- 1School of Chemical Engineering and Technology, Hebei University of Technology, Tianjin, China
- 2CAS Center for Excellence in Nanoscience, CAS Key Laboratory for Biomedical Effects of Nanomaterials and Nanosafety, National Center for Nanoscience and Technology (NCNST), Beijing, China
- 3Center of Materials Science and Optoelectronics Engineering, University of Chinese Academy of Sciences, Beijing, China
Mitochondria are well known to serve as the powerhouse for cells and also the initiator for some vital signaling pathways. A variety of diseases are discovered to be associated with the abnormalities of mitochondria, including cancers. Thus, targeting mitochondria and their metabolisms are recognized to be promising for cancer therapy. In recent years, great efforts have been devoted to developing mitochondria-targeted pharmaceuticals, including small molecular drugs, peptides, proteins, and genes, with several molecular drugs and peptides enrolled in clinical trials. Along with the advances of nanotechnology, self-assembled peptide-nanomaterials that integrate the biomarker-targeting, stimuli-response, self-assembly, and therapeutic effect, have been attracted increasing interest in the fields of biotechnology and nanomedicine. Particularly, in situ mitochondria-targeted self-assembling peptides that can assemble on the surface or inside mitochondria have opened another dimension for the mitochondria-targeted cancer therapy. Here, we highlight the recent progress of mitochondria-targeted peptide-nanomaterials, especially those in situ self-assembly systems in mitochondria, and their applications in cancer treatments.
Introduction
Mitochondria, the dynamic sub-organelles in mammalian cells, are well known to be involved in the generation of adenosine triphosphate (ATP) (Roger et al., 2017). They are composed of mitochondrial membranes that include a porous outer membrane and an inner membrane with a space, and a mitochondrial matrix inside (Frey and Mannella, 2000). With their own genome and fission signatures (Gray et al., 1999; Jiao et al., 2021; Kleele et al., 2021), mitochondria also participate in other essential physiological functions in the body, such as macromolecule biosynthesis (Spinelli and Haigis, 2018) and cell proliferation (Diebold et al., 2019), differentiation (Seo et al., 2018), apoptosis (Bock and Tait, 2020), information transmission (Chandel, 2015), etc. Dysfunctions of mitochondria are discovered to be associated with a series of diseases that threaten human health (Nunnari and Suomalainen, 2012), including neurodegeneration (Devine and Kittler, 2018), cardiovascular disease (Siasos et al., 2018), and cancer (Ward and Thompson, 2012). Increasing evidence has revealed the relevance between the energetic production, metabolic biosynthesis, and singling pathways of mitochondria with the carcinogenesis (Weinberg and Chandel, 2015). Therefore, the mitochondrion has been recognized as a promising target to improve cancer therapeutics (Fulda et al., 2010; Vasan et al., 2020; Gao et al., 2021).
Currently, mitochondria can be intervened by either using the mitochondria-targeted reagents or modulating the specific targets, gene transcriptions, and kinase activities within or outside mitochondria (Smith et al., 2012). Particularly, with the discovery of several types of targeted compounds, the mitochondria-targeted approach attracts increasing attentions (Zinovkin and Zamyatnin, 2019). For instance, lipophilic cations, such as triphenylphosphonium (TPP) and dequalinium, are first discovered to target mitochondria (Murphy and Smith, 2007). TPP contains a positively charged phosphorus atom delocalized over three hydrophobic benzene rings (Liberman et al., 1969). The unique structure allows TPP to target the mitochondrial membrane due to the negative membrane potential and the favored activation energy when crossing phospholipid bilayers, resulting in a thousand-fold enhancement in the mitochondrial accumulation (Murphy and Hartley, 2018). Till now, two TPP-based small molecular antioxidants, MitoQ and SkQ1 (Kelso et al., 2001; Skulachev, 2007), have been enrolled in clinical trials for treatments of Parkinson disease, chronic kidney disease, and hepatitis C, as well as the dry-eye syndrome, respectively (Battogtokh et al., 2018; Kang, 2018; Jeena MT. et al., 2020). The second category is the mitochondria-targeted peptides, mainly including Szeto-Schiller (SS) peptides and mitochondria-penetrating peptides (MMPs) (Horton et al., 2008; Li and Huang, 2020). These peptides usually consist of hydrophobic and positively charged amino acids, which are assumed to target mitochondria driven by the negative membrane potential and the interaction with phospholipids on mitochondrial inner membranes (Zhao et al., 2004; Birk et al., 2013; Jean et al., 2016). One formulation of SS peptide (MTP-131) has been enrolled in clinical trials for treatments of heart attack and skeletal muscle mitochondrial dysfunction in the elderly. Besides, the mitochondrial precursor protein is a natural mitochondria-targeted species, which enters mitochondria via the mitochondrial protein import machinery (Neupert and Herrmann, 2007). These proteins contain a cleavable N-terminal targeted sequence, which is cleaved by mitochondrial peptidases once entering mitochondria (Wasilewski et al., 2017). In recent years, self-assembled peptide-nanomaterials are emerging as a new type of mitochondria-targeted category, due to their designable feature to combine the targeting, biological responsive, self-assembling, and therapeutic properties (Qi et al., 2018).
In this review, we focus on the mitochondria-targeted self-assembled peptide-nanomaterials developed in recent years. The latest strategies and advances for constructing self-assembling peptides that target and assemble in mitochondria are highlighted, including the equipment of mitochondria-targeted ligands, the introduction of a stimuli-responsive mechanism to trigger the self-assembly in situ, and so on. Meanwhile, we briefly discuss the application of these nanomaterials in cancer therapy.
Self-Assembling Peptide
Peptides are usually defined as the biomacromolecules that contain less than 50 amino acids linked by peptide bonds, with the intrinsic characteristics of folding and bioactivities like recognition and response. Since the emergences of technologies for the peptide manufacture and screening, especially the solid-phase peptide synthesis proposed by Merrifield in 1963 (Merrifield, 1963) and the phage display described by Smith in 1985 (Smith, 1985), numerous peptide-based pharmaceuticals and functional materials have been developed (Figure 1A) (Hosoyama et al., 2019; Li et al., 2020; Lopez-Silva and Schneider, 2021; Muttenthaler et al., 2021). Particularly, self-assembling peptides have attracted increasing interest due to their improved stability and biological performance (Guyon et al., 2018; Levin et al., 2020), and have been applied in a wide range of fields including the tissue engineering (Gelain et al., 2020; Zhang et al., 2021), drug delivery (Moitra et al., 2014; Abbas et al., 2017; Yang J. et al., 2020; Kumar et al., 2020; Ji et al., 2021), catalysis (Rufo et al., 2014; Wang M. et al., 2020; Liu Q. et al., 2021; Chen et al., 2021), semi-conducting device (Tao et al., 2017), and energy materials (Hu et al., 2018; Lee et al., 2018; Nguyen et al., 2021). With the molecular basis to form secondary structures including the α-helix and β-sheet, the self-assembling peptides can assemble into well-defined nanostructures like nanofibrils driven by non-covalent interactions, such as the hydrophobic interaction, electrostatic interaction, π-π stacking, hydrogen bond, etc (Hendricks et al., 2017; Hu et al., 2020; Zheng et al., 2021). For instance, the di-phenylalanine peptide (FF), the most widely investigated self-assembling peptide, can assemble into either the nanofiber, nanotube, nanosphere, or nanoarray on the surface, by using properly mixed solvents and kinetic controls, or vapor deposition (Reches and Gazit, 2003; Wei et al., 2017; Zhao et al., 2019). The rapid development of self-assembling peptides can be traced back to 1990s (Zhang, 2020). The peptides in early researches are limited and mainly inspired from natural proteins especially amyloid proteins (Zhang, 2003), like FF and KLVFF. The developments of machine learning (Jumper et al., 2021) and one-bead one-compound (OBOC) combinatorial library (Lam et al., 1991) technologies make the screening of self-assembling peptides in a large-scale manner possible. For instance, Frederix et al. developed a computational simulation tool based on the aggregation propensity and amphiphilicity of peptides, to rapidly screen the self-assembling tripeptides in all 8,000 possible sequences and guided the discoveries of several unreported tripeptides that could form hydrogels (Frederix et al., 2015). In another work, Li et al. focused on the relationship between the chemical structures of peptides and their albitites to form hydrogels, applying machine learning to generate a hydrogel library with more than 2,000 self-assembling dipeptides (Li F. et al., 2019). Besides computational simulations (Moitra et al., 2017), Yang et al. recently capped a hydrophobicity-sensitive probe, the nitro-1,2,3-benzoxadiazole (NBD), to the N-terminus of peptides on beads in the OBOC library, achieving the rapid screening of self-assembling pentapeptides experimentally (Yang et al., 2021).
