- 1Institute of Orthopedic Research and Biomechanics, University Medical Center Ulm, Ulm, Germany
- 2Institute of Comparative Molecular Endocrinology (CME), Ulm University, Ulm, Germany
Biomechanical stimulation by whole-body low-magnitude high-frequency vibration (LMHFV) has demonstrated to provoke anabolic effects on bone metabolism in both non-osteoporotic and osteoporotic animals and humans. However, preclinical studies reported that vibration improved fracture healing and bone formation in osteoporotic, ovariectomized (OVX) mice representing an estrogen-deficient hormonal status, but impaired bone regeneration in skeletally healthy non-OVX mice. These effects were abolished in general estrogen receptor α (ERα)-knockout (KO) mice. However, it remains to be elucidated which cell types in the fracture callus are targeted by LMHFV during bone healing. To answer this question, we generated osteoblast lineage-specific ERα-KO mice that were subjected to ovariectomy, femur osteotomy and subsequent vibration. We found that the ERα specifically on osteoblastic lineage cells facilitated the vibration-induced effects on fracture healing, because in osteoblast lineage-specific ERα-KO (ERαfl/fl; Runx2Cre) mice the negative effects in non-OVX mice were abolished, whereas the positive effects of vibration in OVX mice were reversed. To gain greater mechanistic insights, the influence of vibration on murine and human osteogenic cells was investigated in vitro by whole genome array analysis and qPCR. The results suggested that particularly canonical WNT and Cox2/PGE2 signaling is involved in the mechanotransduction of LMHFV under estrogen-deficient conditions. In conclusion, our study demonstrates a critical role of the osteoblast lineage-specific ERα in LMHFV-induced effects on fracture healing and provides further insights into the molecular mechanism behind these effects.
Introduction
In bone repair, an optimal mechanical environment is required for the successful healing of the fractured bone (Claes et al., 1995; Haffner-Luntzer et al., 2015; Claes, 2017). Long-bone fracture healing critically depends on the stability and type of fracture fixation resulting in different degrees of interfragmentary movement, which either guide intramembranous or endochondral bone formation. A too rigid fixation with less interfragmentary movements results in lower strains around the fracture area and can even hinder successful healing (Perren, 1979; Claes et al., 1998). Clinical and preclinical studies suggest, that the endocrine status is also important because a disturbed bone formation during fracture healing is commonly observed in postmenopausal osteoporotic patients and OVX rodents (Kim et al., 2001; Nikolaou et al., 2009; Beil et al., 2010; He et al., 2011; Cheung et al., 2016). This can be explained by the importance of the osteoanabolic hormone estrogen for bone health (Cauley, 2015). Therefore, external mechanostimulation applied by whole-body low-magnitude high-frequency vibration (LMHFV) was suggested as a promising approach to improve compromised bone repair in osteoporotic subjects (Thompson et al., 2014; Edwards and Reilly, 2015). Several clinical trials investigating fracture healing and LMHFV are ongoing with no published results yet. Regarding the intact skeleton, clinical studies mainly reports anabolic effects of LMHFV on the osteoporotic skeleton (Rubin et al., 2004), whereas one study demonstrates no effect on bone mineral density (Leung et al., 2014). Preclinical studies demonstrate that LMHFV application provokes positive effects on bone healing in sheep (Li et al., 2018) and in ovariectomy-induced estrogen-deficient rodents (Stuermer et al., 2010; Komrakova et al., 2013; Chung et al., 2014; Wehrle et al., 2015; Wang et al., 2017; Steppe et al., 2020). By contrast, no or negative effects on bone repair were observed in non-OVX rodents (Shi et al., 2010; Stuermer et al., 2010; Chung et al., 2014; Wehrle et al., 2014, 2015; Wang et al., 2017; Steppe et al., 2020).
Therefore, estrogen seems to play an important role in regulating the mechanotransduction of LMHFV during fracture healing. The effect of estrogen on bone is mainly mediated via its interaction with two estrogen receptors (ERs), ERα and ERβ. Both are expressed by chondrocytes and osteogenic cells (Manolagas et al., 1995; Almeida et al., 2017), but regulate the expression of different target genes. In addition to the classic ER pathway involving ligand-binding and the subsequent interaction with transcription factors or estrogen-responsive elements or, also ligand-independent signaling can be exerted by ERα and ERβ in the absence of estrogen. Several studies report that ERα expression is decreased in the fracture callus of OVX mice compared to non-OVX animals (He et al., 2011), whereas mechanical stimulation enhanced ERα expression in the fracture callus particularly in OVX rodents (Wehrle et al., 2015; Chow et al., 2016; Haffner-Luntzer et al., 2018a). Furthermore, other reports showed that ERα is required for mediating the anabolic effects of mechanical strain (Lee et al., 2003; Jessop et al., 2004; Windahl et al., 2013; Chow et al., 2016), indicating that ERα is involved in mechanotransduction in bone. By using a global ERα-knockout (ERα-KO) mouse model, we previously demonstrated that in the absence of ERα, both the improved fracture healing and bone formation upon vibration in OVX animals and the impaired bone regeneration in non-OVX mice were not observed anymore (Haffner-Luntzer et al., 2018a). ERβ-KO did not display any effect, indicating only a minor role of this receptor-however, by using global KO mice we were unable to conclude about systemic versus local effects of ERα during mechanostimulation in fracture healing. Notably, we found that the number and the contact area between osteoblasts and bone (osteoblast surface) in the fracture callus of wildtype mice were decreased in non-OVX but increased in OVX animals by vibration, whereas these parameters were unaltered in ERα-KO OVX mice upon LMHFV treatment (Haffner-Luntzer et al., 2018a). Since osteoblasts are involved in bone regeneration (Dirckx et al., 2013; Bahney et al., 2019) and as ERα is highly expressed by osteoblasts (Haffner-Luntzer et al., 2018a), we hypothesized that osteoblasts are direct target cells of LMHFV via ERα signaling. To investigate this hypothesis, we generated mice with an osteoblast lineage-specific ERα-KO (ERαfl/fl; Runx2Cre), which underwent ovariectomy followed by a femur osteotomy and LMHFV treatment.
Materials and Methods
Animal Care and Animal Models
All experiments were performed according to the German Guidelines of Animal Research on the Protection of Animals as well as the ARRIVE guidelines and were approved by the local ethical committee (No. 1455, Regierungspräsidium Tübingen, Germany). Osteoblast lineage-specific ERα-KO mice (Tg (Runx2-cre)1Jtuc x Esr1tm1.2Mma) were generated by crossing Runx2-Cre with ERαfl/fl mice on a C57BL/6 background, both provided by Prof. J. Tuckermann (Ulm University). Cre− littermates (ERαfl/fl) were used as control. Cre+ mice (ERαfl/fl; Runx2Cre) were shown to lack the ERα in cells of the osteogenic lineage (Supplementary Figure S1) including osteoblasts, osteocytes and hypertrophic chondrocytes. All animals were housed in groups of up to five mice per cage with a 12 h light, 12 h dark rhythm, and received water ad libitum as well as a standard mouse feed (Ssniff R/M-H, V1535-300; Ssniff, Soest, Germany) until the day of ovariectomy/sham-ovariectomy. Subsequently, the food was switched to a phytoestrogen-free diet (Ssniff). Mouse genotyping was conducted by lysed ear punch PCR using the primers: 5′-CCAGGAAGACTGCCAGAAGG-3′, 5′-TGGCTTGCAGGT ACAGGAG-3′ and 5′-GGAGCTGCCGAGTCAATAAC-3′ to detect the Cre transgene, whereas the ERα loxP sites were detected using the primers: 5′- TAGGCTTTGTCTCGCTTT CC-3′, 5′- CCCTGG CAAGATAAGACAGC-3′ and 5′-AGGAGAATGAGGTGGCACAG-3′.
Surgical Procedures
When aged 12 weeks, female mice (n = 24 per genotype) were randomly assigned (Table 1) to bilateral ovariectomy (n = 12 per genotype) or sham-operated (non-OVX, n = 12 per genotype) as described previously (Haffner-Luntzer et al., 2017). 4 weeks after OVX or non-OVX surgery, all mice underwent standardized unilateral femur osteotomy as described previously (Röntgen et al., 2010; Wehrle et al., 2015; Haffner-Luntzer et al., 2017). Briefly, the osteotomy was created at the right femur diaphysis using a 0.4 mm Gigli wire saw (RISystem, Davos, Switzerland) and stabilized by a semi-rigid external fixator (RISystem). Half of the OVX (n = 6 per genotype) and non-OVX mice received LMHFV. Three weeks after osteotomy surgery (day 21), all mice were sacrificed using an isoflurane overdose and cardiac blood withdrawal.
LMHFV
The LMHFV regimen was chosen due to our previous studies, showing that 45 Hz significantly improved fracture healing in OVX mice (Wehrle et al., 2015; Haffner-Luntzer et al., 2018a). Starting on the third day after osteotomy surgery, mice were placed on custom-made vibration platforms for 20 min per day for 5 days per week and received vertical whole-body vibration with 0.3 g sinusoidal peak-to-peak acceleration and 45 Hz frequency, as described previously (Wehrle et al., 2015) (Figure 1A). The amplitude and frequency were continuously recorded using integrated accelerometers at the platform (Sensor KS95B.100, measurement amplifier Innobeamer L2, Software Vibromatrix; IDS Innomic GmbH, Salzwedel, Germany). The control mice were sham-vibrated on the same platforms without activation of the vibration generator. All mice were allowed to move freely on the platforms during the vibration or sham-vibration treatment and afterwards returned to their home cages.
