a technical dossier and, in selected cases, a chemical safety report (Article 10 (a) and (b)). It also includes a requirement to prepare a chemical safety report for substances subject to registration in quantities of 10 tonnes or more per year (Article 14 (1)), unless an exemption within the meaning of Article 14 (2) is granted.
Not all substances are subject to registration as few are exempted due to their use for research and development. Substances that are manufactured or imported for research and development purposes are not subject to registration for 5 years (Article 9 (1)). However, even in such a case, the importer and the manufacturer are obliged to provide the Agency with sufficient information, such as the identification of the manufacturer and the substance (Article 9 (2)).
The application for registration is submitted to the Agency, which then checks all the details and assigns a registration number to the substance (Article 20 (1) and (3)). This is followed by an evaluation of the record, where the Agency reviews the testing proposals and then evaluates the substances (Article 44). The Agency then develops the criteria for prioritizing substances.
The Agency shall subsequently notify the registrants or users of the draft decision on the evaluation of the substance and shall inform them of the right to comment on that decision within 30 days of receipt of the proposal. The draft marketing authorization shall also be notified to the competent authorities of the Member States, including any comments from applicants. Member States then can also propose amendments to the decision (Article 50 (1) and Article 51 (1) and (2)).
Certain substances of very high concern that will be included in Annex XIV REACH require marketing authorization. Their placement on the market requires a justification as to why they must be used and whether they cannot be replaced by suitable alternatives or technologies; however, such implementation must be economically and technically feasible (Articles 55 and 56). These are, for example, carcinogenic or toxic substances. The European Commission then decides on the authorization of these substances (Article 60).
Terms of Registration
REACH sets out the general conditions for the registration of a substance, i.e., nanocellulose in this case. The notification includes both the identification of the manufacturer or importer and, above all, the identification of the substance according to the Annex to REACH. Of particular importance is the technical record, which includes study summaries (Article 10 (a) (vi)). Nanocellulose may also be exempted from registration if it is for research and development (Article 9). However, even in the case of an exemption, it is necessary to identify the substance and duly justify its use for research and development.
Reach and Nanocellulose
Nanocellulose from plants is a polymer that exists in nanoforms. It is a substance within the meaning of Article 3 (1) of REACH. This substance is subject to registration if more than 1 tonne per year is manufactured or imported into the EU. As these are nanoforms, the substance must be registered. However, if a substance is exempted under REACH, it would not be subject to registration. Conversely, if a substance meets the definition of nanoform in Annex VI of REACH and the substance is subject to registration, the nanoform condition must also be met. “Cellulose pulp” is exempted from registration in Annex IV of REACH; however, cellulose pulp is not nanocellulose. If they are naturally occurring substances and are not chemically modified, they shall also be exempted from registration under Annex V, provided it is not a dangerous substance.
European Medicines Agency and Nanocellulose
The European Medicines Agency (EMA) is an agency of the European Union established by Regulation (EC) No 726/2004 of the European Parliament and of the Council of 31 March 2004 laying down community procedures for the authorization and supervision of medicinal products for human and veterinary use and establishing a European Medicines Agency. This regulation regulates three main areas: 1) the authorization and supervision of medicinal products for human use, 2) the authorization of veterinary medicinal products, and 3) the functioning of the European Medicines Agency. The EMA is responsible for coordinating the scientific resources and for the evaluation of medicinal products. The EMA must also cooperate with the Member States authorities and provide them the scientific knowledge about the specific effects of medicinal products. To achieve this goal, it creates, for example, a database of medicinal products (Articles 56 and 57).
No medicinal product, neither veterinary nor human, could be introduced in the EU market without authorization (Article 3). Registration applications are submitted to the EMA. Each application shall contain the particulars referred to in Article 6. The Agency shall decide on the registration in a specific period under Article 6 (3). The registration is then valid for a renewable period of 5 years (Article 14 1) and (2)). Marketing authorization is thus done by EMA. If the EMA carries out a marketing authorization, it is valid in all EU Member States and in Norway, Iceland, and Liechtenstein. The Regulation also addresses the issue of criminal and civil liability in such a way that the granting of a marketing authorization does not affect the civil and criminal liability of the manufacturer or marketing authorization holder (Article 15). The regulation also describes pharmacovigilance, which is crucial for placing on the internal market. The holder of a marketing authorization for a medicinal product for human use must always have at his disposal a qualified person responsible for pharmacovigilance (Article 23).