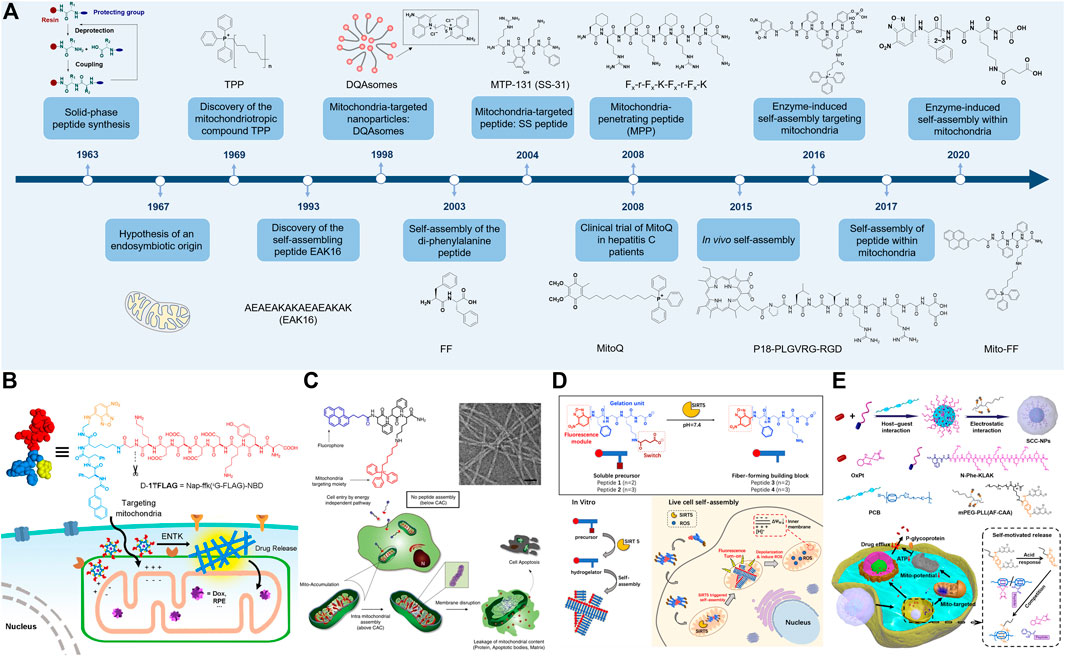
FIGURE 1. (A) A brief timeline of selected events for mitochondria-targeted self-assembly of peptide-nanomaterials. The references are shown in Supplementary Table S1. (B) In situ enzyme-instructed self-assembly of branched peptides around mitochondria. Reproduced with permission from ref (He et al., 2018). Copyright 2018 American Chemical Society. (C) In situ self-assembly of peptide amphiphiles in mitochondria due to the enhanced accumulation by targeting. Reproduced with permission under a Creative Commons CC BY License from ref (Jeena et al., 2017). Copyright 2017 Springer Nature. (D) In situ enzyme-instructed self-assembly of peptides in mitochondria. Reproduced with permission from ref (Yang L. et al., 2020). Copyright 2020 American Chemical Society. (E) Self-motivated release of the mitochondria-cytotoxic peptide to strengthen the chemotherapy toward drug-resistant cancer cells. This figure has been published in CCS Chemistry 2021; Self-Motivated Supramolecular Combination Chemotherapy for Overcoming Drug Resistance Based on Acid-Activated Competition of Host–Guest Interactions is available online at 10.31635/ccschem.021.202100964; https://www.chinesechemsoc.org/doi/10.31635/ccschem.021.202100964.
Self-Assembled Peptide-Nanomaterials for Targeting Mitochondria
In recent years, targeted drug delivery systems have shown promising potentials in precision and personalized medicine, with reduced side effects (Yi et al., 2019; Mi et al., 2020; Manzari et al., 2021). In this regard, the integration with the biomarker-targeting, enzyme-response, and treatment makes the self-assembling peptides as candidates for functional nanomaterials and smart nanomedicines more than scaffolds in hydrogels. This endeavor has been promoted by the establishment of several important concepts including the peptide amphiphiles (Lowik and van Hest, 2004; Cui et al., 2010), the enzyme-instructed self-assembly (EISA) (Zhou and Xu, 2015; He et al., 2020c), and the in vivo self-assembly (Zhang et al., 2015; He P.-P. et al., 2020; Mamuti et al., 2021). Since mitochondria serve as a potential target for cancers (Guo et al., 2021; Liew et al., 2021), dozens of mitochondria-targeted self-assembled peptide-nanomaterials have been reported (Table 1), including the pre-assembled peptide-nanomaterials that locate mitochondria and in situ self-assembling peptides that assemble in mitochondria. In this section, we discuss the former.
A typical approach to construct the mitochondria-targeted self-assembling peptide is to equip a targeting motif to a self-assembling peptide. For instance, Standley et al. combined the α-helical (KLAKLAK)2 (KLAK) peptide, a cytotoxic peptide that breaks mitochondrial membranes (Ellerby et al., 1999), with a hydrophobic alkyl tail and a β-sheet forming peptide to afford a mitochondria-targeted peptide amphiphile (Standley et al., 2010). This peptide amphiphile could assemble into nanofibers, which were demonstrated to enter the breast cancer cells, then locate and disrupt the mitochondrial membranes. To increase the targeting efficacy, stimuli such as the endogenous enzyme, redox, and acidic environments, as well as the exogenous light and ultrasound, are employed to guide the targeted self-assemblies (Chen et al., 2018b; Jiang et al., 2021). For instance, Wang et al. reported an alkaline phosphatase (ALP)-instructed self-assembling peptide for targeting mitochondria (Wang et al., 2016). The peptide consisted of a mitochondria-targeting motif (TPP), a self-assembling backbone (FFYK), an enzyme-responsive phosphorylated tyrosine, and a fluorophore (NBD). Upon dephosphorylation by ALP overexpressed on cancer cell membranes, the peptide became more hydrophobic and self-assembled into nanofibers. After endocytosis and endosomal escape, the peptide-assemblies accumulated to mitochondria assisted by TPP, resulting in mitochondrial dysfunction and cell death. Notably, under the experimental concentration, the peptide did not form assemblies in the low ALP-expressed HS-5 (normal human bone marrow stromal) cells.
Despite the successes in cultured cells, the self-assembling peptides still face the intrinsic nature of instability in physiological environments, as well as several physiological barriers when applied in the body, such as the rapid clearance in blood, uncontrolled transportation to diseased tissues, insufficient cellular internalization and endosomal escape, etc (Sun et al., 2017). To improve the biological performances in the body, Wang and coworkers reported a series of polymer-peptide conjugates (PPCs) as in vivo self-assembled nanomedicines (Li LL. et al., 2019). For instance, Cong et al. reported a type of PPCs that targeted tumoral mitochondria with long blood circulation times (Cong et al., 2019). The PPCs consisted of a poly (β-thioester) backbone and KLAK peptides modified with pH-cleavable cis-aconitic anhydride (CAA) moieties and cell-penetrating peptides (TAT: CYGRKKRRQRRR) on the side chains. Upon systemic administration, the hydrophilic PPCs remained soluble as monomers for circulating long in the bloodstream and penetrating deeply into the solid tumors. Once arrived at the tumor tissue, the acidic tumor microenvironment trigged the cleaving of the hydrophilic CAA moieties on PPCs, resulting in the formation of 100 nm-sized nanoparticles decorated with KLAK and TAT peptides. These newly formed nanoparticles entered cancer cells facilitated by TAT peptides, then further located mitochondria and induced apoptosis associated with KLAK peptides.
