- 1Université de Sherbrooke Water Research Group, Department of Civil and Building Engineering, Université de Sherbrooke, Sherbrooke, QC, Canada
- 2Laboratory of Bioengineering, Earth and Life Institute, Catholic University of Louvain, Louvain-la-Neuve, Belgium
Τhe ligninolytic enzyme laccase has proved its potential for environmental applications. However, there is no documented industrial application of free laccase due to low stability, poor reusability, and high costs. Immobilization has been considered as a powerful technique to enhance laccase’s industrial potential. In this technology, appropriate support selection for laccase immobilization is a crucial step since the support could broadly affect the properties of the resulting catalyst system. Through the last decades, a large variety of inorganic, organic, and composite materials have been used in laccase immobilization. Among them, carbon-based materials have been explored as a support candidate for immobilization, due to their properties such as high porosity, high surface area, the existence of functional groups, and their highly aromatic structure. Carbon-based materials have also been used in culture media as supports, sources of nutrients, and inducers, for laccase production. This study aims to review the recent trends in laccase production, immobilization techniques, and essential support properties for enzyme immobilization. More specifically, this review analyzes and presents the significant benefits of carbon-based materials for their key role in laccase production and immobilization.
Introduction
Water is one of the fundamental resources on which all life on earth is anchored. Over the past few decades, concerns regarding the shortage in freshwater supply and its effect on the sustainability of human societies have increased (Rathi et al., 2021). Rapid population growth, industrialization, climate change, and environmental destruction are factors directly involved in increasing water demand (Jéquier and Constant, 2010; Rathi et al., 2021). Water recycling and reuse through proper treatment is a potential solution to meet the current and rising water demand. In this process, polluted water from different sources including households, industries, hospitals and agriculture may be treated to an acceptable standard and recovered for further use (Englande et al., 2015). However, non-regulated micropollutants termed emerging contaminants (ECs) such as pharmaceuticals and personal care products, certain pesticides, food additives and synthetic hormones constitute a major challenge to existing water treatment methods (Taheran et al., 2018).
ECs is a standard term created to identify environmental risks of pollutants released into the environment with unpredictable consequences (Rathi et al., 2021). According to the United Nations Educational, Scientific and Cultural Organization (UNESCO), the term ECs refers to a group of natural or synthetic chemicals or microorganisms with known or suspected negative effect on humans’ health or the environment (UNESCO, 2019). The word “emerging” does not imply the pollutants that are recently accumulated in the environment; in contrast, this term defines the concern and awareness regarding their negative impacts that are emerging in the world (Scaria et al., 2021). The best-known and widely occurring ECs are hormones such as contraceptives, personal care products such as fragrances and deodorants, pesticides such as insect repellents, and pharmaceutical compounds such as painkillers. At hospital wastewaters, landfills, municipal sewage, fertilizer industries, pharmaceutical production plants, concentrations of ECs could be detected (Ahmed et al., 2017). Up to now, there is no regulation regarding ECs concentration in the environment but several attempts can be found in Europe and North America to reduce their released levels (Taheran et al., 2018). For instance, in Canada and Switzerland, different projects have proposed potential strategies to reduce EC concentration in wastewater treatment plants (WWTPs) (Morales-Caselles et al., 2016; Schmidt, 2018).
Usually, EC concentrations in the environment range from parts per trillion (ppt or ng L−1) to parts per billion (ppb or µg L−1) (Petrie et al., 2015; Rout et al., 2021). Figure 1 demonstrates routes of EC spread into the environment (Gomes et al., 2020).
Conventional WWTPs are not capable of properly removing all ECs especially pesticides, detergents, pharmaceuticals and personal care products (PPCPs) at ng L−1 or µg L−1 from the wastewater and, consequently, ECs will get discharged into the environment (Mohapatra and Kirpalani, 2019). These pollutants could last for a long period of time and circulate, migrate, and transform in the different environmental matrices (Tran et al., 2019). Previous studies have demonstrated that the ECs might be found in conventionally treated wastewater, urban sewage, agricultural runoff, freshwater, and drinking water (Husk et al., 2019; Tran et al., 2019).
The existence of ECs in the environment is a global concern since in the long run their presence could have adverse effects on living organisms (Gomes et al., 2020). These could include bacterial resistance, feminization of aquatic organisms, neurotoxicity, endocrine disruption, and cancer along with other unidentified adverse effects (Mohapatra and Kirpalani, 2019). Several studies have explained the possibility of animal behavior alteration due to exposure to ECs. For instance, Barry (2014) found that tadpoles (Bufo arabicus) became more vulnerable to predation after exposure to fluoxetine (concentration around 3 µg L−1). In Denmark, from 1993 to 2006 a study demonstrated that exposure of patients 56–61 years old to Perfluorooctanoic acid (PFOA) and Perfluorooctane sulfonate (PFOS) could lead to cancer development (Lei et al., 2015).
Even though the concentration of ECs in the environment is relatively low, they still could affect negatively the food chain. Consequently, it is important to understand how to eliminate them from water and wastewater. EC removal methods may be categorized into four different groups, namely physical (such as sedimentation, precipitation, adsorption, and filtration), chemical (such as ozonation, photolysis, and Fenton), biological (such as activated sludge, aerobic microbial treatment, and enzymatic treatment), and hybrid systems (Ahmed et al., 2017; Taheran et al., 2018). Table 1 summarizes the limitations and advantages of each procedure. Among these four categories, biological treatment can be identified as an eco-friendly and cost-effective methodology. In this approach, large molecules could be degraded into smaller ones using different microorganisms such as bacteria, fungi, and algae (Unuofin et al., 2019).
Among microorganisms that potentially can be implemented in biological treatment, fungal systems have been mostly studied due to their significant ability to degrade ECs (Viancelli et al., 2020). Another advantage of fungal treatment is the flexibility in carbon or energy sources due to the fact that EC removal is essentially the result of the secondary metabolic action of fungi (Harms et al., 2011; Touahar et al., 2014).
Among different types of fungi utilized in ECs removal, white-rot fungi (WRF) and their oxidative enzymes have been mostly reported. Due to being non-specific, ligninolytic enzymes including laccase (Lac; EC 1.10.3.2), manganese peroxidase (MnP; EC 1.11.1.13), versatile peroxidase (VP; EC 1.11.1.16), and lignin peroxidase (LiP; EC 1.11.1.14) secreted by WRF have shown great ability to transform numerous compounds through an oxidation process (Bilal et al., 2019a). Even though each of these enzymes has its specific realm of catalytic action, the principal outcome of the reaction is to produce free radicals and ions in the medium and degrade chemical compounds such as dyes, pharmaceuticals and pesticides (Zdarta et al., 2018a). Among these enzymes, laccase has shown a significant capability of chemical compound transformation and has become a strong potential candidate in wastewater treatment applications (Unuofin et al., 2019).
Laccases are identified as a group of multicopper oxidases that are widely distributed in plants, bacteria and fungi (Senthivelan et al., 2016). Natural lignin degradation ability is the key feature of laccases; however, thanks to its low-substrate specificity, this enzyme could be implemented in different industries such as biofuel production, bioremediation, pulp and paper, food processing, biosensors, and dye decolorization (Mate and Alcalde, 2017; Antecka et al., 2021). A major application of this enzyme is in the bioremediation area as laccase could oxidize different pollutants such as phenolics, non-phenolics, aromatics, non-aromatics, and carbohydrates (Antecka et al., 2021). Through oxidation, laccase transforms contaminants into smaller components or into high molecular weight oligomers with the concomitant reduction of oxygen molecules into water (Arregui et al., 2019). Figure 2 presents the different percentages of laccase application in different industries.
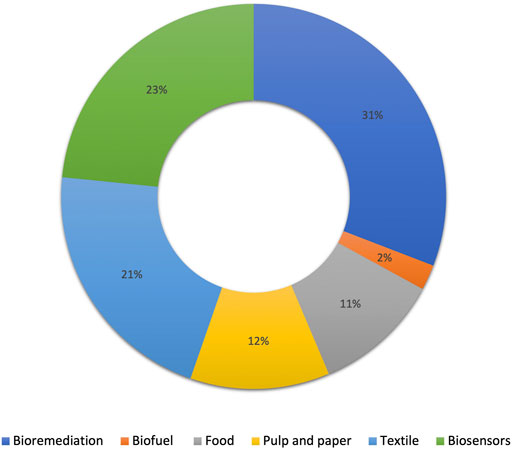
FIGURE 2. Percentage of laccase application in different industries (adapted from Mate and Alcalde 2017).
Although laccase’s ability to eliminate a wide range of contaminants has propelled this enzyme to become a potential candidate for wastewater treatment applications, there are some obstacles regarding its industrial usage, including high production costs, low stability of the enzyme, and its recovery (Hafid et al., 2021). All of these factors directly influence the economic sustainability of such processes. Large-scale production of laccase for industrial application requires a multistep process which can be expensive (Antecka et al., 2021; Hafid et al., 2021). In addition, laccases are generally secreted during fungal secondary metabolism and, unfortunately, the amount of produced laccase from its host is not generally considered sufficient for industrial applications (Antecka et al., 2021; Hafid et al., 2021). A common approach to minimize laccase production cost is to optimize fermentation (process) conditions and reduce the cost of the growth medium (Olivieri et al., 2006). Usually, laccase is produced by fungi grown in single-cell mode in liquid culture. However, through solid-state fermentation laccase could demonstrate higher productivity (Galhaup and Haltrich, 2001). For instance, Xu et al. (2020) reported the noteworthy enhancement in laccase activity secreted from Trametes versicolor cultured through solid-state fermentation on tea residue (Xu et al., 2020). Since laccase production efficiency is greatly dependent on growth medium composition (Antecka et al., 2021), the latter can also be optimized towards lower production cost. Myrothecium roridum laccase production was significantly increased when hay and rapeseed press cake extract were implemented as carbon sources (Jasińska et al., 2019).
Laccase structure could be distorted and deactivated through changing reaction conditions (Yavaşer and Karagözler, 2021). Moreover, there is no documented industrial application of free laccase (Zerva et al., 2019) due to low stability, poor reusability, and high costs. Laccase immobilization can be used to deal practically with its low stability and recovery. Laccase immobilization over solid supports could crucially increase stability and enable its reuse (Zhang et al., 2021) which, in turn, can contribute to cost reduction of the overall process. For instance, laccase immobilized over rice straw biochar showed increased stability (Imam et al., 2021): after six cycles of usage, immobilized laccase still maintained 47% of its initial activity. Overall, immobilization of laccase on solid supports can increase its stability and reusability along with boosting its activity. However, the efficiency depends upon the methods of immobilization employed. Moreover, the immobilized laccase properties such as immobilization yields, residual activity, subtrate specificity and kinetic parameters depend upon the immobilization methods and supports used (Patel et al., 2016; Patel et al., 2018; Patel et al., 2019).
Various solid supports have been used for immobilization of laccase including materials of various origin and chemical composition such as silica and inorganic materials (Girelli et al., 2020), chitosan (Bilal et al., 2019b), and metal oxides (Zdarta et al., 2018a). The extent of laccase immobilization on these solid supports depends upon their properties such as chemical composition, surface area and functional groups on the surface (Zdarta et al., 2018a). Among these divese supports, carbon based materials have been considered as an ideal candidate for enzyme immobilization (Zdarta et al., 2018a). Carbon-based materials such as activated carbons, graphene, and biochars have been employed efficiently for enzyme immobilization (Zhou et al., 2021). Due to well developed pore structures, high surface area (up to 1000 m2 g−1), existence of numerous functional groups on the surface, these materials are a valuable candidate for laccase immobilization (Zdarta et al., 2018a).