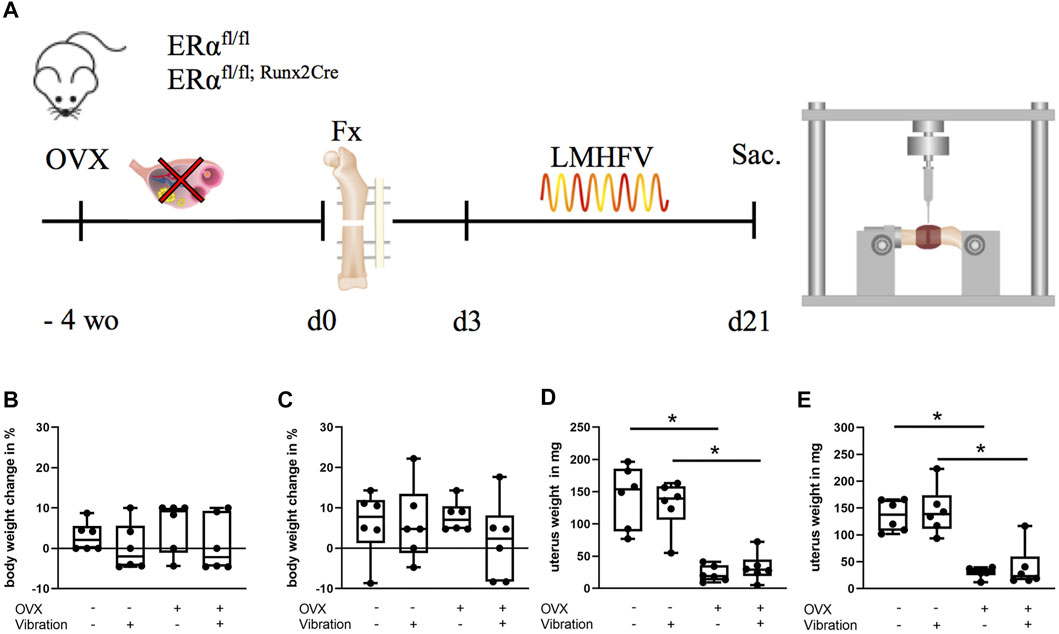
FIGURE 1. Schematic illustration of the experimental timeline and physiological parameters of ERαfl/fl and ERαfl/fl; Runx2Cre mice. (A) When aged 12 weeks, mice were ovariectomized (OVX) or sham-operated followed by fracture surgery (Fx) 4 weeks later. Vibration (LMHFV) or sham-vibration treatment started on the third postoperative day for 20 min/day and 5 days/week. On day 21 after fracture, the mice were sacrificed (Sac.) and their bones were analyzed. Body weight of ERαfl/fl (B) and ERαfl/fl; Runx2Cre (C) mice as well as their respective uterus weight [(D): ERαfl/fl, (E): ERαfl/fl; Runx2Cre] was assessed. Data are shown as box-and-whisker plots (with median and interquartile range) from max. to min., showing all data points. *indicates significant effects with p ≤ 0.05.
Biomechanical Testing and μCT Analysis
To evaluate the mechanical properties of intact and osteotomized femurs explanted on day 21, a non-destructive three-point bending test was performed as described previously (Röntgen et al., 2010; Wehrle et al., 2015). Briefly, following removal of the fixator, an axial load with a maximum of 2 N was applied on top of the callus side in a cranio-lateral position using a material-testing machine (1454, Zwick GmbH & Co KG, Ulm, Germany). The bending stiffness was calculated from the slope of the load-deflection curve as described previously (Röntgen et al., 2010). If the bones were too fragile for reaching a preload of 0.05 N, the three-point bending test was not performed and a flexural rigidity value of 0 was given. Following biomechanical testing, femora were fixed in 4% phosphate-buffered formaldehyde solution and scanned using a μCT scanning device (Skyscan 1172 version 1.5; Skyscan, Kontich, Belgium) at a resolution of 8 μm using a peak voltage of 50 kV and 200 μA. Analyses and calibration steps were performed according to the guidelines of the American Society for Bone and Mineral Research (Bouxsein et al., 2010). The volume of interest was defined as the entire callus between the fractured cortices. Within each scan, two phantoms with a defined density of hydroxyapatite (250 and 750 mg hydroxyapatite/cm3) were included to determine the bone mineral density. To distinguish between mineralized and nonmineralized tissue, a threshold for cortical bone set at 641.9 mg hydroxyapatite/cm3 was used (Morgan et al., 2009). The trabecular bone of the intact left femora was assessed 200 μm proximal of the metaphyseal growth plate over a length of 280 μm in the distal femur, excluding the cortex. For assessing the trabecular bone parameters, a threshold set at 394.8 mg hydroxyapatite/cm3 was used (Morgan et al., 2009). Analyses were performed by means of Skyscan software (NRecon version 1.7.1.0, DataViewer version 1.5.1.2, and CTAn version 1.17.2.2). Bony bridging score was determined in two perpendicular planes with one bridged cortex counting for one scoring point. A bony bridging score of 3 or 4 represented successful healing.
Histomorphometry of the Fracture Callus and Immunohistochemical Staining
Following μCT analysis, bone specimens were subjected to decalcified histology as described previously (Haffner-Luntzer et al., 2014). Sections of 7 μm were stained with Safranin O for histomorphometric tissue quantification. The amounts of bone, cartilage and fibrous tissue in the fracture gap were determined using an image analysis software (Leica DMI6000 B; Software MMAF Version 1.4.0 MetaMorph®; Leica, Heerbrugg, Switzerland). The number and surface of osteoclasts (NOc/BPm, OcS/BS) were quantitatively assessed using tartrate-resistant alkaline phosphatase (TRAP) staining. Osteoclasts were defined as TRAP-positive cells with two or more nuclei, directly located on the bone surface with a visible resorption lacuna between the bone matrix and the cell. The number and surface of osteoblasts were determined using Safranin-O staining. Osteoblasts were defined as cubic-shaped cells with visible cytoplasm, located directly on the bone surface. Bone cells and surface were evaluated in a rectangular area (650 × 450 μm) using the Osteomeasure system (Osteometrics, Decatur, United States). Images were obtained using a Leica microscope (DMI 6000B). All analyses were performed according to the American Society for Bone and Mineral Research guidelines for bone histomorphometry (Dempster et al., 2013).
For immunohistochemical staining, paraffin-embedded sections of 4 µm were deparaffinized, enzymatically demasked by trypsin, blocked with 3% peroxidase followed by a serum block with 5% goat serum in TBS-T for 2 h. Staining for ERα was performed at 4°C overnight using the following primary antibody: rabbit anti-mouse ERα (1:75, # PA5-16440, Invitrogen). Rabbit IgG was included as isotype control to confirm specific staining. Next, sections were washed with TBS and incubated with a goat anti-rabbit secondary antibody (1:100, #B2770, Life Technologies) for an hour at RT. After another washing step, horseradish peroxidase (HRP)-conjugated streptavidin (#PK-6100, VECTASTAIN® Elite ABC-HRP Kit, Peroxidase, Vector Laboratories) was applied according to the manufacturer`s guidelines. NovaRED (#SK-4800, Vector® NovaRED® Substrate Kit, Peroxidase (HRP), Vector laboratories) was used as chromogen and the sections were counterstained with hematoxylin (1:5; #2C-306, Waldeck, Münster, Germany) and rinsed. This staining protocol was previously established by using biological negative controls (bone sections from ERα-general KO mice). In ERα-stained sections, osteoblasts were identified by their cubic shape and direct contact to the bone trabeculae, whereas osteocytes were defined as ellipsoidal shaped cells embedded in the mineralized bone matrix. Hypertrophic chondrocytes were identified as spherical or polygonal shaped cells within a population of other chondrocytes.
Cell Culture Experiments and Transcriptome Analysis
Murine MC3T3-E1 cells were purchased from the American Type Culture Collection (ATCC) and seeded at a density of 4,000 cells per cm2. On day 3 after seeding, cells were differentiated by adding 50 mg/ml ascorbic acid and 10 mM β-glycerophosphate to the phenol-red free culture medium containing 10% charcoal-stripped (estrogen-free) fetal calf serum (FCS), 1% L-glutamine and 1% penicillin/streptomycin (all ThermoFisher Scientific). Half of the cultures were subjected to LMHFV on a custom-made vibration platform at 0.3 g peak-to-peak acceleration/45 Hz for 20 min/day for 5 days. The other half of the cultures (sham-vibrated) were placed on the same platform without turning on the vibration device. Total RNA was isolated as described previously (Haffner-Luntzer et al., 2018b) and microarray-based gene expression analysis with mouse Gene 1.0 ST GeneChip® arrays was performed as published previously (Mödinger et al., 2018). Differentially expressed probesets were determined by t test and considered statistically significant when p < 0.05 and fold change ≤1.5, as described previously (Zoller et al., 2017). To identify the involved molecular pathways, the GoMINER tool (Zeeberg et al., 2003) was used, whereas functional protein interaction networks were identified using the STRING 10 program (http://string-db.org/). Complete microarray data are available in the Supplementary Material. For the Cox2 antagonist experiment, celecoxib was obtained from Sigma (SML3031) and prepared using dimethyl sulfoxide (stock: 2 mg/ml). AH23848 (EP4 antagonist) was purchased from Cayman Chemical (19023) and dissolved in dimethyl sulfoxide (stock: 5 mg/ml). Both antagonists were used at a concentration of 1 × 10−6 M.
Human bone marrow-derived mesenchymal stem cells (hMSCs) were obtained by Lonza (PT-2501) and seeded in 24-well plates (10,000 cells/well) 2 days prior to the differentiation experiments. The medium was changed to differentiation medium (phenol-red free α-MEM, 10% FCS + 1% penicillin/streptomycin + 1% L-glutamine, 50 mg/ml ascorbic acid, 10 mM β-glycerophosphate) at day 0 and the cells were allowed to differentiate for 10 days. At day 10, the medium was switched to estrogen-free medium and vibration was performed 4 h after the medium-switch for 20 min at 45 Hz and 0.3 g. Thirty minutes after the end of the vibration treatment, the experiment was stopped and RNA was isolated (RNeasy Mini kit, Qiagen) for analyzing gene expression by qPCR and human Gene 1.0 ST GeneChip® arrays.
qPCR
The SensiFAST SYBR Hi-ROX One-Step Kit (Bioline, Mempis, United States) was used according to the manufacturer’s guidelines to perform quantitative PCR. B2M (murine F: 5′-ATACGCCTGCAGAGTTAAGCA-3′, murine R: 5′-TCACATGTCTCGATCCCAGT-3′, human F: 5′-CTCACGTCATCCAGCAGAGA-3′, human R: 5′-GGATGGATGAAACCCAGACA-3′) was used as the housekeeping gene. Relative gene expression of Lef1 (murine F: 5′-TCA CCT ACA GCG ACG AG-3′, murine R: 5′-TGACATCTGACGGGATGTGT-3′, human F: 5′ GAGATTTCTCTGTATGGCACC-3′, human R: 5′-CTGCAATGAGACACTTTCTC-3′), Ptgs2 (murine F: 5′-AGGGGTGTCCCTTCACTTCT-3′, murine R: 5′-CATTGATGGTGGCTGTTTTG-3′, human F: 5′-TAAGGGGAGAGGAGGGAAAA-3′, human R: 5′-CTGCTGAGGAGTTCCTGGAC-3′) and Mdk (human F: 5′-TGCCCTGCAACTGGAAGAA-3′, human R: 5′-GCCTGTGCCCCC ATCAC-3′) was calculated using the delta-delta CT method.
Statistics
Group size was n = 6 per group and per genotype (ERαfl/fl, ERαfl/fl; Runx2Cre). In one animal, the histological sections were not evaluable, therefore analyzing this group with n = 5 (Figures 3E–H, second boxplot). Data were tested for normal distribution using the Shapiro-Wilk test, and data sets were normally distributed. Statistical testing between two groups was done using two-tailed Student’s t-test, whereas comparisons between more than two groups were performed by one-way ANOVA with post hoc Tukey’s test for adjusting the p-value for multiple comparisons using GraphPad Prism 8.4.3 (GraphPad Software, La Jolla, CA). The level of significance was set at p ≤ 0.05. Results are presented as box-and-whisker plots (with median and interquartile range) from max. to min., showing all data points.