In Situ Self-Assembly of Peptide-Nanomaterials in Mitochondria
In the previous section, we discussed the assembled peptide-nanomaterials that locate mitochondria. Recently, the in situ self-assembly attracts much attention due to its spatiotemporal precision and activable bioeffects, emerging as a frontier in the biomedical field (Deng et al., 2021; Kwek et al., 2021; Liu et al., 2021a; Wang et al., 2021b). The specific enzymes and overexpressed ROS can be used to trigger the self-assembly around or on the surface of mitochondria in situ. As shown in Figure 1B, He et al. reported a mitochondrial enterokinase (ENTK)-instructed branched peptide for self-assembly around mitochondria (He et al., 2018). A hydrophilic ENTK-cleavable Flag-tag (DDDDK) was conjugated to the peptide scaffold, resulting in a micelle-like structure. After cell internalization, the micelles transformed into nanofibers mainly at mitochondria due to the enzymatic cleaving by ENTK. This construction of self-assembling peptides has successfully delivered the chloramphenicol and histone proteins (H2B) to mitochondria of cancer cells in vitro (He et al., 2020a; He et al., 2020b). Furthermore, to achieve mitochondria-targeting in the body, Cheng et al. constructed a type of ROS-trigged morphology-transformable PPCs driven by the responsive detachment of poly (ethylene glycol) (PEG) chains (Cheng et al., 2019). The PPCs consisted of a poly (vinyl alcohol) backbone with side chain-modifications of PEGylated KLVFF peptides linked by ROS-cleavable thioketals and KLAK peptides. After administration, the micelle-like PPCs transported in the bloodstream with the shield of PEG chains. Once closing to mitochondria, the over-generated ROS cleaved the thioketal linker to detach PEG chains, resulting in a transformation of micelles to nanofibers that exposed KLAK peptides to disrupt mitochondrial membranes.
To further achieve the precise self-assembly of peptides inside mitochondria, the targeted accumulation-induced assembly and the intramitochondrial protease-instructed assembly have shown promising potentials. Since enough concentration higher than the critical aggregation concentration (CAC) is the basis for molecular assemblies, making the self-assembling peptides with a spatial concentration above CAC in mitochondria selectively and a concentration below CAC in the cytoplasm is a feasible approach to achieve in situ self-assembly in mitochondria. For instance, Jeena et al. reported a system of in situ self-assembly (Mito-FF) that assembled inside mitochondria through targeted accumulation-induced assembly (Figure 1C) (Jeena et al., 2017). Mito-FF consisted of FFK peptide backbone with a fluorescent pyrene at the N-terminus and TPP at the lysine side chain, with a CAC of 60 µM. Using a culture medium containing 5 and 10 µM Mito-FF, the amphiphilic Mito-FF could efficiently and selectively gather inside mitochondria of cervical cancer (HeLa) cells with a concentration of 3 and 11 mM, respectively, thereby aggregating into nanofibers inside mitochondria in situ. Besides the targeted accumulation-induced aggregation, Yang et al. recently utilized the mitochondrial localization enzyme sirtuin 5 (SIRT5) to induce peptide self-assembly inside mitochondria in situ (Figure 1D) (Yang L. et al., 2020). The peptide had a backbone of FFFGKG, with a fluorescent probe NBD at the N-terminus and a succinylated lysine residue. Once entering into mitochondria, the peptide was desuccinylated by SIRT5 to become more hydrophobic, resulting in the formation of nanofibers inside mitochondria.
Applications in Cancer Therapy
Although peptides and peptide-based vaccines and nanomaterials have been frequently reported for cancer therapy (Chiangjong et al., 2020; Malonis et al., 2020; Rong et al., 2020; Fan et al., 2021), the self-assembled peptide-nanomaterials especially those targeting mitochondria as anticancer reagents are still in the primary stage. Currently, approaches of these nanomaterials for cancer therapy mainly include the delivery of anticancer drugs, destructions of mitochondria by mitochondria-cytotoxic peptides or peptide-assemblies, and the combination with chemotherapy or photothermal therapy (PTT) (Supplementary Table S2). For instance, to deliver the chloramphenicol selectively to mitochondria, He et al. employed ENTK-instructed self-assembled peptide-nanoparticles as nanocarriers (He et al., 2020b). Once arrived at mitochondria, the hydrophilic Flag-tag on the peptide was cleaved by ENTK, resulting in the release of chloramphenicol to mitochondria. The chloramphenicol further interrupted the mitochondrial metabolism by inhibiting the synthesis of mitochondrial proteins, resulting in the release of cytochrome c for apoptosis. This self-assembled nanoparticle showed selective cytotoxicity to tumor cells, with lower half-maximal inhibitory concentration (IC50) values toward human hepatoma (HepG2) cells (54 µM) and HeLa cells (73 µM) than those for normal HS-5 cells (143 µM) and murine hepatocyte (AML12) cells (142 µM). The selectivity might be due to either the lack of ENTK enzymes in HS-5 cells or the less polarized mitochondria in AML12 cells. In addition, the peptide-assemblies in mitochondria also show strong anticancer activities. For instance, Jeena et al. showed that the co-assembly of Mito-FF and its mirror formulation Mito-ff led to nanofibers with a diameter of 100 nm, resulting in the enhanced antitumor efficacy in subcutaneous colorectal adenocarcinoma (HT-29)-bearing mouse model via intraperitoneal injections (Jeena et al., 2019).
To realize the mitochondria-cytotoxic peptides for improved cancer therapy, Qiao et al. developed a type of PPCs containing KLAK peptides prepared by Michael-type addition (Qiao et al., 2016a). This synthetic method allowed the facile conjugations of targeted and therapeutic peptides together with PEG chains to a polymer backbone, achieving both the improved biological stability and enhanced anticancer efficacy toward the subcutaneous glioblastoma (U87)-bearing mouse model. However, this covalent approach suffers from the long reaction time (e.g., 2 days) and the competitive reactions from the thiol and amine groups. To construct a fast and simple method for systemic delivery of peptide pharmaceuticals, Wang et al. based on the concept of non-covalent supramolecular chemotherapy (Chen et al., 2017; Chen et al., 2018a; Wu et al., 2021), proposed a strategy of supramolecular peptide therapeutics (Wang H. et al., 2020). Owning to the strong host-guest interaction between the cucurbit[7]uril (CB[7]) and N-terminal phenylalanine (N-Phe) residue in the peptide (binding constant ∼2 × 106 M−1), the N-Phe-containing KLAK peptides were carried by the CB[7]-PEG copolymers in a simple (mixing in the aqueous solution) and fast (several minutes) manner with a high peptide encapsulation efficiency (>97%) under the peptide concentration of 0.5 mM. This strategy achieved prolonged blood circulation (25% remained at 1 h after intravenous injections compared with 13% for the peptide alone), enhanced tumor accumulation (2.8-fold enhancement), and increased anticancer efficacy (4-fold enhancement in the tumor inhibition rate) toward the subcutaneous colorectal tumor (HCT116)-bearing mouse model via intravenous injections, with minimal hematologic, hepatic, and nephric toxicities. In addition, to overcome the drug resistance of cancer cells, the same group further combined the oxaliplatin and KLAK peptide that disrupted ATP generations synergistically, in an acid-trigged on-demand drug release system (Figure 1E), achieving the improved anticancer activity toward oxaliplatin-resistant HCT116 cells, with IC50 decreased from 76.5 µM (oxaliplatin) to 31.2 µM (Wang H. et al., 2021). Besides chemotherapy, Zhang et al. utilized the photothermal effect irradiated by the near-infrared (NIR) light to promote the self-assembly of a purpurin-18-containing peptide, resulting in a four times increase in the self-assembly rate and a 2-fold enhancement in the tumor accumulation (Zhang et al., 2020).