Among carbon-based materials, biochar, due to its properties, has attracted special attention (Madadi and Bester, 2021). Biochar is a solid carbonaceous material produced through hydrothermal and thermochemical methods (Madadi and Bester, 2021). Biochar is made up of numerous polyaromatic carbon units which enable this material to remove organic and inorganic pollutants from wastewater (Xiang et al., 2020). Further, biochar’s low cost and reasonable adsorption capacity make it a potential candidate for laccase immobilization (Madadi and Bester, 2021). Moreover, biochar has already proved its compatibility with a carbon negative, circular and sustainable economy (Glaser et al., 2009; Bolognesi et al., 2021).
In recent decades, a number of studies developed different immobilized laccase systems to eliminate ECs from wastewater systems. This review is focusing on carbonaceous materials and their role as a growth support for WRF as well as a solid support for laccase immobilization. Moreover, this review highlights the properties of various carbonaceous materials, recent trends in laccase production, and various strategies/mechanisms used for laccase immobilization. It also analyzes and presents the significant benefits of carbon-based materials for their key role in laccase production and immobilization. Furthermore, this review aims to eliminate current research gaps on the immobilization of laccase on carbonaceous materials and provide insights on future research directions in this domain.
Laccases
Lignin and Laccases
Lignin is an irregular branched three-dimensional polyphenolic biopolymer, which contributes to plant cell wall structural integrity and stability, resulting in the overall strength and rigidity of woody plants (Joffres et al., 2013; Figueiredo et al., 2018; Bugg et al., 2020). Its complex chemical structure consisting of three basic phenylpropanolic monomers (monolignols), i.e., coniferyl, p-coumaryl, and sinapyl alcohols makes lignin a highly resistant compound (Joffres et al., 2013; Bagewadi et al., 2017; Figueiredo et al., 2018). Besides, the presence of functional groups such as phenolic hydroxyl, benzylic hydroxyl and carbonyl moieties linked to the monolignols adds to this macromolecule’s heterogeneity and complexity (Bagewadi et al., 2017).
Laccases are one of the best characterized classes of extracellular lignin modifying enzymes (LME) (Zerva et al., 2017; Elisashvili et al., 2018). Owing to their capacity to depolymerize/degrade lignin, laccases attract biotechnological interest as one of the promising “green” tools for phenolic and non-phenolic compounds transformation and environmental bioremediation (Kameshwar and Qin, 2017; Zerva et al., 2017). Laccases are naturally expressed in bacteria, plants, or fungi (Kameshwar and Qin, 2017). WRF species, which play a major role in the wood decay process, are under considerable scrutiny in research for LME production (Ergun and Urek, 2017; Elisashvili et al., 2018).
Enzyme production is an important field in biotechnology. Given the promising biotechnological and industrial applications of laccases, continuous efforts have been deployed for the optimization of their production, aiming at their catalytic property enhancement and minimizing production costs. Bioengineering of new producing fungal species, optimization of the production methods and cultivation media, or bioprocess technologies are the avenues usually exploited (Pollegioni et al., 2015; Elisashvili et al., 2017; Kumar and Chandra, 2020).
Fermentation Strategies for the Production of Laccases
Typically, submerged (SmF) and solid-state fermentations (SSF) of lignocellulosic materials by WRF are used for laccase production (Elisashvili et al., 2018). SSF involves the growth of microorganisms on solid natural (e.g., organic substrates) or synthetic inert materials in the absence or near absence of free liquid medium (Palma et al., 2016; Ergun and Urek, 2017). This approach offers attractive features such as the use of cheap and underutilized agroforestry wastes as growth substrates to produce high value-added enzymes, high volumetric productivity, low energy and operational cost, low wastewater production, and low susceptibility to bacterial contamination (Karp et al., 2015; Soumya et al., 2016; Ergun and Urek, 2017; Ariste et al., 2020). SSF has been shown to be particularly fitting for filamentous fungi, since it provides adequate surface adherence and tends to mimic their natural habitat and growth conditions (Chenthamarakshan et al., 2017; Soccol et al., 2017).
Under SmF, microorganisms are grown in carbohydrate-based liquid media usually supplemented with nitrogen and other nutrients, under aerobic conditions. Unlike SSF, SmF allows easy monitoring of operating parameters such as pH, dissolved oxygen, or concentration of water-soluble substrates. In addition, this system is characterized by an easy mixing of the broth and separation of the biomass after fermentation. Due to its relatively easy scale-up, industrial production of enzymes is mainly performed under SmF (Wang et al., 2019). However, SmF can be limited by uncontrolled mycelial growth resulting in an overabundant biomass. Expansion of biomass can increase broth viscosity and limit mass and oxygen transfer, thereby reducing metabolic rate and enzyme secretion (Krull et al., 2013; Silvério et al., 2013).
Co-culture: An Effective Strategy for the Enhanced Production of Laccase
In recent years, microbial co-culture has developed rapidly as a promising alternative for the biosynthesis of various natural bioproducts of interest (Qian et al., 2020). This technique, which can be performed under SSF or SmF, brings together different species. It is therefore a convenient way to exploit the interactions of different species and stimulate individual strain cryptic genes and trigger the generation of new products. Yet, the exact biosynthetic mechanisms and pathways behind the overall process are complex and still await elucidation (Maglangit et al., 2020; Zhuang and Zhang, 2021). To be successful, biosynthesis of new products in co-culture requires appropriate conditions for the compatible coexistence of the different microbial species involved (Zhuang and Zhang, 2021). In terms of compatibility, different interactions have been highlighted between species in co-culture fermentations: one species develops at the expense of the others, the species inhibit each other (deadlock), or they collaborate (Wiberth et al., 2019).
Several recent studies on co-culture have proven its feasibility and viability as an experimental approach to enhance the chemical diversity of microorganisms. Co-culture of Pycnoporus sanguineus and Beauveria brongniartii strains under SSF by Jiménez-Barrera et al. (2018) yielded a six-fold increase in laccase activity. Also, a co-culture of Pycnoporus sanguineus and Trametes maxima and eight soil-borne micromycetes under SmF showed different competitive antagonism and collaboration interactions while, overall, ligninolytic enzymes including laccase showed increased activity (Wiberth et al., 2019). Laccase enzyme systems have been produced by co-cultures of Alcaligenes faecalis / P. sanguineus (Li et al., 2016) and T. maxima / Paecilomyces carneus (Chan-Cupul et al., 2016) under SmF; both yielded higher laccase activity compared to monocultures.
Factors Affecting Laccase Production Under SmF and SSF
Under solid-state or submerged fermentation, several factors can influence enzyme production. Successful production implies selection of appropriate fungi species, supports/substrates, growth media and conditions, and inducers (Soccol et al., 2017). In general, the key factors that regulate laccase production can be clustered into two broad sets. The first category includes the media composition (in particular the carbon and nitrogen sources and concentrations), the concentration of dissolved oxygen (DO) and the type and concentration of inducers (Kumar et al., 2016; Elisashvili et al., 2017; Schneider et al., 2020). Second, the operating parameters, which comprise pH, temperature, agitation, and incubation time can significantly affect fungal laccase production. As the effects of these factors combine, it is quite complex to establish a standardized model for the regulation of laccase synthesis (Chenthamarakshan et al., 2017; Elisashvili et al., 2018).
Importance of Carbon and Nitrogen Sources on Laccase Production
As a first note, different fungi may require different sources of carbon and nitrogen to fully release their laccase expression potential. Under submerged conditions, Hariharan and Nambisan (2012) tested many sources of carbon including glucose, sucrose, starch, maltose, and lactose. Their results suggested that glucose and sucrose enhanced the enzyme expression, but other carbon sources contributed to activity decrease. These results are consistent with those recently unveiled by other researchers, where glucose effectively promoted laccase activity (Schneider et al., 2019; Marin et al., 2020). Furthermore, Schneider et al. (2019) found that the secretion of laccase was related to the nitrogen source in the media, with casein being a better enzyme promoter than peptone. In the same sense, Lentinus strigosus 1566 showed highest laccase activity in a peptone-yeast extract medium supplemented with galactose, arabinose, and xylose, while glucose, sucrose, or maltose decreased its activity (Myasoedova et al., 2015). These authors also found that glucose slightly increased laccase activity compared to malt dextrin, whereas fructose decreased laccase production. As for sucrose and glycerol, they lowered laccase activity yield but substitution by maltose had no effects on laccase production. Overall, diverse carbon sources have a significant role in laccase production. Determining the best carbon source is the first step towards optimal growth medium design and eventually optimal laccase production.
Typically, culture media are supplemented with organic or inorganic nitrogen sources. Depending upon these two forms, different levels of laccase expression and activity can be observed with the same strain and from one strain to another. A direct positive correlation between peptone concentration and biomass development and laccase activity increase was observed in a culture of Coriolopsis gallica 142 strain (Mikiashvili et al., 2006; Elisashvili et al., 2017). However, at a certain threshold, the subsequent increase in peptone concentration led to an opposite effect on the activity. In the aforementioned study, nitrogen sources such as peptone, yeast extract, beef extract, ammonium sulphate, ammonium nitrate, and urea, were also tested for laccase production. The authors found that beef extract was the best nitrogen source for highest activity expression after 120 h of incubation (Hariharan and Nambisan, 2012). Previously, Zerva et al. (2017) studied the comparative influence of five different nitrogen sources including diammonium tartrate, potassium nitrate, ammonium nitrate, yeast extract and corn steep liquor (CSL) on laccase expression by Pleurotus citrinopileatus and Irpex lacteus. It was observed that both species developed highest biomass and laccase activities in samples supplemented with CSL. Besides, inorganic nitrogen sources were found to promote less fungal growth. In another study, Chauhan (2019) obtained a similar result with Grammothele fuligo cultured in glucose-based medium, where inorganic nitrogen sources tested failed to promote abundant biomass and further to secrete laccase. The fermentation of P. ostreatus Pl 22 strain using different nitrogen sources showed that yeast extract increased laccase activity by almost six-fold in comparison with ammonium sulfate (Karp et al., 2015). Mikiashvili et al. (2006) determined that ammonium sulfate and ammonium nitrate were good sources of nitrogen for laccase production by Trametes multicolor. Besides their individual effects, the Carbon/Nitrogen (C/N) ratio can significantly influence the synthesis and secretion of fungal laccase (Rivera-Hoyos et al., 2013; Elisashvili et al., 2018). Globally, depending upon the strains, low or high C/N ratio can alternately improve or decrease the production (Elisashvili et al., 2018). Interestingly, Yang et al. (2016) determined that the combination of high concentrations of carbon and nitrogen led to higher production of laccase from Cerrena sp.
In summary, a wide range of nitrogen sources has been studied and can induce diverse effects on laccase production, hence there is considerable uncertainty regarding the selection of the optimal nitrogen concentration for laccase production (Elisashvili et al., 2018).