Results
Body Weight and Uterus Weight Changes
All mice received the selected treatments including ovariectomy/sham-operation, fracture and LMHFV/sham-vibration as illustrated in Figure 1A. The body weight of the animals was not significantly influenced by the treatments or the genotype during the entire experimental period (Figures 1B,C). To control for the success of OVX in ERαfl/fl and ERαfl/fl; Runx2Cre mice, uterus weight at day 21 after osteotomy was assessed. In both mouse strains, OVX resulted in a significantly lower uterus weight (Figures 1D,E). Additionally, we performed µCT analysis of the intact left femora to determine if our mice developed an osteoporotic bone phenotype after OVX treatment. We found that non-vibrated and vibrated OVX groups from both genotypes displayed lower bone volume fraction and trabecular number, either significantly or by strong trend (Supplementary Figure S2).
Fracture Healing in Control (ERαfl/fl) Mice
In non-OVX mice that received LMHFV treatment, a significant reduction of flexural rigidity (p < 0.0001) and bony bridging (p = 0.0047) of the fracture gap was displayed, whereas the bone mineral density and the relative bone volume ratio did not differ significantly (Figures 2A–D). Furthermore, histomorphometrical analysis revealed a by trend reduced relative bone area (p = 0.0923), an increased cartilage area (p = 0.0564) as well as a significantly reduced osteoblast count (p = 0.0539) and a significantly lower osteoblast activity (p = 0.0159) in vibrated non-OVX ERαfl/fl mice (Figures 3A–F). All these data indicated impaired fracture healing at day 21 in non-OVX mice subjected to vibration treatment.
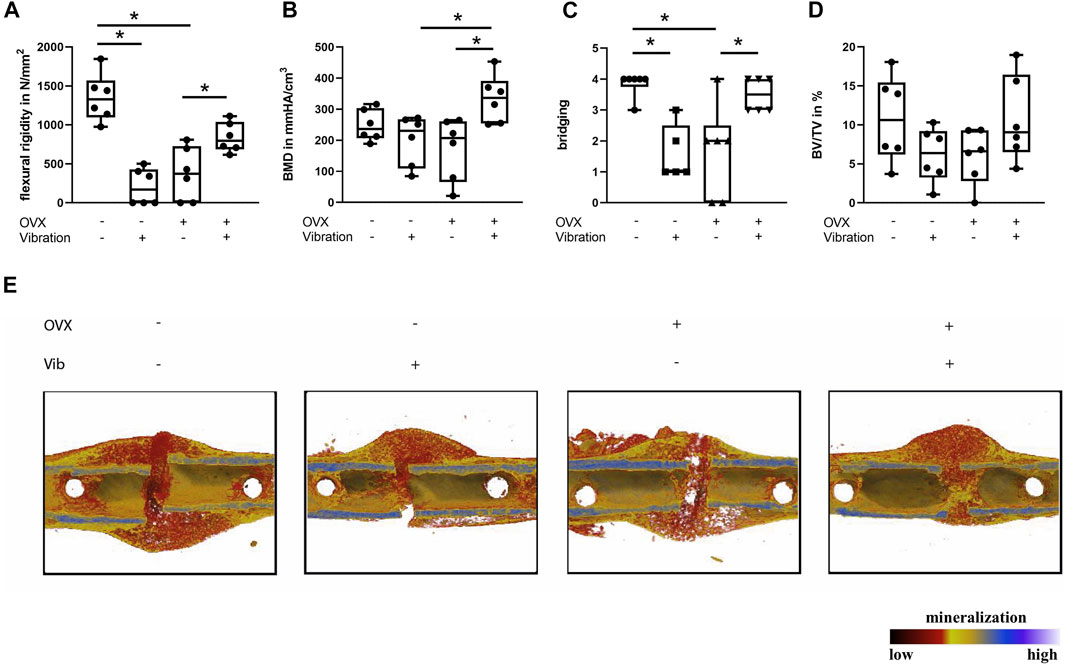
FIGURE 2. Influence of LMHFV on fracture healing in ERαfl/fl mice on day 21. Biomechanical testing and μCT analysis. (A) Bending stiffness of the fracture callus. (B) Bone mineral density of the fracture gap. (C) Bony bridging of the fracture gap evaluated in two perpendicular planes. (D) Relative bone volume (BV/TV) in the fracture gap. (E) Representative μCT images from the fractured femurs. Red indicates weakly mineralized bone, whereas yellow and light blue indicate highly mineralized bone. Data are shown as box-and-whisker plots (with median and interquartile range) from max. to min., showing all data points. *indicates significant effects with p ≤ 0.05.
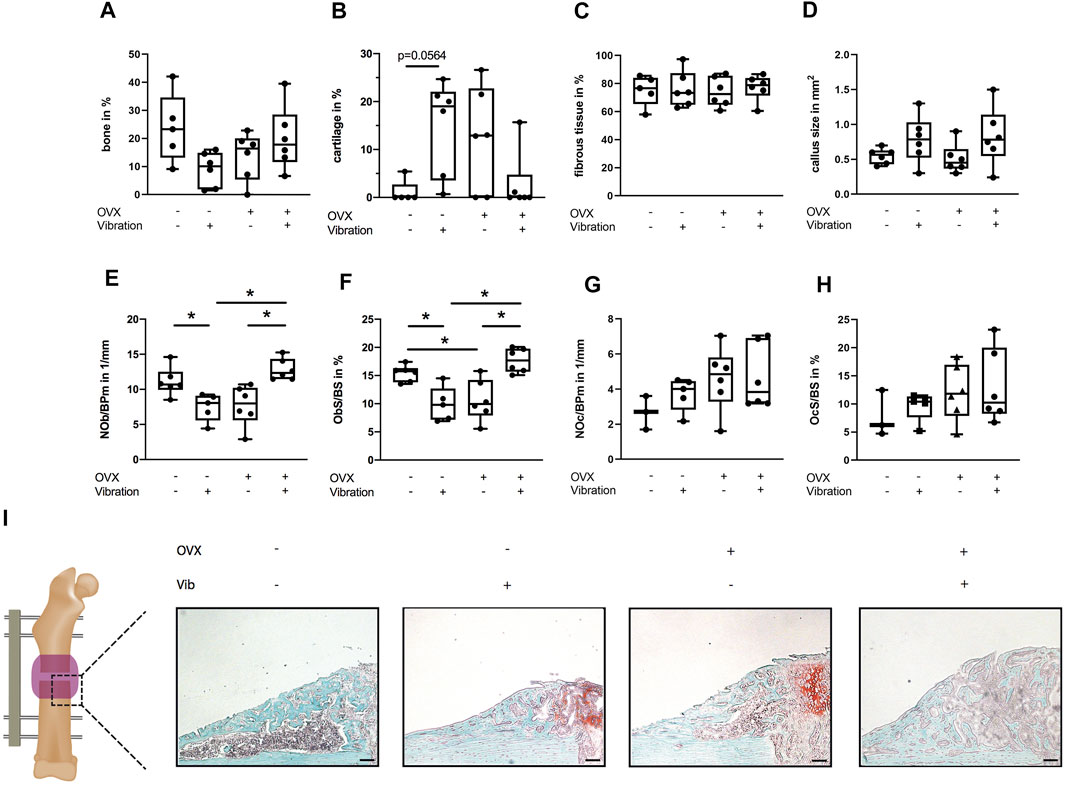
FIGURE 3. Histomorphometrical analysis of the fracture gap in ERαfl/fl mice on day 21. Paraffin sections of fractured femurs were stained with Safranin O and histomorphometrical analysis was performed to determine (A) relative bone area, (B) relative cartilage area, (C) relative fibrous tissue area and (D) callus size. (E) Number of −6+s per bone perimeter (NOb/BPm) and (F) osteoblast surface per bone surface (ObS/BS) in the fracture gap. (G) Number of osteoclasts per bone perimeter (NOc/BPm) and (H) osteoclast surface per bone surface (OcS/BS). (I) Representative images from the fracture callus at day 21 after surgery stained with Safranin O. Scale bar = 100 μm. Data are shown as box-and-whisker plots (with median and interquartile range) from max. to min., showing all data points. *indicates significant effects with p ≤ 0.05.
Bone regeneration was also compromised in OVX mice compared to non-OVX mice, as indicated by an inferior flexural rigidity (p < 0.0001), significantly reduced bony bridging (p = 0.0041) and by trend increased amount of cartilage (p = 0.1469) in the fracture callus (Figures 2, 3). Similarly, osteoblast number (p = 0.0576) and surface (p = 0.0296) were also diminished in OVX mice compared to non-OVX mice (Figures 3E,F).
Bone healing was improved by LMHFV in OVX mice in comparison to OVX animals as indicated by significantly enhanced flexural rigidity (p = 0.0374), bone mineral density (p = 0.0108) and bony bridging (p = 0.0155) of the fracture gap and a by trend reduced cartilage area (p = 0.2248) (Figures 2, 3). Furthermore, LMHFV resulted in a significantly increased number (p = 0.0031) and activity of osteoblasts (p = 0.0012) in OVX ERαfl/fl mice compared to OVX animals without vibration (Figures 3E,F). The callus size as well as the number and surface of osteoclasts did not differ significantly between all the ERαfl/fl groups (Figures 3D,G,H). Overall, these data demonstrated that OVX and LMHFV both disturb bone regeneration in control ERαfl/fl mice, whereas in combination both treatments resulted in an improved fracture healing.
Fracture Healing in Osteoblast-Specific ERα-KO (ERαfl/fl; Runx2Cre) Mice
Although mice with a specific deletion of the ERα in the osteoblast lineage (ERαfl/fl; Runx2Cre) were previously shown to have a preexisting bone phenotype with decreased trabecular bone mass in the spine and tibiae (Seitz et al., 2012), we found that this does not influence fracture healing (p = 0.439) when directly comparing non-OVX non-vibrated animals of both genotypes (Supplementary Figure S3).
Furthermore, LMHFV did not exert negative effects on fracture healing in non-OVX ERαfl/fl; Runx2Cre mice, because there were no significant differences in flexural rigidity, bone content or bridging of the fracture gap in comparison to ERαfl/fl; Runx2Cre mice that did not receive LMHFV (Figure 4). In addition, histomorphometrical parameters were unaltered by LMHFV treatment in non-OVX mice except the osteoblast number (p = 0.0005) and surface (p = 0.0004) that were significantly increased in vibrated non-OVX mice lacking the ERα in osteoblasts (Figure 5).
