- 1Department of Biological Sciences and Technology, School of Environmental Studies, China University of Geosciences, Wuhan, China
- 2Key Laboratory of Systems Bioengineering (Ministry of Education), SynBio Research Platform, Collaborative Innovation Center of Chemical Science and Engineering, School of Chemical Engineering and Technology, Tianjin University, Tianjin, China
- 3State Key Laboratory of Biogeology and Environmental Geology, China University of Geosciences, Wuhan, China
- 4Hubei Key Laboratory of Yangtze Catchment Environmental Aquatic Science, China University of Geosciences, Wuhan, China
- 5State Environmental Protection Key Laboratory of Source Apportionment and Control of Aquatic Pollution, Ministry of Ecology and Environment, Wuhan, China
Geobacter and Shewanella spp. were discovered in late 1980s as dissimilatory metal-reducing microorganisms that can transfer electrons from cytoplasmic respiratory oxidation reactions to external metal-containing minerals. In addition to mineral-based electron acceptors, Geobacter and Shewanella spp. also can transfer electrons to electrodes. The microorganisms that have abilities to transfer electrons to electrodes are known as exoelectrogens. Because of their remarkable abilities of electron transfer, Geobacter and Shewanella spp. have been the two most well studied groups of exoelectrogens. They are widely used in bioelectrochemical systems (BESs) for various biotechnological applications, such as bioelectricity generation via microbial fuel cells. These applications mostly associate with Geobacter and Shewanella biofilms grown on the surfaces of electrodes. Geobacter and Shewanella biofilms are electrically conductive, which is conferred by matrix-associated electroactive components such as c-type cytochromes and electrically conductive nanowires. The thickness and electroactivity of Geobacter and Shewanella biofilms have a significant impact on electron transfer efficiency in BESs. In this review, we first briefly discuss the roles of planktonic and biofilm-forming Geobacter and Shewanella cells in BESs, and then review biofilm biology with the focus on biofilm development, biofilm matrix, heterogeneity in biofilm and signaling regulatory systems mediating formation of Geobacter and Shewanella biofilms. Finally, we discuss strategies of Geobacter and Shewanella biofilm engineering for improving electron transfer efficiency to obtain enhanced BES performance.
Introduction
Dissimilatory metal-reducing microorganisms (DMRM) can oxidize organic matters or hydrogen and then transfer the derived electrons to extracellular metal-containing minerals for respiration under anoxic conditions (Lovley et al., 1987; Lovley and Phillips, 1988). DMRM, ranging from Gram-negative and positive bacteria to archaea, inhabit various environments where they directly contribute to biogeochemical cycling of carbon and metals, such as iron (Fe) and manganese (Mn) (Lovley and Phillips, 1988; Myers and Nealson, 1988; Lovley et al., 1989). Geobacter metallireducens and Shewanella oneidensis were the first isolated organisms that were unequivocally shown the ability to gain energy for their growth through Fe(III) or Mn(IV) reduction (Lovley et al., 1987; Myers and Nealson, 1988). Since these pioneering studies in late 1980s, it has been found that most organisms conserving energy to support their growth by the reduction of Fe(III) or Mn(IV) fall within Geobacter spp. that predominate over other DMRM and play important roles in the oxidation of organic compounds in subsurface environments. On the other hand, the Gram-negative bacteria Shewanella spp. are facultative anaerobic microbes capable of using a broad range of electron donors and acceptors. They are found in different ecological niches, such as marine and freshwater ecosystems (Heidelberg et al., 2002). Over the past 40 years, thorough and rigorous studies have made Geobacter and Shewanella spp. the two widely investigated groups of DMRM.
Geobacter and Shewanella spp. have been of scientific interest not only due to their involvement in a range of biogeochemical cycles, but also the potential of harnessing their electron transfer activities for a range of biotechnological applications (Lloyd et al., 2003). For example, in addition to mineral-based acceptors, G. metallireducens can transfer electrons directly to methanogens, such as Methanotrix harundinacea and Methanosarcina barkeri, for methane production in wetlands and anaerobic digesters (Summers et al., 2010; Rotaru et al., 2014). This process is referred to as direct interspecies electron transfer that permits the conversion of wastes to methane. Another broadly studied attributes of Geobacter and Shewanella spp. are their capability to transfer electrons to or accept electrons from electrodes (Bond and Lovley, 2003; Gregory et al., 2004; Ueki et al., 2018). Microorganisms with the ability of exocellular electron transfer to electrodes are known as exoelectrogens (Logan, 2009; Logan et al., 2019). Meanwhile, they also have been defined as electrochemically active bacteria (Chang et al., 2006), anode respiring bacteria (Rittmann et al., 2008) and electricigens (Lovley, 2006a). This group of microorganisms are widely used in bioelectrochemical systems (BESs) for various biotechnological applications (Nealson and Rowe, 2016; Kumar et al., 2017). For example, exoelectrogens show promise as biocatalysts for efficient conversion of a wide range of organic wastes and renewable biomass to electricity (i.e., microbial fuel cells, MFCs) (Rabaey and Verstraete, 2005; Lovley, 2006b; Logan, 2009). It should be pointed out that the highest current densities to date are produced by mixed cultures that are often dominated by Geobacter spp. (Koch and Harnisch, 2016; Logan et al., 2019). Moreover, Geobacter sulfurreducens KN400 generated one of the highest known power densities in pure cultures (Yi et al., 2009), highlighting the promise of Geobacter spp. in energy applications. Thus, Geobacter and Shewanella spp. have been used in BESs for various environmental and energy applications (Koch and Harnisch, 2016; TerAvest and Ajo-Franklin, 2016; Li et al., 2018; Logan et al., 2019).
Most biotechnological applications of Geobacter and Shewanella spp. associate with the formation and conductivity of Geobacter and Shewanella biofilms (Hu et al., 2019; Mukherjee et al., 2020). A biofilm is a well-organized 3-dimensional (3D) architecture in which cells are enclosed by a matrix of self-produced extracellular polymeric substances (EPS) (Wingender et al., 1999). Geobacter and Shewanella biofilms are electrically conductive, which is conferred by matrix-associated electroactive components such as c-type cytochromes (c-Cyts) and nanowires (Borole et al., 2011; Bond et al., 2012; Snider et al., 2012; Xu et al., 2018). The electroactivity of these redox molecules permits the cells to grow at a distance from electrodes. To improve Geobacter and Shewanella biofilm-mediated bioprocesses for various biotechnological applications, engineering their biofilms is a promising strategy.
The state-of-the-art understanding of biofilm biology has enabled biofilm engineering for desirable performances in practical applications. This review first briefly discusses extracellular electron transfer of Geobacter and Shewanella and compares the roles of planktonic and biofilm-forming cells in BESs. We then review biofilm biology with the focus on biofilm development, biofilm matrix, heterogeneity in biofilm and signaling regulatory systems that mediate formation of Geobacter and Shewanella biofilms. Finally, we discuss strategies of biofilm engineering for improving electron transfer performances of Geobacter and Shewanella biofilms in BESs.
Extracellular Electron Transfer of Geobacter and Shewanella: Biofilm vs. Planktonic Exoelectrogens
Microbial electron exchange with extracellular substrates, such as electrodes in BESs, is often called microbial extracellular electron transfer (EET). The most well characterized microbial EET pathway is the Mtr pathway of S. oneidensis MR-1. S. oneidensis MR-1 transfers electrons from the quinone/quinol pool in the cytoplasmic membrane to the bacterial surface through redox and structural proteins, including the inner membrane c-Cyt CymA, the periplasmic c-Cyts Fcc3 and STC, and the outer membrane porin-c-Cyt complex MtrCAB (Figure 1A) (Nealson et al., 2002; Shi et al., 2016; Jiang et al., 2019). Eventually, electrons are transferred from the bacterial surface to extracellular acceptors directly via the outer membrane c-Cyts or via nanowires (Figures 1A,B), or indirectly via self-secreted electron mediator flavins (Figure 1C) (Bretschger et al., 2007; Marsili et al., 2008; Meitl et al., 2009; Coursolle et al., 2010; Kotloski et al., 2013; Okamoto et al., 2013; Xu et al., 2018). Shewanella nanowires are the extensions of the outer membrane and periplasm in which the cell surface c-Cyts MtrC and OmcA are the key players for long-distance electron transfer (Gorby et al., 2006; Pirbadian et al., 2014). Another widely studied EET pathway is Pcc EET pathways of G. sulfurreducens. Like Mtr pathway of S. oneidensis MR-1, Pcc EET pathways transfer electrons from quinone/quinol pool in the cytoplasmic membrane to the bacterial surface using a series of multiheme c-Cyts (Liu Y. et al., 2014; Shi et al., 2014; Liu Y. et al., 2015; Shi et al., 2016). It should be noted that nanowires produced by G. sulfurreducens are important for long-range EET (Reguera et al., 2006; Reguera, 2018; Lovley and Walker, 2019). G. sulfurreducens nanowires were previously thought to be electrically conductive type IV Pili assembled by PilA protein. (Reguera et al., 2005; Malvankar and Lovley, 2014; Lovley, 2017; Tan et al., 2017; Lovley and Walker, 2019; Malvankar et al., 2012b). However, it is based on genetic studies that pilA mutant did not produce filaments (Reguera et al., 2005), which is lack of a direct evidence. By using cryo-electron microscopy with atomic force microscopy, Wang et al. (2019), Yalcin et al. (2020) determined composition and structure of nanowires and found that nanowires are polymerized by c-Cyts OmcS and OmcZ. They further correlated their protein structures with functions including their conductivity and stiffness. The results revealed that OmcS nanowires show 100-fold higher conductivity than noncytochrome filaments, whereas OmcZ nanowires exhibit 1000-fold higher conductivity and 3-fold higher stiffness than OmcS nanowires. Moreover, they recently demonstrated the conductivity of pili is very low based on the predicted and observed results, as well as found that OmcS and OmcZ for ΔpilA-N cells remain in the periplasm rather than extracellular fraction. Thus, they proposed that Geobacter pili exhibit secretory behaviour (Gu et al., 2021). On the other hand, a recent study by Liu et al. (2021) demonstrated that G. sulfurreducens produces abundant electrically conductive pili but expresses few OmcS-based filaments by directly examining filaments emanating from cells. The debate on the composition of nanowires and their role in long-range electron transfer continues. Moreover, self-secreted flavins contribute to Geobacter EET as cofactors by binding to outer membrane c-Cyts when electrodes are used as electron acceptors (Okamoto et al., 2014a; Okamoto et al., 2014b; Huang et al., 2018; Thirumurthy and Jones, 2020). Based on these studies, two microbial EET pathways have been proposed: direct EET via c-Cyts or electrically conductive nanowires and indirect EET via exogenous or endogenous electron shuttles.
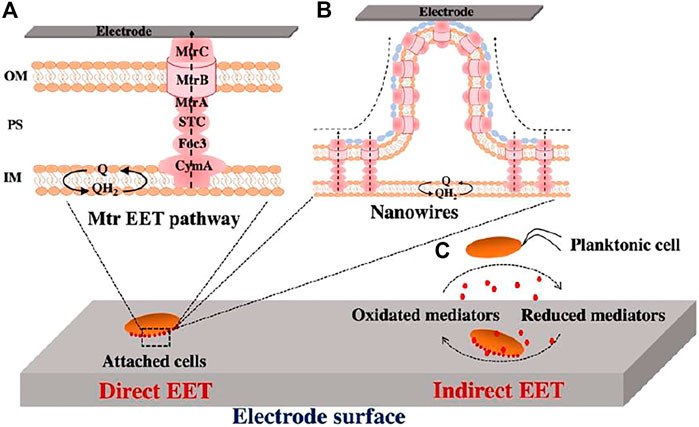
FIGURE 1. Extracellular electron transfer (EET) pathways of Shewanella oneidensis MR-1 to electrodes. (A) Direct electron transfer via Mtr pathway. (B) Direct electron transfer via the outer membrane and periplasm extensions (previously described as nanowires). (C) Indirect electron transfer via electron shuttle flavins. Q, quinone, QH2, quinol, IM, inner membrane, PS, periplasmic space, OM, outer membrane.