Effect of Inducers on Laccase Production
Lignin degradation metabolites and metals naturally present in the environment can act as promoters of fungal laccase production. In a laboratory context, phenolic and aromatic compounds, especially those structurally related to lignin (Furukawa et al., 2014; Pollegioni et al., 2015; Elisashvili et al., 2017), and metals such as copper, manganese, cadmium, and magnesium can play an important role in laccase production (Valle et al., 2014; Martani et al., 2017; Lallawmsanga et al., 2019). However, these compounds have also been depicted to be playing dual roles as they can act as inducer or repressor, depending notably on their concentration, the media composition, the fungal species, and the enzyme tested (Elisashvili et al., 2017). Under submerged fermentation, hydroquinone was found to cause an increase in laccase production by T. versicolor, whereas C. unicolor rather decreased laccase activity (Elisashvili et al., 2010). Under laboratory conditions, compounds such as 2,5-xylidine, guaiacol, veratryl alcohol (VA) and catechol are often used as laccase inducers (Krull et al., 2013; Martani et al., 2017). In a submerged fermentation of T. multicolor 511, VA and guaiacol enhanced laccase specific activity by two-fold (Mikiashvili et al., 2006). Similarly, gallic acid (1 mM), tartaric acid (20 mM), and citric acid (20 mM) could elevate laccase activity (Chang and Chang, 2016). It was also observed that among several organic inducers, ethanol and guaiacol induced laccase production by Lentinus crinitus while pyrogallol, veratryl alcohol, xylidine, and vanillin were ineffective (Valle et al., 2014). It was also determined that the induction of laccase activity by ethanol was concentration-dependent, as concentrations of 1% v/v and 3% v/v have increased Ganoderma lucidum laccase activity production by 6.5 and 14 times compared to the control, repectively. However, with up to 5% v/v ethanol, the activity reached only 10 times that of the control, showing that the correlation of activity induction with ethanol concentration was positive up to a certain level, beyond which the ethanol concentration could be less effective in increasing laccase activity (Manavalan et al., 2013). Resveratrol, tannic acid, and guaiacol were found to be the best laccase inducers in a culture of C. gallica, however, 2–2′-azinobis (3-ethylbenzothiazoline-6-sulfonic acid) (ABTS) and gallic were ineffective (Xu et al., 2016) under the same fermentation conditions. On the contrary, laccase activity was increased in Cerrena sp. HYB07 fermentation by ABTS and guaiacol, though other aromatic compounds had no significant effects (Yang et al., 2016).
Several inorganics can modulate laccase expression. In general, trace metallic elements at high concentrations can be toxic to ligninolytic fungi growth and repress their laccase expression. Besides, it was demonstrated early on that tolerance to high concentrations of trace metallic elements can largely be species dependent (Giller et al., 1998; Valle et al., 2014). Meanwhile, some metallic compounds such as Cu, Mn, Co, and Zn, present at low concentrations in the culture medium are essential for fungal growth and biological functions (Baldrian, 2003; Asif et al., 2017). Among microelements, copper is largely used as an inducer in enzyme production. The positive correlation between laccase production and copper, often added to the media as copper sulfate, has been well described in previous studies (Valle et al., 2014; Karp et al., 2015; Chang and Chang, 2016; Vrsanska et al., 2016; Yang et al., 2016; Zhu et al., 2016; Schneider et al., 2019). Moreover, the influence of copper on laccase expression is likely to be magnified or minimized concomitantly with high or low nitrogen concentration, respectively (Valle et al., 2014). However, under certain conditions, the negative effect of copper has also been highlighted (Dao et al., 2019). Thus, as reported by Martani et al. (2017), the overall influence of copper on laccase production depends on its concentration in the culture medium, the microbial strains involved, and the presence of other components in the medium.
Effect of Fermentation Operating Parameters on Laccase Production
In addition to the design of the nutritional environment, operational factors such as temperature, pH, time, agitation rate, and dissolved oxygen can significantly influence the fungal growth and enzyme production.
Temperature does not correlate significantly with fungal growth rate and biomass development (Martani et al., 2017). However, it importantly influences the potential and the level of laccase activity expressed, as revealed by several studies. Schneider et al. (2019) showed that laccase activity of Marasmiellus palmivorus VE111 was maximum at 28°C and decreased when this temperature was either lowered or raised by 5°C. The decrease of laccase activity below or above 28°C was explained by the reduction of expression of some genes involved in the transcription of this enzyme (Rivera-Hoyos et al., 2013; Schneider et al., 2019). A previous study on M. palmivorus LA1 laccase secretion under SSF using pineapple leaf as substrate led to a similar conclusion (Chenthamarakshan et al., 2017). Hariharan and Nambisan (2012) found that 27°C was the best temperature for laccase production by Ganoderma lucidum under SSF, while temperatures lower than 23°C or higher than 33°C led to a significant reduction in enzyme production. Yet, Chang and Chang (2016) determined 30°C as the optimum temperature for laccase production from Pleurotus eryngii, under submerged conditions.
The pH can have an important influence on fungal growth and thereby on laccase expression. According to previous studies, highly acidic or basic media negatively affect fungal growth and laccase activity, and this can be noticed either under SSF or SmF. Chang and Chang (2016) noted an increase of laccase activity of P. eryngii between pH 2 and 5, before its decrease in the 5–9 pH-range. In another study, Chenthamarakshan et al. (2017) determined that pH 5 was the optimum for best growth of M. palmivorus LA1 on pineapple leaf for laccase secretion and maximum activity. In the same vein, pH 5 was determined as optimal for production of laccase from G. lucidum under SSF, after an optimization process (Hariharan and Nambisan, 2012) while Zerva et al. (2017) got the best results at pH 5 and 6 with Pleurotus citrinopileatus and Irpex lacteus strains using supplemented olive mill wastewater as culture medium. For Schneider et al. (2019), pH 4 and below or pH 8 and above led to laccase activity decrease, whereas it reached maximum activity at pH 7.
Incubation time for an enzyme to reach maximum activity expression varies from one strain to another and according to fermentation conditions. In general, microorganisms are characterized by a period of acclimation followed by growth and biomass production accompanying the substrate consumption. Overall, thanks to the ready availability of nutrients, the culture period for enzyme production in SmF is generally shorter than that of SSF (Wang et al., 2019). The Ganoderma lucidum 447 culture for enzyme production in olive mill by-products medium achieved highest laccase activity after 6 days, i.e. earlier than with other fungi tested in the same study. In contrast, Cerrena unicolor 302 attained maximum laccase activity after 2 weeks of fermentation (Elisashvili et al., 2017). A 2-week period was also the cultivation time necessary for Ganoderma applanatum with rice bran as media to achieve maximal laccase activity (Wang et al., 2019). The culture of Coriolus versicolor on sweet sorghum bagasse in SSF supplemented with CuSO4, gallic acid and syringic acid produced maximum laccase activity within 16 days (Mishra et al., 2017). Under SmF, P. citrinopileatus and I. lacteus produced highest laccase activity in 10 and 24 days of cultivation in olive mil wastewater, respectively (Zerva et al., 2017), however Cerrena consors took much more time (30 days) for the laccase activity peak in a 50% olive mill wastewater (Mann et al., 2015).
Under submerged fermentation conditions, the availability and transfer of oxygen is essential for fungal growth. As mentioned earlier, mycelial uncontrolled expansion can limit oxygen transfer (Krull et al., 2013; Silvério et al., 2013). To promote oxygen transfer, it is important that the culture must remain continuously under shaking conditions. This was corroborated by Domingos et al. (2017) who found that unshaken culture resulted in incomplete sugar consumption partially due to lack of proper oxygen transfer. In another study, Schneider et al. (2019) have analyzed the influence of the concentration of dissolved oxygen on enzymatic activity from Marasmiellus palmivorus VE111 strain. Thus, in general, it is proved that increased laccase activity is directly related to DO concentration.
The monitoring of agitation has shown a positive correlation between biomass growth and agitation rate. However, above a certain threshold, agitation can lead to a negative effect on biomass growth and enzyme expression. In fact, under excessive agitation, hydrodynamic shear stress on biomass can result in changes in its morphology, leading to subsequent enzyme under-expression (Zerva et al., 2017).
Sustainable and Cost-Effective Growth Media for Enhanced Production of Laccases
Recently, several fungal strains have been screened for their potential growth under SmF conditions for laccase production, using various natural carbonaceous substrates such as agro-residues. For instance, Elisashvili et al. (2018) used mandarin peels (MDP), olive tree sawdust (OTS), olive pomace (OP), and olive mill wastewater (OMW) as growth substrates under SmF and SSF conditions. They have tested seven strains belonging to C. unicolor, Fomes fomentarius, Ganoderma lucidum, P. ostreatus, P. coccineus, T. trogii, and T. versicolor species. The culture media were initially supplemented with 0.3% peptone as additional nitrogen source and 1 mM CuSO4 as laccase inducer. Overall, C. unicolor and T. trogii 146 strains showed the highest laccase activity. MDP were good substrates for laccase secretion by the C. unicolor strains, and OTS promoted best secretion of laccase by C. unicolor 302, whereas OP appeared to be ideal for laccase production by C. unicolor strains and T. versicolor (Elisashvili et al., 2018). Cultures with OMW favored enhanced production of laccase by G. lucidum 447, P. ostreatus 2175, and P. coccineus 310. Overall, highest laccase activity was obtained from C. unicolor 301 and T. trogii 146 with OMW-based medium. In a similar study, Zhao S.-X. et al. (2017) grew P. ostreatus under SmF conditions using tea, peanut shells, orange peel, corn cob, and bagasse as substrates in glucose-based medium. Laccase production was enhanced in all the cultures except in those using peanut shells as substrates. The cultures with orange peel showed the highest laccase activity which was nine times higher than the control.
Immobilization of Laccase
Free laccase can have high activity. However, due to not being able to separate and be reused, activity can be lost in a continuous process thus increasing the operational cost (Masjoudi et al., 2021). In addition, it has been proved that free laccase may exhibit poor stability while exposed to harsh operating conditions and over time (Wen et al., 2019). In order to tackle these challenges, the immobilization strategy is considered the most successful method. Attachment of laccase over solid supports could significantly enhance its capability to maintain its activity over time and its resistance to operational conditions (e.g. temperature, pH, and exposure to different chemical agents) (Shakerian et al., 2020). Moreover, reusability of immobilized laccase can crucially decrease operational cost in continuous systems (Naghdi et al., 2017). However, immobilization could result in laccase conformational change, and a decrease in activity (Ji et al., 2017). For an efficient immobilization, mode of immobilization, support material, and initial activity of laccase are critical parameters to be considered (Yavaşer and Karagözler, 2021). Figure 3 shows the important factors regarding biocatalyst preparation.
Modes of Immobilization
Immobilization procedures are categorized into two groups including physical and chemical interactions (Fernández-Fernández et al., 2013). The difference between chemical and physical immobilization procedure refers to how the enzyme attaches onto/into the support (Zdarta et al., 2018a). During physical immobilization, there is no or minimal enzyme conformation change, and the enzyme could keep its activity (Zhou et al., 2021). In this methodology, there are no strong interactions between enzyme and carrier and the two can be connected through weak intermolecular forces such as hydrogen bonds, ionic, and hydrophobic interactions (Ba et al., 2013; Zdarta et al., 2018b). Entrapment and adsorption stand out as the main physical procedures (Zhou et al., 2021).