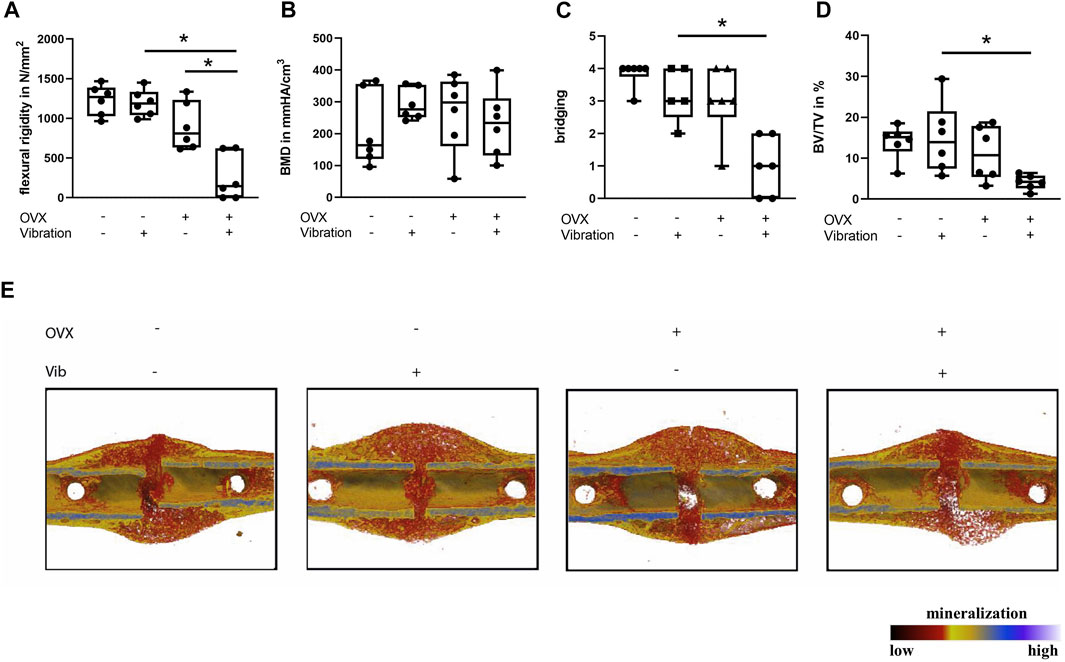
FIGURE 4. Influence of LMHFV on fracture healing in ERαfl/fl; Runx2Cre mice on day 21. Biomechanical testing and μCT analysis. (A) Bending stiffness of the fracture callus. (B) Bone mineral density of the fracture gap. (C) Bony bridging of the fracture gap evaluated in two perpendicular planes. (D) Relative bone volume (BV/TV) in the fracture gap. (E) Representative μCT images from the fractured femurs. Red indicates weakly mineralized bone whereas yellow and light blue indicate highly mineralized bone. Data are shown as box-and-whisker plots (with median and interquartile range) from max. to min., showing all data points. *indicates significant effects with p ≤ 0.05.
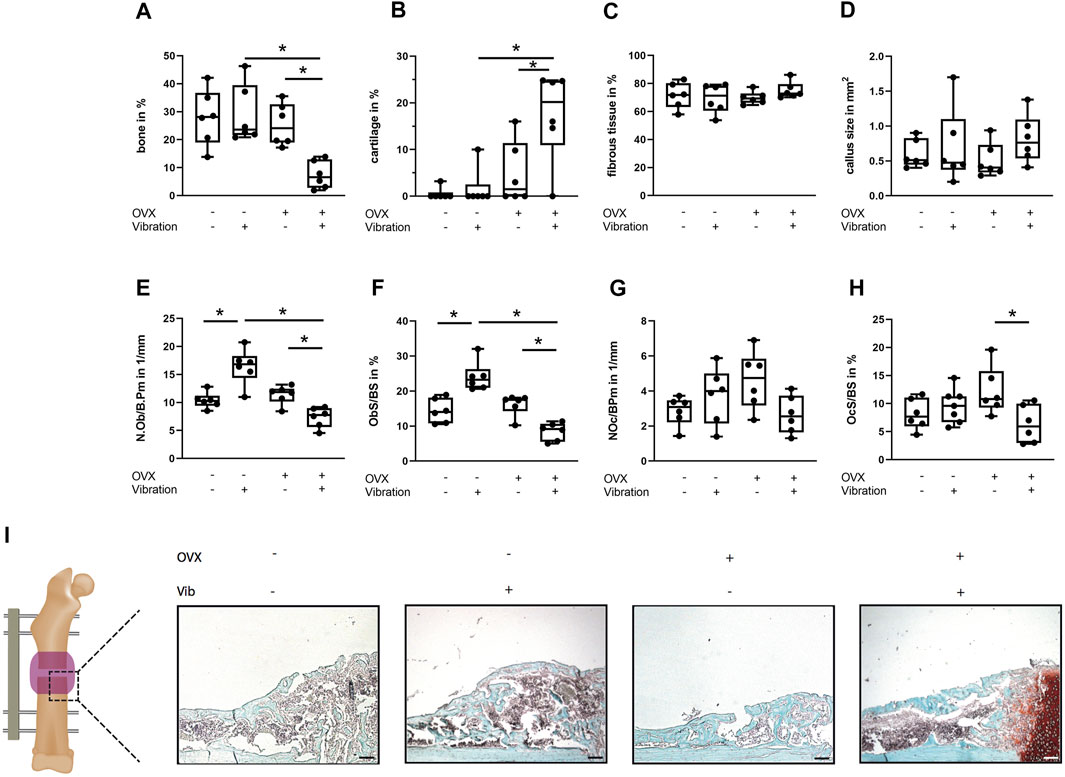
FIGURE 5. Histomorphometrical analysis of the fracture gap in ERαfl/fl; Runx2Cre mice on day 21. Paraffin sections of fractured femurs were stained with Safranin O and histomorphometrical analysis was performed to determine (A) relative bone area, (B) cartilage area, (C) relative fibrous tissue area and (D) callus size. (E) Number of osteoblasts per bone perimeter (NOb/BPm) and (F) osteoblast surface per bone surface (ObS/BS) in the fracture gap. (G) Number of osteoclasts per bone perimeter (NOc/BPm) and (H) osteoclast surface per bone surface (OcS/BS). (I) Representative images from the fracture callus at day 21 after surgery stained with Safranin O. Scale bar = 100 μm. Data are shown as box-and-whisker plots (with median and interquartile range) from max. to min., showing all data points. *indicates significant effects with p ≤ 0.05.
ERαfl/fl; Runx2Cre OVX mice without vibration were protected from impaired fracture healing due to OVX, because a similar flexural rigidity, bony bridging and bone formation in the fracture callus was observed compared to non-OVX ERαfl/fl; Runx2Cre mice (Figure 4). Furthermore, histomorphometrical analysis did not reveal any significant differences in callus composition or cellular parameters compared to non-OVX ERαfl/fl; Runx2Cre mice (Figure 5).
Interestingly, reversed effects of vibration on bone formation during fracture healing in OVX ERαfl/fl; Runx2Cre mice were observed, which resulted in a considerably reduced flexural rigidity (p = 0.001), by trend diminished bone formation (p = 0.1975) and significantly reduced bony bridging (p = 0.0032) compared to OVX ERαfl/fl; Runx2Cre mice (Figures 4A,C,D) in contrast to the improved fracture healing seen in vibrated OVX control ERαfl/fl mice. Histomorphometrical analysis further revealed a significantly increased cartilage area (p = 0.0114) in the fracture callus of OVX ERαfl/fl; Runx2Cre animals subjected to vibration treatment (Figure 5B). In addition, the osteoblast surface (p = 0.0039) and number (p = 0.0188) were significantly decreased in vibrated OVX ERαfl/fl; Runx2Cre mice (Figures 5E,F), whereas osteoclast activity was significantly reduced (p = 0.0315) (Figure 5H).
Molecular Mechanisms
We performed whole genome microarray-based gene expression analysis of vibrated and sham-vibrated samples and found that 304 genes were differentially regulated upon LMHFV treatment (Figure 6). Among the 184 upregulated genes, the mechanosensitive enzyme Cox2, encoded by the gene Ptgs2, appeared in our array (Table 2), as well as bone remodeling and extracellular matrix-associated genes (Table 3). Downregulated genes were found to be involved in Wnt/β-catenin signaling (Table 4), with most of the genes encoding for proteins that act as Wnt inhibitors (Axin2, Sostdc1, Dkk2) except the gene encoding for the Wnt receptor Fzd9. STRING analysis was performed to investigate the interactions of the respective pathways (Figures 6A,D). As expected, the Wnt pathway genes were clustering as well as the genes for extracellular matrix and Cox2/PGE2 signaling (Figure 6D). Confirming the microarray findings, gene expression of Cox2 was validated by qPCR (Figure 6C). Consequently, we were interested whether the Cox2/PGE2 pathway might interact with canonical Wnt signaling. To investigate whether the signaling via the Cox2/EP4 axis might affect downstream signaling of the Wnt pathway, we performed further in vitro experiments with MC3T3-E1 cells pretreated with either the EP4 inhibitor AH23848 or the Cox2 inhibitor celecoxib. We demonstrated that the prostaglandin pathway via EP4 positively modulates the Wnt target gene Lef1 in response to LMHFV, because the EP4 and Cox2 inhibitors abolished the LMHFV-induced Lef1 upregulation (Figures 6E–G). Because we aimed to study the effects of LMHFV also in human cells, hMSCs were subjected to vibration and gene expression was assessed. We demonstrated that Cox2 in hMSCs was also upregulated by vibration (Figure 6H). Furthermore, the Wnt inhibitor Mdk was downregulated upon LMHFV, whereas the Wnt transcription factor Lef1 was significantly upregulated (Figures 6I,J). In addition, microarray-based gene analysis of vibrated vs. non-vibrated hMSCs revealed that a further 56 genes were differentially regulated upon vibration. After excluding non-coding transcripts, 26 probesets remained for analysis. Among them, there were five micro RNAs (miRNAs) that are known to be involved in regulating bone metabolism, whereof three were upregulated and two downregulated (Table 5).