The exoelectrogens grown in BESs display two microbial lifestyles, i.e., biofilms on anodes and planktonic cells in anode chambers. Both biofilm cells and planktonic cells can transfer electrons to electrodes through direct EET or indirect EET (Marsili et al., 2008; Harris et al., 2010; Borole et al., 2011). Previous studies showed that S. oneidensis MR-1 can form a biofilm on the anode in lactate-fed MFCs (McLean et al., 2010; Watson and Logan, 2010; Roy et al., 2014), and both planktonic cells and biofilms synergistically contribute to current generation (Biffinger et al., 2007). In contrast, the replacement of the medium surrounding biofilm-coated electrodes rarely affects the electron transfer of Geobacter spp., indicating that indirect EET mediated by electron shuttles is not involved in the current generation of Geobacter MFCs (Bond et al., 2002; Bond and Lovley, 2003). The level of flavins in planktonic cultures of G. sulfurreducens is too low to function as electron shuttles for respiration (Okamoto et al., 2014a; Okamoto et al., 2014b). Moreover, characterization of metabolism in G. sulfurreducens by constraint-based modeling revealed that EET by direct contact for G. sulfurreducens has an energetic advantage over electron shuttling by flavins (Mahadevan et al., 2006). Thus, G. sulfurreducens requires direct electrical contact with the surface of the anode electrode for electron transfer (Bond and Lovley, 2003; Reguera et al., 2006). Additionally, previous studies showed that direct EET likely enables higher kinetic rates of electron transfer than the process solely depending on diffusion of electron mediators (Marsili et al., 2008; Srikanth et al., 2008; Marsili et al., 2010). Thus, the ability of an exoelectrogen to form a biofilm is one of key factors for increasing current generation in MFCs.
Biofilm Biology of Geobacter and Shewanella
Biofilm Development
The life cycle of a biofilm is a continuous, highly dynamic process. Biofilms develop through several stages including microbial attachment on surfaces or interfaces, biofilm maturation and biofilm dispersal (Costerton, 1999; O'Toole et al., 2000; Watnick and Kolter, 2000; Flemming et al., 2016). Similar to other biofilm-forming bacteria, exoelectrogens first physically contact and attach to the surfaces of external electron acceptors, such as metal particles, electrode surfaces, or partner bacteria. Attachment stages of Geobacter and Shewanella biofilms are mediated by functional flagellar system, type IV pilus system and extracellular DNA (eDNA) (Thormann et al., 2004; Gödeke et al., 2011a; Gödeke et al., 2011b; Cologgi et al., 2014; Bennett et al., 2016). Then, biofilms become mature, during which cells form a fixed EPS matrix (Allison, 2003; Flemming and Wingender, 2010). Different from that of nonelectrogenic bacteria, the biofilm matrix of Geobacter and Shewanella spp. is electrically conductive and contains extracellular electron transfer components, such as cytochromes and other electron mediators (Borole et al., 2011; Reguera, 2018; Xu et al., 2018). This is required for the growth of daughter cells or new cell layers at a distance from external electron accepters. The final stage of a biofilm life cycle is biofilm dispersal in which the planktonic cells are released from a mature biofilm, migrate and attach to new surfaces, and subsequently start a new life cycle (McDougald et al., 2012; Rumbaugh and Sauer, 2020).
Biofilm Matrix
Cells in a mature biofilm are enclosed in a self-produced EPS matrix that occupies most of biofilm biomass (Allison, 2003). EPS matrix is composed of soluble, gel-forming components such as polysaccharides, proteins and extracellular DNA (eDNA) (Flemming and Wingender, 2010), as well as insoluble components including cellulose, amyloid, flagella and pili (Hobley et al., 2015). By filling and shaping the space between the cells of biofilms, EPS molecules offer a microenvironment for biofilm cells and provide a structural scaffold for biofilm stability (Persat et al., 2015). EPS matrix may also form an external digestion system due to its ability to accumulate enzymes (Tielen et al., 2010). These enzymes, such as protein-, polysaccharide-, oligosaccharide-, or lipid-degrading enzymes and phosphomonoesterases, can degrade diverse matrix components or other nutrients (Allison, 2003; Flemming and Wingender, 2010). After degradation, the products in close proximity to cells are efficiently captured and taken up by cells. Another important role of EPS matrix is to serve as a protective shield against toxins, antimicrobials and desiccation (Flemming and Wingender, 2010). Thus, the matrix is a functionally crucial component of microbial biofilms.
Shewanella matrix contains extracellular polysaccharides, proteins and eDNA (Figure 2) (Mukherjee et al., 2020). Proteomics analysis of EPS extracted from Shewanella sp. HRCR-1 biofilm found the presence of 58 extracellular and outer membrane proteins, such as redox active proteins MtrC and OmcA (Cao et al., 2011). The presence of c-Cyts in the biofilm matrix of S. oneidensis MR-1 is further confirmed by a recent study that detected the electrochemical activities and redox peaks of c-Cyts using voltammetry measurement (Xiao et al., 2017). This study also clearly showed the redox peaks of flavins in EPS of S. oneidensis MR-1. The EPS matrix of Shewanella biofilm likely affects extracellular electron transfer through biofilm via redox proteins and electron shuttles.
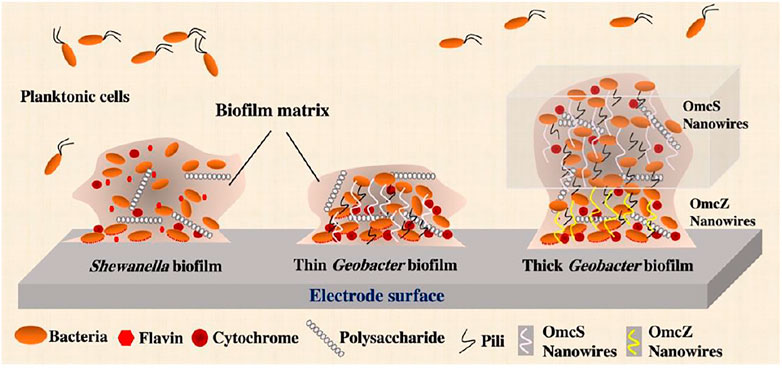
FIGURE 2. The complex structure of Shewanella and Geobacter biofilms in a bioelectrochemical system. Microcolonies in the mature biofilm are characterized by an extracellular polymeric substances (EPS) matrix consisting of polysaccharides, proteins and extracellular DNA (eDNA). Different from that of nonelectrogenic bacteria, the biofilm matrix of exoelectrogens is electrically conductive and contains extracellular electron transfer components, such as cytochromes and other electron mediators in Shewanella EPS matrix, as well as cytochromes, pili and OmcS/OmcZ-based nanowires in Geobacter EPS matrix. Because of higher abundance of cytochromes that form conductive nanowires, Geobacter biofilm is much more conductive and thicker than Shewanella biofilm.
It has been reported that Geobacter spp. are among the major phylotypes in the attached fraction of environment samples (Dong et al., 2021). Geobacter biofilms have an electroactive EPS matrix with matrix-associated c-Cyts and pili that spatially organize cells well for growth and electron transfer to mineral oxides and electrodes (Figure 2). The studies that showed the reversible reduction and oxidation of the cytochromes demonstrated their functionality as electron carriers for electron transfer in electricity-producing G. sulfurreducens biofilms (Liu et al., 2011; Liu and Bond, 2012). The roles of outer membrane cytochromes in biofilm electroactivity were further investigated by the studies that constructed diverse cytochrome-defective mutants and tested their current outputs. Deleting outer membrane c-Cyt gene omcE or omcB from the G. sulfurreducens genome has no effect on electricity outputs of MFCs (Holmes et al., 2006; Nevin et al., 2009; Richter et al., 2009). Outer membrane c-Cyt OmcS is required for optimum current production in fuel cells in which electrochemical restrictions limit electricity outputs (Holmes et al., 2006), whereas deleting omcS has no impact on current production when there is no electrochemical limitation (Nevin et al., 2009). However, the genetic deletion of OmcZ leads to a significant reduction in biofilm electroactivity and electricity output (Nevin et al., 2009; Richter et al., 2009). OmcZ preferentially locates at or closer (<10 µm) to the electrode surface in the anodic G. sulfurreducens biofilm (Inoue et al., 2011), suggesting a critical role of OmcZ in the last step of EET, i.e., electron transfer from biofilms to electrodes. Moreover, a recent study discovered that the production of OmcZ can be simulated by electric field (Yalcin et al., 2020). OmcZ has been identified to be an essential matrix-associated c-Cyt for electron transfer in thick biofilms. Other matrix-associated c-Cyts may be required, but compensatory effects observed in encoding gene-defective strains make their genetic identification challenging (Kim et al., 2005; Mehta et al., 2005). For example, the compensatory effect of OmcZ nanowires probably makes ΔomcS G. sulfurreducens biofilms show high current production when the biofilms mature. Thus, OmcZ and OmcS nanowires may have overlapping roles in G. sulfurreducens MFC performance (Nevin et al., 2009). Based on these previous studies, Yelcin et al. proposed a model that OmcS nanowires play an important role in EET during the early stage of biofilm growth, while the high conductivity of OmcZ nanowires enables G. sulfurreducens to form thick biofilms on the electrode surface of MFC (Figure 2) (Yalcin and Malvankar, 2020). On the other hand, Steidl et al. (2016) found that G. sulfurreducens pili have critical impacts on the formation of relatively thick (>10 µm) biofilms on the electrodes.
Heterogeneity in Geobacter and Shewanella Biofilms
Steep chemical gradients exist in the EPS matrix of microbial biofilms (Chang et al., 2015). The microbial cells living in these heterogenous environments display different metabolic activities for their adaption to different local environmental conditions by expressing distinct set of genes (Stewart and Franklin, 2008). Cao et al. monitored the spatiotemporal metabolic responses of metabolically active S. oneidensis MR-1 biofilms to contaminant exposure, which demonstrated that metabolic responses were spatially stratified based on local microenvironments (Cao et al., 2012). In an aerobic heterotrophic biofilm, organisms are stratified based on oxygen and nutrient availabilities, which become depleted in the lower layers of the biofilm when the consumption rates of oxygen and nutrients in the upper layers of the biofilm are faster than their diffusion rates (Stewart, 2003). The cells in the upper layers of the biofilm often have higher metabolic activities because they have optimal access to nutrients and electron acceptors.
Unlike a biofilm on a surface, the cells in the upper layers of the anode-respiring biofilm have optimal access to soluble electron donors, nutrients and buffering agents, whereas cell layers close to electrodes have optimal access to electron acceptors (Bond et al., 2002). Optimizing electrode-respiring biofilm systems for practical applications greatly requires the understanding of their internal chemical and electrical fluxes including nutrient diffusion, electron transfer and the movement of protons and pH-buffering compounds (Renslow R. S. et al., 2013). Babauta et al. used microelectrodes to quantify pH and redox potential variations in electrode-respiring S. oneidensis MR-1 and G. sulfurreducens biofilms (Babauta et al., 2011; Babauta et al., 2012). Their results demonstrated that observable pH variation was not detected, and that the redox potential decreased toward the bottom of the biofilm in the S. oneidensis MR-1 biofilm. It implies that there is no proton transfer limitation in S. oneidensis MR-1 biofilms. On the other hand, they found that pH continued to decrease in the G. sulfurreducens biofilm through different growth phase, suggesting that pH was not always a limiting factor for biofilm growth. Physiological stratification in electricity-producing G. sulfurreducens biofilms was further investigated by the study showing that the progressive accumulation of cells did not contribute to current production after a maximal current was achieved (Schrott et al., 2014). Furthermore, Chadwick et al. revealed metabolic stratification within current-producing G. sulfurreducens biofilms using stable isotope probing coupled to nanoscale secondary ion mass spectrometry (nanoSIMS) (Chadwick et al., 2019). The results demonstrated that cells at the anode surface were most active, and that cell activity decreased with an increase in distance from electrodes. This work also found that the cells nearest the electrode could keep their maximum growth rate in 80-μm-thick biofilms, suggesting that nutrient or buffer diffusion into the biofilm was not rate-limiting. In another interesting study, azo dye was used as an alternative electron acceptor in MFCs with Shewanella decolorationis (Yang et al., 2015). They observed electron acceptor-dependent physiological stratification within anode biofilm in the presence of multiple electron acceptors. Collectively, these findings demonstrate the physicochemical and physiological stratifications within electrode-respiring biofilms of Geobacter and Shewanella spp. It helps improve understanding of the electron transfer mechanism within anode-respiring biofilm.