The EMA does not contain any specific regulation for nanocellulose. According to the EMA, however, nanocellulose from plants can be used as an excipient. Specific conditions then apply to excipients (Committee for Medicinal Products for Human Use, 2018). For example, the choice of this substance and other characteristics for determining the active substance must be explained. The EMA is related to REACH. If the excipient, including nanocellulose from plants, is a new substance then that would be subjected to the registration process under REACH. It therefore depends on the specific composition and use of nanocellulose from plants for therapeutic purposes. It can hence be concluded that if the botanical NC is to serve as a medicinal product, human or veterinary, it must be approved by the EMA. The registrant must then also submit the relevant dossiers to be assessed by the EMA.
Future of Nanocellulose
In addition to renewability, better carbon footprint, sustainability, indispensability to bioeconomy, and environmentally benignity of nanocellulose, especially in comparison to petroleum-based products, the recent developments in science and technology have placed nanocellulose in the categories of future material of innovation and production. To understand the future scope of nanocellulose, we will consider different fields individually. In material science, nanocellulose based and/or reinforced composites for materials application in food packaging involving active packaging have been reported numerous times and some commercial products are already available. Active or smart packaging, which is a developing branch of food packaging technology, can be seen as a promising application of cellulose. The transparent paper and its applications in thin film transistors, organic light-emitting-diode, organic photovoltaic devices, printed foldable antenna, and resistive paper touch screen can give some idea of future applications of cellulose in high value areas. In the biomedical field, newly reported applications in tissue engineering, implants, prosthetics, drug delivery, patches, and wound healing using films, aerogels, and hydrogels, 3D printed structures add a very specific dimension to the high value applications of cellulose. Additionally, the already marketed bio-cellulose based products for tissue engineering, wound care, and wound healing are relatively new entrants to the catalog of cellulosic biomedical products. In light of the above arguments, the future of cellulosic materials in interdisciplinary and especially in high-value applications seems very bright and these materials can be assumed to be major contributors to future sustainable bio-economies at the global scale.
Conclusion
Utilization of cellulose in human society is as old as utilization of paper. It has been a traditional product of the pulp and paper industry. Along with the development in the field of science, the understanding of structure and properties of cellulose has improved, which in turn has opened the doors for its newer applications. Better understanding of delignification process of wood and other lignocellulosic biomass has led to greener, economical, and environmentally favorable processes of cellulose production. Recent reports on biological compatibility and toxicity properties of cellulose have pulled cellulose from just being a tableting excipient and pH sensitive coating agent to being a drug carrier molecule and more. Cellulose is now being used in its nano form as nanocrystals and nanofibers, which are now extensively explored as drug carriers, tissue engineering material, in wound healing, 3D bioprinting, etc. A good number of pilot plant and semi-commercial scale demonstration and production units are already working across the globe and bodes well for the future of nanocellulose-based materials.
There is no specific legislative act in EU legislation regulating nanocellulose from plants. Thus, only the general REACH regulation, which regulates the manufacture, import, and registration of chemicals, can be applied. Several other regulations that follow the content of the REACH regulation will also be applied. For the registration of nanocellulose from plants, it will be necessary to submit the necessary documents for registration, in particular the exact identification of the substance. The condition of substance identification will also be necessary if nanocellulose from plants is exempted from registration for scientific and research purposes; however, this purpose must always be demonstrated by the importer or manufacturer.
Author Contributions
PKM and MR contributed to the conception and design of the study. OP, AE and HM organized the database. PKM wrote the first draft of the manuscript. MR, AE, OP, and HM wrote/revised sections of the manuscript. All authors contributed to manuscript revision, read, and approved the submitted version.
Conflict of Interest
The authors declare that the research was conducted in the absence of any commercial or financial relationships that could be construed as a potential conflict of interest.
Publisher’s Note
All claims expressed in this article are solely those of the authors and do not necessarily represent those of their affiliated organizations, or those of the publisher, the editors and the reviewers. Any product that may be evaluated in this article, or claim that may be made by its manufacturer, is not guaranteed or endorsed by the publisher.
Acknowledgments
We thank the European Chemical Agency and the European Medicines Agency for the opportunity of consultation.