In contrast to physical attachment, chemical interactions are involved through the creation of covalent bonds between enzyme and solid support (Daronch et al., 2020). Chemical immobilization is based on the interaction between functional groups of the solid support and enzyme functional groups (mostly –NH2, –SH, and –OH). Covalent binding and cross-linking can be considered as two methodologies in this category.
Since physical bonding is relatively weak, it will maintain the enzyme bound to the support for a shorter period of time (Datta et al., 2013). In addition, changes in operational conditions (e.g. ionic strength, pH, and temperature) could result in loss of enzyme activity. As a result, preference is given to chemical immobilization (Wahab et al., 2020) for industrial applications such as wastewater treatment. Generally, it is expected that chemical immobilization reduces enzyme leakage and significantly improves its reusability (Zdarta et al., 2018b). Figure 4 illustrates different immobilization techniques.
Entrapment
Entrapment is identified as the simplest immobilization technique in which enzyme molecules disperse into a porous solid matrix; hence no direct attachment may be formed between carrier and enzyme (Fernández-Fernández et al., 2013; Karthik et al., 2021). Alginate, collagen, silicon rubber, gelatin, carrageenan, polyurethane, polyacrylamide, and polyvinyl alcohol with styryl pyridinium groups are solid matrices that can be used for enzyme entrapment (Dayaram and Dasgupta, 2008; Phetsom et al., 2009; Fernández-Fernández et al., 2013). Enzyme entrapment can be carried out in two steps: first enzyme molecules are dispersed into monomer solution, and then a polymerization process ensues which maintains enzyme molecules trapped (Karthik et al., 2021). Entrapment technology could increase laccase stability considerably and it can be helpful to avoid enzyme denaturation. Despite its benefits, this method has some limitations which restrict its application. One such issue is enzyme leakage which can be significant when a support with a large pore size is used.
Adsorption
In the adsorption immobilization technique, the enzyme is linked to the carrier through weak interactions (Sirisha et al., 2016). Based on the types of weak forces, adsorption immobilization can be divided into two categories, namely ionic attachment (electrostatic interaction is dominant) and physical attachment (mainly through van der Waals forces, hydrophobic interactions or hydrogen bond formation) (Karthik et al., 2021; Zhou et al., 2021). Compared with other techniques, adsorption methodology is recognized as a simple and low-cost procedure for enzyme immobilization (Fernández-Fernández et al., 2013). Despite its benefits, the amount of enzyme leakage in this method is high, therefore the application of adsorption immobilization for long-term processes or processes with varying operational conditions is not recommended (Zhou et al., 2021). pH, ionic strength of the solution and solid support surface area are three factors that should be considered during adsorption immobilization (Rekuć et al., 2008; Huajun et al., 2009; Xu et al., 2009; Forde et al., 2010).
Covalent Binding
Covalent binding is considered as the most reliable method for industrial application (Fernández-Fernández et al., 2013). In this methodology, strong bonds are formed between non-essential amino acids at the surface of enzymes and carrier chemical groups. Due to the formation of these strong bonds between supports and enzymes, the amount of leakage decreases significantly (Hernandez and Fernandez-Lafuente, 2011; Zdarta et al., 2018b). Based on the functional groups on the supports, various reagents could be implemented to prepare the support for covalent immobilization. For supports with hydroxyl groups, cyanogen bromide (CNBr) and carbonyl diimidazole (CDI) are recommended (Karthik et al., 2021). For supports with carboxyl groups, zero length reagents such as EDC (1-ethyl-3-(3-dimethylaminopropyl) carbodiimide hydrochloride), NHS (N-hydroxysulfosuccinimide), and EDC coupling with Sulfo-NHS are recommended (Hermanson, 2013). In addition to these reagents, ionic liquids have been frequently used in enzyme immobilization as they are eco-friendly solvent media (Hermanson, 2013). However, selection of ionic liquid types is a key step since cation or anion changes in such a liquid could affect activity, structure and enzyme stability (Hermanson, 2013). The possibility of laccase immobilization on magnetic nanoparticles was also investigated (Qiu et al., 2020). In this study, the surface of magnetic nanoparticles was modified with an amino-functionalized ionic liquid. Through surface modification with 3-(chloropropyl) trimethoxysilane (CPTMO) and (3-aminopropyl) trimethoxysilane (APTES), laccase was covalently immobilized on the surface (Qiu et al., 2020). Stability-wise, the biocatalyst could maintain around 70% of its initial activity after six cycles (Qiu et al., 2020). In the context of magnetic supports, bioinspired magnetic particles bearing laccase (laccase-biotitania, lac-bioTiO2) were applied for the efficient removal of bisphenol A, 17α-ethinylestradiol and diclofenac in a mixture of six model endocrine disrupting compounds (EDCs) and retained 90% of activity after five reaction cycles and 60% after 10 cycles (Ardao et al., 2015).
Cross-Linking of Enzyme Aggregates
Cross-linking of enzyme aggregates is a carrier-free insolubilization procedure in which multifunctional or bifunctional reagents are implemented to assist enzyme cross-linking into a unified structure with no added carriers (Mateo et al., 2004; Zhou et al., 2021). Since, in this methodology, enzymes act as their own solid supports, this procedure is also called a self-immobilization technique (Karthik et al., 2021). Among different cross-linker reagents such as diiminoesters, diisocyanates, and diamines activated by carbodiimide, the best-known is glutaraldehyde (GA) as it is inexpensive, widely available, and easy to manipulate (Fernández-Fernández et al., 2013; Xiang et al., 2018). However, currently this cross-linker is raising potential toxicity concerns (Yang et al., 2019). This method is highly dependent on pH which includes Schiff’s base formation and Michael-type 1,4 in addition to α, β-unsaturated aldehyde moieties (Migneault et al., 2004). There are two kinds of enzyme cross-linking techniques, namely formation of cross-linking enzyme crystals (CLEC), and of cross-linking aggregates (CLEA) (Ba et al., 2013). In CLEA (Schoevaart et al. 2004), first enzyme molecules are clustered in chemical precipitant solutions such as acetone, ammonium sulfate or ethanol and subsequently a cross-linking reaction completes the process, as initially demonstrated with laccase CLEA by Cabana et al. (2007) and then by others (Matijošyte et al., 2010; Nguyen et al., 2017). CLEC techniques demonstrate good stability and promising activity, however for this process high purity of enzyme is required (Sirisha et al., 2016). Finally, cross-linking with the concomitant enzyme immobilization on an inert porous support may confer additional stability. For istance, Nair et al. (2013) described the deactivation of free and immobilized enzymes during their incubation at 45, 55, 65 and 75°C at pH 5 in absence of electron-donor substrate by periodically measuring the residual activity with ABTS as a substrate. An apparent higher stability of immobilized laccase was evidenced with greater half-lives for the immobilized laccase than soluble laccase. Table 2 presents indicative properties of enzyme immobilization techniques applicable to laccases.
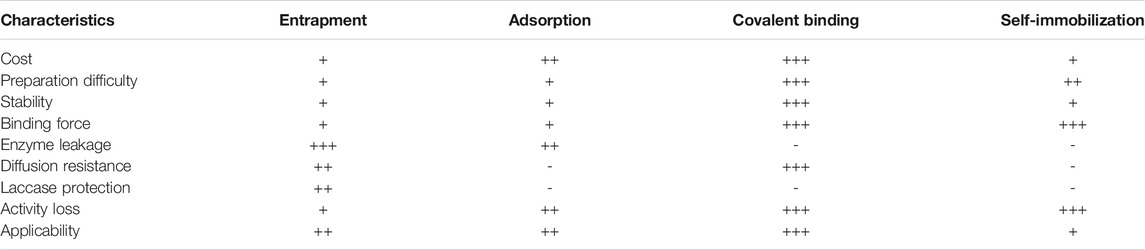
TABLE 2. Inherent characteristics of immobilization methods (Zhou et al., 2021).
Immobilization Carriers/Solid Supports and Their Properties on Laccase Immobilization
The selection of appropriate solid support for laccase immobilization is crucial for biocatalyst efficiency (Daronch et al., 2020). Generally, carriers are sought to enhance laccase catalytic activity and stability (Zhou et al., 2021). An ideal support should protect both enzyme structure and activity under a variety of operational conditions (Zdarta et al., 2018a) while keeping its own physical integrity. Here below, important characteristics of a solid support are discussed (Figure 5).
Particle Size
Solid support particle size plays a significant role in the success of immobilization. In industrial applications, large particles may be handled better than small ones (Santos et al., 2015). Nanoporous gold supports were employed to study the effect of particle size on laccase immobilization (Huajun et al., 2009). The results obtained from three different particle size samples demonstrated that the larger particle size support had the ability to keep more enzyme on its surface due to laccase accessibility to inner pore structures (Huajun et al., 2009). However, having larger support particles could have some drawbacks as well. Large particles could enhance diffusional limitations which could, in turn, affect negatively the enzyme activity (Bortone et al., 2014). In the case of the substrate, if its consumption rate by the enzyme is higher than its diffusion rate, there is a possibility of the enzyme located at the support core not receiving any substrate and therefore the biocatalyst’s apparent enzyme activity could decrease (Lortie and André, 1991; Boniello et al., 2010). At the same time, even though nanoparticles present handling issues, the diffusion problems can be prevented by the use of nanoparticles instead of microparticles and for non-porous supports the enzyme is always exposed to the substrate (Bilal and Iqbal, 2019; Bilal et al., 2020). Moreover, to produce effective multipoint covalent immobilization on nanoparticles, epoxy, glyoxyl or divinylsulfone activated nanoparticles can be used (Bilal and Iqbal, 2019; Bilal et al., 2020).
Pore Size/Specific Area
There is a connection between pore size and surface area in which larger pores result in a lower specific area. Specific surface area determines the amount of enzyme that could be loaded over the carrier (Di Cosimo et al., 2013). From an economics perspective, a larger specific surface area could result in a higher amount of enzyme that could be loaded over the support (Santos et al., 2015). Pore diameter determines the size of the enzyme which could be immobilized over the solid support. Importantly, the size of the pore should be big enough to allow the new enzyme molecules to enter in the support (Hudson et al., 2008). In general, the diameter of the pore should be four to five fold larger than the enzyme’s molecule size (Hanefeld et al., 2009). According to a comprehensive analysis of 182 experiments with emphasis on the effect of pore size and surface area on enzyme immobilization, a general trend emerged: higher surface area would result in higher enzyme load on the support (Bayne et al., 2013). However, this general trend for pore size was divided into three ranges in which for the supports with pore size less than 10 nm, the amount of loading is less (apparently due to physical restrictions in accessing the augmenting surface inherent in this pore diameter range), for the supports with pore size between 10 and 100 nm, the amount of enzyme loading tends to be constant (possibly due to protein–protein interaction blocking pores and restricting access to the higher surface area available at lower pore diameters), and for supports with pore size higher than 100 nm, the amount of enzyme loading per unit mass would decline due to a parallel reduction in available surface area (Bayne et al., 2013). Thus, upon a critical analysis even if the surface area is larger for solid supports with small pores the possibility of enzyme loading is lower. Moreover, there was no clear trend between pore characteristics and retention of catalytic activity (Bayne et al., 2013).