Cyclic Dinucleotide Signaling System in the Development of Geobacter and Shewanella Biofilms
Bis-(3′-5′)-cyclic dimeric GMP (c-di-GMP) is a key regulator in biofilm formation (Hengge, 2009). It is respectively synthesized and degraded by diguanylate cyclases (DGCs) containing GGDEF domain and phosphodiesterases (PDEs) harboring EAL/HD-GYP domain (Figure 3) (Jenal et al., 2017). The dynamic change of c-di-GMP level is converted ultimately into specific cellular responses by mediating the responsive effectors, such as riboswitches and c-di-GMP receipting proteins (Römling et al., 2005; Hengge, 2009).
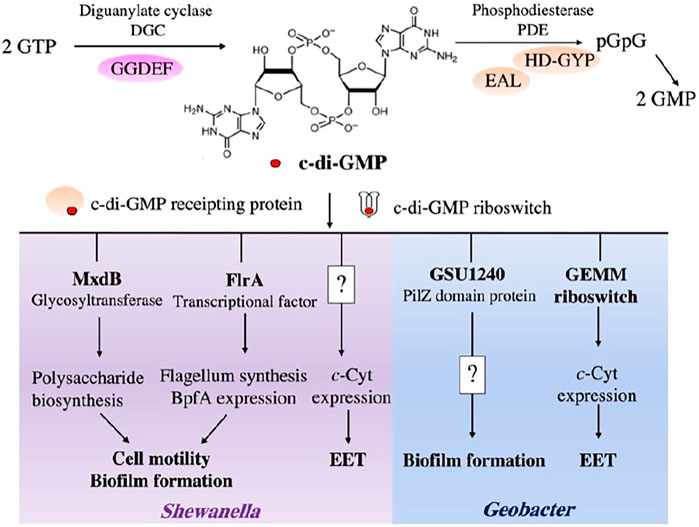
FIGURE 3. c-di-GMP signaling in Shewanella and Geobacter cells. The intracellular level of c-di-GMP is mediated by DGCs with GGDEF domains and PDEs with EAL/HD-GYP domains. In Shewanella spp., the c-di-GMP signaling network coordinates the biosynthesis of extracellular polysaccharides through interaction with MxdB glycosyltransferase, a polysaccharide biosynthetic enzyme. Flagellum synthesis and BpfA expression are controlled by c-di-GMP through its responsive transcriptional factor, FlrA. c-di-GMP also positively regulates c-cytochrome (c-Cyt) expression in S. oneidensis MR-1, but molecular mechanism is unclear. In Geobacter spp., c-di-GMP regulates biofilm formation and c-Cyt expression using PilZ domain-containing protein and GEMM riboswitches, respectively. How c-di-GMP regulates formation of Geobacter biofilm is currently unknown.
Environmental cues, such as nitric oxide, affect biofilm formation through the turnover of c-di-GMP signaling network, which has been discovered in both Shewanella woodyi and S. oneidensis (Liu et al., 2012; Plate and Marletta, 2012). It indicates that c-di-GMP is a crucial intracellular regulator for controlling formation of Shewanella biofilm. Moreover, a high abundance of genes encoding DGCs and PDEs in Shewanella genomes further emphasize the importance of c-di-GMP signaling network in the regulation of physiological processes of Shewanella spp. (Mukherjee et al., 2020). For example, S. oneidensis are predicted to carry 51 GGDEF-containing proteins, 27 EAL-containing proteins, and 20 proteins that contain both GGDEF and EAL/HD-GYP. In Shewanella spp., c-di-GMP mediates biofilm formation by interacting with polysaccharide biosynthetic enzymes (e.g., MxdB glycosyltransferase) to activate the biosynthesis of extracellular polysaccharides (Gambari et al., 2019). Previous study showed that c-di-GMP-responsive transcriptional factor FlrA controls the expression of genes for the synthesis of flagella and an adhesin protein BpfA (Figure 3) (Cheng et al., 2017; Gao et al., 2018). In addition to mediating the biofilm development, a recent study showed that c-di-GMP signaling network regulates the expression of c-Cyts, such as CymA, OmcA and MtrCAB, in S. oneidensis MR-1, which provides new insights into the physiological functions of c-di-GMP in Shewanella spp. (Ng et al., 2020) (Figure 3). Notably, a cyclic adenosine 3′,5′-monophosphate (cAMP) also commonly exists in Shewanella spp. Previous study demonstrated that cAMP-CRP (cyclic adenosine 3′,5′-monophosphate receptor protein) regulatory system regulates multiple bidirectional EET-related pathways (Saffarini et al., 2003; Charania et al., 2009). Collectively, cyclic dinucleotides (CDNs) regulate both Shewanella biofilm formation and the expression of EET-related genes or pathways, such as c-Cyts and flavin biosynthesis pathway.
Numerous genes that encode GGDEF, EAL, and both GGDEF and EAL/HD-GYP domain-containing proteins are found in Geobacter genomes. Deletion of esnD gene encoding a DGC reduces c-di-GMP levels in the cells of G. sulfurreducens. This disrupts the growth of G. sulfurreducens biofilm on −0.1 V vs. SHE electrodes, and decreases the current levels (Hallberg et al., 2019). Leang et al. deleted the gene GSU1240 of G. sulfurreducens, which was predicted to encode a protein with the PilZ domain (Figure 3). They found that the GSU1240-deficient mutant formed a highly cohesive and more conductive biofilm and produced 70% higher power densities than the wild-type strain (Leang et al., 2013). Moreover, Tremblay et al. (2011) found that a nucleotide insertion in a GEMM (Genes for the Environment, for Membranes and for Motility) riboswitch of G. sulfurreducens increased the expression of its downstream pgcA gene. This results in an enhanced Fe(III) reduction rate (Figure 3). GEMM riboswitch is a typical c-di-GMP binding riboswitch. Similar to G. sulfurreducens, the G. metallireducens genome also contains GEMM riboswitches and a high abundance of genes encoding the proteins with GGDEF, EAL, and both GGDEF and EAL domain (Kellenberger et al., 2015), indicating the important role of c-di-GMP signaling network in Geobacter spp. Despite these advances, our understanding of how c-di-GMP impacts the formation of Geobacter biofilm is far from complete.
Geobacter spp. produce another CDNs, i.e., cyclic AMP-GMP (cGAMP or 3′, 3′-cGAMP). Thirty years ago, the GGDEF domain-containing enzymes were considered as c-di-GMP synthases. However, Hallberg et al. recently found that the hybrid promiscuous GGDEF enzyme GacA specifically produced cGAMP in G. sulfurreducens (Hallberg et al., 2019). Moreover, GEMM riboswitches preferentially recognize cGAMP (Kellenberger et al., 2015; Nelson et al., 2015). GEMM riboswitches are conserved upstream of EET-associated genes, such as omcST, pilMNPPQ and omcAHG in G. sulfurreducens (Hallberg et al., 2019). These observations suggest a possible role of cGAMP in regulating EET process of G. sulfurreducens. Hallberg et al. found that the ΔgacA deletion strain of G. sulfurreducens was defective in the reduction of insoluble Fe(III) oxide particles, demonstrating the importance of cGAMP in the growth of G. sulfurreducens with Fe(III) oxides as electron acceptors (Hallberg et al., 2019).
Quorum Sensing Systems in the Development of Geobacter and Shewanella Biofilms
Microbes or microbial communities can synchronize behaviors in response to population density by detecting the accumulation of self-produced extracellular chemical signals. This process is known as quorum sensing (QS). A high cell density allows accumulation of the signals in a local environment to the levels that are required to activate signal-responsive transcriptional factors via specific binding. The activated transcriptional factors then regulate the expressions of the genes with QS responsive promoters (Mukherjee and Bassler, 2019; Mukherjee et al., 2020). Quorum sensing occurs in both Gram-positive and negative bacteria via a diversity of chemical signals typically classified to acyl homoserine lactones (AHLs), fautoinducer-2 (AI2), diffusible signal factor (DSF) and peptides (Papenfort and Bassler, 2016). Abundant literatures show that QS participates in different stages of biofilm development for different species (O'Toole et al., 2000; Flemming et al., 2016; Rumbaugh and Sauer, 2020).
One of the most well-studied QS system in Gram-negative bacteria are AHL-based QS system that is characterized by two core proteins, the LuxI-type protein (i.e., the AHL synthase), and the LuxR-type protein (i.e., the AHL-responsive transcriptional factor) (Papenfort and Bassler, 2016). LuxI and LuxR homologs were found in Geobacter uraniireducens genome (Gelencsér et al., 2012). Chen et al. found that the addition of endogenous AHLs enhanced the electroactivity of a mix-culture biofilm grown on the anodes of MFCs most likely by increasing the relative abundance of electroactive bacteria assigned to Geobacter spp. (Chen et al., 2017). The role of AHL signal in a pure culture of Geobacter spp. was further investigated. The results showed that the detectable self-secreted AHLs of Geobacter soli GSS01 enhanced the formation and electrochemical activity of the biofilm grown on the anode of MFC. Exogenous addition of AHLs further facilitated EPS production (Jing et al., 2019). They also showed that AHL addition increased the biomass, cell viability and EPS abundance of G. soli GSS01 biofilm grown on the cathode. Their studies demonstrated, for the first time, the critical role of AHL-type signal in the formation and electroactivity of Geobacter biofilm (Jing et al., 2019). Moreover, AHL was detected in cocultured aggregates of G. metallireducens and G. sulfurreducens, indicating AHL-based QS system may regulate Geobacter syntrophic growth and aggregation (Wei et al., 2017).
The QS systems in Shewanella spp. are well summarized in a recent review (Mukherjee et al., 2020). Briefly, several LuxR homologous genes are predicted in the genomes of Shewanella spp. including S. woodyi MS32 and S. baltica (Li J. et al., 2020; Hayek et al., 2020). The genome of S. oneidensis is predicted to contain seven putative LuxR family proteins. However, they are not LuxR-type QS transcriptional factors (Learman, 2008). Different from Geobacter spp., detectable AHL signals are rarely present in Shewanella spp. (Zhang et al., 2017; Mukherjee et al., 2020). Moreover, there has been no evidence showing the involvement of QS systems in formation of Shewanella biofilm.