References
Abdul Khalil, H. P. S., Davoudpour, Y., Islam, M. N., Mustapha, A., Sudesh, K., Dungani, R., et al. (2014). Production and Modification of Nanofibrillated Cellulose Using Various Mechanical Processes: A Review. Carbohydr. Polym. 99, 649–665. doi:10.1016/j.carbpol.2013.08.069
Abitbol, T., Rivkin, A., Cao, Y., Nevo, Y., Abraham, E., Ben-Shalom, T., et al. (2016). Nanocellulose, a Tiny Fiber with Huge Applications. Curr. Opin. Biotechnol. 39, 76–88. doi:10.1016/j.copbio.2016.01.002
Akhlaghi, S. P., Berry, R. C., and Tam, K. C. (2013). Surface Modification of Cellulose Nanocrystal with Chitosan Oligosaccharide for Drug Delivery Applications. Cellulose 20, 1747–1764. doi:10.1007/s10570-013-9954-y
Almeida, I. F., Pereira, T., Silva, N. H. C. S., Gomes, F. P., Silvestre, A. J. D., Freire, C. S. R., et al. (2014). Bacterial Cellulose Membranes as Drug Delivery Systems: an In Vivo Skin Compatibility Study. Eur. J. Pharmaceutics Biopharmaceutics 86, 332–336. doi:10.1016/j.ejpb.2013.08.008
Anderson, R. L., Owens, J. W., and Timms, C. W. (1992). The Toxicity of Purified Cellulose in Studies with Laboratory Animals. Cancer Lett. 63, 83–92. doi:10.1016/0304-3835(92)90057-3
Asabuwa Ngwabebhoh, F., Ilkar Erdagi, S., and Yildiz, U. (2018). Pickering Emulsions Stabilized Nanocellulosic-Based Nanoparticles for Coumarin and Curcumin Nanoencapsulations: In Vitro Release, Anticancer and Antimicrobial Activities. Carbohydr. Polym. 201, 317–328. doi:10.1016/j.carbpol.2018.08.079
Basu, A., Lindh, J., Ålander, E., Strømme, M., and Ferraz, N. (2017). On the Use of Ion-Crosslinked Nanocellulose Hydrogels for Wound Healing Solutions: Physicochemical Properties and Application-Oriented Biocompatibility Studies. Carbohydr. Polym. 174, 299–308. doi:10.1016/j.carbpol.2017.06.073
Bhandari, J., Mishra, H., Mishra, P. K., Wimmer, R., Ahmad, F. J., and Talegaonkar, S. (2017). Cellulose Nanofiber Aerogel as a Promising Biomaterial for Customized Oral Drug Delivery. Ijn Vol. 12, 2021–2031. doi:10.2147/IJN.S124318
Burt, H. M., Jackson, J. K., and Hamad, W. Y. (2014). Binding Drugs with Nanocrystalline Cellulose (Ncc). Available at: https://patents.google.com/patent/US20140335132A1/en (Accessed January 18, 2022).
Chawla, P. R., Bajaj, I. B., Survase, S. A., and Singhal, R. S. (2009). Microbial Cellulose: Fermentative Production and Applications. Food Technol. Biotechnol. 47, 107–124.
Chen, P., Yu, H., Liu, Y., Chen, W., Wang, X., and Ouyang, M. (2013). Concentration Effects on the Isolation and Dynamic Rheological Behavior of Cellulose Nanofibers via Ultrasonic Processing. Cellulose 20, 149–157. doi:10.1007/s10570-012-9829-7
Colombo, L., Zoia, L., Violatto, M. B., Previdi, S., Talamini, L., Sitia, L., et al. (2015). Organ Distribution and Bone Tropism of Cellulose Nanocrystals in Living Mice. Biomacromolecules 16, 2862–2871. doi:10.1021/acs.biomac.5b00805
Committee for Medicinal Products for human Use (2018). Excipients in the Dossier for Application Marketing Authorisation of a Medicinal Product. Eur. Med. Agency. Available at: https://www.ema.europa.eu/en/excipients-dossier-application-marketing-authorisation-medicinal-product (Accessed October 5, 2021).