Functional Groups
The existence of functional groups on the solid supports is another factor that controls enzyme-support interactions (Santos et al., 2015). Favorable functional groups on the solid support are essential to ensure that strong multiple interactions would occur between enzyme, binding agent, and support leading to decreased leakage (Pandey et al., 2020). While the density of active groups on the solid support is crucial, the nature of functional groups is also critical. Most active groups are stable and do not require further consideration (Garcia-Galan et al., 2011). However, covalent immobilization merits further analysis (Garcia-Galan et al., 2011). An ideal functional group for successful covalent immobilization should have the following properties:
- Allow reaction between enzyme and support with low steric hindrances (Mateo et al., 2005);
-Maintain the physical properties of the enzyme after immobilization (Bolivar et al., 2009);
- Be stable over a wide range of conditions (Pedroche et al., 2007);
- Require a simple immobilization protocol with no additional treatment (Santos et al., 2015).
Inertness and Mechanical Properties
Support inertness could affect both immobilization and the substrate on which immobilized laccase is expected to act (Daronch et al., 2020). Commonly, a solid support should maintain its physical integrity and be inert after immobilization to avoid interfering with desired reactions (Ba et al., 2013). Polysaccharide matrices such as agarose and cellulose beads, carbonaceous materials, as well as silica compounds are considered as inert solid supports (Santos et al., 2015). Mechanical properties of solid supports are highly dependent on the process use intended for the immobilized laccase (Garcia-Galan et al., 2011). For instance, in a fixed-bed reactor, the solid support should have high rigidity to tolerate high pressure (Santos et al., 2015), hence silica materials, carbon-based materials, and inorganic oxides are recommended (Kim et al., 2008; Tartaj, 2011; Hartmann and Kostrov, 2013). However, the situation would be different in a stirred-tank reactor (Santos et al., 2015)where, instead of mineral materials, more flexible compounds such as agarose beads, cellulose beads, and lentikats can be used (Grazu et al., 2006; Cárdenas-Fernández et al., 2012; Lam et al., 2012).
Besides the above-mentioned properties, the ideal solid support should be low cost and eco-friendly (not increasing operation cost and generating environmental problems), with high affinity toward the enzyme to be amenable to regeneration (Ba et al., 2013; Daronch et al., 2020). Table 3 categorizes three major types of support materials used for immobilization and their specific properties.
Carbonaceous Materials in Laccase Production and as a Support for Their Immobilization
Perspectives of Carbon-Based Materials for Laccase Production As Inducers and Growth Medium
The prospect of using carbon-based materials is very interesting for laccase production. However, there are few reports in the literature on biochar utilization in laccase production, in contrast to more abundant trends towards biochar immobilization of enzymes produced conventionally. Another technique involves the concomitant production and immobilization of enzymes on solid supports in a single-step process. However, to the best of our knowledge, this has not been explored further and future studies can further explore the concerted production and immobilization of enzymes within the same process. Fortunately, due to the eclectic and rich composition of biochar and its overall physicochemical characteristics (see below), the use of this material can be considered a multi-in-one technique to enhance laccase production and immobilization.
Biochar as a Substrate for Production and Support for Immobilization
The study of biochar’s composition has revealed that, depending on the feedstocks and pyrolysis conditions, this material can present incompletely degraded lignocellulosic biomass and nitrogen-content residues such amine groups (see below). Furthermore, functionalization can introduce new chemical groups to the biochar structure. These elements make biochar a complementary source among the common carbonaceous nutrients provided in culture media for laccase production WRF. Besides, the large specific area and pore size, and the existence of specific chemical groups on biochar surface favor its adsorptive capacity, which can also be related to the molecular size of the enzyme (Rajapaksha et al., 2016; Li et al., 2018; Fernandez-Sanroman et al., 2020; Pandey et al., 2020). Several studies have reported the successful enhancement of laccase production and immobilization on biochar either by adsorption or covalent bonds (Lonappan et al., 2018a; Li et al., 2018; Fernandez-Sanroman et al., 2020; Pandey et al., 2020; Imam et al., 2021). A summary of such studies is shown in Table 4.
Biochar as an Inducer of Laccase Production
In a biochar-based medium for laccase production, laccase can adsorb onto biochar or some of its components can be released in the culture medium and absorbed by the fungus. In both cases, as discussed in other sections, these organic and inorganic components in the biochar exert regulatory actions on laccase production, either as promoting or inhibiting agents (Giller et al., 1998). Due to its physicochemical characteristics, i.e., its high porosity and hydrophobicity (Taskin et al., 2019b), biochar can demonstrate high affinity for organic and inorganic contaminants (Taskin et al., 2019b; Fernandez-Sanroman et al., 2020). This property allows its use as a sorbent of organic or inorganic pollutants for soil amendments (Taskin et al., 2019b). Biochar has also been used in wastewater as additive/support media during anaerobic digestion, filtration matrix for the removal of suspended matter, heavy metals, or pathogens (Madadi and Bester, 2021).
The presence in biochar of bioavailable organic components like hydrophilic compounds and thermally labile fractions (Rombolà et al., 2016), adsorbed volatile organic compounds (Spokas et al., 2011), and polycyclic aromatic hydrocarbons (Buss et al., 2015) is well established. Many inorganic compounds including essential elements for the improvement of fungal laccase production such as Cu, Mn, or Fe have also been found in the biochar structure (see below). On the other hand, some of these compounds are potentially toxic and can be detrimental to laccase production or immobilization (Singh et al., 2010; Zhang G. et al., 2018). In some cases, it all depends on biochar level in culture media (Taskin et al., 2019b). Ultimately, the use of biochar as a substrate for laccase production or immobilization remains an open question.
Regarding carbon-based stimulation of WRF enzyme production, Liu et al. (2019) investigated the impact of single-walled carbon nanotubes, graphene and oxidized graphene (graphene oxide, GO) on the extracellular LME activities of a Cladosporium sp. strain, using a SmF with basal medium made of peptone and yeast extracts. It was found that, among the three carbon-based materials tested, single-walled carbon nanotubes and graphene increased laccase production, while GO caused a slight decrease in laccase activity (Liu et al., 2019). The effects on laccase expression of two carbon-based materials, i.e., biochar (BC) and hydrochar (HC) prepared from four feedstocks were also studied using T. versicolor, P. ostreatus and P. eryngii strains (Taskin et al., 2019a). At two different doses (0.4 and 2% w/v), the two materials significantly stimulated laccase production and increased its activity for T. versicolor and P. eryngii strains, but P. ostreatus did not release any detectable laccase. Hence, BC from red spruce pellets at 0.4% w/v and HC from urban pruning residues at 2% w/v have promoted T. versicolor laccase activity by 6.4 and 21-fold with respect to the controls, respectively. Similarly, BC from vine pruning residues at 0.4% w/v and HC from urban pruning residues at 2% w/v induced a 6.4- and 21-fold increase in P. eryngii laccase activity over controls, respectively. Despite the promoting impacts of BC on laccase production, some inhibitory effects were noticed in connection with higher doses of BC (2%, w/v) in laccase expression by T. versicolor and P. ostreatus (Taskin et al., 2019a). On the other hand, Ascough et al. (2010) previously found depressive effects of BC at concentrations as low as 0.5% (w/v) on the growth of P. pulmonarius and T. versicolor. As for the effects of microelements such as Cu, Fe and Mn, Taskin et al. (2019a) could relate laccase expression induction to high levels of Fe (about 4.3 mM) and Mn (2.5 mM) in BC. In contrast, the absence of Mn, coupled with the presence of As, Pb, and Cl at relatively high levels, may have contributed to the decrease of laccase expression by P. ostreatus at both BC doses.
Lonappan et al. (2018a) immobilized laccase on BC from three different feedstocks, i.e., pine wood (BC-PW), pig manure (BC-PM) and almond shell (BC-AS) produced in different pyrolysis conditions, for diclofenac elimination. The specific surface areas of the three BCs, determined using the Brunauer, Emmett, and Teller (BET) method were 14.1 m2 g−1 (BC-PW), 46.1 m2 g−1 (BC-PM) and 17 m2 g−1 (BC-AS), respectively. The BCs exhibited different surface texture, morphology, surface chemistry and functional groups. In addition, they demonstrated good results in covalent laccase immobilization, with BC-PM being the best immobilization support, mostly due to its higher specific area. In a similar study, two BCs prepared from maple (MB) and spruce (SB) were used as supports for laccase immobilization and for chlorinated biphenyl removal in wastewater (Li et al., 2018). FT-IR, SEM and BET analyses showed a honeycomb structure in the MB with a specific area of 613.6 m2g−1 and pore volume 0.695 cm3g−1 while SB exhibited 86.3 m2g−1 specific area and 0.065 cm3g−1 pore volume. Maple-based BC displayed the higher immobilization yield (Li et al., 2018).
As mentioned earlier, several studies have demonstrated the potential of ethanol to induce laccase production (Meza et al., 2005; Manavalan et al., 2013; Valle et al., 2014, 2015). Furthermore, due to its antimicrobial activity, ethanol has also been used as inactivating agent of competing fungal strains (Peters et al., 2013; Lucas et al., 2017) and other undesired microorganisms. In addition, ethanol is a safe, stable, and affordable solvent that can easily permeate the BC structure. Therefore, ethanol-based sterilization of BC and the subsequent use of the soaked BC as a substrate and carrier for laccase production and immobilization may be considered as an attractive means of enhancing the expression of specific fungal laccases. More generally, BC could be soaked in inducer solutions (e.g., copper containing solution) to serve as a complete culture medium of laccase production.
Carbonaceous Materials as a Support for Laccase Immobilization
Carbon-based materials have been identified as effective and valuable supports in enzyme immobilization and have been implemented especially in the past two decades (Daoud et al., 2010). Carbon-based materials usually have fully developed pore structures with adequate pore size and high surface area (up to 1000 m2 g−1) which make them appropriate candidates for enzyme immobilization (Zdarta et al., 2018a). Besides these properties, carbon-based materials contain a great number of functional groups (i.e. carboxyl, and hydroxyl) on their surface which makes them ideal candidates for covalent and adsorption immobilization (Zdarta et al., 2018b).
Graphene and Graphene-Related Materials
Graphene-based materials are promising immobilization supports due to inherent properties such as their high surface area (approximately 2630 m2 g−1), and functional groups such as epoxide, carboxylic, and hydroxyl on their surface (Daneshmandi et al., 2021; Karthik et al., 2021). Graphene materials have been used for enzyme immobilization through adsorption or covalent methodologies (Zhou et al., 2021). For instance, Skoronski et al. (2017) studied immobilization of laccase from Aspergillus sp. on commercial graphene nanoplatelets as a support (Skoronski et al., 2017). In this study, laccase activity immobilized on graphene through adsorption and covalent binding was evaluated. For covalent binding, graphene was modified through a nitration process to ensure that -NH2 groups would be created on its surface. Then using glutaraldehyde as a cross-linker agent, laccase was immobilized on the modified graphene surface. The obtained results demonstrated that laccase immobilized on graphene covalently could maintain its activity (around 80% of initial activity) after six cycles while the other forms of immobilizations such as adsorptive immobilization could not keep the activity after five cycles of operation.