Geobacter and Shewanella Biofilm Engineering for Energy Applications
EPS Matrix-Targeted Biofilm Engineering for Electricity Generation in MFCs
MFCs show potential to power electronic devices in remote environments such as sea-floors and to remove organic wastes from contaminated areas in conjunction of electricity generation (Lovley, 2006b; Logan, 2009). But most perceived practical applications are limited by the low current output of MFC. The previous study has shown that the conductivity of the biofilm grown on anodes greatly affected G. sulfurreducens MFC performance (Malvankar et al., 2012a). The conductivity through G. sulfurreducens biofilm is mainly attributed to cytochromes and nanowires that mediate the short- and long-range electron transfer between cells and electrodes (Reguera et al., 2006; Richter et al., 2009; Malvankar et al., 2011). The expression of G. sulfurreducens pili in Pseudomonas aeruginosa resulted in a dramatically increased bioelectricity output of P. aeruginosa MFCs (Liu et al., 2019a). In contrast, Liu et al. expressed P. aeruginosa type IV Pili into G. sulfurreducens, resulting in the decreased electricity output (Liu X. et al., 2014). Yi et al. used a selective strategy to obtain a strain of G. sulfurreducens KN400 that showed a lower internal resistance and generated a higher electricity output than G. sulfurreducens DL-1. The increased electricity output is due to the increased production of pili (Yi et al., 2009). Additionally, the expression of flagella enables G. sulfurreducens KN400 to form a thicker biofilm on the anode as compared to G. sulfurreducens DL-1 without flagella. Although they are nonconductive, flagella also contribute to the conductivity of G. sulfurreducens biofilm. Liu and his collaborators found that flagella can act as Geobacter biofilm scaffolds to stabilize the biofilm and anchor extracellular cytochromes with an orderly arrangement, which facilitates extracellular electron transfer (Liu et al., 2019b). In a recent study, overexpression of GSU1501 (a gene for ATP-dependent exporter within an operon of polysaccharide biosynthesis) promoted polysaccharide secretion. The enhanced production of polysaccharides stimulated the formation of the biofilm with higher cell viability. This enhanced the current generation of G. sulfurreducens MFCs (Zhuang et al., 2020). Collectively, these studies demonstrate that biofilm matrix is a desirable target to engineer Geobacter biofilm for improving MFC performance.
Chemical Signaling-Targeted Biofilm Engineering for Electricity Generation in MFCs
The switch of bacterial lifestyle between planktonic and sessile modes is often mediated by sophisticated signaling networks that control intracellular levels of small molecules, such as c-di-GMP and quorum sensing signaling (Parsek and Greenberg, 2005; Valentini and Filloux, 2016). Therefore, the manipulations of these signaling networks can efficiently engineer biofilms to improve biofilm-based bioprocesses. Liu et al. and Hu et al. enhanced the formation of S. oneidensis biofilm on the anodes by overexpressing a DGC YdeH and a near-infrared-light responsive DGC BphS in S. oneidensis, respectively (Liu T. et al., 2015; Hu et al., 2017). The overexpression of these two genes enhanced the biofilm formation and increased the electricity generation of S. oneidensis MFCs. Cheng et al. functionally expressed the gene for an adenylate cyclase in S. oneidensis and used the engineered strain in microbial electrochemical systems (Cheng et al., 2020). The engineered strain exhibited the enhanced bidirectional EET capacities and the enhanced rate of reducing Cr(VI). The biofilm of G. sulfurreducens was engineered by the manipulation of c-di-GMP effectors. The deletion of the gene encoding a c-di-GMP binding effector resulted in the formation of a thicker and much more conductive G. sulfurreducens biofilm, which subsequently increased the power density of MFCs (Leang et al., 2013).
QS systems are also targeted to engineer S. oneidensis biofilms. Hu et al. used LuxR/AHL QS system originated from Vibrio fischeri to construct a gene circuit of AND logic gate. This circuit includes three parts: an isopropyl β-D- thiogalactopyranoside (IPTG)-inducible module, a QS module and an output module (Hu et al., 2015). IPTG inducible module is composed of an IPTG inducible promoter to control the expression of the transcriptional factor gene luxR. The expressed LuxR is activated by AHL to drive the expression of the gene, i.e., mtrA, in the output module. They introduced this gene circuit into S. oneidensis mtrA-deletion mutant and applied the engineered strain in MFCs. The presence of both IPTG and AHL activates MtrA expression, which restores the EET pathway and increases electricity output (Hu et al., 2015). In another study, the lux QS system was used to construct a population-state decision system for intelligently reprogramming the EET regulation system (Li F.-H. et al., 2020). The EET-related genes or pathways, such as cymA, shuttle exporter genes or flavin biosynthesis pathway, were located at the downstream of QS system and could be expressed at a certain population-state threshold. This system permitted the bacterial growth first and then shifted the metabolic flux form bacterial growth to EET. The engineered strain exhibited a higher EET efficiency and an improved pollutant reduction ability (Li F.-H. et al., 2020).
Artificial Biofilm
Probably because of nutrient diffusion limitation and insufficient interaction between bacteria and electrodes, the thickness of the biofilms on the anode is often limited to several tens of micron (Renslow R. et al., 2013). To circumvent this limitation, the biofilms are prepared by embedding microbial cells in a matrix made of synthetic polymers. The biofilms prepared in this way is often referred to as “artificial electroactive biofilm” (Adachi et al., 2008). The materials for the building up of an artificial electroactive biofilm are required to provide a high cell viability and ensure an efficient electrical contact between cells and materials. Yu et al. (2011) designed an artificial S. oneidensis biofilm with a conductive matrix interlocked with the conductive polymer chains of polypyrrole. Similarly, ready-to-use artificial bioelectrodes were constructed through the immobilization of G. sulfurreducens cells in composite materials associating with silica gel and carbon felt fibers (Estevez-Canales et al., 2018). In another study by Yong et al. (2014) a self-assembled 3D reduced graphene oxide (rGO)/S. oneidensis hybrid biofilm was formed by a “fishing” process where the GO nanosheets serving as nets caught the bacterial “fish.” Compared to a natural biofilm, this artificial electroactive biofilm exhibits a dramatical increase in power densities due to enhanced electrical conductivity of biofilms. However, the further improvement of BES performance is largely limited by sluggish biotic/abiotic interfacial electron exchange between cells and materials. To improve electron exchange between living cells and abiotic surfaces, a recent study developed a single cell electron collector. In this collector, FeS nanoparticles are confined in the periplasm and on the outer membrane surface to in-situ build an interconnected intact conductive layer on and cross the individual cell membrane (Yu et al., 2020). A single cell electron collector permits intimate association of the conductive nanoparticles with the cellular electron transfer machinery and maximizes the interfacial area between microbial cells and electrodes, which substantially enhanced high electron transfer rate and BES performance. Similarly, by introducing trans-outer-membrane silver nanoparticles, Cao et al. (2021) obtained the highest power density of Shewanella MFCs reported to date. Collectively, all of these results demonstrate a promising and new platform for wiring-up cells with abiotic interface.
Outlook
Biofilm is the prevalent lifestyle of microbial growth in natural and engineered environments (Flemming et al., 2016). The electroactive Geobacter and Shewanella biofilms have attracted increasing attention from academic and industrial communities because of their promise in various biotechnological applications, such as bioelectricity generation via MFCs. However, the low electricity output largely limits the practical applications of MFCs. The improved understanding of their EET molecular mechanisms and biofilm biology have greatly helped Geobacter and Shewanella biofilm engineering for improving MFC performance. The rapid advance of synthetic biology has also significantly helped the genetical modification of Geobacter and Shewanella spp. (Leang et al., 2013; Li et al., 2018; Cao et al., 2019). Furthermore, conductive metal nanoparticles have been used to improve bacteria loading capacity and the conductivities of electrodes and microbial cells. However, few studies couple genetically modified living cell catalysis and material catalysts. Thus, future investigation should focus on the genetically engineered cell-nanoparticle hybrid systems to further push the limits of MFCs.
Most studies of Geobacter and Shewanella biofilms focus on their biofilm formation on the anodes of MFCs for electricity generation. Although it is still at proof-of-principle stage, Geobacter and Shewanella spp. as the two best studied groups of exoelectrogens also show the promise to grow on the cathodes for the synthesis of valuable chemicals by using electrode-feeding electrons as reducing power and CO2 as a feedstock (i.e., electrobiosynthesis) (Dumas et al., 2008; Ross et al., 2011; Soussan et al., 2013; Ueki et al., 2018). This technology is attractive for both the alleviation of the world demands for fossil-based fuel and the decrease of CO2 emissions. The future research should also focus on the Geobacter and Shewanella biofilms on the cathodes. Previous studies demonstrated that both Geobacter and Shewanella cells express different cytochromes and regulate metabolism pathways when they are grown at different electrode potentials (Levar et al., 2014; Hirose et al., 2018). Thus, one of future directions is to investigate the mechanism of electron transfer from cathodes to the cells. Furthermore, the strategies to engineer Geobacter and Shewanella biofilms formed on the cathodes for the enhanced performance of electrobiosynthesis should also be explored.
Author Contributions
YH is credited with the conception and writing of the manuscript. YW, XH, and YS assisted figure preparation and manuscript proofreading. FL assisted manuscript proofreading and provided valuable suggestions. LS provided valuable edits, suggestions and feedback. All authors contributed to the article and approved the submitted version.
Funding
This work was supported by The National Key Research and Development Program of China (2018YFA0901303) and the National Natural Science Foundation of China (NSFC91851211, 41772363).
Conflict of Interest
The authors declare that the research was conducted in the absence of any commercial or financial relationships that could be construed as a potential conflict of interest.
Publisher’s Note
All claims expressed in this article are solely those of the authors and do not necessarily represent those of their affiliated organizations, or those of the publisher, the editors and the reviewers. Any product that may be evaluated in this article, or claim that may be made by its manufacturer, is not guaranteed or endorsed by the publisher.
Acknowledgments
We would like to thank our University for providing the literature services.
References
Adachi, M., Shimomura, T., Komatsu, M., Yakuwa, H., and Miya, A. (2008). A Novel Mediator-Polymer-Modified Anode for Microbial Fuel Cells. Chem. Commun. 7 (17), 2055–2057. doi:10.1039/B717773A
Allison, D. G. (2003). The Biofilm Matrix. Biofouling 19 (2), 139–150. doi:10.1038/nrmicro241510.1080/0892701031000072190
Babauta, J. T., Nguyen, H. D., and Beyenal, H. (2011). Redox and pH Microenvironments within Shewanella Oneidensis MR-1 Biofilms Reveal an Electron Transfer Mechanism. Environ. Sci. Technol. 45 (15), 6654–6660. doi:10.1021/es200865u
Babauta, J. T., Nguyen, H. D., Harrington, T. D., Renslow, R., and Beyenal, H. (2012). pH, Redox Potential and Local Biofilm Potential Microenvironments within Geobacter Sulfurreducens Biofilms and Their Roles in Electron Transfer. Biotechnol. Bioeng. 109 (10), 2651–2662. doi:10.1002/bit.24538
Bennett, R. R., Lee, C. K., De Anda, J., Nealson, K. H., Yildiz, F. H., O'Toole, G. A., et al. (2016). Species-dependent Hydrodynamics of Flagellum-Tethered Bacteria in Early Biofilm Development. J. R. Soc. Interf. 13 (115), 20150966. doi:10.1098/rsif.2015.0966
Biffinger, J. C., Pietron, J., Ray, R., Little, B., and Ringeisen, B. R. (2007). A Biofilm Enhanced Miniature Microbial Fuel Cell Using Shewanella Oneidensis DSP10 and Oxygen Reduction Cathodes. Biosens. Bioelectron. 22 (8), 1672–1679. doi:10.1016/j.bios.2006.07.027
Bond, D. R., Holmes, D. E., Tender, L. M., and Lovley, D. R. (2002). Electrode-reducing Microorganisms that Harvest Energy from marine Sediments. Science 295(5554), 483–485. doi:10.1126/science.1066771
Bond, D. R., and Lovley, D. R. (2003). Electricity Production by Geobacter Sulfurreducens Attached to Electrodes. Appl. Environ. Microbiol. 69 (3), 1548–1555. doi:10.1128/AEM.69.3.1548-1555.2003
Bond, D. R., Strycharz-Glaven, S. M., Tender, L. M., and Torres, C. I. (2012). On Electron Transport through Geobacter Biofilms. ChemSusChem 5 (6), 1099–1105. doi:10.1002/cssc.201100748
Borole, A. P., Reguera, G., Ringeisen, B., Wang, Z.-W., Feng, Y., and Kim, B. H. (2011). Electroactive Biofilms: Current Status and Future Research Needs. Energy Environ. Sci. 4 (12), 4813–4834. doi:10.1039/C1EE02511B
Bretschger, O., Obraztsova, A., Sturm, C. A., Chang, I. S., Gorby, Y. A., Reed, S. B., et al. (2007). Current Production and Metal Oxide Reduction by Shewanella Oneidensis MR-1 Wild Type and Mutants. Appl. Environ. Microbiol. 73 (21), 7003–7012. doi:10.1128/AEM.01087-07
Cao, B., Majors, P. D., Ahmed, B., Renslow, R. S., Silvia, C. P., Shi, L., et al. (2012). Biofilm Shows Spatially Stratified Metabolic Responses to Contaminant Exposure. Environ. Microbiol. 14 (11), 2901–2910. doi:10.1111/j.1462-2920.2012.02850.x
Cao, B., Shi, L., Brown, R. N., Xiong, Y., Fredrickson, J. K., Romine, M. F., et al. (2011). Extracellular Polymeric Substances from Shewanella Sp. HRCR-1 Biofilms: Characterization by Infrared Spectroscopy and Proteomics. Environ. Microbiol. 13 (4), 1018–1031. doi:10.111/1j.1462-2920.2010.02407.x10.1111/j.1462-2920.2010.02407.x
Cao, B., Zhao, Z., Peng, L., Shiu, H.-Y., Ding, M., Song, F., et al. (2021). Silver Nanoparticles Boost Charge-Extraction Efficiency in Shewanella Microbial Fuel Cells. Science 373 (6561), 1336–1340. doi:10.1126/science.abf3427
Cao, Y., Song, M., Li, F., Li, C., Lin, X., Chen, Y., et al. (2019). A Synthetic Plasmid Toolkit for Shewanella Oneidensis MR-1. Front. Microbiol. 10 (10), 410. doi:10.3389/fmicb.2019.00410
Chadwick, G. L., Jiménez Otero, F., Gralnick, J. A., Bond, D. R., and Orphan, V. J. (2019). Nanosims Imaging Reveals Metabolic Stratification Within Current-Producing Biofilms. Proc. Nat. Acad. Sci. 116 (41), 20716–20724. doi:10.1073/pnas.1912498116
Chang, I.-S., Moon, H.-S., Bretschger, O., Jang, J.-K., Park, H.-I., Nealson, K. H., et al. (2006). Electrochemically Active Bacteria (EAB) and Mediator-Less Microbial Fuel Cells. J. Microbiol. Biotechnol. 16 (2), 163–177.