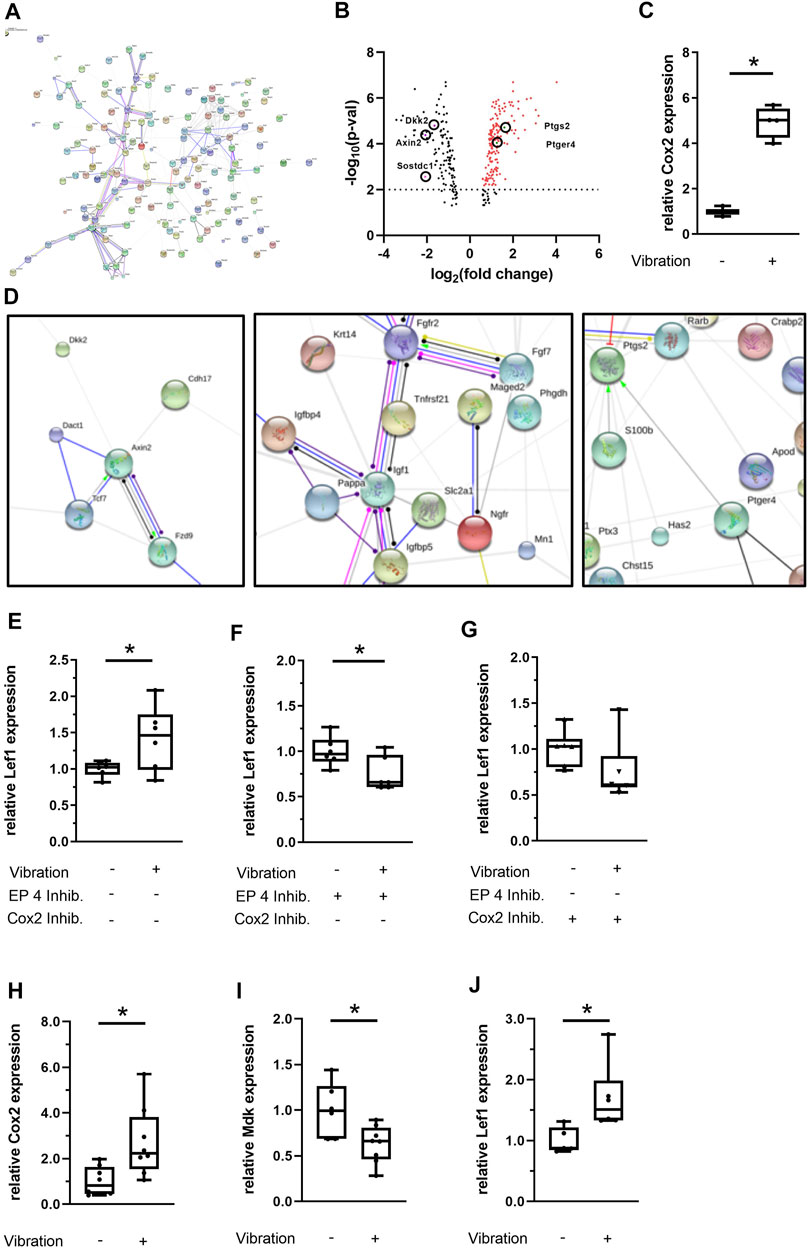
FIGURE 6. Transcriptome analysis of MC3T3-E1 cells and gene expression analysis of hMSCs upon vibration treatment under estrogen-free conditions. (A) Nondetailed STRING protein-association network (action) derived from the MC3T3-E1 microarray data. The image in high quality can be found in the supplemental material (Supplementary Figure S4). (B) Vulcano plot of differentially regulated genes and (C) qPCR validation of Cox2 expression in MC3T3-E1 cells. (D) Detailed STRING network (MC3T3-E1 microarray). (E–G) Effect of LMHFV in MC3T3-E1 cells pretreated with the EP4 antagonist AH23848 (1 × 10−6 M) or with the Cox2 antagonist celecoxib (1 × 10−6 M). (H–J) Effect of LMHFV in hMSCs. Data are shown as box-and-whisker plots (with median and interquartile range) from max. to min., showing all data points. N = 4–6 per group (vibrated and sham-vibrated), *indicates significant effects with p ≤ 0.05.

TABLE 2. Selected differentially regulated mechanosensitive genes upon vibration treatment in MC3T3-E1 cells under estrogen-free conditions.
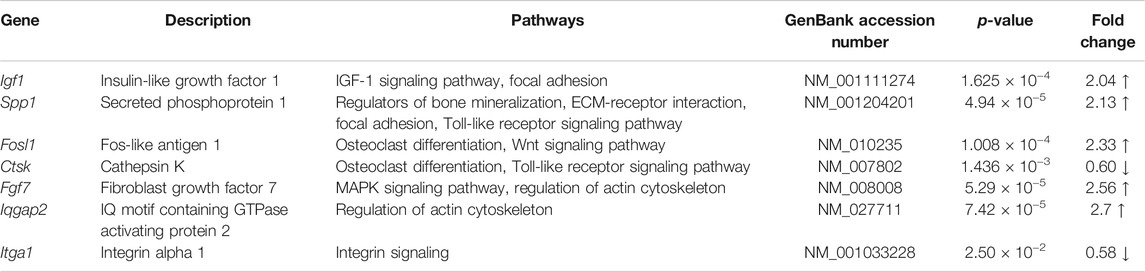
TABLE 3. Selected differentially regulated bone remodeling and ECM-associated genes upon vibration treatment in MC3T3-E1 cells under estrogen-free conditions.

TABLE 4. Selected differentially regulated Wnt signaling genes upon vibration treatment in MC3T3-E1 cells under estrogen-free conditions.
Discussion
Estrogen is a key hormone for bone homeostasis, therefore, estrogen-deficiency in postmenopausal females or after ovariectomy in rodents lead to osteoporosis development. Furthermore, fracture healing was found to be disturbed in osteoporotic women and in female mice after ovariectomy because of impaired endochondral bone formation (Beil et al., 2010; Wehrle et al., 2015; Haffner-Luntzer et al., 2017; Wildemann et al., 2021). Biomechanical stimulation by whole-body vibration was proposed as a readily applicable and non-invasive treatment approach for osteoporotic fracture healing. However, preclinical studies demonstrate that the estrogen and ERα signaling play an important role in the response to vibration treatment. Therefore, the aim of this study was to further investigate the target cells of vibration during fracture healing and the role of estrogen-dependent and -independent ERα signaling particularly in cells of the osteoblast lineage.
In the present study, mice lacking the ERα receptors on osteoblast-lineage cells were protected from ovariectomy-induced compromised fracture healing, indicating that the ERα in these cells contributes to the pathomechanisms of osteoporosis-induced impaired bone repair. As expected, osteoblast number and activity were reduced in ERαfl/fl mice after ovariectomy, whereas these parameters were unaltered in OVX ERαfl/fl; Runx2Cre mice. This suggests that ligand-independent ERα signaling negatively regulates osteoblast formation and activity in the fracture callus of estrogen-deficient mice during bone regeneration. Regarding the role of ERα during fracture healing, to our knowledge, there is only one other study using mice with an ERα deletion in mature osteoblasts and osteocytes that uses a monocortical defect model on the tibiae of female mice (Ikedo and Imai, 2021). In agreement with our findings, they also reported that the ERα on mature osteoblasts controls osteoblast number and osteoblast surface. However, their defect model does not represent a full fracture of the bone, thus lacking the endochondral ossification process. Another difference between the two studies is that Ikedo et al. deleted the ERα by expressing the Cre recombinase under the control of the osteocalcin promotor, whereas we used the Runx2-Cre model. In comparison to osteocalcin, which is expressed by mature osteoblasts, Runx2 is necessary for the differentiation of osteoblastic precursors, thus the deletion of the ERα receptors on osteoblast lineage cells affected these cells earlier (Komori et al., 1998).
The presence or absence of estrogen plays a crucial role in regulating the mechanotransduction pathways upon LMHFV. In this study, we confirmed that while the application of LMHFV impaired bone formation in the fracture callus of non-OVX ERαfl/fl mice, it improved the healing outcome in OVX ERαfl/fl animals, consistent with previous reports (Stuermer et al., 2010; Cheung et al., 2012; Wehrle et al., 2014; Wehrle et al., 2015; Chow et al., 2016; Haffner-Luntzer et al., 2018a). We demonstrated that the vibration-induced effects on fracture healing are mediated via osteoblast lineage-specific ERα signaling because the effects of vibration on bone repair were abolished or even reversed in osteoblast lineage-specific ERα-KO animals (ERαfl/fl; Runx2Cre). On a cellular level, osteoblast number and surface were unaffected by LMHFV in non-OVX KO animals, whereas a reduction of both parameters was found in the fracture callus of OVX ERαfl/fl; Runx2Cre mice that were subjected to LMHFV. Histological characterization of the fractures revealed the persistence of residual cartilage in OVX ERαfl/fl; Runx2Cre mice that were subjected to vibration, consistent with the impaired healing and the poor biomechanical properties of the fractured bones. We conclude from our data that ligand-dependent ERα signaling in osteoblast lineage cells might be responsible for the negative effects of LMHFV in estrogen-competent mice and that ligand-independent ERα signaling in osteoblast lineage cells is important for the positive effects of LMHFV under estrogen-deficient conditions. The reasons why deletion of the ERα specifically on cells of the osteoblast lineage even reversed the effects of vibration in OVX mice remains to be elucidated.
To further investigate which pathways might contribute to the vibration-mediated improved bone healing under estrogen-deficient conditions, we performed microarray-based gene expression analysis in vitro in vibrated osteogenic cells. We identified the Cox2/PGE2 and Wnt pathway as critical signaling pathways that are regulated by LMHFV under estrogen-deficient conditions in MC3T3-E1 cells. Specifically, our array data revealed that Cox2, involved in prostaglandin biosynthesis, and the prostaglandin receptor EP4 were significantly upregulated. Cox2 is well known to be induced by mechanical strain and was recently reported to be regulated by LMHFV in vitro (Haffner-Luntzer et al., 2018b). In this study, siRNA knockdown of ERα in MC3T3 cells followed by LMHFV both with and without estrogen supplementation was performed, showing that Cox2 expression is significantly increased upon LMHFV in the absence of estrogen and significantly reduced by LMHFV in the presence of estrogen (Haffner-Luntzer et al., 2018b). Furthermore, it was demonstrated by Haffner-Luntzer et al., that these effects of LMHFV on Cox2 expression were both abrogated by siRNA knockdown of ERα. Additionally, the metabolic activity was also upregulated by vibration in an estrogen-depleted environment, but not in the presence of estrogen. In primary osteoblasts isolated from C57BL/6J wildtype mice, the significant downregulation of Cox2 upon vibration in estrogen-supplemented medium was confirmed, whereas this effect was abrogated in primary osteoblasts derived from ERα general KO mice (Haffner-Luntzer et al., 2018b). Because of these data and the fact that Cox2 upregulation is indispensable for mechanotranduction, we performed all in vitro experiments in an estrogen-depleted environment and with the same vibration regime that was used in our previous study (Haffner-Luntzer et al., 2018b).
Studies using mechanical loading via dynamic cell stretching (Liedert et al., 2010) or via fluid shear stress (Yeh et al., 2010) have shown a synergistic effect of estrogen and mechanical stimulation regarding Cox2 upregulation in osteoblasts, however, this effect might not account for vibration and seems to be dependent on the type of stimulus. Regarding fracture healing, studies with Cox2-selective inhibitors or Cox2-KO mouse models demonstrated a significantly disturbed bone healing (Simon et al., 2002; Zhang et al., 2002), indicating an important role of this pathway during bone regeneration. This is supported by a study with local Cox2 overexpression at the fracture site revealing an acceleration of fracture healing (Lau et al., 2013). Furthermore, the application of EP4 receptor agonist was shown to rescue impaired fracture healing in Cox2-KO mice (Xie et al., 2009), which highlights the importance of Cox2-and EP4 for endochondral bone repair. These studies together with our array data might explain the improved bone healing by LMHFV in our OVX ERαfl/fl animals, possibly mediated via Cox2 upregulation. We have further provided evidence for Cox2 upregulation upon LMHFV in hMSCs and that several miRNAs might also be involved in the effects of LMHFV on hMSCs. However, while these findings regarding the regulation of miRNAs upon vibration are interesting, they still require further detailed investigation in future projects.