Conclusion and Outlook
The advances in nanotechnology and biotechnology have witnessed the great progress of the self-assembling peptides from simple scaffolds of hydrogels to smart nanomaterials for versatile biomedical applications, bridging the gap between simple synthetic molecules with sophisticated biological machinery in the body. Owning to the advantages including the excellent biocompatibility and the inclusivity for multiple biological and physicochemical activities, the self-assembling peptide achieves the self-assembled accuracy from levels of the tissue, through cells, to cellular organelles. However, several challenges still exist for the further development of mitochondria-targeted self-assembling peptides. The first is the precise self-assembly. Besides the membrane potential, enzyme, and ROS, other candidates such as the mitochondrial protein import machinery and nucleic acids (Zhao et al., 2021) are also promising targets. The second one is the characterization in situ. High-resolution, real-time, and in situ technologies are highly desirable to investigate the process and kinetics of the self-assembly in organelles. The third one is the rapid design and screening. The technology of machine learning may provide a high-throughput method to develop self-assembling peptides equipped with multiple bioactive and functional modules. Last but not least, biological safety should be highly concerned. Since the prolonged retention nature of the peptide nanofibers, careful studies in the degradation, metabolism, and long-term toxicology of the self-assembling peptides are important for their further clinical applications. Despite the challenges, we believe the self-assembled peptide-nanomaterials, especially the organelle-precise self-assembling peptides, will contribute to the new paradigms of biomedical technologies and products.
Author Contributions
All authors listed have made a substantial, direct, and intellectual contribution to the work and approved it for publication.
Funding
This research was financially supported by the National Key R&D Program of China (No. 2018YFE0205400) and the National Natural Science Foundation of China (Nos. 21805058 and 51890892).
Conflict of Interest
The authors declare that the research was conducted in the absence of any commercial or financial relationships that could be construed as a potential conflict of interest.
Publisher’s Note
All claims expressed in this article are solely those of the authors and do not necessarily represent those of their affiliated organizations, or those of the publisher, the editors and the reviewers. Any product that may be evaluated in this article, or claim that may be made by its manufacturer, is not guaranteed or endorsed by the publisher.
Supplementary Material
The Supplementary Material for this article can be found online at: https://www.frontiersin.org/articles/10.3389/fbioe.2021.782234/full#supplementary-material
References
Abbas, M., Zou, Q., Li, S., and Yan, X. (2017). Self-Assembled Peptide- and Protein-Based Nanomaterials for Antitumor Photodynamic and Photothermal Therapy. Adv. Mater. 29, 1605021. doi:10.1002/adma.201605021
Battogtokh, G., Choi, Y. S., Kang, D. S., Park, S. J., Shim, M. S., Huh, K. M., et al. (2018). Mitochondria-Targeting Drug Conjugates for Cytotoxic, Anti-Oxidizing and Sensing Purposes: Current Strategies and Future Perspectives. Acta Pharm. Sin. B 8, 862–880. doi:10.1016/j.apsb.2018.05.006
Birk, A. V., Liu, S., Soong, Y., Mills, W., Singh, P., Warren, J. D., et al. (2013). The Mitochondrial-Targeted Compound SS-31 Re-Energizes Ischemic Mitochondria by Interacting with Cardiolipin. J. Am. Soc. Nephrol. 24, 1250–1261. doi:10.1681/asn.2012121216
Bock, F. J., and Tait, S. W. G. (2020). Mitochondria as Multifaceted Regulators of Cell Death. Nat. Rev. Mol. Cell Biol. 21, 85–100. doi:10.1038/s41580-019-0173-8
Chandel, N. S. (2015). Evolution of Mitochondria as Signaling Organelles. Cell Metab. 22, 204–206. doi:10.1016/j.cmet.2015.05.013
Chandra Saha, P., Das, R. S., Chatterjee, T., Bhattacharyya, M., and Guha, S. (2020). Supramolecular β-Sheet Forming Peptide Conjugated with Near-Infrared Chromophore for Selective Targeting, Imaging, and Dysfunction of Mitochondria. Bioconjug. Chem. 31, 1301–1306. doi:10.1021/acs.bioconjchem.0c00153
Chen, H., Chen, Y., Wu, H., Xu, J.-F., Sun, Z., and Zhang, X. (2018a). Supramolecular Polymeric Chemotherapy Based on Cucurbit[7]uril-PEG Copolymer. Biomaterials 178, 697–705. doi:10.1016/j.biomaterials.2018.02.051
Chen, H., Gu, Z., An, H., Chen, C., Chen, J., Cui, R., et al. (2018b). Precise Nanomedicine for Intelligent Therapy of Cancer. Sci. China Chem. 61, 1503–1552. doi:10.1007/s11426-018-9397-5
Chen, Y., Huang, Z., Zhao, H., Xu, J.-F., Sun, Z., and Zhang, X. (2017). Supramolecular Chemotherapy: Cooperative Enhancement of Antitumor Activity by Combining Controlled Release of Oxaliplatin and Consuming of Spermine by Cucurbit[7]uril. ACS Appl. Mater. Inter. 9, 8602–8608. doi:10.1021/acsami.7b01157
Chen, Y., Yang, Y., Orr, A. A., Makam, P., Redko, B., Haimov, E., et al. (2021). Self‐Assembled Peptide Nano‐Superstructure towards Enzyme Mimicking Hydrolysis. Angew. Chem. Int. Ed. 60, 17164–17170. doi:10.1002/anie.202105830
Cheng, D.-B., Yang, P.-P., Cong, Y., Liu, F.-H., Qiao, Z.-Y., and Wang, H. (2017). One-Pot Synthesis of pH-Responsive Hyperbranched Polymer-Peptide Conjugates with Enhanced Stability and Loading Efficiency for Combined Cancer Therapy. Polym. Chem. 8, 2462–2471. doi:10.1039/c7py00101k
Cheng, D.-B., Zhang, X.-H., Chen, Y., Chen, H., Qiao, Z.-Y., and Wang, H. (2020). Ultrasound-Activated Cascade Effect for Synergistic Orthotopic Pancreatic Cancer Therapy. iScience 23, 101144. doi:10.1016/j.isci.2020.101144
Cheng, D.-B., Zhang, X.-H., Gao, Y.-J., Ji, L., Hou, D., Wang, Z., et al. (2019). Endogenous Reactive Oxygen Species-Triggered Morphology Transformation for Enhanced Cooperative Interaction with Mitochondria. J. Am. Chem. Soc. 141, 7235–7239. doi:10.1021/jacs.8b07727
Chiangjong, W., Chutipongtanate, S., and Hongeng, S. (2020). Anticancer Peptide: Physicochemical Property, Functional Aspect and Trend in Clinical Application (Review). Int. J. Oncol. 57, 678–696. doi:10.3892/ijo.2020.5099