Chang, Y.-W., Fragkopoulos, A. A., Marquez, S. M., Kim, H. D., Angelini, T. E., and Fernández-Nieves, A. (2015). Biofilm Formation in Geometries with Different Surface Curvature and Oxygen Availability. New J. Phys. 17 (3), 033017–033026. doi:10.1088/1367-2630/17/3/033017
Charania, M. A., Brockman, K. L., Zhang, Y., Banerjee, A., Pinchuk, G. E., Fredrickson, J. K., et al. (2009). Involvement of a Membrane-Bound Class III Adenylate Cyclase in Regulation of Anaerobic Respiration in Shewanella Oneidensis MR-1. J. Bacteriol. 191 (13), 4298–4306. doi:10.1128/JB.01829-08
Chen, S., Jing, X., Tang, J., Fang, Y., and Zhou, S. (2017). Quorum sensing Signals Enhance the Electrochemical Activity and Energy Recovery of Mixed-Culture Electroactive Biofilms. Biosens. Bioelectron. 97 (15), 369–376. doi:10.1016/j.bios.2017.06.024
Cheng, Y.-Y., Wu, C., Wu, J.-Y., Jia, H.-L., Wang, M.-Y., Wang, H.-Y., et al. (2017). FlrA Represses Transcription of the Biofilm-Associated bpfA Operon in Shewanella Putrefaciens. Appl. Environ. Microbiol. 83 (4), e02410–02416. doi:10.1128/AEM.02410-16
Cheng, Z. H., Xiong, J. R., Min, D., Cheng, L., Liu, D. F., Li, W. W., et al. (2020). Promoting Bidirectional Extracellular Electron Transfer of Shewanella Oneidensis MR‐1 for Hexavalent Chromium Reduction via Elevating Intracellular cAMP Level. Biotechnol. Bioeng. 117 (5), 1294–1303. doi:10.1002/bit.27305
Cologgi, D. L., Speers, A. M., Bullard, B. A., Kelly, S. D., Reguera, G., and Parales, R. E. (2014). Enhanced Uranium Immobilization and Reduction by Geobacter Sulfurreducens Biofilms. Appl. Environ. Microbiol. 80 (21), 6638–6646. doi:10.1128/AEM.02289-14
Costerton, J. W. (1999). Introduction to Biofilm. Int. J. Antimicrob. Agents 11 (3-4), 217–219. doi:10.1016/s0924-8579(99)00018-7
Coursolle, D., Baron, D. B., Bond, D. R., and Gralnick, J. A. (2010). The Mtr Respiratory Pathway Is Essential for Reducing Flavins and Electrodes in Shewanella Oneidensis. J. Bacteriol. 192 (2), 467–474. doi:10.1128/JB.00925-09
Dong, Y., Sanford, R. A., Connor, L., Chee-Sanford, J., Wimmer, B. T., Iranmanesh, A., et al. (2021). Differential Structure and Functional Gene Response to Geochemistry Associated with the Suspended and Attached Shallow Aquifer Microbiomes from the Illinois Basin, IL. Water Res. 202, 117431. doi:10.1016/j.watres.2021.117431
Dumas, C., Basseguy, R., and Bergel, A. (2008). Microbial Electrocatalysis With Geobacter Sulfurreducens Biofilm on Stainless Steel Cathodes. Electro. Acta 53 (5), 2494–2500. doi:10.1016/j.electacta.2007.10.018
Estevez-Canales, M., Pinto, D., Coradin, T., Laberty-Robert, C., and Esteve-Núñez, A. (2018). Silica Immobilization ofGeobacter Sulfurreducensfor Constructing Ready-To-Use Artificial Bioelectrodes. Microb. Biotechnol. 11 (1), 39–49. doi:10.1111/1751-7915.12561
Flemming, H.-C., Wingender, J., Szewzyk, U., Steinberg, P., Rice, S. A., and Kjelleberg, S. (2016). Biofilms: an Emergent Form of Bacterial Life. Nat. Rev. Microbiol. 14 (9), 563–575. doi:10.1038/nrmicro.2016.94
Flemming, H.-C., and Wingender, J. (2010). The Biofilm Matrix. Nat. Rev. Microbiol. 8 (9), 623–633. doi:10.1038/nrmicro2415
Gambari, C., Boyeldieu, A., Armitano, J., Méjean, V., and Jourlin-Castelli, C. (2019). Control of Pellicle Biogenesis Involves the Diguanylate Cyclases PdgA and PdgB, the C-Di-GMP Binding Protein MxdA and the Chemotaxis Response Regulator CheY3 in Shewanella Oneidensis. Environ. Microbiol. 21 (1), 81–97. doi:10.1111/1462-2920.14424
Gao, T., Shi, M., Gao, H., and O'Toole, G. (2018). Partially Reciprocal Replacement of FlrA and FlrC in Regulation of Shewanella Oneidensis Flagellar Biosynthesis. J. Bacteriol. 200 (7), e00796–00717. doi:10.1128/JB.00796-17
Gelencsér, Z., Choudhary, K. S., Coutinho, B. G., Hudaiberdiev, S., Galbáts, B., Venturi, V., et al. (2012). Classifying the Topology of AHL-Driven Quorum Sensing Circuits in Proteobacterial Genomes. Sensors 12 (5), 5432–5444. doi:10.3390/s120505432
Gödeke, J., Heun, M., Bubendorfer, S., Paul, K., and Thormann, K. M. (2011a). Roles of Two Shewanella Oneidensis MR-1 Extracellular Endonucleases. Appl. Environ. Microbiol. 77 (15), 5342–5351. doi:10.1128/AEM.00643-11
Gödeke, J., Paul, K., Lassak, J., and Thormann, K. M. (2011b). Phage-induced Lysis Enhances Biofilm Formation in Shewanella Oneidensis MR-1. Isme J. 5 (4), 613–626. doi:10.1038/ismej.2010.153
Gorby, Y. A., Yanina, S., McLean, J. S., Rosso, K. M., Moyles, D., Dohnalkova, A., et al. (2006). Electrically Conductive Bacterial Nanowires Produced by Shewanella Oneidensis Strain MR-1 and Other Microorganisms. Proc. Natl. Acad. Sci. 103 (30), 11358–11363. doi:10.1073/pnas.0604517103
Gregory, K. B., Bond, D. R., and Lovley, D. R. (2004). Graphite Electrodes as Electron Donors for Anaerobic Respiration. Environ. Microbiol. 6 (6), 596–604. doi:10.1111/j.1462-2920.2004.00593.x
Gu, Y., Srikanth, V., Salazar-Morales, A. I., Jain, R., O’Brien, J. P., Yi, S. M., et al. (2021). Structure of Geobacter Pili Reveals Secretory rather Than Nanowire Behaviour. Nature 597 (1), 430–434. doi:10.1038/s41586-021-03857-w
Hallberg, Z. F., Chan, C. H., Wright, T. A., Kranzusch, P. J., Doxzen, K. W., Park, J. J., et al. (2019). Structure and Mechanism of a Hypr GGDEF Enzyme that Activates cGAMP Signaling to Control Extracellular Metal Respiration. eLife Sci. 8, e43959. doi:10.7554/eLife.43959
Harris, H. W., El-Naggar, M. Y., Bretschger, O., Ward, M. J., Romine, M. F., Obraztsova, A. Y., et al. (2010). Electrokinesis Is a Microbial Behavior that Requires Extracellular Electron Transport. Proc. Natl. Acad. Sci. 107 (1), 326–331. doi:10.1073/pnas.0907468107
Hayek, M., Baraquet, C., Lami, R., Blache, Y., and Molmeret, M. (2020). The marine Bacterium Shewanella Woodyi Produces C8-HSL to Regulate Bioluminescence. Microb. Ecol. 79 (4), 865–881. doi:10.1007/s00248-019-01454-z
Heidelberg, J. F., Paulsen, I. T., Nelson, K. E., Gaidos, E. J., Nelson, W. C., Read, T. D., et al. (2002). Genome Sequence of the Dissimilatory Metal Ion-Reducing Bacterium Shewanella Oneidensis. Nat. Biotechnol. 20 (11), 1118–1123. doi:10.1038/nbt749
Hengge, R. (2009). Principles of C-Di-GMP Signalling in Bacteria. Nat. Rev. Microbiol. 7 (4), 263–273. doi:10.1038/nrmicro2109
Hirose, A., Kasai, T., Aoki, M., Umemura, T., Watanabe, K., and Kouzuma, A. (2018). Electrochemically Active Bacteria Sense Electrode Potentials for Regulating Catabolic Pathways. Nat. Commun. 9 (1), 1083. doi:10.1038/s41467-018-03416-4
Hobley, L., Harkins, C., MacPhee, C. E., and Stanley-Wall, N. R. (2015). Giving Structure to the Biofilm Matrix: an Overview of Individual Strategies and Emerging Common Themes. FEMS Microbiol. Rev. 39 (5), 649–669. doi:10.1093/femsre/fuv015
Holmes, D. E., Chaudhuri, S. K., Nevin, K. P., Mehta, T., Methe, B. A., Liu, A., et al. (2006). Microarray and Genetic Analysis of Electron Transfer to Electrodes in Geobacter Sulfurreducens. Environ. Microbiol. 8 (10), 1805–1815. doi:10.1111/j.1462-2920.2006.01065.x
Hu, Y., Mukherjee, M., and Cao, B. (2019). “Biofilm-Biology-Informed Biofilm Engineering for Environmental Biotechnology,” in Introduction to Biofilm Engineering (American Chemical Society), 59–82. doi:10.1021/bk-2019-1323.ch003
Hu, Y., Wu, Y., Mukherjee, M., and Cao, B. (2017). A Near-Infrared Light Responsive C-Di-GMP Module-Based and Logic Gate in Shewanella Oneidensis. Chem. Commun. 53 (10), 1646–1648. doi:10.1039/C6CC08584A
Hu, Y., Yang, Y., Katz, E., and Song, H. (2015). Programming the Quorum Sensing-Based and Gate in Shewanella Oneidensis for Logic Gated-Microbial Fuel Cells. Chem. Commun. 51 (20), 4184–4187. doi:10.1039/C5CC00026B
Huang, L., Tang, J., Chen, M., Liu, X., and Zhou, S. (2018). Two Modes of Riboflavin-Mediated Extracellular Electron Transfer in Geobacter Uraniireducens. Front. Microbiol. 9 (9), 2886–2894. doi:10.3389/fmicb.2018.02886
Inoue, K., Leang, C., Franks, A. E., Woodard, T. L., Nevin, K. P., and Lovley, D. R. (2011). Specific Localization of the C-type Cytochrome OmcZ at the Anode Surface in Current-Producing Biofilms of Geobacter Sulfurreducens. Environ. Microbiol. Rep. 3 (2), 211–217. doi:10.1111/j.1758-2229.2010.00210.x
Jenal, U., Reinders, A., and Lori, C. (2017). Cyclic Di-GMP: Second Messenger Extraordinaire. Nat. Rev. Microbiol. 15 (5), 271–284. doi:10.1038/nrmicro.2016.190
Jiang, Y., Shi, M., and Shi, L. (2019). Molecular Underpinnings for Microbial Extracellular Electron Transfer during Biogeochemical Cycling of Earth Elements. Sci. China Life Sci. 62 (10), 1275–1286. doi:10.1007/s11427-018-9464-3