Regarding the molecular mechanism that is involved in Cox2-induced osteogenesis, it has been recently demonstrated that the Cox2/PGE2/EP4 axis is involved in mechanosensing of primary cilia after LMHFV (Li et al., 2021). Primary cilia act as important mechanosensors on osteoblasts, with EP4 was found to be expressed at the bases of these cilia. Under LMHFV treatment, MC3T3-E1 cells were shown to upregulate EP4 and displayed a lower number and length of cilia. By blocking the EP4 receptor, primary cilia mechanotransduction and LMHFV-mediated osteogenesis were abrogated, suggesting a crosstalk of primary cilia and the Cox2/PGE2/EP4 pathway to facilitate mechanotransduction signaling.
One signaling pathway that might be activated by the Cox2/PGE2/EP4-mediated mechanotransduction is the Wnt signaling pathway. Wnt signaling plays a crucial role in bone homeostasis and fracture healing, whereby an activated Wnt signaling is associated with an improved fracture repair (Kim et al., 2007; Chen and Alman, 2009), whereas inhibited Wnt signaling led to delayed fracture healing (Huang et al., 2012; Beier et al., 2014; Haffner-Luntzer et al., 2014; Liedert et al., 2014; Haffner-Luntzer et al., 2016; Teng et al., 2018). In our microarray data, we found a downregulation of canonical Wnt/β-catenin pathway inhibitors (Axin2, Sostdc1, Dkk2), indicating that the canonical Wnt pathway is activated by LMHFV. This was further substantiated by the study of Gao et al. showing that vibration treatment of primary osteoblasts resulted in an upregulation of the Wnt signaling genes Wnt3a, Lrp6 and β-catenin in vitro (Gao et al., 2017). By contrast, the Wnt receptor Fzd9, which is essential for activating the non-canonical Wnt pathway, was downregulated. It is known that Fzd9-KO mice display an impaired bone strength during fracture healing, whereas it was found that the canonical Wnt signaling was unaffected by Fzd9 deficiency in osteoblasts (Heilmann et al., 2013). Therefore, the mechanism involved in the downregulation of Fzd9 and its effects on osteogenesis after LMHFV is not completely clear. Even so, our data strongly suggests that the canonical Wnt signaling is activated upon LMHFV via downregulation of Wnt inhibitory molecules. Several studies suggested a strong association between Cox2 and the Wnt/β-catenin signaling, showing that Cox2 regulates the transcriptional and translational levels of β-catenin (Zheng et al., 2019) and that Cox2 is important for osteoblast maturation via Wnt pathway genes (Nagano et al., 2017). Other studies reported that PGE2 is able to induce bone formation via promoting the production of insulin-like growth factor 1 (IGF-1) and activating the protein kinase B (Akt) (Sunters et al., 2010; Xia et al., 2010). Since there is no data available regarding a possible connection between Cox2/PGE2 and Wnt signaling in the context of LMHFV, we did perform in vitro experiments with EP4 or Cox2 inhibitors and could prove that the presence of Cox2 or EP4 is crucial for LMHFV-induced Lef1 upregulation in MC3T3-E1 cells in vitro, indicating an interaction of both pathways upon LMHFV.
We therefore hypothesize that mechanotransduction in vivo first requires estrogen-independent ERα signaling in osteoblast lineage cells, which subsequently triggers Cox2 expression and PGE2 production that might lead to differentially expressed Wnt-related target genes. Ultimately, this might favor an activation of the Wnt pathway, which could further promote fracture healing.
A limitation of our study was that we used the OVX model to induce estrogen-deficiency. Because the ovaries are not the only source of estrogen, small amounts of circulating estrogen might still be present in OVX mice (Haisenleder et al., 2011). Another limitation is that we used a diaphyseal femur fracture model which does not reflect the most common fracture sites (vertebral compression fractures, hip fractures, distal radius fractures, proximal humerus fractures) or the fracture type (metaphyseal), that commonly occur in postmenopausal women. However, it was shown that molecular mechanisms did not differ greatly between diaphyseal and metaphyseal fracture healing (Haffner-Luntzer et al., 2020).
Furthermore, with the performed three-point bending test, we did not evaluate ultimate bone strength. This test is also critically dependent on the callus geometry, which was not consistent between all mice. Therefore, a torsion test would be the best way to analyze mechanical stability, however, there is also evidence that torsion testing is not superior to our three-point bending test in small rodents (Steiner et al., 2015). Also, it should be also considered that hypertrophic chondrocytes in the cartilaginous fracture callus undergoing transdifferentiation to osteoblasts (Zhou et al., 2014) might express the Cre recombinase under the Runx2-promotor and might also be affected by the KO. Therefore, we cannot discriminate in our model between the effects of LMHFV on endochondral ossification and direct ossification, which both occur in the fracture callus in the chosen surgical model. Another limitation is that we did not compare all eight experimental groups to each other to investigate direct interaction between genotype and OVX/vibration treatment. This was due to considerations to reduce the number of needed animals.
In conclusion, our study suggests a critical role of the ERα in osteoblast lineage cells during mechanotransduction in the fracture callus both under estrogen-deficient and -sufficient conditions. This work provides further insights into the molecular mechanism responsible for the effects of estrogen or estrogen-deficiency on mechanostimulation during fracture healing and might result in improved treatment strategies for osteoporotic fracture patients, because we demonstrated that in addition to the estrogen status, the presence of ERα is important for mediating the positive effects of LMHFV on fracture healing. To ensure a safe treatment with LMHFV, further research is needed to define eligible patient cohorts that would benefit most from this treatment.
Data Availability Statement
The original contributions presented in the study are included in the article/Supplementary Material, further inquiries can be directed to the corresponding author.
Ethics Statement
The animal study was reviewed and approved by Regierungspräsidium Tübingen, Germany.
Author Contributions
LS: conceptualization, investigation, visualization, data analysis, writing original draft; BK: investigation; MT: visualization; VF: visualization; JT: provided mouse models; AI: conceptualization, funding acquisition, writing original draft, supervision; MH-L: conceptualization, investigation, funding acquisition, writing original draft, supervision.
Funding
This research was supported by the German Research Foundation (DFG, Project-ID HA 8470/1-1; Collaborative Research Center CRC1149, Project-ID 251293561) and an ASBMR Rising Star Award to MH-L.
Conflict of Interest
The authors declare that the research was conducted in the absence of any commercial or financial relationships that could be construed as a potential conflict of interest.
Publisher’s Note
All claims expressed in this article are solely those of the authors and do not necessarily represent those of their affiliated organizations, or those of the publisher, the editors and the reviewers. Any product that may be evaluated in this article, or claim that may be made by its manufacturer, is not guaranteed or endorsed by the publisher.
Acknowledgments
We thank Kristina Karremann, Tina Hieber, Andrea Böhmler and Iris Baum for excellent technical assistance.
Supplementary Material
The Supplementary Material for this article can be found online at: https://www.frontiersin.org/articles/10.3389/fbioe.2021.782355/full#supplementary-material
Supplementary Figure S1 | : Osteoblasts, osteocytes and hypertrophic chondrocytes are affected by the deletion of ERα, but not bone marrow cells. Immunohistochemical staining for ERα were performed on paraffin sections of (A,C) ERαfl/fl and (B,D) ERαfl/fl; Runx2Cre mice. Representative images of the fracture callus, growth plate and bone marrow are shown. Scale bar = 100 μm in overview images of the fracture callus and 50 μm in images 1–9; g= gap.
Supplementary Figure S2 | : Influence of OVX treatment on trabecular bone parameters. μCT analysis of the left femora was performed to determine the relative bone volume (BV/TV) in the trabecular bone compartment of ERαfl/fl (A) and ERαfl/fl; Runx2Cre (B) mice. The trabecular number (Tb.N) was also determined in ERαfl/fl (C) and ERαfl/fl; Runx2Cre (D) mice. Data are shown as box-and-whisker plots (with median and interquartile range) from max. to min., showing all data points. *indicates significant effects with p ≤ 0.05; n = 4–5.
Supplementary Figure S3 | : Influence of the respective genotype on fracture healing outcome on day 21. Biomechanical testing data (bending stiffness), bony bridging score and BV/TV of the fracture calli from control groups were directly compared between ERαfl/fl and ERαfl/fl; Runx2Cre mice. Data are shown as box-and-whisker plots (with median and interquartile range) from max. to min., showing all data points. *indicates significant effects with p ≤ 0.05.
Supplementary Figure S4 | : Nondetailed STRING protein-association network (action) derived from the MC3T3-E1 microarray data in high quality.