Cullen, R. T., Miller, B. G., Jones, A. D., and Davis, J. M. G. (2002). Toxicity of Cellulose Fibres. Ann. Occup. Hyg. 46, 81–84. doi:10.1093/annhyg/46.suppl_1.81
Curvello, R., Raghuwanshi, V. S., and Garnier, G. (2019). Engineering Nanocellulose Hydrogels for Biomedical Applications. Adv. Colloid Interf. Sci. 267, 47–61. doi:10.1016/j.cis.2019.03.002
de la Motte, H., Hasani, M., Brelid, H., and Westman, G. (2011). Molecular Characterization of Hydrolyzed Cationized Nanocrystalline Cellulose, Cotton Cellulose and Softwood Kraft Pulp Using High Resolution 1D and 2D NMR. Carbohydr. Polym. 85, 738–746. doi:10.1016/j.carbpol.2011.03.038
Devarayan, K., and Kim, B.-S. (2015). Reversible and Universal pH Sensing Cellulose Nanofibers for Health Monitor. Sensors Actuators B: Chem. 209, 281–286. doi:10.1016/j.snb.2014.11.120
Díez, I., Eronen, P., Österberg, M., Linder, M. B., Ikkala, O., and Ras, R. H. A. (2011). Functionalization of Nanofibrillated Cellulose with Silver Nanoclusters: Fluorescence and Antibacterial Activity. Macromol. Biosci. 11, 1185–1191. doi:10.1002/mabi.201100099
Dong, L., Zhang, X., Ren, S., Lei, T., Sun, X., Qi, Y., et al. (2016). Poly(diallyldimethylammonium Chloride)-Cellulose Nanocrystals Supported Au Nanoparticles for Nonenzymatic Glucose Sensing. RSC Adv. 6, 6436–6442. doi:10.1039/C5RA23935D
Dong, S., Cho, H. J., Lee, Y. W., and Roman, M. (2014). Synthesis and Cellular Uptake of Folic Acid-Conjugated Cellulose Nanocrystals for Cancer Targeting. Biomacromolecules 15, 1560–1567. doi:10.1021/bm401593n
Drury, J. L., and Mooney, D. J. (2003). Hydrogels for Tissue Engineering: Scaffold Design Variables and Applications. Biomaterials 24, 4337–4351. doi:10.1016/s0142-9612(03)00340-5
Edwards, J. V., Prevost, N., Sethumadhavan, K., Ullah, A., and Condon, B. (2013). Peptide Conjugated Cellulose Nanocrystals with Sensitive Human Neutrophil Elastase Sensor Activity. Cellulose 20, 1223–1235. doi:10.1007/s10570-013-9901-y
Emara, L., El-Ashmawy, A., Taha, N., El-Shaffei, K., Mahdey, E.-S., and El-kholly, H. (2016). Nano-crystalline Cellulose as a Novel Tablet Excipient for Improving Solubility and Dissolution of Meloxicam. J. App Pharm. Sci. 6, 032–043. doi:10.7324/japs.2016.60205
Esmaeili, C., Abdi, M., Mathew, A., Jonoobi, M., Oksman, K., and Rezayi, M. (2015). Synergy Effect of Nanocrystalline Cellulose for the Biosensing Detection of Glucose. Sensors 15, 24681–24697. doi:10.3390/s151024681
European Commission (2012). COMMUNICATION from the COMMISSION to the EUROPEAN PARLIAMENT, the COUNCIL and the EUROPEAN ECONOMIC and SOCIAL COMMITTEE Second Regulatory Review on Nanomaterials. Available at: https://eur-lex.europa.eu/legal-content/EN/TXT/?_csrf=5bd9bd61-f83a-418b-8f7a-7426953419a4&lang1=EN&lang2=choose&lang3=choose&uri=CELEX%3A52012DC0572 (Accessed September 24, 2021).
Eyholzer, C., Borges de Couraça, A., Duc, F., Bourban, P. E., Tingaut, P., Zimmermann, T., et al. (2011). Biocomposite Hydrogels with Carboxymethylated, Nanofibrillated Cellulose Powder for Replacement of the Nucleus Pulposus. Biomacromolecules 12, 1419–1427. doi:10.1021/bm101131b
Ferrer, A., Quintana, E., Filpponen, I., Solala, I., Vidal, T., Rodríguez, A., et al. (2012). Effect of Residual Lignin and Heteropolysaccharides in Nanofibrillar Cellulose and Nanopaper from wood Fibers. Cellulose 19, 2179–2193. doi:10.1007/s10570-012-9788-z
Fink, H.-P., Philipp, B., Paul, D., Serimaa, R., and Paakkari, T. (1987). The Structure of Amorphous Cellulose as Revealed by Wide-Angle X-ray Scattering. Polymer 28, 1265–1270. doi:10.1016/0032-3861(87)90435-6
Fink, H., Hong, J., Drotz, K., Risberg, B., Sanchez, J., and Sellborn, A. (2011). An In Vitro Study of Blood Compatibility of Vascular Grafts Made of Bacterial Cellulose in Comparison with Conventionally-Used Graft Materials. J. Biomed. Mater. Res. 97A, 52–58. doi:10.1002/jbm.a.33031
Foth, H., and Hayes, A. (2008). Background of REACH in EU Regulations on Evaluation of Chemicals. Hum. Exp. Toxicol. 27, 443–461. doi:10.1177/0960327108092296