Jing, X., Liu, X., Deng, C., Chen, S., and Zhou, S. (2019). Chemical Signals Stimulate Geobacter Soli Biofilm Formation and Electroactivity. Biosens. Bioelectron. 127, 1–9. doi:10.1016/j.bios.2018.11.051
Kellenberger, C. A., Wilson, S. C., Hickey, S. F., Gonzalez, T. L., Su, Y., Hallberg, Z. F., et al. (2015). GEMM-I Riboswitches from Geobacter Sense the Bacterial Second Messenger Cyclic AMP-GMP. Proc. Natl. Acad. Sci. USA 112 (17), 5383–5388. doi:10.1073/pnas.1419328112
Kim, B.-C., Leang, C., Ding, Y.-H. R., Glaven, R. H., Coppi, M. V., and Lovley, D. R. (2005). OmcF, a Putative C -Type Monoheme Outer Membrane Cytochrome Required for the Expression of Other Outer Membrane Cytochromes in Geobacter Sulfurreducens. J. Bacteriol. 187 (13), 4505–4513. doi:10.1128/JB.187.13.4505-4513.2005
Koch, C., and Harnisch, F. (2016). Is There a Specific Ecological Niche for Electroactive Microorganisms? ChemElectroChem 3 (9), 1282–1295. doi:10.1002/celc.201600079
Kotloski, N. J., Gralnick, J. A., and Newman, D. K. (2013). Flavin Electron Shuttles Dominate Extracellular Electron Transfer by Shewanella Oneidensis. mBio 4 (1), e00553–00512. doi:10.1128/mBio.00553-12
Kumar, A., Hsu, L. H.-H., Kavanagh, P., Barrière, F., Lens, P. N. L., Lapinsonnière, L., et al. (2017). The Ins and Outs of Microorganism-Electrode Electron Transfer Reactions. Nat. Rev. Chem. 1 (3), 0024–0032. doi:10.1038/s41570-017-0024
Leang, C., Malvankar, N. S., Franks, A. E., Nevin, K. P., and Lovley, D. R. (2013). Engineering Geobacter Sulfurreducens to Produce a Highly Cohesive Conductive Matrix with Enhanced Capacity for Current Production. Energ. Environ. Sci. 6 (6), 1901–1908. doi:10.1039/c3ee40441b
Learman, D. R. (2008). Shewanella Oneidensis MR-1 Cell-To-Cell Signaling and its Influences on Biogeochemical Processes. PhD thesis. Blacksburg (Virginia): Virginia Polytechnic Institute and State University
Levar, C. E., Chan, C. H., Mehta-Kolte, M. G., Bond, D. R., and Newman, D. K. (2014). An Inner Membrane Cytochrome Required Only for Reduction of High Redox Potential Extracellular Electron Acceptors. mBio 5 (6), e02034–02014. doi:10.1128/mBio.02034-14
Li, F.-H., Tang, Q., Fan, Y.-Y., Li, Y., Li, J., Wu, J.-H., et al. (2020a). Developing a Population-State Decision System for Intelligently Reprogramming Extracellular Electron Transfer in Shewanella Oneidensis. Proc. Natl. Acad. Sci. USA 117 (37), 23001–23010. doi:10.1073/pnas.2006534117
Li, F., Wang, L., Liu, C., Wu, D., and Song, H. (2018). Engineering Exoelectrogens by Synthetic Biology Strategies. Curr. Opin. Electrochemistry 10, 37–45. doi:10.1016/j.coelec.2018.03.030
Li, J., Yu, H., Yang, X., Dong, R., Liu, Z., and Zeng, M. (2020b). Complete Genome Sequence Provides Insights into the Quorum Sensing-Related Spoilage Potential of Shewanella Baltica 128 Isolated from Spoiled Shrimp. Genomics 112 (1), 736–748. doi:10.1016/j.ygeno.2019.05.010
Liu, N., Xu, Y., Hossain, S., Huang, N., Coursolle, D., Gralnick, J. A., et al. (2012). Nitric Oxide Regulation of Cyclic Di-GMP Synthesis and Hydrolysis in Shewanella Woodyi. Biochemistry 51 (10), 2087–2099. doi:10.1021/bi201753f
Liu, T., Yu, Y.-Y., Deng, X.-P., Ng, C. K., Cao, B., Wang, J.-Y., et al. (2015a). EnhancedShewanellabiofilm Promotes Bioelectricity Generation. Biotechnol. Bioeng. 112 (10), 2051–2059. doi:10.1002/bit.25624
Liu, X., Tremblay, P.-L., Malvankar, N. S., Nevin, K. P., Lovley, D. R., and Vargas, M. (2014a). A Geobacter Sulfurreducens Strain Expressing Pseudomonas aeruginosa Type IV Pili Localizes OmcS on Pili but Is Deficient in Fe(III) Oxide Reduction and Current Production. Appl. Environ. Microbiol. 80 (3), 1219–1224. doi:10.1128/AEM.02938-13
Liu, X., Walker, D. J. F., Nonnenmann, S. S., Sun, D., Lovley, D. R., and Papoutsakis, E. T. (2021). Direct Observation of Electrically Conductive Pili Emanating from Geobacter Sulfurreducens. mBio 12 (4), e02209–02221. doi:10.1128/mBio.02209-21
Liu, X., Wang, S., Xu, A., Zhang, L., Liu, H., and Ma, L. Z. (2019a). Biological Synthesis of High-Conductive Pili in Aerobic Bacterium Pseudomonas aeruginosa. Appl. Microbiol. Biotechnolapplied Microbiol. Biotechnol. 103 (3), 1535–1544. doi:10.1007/s00253-018-9484-5
Liu, X., Zhuo, S., Jing, X., Yuan, Y., Rensing, C., and Zhou, S. (2019b). Flagella Act as Geobacter Biofilm Scaffolds to Stabilize Biofilm and Facilitate Extracellular Electron Transfer. Biosens. Bioelectron. 146, 111748–111757. doi:10.1016/j.bios.2019.111748
Liu, Y., and Bond, D. R. (2012). Long-distance Electron Transfer by G. Sulfurreducens Biofilms Results in Accumulation of Reduced C-type Cytochromes. ChemSusChem 5 (6), 1047–1053. doi:10.1002/cssc.201100734
Liu, Y., Fredrickson, J. K., Zachara, J. M., and Shi, L. (2015b). Direct Involvement of ombB, omaB, and omcB Genes in Extracellular Reduction of Fe(III) by Geobacter Sulfurreducens PCA. Front. Microbiol. 6 (1075). doi:10.3389/fmicb.2015.01075
Liu, Y., Kim, H., Franklin, R. R., and Bond, D. R. (2011). Linking Spectral and Electrochemical Analysis to Monitor C-type Cytochrome Redox Status in Living Geobacter Sulfurreducens Biofilms. ChemPhysChem 12 (12), 2235–2241. doi:10.1002/cphc.201100246
Liu, Y., Wang, Z., Liu, J., Levar, C., Edwards, M. J., Babauta, J. T., et al. (2014b). A Trans‐outer Membrane Porin‐cytochrome Protein Complex for Extracellular Electron Transfer by G Eobacter Sulfurreducens PCA. Environ. Microbiol. Rep. 6 (6), 776–785. doi:10.1111/1758-2229.12204
Lloyd, J. R., Leang, C., Myerson, A. L. H., Coppi, M. V., Cuifo, S., Methe, B., et al. (2003). Biochemical and Genetic Characterization of PpcA, a Periplasmic C-type Cytochrome in Geobacter Sulfurreducens. Biochem. J. 369 (1), 153–161. doi:10.1042/bj20020597
Logan, B. E. (2009). Exoelectrogenic Bacteria that Power Microbial Fuel Cells. Nat. Rev. Microbiol. 7 (5), 375–381. doi:10.1038/nrmicro2113
Logan, B. E., Rossi, R., Ragab, A. a., and Saikaly, P. E. (2019). Electroactive Microorganisms in Bioelectrochemical Systems. Nat. Rev. Microbiol. 17 (5), 307–319. doi:10.1038/s41579-019-0173-x
Lovley, D. R. (2006a). Bug Juice: Harvesting Electricity with Microorganisms. Nat. Rev. Microbiol. 4 (7), 497–508. doi:10.1038/nrmicro1442
Lovley, D. R. (2017). Electrically Conductive Pili: Biological Function and Potential Applications in Electronics. Curr. Opin. Electrochemistry 4 (1), 190–198. doi:10.1016/j.coelec.2017.08.015
Lovley, D. R. (2006b). Microbial Fuel Cells: Novel Microbial Physiologies and Engineering Approaches. Curr. Opin. Biotechnol. 17 (3), 327–332. doi:10.1016/j.copbio.2006.04.006
Lovley, D. R., Phillips, E. J. P., and Lonergan, D. J. (1989). Hydrogen and Formate Oxidation Coupled to Dissimilatory Reduction of Iron or Manganese by Alteromonas Putrefaciens. Appl. Environ. Microbiol. 55 (3), 700–706. doi:10.1128/aem.55.3.700-706.1989
Lovley, D. R., and Phillips, E. J. P. (1988). Novel Mode of Microbial Energy Metabolism: Organic Carbon Oxidation Coupled to Dissimilatory Reduction of Iron or Manganese. Appl. Environ. Microbiol. 54 (6), 1472–1480. doi:10.1128/aem.54.6.1472-1480.1988
Lovley, D. R., Stolz, J. F., Nord, G. L., and Phillips, E. J. P. (1987). Anaerobic Production of Magnetite by a Dissimilatory Iron-Reducing Microorganism. Nature 330 (6145), 252–254. doi:10.1038/330252a0
Lovley, D. R., and Walker, D. J. F. (2019). Geobacter Protein Nanowires. Front. Microbiol. 10 (10), 2078–2095. doi:10.3389/fmicb.2019.02078
Mahadevan, R., Bond, D. R., Butler, J. E., Esteve-Nuñez, A., Coppi, M. V., Palsson, B. O., et al. (2006). Characterization of Metabolism in the Fe(III)-reducing Organism Geobacter Sulfurreducens by Constraint-Based Modeling. Appl. Environ. Microbiol. 72 (2), 1558–1568. doi:10.1128/AEM.72.2.1558-1568.2006
Malvankar, N. S., and Lovley, D. R. (2014). Microbial Nanowires for Bioenergy Applications. Curr. Opin. Biotechnol. 27, 88–95. doi:10.1016/j.copbio.2013.12.003
Malvankar, N. S., Tuominen, M. T., and Lovley, D. R. (2012a). Biofilm Conductivity Is a Decisive Variable for High-Current-Density Geobacter Sulfurreducens Microbial Fuel Cells. Energ. Environ. Sci. 5 (2), 5790–5797. doi:10.1039/C2EE03388G
Malvankar, N. S., Tuominen, M. T., and Lovley, D. R. (2012b). Lack of Cytochrome Involvement in Long-Range Electron Transport through Conductive Biofilms and Nanowires of Geobacter Sulfurreducens. Energ. Environ. Sci. 5 (9), 8651–8659. doi:10.1039/C2EE22330A
Malvankar, N. S., Vargas, M., Nevin, K. P., Franks, A. E., Leang, C., Kim, B.-C., et al. (2011). Tunable Metallic-like Conductivity in Microbial Nanowire Networks. Nat. Nanotech 6 (9), 573–579. doi:10.1038/nnano.2011.119