References
Almeida, M., Laurent, M. R., Dubois, V., Claessens, F., O'Brien, C. A., Bouillon, R., et al. (2017). Estrogens and Androgens in Skeletal Physiology and Pathophysiology. Physiol. Rev. 97, 135–187. doi:10.1152/physrev.00033.2015
Bahney, C. S., Zondervan, R. L., Allison, P., Theologis, A., Ashley, J. W., Ahn, J., et al. (2019). Cellular Biology of Fracture Healing. J. Orthop. Res. 37, 35–50. doi:10.1002/jor.24170
Beier, E. E., Sheu, T.-j., Buckley, T., Yukata, K., O'Keefe, R., Zuscik, M. J., et al. (2014). Inhibition of Beta-Catenin Signaling by Pb Leads to Incomplete Fracture Healing. J. Orthop. Res. 32, 1397–1405. doi:10.1002/JOR.22677
Beil, F. T., Barvencik, F., Gebauer, M., Seitz, S., Rueger, J. M., Ignatius, A., et al. (2010). Effects of Estrogen on Fracture Healing in Mice. J. Trauma 69, 1259–1265. doi:10.1097/TA.0b013e3181c4544d
Bouxsein, M. L., Boyd, S. K., Christiansen, B. A., Guldberg, R. E., Jepsen, K. J., and Müller, R. (2010). Guidelines for Assessment of Bone Microstructure in Rodents Using Micro-computed Tomography. J. Bone Miner. Res. 25, 1468–1486. doi:10.1002/jbmr.141
Cauley, J. A. (2015). Estrogen and Bone Health in Men and Women. Steroids 99, 11–15. doi:10.1016/J.STEROIDS.2014.12.010
Chen, Y., and Alman, B. A. (2009). Wnt Pathway, an Essential Role in Bone Regeneration. J. Cel. Biochem. 106, 353–362. doi:10.1002/JCB.22020
Cheung, W.-H., Sun, M.-H., Zheng, Y.-P., Chu, W. C.-W., Leung, A. H.-C., Qin, L., et al. (2012). Stimulated Angiogenesis for Fracture Healing Augmented by Low-Magnitude, High-Frequency Vibration in A Rat Model-Evaluation of Pulsed-Wave Doppler, 3-D Power Doppler Ultrasonography and Micro-CT Microangiography. Ultrasound Med. Biol. 38, 2120–2129. doi:10.1016/j.ultrasmedbio.2012.07.025
Cheung, W. H., Miclau, T., Chow, S. K.-H., Yang, F. F., and Alt, V. (2016). Fracture Healing in Osteoporotic Bone. Injury 47, S21–S26. doi:10.1016/S0020-1383(16)47004-X
Chow, S. K. H., Leung, K. S., Qin, J., Guo, A., Sun, M., Qin, L., et al. (2016). Mechanical Stimulation Enhanced Estrogen Receptor Expression and Callus Formation in Diaphyseal Long Bone Fracture Healing in Ovariectomy-Induced Osteoporotic Rats. Osteoporos. Int. 27, 2989–3000. doi:10.1007/s00198-016-3619-2
Chung, S.-L., Leung, K.-S., and Cheung, W.-H. (2014). Low-magnitude High-Frequency Vibration Enhances Gene Expression Related to Callus Formation, Mineralization and Remodeling during Osteoporotic Fracture Healing in Rats. J. Orthop. Res. 32, 1572–1579. doi:10.1002/jor.22715
Claes, L. E., Heigele, C. A., Neidlinger-Wilke, C., Kaspar, D., Seidl, W., Margevicius, K. J., et al. (1998). Effects of Mechanical Factors on the Fracture Healing Process. Clin. Orthopaedics Relat. Res. 355S, S132–S147. doi:10.1097/00003086-199810001-00015
Claes, L. (2017). Mechanobiologie der Frakturheilung Teil 1. Unfallchirurg 120, 14–22. doi:10.1007/s00113-016-0280-3
Claes, L., Wilke, H.-J., Augat, P., Rübenacker, S., and Margevicius, K. (1995). Effect of Dynamization on gap Healing of Diaphyseal Fractures under External Fixation. Clin. Biomech. 10, 227–234. doi:10.1016/0268-0033(95)99799-8
Dempster, D. W., Compston, J. E., Drezner, M. K., Glorieux, F. H., Kanis, J. A., Malluche, H., et al. (2013). Standardized Nomenclature, Symbols, and Units for Bone Histomorphometry: A 2012 Update of the Report of the ASBMR Histomorphometry Nomenclature Committee. J. Bone Miner. Res. 28, 2–17. doi:10.1002/jbmr.1805
Dirckx, N., Van Hul, M., and Maes, C. (2013). Osteoblast Recruitment to Sites of Bone Formation in Skeletal Development, Homeostasis, and Regeneration. Birth Defect Res. C 99, 170–191. doi:10.1002/bdrc.21047
Edwards, J. H., and Reilly, G. C. (2015). Vibration Stimuli and the Differentiation of Musculoskeletal Progenitor Cells: Review of Resultsin Vitroandin Vivo. Wjsc 7, 568. doi:10.4252/wjsc.v7.i3.568
Gao, H., Zhai, M., Wang, P., Zhang, X., Cai, J., Chen, X., et al. (2017). Low-level Mechanical Vibration Enhances Osteoblastogenesis via a Canonical Wnt Signaling-Associated Mechanism. Mol. Med. Rep. 16, 317–324. doi:10.3892/mmr.2017.6608
Haffner‐Luntzer, M., Weber, B., Lam, C., Fischer, V., Lackner, I., Ignatius, A., et al. (2020). A Novel Mouse Model to Study Fracture Healing of the Proximal Femur. J. Orthop. Res. 38, 2131–2138. doi:10.1002/jor.24677
Haffner-Luntzer, M., Fischer, V., Prystaz, K., Liedert, A., and Ignatius, A. (2017). The Inflammatory Phase of Fracture Healing Is Influenced by Oestrogen Status in Mice. Eur. J. Med. Res. 22, 23. doi:10.1186/s40001-017-0264-y
Haffner-Luntzer, M., Heilmann, A., Rapp, A. E., Beie, S., Schinke, T., Amling, M., et al. (2014). Midkine-deficiency Delays Chondrogenesis during the Early Phase of Fracture Healing in Mice. PLoS One 9, e116282. doi:10.1371/journal.pone.0116282
Haffner-Luntzer, M., Kemmler, J., Heidler, V., Prystaz, K., Schinke, T., Amling, M., et al. (2016). Inhibition of Midkine Augments Osteoporotic Fracture Healing. PLoS One 11, e0159278. doi:10.1371/journal.pone.0159278
Haffner-Luntzer, M., Kovtun, A., Lackner, I., Mödinger, Y., Hacker, S., Liedert, A., et al. (2018a). Estrogen Receptor α- (ERα), but Not ERβ-Signaling, Is Crucially Involved in Mechanostimulation of Bone Fracture Healing by Whole-Body Vibration. Bone 110, 11–20. doi:10.1016/j.bone.2018.01.017
Haffner-Luntzer, M., Lackner, I., Liedert, A., Fischer, V., and Ignatius, A. (2018b). Effects of Low-Magnitude High-Frequency Vibration on Osteoblasts Are Dependent on Estrogen Receptor α Signaling and Cytoskeletal Remodeling. Biochem. Biophysical Res. Commun. 503, 2678–2684. doi:10.1016/j.bbrc.2018.08.023
Haffner-Luntzer, M., Liedert, A., and Ignatius, A. (2015). Mechanobiologie und Knochenstoffwechsel. Unfallchirurg 118, 1000–1006. doi:10.1007/s00113-015-0102-z
Haisenleder, D. J., Schoenfelder, A. H., Marcinko, E. S., Geddis, L. M., and Marshall, J. C. (2011). Estimation of Estradiol in Mouse Serum Samples: Evaluation of Commercial Estradiol Immunoassays. Endocrinology 152, 4443–4447. doi:10.1210/EN.2011-1501
He, Y.-X., Zhang, G., Pan, X.-H., Liu, Z., Zheng, L.-z., Chan, C.-W., et al. (2011). Impaired Bone Healing Pattern in Mice with Ovariectomy-Induced Osteoporosis: A Drill-Hole Defect Model. Bone 48, 1388–1400. doi:10.1016/j.bone.2011.03.720
Heilmann, A., Schinke, T., Bindl, R., Wehner, T., Rapp, A., Haffner-Luntzer, M., et al. (2013). The Wnt Serpentine Receptor Frizzled-9 Regulates New Bone Formation in Fracture Healing. PLoS One 8, e84232–10. doi:10.1371/journal.pone.0084232
Huang, Y., Zhang, X., Du, K., Yang, F., Shi, Y., Huang, J., et al. (2012). Inhibition of β-catenin Signaling in Chondrocytes Induces Delayed Fracture Healing in Mice. J. Orthop. Res. 30, 304–310. doi:10.1002/jor.21505
Ikedo, A., and Imai, Y. (2021). Estrogen Receptor α in Mature Osteoblasts Regulates the Late Stage of Bone Regeneration. Biochem. Biophysical Res. Commun. 559, 238–244. doi:10.1016/j.bbrc.2021.04.112
Jessop, H. L., Suswillo, R. F., Rawlinson, S. C., Zaman, G., Lee, K., Das-Gupta, V., et al. (2004). Osteoblast-Like Cells from Estrogen Receptor α Knockout Mice Have Deficient Responses to Mechanical Strain. J. Bone Miner. Res. 19, 938–946. doi:10.1359/jbmr.2004.19.6.938
Kim, J.-B., Leucht, P., Lam, K., Luppen, C., Ten Berge, D., Nusse, R., et al. (2007). Bone Regeneration Is Regulated by Wnt Signaling. J. Bone Miner. Res. 22, 1913–1923. doi:10.1359/JBMR.070802
Kim, W.-Y., Han, C.-H., Park, J.-I., and Kim, J.-Y. (2001). Failure of Intertrochanteric Fracture Fixation with a Dynamic Hip Screw in Relation to Pre-operative Fracture Stability and Osteoporosis. Int. Orthopaedics (Sicot) 25, 360–362. doi:10.1007/s002640100287
Komori, T., Yagi, H., Nomura, S., and Yamaguchi, A. (1998). Targeted Disruption of Cbfa1 Results in a Complete Lack of Bone Formation Owing to Maturational Arrest of Osteoblasts. Proc. Natl. Acad. Sci. U. S. A. 95, 8692–8697. doi:10.1073/pnas.95.15.8692
Komrakova, M., Sehmisch, S., Tezval, M., Ammon, J., Lieberwirth, P., Sauerhoff, C., et al. (2013). Identification of a Vibration Regime Favorable for Bone Healing and Muscle in Estrogen-Deficient Rats. Calcif. Tissue Int. 92, 509–520. doi:10.1007/s00223-013-9706-x
Lau, K.-H. W., Kothari, V., Das, A., Zhang, X.-B., and Baylink, D. J. (2013). Cellular and Molecular Mechanisms of Accelerated Fracture Healing by COX2 Gene Therapy. Bone 53, 369–381. doi:10.1016/J.BONE.2013.01.003
Lee, K., Jessop, H., Suswillo, R., Zaman, G., and Lanyon, L. (2003). Bone Adaptation Requires Oestrogen Receptor-α. Nature 424, 389. doi:10.1038/424389a
Leung, K. S., Li, C. Y., Tse, Y. K., Choy, T. K., Leung, P. C., Hung, V. W. Y., et al. (2014). Effects of 18-month Low-Magnitude High-Frequency Vibration on Fall Rate and Fracture Risks in 710 Community Elderly-A Cluster-Randomized Controlled Trial. Osteoporos. Int. 25, 1785–1795. doi:10.1007/s00198-014-2693-6
Li, Y., Liu, G., Yu, J., Li, C., Tan, L., Hao, B., et al. (2018). Effects of Continuous or Intermittent Low-Magnitude High-Frequency Vibration on Fracture Healing in Sheep. Int. Orthopaedics (Sicot) 42, 939–946. doi:10.1007/s00264-018-3759-4
Li, Y.H., Zhu, D., Yang, T., Cheng, L., Sun, J., and Tan, L. (2021). Crosstalk Between The COX2-PGE2-EP4 Signaling Pathway And Primary Cilia In Osteoblasts After Mechanical Stimulation. J. Cell Physiol. 236, 4764–4777. doi:10.1002/jcp.30198
Liedert, A., Röntgen, V., Schinke, T., Benisch, P., Ebert, R., Jakob, F., et al. (2014). Osteoblast-specific Krm2 Overexpression and Lrp5 Deficiency Have Different Effects on Fracture Healing in Mice. PLoS One 9, e103250. doi:10.1371/journal.pone.0103250
Liedert, A., Wagner, L., Seefried, L., Ebert, R., Jakob, F., and Ignatius, A. (2010). Estrogen Receptor and Wnt Signaling Interact to Regulate Early Gene Expression in Response to Mechanical Strain in Osteoblastic Cells. Biochem. Biophysical Res. Commun. 394, 755–759. doi:10.1016/j.bbrc.2010.03.065
Manolagas, S. C., Bellido, T., and Jilka, R. L. (1995). Sex Steroids, Cytokines and the Bone Marrow: New Concepts on the Pathogenesis of Osteoporosis. Ciba Found. Symp. 191, 187–202. doi:10.1002/9780470514757.ch11
Mödinger, Y., Rapp, A., Pazmandi, J., Vikman, A., Holzmann, K., Haffner-Luntzer, M., et al. (2018). C5aR1 Interacts with TLR2 in Osteoblasts and Stimulates the Osteoclast-Inducing Chemokine CXCL10. J. Cel. Mol. Med. 22, 6002–6014. doi:10.1111/jcmm.13873
Morgan, E. F., Mason, Z. D., Chien, K. B., Pfeiffer, A. J., Barnes, G. L., Einhorn, T. A., et al. (2009). Micro-computed Tomography Assessment of Fracture Healing: Relationships Among Callus Structure, Composition, and Mechanical Function. Bone 44, 335–344. doi:10.1016/j.bone.2008.10.039
Nagano, A., Arioka, M., Takahashi-Yanaga, F., Matsuzaki, E., and Sasaguri, T. (2017). Celecoxib Inhibits Osteoblast Maturation by Suppressing the Expression of Wnt Target Genes. J. Pharmacol. Sci. 133, 18–24. doi:10.1016/j.jphs.2016.11.003
Nikolaou, V. S., Efstathopoulos, N., Kontakis, G., Kanakaris, N. K., and Giannoudis, P. V. (2009). The Influence of Osteoporosis in Femoral Fracture Healing Time. Injury 40, 663–668. doi:10.1016/j.injury.2008.10.035
Perren, S. M. (1979). Physical and Biological Aspects of Fracture Healing with Special Reference to Internal Fixation. Clin. Orthop. Relat. Res. 138, 175–196.