Chuah, J.-A., Matsugami, A., Hayashi, F., and Numata, K. (2016). Self-Assembled Peptide-Based System for Mitochondrial-Targeted Gene Delivery: Functional and Structural Insights. Biomacromolecules 17, 3547–3557. doi:10.1021/acs.biomac.6b01056
Cong, Y., Ji, L., Gao, Y. J., Liu, F. H., Cheng, D. B., Hu, Z., et al. (2019). Microenvironment‐Induced In Situ Self‐Assembly of Polymer-Peptide Conjugates that Attack Solid Tumors Deeply. Angew. Chem. Int. Ed. 58, 4632–4637. doi:10.1002/anie.201900135
Cui, H., Webber, M. J., and Stupp, S. I. (2010). Self-Assembly of Peptide Amphiphiles: From Molecules to Nanostructures to Biomaterials. Biopolymers 94, 1–18. doi:10.1002/bip.21328
Deng, Y., Zhan, W., and Liang, G. (2021). Intracellular Self-Assembly of Peptide Conjugates for Tumor Imaging and Therapy. Adv. Healthc. Mater. 10, e2001211. doi:10.1002/adhm.202001211
Devine, M. J., and Kittler, J. T. (2018). Mitochondria at the Neuronal Presynapse in Health and Disease. Nat. Rev. Neurosci. 19, 63–80. doi:10.1038/nrn.2017.170
Diebold, L. P., Gil, H. J., Gao, P., Martinez, C. A., Weinberg, S. E., and Chandel, N. S. (2019). Mitochondrial Complex III Is Necessary for Endothelial Cell Proliferation during Angiogenesis. Nat. Metab. 1, 158–171. doi:10.1038/s42255-018-0011-x
Ellerby, H. M., Arap, W., Ellerby, L. M., Kain, R., Andrusiak, R., Rio, G. D., et al. (1999). Anti-Cancer Activity of Targeted pro-Apoptotic Peptides. Nat. Med. 5, 1032–1038. doi:10.1038/12469
Fan, Y., Liu, S., Yi, Y., Rong, H., and Zhang, J. (2021). Catalytic Nanomaterials toward Atomic Levels for Biomedical Applications: From Metal Clusters to Single-Atom Catalysts. ACS Nano 15, 2005–2037. doi:10.1021/acsnano.0c06962
Frederix, P. W. J. M., Scott, G. G., Abul-Haija, Y. M., Kalafatovic, D., Pappas, C. G., Javid, N., et al. (2015). Exploring the Sequence Space for (Tri-)peptide Self-Assembly to Design and Discover New Hydrogels. Nat. Chem. 7, 30–37. doi:10.1038/nchem.2122
Frey, T. G., and Mannella, C. A. (2000). The Internal Structure of Mitochondria. Trends Biochem. Sci. 25, 319–324. doi:10.1016/s0968-0004(00)01609-1
Fulda, S., Galluzzi, L., and Kroemer, G. (2010). Targeting Mitochondria for Cancer Therapy. Nat. Rev. Drug Discov. 9, 447–464. doi:10.1038/nrd3137
Gao, Y., Tong, H., Li, J., Li, J., Huang, D., Shi, J., et al. (2021). Mitochondria-Targeted Nanomedicine for Enhanced Efficacy of Cancer Therapy. Front. Bioeng. Biotechnol. 9, 720508. doi:10.3389/fbioe.2021.720508
Gelain, F., Luo, Z., and Zhang, S. (2020). Self-Assembling Peptide EAK16 and RADA16 Nanofiber Scaffold Hydrogel. Chem. Rev. 120, 13434–13460. doi:10.1021/acs.chemrev.0c00690
Gray, M. W., Burger, G., and Lang, B. F. (1999). Mitochondrial Evolution. Science 283, 1476–1481. doi:10.1126/science.283.5407.1476
Guo, X., Yang, N., Ji, W., Zhang, H., Dong, X., Zhou, Z., et al. (2021). Mito‐Bomb: Targeting Mitochondria for Cancer Therapy. Adv. Mater. 33, 2007778. doi:10.1002/adma.202007778
Guyon, L., Lepeltier, E., and Passirani, C. (2018). Self-Assembly of Peptide-Based Nanostructures: Synthesis and Biological Activity. Nano Res. 11, 2315–2335. doi:10.1007/s12274-017-1892-9
He, H., Guo, J., Lin, X., and Xu, B. (2020a). Enzyme‐Instructed Assemblies Enable Mitochondria Localization of Histone H2B in Cancer Cells. Angew. Chem. Int. Ed. 59, 9330–9334. doi:10.1002/anie.202000983
He, H., Lin, X., Guo, J., Wang, J., and Xu, B. (2020b). Perimitochondrial Enzymatic Self-Assembly for Selective Targeting the Mitochondria of Cancer Cells. ACS Nano 14, 6947–6955. doi:10.1021/acsnano.0c01388
He, H., Tan, W., Guo, J., Yi, M., Shy, A. N., and Xu, B. (2020c). Enzymatic Noncovalent Synthesis. Chem. Rev. 120, 9994–10078. doi:10.1021/acs.chemrev.0c00306
He, H., Wang, J., Wang, H., Zhou, N., Yang, D., Green, D. R., et al. (2018). Enzymatic Cleavage of Branched Peptides for Targeting Mitochondria. J. Am. Chem. Soc. 140, 1215–1218. doi:10.1021/jacs.7b11582
He, P.-P., Li, X.-D., Fan, J.-Q., Fan, Y., Yang, P.-P., Li, B.-N., et al. (2020d). Live Cells Process Exogenous Peptide as Fibronectin Fibrillogenesis In Vivo. CCS Chem. 2, 539–554. doi:10.31635/ccschem.020.201900117
Hendricks, M. P., Sato, K., Palmer, L. C., and Stupp, S. I. (2017). Supramolecular Assembly of Peptide Amphiphiles. Acc. Chem. Res. 50, 2440–2448. doi:10.1021/acs.accounts.7b00297
Hong, T. H., Jeena, M. T., Kim, O.-H., Kim, K.-H., Choi, H. J., Lee, K. H., et al. (2021). Application of Self-Assembly Peptides Targeting the Mitochondria as a Novel Treatment for Sorafenib-Resistant Hepatocellular Carcinoma Cells. Sci. Rep. 11, 874. doi:10.1038/s41598-020-79536-z
Horton, K. L., Stewart, K. M., Fonseca, S. B., Guo, Q., and Kelley, S. O. (2008). Mitochondria-Penetrating Peptides. Chem. Biol. 15, 375–382. doi:10.1016/j.chembiol.2008.03.015
Hosoyama, K., Lazurko, C., Muñoz, M., Mctiernan, C. D., and Alarcon, E. I. (2019). Peptide-Based Functional Biomaterials for Soft-Tissue Repair. Front. Bioeng. Biotechnol. 7, 205. doi:10.3389/fbioe.2019.00205
Hu, K., Jiang, Y., Xiong, W., Li, H., Zhang, P. Y., Yin, F., et al. (2018). Tuning Peptide Self-Assembly by an In-Tether Chiral Center. Sci. Adv. 4, eaar5907. doi:10.1126/sciadv.aar5907
Hu, K., Xiong, W., Sun, C., Wang, C., Li, J., Yin, F., et al. (2020). Self-Assembly of Constrained Cyclic Peptides Controlled by Ring Size. CCS Chem. 2, 42–51. doi:10.31635/ccschem.020.201900047
Jean, S. R., Ahmed, M., Lei, E. K., Wisnovsky, S. P., and Kelley, S. O. (2016). Peptide-Mediated Delivery of Chemical Probes and Therapeutics to Mitochondria. Acc. Chem. Res. 49, 1893–1902. doi:10.1021/acs.accounts.6b00277
Jeena, M. T., Kim, S., Jin, S., and Ryu, J. H. (2020a). Recent Progress in Mitochondria-Targeted Drug and Drug-free Agents for Cancer Therapy. Cancers (Basel) 12, 4. doi:10.3390/cancers12010004
Jeena, M. T., Jeong, K., Go, E. M., Cho, Y., Lee, S., Jin, S., et al. (2019). Heterochiral Assembly of Amphiphilic Peptides inside the Mitochondria for Supramolecular Cancer Therapeutics. ACS Nano 13, 11022–11033. doi:10.1021/acsnano.9b02522
Jeena, M. T., Lee, S., Barui, A. K., Jin, S., Cho, Y., Hwang, S.-W., et al. (2020b). Intra-Mitochondria Self-Assembly to Overcome the Intracellular Enzymatic Degradation of L-Peptides. Chem. Commun. 56, 6265–6268. doi:10.1039/d0cc02029j