Galkina, O. L., Ivanov, V. K., Agafonov, A. V., Seisenbaeva, G. A., and Kessler, V. G. (2015). Cellulose Nanofiber-Titania Nanocomposites as Potential Drug Delivery Systems for Dermal Applications. J. Mater. Chem. B 3, 1688–1698. doi:10.1039/c4tb01823k
Gardiner, E. S., and Sarko, A. (1985). Packing Analysis of Carbohydrates and Polysaccharides. 16. The crystal Structures of Celluloses IVI and IVII. Can. J. Chem. 63, 173–180. doi:10.1139/v85-027
Gardner, K. H., and Blackwell, J. (1974). The Structure of Native Cellulose. Biopolymers 13, 1975–2001. doi:10.1002/bip.1974.360131005
Garvey, C. J., Parker, I. H., and Simon, G. P. (2005). On the Interpretation of X-Ray Diffraction Powder Patterns in Terms of the Nanostructure of Cellulose I Fibres. Macromol. Chem. Phys. 206, 1568–1575. doi:10.1002/macp.200500008
Gautam, S., Bundela, P., Pandey, A., Awasthi, M., and Sarsaiya, S. (2010). A Review on Systematic Study of Cellulose. Appl. Nat. Sci. Found. 2, 333–343. doi:10.31018/jans.v2i2.143
Guo, T., Pei, Y., Tang, K., He, X., Huang, J., and Wang, F. (2017). Mechanical and Drug Release Properties of Alginate Beads Reinforced with Cellulose. J. Appl. Polym. Sci. 134 (44495), 1–9. doi:10.1002/app.44495
Habibi, Y., Lucia, L. A., and Rojas, O. J. (2010). Cellulose Nanocrystals: Chemistry, Self-Assembly, and Applications. Chem. Rev. 110, 3479–3500. doi:10.1021/cr900339w
Hakkarainen, T., Koivuniemi, R., Kosonen, M., Escobedo-Lucea, C., Sanz-Garcia, A., Vuola, J., et al. (2016). Nanofibrillar Cellulose Wound Dressing in Skin Graft Donor Site Treatment. J. Controlled Release 244, 292–301. doi:10.1016/j.jconrel.2016.07.053
He, J., Cui, S., and Wang, S.-y. (2008). Preparation and Crystalline Analysis of High-Grade Bamboo Dissolving Pulp for Cellulose Acetate. J. Appl. Polym. Sci. 107, 1029–1038. doi:10.1002/app.27061
He, X., Xiao, Q., Lu, C., Wang, Y., Zhang, X., Zhao, J., et al. (2014). Uniaxially Aligned Electrospun All-Cellulose Nanocomposite Nanofibers Reinforced with Cellulose Nanocrystals: Scaffold for Tissue Engineering. Biomacromolecules 15, 618–627. doi:10.1021/bm401656a
Herrick, F. W., Casebier, R. L., Hamilton, J. K., and Sandberg, K. R. (1983). Microfibrillated Cellulose: Morphology and Accessibility. Shelton, WA: ITT Rayonier Inc.
Horii, F., Hirai, A., and Kitamaru, R. (1982). Solid-state High-Resolution 13 C-NMR Studies of Regenerated Cellulose Samples with Different Crystallinities. Polym. Bull. 8, 163–170. doi:10.1007/bf00263023
Hult, E.-L., Iversen, T., and Sugiyama, J. (2003). Characterization of the Supermolecular Structure of Cellulose in wood Pulp Fibres. Cellulose 10, 103–110. doi:10.1023/a:1024080700873
Jack, A. A., Nordli, H. R., Powell, L. C., Powell, K. A., Kishnani, H., Johnsen, P. O., et al. (2017). The Interaction of wood Nanocellulose Dressings and the Wound Pathogen P. aeruginosa. Carbohydr. Polym. 157, 1955–1962. doi:10.1016/j.carbpol.2016.11.080
Jeong, S. I., Lee, S. E., Yang, H., Jin, Y.-H., Park, C.-S., and Park, Y. S. (2010). Toxicologic Evaluation of Bacterial Synthesized Cellulose in Endothelial Cells and Animals. Mol. Cell. Toxicol. 6, 370–377. doi:10.1007/s13273-010-0049-7
Jorfi, M., and Foster, E. J. (2015). Recent Advances in Nanocellulose for Biomedical Applications. J. Appl. Polym. Sci. 132, a–n. doi:10.1002/app.41719
Jozala, A. F., de Lencastre-Novaes, L. C., Lopes, A. M., de Carvalho Santos-Ebinuma, V., Mazzola, P. G., Pessoa-Jr, A., et al. (2016). Bacterial Nanocellulose Production and Application: a 10-year Overview. Appl. Microbiol. Biotechnol. 100, 2063–2072. doi:10.1007/s00253-015-7243-4
Kautto, P., and Valve, H. (2019). Cosmopolitics of a Regulatory Fit: The Case of Nanocellulose. Sci. as Cult. 28, 25–45. doi:10.1080/09505431.2018.1533935
Klemm, D., Kramer, F., Moritz, S., Lindström, T., Ankerfors, M., Gray, D., et al. (2011). Nanocelluloses: A New Family of Nature-Based Materials. Angew. Chem. Int. Ed. 50, 5438–5466. doi:10.1002/anie.201001273
Kolakovic, R., Peltonen, L., Laukkanen, A., Hirvonen, J., and Laaksonen, T. (2012). Nanofibrillar Cellulose Films for Controlled Drug Delivery. Eur. J. Pharmaceutics Biopharmaceutics 82, 308–315. doi:10.1016/j.ejpb.2012.06.011