Marsili, E., Baron, D. B., Shikhare, I. D., Coursolle, D., Gralnick, J. A., and Bond, D. R. (2008). Shewanella Secretes Flavins that Mediate Extracellular Electron Transfer. Proc. Natl. Acad. Sci. 105 (10), 3968–3973. doi:10.1073/pnas.0710525105
Marsili, E., Sun, J., and Bond, D. R. (2010). Voltammetry and Growth Physiology of Geobacter Sulfurreducens Biofilms as a Function of Growth Stage and Imposed Electrode Potential. Electroanalysis 22 (7-8), 865–874. doi:10.1002/elan.200800007
McDougald, D., Rice, S. A., Barraud, N., Steinberg, P. D., and Kjelleberg, S. (2012). Should We Stay or Should We Go: Mechanisms and Ecological Consequences for Biofilm Dispersal. Nat. Rev. Microbiol. 10 (1), 39–50. doi:10.1038/nrmicro2695
McLean, J. S., Wanger, G., Gorby, Y. A., Wainstein, M., McQuaid, J., Ishii, S. I., et al. (2010). Quantification of Electron Transfer Rates to a Solid Phase Electron Acceptor through the Stages of Biofilm Formation from Single Cells to Multicellular Communities. Environ. Sci. Technol. 44 (7), 2721–2727. doi:10.1021/es903043p
Mehta, T., Coppi, M. V., Childers, S. E., and Lovley, D. R. (2005). Outer Membrane C -Type Cytochromes Required for Fe(III) and Mn(IV) Oxide Reduction in Geobacter Sulfurreducens. Appl. Environ. Microbiol. 71 (12), 8634–8641. doi:10.1128/AEM.71.12.8634-8641.2005
Meitl, L. A., Eggleston, C. M., Colberg, P. J. S., Khare, N., Reardon, C. L., and Shi, L. (2009). Electrochemical Interaction of Shewanella Oneidensis MR-1 and its Outer Membrane Cytochromes OmcA and MtrC with Hematite Electrodes. Geochimica et Cosmochimica Acta 73 (18), 5292–5307. doi:10.1016/j.gca.2009.06.021
Mukherjee, M., Zaiden, N., Teng, A., Hu, Y., and Cao, B. (2020). Shewanella Biofilm Development and Engineering for Environmental and Bioenergy Applications. Curr. Opin. Chem. Biol. 59, 84–92. doi:10.1016/j.cbpa.2020.05.004
Mukherjee, S., and Bassler, B. L. (2019). Bacterial Quorum Sensing in Complex and Dynamically Changing Environments. Nat. Rev. Microbiol. 17 (6), 371–382. doi:10.1038/s41579-019-0186-5
Myers, C. R., and Nealson, K. H. (1988). Bacterial Manganese Reduction and Growth with Manganese Oxide as the Sole Electron Acceptor. Science 240 (4857), 1319–1321. doi:10.1126/science.240.4857.1319
Nealson, K. H., Belz, A., and McKee, B. (2002). Breathing Metals as a Way of Life: Geobiology in Action. Antonie van Leeuwenhoek 81 (1), 215–222. doi:10.1023/A:1020518818647
Nealson, K. H., and Rowe, A. R. (2016). Electromicrobiology: Realities, Grand Challenges, Goals and Predictions. Microb. Biotechnol. 9 (5), 595–600. doi:10.1111/1751-7915.12400
Nelson, J. W., Sudarsan, N., Phillips, G. E., Stav, S., Lünse, C. E., McCown, P. J., et al. (2015). Control of Bacterial Exoelectrogenesis by C-AMP-GMP. Proc. Natl. Acad. Sci. USA 112 (17), 5389–5394. doi:10.1073/pnas.1419264112
Nevin, K. P., Kim, B.-C., Glaven, R. H., Johnson, J. P., Woodard, T. L., Methé, B. A., et al. (2009). Anode Biofilm Transcriptomics Reveals Outer Surface Components Essential for High Density Current Production in Geobacter Sulfurreducens Fuel Cells. PLOS ONE 4 (5), e5628–5638. doi:10.1371/journal.pone.0005628
Ng, C. K., Xu, J., Cai, Z., Yang, L., Thompson, I. P., Huang, W. E., et al. (2020). Elevated Intracellular cyclic‐di‐GMP Level in Shewanella Oneidensis Increases Expression of C ‐type Cytochromes. Microb. Biotechnol. 13 (6), 1904–1916. doi:10.1111/1751-7915.13636
O'Toole, G., Kaplan, H. B., and Kolter, R. (2000). Biofilm Formation as Microbial Development. Annu. Rev. Microbiol. 54 (1), 49–79. doi:10.1146/annurev.micro.54.1.49
Okamoto, A., Hashimoto, K., Nealson, K. H., and Nakamura, R. (2013). Rate Enhancement of Bacterial Extracellular Electron Transport Involves Bound Flavin Semiquinones. Proc. Natl. Acad. Sci. 110 (19), 7856–7861. doi:10.1073/pnas.1220823110
Okamoto, A., Nakamura, R., Nealson, K. H., and Hashimoto, K. (2014a). Bound Flavin Model Suggests Similar Electron-Transfer Mechanisms inShewanellaandGeobacter. ChemElectroChem 1 (11), 1808–1812. doi:10.1002/celc.201402151
Okamoto, A., Saito, K., Inoue, K., Nealson, K. H., Hashimoto, K., and Nakamura, R. (2014b). Uptake of Self-Secreted Flavins as Bound Cofactors for Extracellular Electron Transfer in Geobacter Species. Energ. Environ. Sci. 7 (4), 1357–1361. doi:10.1039/c3ee43674h
Pirbadian, S., Barchinger, S. E., Leung, K. M., Byun, H. S., Jangir, Y., and Bouhenni, R. A. (2014). Shewanella Oneidensis MR-1 Nanowires are Outer Membrane and Periplasmic Extensions of the Extracellular Electron Transport Components. Proc. Nat. Acad. Sci. 111 (35), 12883–12888. doi:10.1073/pnas.1410551111
Papenfort, K., and Bassler, B. L. (2016). Quorum sensing Signal-Response Systems in Gram-Negative Bacteria. Nat. Rev. Microbiol. 14 (9), 576–588. doi:10.1038/nrmicro.2016.89
Parsek, M. R., and Greenberg, E. P. (2005). Sociomicrobiology: the Connections between Quorum Sensing and Biofilms. Trends Microbiol. 13 (1), 27–33. doi:10.1016/j.tim.2004.11.007
Persat, A., Nadell, C. D., Kim, M. K., Ingremeau, F., Siryaporn, A., Drescher, K., et al. (2015). The Mechanical World of Bacteria. Cell 161 (5), 988–997. doi:10.1016/j.cell.2015.05.005
Plate, L., and Marletta, M. A. (2012). Nitric Oxide Modulates Bacterial Biofilm Formation through a Multicomponent Cyclic-Di-GMP Signaling Network. Mol. Cel 46 (4), 449–460. doi:10.1016/j.molcel.2012.03.023
Rabaey, K., and Verstraete, W. (2005). Microbial Fuel Cells: Novel Biotechnology for Energy Generation. Trends Biotechnol. 23 (6), 291–298. doi:10.1016/j.tibtech.2005.04.008
Reguera, G., McCarthy, K. D., Mehta, T., Nicoll, J. S., Tuominen, M. T., and Lovley, D. R. (2005). Extracellular Electron Transfer via Microbial Nanowires. Nature 435 (7045), 1098–1101. doi:10.1038/nature03661
Reguera, G. (2018). Microbial Nanowires and Electroactive Biofilms. FEMS Microbiol. Ecol. 94 (7), 086–098. doi:10.1093/femsec/fiy086
Reguera, G., Nevin, K. P., Nicoll, J. S., Covalla, S. F., Woodard, T. L., and Lovley, D. R. (2006). Biofilm and Nanowire Production Leads to Increased Current in Geobacter Sulfurreducens Fuel Cells. Appl. Environ. Microbiol. 72 (11), 7345–7348. doi:10.1128/AEM.01444-06
Renslow, R., Babauta, J., Kuprat, A., Schenk, J., Ivory, C., Fredrickson, J., et al. (2013a). Modeling Biofilms with Dual Extracellular Electron Transfer Mechanisms. Phys. Chem. Chem. Phys. 15 (44), 19262–19283. doi:10.1039/c3cp53759e
Renslow, R. S., Babauta, J. T., Majors, P. D., and Beyenal, H. (2013b). Diffusion in Biofilms Respiring on Electrodes. Energ. Environ. Sci. 6 (2), 595–607. doi:10.1039/C2EE23394K
Richter, H., Nevin, K. P., Jia, H., Lowy, D. A., Lovley, D. R., and Tender, L. M. (2009). Cyclic Voltammetry of Biofilms of Wild Type and Mutant Geobacter Sulfurreducens on Fuel Cell Anodes Indicates Possible Roles of OmcB, OmcZ, Type IV Pili, and Protons in Extracellular Electron Transfer. Energ. Environ. Sci. 2 (5), 506–516. doi:10.1039/B816647A
Rittmann, B. E., Krajmalnik-Brown, R., and Halden, R. U. (2008). Pre-genomic, Genomic and post-genomic Study of Microbial Communities Involved in Bioenergy. Nat. Rev. Microbiol. 6 (8), 604–612. doi:10.1038/nrmicro1939
Römling, U., Gomelsky, M., and Galperin, M. Y. (2005). C-di-GMP: the Dawning of a Novel Bacterial Signalling System. Mol. Microbiol. 57 (3), 629–639. doi:10.1111/j.1365-2958.2005.04697.x
Ross, D. E., Flynn, J. M., Baron, D. B., Gralnick, J. A., and Bond, D. R. (2011). Towards Electrosynthesis in Shewanella: Energetics of Reversing the Mtr Pathway for Reductive Metabolism. PloS one 6 (2), e16649. doi:10.1371/journal.pone.0016649
Rotaru, A.-E., Shrestha, P. M., Liu, F., Markovaite, B., Chen, S., Nevin, K. P., et al. (2014). Direct Interspecies Electron Transfer between Geobacter Metallireducens and Methanosarcina Barkeri. Appl. Environ. Microbiol. 80 (15), 4599–4605. doi:10.1128/AEM.00895-14
Roy, J. N., Babanova, S., Garcia, K. E., Cornejo, J., Ista, L. K., and Atanassov, P. (2014). Catalytic Biofilm Formation by Shewanella Oneidensis MR-1 and Anode Characterization by Expanded Uncertainty. Electrochimica Acta 126, 3–10. doi:10.1016/j.electacta.2013.07.075
Rumbaugh, K. P., and Sauer, K. (2020). Biofilm Dispersion. Nat. Rev. Microbiol. 18 (10), 571–586. doi:10.1038/s41579-020-0385-0
Saffarini, D. A., Schultz, R., and Beliaev, A. (2003). Involvement of Cyclic AMP (cAMP) and cAMP Receptor Protein in Anaerobic Respiration of Shewanella Oneidensis. J. Bacteriol. 185 (12), 3668–3671. doi:10.1128/JB.185.12.3668-3671.2003
Schrott, G. D., Ordoñez, M. V., Robuschi, L., and Busalmen, J. P. (2014). Physiological Stratification in Electricity-Producing Biofilms ofGeobacter Sulfurreducens. Geobacter sulfurreducensChemSusChem 7 (2), 598–603. doi:10.1002/cssc.201300605