Two other forms of graphene are graphene oxide (GO) and reduced graphene oxide (rGO). GO could be prepared through various methods such as Brodie, Staudenmaier, and Hummers processes in which graphite layers are separated followed by an oxidation step with strong oxidizing agents (Adeel et al., 2018). The oxidation step increases the distance between layers (Adeel et al., 2018). In a study on GO, atomic force microscopy (AFM) analysis demonstrated that a fully enriched surface of GO with abundant oxygen-containing functional groups such as epoxide, hydroxyl, and carboxyl could possibly enable laccase to attach to GO sheets without the need for further modification or cross-linking reagents (Zhang J. et al., 2010). In addition, it was demonstrated that as the extend of reduction of GO increases, the obtained support would have better enzyme loading capability and stability (Skoronski et al., 2017; Catania et al., 2021; Olabi et al., 2021). Kashefi et al. (2019) investigated laccase immobilization on GO covalently. Through addition of glutaraldehyde, it was demonstrated that in the final biocatalyst laccase obtained from Aspergillus sp. was covalently attached to GO sheets. Additionally, the final catalyst maintained 75% of laccase initial activity after six cycles.
Reduced GO is produced through removing oxygen functional groups from GO using different methodologies such as thermal reduction (Mcallister et al., 2007), photo-reduction (Zhang Y. et al., 2010), electrochemical reduction (Ramesha and Sampath, 2009), microwave reduction (Zhu et al., 2010), and chemical reduction (Stankovich et al., 2007; Olabi et al., 2021). Various reducing agents can be implemented in each procedure such as hydroiodic acid, ascorbic acid, hydrazine, and NaBH4 (Pei and Cheng, 2012; Lavin-Lopez et al., 2017). In a study by Patel et al. (2017) laccase was immobilized on a composite support produced through doping Fe3O4 on the rGO surface. The results illustrated that laccase stability was improved 15-fold at room temperature. Furthermore, the biocatalyst maintained 92% of initial activity after 10 cycles (Patel et al., 2017). Table 5 describes each type of graphene and its properties.
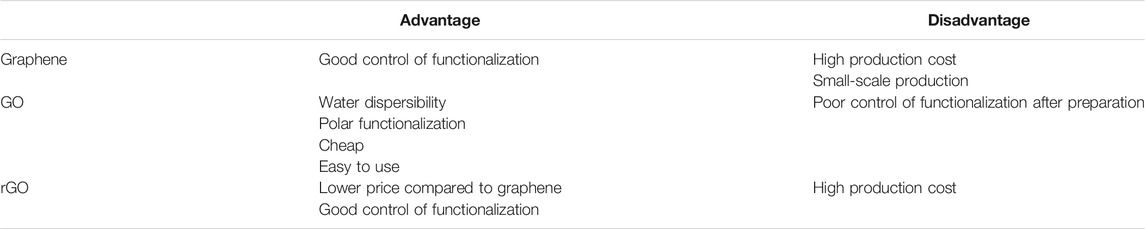
TABLE 5. Advantages and disadvantage of graphene materials (Catania et al., 2021).
Carbon Nanotubes
Carbon nanotubes (CNTs) or buckytubes are hollow cylinders in which carbon atoms are located in hexagonal arrangements (Assi et al., 2021). Since CNT materials are formed from graphene sheets, they demonstrate similar properties to graphene materials like thermal and chemical stability, high tensile strength, and biocompatibility (Karthik et al., 2021). However, graphene atoms are in a two-dimensional arrangement while carbon atoms of CNTs are in a one-dimensional arrangement (Anzar et al., 2020). Moreover, CNTs exhibit radical breathing mode (RBM) in Raman spectrum which is unique to CNTs in comparison with other carbon systems, where all of the carbon atoms move in the radial direction synchronously thus generating an effect similar to breathing (Lei et al., 2011). CNTs can be formed through three different methods, i.e., arc discharge method, laser ablation method and chemical vapor deposition procedure. Commonly, two forms of CNTs can be developed: single wall carbon nanotubes (SWCNTs), and multiple wall carbon nanotube (MWCNTs).
- Single-walled carbon nanotubes:
SWCNTs may be developed from a single graphene sheet rolling upon itself (1–2 nm diameter) (Sabzehmeidani et al., 2021). SWCNTs were first reported in 1993 (Karthik et al., 2021). They have unique properties such as strong covalent bonding, one-dimensional structure, and nanometer size (Liu et al., 2015). Based on how graphene sheets are rolled up, two forms of SWCNTs can be obtained: a zigzag structure, and an armchair structure (Shoukat and Khan, 2021).
- Multi-walled carbon nanotubes:
MWCNTs are prepared by rolling up multiple layers of graphene sheets on themselves (Ali et al., 2021). Based on the number of graphene tubes being rolled up, MWCNT diameter varies from 2 to 50 nm (Ibrahim, 2013). The simplest form of MWCNT is a double-walled carbon nanotube (DWCNT) (Karthik et al., 2021).
Recently, studies on enzyme immobilization over CNTs have increased rapidly since these materials have high surface area, capability of enhanced enzyme loading, and low mass transfer hindrances. For instance, in a study conducted by Xu et al. (2015) laccase was immobilized on a novel composite membrane (polyvinyl alcohol/chitosan/MWCNTs) (Xu et al., 2015). The immobilization was completed through surface modification of the membrane with glutaraldehyde. The final product was shown to maintain 80% of initial laccase activity after seven cycles of operation. As mentioned previously, industrial application of nanoparticles due to their small sizes could be challenging, especially their handling in the environmental arena. Most studies in the field of enzyme immobilization on graphene and carbon nanotubes are related to biosensor applications. In addition, plasma based treatment/production of CNTs may result in better immobilization/loading of laccase. Plasma based treatments are non-polluting in nature and can provide a wide range of functional groups (Ruelle et al., 2011). To the best of our knowledge, this technique has not been used for the immobilization of laccase on plasma treated CNTs. However, Othman et al. (2016) used MWCNTs synthesized using plasma enhanced chemical vapor deposition for the immobilization of laccase (Othman et al., 2016)
Activated Carbon
Activated carbon (AC) denotes amorphous carbonaceous materials with good chemical and physical characteristics (Barroso Bogeat, 2021). Its high surface area (600–1300 m2 g−1) with large number of contact sites makes activated carbon a valuable support for enzyme immobilization (Karthik et al., 2021). Previous studies have demonstrated that natural activated carbon or functionalized activated carbon with HCl could act as a support in laccase immobilization (Sirisha et al., 2016). Recently mesoporous activated carbon with large contact sites has been using for laccase immobilization as well as acid protease and acid lipases immobilization (Ganesh Kumar et al., 2010; Datta et al., 2013). In a study, activated carbon fibers modified with dopamine was utilized as a support for laccase obtained from Aspergillus sp. immobilization (Zhang C. et al., 2018). The results indicated that the biocatalyst had the capability of maintaining its activity (around 60% of initial laccase activity) after six cycles of operation while free laccase only kept 40% of initial activity after the same number of operations (Zhang C. et al., 2018). Table 4 presented various studies of immobilization of enzymes on carbon based materials.
Kinetic Parameters of Immobilized Laccase
Kinetic parameters such as Km, Vmax and the catalytic efficiency kcat/Km determine the catalytic action of enzymes. These parameters can vary considerably depending on the types of enzymes, support materials and process conditions. The Michaelis constant (Km) expresses the affinity of the laccase to the substrate. Vmax is the maximum reaction rate. Low apparent Vmax can result from mass transfer limitations and reduction in enzyme–substrate affinity after immobilization (Gahlout et al., 2017). The Vmax/Km ratio reflects the catalytic efficiency of the enzyme-substrate system. Some values of kinetic parameters related to free laccase and its immobilized counterparts formed using different techniques and carriers are reported in Table 6.
Biodegradation of Organic Contaminants by Immobilized Laccase
A number of studies have been performed using immobilized laccases for the biotransformation of organic contaminants. Most of these studies have been conducted using synthetic wastewater, however a few of them also involved real wastewater, at laboratory or pilot scale. Due to the immobilized enzymes’ overall stability over free enzymes and their recyclability, they generally exhibited higher removal. Table 7 summarizes some of the very recent studies on the application of immobilized laccase for emerging contaminant removal.
Biochar as an Emerging “Carbon Negative” Carbonaceous Solid Support for Immobilization of Laccase
Biochar Properties and Sustainability
BC is a porous carbonaceous solid residue that can be obtained through biomass conversion via hydrothermal and thermochemical processes such as pyrolysis and gasification in the absence of oxygen under various temperatures (Kuzyakov et al., 2009; Liu et al., 2019; Cheng et al., 2021; Madadi and Bester, 2021). BC production is adding value to the economy because in this process wastes and biomass residues can be recycled and reused as secondary resources (Janu et al., 2021). Moreover, BC is carbon negative (Glaser et al., 2009) and its production and application feeds directly into the circular and sustainable economy (Bolognesi et al., 2021). In comparison to activated carbon, BC can be obtained from various types of resources requiring less production energy (Madadi and Bester, 2021). Also, in contrast to activated carbon, BC production is a chemical-free process (Frišták et al., 2018; Madadi and Bester, 2021). The existence of large numbers of polyaromatic carbon groups on BC surfaces with abundant functional groups (carboxyl and hydroxyl) makes it an efficient and low-cost support for immobilization (Komkiene and Baltrenaite, 2016; Kong et al., 2017; Tong et al., 2019). Surface area, existence of functional groups with affinity to laccase and pore size are the crucial parameters affecting laccase immobilization on BC (Madadi and Bester, 2021). BCs with high surface area, activated sites, and the proper porous structure can be considered as a cost-effective candidate compared to activated carbons for enzyme immobilization (Madadi and Bester, 2021). The physical and chemical properties of BC are highly dependent on the feedstock and conditions of production (Barroso Bogeat, 2021; Madadi and Bester, 2021).
Feedstock Composition
BC sources can be divided into two categories, i.e., BCs produced from lignocellulosic materials and BCs produced from non-lignocellulosic materials (Stella Mary et al., 2016; Karim et al., 2019). Lignocellulosic biochars can be divided into three different subcategories namely: wood (hardwood or softwood), crop waste, and grass and leaves (Ippolito et al., 2020). Non-lignocellulosic biochars mainly come from sewage sludge, manure, and algae (Ippolito et al., 2020; Pandey et al., 2020). From lignocellulosic sources, corn, wheat straw, and rice/husk straw are commonly used (Ippolito et al., 2020). Regarding non-lignocellulosic sources poultry, pig, and cattle manure are the most common sources for biochar production (Ippolito et al., 2020). Feedstock significantly affect the carbon content, surface area, and functional groups of final products (Novak et al., 2019). Normally carbon content is proportionally related to biomass lignin content. Biochars produced from wood feedstock demonstrates higher carbon content compared to other sources (Wang et al., 2016). Biochars produced from manure normally have higher content of N, S, and P (Ippolito et al., 2020). In the terms of surface area, lignocellulosic biochars have higher surface and among different sources, wood-based biochar represent higher surface area (Lehmann and Joseph, 2009; Weber and Quicker, 2018). Biochar produced from manure usually have low surface area due to structural cracking or micropore blockage (Ahmad et al., 2014; Ippolito et al., 2017). Regarding functional groups, normally lignocellulosic biochars exhibit content of hydroxyl and carboxyl bonds on their surface (Pandey et al., 2020). However, manure-based biochars demonstrate amine groups on their structures (Leng et al., 2019). The amine content on biochars obtained from different biomasses is followed the pattern in order of wood biochars<crop biochars<grass biochars<manure biochars (Ippolito et al., 2020).