Kupnik, K., Primožič, M., Kokol, V., and Leitgeb, M. (2020). Nanocellulose in Drug Delivery and Antimicrobially Active Materials. Polymers 12, 2825. doi:10.3390/polym12122825
Kurosumi, A., Sasaki, C., Yamashita, Y., and Nakamura, Y. (2009). Utilization of Various Fruit Juices as Carbon Source for Production of Bacterial Cellulose by Acetobacter Xylinum NBRC 13693. Carbohydr. Polym. 76, 333–335. doi:10.1016/j.carbpol.2008.11.009
Laurén, P., Lou, Y. R., Raki, M., Urtti, A., Bergström, K., and Yliperttula, M. (2014). Technetium-99m-labeled Nanofibrillar Cellulose Hydrogel for In Vivo Drug Release. Eur. J. Pharm. Sci. 65, 79–88. doi:10.1016/j.ejps.2014.09.013
Laurén, P., Paukkonen, H., Lipiäinen, T., Dong, Y., Oksanen, T., Räikkönen, H., et al. (2018). Pectin and Mucin Enhance the Bioadhesion of Drug Loaded Nanofibrillated Cellulose Films. Pharm. Res. 35, 1–14. doi:10.1007/s11095-018-2428-z
Lin, N., and Dufresne, A. (2014). Nanocellulose in Biomedicine: Current Status and Future prospect. Eur. Polym. J. 59, 302–325. doi:10.1016/j.eurpolymj.2014.07.025
Liu, J., Chinga-Carrasco, G., Cheng, F., Xu, W., Willför, S., Syverud, K., et al. (2016). Hemicellulose-reinforced Nanocellulose Hydrogels for Wound Healing Application. Cellulose 23, 3129–3143. doi:10.1007/s10570-016-1038-3
Mandrycky, C., Wang, Z., Kim, K., and Kim, D.-H. (2016). 3D Bioprinting for Engineering Complex Tissues. Biotechnol. Adv. 34, 422–434. doi:10.1016/j.biotechadv.2015.12.011
Markstedt, K., Mantas, A., Tournier, I., Martínez Ávila, H., Hägg, D., and Gatenholm, P. (2015). 3D Bioprinting Human Chondrocytes with Nanocellulose-Alginate Bioink for Cartilage Tissue Engineering Applications. Biomacromolecules 16, 1489–1496. doi:10.1021/acs.biomac.5b00188
Martínez Ávila, H., Schwarz, S., Rotter, N., and Gatenholm, P. (2016). 3D Bioprinting of Human Chondrocyte-Laden Nanocellulose Hydrogels for Patient-specific Auricular Cartilage Regeneration. Bioprinting 1-2, 22–35. doi:10.1016/j.bprint.2016.08.003
Mishra, P. K., Ekielski, A., Mukherjee, S., Sahu, S., Chowdhury, S., Mishra, M., et al. (2019). Wood-based Cellulose Nanofibrils: Haemocompatibility and Impact on the Development and Behaviour of Drosophila melanogaster. Biomolecules 9, 363. doi:10.3390/biom9080363
Mishra, P. K., Gregor, T., and Wimmer, R. (2017). Utilising brewer’s Spent Grain as a Source of Cellulose Nanofibres Following Separation of Protein-Based Biomass. BioResources 12, 107–116. doi:10.15376/biores.12.1.107-116
Mishra, P. K., and Wimmer, R. (2017). Aerosol Assisted Self-Assembly as a Route to Synthesize Solid and Hollow Spherical Lignin Colloids and its Utilization in Layer by Layer Deposition. Ultrason. Sonochem. 35, 45–50. doi:10.1016/j.ultsonch.2016.09.001
Mohammadkazemi, F., Azin, M., and Ashori, A. (2015). Production of Bacterial Cellulose Using Different Carbon Sources and Culture media. Carbohydr. Polym. 117, 518–523. doi:10.1016/j.carbpol.2014.10.008
Mohanta, V., Madras, G., and Patil, S. (2014). Layer-by-layer Assembled Thin Films and Microcapsules of Nanocrystalline Cellulose for Hydrophobic Drug Delivery. ACS Appl. Mater. Inter. 6, 20093–20101. doi:10.1021/am505681e
Moroni, L., Schrooten, J., Truckenmüller, R., Rouwkema, J., Sohier, J., and van Blitterswijk, C. A. (2014). Tissue Engineering. London: Elsevier, 1–21. doi:10.1016/b978-0-12-420145-3.00001-8
Müller, M., Öztürk, E., Arlov, Ø., Gatenholm, P., and Zenobi-Wong, M. (2017). Alginate Sulfate-Nanocellulose Bioinks for Cartilage Bioprinting Applications. Ann. Biomed. Eng. 45, 210–223. doi:10.1007/s10439-016-1704-5
Navarro, J. R. G., Wennmalm, S., Godfrey, J., Breitholtz, M., and Edlund, U. (2016). Luminescent Nanocellulose Platform: From Controlled Graft Block Copolymerization to Biomarker Sensing. Biomacromolecules 17, 1101–1109. doi:10.1021/acs.biomac.5b01716
Nelson, K., Retsina, T., Pylkkanen, V., and O’Connor, R. (2014). Processes and Apparatus for Producing Nanocellulose, and Compositions and Products Produced Therefrom. Available at: https://www.google.com/patents/US20140154757.