Jeena, M. T., Palanikumar, L., Go, E. M., Kim, I., Kang, M. G., Lee, S., et al. (2017). Mitochondria Localization Induced Self-Assembly of Peptide Amphiphiles for Cellular Dysfunction. Nat. Commun. 8, 26. doi:10.1038/s41467-017-00047-z
Ji, T., Li, Y., Deng, X., Rwei, A. Y., Offen, A., Hall, S., et al. (2021). Delivery of Local Anaesthetics by a Self-Assembled Supramolecular System Mimicking Their Interactions with a Sodium Channel. Nat. Biomed. Eng. 5, 1099–1109. doi:10.1038/s41551-021-00793-y
Jiang, T., Liu, C., Xu, X., He, B., and Mo, R. (2021). Formation Mechanism and Biomedical Applications of Protease-Manipulated Peptide Assemblies. Front. Bioeng. Biotechnol. 9, 598050. doi:10.3389/fbioe.2021.598050
Jiao, H., Jiang, D., Hu, X., Du, W., Ji, L., Yang, Y., et al. (2021). Mitocytosis, a Migrasome-Mediated Mitochondrial Quality-Control Process. Cell 184, 2896–2910. doi:10.1016/j.cell.2021.04.027
Jumper, J., Evans, R., Pritzel, A., Green, T., Figurnov, M., Ronneberger, O., et al. (2021). Highly Accurate Protein Structure Prediction with Alphafold. Nature 596, 583–589. doi:10.1038/s41586-021-03819-2
Kang, H. C. (2018). Mitochondria-Targeting Theranostics. Biomater. Res. 22, 34. doi:10.1186/s40824-018-0145-7
Kelso, G. F., Porteous, C. M., Coulter, C. V., Hughes, G., Porteous, W. K., Ledgerwood, E. C., et al. (2001). Selective Targeting of a Redox-Active Ubiquinone to Mitochondria within Cells. J. Biol. Chem. 276, 4588–4596. doi:10.1074/jbc.m009093200
Kleele, T., Rey, T., Winter, J., Zaganelli, S., Mahecic, D., Perreten Lambert, H., et al. (2021). Distinct Fission Signatures Predict Mitochondrial Degradation or Biogenesis. Nature 593, 435–439. doi:10.1038/s41586-021-03510-6
Kumar, K., Moitra, P., Bashir, M., Kondaiah, P., and Bhattacharya, S. (2020). Natural Tripeptide Capped pH-Sensitive Gold Nanoparticles for Efficacious Doxorubicin Delivery Both In Vitro and In Vivo. Nanoscale 12, 1067–1074. doi:10.1039/c9nr08475d
Kwek, G., Do, T. C., Lu, X., Lin, J., and Xing, B. (2021). Scratching the Surface of Unventured Possibilities with In Situ Self-Assembly: Protease-Activated Developments for Imaging and Therapy. ACS Appl. Bio Mater. 4, 2192–2216. doi:10.1021/acsabm.0c01340
Lam, K. S., Salmon, S. E., Hersh, E. M., Hruby, V. J., Kazmierski, W. M., and Knapp, R. J. (1991). A New Type of Synthetic Peptide Library for Identifying Ligand-Binding Activity. Nature 354, 82–84. doi:10.1038/354082a0
Lee, J.-H., Heo, K., Schulz-Schönhagen, K., Lee, J. H., Desai, M. S., Jin, H.-E., et al. (2018). Diphenylalanine Peptide Nanotube Energy Harvesters. ACS Nano 12, 8138–8144. doi:10.1021/acsnano.8b03118
Levin, A., Hakala, T. A., Schnaider, L., Bernardes, G. J. L., Gazit, E., and Knowles, T. P. J. (2020). Biomimetic Peptide Self-Assembly for Functional Materials. Nat. Rev. Chem. 4, 615–634. doi:10.1038/s41570-020-0215-y
Li, C., Iscen, A., Sai, H., Sato, K., Sather, N. A., Chin, S. M., et al. (2020). Supramolecular-Covalent Hybrid Polymers for Light-Activated Mechanical Actuation. Nat. Mater. 19, 900–909. doi:10.1038/s41563-020-0707-7
Li, F., Han, J., Cao, T., Lam, W., Fan, B., Tang, W., et al. (2019a). Design of Self-Assembly Dipeptide Hydrogels and Machine Learning via Their Chemical Features. Proc. Natl. Acad. Sci. USA 116, 11259–11264. doi:10.1073/pnas.1903376116
Li, L. L., Qiao, Z. Y., Wang, L., and Wang, H. (2019b). Programmable Construction of Peptide-Based Materials in Living Subjects: From Modular Design and Morphological Control to Theranostics. Adv. Mater. 31, e1804971. doi:10.1002/adma.201804971
Li, Q., and Huang, Y. (2020). Mitochondrial Targeted Strategies and Their Application for Cancer and Other Diseases Treatment. J. Pharm. Investig. 50, 271–293. doi:10.1007/s40005-020-00481-0
Liberman, E. A., Topaly, V. P., Tsofina, L. M., Jasaitis, A. A., and Skulachev, V. P. (1969). Mechanism of Coupling of Oxidative Phosphorylation and the Membrane Potential of Mitochondria. Nature 222, 1076–1078. doi:10.1038/2221076a0
Liew, S. S., Qin, X., Zhou, J., Li, L., Huang, W., and Yao, S. Q. (2021). Smart Design of Nanomaterials for Mitochondria‐Targeted Nanotherapeutics. Angew. Chem. Int. Ed. 60, 2232–2256. doi:10.1002/anie.201915826
Liu, D., Angelova, A., Liu, J., Garamus, V. M., Angelov, B., Zhang, X., et al. (2019). Self-Assembly of Mitochondria-Specific Peptide Amphiphiles Amplifying Lung Cancer Cell Death through Targeting the VDAC1-Hexokinase-II Complex. J. Mater. Chem. B 7, 4706–4716. doi:10.1039/c9tb00629j
Liu, F.-H., Cong, Y., Qi, G.-B., Ji, L., Qiao, Z.-Y., and Wang, H. (2018a). Near-Infrared Laser-Driven In Situ Self-Assembly as a General Strategy for Deep Tumor Therapy. Nano Lett. 18, 6577–6584. doi:10.1021/acs.nanolett.8b03174
Liu, F.-H., Hou, C.-Y., Zhang, D., Zhao, W.-J., Cong, Y., Duan, Z.-Y., et al. (2018b). Enzyme-Sensitive Cytotoxic Peptide-Dendrimer Conjugates Enhance Cell Apoptosis and Deep Tumor Penetration. Biomater. Sci. 6, 604–613. doi:10.1039/c7bm01182b
Liu, N., Zhu, L., Li, Z., Liu, W., Sun, M., and Zhou, Z. (2021a). In Situ Self-Assembled Peptide Nanofibers for Cancer Theranostics. Biomater. Sci. 9, 5427–5436. doi:10.1039/d1bm00782c
Liu, Q., Wan, K., Shang, Y., Wang, Z.-G., Zhang, Y., Dai, L., et al. (2021b). Cofactor-Free Oxidase-Mimetic Nanomaterials from Self-Assembled Histidine-Rich Peptides. Nat. Mater. 20, 395–402. doi:10.1038/s41563-020-00856-6
Lopez-Silva, T. L., and Schneider, J. P. (2021). From Structure to Application: Progress and Opportunities in Peptide Materials Development. Curr. Opin. Chem. Biol. 64, 131–144. doi:10.1016/j.cbpa.2021.06.006
Löwik, D. W. P. M., and Van Hest, J. C. M. (2004). Peptide Based Amphiphiles. Chem. Soc. Rev. 33, 234–245. doi:10.1039/b212638a
Malonis, R. J., Lai, J. R., and Vergnolle, O. (2020). Peptide-Based Vaccines: Current Progress and Future Challenges. Chem. Rev. 120, 3210–3229. doi:10.1021/acs.chemrev.9b00472
Mamuti, M., Zheng, R., An, H.-W., and Wang, H. (2021). In Vivo Self-Assembled Nanomedicine. Nano Today 36, 101036. doi:10.1016/j.nantod.2020.101036
Manzari, M. T., Shamay, Y., Kiguchi, H., Rosen, N., Scaltriti, M., and Heller, D. A. (2021). Targeted Drug Delivery Strategies for Precision Medicines. Nat. Rev. Mater. 6, 351–370. doi:10.1038/s41578-020-00269-6