Pyrolysis Type
There are two kinds of pyrolysis, slow and fast. During slow pyrolysis, low temperature heating rate (0.01–2 Cs−1) would be implemented (Sohi et al., 2009). However, temperature heating rate would be higher than 2°Cs−1 in fast pyrolysis. Pyrolysis type would affect surface area and average particle size (Ippolito et al., 2020). Biochar produced through fast pyrolysis usually have higher surface area compared to biochars produced with slow pyrolysis; however, fast pyrolysis biochars demonstrate lower average particle size compared to slow pyrolysis biochars (Asadullah et al., 2010; Qambrani et al., 2017).
Pyrolysis Temperature
Temperature is considered as a significant parameter that affects biochar physiochemical properties. Biochar porosity and surface area would crucially change by pyrolysis temperature variation. Generally, at higher temperature, larger pore volume and surface area would be expected (Mendonça et al., 2017; Weber and Quicker, 2018). Pyrolysis temperature could also affect the content of functional groups and aromatic structure of biochar. Biochar produced at temperature above 500°C demonstrate lower amount of O- and H-containing functional groups (Janu et al., 2021). However, biochars produced below 500°C exhibits higher O- containing functional groups (Janu et al., 2021). For instance, Li X. et al. (2013) studied how variation in pyrolysis temperature could affect biochar properties. The obtained results from two-dimensional (2D) 13C nuclear magnetic resonance (NMR) demonstrated the lower aromaticity ratio (H/C) and lower polarity (O/C and (O+N)/C ratios. This could happen because at higher temperature, the carbon content would increase while H, N, and O contents would decrease (Li X. et al., 2013).
Biochar Engineering
BC engineering is identified as a procedure to manipulate BC properties to enhance its surface area, porosity and the content of functional groups. BC could be engineered through physical and chemical modification procedures.
Physical Activation
In the physical activation approach, no chemical agents are implemented, and this methodology is considered as an economical and simple approach (Rajapaksha et al., 2016). Physical activation of biochar involves the use of gases such steam, CO2, and ozone at temperatures above 700°C (Jimenez-Cordero et al., 2015; Shen et al., 2015; Shim et al., 2015). This modification can be summarized into two steps: first biochar surface area is increased through modification of its unstructured parts and second its crystalline-C formation is improved (Jung and Kim, 2014, 2014; Cha et al., 2016). Park et al. (2016) studied the effect of steam modification on BC surface. In this study BC was produced from P. tenera at 500°C and steam modification was carried out at 700°C for 1 h. The results confirmed that while the surface area of untreated BC was close to zero, that of treated BC increased to 22 m2 g−1 (Park et al., 2016).
Chemical Activation
During chemical modification, BC is mixed with a chemical agent and through dehydration and oxidation, its properties can change (Xiang et al., 2020). Despite its drawback such as the high cost of chemicals, and inability to recover and reuse such chemical agents, this method has a higher efficiency compared to physical activation (Cha et al., 2016). Chemical treatment of BC is achieved using strong acids such as H3PO4, HCl, and H2SO4, and strong bases such as KOH, NaOH, and NH3 (Cha et al., 2016; Zhang C. et al., 2020; Pandey et al., 2020).
Acid treatments normally promote the emergence of oxygen-containing functional groups together with increasing surface area (Rajapaksha et al., 2016). In a study of covalent laccase immobilization on modified BC Lonappan et al. (2018b) used raw BC from pinewood, pig manure, and almond shell. Through BC modification with citric acid, more carboxylic groups were observed on its surface compared to untreated BC (Lonappan et al., 2018b).
BC alkalinization enhances non-polarity with increasing surface area and functional group content. Jin et al., 2014) studied the effects of KOH on the BC produced from municipal solid wastes (Jin et al., 2014). FTIR analysis demonstrated that the number of hydroxyl and carboxyl groups on the surface of treated BC was increased (Jin et al., 2014). In addition, surface area was increased from 14.4 m2 g−1 for raw BC to 49.1 m2 g−1 for treated BC (Jin et al., 2014).
Specific Properties of Biochar for Immobilization of Enzymes
Previously, several studies were carried out on the “carbon negative” biochar to be used as a sustainable and green solid support for the immobilization of laccase (Kuzyakov et al., 2009). Porosity, existence of functional groups, stability, and surface area are important BC properties which could affect immobilization. Previous studies have been conducted to illustrate how feedstock, activation processes, and pyrolysis temperature could affect these properties. For instance, Jin et al. (2014) investigated the effect of chemical activation on BC produced from municipal solid wastes. The obtained results confirmed that KOH activation would increase the surface area from 14.4 m2 g−1 to 49 m2 g−1 (Jin et al., 2014). In another study, Kloss et al. (2012) studied how feedstock could affect surface area. The results illustrated that BCs derived from wood biomass often have higher surface area (Kloss et al., 2012). Furthermore, Zhao L. et al. (2017) studied the effect of pyrolysis temperature on physicochemical properties of produced BCs from apple tree branches. The final results explained that surface area increases with increasing pyrolysis temperature (Zhao S.-X. et al., 2017). More details on BC properties are given in the review by Madadi and Bester (2021).
As a waste management alternative, immobilization of enzymes on BC paves a sustainable pathway in environmental management. However, the disposal/management of used catalyst (i.e. enzyme immobilized on BC) is a potential concern. Despite the environmental friendliness and effectiveness of the BC-laccase catalyst, the disposal of the used catalyst must be carried out properly otherwise the used catalyst itself will end as another potential “emerging contaminant.” Consequently, the used catalyst could be valorized as a fertilizer in soil given the proved ability of BC in fertilizing agricultural lands (Ding et al., 2016). The unused enzyme present on the BC surface can further eliminate pesticides and other organic contaminants present in the soil. In addition, the accumulated nutrients on BC after its application in wastewater treatment (given that wastewater also contains several nutrients (Kaetzl et al., 2020)) will reach the soil as well. Therefore, re-using already utilized BC-enzyme catalyst after wastewater applications is bound to have a considerable positive effect as a soil amendment.
On the other hand, these BC-based biocatalysts could adsorb organic, inorganic and biological contaminants that could have negative impacts on the yield or the crop quality. Thus, the potential impacts as well as the fate of the adsorbed contaminants at the surface of BC should be further studied. Nonetheless, certain contaminants could be further degraded by the residual laccase present on the biochar surface.
Conclusions, Current Research Challenges and Future Perspectives
This review provides a survey on the recent developments of laccase production, immobilization techniques, and application of carbon-based materials as supports.
Low productivity, low stability and limited reusability are the major concerns which challenge the industrial production and application of laccase. Although past studies have concentrated on enhanced laccase production through various methods and then through its immobilization, concerns regarding the cost-effectiveness of these approaches still exist and raise questions regarding their industrial feasibility.
• In the recent past, co-culture has been studied as an effective strategy for the enhanced production of laccase (Chan-Cupul et al., 2016). However, to be a successful process this approach requires the compatible coexistence of the different microbial species involved. Optimization of this process is often challenging. More studies are required to further elucidate the complex pathways behind the co-culture approach for laccase production.
• Inducers were previously proven to be a factor for the enhanced production of laccase if added at the correct concentration. Inducers such as Cu, 2,5-xylidine, guaiacol, etc. enhance laccase production and are usually added in the form of a more complex medium ingredient containing these elements/chemicals. However, addition of expensive chemicals may endanger process economics. Thus, waste/residual materials/inexpensive materials such as biochar can be an economically attractive alternative for industrial production. Nevertheless, it has to be noted that the process must be optimized on the basis of the inducer concentration in the residual materials. In addition, the choice of these “residual material-based” inducers must be made wisely as the presence of potential toxic molecules can inhibit fungal growth and thus laccase production.
• For a given species, the culture media composition is one of the key determining factors which dictates the overall productivity of the process. This is also the most cost-intensive factor (Alessandrello and Vullo, 2016). Thus, inexpensive “culture media alternatives” could significantly reduce the overall cost of the process. In the recent past, the quest for inexpensive and sustainable alternatives for growth media/substrates has resulted in various waste/residual materials such as olive tree saw dust, olive pomace, apple pomace, etc. However, fermentation using these materials must be carried out under solid state conditions for which process control such as maintaining pH, mixing and aeration cannot be easily obtained. On the other hand, for liquid residual materials, submerged fermentations can be carried out and thus adequate process control can be implemented. Research in this domain is minimal and further studies in these directions will improve the sustainability and cost-effectiveness of the overall process at industrial scale.
• Future studies should be carried out in the direction of concomitant enzyme production and immobilization. Instead of completing each procedure separately, the development of procedure using feedstocks which can satisfy both enzyme production enhancement and efficient immobilization would be cost-effective and efficient.
Free laccase is comparatively unstable and expensive and thus it has to be immobilized/cross linked for real life applications. Immobilization of laccase over solid supports could significantly enhance the capability of laccase to maintain its activity over time and its resilience to operational conditions (such as temperature, pH, and exposure to different chemical agents) (Shakerian et al., 2020). Various immobilization methods such as entrapment, adsorptive and covalent immobilization and cross linking have been employed and extensively studied in the past. For immobilization supports the particle size, specific surface area, porosity, mechanical properties and surface functional groups play important roles in the extent of immobilization. Various immobilization supports were studied in the past and application of carbonceous materials is interesting owing to their organic/renewable origin and nature. In particularly, activated carbon, carbon nano tubes, and graphene are well known immobilization supports and which have been used frequently for laccase immobilization.
The application of biochar as an immobilization support for laccase is under-explored and this review is an attempt to summarize the existing studies and further explore biochar’s potential as an immobilization support for laccase. Because of its carbon negative nature (Glaser et al., 2009) application of biochar can be a further step towards sustainability and integration into the circular economy. As previously described, particle size, specific surface area, porosity, mechanical properties and surface functional groups play important roles in the extent of the immobilization. For biochar these properties are often dictated by feedstock composition and method of production. Thus, properly designed and engineered biochar materials can result in excellent immobilization/loading of laccase on their surface. Moreover, biochar activation can be an effective tool for enhanced laccase loading/immobilization.
In summary, the following gaps in research, technological challenges and perspectives for future studies may be noted:
• The application of BC itself as a substrate for fungi can be interesting and challenging at the same time. The limiting factors here may reflect nutrient deficiencies and presence of growth inhibitors. However, further research in this direction could be profitable, given the sustainable and cost-effective nature of BC.
• The minimal cost of BC production and its special features such as the existence of functional groups, porosity, and surface area are key positive factors in considering BC as an immobilization support. However, raw BC is not sufficiently diversified in terms of functional groups. Although a number of past studies have focused on BC functionalization, future work should be directed towards increasing amino groups on the BC surface to enhance the potential of chemical immobilization of laccase and similar enzymes.
• While glutaraldehyde has been identified as a common and efficient cross-linker for chemical immobilization, it can be harmful for the environment or exposed workers. Therefore, future studies should be concentrated to identify green and environmental friendly alternatives.
• BC is rich with many molecules which can act as potential mediators for laccase-based elimination of ECs. Thus, BC-immobilized laccase has already the potential for enhanced elimination of ECs. In addition, further engineering BC with functional groups which can act as mediators for laccase-based elimination of ECs can be a promising area for further research.