Nguyen, D., Hägg, D. A., Forsman, A., Ekholm, J., Nimkingratana, P., Brantsing, C., et al. (2017). Cartilage Tissue Engineering by the 3D Bioprinting of iPS Cells in a Nanocellulose/Alginate Bioink. Sci. Rep. 7, 658. doi:10.1038/s41598-017-00690-y
Olkowski, A. A., and Laarveld, B. (2013). Catalytic Biomass Conversion. Available at: https://www.google.com/patents/WO2013000074A1?cl=en.
Paakkari, T., Serimaa, R., and Fink, H.-P. (1989). Structure of Amorphous Cellulose. Acta Polym. 40, 731–734. doi:10.1002/actp.1989.010401205
Park, S., Baker, J. O., Himmel, M. E., Parilla, P. A., and Johnson, D. K. (2010). Cellulose Crystallinity index: Measurement Techniques and Their Impact on Interpreting Cellulase Performance. Biotechnol. Biofuels 3, 10. doi:10.1186/1754-6834-3-10
Patil, M. D., Patil, V. D., Sapre, A. A., Ambone, T. S., Torris A. T., A., Shukla, P. G., et al. (2018). Tuning Controlled Release Behavior of Starch Granules Using Nanofibrillated Cellulose Derived from Waste Sugarcane Bagasse. ACS Sustainable Chem. Eng. 6, 9208–9217. doi:10.1021/acssuschemeng.8b01545
Pérez, J., Muñoz-Dorado, J., de la Rubia, T., and Martínez, J. (2002). Biodegradation and Biological Treatments of Cellulose, Hemicellulose and Lignin: an Overview. Int. Microbiol. 5, 53–63. doi:10.1007/s10123-002-0062-3
Petersen, N., and Gatenholm, P. (2011). Bacterial Cellulose-Based Materials and Medical Devices: Current State and Perspectives. Appl. Microbiol. Biotechnol. 91, 1277–1286. doi:10.1007/s00253-011-3432-y
Rauscher, H., Rasmussen, K., and Sokull-Klüttgen, B. (2017). Regulatory Aspects of Nanomaterials in the EU. Chem. Ingenieur Technik 89, 224–231. doi:10.1002/cite.201600076
Ruland, W. (1961). X-ray Determination of Crystallinity and Diffuse Disorder Scattering. Acta Crystallogr. 14, 1180–1185. doi:10.1107/s0365110x61003429
Salimi, S., Sotudeh-Gharebagh, R., Zarghami, R., Chan, S. Y., and Yuen, K. H. (2019). Production of Nanocellulose and its Applications in Drug Delivery: A Critical Review. ACS Sustainable Chem. Eng. 7, 15800–15827. doi:10.1021/acssuschemeng.9b02744
Sarkar, G., Orasugh, J. T., Saha, N. R., Roy, I., Bhattacharyya, A., Chattopadhyay, A. K., et al. (2017). Cellulose Nanofibrils/chitosan Based Transdermal Drug Delivery Vehicle for Controlled Release of Ketorolac Tromethamine. New J. Chem. 41, 15312–15319. doi:10.1039/c7nj02539d
Segal, L., Creely, J. J., Martin, A. E., and Conrad, C. M. (1959). An Empirical Method for Estimating the Degree of Crystallinity of Native Cellulose Using the X-Ray Diffractometer. Textile Res. J. 29, 786–794. doi:10.1177/004051755902901003
Shahrokhian, S., Naderi, L., and Ghalkhani, M. (2015). Nanocellulose/Carbon Nanoparticles Nanocomposite Film Modified Electrode for Durable and Sensitive Electrochemical Determination of Metoclopramide. Electroanalysis 27, 2637–2644. doi:10.1002/elan.201500266
Siqueira, P., Siqueira, É., De Lima, A. E., Siqueira, G., Pinzón-Garcia, A. D., Lopes, A. P., et al. (2019). Three-Dimensional Stable Alginate-Nanocellulose Gels for Biomedical Applications: Towards Tunable Mechanical Properties and Cell Growing. Nanomaterials 9, 78. doi:10.3390/nano9010078