Röntgen, V., Blakytny, R., Matthys, R., Landauer, M., Wehner, T., Göckelmann, M., et al. (2010). Fracture Healing in Mice under Controlled Rigid and Flexible Conditions Using an Adjustable External Fixator. J. Orthop. Res. 28, 1456–1462. doi:10.1002/jor.21148
Rubin, C., Recker, R., Cullen, D., Ryaby, J., McCabe, J., and McLeod, K. (2004). Prevention of Postmenopausal Bone Loss by a Low-Magnitude, High-Frequency Mechanical Stimuli: A Clinical Trial Assessing Compliance, Efficacy, and Safety. J. Bone Miner. Res. 19, 343–351. doi:10.1359/JBMR.0301251
Seitz, S., Keller, J., Schilling, A. F., Jeschke, A., Marshall, R. P., Stride, B. D., et al. (2012). Pharmacological Estrogen Administration Causes a FSH-independent Osteo-Anabolic Effect Requiring ER Alpha in Osteoblasts. PLoS One 7, e50301–9. doi:10.1371/journal.pone.0050301
Shi, H.-F., Cheung, W.-H., Qin, L., Leung, A. H.-C., and Leung, K.-S. (2010). Low-magnitude High-Frequency Vibration Treatment Augments Fracture Healing in Ovariectomy-Induced Osteoporotic Bone. Bone 46, 1299–1305. doi:10.1016/j.bone.2009.11.028
Simon, A. M., Manigrasso, M. B., and O'Connor, J. P. (2002). Cyclo-oxygenase 2 Function Is Essential for Bone Fracture Healing. J. Bone Miner. Res. 17, 963–976. doi:10.1359/jbmr.2002.17.6.963
Steiner, M., Volkheimer, D., Meyers, N., Wehner, T., Wilke, H.-J., Claes, L., et al. (2015). Comparison between Different Methods for Biomechanical Assessment of Ex Vivo Fracture Callus Stiffness in Small Animal Bone Healing Studies. PLoS ONE 10, e0119603–16. doi:10.1371/journal.pone.0119603
Steppe, L., Liedert, A., Ignatius, A., and Haffner-Luntzer, M. (2020). Influence of Low-Magnitude High-Frequency Vibration on Bone Cells and Bone Regeneration. Front. Bioeng. Biotechnol. 8, 595139. doi:10.3389/fbioe.2020.595139
Stuermer, E. K., Komrakova, M., Werner, C., Wicke, M., Kolios, L., Sehmisch, S., et al. (2010). Musculoskeletal Response to Whole-Body Vibration during Fracture Healing in Intact and Ovariectomized Rats. Calcif. Tissue Int. 87, 168–180. doi:10.1007/s00223-010-9381-0
Sunters, A., Armstrong, V. J., Zaman, G., Kypta, R. M., Kawano, Y., Lanyon, L. E., et al. (2010). Mechano-transduction in Osteoblastic Cells Involves Strain-Regulated Estrogen Receptor α-mediated Control of Insulin-like Growth Factor (IGF) I Receptor Sensitivity to Ambient IGF, Leading to Phosphatidylinositol 3-Kinase/AKT-dependent Wnt/LRP5 Receptor-independent Activation of β-Catenin Signaling. J. Biol. Chem. 285, 8743–8758. doi:10.1074/jbc.M109.027086
Teng, J. W., Ji, P. F., and Zhao, Z. G. (2018). MiR-214-3p Inhibits β-catenin Signaling Pathway Leading to Delayed Fracture Healing. Eur. Rev. Med. Pharmacol. Sci. 22, 17–24. doi:10.26355/eurrev_201801_14095
Thompson, W. R., Yen, S. S., and Rubin, J. (2014). Vibration Therapy. Curr. Opin. Endocrinol. Diabetes Obes. 21, 447–453. doi:10.1097/MED.0000000000000111
Wang, J., Leung, K. S., Leung, K., Chow, S., and Cheung, W. (2017). The Effect of Whole Body Vibration on Fracture Healing - a Systematic Review. eCM 34, 108–127. doi:10.22203/eCM.v034a08
Wehrle, E., Liedert, A., Heilmann, A., Wehner, T., Bindl, R., Fischer, L., et al. (2015). The Impact of Low-Magnitude High-Frequency Vibration on Fracture Healing Is Profoundly Influenced by the Oestrogen Status in Mice. DMM Dis. Model. Mech. 8, 93–104. doi:10.1242/dmm.018622
Wehrle, E., Wehner, T., Heilmann, A., Bindl, R., Claes, L., Jakob, F., et al. (2014). Distinct Frequency Dependent Effects of Whole-Body Vibration on Non-fractured Bone and Fracture Healing in Mice. J. Orthop. Res. 32, 1006–1013. doi:10.1002/jor.22629
Wildemann, B., Ignatius, A., Leung, F., Taitsman, L. A., Smith, R. M., Pesántez, R., et al. (2021). Non-union Bone Fractures. Nat. Rev. Dis. Primers 7, 57. doi:10.1038/S41572-021-00289-8
Windahl, S. H., Saxon, L., Börjesson, A. E., Lagerquist, M. K., Frenkel, B., Henning, P., et al. (2013). Estrogen Receptor‐α Is Required for the Osteogenic Response to Mechanical Loading in a Ligand‐independent Manner Involving its Activation Function 1 but Not 2. J. Bone Miner. Res. 28, 291–301. doi:10.1002/jbmr.1754
Xia, X., Batra, N., Shi, Q., Bonewald, L. F., Sprague, E., and Jiang, J. X. (2010). Prostaglandin Promotion of Osteocyte Gap Junction Function through Transcriptional Regulation of Connexin 43 by Glycogen Synthase Kinase 3/β-Catenin Signaling. Mol. Cel. Biol. 30, 206–219. doi:10.1128/mcb.01844-08
Xie, C., Liang, B., Xue, M., Lin, A. S. P., Loiselle, A., Schwarz, E. M., et al. (2009). Rescue of Impaired Fracture Healing in COX-2−/− Mice via Activation of Prostaglandin E2 Receptor Subtype 4. Am. J. Pathol. 175, 772–785. doi:10.2353/AJPATH.2009.081099
Yeh, C.-R., Chiu, J.-J., Lee, C.-I., Lee, P.-L., Shih, Y.-T., Sun, J.-S., et al. (2010). Estrogen Augments Shear Stress-Induced Signaling and Gene Expression in Osteoblast-like Cells via Estrogen Receptor-Mediated Expression of β1-integrin. J. Bone Miner. Res. 25, 627–639. doi:10.1359/jbmr.091008
Zeeberg, B. R., Feng, W., Wang, G., Wang, M. D., Fojo, A. T., Sunshine, M., et al. (2003). GoMiner: a Resource for Biological Interpretation of Genomic and Proteomic Data. Genome Biol. 4, R28. doi:10.1186/gb-2003-4-4-r28
Zhang, X., Schwarz, E. M., Young, D. A., Puzas, J. E., Rosier, R. N., and O’Keefe, R. J. (2002). Cyclooxygenase-2 Regulates Mesenchymal Cell Differentiation into the Osteoblast Lineage and Is Critically Involved in Bone Repair. J. Clin. Invest. 109, 1405–1415. doi:10.1172/JCI1568110.1172/jci0215681
Zheng, C., Qu, Y. X., Wang, B., Shen, P. F., Xu, J. D., and Chen, Y. X. (2019). COX-2/PGE2 Facilitates Fracture Healing by Activating the Wnt/β-Catenin Signaling Pathway. Eur. Rev. Med. Pharmacol. Sci. 23, 9721–9728. doi:10.26355/eurrev_201911_19534
Zhou, X., von der Mark, K., Henry, S., Norton, W., Adams, H., and de Crombrugghe, B. (2014). Chondrocytes Transdifferentiate into Osteoblasts in Endochondral Bone during Development, Postnatal Growth and Fracture Healing in Mice. Plos Genet. 10, e1004820. doi:10.1371/journal.pgen.1004820
Keywords: osteoblasts, estrogen receptor signaling, whole-body vibration, fracture healing, LMHFV, wnt signaling, prostaglandin signaling
Citation: Steppe L, Krüger BT, Tschaffon MEA, Fischer V, Tuckermann J, Ignatius A and Haffner-Luntzer M (2021) Estrogen Receptor α Signaling in Osteoblasts is Required for Mechanotransduction in Bone Fracture Healing. Front. Bioeng. Biotechnol. 9:782355. doi: 10.3389/fbioe.2021.782355
Received: 24 September 2021; Accepted: 24 November 2021;
Published: 07 December 2021.
Edited by:
Dmitriy Sheyn, Board of Governors Regenerative Medicine Institute, United StatesReviewed by:
Evan Buettmann, Virginia Commonwealth University, United StatesMelodie Metzger, Cedars Sinai Medical Center, United States
Doron Cohn-Schwartz, Rambam Health Care Campus, Israel
Copyright © 2021 Steppe, Krüger, Tschaffon, Fischer, Tuckermann, Ignatius and Haffner-Luntzer. This is an open-access article distributed under the terms of the Creative Commons Attribution License (CC BY). The use, distribution or reproduction in other forums is permitted, provided the original author(s) and the copyright owner(s) are credited and that the original publication in this journal is cited, in accordance with accepted academic practice. No use, distribution or reproduction is permitted which does not comply with these terms.
*Correspondence: Melanie Haffner-Luntzer, bWVsYW5pZS5oYWZmbmVyLWx1bnR6ZXJAdW5pLXVsbS5kZQ==
†ORCID ID: Lena Steppe, orcid.org/0000-0003-0968-5627