Merrifield, R. B. (1963). Solid Phase Peptide Synthesis. I. The Synthesis of a Tetrapeptide. J. Am. Chem. Soc. 85, 2149–2154. doi:10.1021/ja00897a025
Mi, P., Cabral, H., and Kataoka, K. (2020). Ligand-Installed Nanocarriers toward Precision Therapy. Adv. Mater. 32, e1902604. doi:10.1002/adma.201902604
Moitra, P., Kumar, K., Kondaiah, P., and Bhattacharya, S. (2014). Efficacious Anticancer Drug Delivery Mediated by a pH-Sensitive Self-Assembly of a Conserved Tripeptide Derived from Tyrosine Kinase NGF Receptor. Angew. Chem. Int. Ed. 53, 1113–1117. doi:10.1002/anie.201307247
Moitra, P., Subramanian, Y., and Bhattacharya, S. (2017). Concentration Dependent Self-Assembly of TrK-NGF Receptor Derived Tripeptide: New Insights from Experiment and Computer Simulations. J. Phys. Chem. B 121, 815–824. doi:10.1021/acs.jpcb.6b10511
Murphy, M. P., and Hartley, R. C. (2018). Mitochondria as a Therapeutic Target for Common Pathologies. Nat. Rev. Drug Discov. 17, 865–886. doi:10.1038/nrd.2018.174
Murphy, M. P., and Smith, R. A. J. (2007). Targeting Antioxidants to Mitochondria by Conjugation to Lipophilic Cations. Annu. Rev. Pharmacol. Toxicol. 47, 629–656. doi:10.1146/annurev.pharmtox.47.120505.105110
Muttenthaler, M., King, G. F., Adams, D. J., and Alewood, P. F. (2021). Trends in Peptide Drug Discovery. Nat. Rev. Drug Discov. 20, 309–325. doi:10.1038/s41573-020-00135-8
Neupert, W., and Herrmann, J. M. (2007). Translocation of Proteins into Mitochondria. Annu. Rev. Biochem. 76, 723–749. doi:10.1146/annurev.biochem.76.052705.163409
Nguyen, T. P., Easley, A. D., Kang, N., Khan, S., Lim, S.-M., Rezenom, Y. H., et al. (2021). Polypeptide Organic Radical Batteries. Nature 593, 61–66. doi:10.1038/s41586-021-03399-1
Nunnari, J., and Suomalainen, A. (2012). Mitochondria: In Sickness and in Health. Cell 148, 1145–1159. doi:10.1016/j.cell.2012.02.035
Qi, G. B., Gao, Y. J., Wang, L., and Wang, H. (2018). Self-Assembled Peptide-Based Nanomaterials for Biomedical Imaging and Therapy. Adv. Mater. 30, e1703444. doi:10.1002/adma.201703444
Qiao, Z.-Y., Lai, W.-J., Lin, Y.-X., Li, D., Nan, X.-H., Wang, Y., et al. (2017a). Polymer-KLAK Peptide Conjugates Induce Cancer Cell Death through Synergistic Effects of Mitochondria Damage and Autophagy Blockage. Bioconjug. Chem. 28, 1709–1721. doi:10.1021/acs.bioconjchem.7b00176
Qiao, Z.-Y., Lin, Y.-X., Lai, W.-J., Hou, C.-Y., Wang, Y., Qiao, S.-L., et al. (2016a). A General Strategy for Facile Synthesis and In Situ Screening of Self-Assembled Polymer-Peptide Nanomaterials. Adv. Mater. 28, 1859–1867. doi:10.1002/adma.201504564
Qiao, Z.-Y., Zhao, W.-J., Cong, Y., Zhang, D., Hu, Z., Duan, Z.-Y., et al. (2016b). Self-Assembled ROS-Sensitive Polymer-Peptide Therapeutics Incorporating Built-In Reporters for Evaluation of Treatment Efficacy. Biomacromolecules 17, 1643–1652. doi:10.1021/acs.biomac.6b00041
Qiao, Z.-Y., Zhao, W.-J., Gao, Y.-J., Cong, Y., Zhao, L., Hu, Z., et al. (2017b). Reconfigurable Peptide Nanotherapeutics at Tumor Microenvironmental pH. ACS Appl. Mater. Inter. 9, 30426–30436. doi:10.1021/acsami.7b09033
Reches, M., and Gazit, E. (2003). Casting Metal Nanowires within Discrete Self-Assembled Peptide Nanotubes. Science 300, 625–627. doi:10.1126/science.1082387
Roger, A. J., Muñoz-Gómez, S. A., and Kamikawa, R. (2017). The Origin and Diversification of Mitochondria. Curr. Biol. 27, R1177–R1192. doi:10.1016/j.cub.2017.09.015
Rong, L., Lei, Q., and Zhang, X. Z. (2020). Recent Advances on Peptide-Based Theranostic Nanomaterials. View 1, 20200050. doi:10.1002/viw.20200050
Rufo, C. M., Moroz, Y. S., Moroz, O. V., Stöhr, J., Smith, T. A., Hu, X., et al. (2014). Short Peptides Self-Assemble to Produce Catalytic Amyloids. Nat. Chem. 6, 303–309. doi:10.1038/nchem.1894
Saha, P. C., Bera, T., Chatterjee, T., Samanta, J., Sengupta, A., Bhattacharyya, M., et al. (2021). Supramolecular Dipeptide-Based Near-Infrared Fluorescent Nanotubes for Cellular Mitochondria Targeted Imaging and Early Apoptosis. Bioconjug. Chem. 32, 833–841. doi:10.1021/acs.bioconjchem.1c00106
Seo, B., Yoon, S., and Do, J. (2018). Mitochondrial Dynamics in Stem Cells and Differentiation. Int. J. Mol. Sci. 19, 3893. doi:10.3390/ijms19123893
Siasos, G., Tsigkou, V., Kosmopoulos, M., Theodosiadis, D., Simantiris, S., Tagkou, N. M., et al. (2018). Mitochondria and Cardiovascular Diseases-From Pathophysiology to Treatment. Ann. Transl. Med. 6, 256. doi:10.21037/atm.2018.06.21
Skulachev, V. P. (2007). A Biochemical Approach to the Problem of Aging: "Megaproject" on Membrane-Penetrating Ions. The First Results and Prospects. Biochem. Mosc. 72, 1385–1396. doi:10.1134/s0006297907120139
Smith, G. P. (1985). Filamentous Fusion Phage: Novel Expression Vectors that Display Cloned Antigens on the Virion Surface. Science 228, 1315–1317. doi:10.1126/science.4001944
Smith, R. A. J., Hartley, R. C., Cochemé, H. M., and Murphy, M. P. (2012). Mitochondrial Pharmacology. Trends Pharmacol. Sci. 33, 341–352. doi:10.1016/j.tips.2012.03.010
Spinelli, J. B., and Haigis, M. C. (2018). The Multifaceted Contributions of Mitochondria to Cellular Metabolism. Nat. Cell Biol. 20, 745–754. doi:10.1038/s41556-018-0124-1
Standley, S. M., Toft, D. J., Cheng, H., Soukasene, S., Chen, J., Raja, S. M., et al. (2010). Induction of Cancer Cell Death by Self-Assembling Nanostructures Incorporating a Cytotoxic Peptide. Cancer Res. 70, 3020–3026. doi:10.1158/0008-5472.can-09-3267
Sun, Q., Zhou, Z., Qiu, N., and Shen, Y. (2017). Rational Design of Cancer Nanomedicine: Nanoproperty Integration and Synchronization. Adv. Mater. 29, 1606628. doi:10.1002/adma.201606628
Tao, K., Makam, P., Aizen, R., and Gazit, E. (2017). Self-Assembling Peptide Semiconductors. Science 358, eaam9756. doi:10.1126/science.aam9756
Vasan, K., Werner, M., and Chandel, N. S. (2020). Mitochondrial Metabolism as a Target for Cancer Therapy. Cell Metab. 32, 341–352. doi:10.1016/j.cmet.2020.06.019
Wang, H., Feng, Z., Wang, Y., Zhou, R., Yang, Z., and Xu, B. (2016). Integrating Enzymatic Self-Assembly and Mitochondria Targeting for Selectively Killing Cancer Cells without Acquired Drug Resistance. J. Am. Chem. Soc. 138, 16046–16055. doi:10.1021/jacs.6b09783