Siró, I., and Plackett, D. (2010). Microfibrillated Cellulose and New Nanocomposite Materials: a Review. Cellulose 17, 459–494. doi:10.1007/s10570-010-9405-y
Staudinger, H. (1933). Viscosity Investigations for the Examination of the Constitution of Natural Products of High Molecular Weight and of Rubber and Cellulose. Trans. Faraday Soc. 29, 18–32. doi:10.1039/tf9332900018
Supramaniam, J., Adnan, R., Mohd Kaus, N. H., and Bushra, R. (2018). Magnetic Nanocellulose Alginate Hydrogel Beads as Potential Drug Delivery System. Int. J. Biol. Macromolecules 118, 640–648. doi:10.1016/j.ijbiomac.2018.06.043
Tanskul, S., Amornthatree, K., and Jaturonlak, N. (2013). A New Cellulose-Producing Bacterium, Rhodococcus Sp. MI 2: Screening and Optimization of Culture Conditions. Carbohydr. Polym. 92, 421–428. doi:10.1016/j.carbpol.2012.09.017
Ullah, H., Wahid, F., Santos, H. A., and Khan, T. (2016). Advances in Biomedical and Pharmaceutical Applications of Functional Bacterial Cellulose-Based Nanocomposites. Carbohydr. Polym. 150, 330–352. doi:10.1016/j.carbpol.2016.05.029
Valo, H., Kovalainen, M., Laaksonen, P., Häkkinen, M., Auriola, S., Peltonen, L., et al. (2011). Immobilization of Protein-Coated Drug Nanoparticles in Nanofibrillar Cellulose Matrices-Enhanced Stability and Release. J. Controlled Release 156, 390–397. doi:10.1016/j.jconrel.2011.07.016
Wada, M., Heux, L., and Sugiyama, J. (2004). Polymorphism of Cellulose I Family: Reinvestigation of Cellulose IVI. Biomacromolecules 5, 1385–1391. doi:10.1021/bm0345357
Wang, Q. Q., Zhu, J. Y., Gleisner, R., Kuster, T. A., Baxa, U., and McNeil, S. E. (2012). Morphological Development of Cellulose Fibrils of a Bleached eucalyptus Pulp by Mechanical Fibrillation. Cellulose 19, 1631–1643. doi:10.1007/s10570-012-9745-x
Zhong, S. P., Zhang, Y. Z., and Lim, C. T. (2010). Tissue Scaffolds for Skin Wound Healing and Dermal Reconstruction. WIREs Nanomed Nanobiotechnol 2, 510–525. doi:10.1002/wnan.100
Keywords: Nanocellulose, Cellulose, REACh regulation, EMA, Bioeconomy, Biomedicine
Citation: Mishra PK, Pavelek O, Rasticova M, Mishra H and Ekielski A (2022) Nanocellulose-Based Biomedical Scaffolds in Future Bioeconomy: A Techno-Legal Assessment of the State-of-the-Art. Front. Bioeng. Biotechnol. 9:789603. doi: 10.3389/fbioe.2021.789603
Received: 05 October 2021; Accepted: 24 December 2021;
Published: 11 February 2022.
Edited by:
Suresh K. Verma, Uppsala University, SwedenReviewed by:
Ranjit Mehta, University of Michigan, United StatesPuja Kumari, Masaryk University, Czechia
Copyright © 2022 Mishra, Pavelek, Rasticova, Mishra and Ekielski. This is an open-access article distributed under the terms of the Creative Commons Attribution License (CC BY). The use, distribution or reproduction in other forums is permitted, provided the original author(s) and the copyright owner(s) are credited and that the original publication in this journal is cited, in accordance with accepted academic practice. No use, distribution or reproduction is permitted which does not comply with these terms.
*Correspondence: Pawan Kumar Mishra, cGF3YW4ubWlzaHJhQG1lbmRlbHUuY3o=