Shi, L., Dong, H., Reguera, G., Beyenal, H., Lu, A., Liu, J., et al. (2016). Extracellular Electron Transfer Mechanisms between Microorganisms and Minerals. Nat. Rev. Microbiol. 14 (10), 651–662. doi:10.1038/nrmicro.2016.93
Shi, L., Fredrickson, J. K., and Zachara, J. M. (2014). Genomic Analyses of Bacterial Porin-Cytochrome Gene Clusters. Front. Microbiol. 5 (657). doi:10.3389/fmicb.2014.00657
Snider, R. M., Strycharz-Glaven, S. M., Tsoi, S. D., Erickson, J. S., and Tender, L. M. (2012). Long-range Electron Transport in Geobacter Sulfurreducens Biofilms Is Redox Gradient-Driven. Proc. Natl. Acad. Sci. USA 109 (38), 15467–15472. doi:10.1073/pnas.1209829109
Soussan, L., Riess, J., Erable, B., Delia, M.-L., and Bergel, A. (2013). Electrochemical Reduction of CO2 Catalysed by Geobacter Sulfurreducens Grown on Polarized Stainless Steel Cathodes. Electrochemistry Commun. 28, 27–30. doi:10.1016/j.elecom.2012.11.033
Srikanth, S., Marsili, E., Flickinger, M. C., and Bond, D. R. (2008). Electrochemical Characterization ofGeobacter Sulfurreducens Cells Immobilized on Graphite Paper Electrodes. Biotechnol. Bioeng. 99 (5), 1065–1073. doi:10.1002/bit.21671
Steidl, R. J., Lampa-Pastirk, S., and Reguera, G. (2016). Mechanistic Stratification in Electroactive Biofilms of Geobacter Sulfurreducens Mediated by Pilus Nanowires. Nat. Commun. 7 (1), 1–11. doi:10.1038/ncomms12217
Stewart, P. S. (2003). Diffusion in Biofilms. J. Bacteriol. 185 (5), 1485–1491. doi:10.1128/jb.185.5.1485-1491.2003
Stewart, P. S., and Franklin, M. J. (2008). Physiological Heterogeneity in Biofilms. Nat. Rev. Microbiol. 6 (3), 199–210. doi:10.1038/nrmicro1838
Summers, Z. M., Fogarty, H. E., Leang, C., Franks, A. E., Malvankar, N. S., and Lovley, D. R. (2010). Direct Exchange of Electrons within Aggregates of an Evolved Syntrophic Coculture of Anaerobic Bacteria. Science 330 (6009), 1413–1415. doi:10.1126/science.1196526
Tan, Y., Adhikari, R. Y., Malvankar, N. S., Ward, J. E., Woodard, T. L., Nevin, K. P., et al. (2017). Expressing the Geobacter Metallireducens PilA in Geobacter Sulfurreducens Yields Pili with Exceptional Conductivity. mBio 8 (1), e02203–02216. doi:10.1128/mBio.02203-16
TerAvest, M. A., and Ajo‐Franklin, C. M. (2016). Transforming Exoelectrogens for Biotechnology Using Synthetic Biology. Biotechnol. Bioeng. 113 (4), 687–697. doi:10.1002/bit.25723
Thirumurthy, M. A., and Jones, A. K. (2020). Geobacter Cytochrome OmcZs Binds Riboflavin: Implications for Extracellular Electron Transfer. Nanotechnology 31 (12), 124001–124008. doi:10.1088/1361-6528/ab5de6
Thormann, K. M., Saville, R. M., Shukla, S., Pelletier, D. A., and Spormann, A. M. (2004). Initial Phases of Biofilm Formation in Shewanella Oneidensis MR-1. J. Bacteriol. 186 (23), 8096–8104. doi:10.1128/JB.186.23.8096-8104.2004
Tielen, P., Rosenau, F., Wilhelm, S., Jaeger, K.-E., Flemming, H.-C., and Wingender, J. (2010). Extracellular Enzymes Affect Biofilm Formation of Mucoid Pseudomonas aeruginosa. Microbiology 156 (7), 2239–2252. doi:10.1099/mic.0.037036-0
Tremblay, P.-L., Summers, Z. M., Glaven, R. H., Nevin, K. P., Zengler, K., Barrett, C. L., et al. (2011). A C-type Cytochrome and a Transcriptional Regulator Responsible for Enhanced Extracellular Electron Transfer inGeobacter Sulfurreducensrevealed by Adaptive Evolution. Environ. Microbiol. 13 (1), 13–23. doi:10.1111/j.1462-2920.2010.02302.x
Ueki, T., Nevin, K. P., Woodard, T. L., Aklujkar, M. A., Holmes, D. E., and Lovley, D. R. (2018). Construction of a Geobacter Strain with Exceptional Growth on Cathodes. Front. Microbiol. 9 (9), 1512. doi:10.3389/fmicb.2018.01512
Valentini, M., and Filloux, A. (2016). Biofilms and Cyclic Di-GMP (C-Di-GMP) Signaling: Lessons from Pseudomonas aeruginosa and Other Bacteria. J. Biol. Chem. 291 (24), 12547–12555. doi:10.1074/jbc.R115.711507
Wang, F., Gu, Y., O’Brien, J. P., Yi, S. M., Yalcin, S. E., Srikanth, V., et al. (2019). Structure of Microbial Nanowires Reveals Stacked Hemes that Transport Electrons over Micrometers. Cell 177 (2), 361–369. e310. doi:10.1016/j.cell.2019.03.029
Watnick, P., and Kolter, R. (2000). Biofilm, City of Microbes. J. Bacteriol. 182 (10), 2675–2679. doi:10.1128/JB.182.10.2675-2679.2000
Watson, V. J., and Logan, B. E. (2010). Power Production in MFCs Inoculated withShewanella oneidensisMR-1 or Mixed Cultures. Biotechnol. Bioeng. 105 (3), 489–498. doi:10.1002/bit.22556
Wei, W., Zhang, Y., Komorek, R., Plymale, A., Yu, R., Wang, B., et al. (2017). Characterization of syntrophicGeobactercommunities Using ToF-SIMS. Biointerphases 12 (5), 05G601–612. doi:10.1116/1.4986832
Wingender, J., Neu, T. R., and Flemming, H.-C. (1999). “What Are Bacterial Extracellular Polymeric Substances?” in Microbial Extracellular Polymeric Substances: Characterization, Structure and Function. Editors J. Wingender, T. R. Neu, and H.-C. Flemming (Berlin, Heidelberg: Springer Berlin Heidelberg), 1–19. doi:10.1007/978-3-642-60147-7_1
Xiao, Y., Zhang, E., Zhang, J., Dai, Y., Yang, Z., Christensen, H. E. M., et al. (2017). Extracellular Polymeric Substances Are Transient media for Microbial Extracellular Electron Transfer. Sci. Adv. 3 (7), e1700623–1700631. doi:10.1126/sciadv.1700623
Xu, S., Barrozo, A., Tender, L. M., Krylov, A. I., and El-Naggar, M. Y. (2018). Multiheme Cytochrome Mediated Redox Conduction through Shewanella Oneidensis MR-1 Cells. J. Am. Chem. Soc. 140 (32), 10085–10089. doi:10.1021/jacs.8b05104
Yalcin, S. E., and Malvankar, N. S. (2020). The Blind Men and the Filament: Understanding Structures and Functions of Microbial Nanowires. Curr. Opin. Chem. Biol. 59, 193–201. doi:10.1016/j.cbpa.2020.08.004
Yalcin, S. E., O’Brien, J. P., Gu, Y., Reiss, K., Yi, S. M., Jain, R., et al. (2020). Electric Field Stimulates Production of Highly Conductive Microbial OmcZ Nanowires. Nat. Chem. Biol. 16 (10), 1136–1142. doi:10.1038/s41589-020-0623-9
Yang, Y., Xiang, Y., Sun, G., Wu, W.-M., and Xu, M. (2015). Electron Acceptor-dependent Respiratory and Physiological Stratifications in Biofilms. Environ. Sci. Technol. 49 (1), 196–202. doi:10.1021/es504546g
Yi, H., Nevin, K. P., Kim, B.-C., Franks, A. E., Klimes, A., Tender, L. M., et al. (2009). Selection of a Variant of Geobacter Sulfurreducens with Enhanced Capacity for Current Production in Microbial Fuel Cells. Biosens. Bioelectron. 24 (12), 3498–3503. doi:10.1016/j.bios.2009.05.004
Yong, Y.-C., Yu, Y.-Y., Zhang, X., and Song, H. (2014). Highly Active Bidirectional Electron Transfer by a Self-Assembled Electroactive Reduced-Graphene-Oxide-Hybridized Biofilm. Angew. Chem. Int. Ed. 53 (17), 4480–4483. doi:10.1002/anie.201400463
Yu, Y.-Y., Chen, H.-L., Yong, Y.-C., Kim, D.-H., and Song, H. (2011). Conductive Artificial Biofilm Dramatically Enhances Bioelectricity Production in Shewanella-Inoculated Microbial Fuel Cells. Chem. Commun. 47 (48), 12825–12827. doi:10.1039/C1CC15874K
Yu, Y.-Y., Wang, Y.-Z., Fang, Z., Shi, Y.-T., Cheng, Q.-W., Chen, Y.-X., et al. (2020). Single Cell Electron Collectors for Highly Efficient Wiring-Up Electronic Abiotic/biotic Interfaces. Nat. Commun. 11 (1), 4087–4096. doi:10.1038/s41467-020-17897-9
Zhang, C., Zhu, S., Jatt, A.-N., Pan, Y., and Zeng, M. (2017). Proteomic Assessment of the Role of N -acyl Homoserine Lactone in Shewanella Putrefaciens Spoilage. Lett. Appl. Microbiol. 65 (5), 388–394. doi:10.1111/lam.12795
Keywords: Geobacter, Shewanella, exoelectrogen, biofilm, biofilm engineering, microbial fuel cells
Citation: Hu Y, Wang Y, Han X, Shan Y, Li F and Shi L (2021) Biofilm Biology and Engineering of Geobacter and Shewanella spp. for Energy Applications. Front. Bioeng. Biotechnol. 9:786416. doi: 10.3389/fbioe.2021.786416
Received: 30 September 2021; Accepted: 18 November 2021;
Published: 03 December 2021.
Edited by:
Bin Lai, Helmholtz Association of German Research Centres (HZ), GermanyReviewed by:
Yonggang Yang, Guangdong Academy of Science, ChinaPier-Luc Tremblay, Wuhan University of Technology, China
Copyright © 2021 Hu, Wang, Han, Shan, Li and Shi. This is an open-access article distributed under the terms of the Creative Commons Attribution License (CC BY). The use, distribution or reproduction in other forums is permitted, provided the original author(s) and the copyright owner(s) are credited and that the original publication in this journal is cited, in accordance with accepted academic practice. No use, distribution or reproduction is permitted which does not comply with these terms.
*Correspondence: Yidan Hu, aHV5aWRhbkBjdWcuZWR1LmNu; Liang Shi, bGlhbmcuc2hpQGN1Zy5lZHUuY24=