Wang, H., Wu, H., Yi, Y., Xue, K.-F., Xu, J.-F., Wang, H., et al. (2021a). Self-Motivated Supramolecular Combination Chemotherapy for Overcoming Drug Resistance Based on Acid-Activated Competition of Host-Guest Interactions. CCS Chem. 3, 1413–1425. doi:10.31635/ccschem.021.202100964
Wang, H., Yan, Y.-Q., Yi, Y., Wei, Z.-Y., Chen, H., Xu, J.-F., et al. (2020a). Supramolecular Peptide Therapeutics: Host-Guest Interaction-Assisted Systemic Delivery of Anticancer Peptides. CCS Chem. 2, 739–748. doi:10.31635/ccschem.020.202000283
Wang, M., Zhang, Q., Jian, H., Liu, S., Li, J., Wang, A., et al. (2020b). Role of Thermolysin in Catalytic-Controlled Self-Assembly of Fmoc-Dipeptides. CCS Chem. 2, 317–328. doi:10.31635/ccschem.020.201900116
Wang, Y., Weng, J., Wen, X., Hu, Y., and Ye, D. (2021b). Recent Advances in Stimuli-Responsive In Situ Self-Assembly of Small Molecule Probes for In Vivo Imaging of Enzymatic Activity. Biomater. Sci. 9, 406–421. doi:10.1039/d0bm00895h
Ward, P. S., and Thompson, C. B. (2012). Metabolic Reprogramming: A Cancer Hallmark Even Warburg Did Not Anticipate. Cancer Cell 21, 297–308. doi:10.1016/j.ccr.2012.02.014
Wasilewski, M., Chojnacka, K., and Chacinska, A. (2017). Protein Trafficking at the Crossroads to Mitochondria. Biochim. Biophys. Acta (Bba) - Mol. Cell Res. 1864, 125–137. doi:10.1016/j.bbamcr.2016.10.019
Wei, G., Su, Z., Reynolds, N. P., Arosio, P., Hamley, I. W., Gazit, E., et al. (2017). Self-Assembled Peptide and Protein Amyloids: From Structure to Tailored Function in Nanotechnology. Chem. Soc. Rev. 46, 4661–4708. doi:10.1039/c6cs00542j
Weinberg, S. E., and Chandel, N. S. (2015). Targeting Mitochondria Metabolism for Cancer Therapy. Nat. Chem. Biol. 11, 9–15. doi:10.1038/nchembio.1712
Wu, H., Wang, H., Qi, F., Xia, T., Xia, Y., Xu, J.-F., et al. (2021). An Activatable Host-Guest Conjugate as a Nanocarrier for Effective Drug Release through Self-Inclusion. ACS Appl. Mater. Inter. 13, 33962–33968. doi:10.1021/acsami.1c09823
Yang, J., An, H.-W., and Wang, H. (2020a). Self-Assembled Peptide Drug Delivery Systems. ACS Appl. Bio Mater. 4, 24–46. doi:10.1021/acsabm.0c00707
Yang, L., Peltier, R., Zhang, M., Song, D., Huang, H., Chen, G., et al. (2020b). Desuccinylation-Triggered Peptide Self-Assembly: Live Cell Imaging of SIRT5 Activity and Mitochondrial Activity Modulation. J. Am. Chem. Soc. 142, 18150–18159. doi:10.1021/jacs.0c08463
Yang, P.-P., Li, Y.-J., Cao, Y., Zhang, L., Wang, J.-Q., Lai, Z., et al. (2021). Rapid Discovery of Self-Assembling Peptides with One-Bead One-Compound Peptide Library. Nat. Commun. 12, 4494. doi:10.1038/s41467-021-24597-5
Yi, Y., Kim, H. J., Zheng, M., Mi, P., Naito, M., Kim, B. S., et al. (2019). Glucose-Linked Sub-50-nm Unimer Polyion Complex-Assembled Gold Nanoparticles for Targeted siRNA Delivery to Glucose Transporter 1-Overexpressing Breast Cancer Stem-Like Cells. J. Control. Release 295, 268–277. doi:10.1016/j.jconrel.2019.01.006
Zhang, D., Qi, G.-B., Zhao, Y.-X., Qiao, S.-L., Yang, C., and Wang, H. (2015). In Situ Formation of Nanofibers from Purpurin18-Peptide Conjugates and the Assembly Induced Retention Effect in Tumor Sites. Adv. Mater. 27, 6125–6130. doi:10.1002/adma.201502598
Zhang, S. (2003). Fabrication of Novel Biomaterials through Molecular Self-Assembly. Nat. Biotechnol. 21, 1171–1178. doi:10.1038/nbt874
Zhang, S. (2020). Self-Assembling Peptides: From a Discovery in a Yeast Protein to Diverse Uses and Beyond. Protein Sci. 29, 2281–2303. doi:10.1002/pro.3951
Zhang, X.-H., Cheng, D.-B., Ji, L., An, H.-W., Wang, D., Yang, Z.-X., et al. (2020). Photothermal-Promoted Morphology Transformation In Vivo Monitored by Photoacoustic Imaging. Nano Lett. 20, 1286–1295. doi:10.1021/acs.nanolett.9b04752
Zhang, Z., Chai, Y., Zhao, H., Yang, S., Liu, W., Yang, Z., et al. (2021). Crosstalk between PC12 Cells and Endothelial Cells in an Artificial Neurovascular Niche Constructed by a Dual-Functionalized Self-Assembling Peptide Nanofiber Hydrogel. Nano Res. doi:10.1007/s12274-021-3684-5
Zhao, J., Li, Z., Shao, Y., Hu, W., and Li, L. (2021). Spatially Selective Imaging of Mitochondrial microRNAs via Optically Programmable Strand Displacement Reactions. Angew. Chem. Int. Ed. 60, 17937–17941. doi:10.1002/anie.202105696
Zhao, K., Zhao, G.-M., Wu, D., Soong, Y., Birk, A. V., Schiller, P. W., et al. (2004). Cell-Permeable Peptide Antioxidants Targeted to Inner Mitochondrial Membrane Inhibit Mitochondrial Swelling, Oxidative Cell Death, and Reperfusion Injury. J. Biol. Chem. 279, 34682–34690. doi:10.1074/jbc.m402999200
Zhao, L., Li, S., Liu, Y., Xing, R., and Yan, X. (2019). Kinetically Controlled Self-Assembly of Phthalocyanine-Peptide Conjugate Nanofibrils Enabling Superlarge Redshifted Absorption. CCS Chem. 1, 173–180. doi:10.31635/ccschem.019.20180017
Zheng, Y., Mao, K., Chen, S., and Zhu, H. (2021). Chirality Effects in Peptide Assembly Structures. Front. Bioeng. Biotechnol. 9, 703004. doi:10.3389/fbioe.2021.703004
Zhou, J., and Xu, B. (2015). Enzyme-Instructed Self-Assembly: A Multistep Process for Potential Cancer Therapy. Bioconjug. Chem. 26, 987–999. doi:10.1021/acs.bioconjchem.5b00196
Keywords: mitochondrion, self-assembly, peptide, enzyme, nanomaterials, cancer therapy
Citation: Luo Z, Gao Y, Duan Z, Yi Y and Wang H (2021) Mitochondria-Targeted Self-Assembly of Peptide-Based Nanomaterials. Front. Bioeng. Biotechnol. 9:782234. doi: 10.3389/fbioe.2021.782234
Received: 24 September 2021; Accepted: 01 November 2021;
Published: 26 November 2021.
Edited by:
Bing Xia, Nanjing Forestry University, ChinaReviewed by:
Parikshit Moitra, University of Maryland, Baltimore, United StatesXuemei Ge, Nanjing Forestry University, China
Copyright © 2021 Luo, Gao, Duan, Yi and Wang. This is an open-access article distributed under the terms of the Creative Commons Attribution License (CC BY). The use, distribution or reproduction in other forums is permitted, provided the original author(s) and the copyright owner(s) are credited and that the original publication in this journal is cited, in accordance with accepted academic practice. No use, distribution or reproduction is permitted which does not comply with these terms.
*Correspondence: Zhongyu Duan, enlkdWFuQGhlYnV0LmVkdS5jbg==; Yu Yi, eWl5dUBuYW5vY3RyLmNu; Hao Wang, d2FuZ2hhb0BuYW5vY3RyLmNu
†These authors have contributed equally to this work