Author Contributions
YA, LL, KA, and HC conceived and researched the work. YA, LL, KA, SA, and HC drafted the article. SA and HC critically revised the article. HC provided the funding.
Funding
The authors express their deepest gratitude to the financial support extended by the Natural Sciences and Engineering Research Council of Canada (grant number RGPIN-2019-06178) as well as by the Government of Canada.
Conflict of Interest
The authors declare that the research was conducted in the absence of any commercial or financial relationships that could be construed as a potential conflict of interest.
Publisher’s Note
All claims expressed in this article are solely those of the authors and do not necessarily represent those of their affiliated organizations, or those of the publisher, the editors and the reviewers. Any product that may be evaluated in this article, or claim that may be made by its manufacturer, is not guaranteed or endorsed by the publisher.
References
Adeel, M., Bilal, M., Rasheed, T., Sharma, A., and Iqbal, H. M. N. (2018). Graphene and Graphene Oxide: Functionalization and Nano-Bio-Catalytic System for Enzyme Immobilization and Biotechnological Perspective. Int. J. Biol. Macromolecules 120, 1430–1440. doi:10.1016/j.ijbiomac.2018.09.144
Ahmad, M., Lee, S. S., Lim, J. E., Lee, S.-E., Cho, J. S., Moon, D. H., et al. (2014). Speciation and Phytoavailability of Lead and Antimony in a Small Arms Range Soil Amended with Mussel Shell, Cow Bone and Biochar: EXAFS Spectroscopy and Chemical Extractions. Chemosphere 95, 433–441. doi:10.1016/j.chemosphere.2013.09.077
Ahmed, M. B., Zhou, J. L., Ngo, H. H., Guo, W., Thomaidis, N. S., and Xu, J. (2017). Progress in the Biological and Chemical Treatment Technologies for Emerging Contaminant Removal from Wastewater: A Critical Review. J. Hazard. Mater. 323, 274–298. doi:10.1016/j.jhazmat.2016.04.045
Alessandrello, M. J., and Vullo, D. L. (2016). Economical Fermentation Media for the Production of a Whole Cell Catalyst for the Treatment of Cr(VI)-containing Wastewaters. Revista Argentina de Microbiología 48, 245–251. doi:10.1016/j.ram.2016.04.001
Ali, I., AlGarni, T. S., Burakova, E., Tkachev, A., Tugolukov, E., Dyachkova, T., et al. (2021). A New Approach to the Economic Synthesis of Multi-Walled Carbon Nanotubes Using a Ni/MgO Catalyst. Mater. Chem. Phys. 261, 124234. doi:10.1016/j.matchemphys.2021.124234
Amin, R., Khorshidi, A., Shojaei, A. F., Rezaei, S., and Faramarzi, M. A. (2018). Immobilization of Laccase on Modified Fe3O4@SiO2@Kit-6 Magnetite Nanoparticles for Enhanced Delignification of Olive Pomace Bio-Waste. Int. J. Biol. Macromolecules 114, 106–113. doi:10.1016/j.ijbiomac.2018.03.086
Antecka, A., Blatkiewicz, M., Głuszcz, P., and Ledakowicz, S. (2021). Improvement of Laccase Biosynthesis by Various Feeding Strategies and In Situ Integration of Biomass Separation. Chem. Eng. Process. - Process Intensification 159, 108237. doi:10.1016/j.cep.2020.108237
Anzar, N., Hasan, R., Tyagi, M., Yadav, N., and Narang, J. (2020). Carbon Nanotube - A Review on Synthesis, Properties and Plethora of Applications in the Field of Biomedical Science. Sensors Int. 1, 100003. doi:10.1016/j.sintl.2020.100003
Arca-Ramos, A., Kumar, V. V., Eibes, G., Moreira, M. T., and Cabana, H. (2016). Recyclable Cross-Linked Laccase Aggregates Coupled to Magnetic Silica Microbeads for Elimination of Pharmaceuticals from Municipal Wastewater. Environ. Sci. Pollut. Res. 23, 8929–8939. doi:10.1007/s11356-016-6139-x
Ardao, I., Magnin, D., and Agathos, S. N. (2015). Bioinspired Production of Magnetic Laccase-Biotitania Particles for the Removal of Endocrine Disrupting Chemicals. Biotechnol. Bioeng. 112, 1986–1996. doi:10.1002/bit.25612
Ariste, A. F., Batista-García, R. A., Vaidyanathan, V. K., Raman, N., Vaithyanathan, V. K., Folch-Mallol, J. L., et al. (2020). Mycoremediation of Phenols and Polycyclic Aromatic Hydrocarbons from a Biorefinery Wastewater and Concomitant Production of Lignin Modifying Enzymes. J. Clean. Prod. 253, 119810. doi:10.1016/j.jclepro.2019.119810
Arregui, L., Ayala, M., Gómez-Gil, X., Gutiérrez-Soto, G., Hernández-Luna, C. E., Herrera de los Santos, M., et al. (2019). Laccases: Structure, Function, and Potential Application in Water Bioremediation. Microb. Cel Fact 18, 200. doi:10.1186/s12934-019-1248-0
Asadullah, M., Zhang, S., and Li, C.-Z. (2010). Evaluation of Structural Features of Chars from Pyrolysis of Biomass of Different Particle Sizes. Fuel Process. Tech. 91, 877–881. doi:10.1016/j.fuproc.2009.08.008
Ascough, P. L., Sturrock, C. J., and Bird, M. I. (2010). Investigation of Growth Responses in Saprophytic Fungi to Charred Biomass. Isotopes Environ. Health Stud. 46, 64–77. doi:10.1080/10256010903388436
Asif, M. B., Hai, F. I., Hou, J., Price, W. E., and Nghiem, L. D. (2017). Impact of Wastewater Derived Dissolved Interfering Compounds on Growth, Enzymatic Activity and Trace Organic Contaminant Removal of White Rot Fungi - A Critical Review. J. Environ. Manage. 201, 89–109. doi:10.1016/j.jenvman.2017.06.014
Assi, L., Alsalman, A., Bianco, D., Ziehl, P., El-Khatib, J., Bayat, M., et al. (2021). Multiwall Carbon Nanotubes (MWCNTs) Dispersion & Mechanical Effects in OPC Mortar & Paste: A Review. J. Building Eng. 43, 102512. doi:10.1016/j.jobe.2021.102512
Ba, S., Arsenault, A., Hassani, T., Jones, J. P., and Cabana, H. (2013). Laccase Immobilization and Insolubilization: From Fundamentals to Applications for the Elimination of Emerging Contaminants in Wastewater Treatment. Crit. Rev. Biotechnol. 33, 404–418. doi:10.3109/07388551.2012.725390
Bagewadi, Z. K., Mulla, S. I., and Ninnekar, H. Z. (2017). Optimization of Laccase Production and its Application in Delignification of Biomass. Int. J. Recycl Org. Waste Agricult 6, 351–365. doi:10.1007/s40093-017-0184-4
Baldrian, P. (2003). Interactions of Heavy Metals with White-rot Fungi. Enzyme Microb. Tech. 32, 78–91. doi:10.1016/S0141-0229(02)00245-4
Barroso Bogeat, A. (2021). Understanding and Tuning the Electrical Conductivity of Activated Carbon: A State-Of-The-Art Review. Crit. Rev. Solid State. Mater. Sci. 46, 1–37. doi:10.1080/10408436.2019.1671800
Barry, M. J. (2014). Fluoxetine Inhibits Predator Avoidance Behavior in Tadpoles. Toxicol. Environ. Chem. 96, 641–649. doi:10.1080/02772248.2014.966713
Bayne, L., Ulijn, R. V., and Halling, P. J. (2013). Effect of Pore Size on the Performance of Immobilised Enzymes. Chem. Soc. Rev. 42, 9000–9010. doi:10.1039/c3cs60270b
Bayramoglu, G., and Arica, M. Y. (2019). Biodegradation of Methylene Blue and Carbaryl by Trametes versicolor Laccase Preparations in the Presence of a Mediator Compound. J. Macromolecular Sci. A 56, 277–285. doi:10.1080/10601325.2019.1565549
Bilal, M., Adeel, M., Rasheed, T., Zhao, Y., and Iqbal, H. M. N. (2019a). Emerging Contaminants of High Concern and Their Enzyme-Assisted Biodegradation - A Review. Environ. Int. 124, 336–353. doi:10.1016/j.envint.2019.01.011
Bilal, M., Anh Nguyen, T., and Iqbal, H. M. N. (2020). Multifunctional Carbon Nanotubes and Their Derived Nano-Constructs for Enzyme Immobilization - A Paradigm Shift in Biocatalyst Design. Coord. Chem. Rev. 422, 213475. doi:10.1016/j.ccr.2020.213475
Bilal, M., and Iqbal, H. M. N. (2019). Chemical, Physical, and Biological Coordination: An Interplay Between Materials and Enzymes as Potential Platforms for Immobilization. Coord. Chem. Rev. 388, 1–23. doi:10.1016/j.ccr.2019.02.024
Bilal, M., Jing, Z., Zhao, Y., and Iqbal, H. M. N. (2019b). Immobilization of Fungal Laccase on Glutaraldehyde Cross-Linked Chitosan Beads and its Bio-Catalytic Potential to Degrade Bisphenol A. Biocatal. Agric. Biotechnol. 19, 101174. doi:10.1016/j.bcab.2019.101174
Bilińska, L., Blus, K., Gmurek, M., and Ledakowicz, S. (2019). Coupling of Electrocoagulation and Ozone Treatment for Textile Wastewater Reuse. Chem. Eng. J. 358, 992–1001. doi:10.1016/j.cej.2018.10.093
Bolivar, J. M., Mateo, C., Godoy, C., Pessela, B. C. C., Rodrigues, D. S., Giordano, R. L. C., et al. (2009). The Co-operative Effect of Physical and Covalent Protein Adsorption on Heterofunctional Supports. Process Biochem. 44, 757–763. doi:10.1016/j.procbio.2009.03.012
Bolognesi, S., Bernardi, G., Callegari, A., Dondi, D., and Capodaglio, A. G. (2021). Biochar Production from Sewage Sludge and Microalgae Mixtures: Properties, Sustainability and Possible Role in Circular Economy. Biomass Conv. Bioref. 11, 289–299. doi:10.1007/s13399-019-00572-5
Boniello, C., Mayr, T., Klimant, I., Koenig, B., Riethorst, W., and Nidetzky, B. (2010). Intraparticle Concentration Gradients for Substrate and Acidic Product in Immobilized Cephalosporin C Amidase and Their Dependencies on Carrier Characteristics and Reaction Parameters. Biotechnol. Bioeng. 106, 528–540. doi:10.1002/bit.22694
Bortone, N., Fidaleo, M., and Moresi, M. (2014). Internal and External Mass Transfer Limitations on the Activity of Immobilised Acid Urease Derivatives Differing in Enzyme Loading. Biochem. Eng. J. 82, 22–33. doi:10.1016/j.bej.2013.10.017
Bugg, T. D. H., Williamson, J. J., and Rashid, G. M. M. (2020). Bacterial Enzymes for Lignin Depolymerisation: New Biocatalysts for Generation of Renewable Chemicals from Biomass. Curr. Opin. Chem. Biol. 55, 26–33. doi:10.1016/j.cbpa.2019.11.007
Buss, W., Mašek, O., Graham, M., and Wüst, D. (2015). Inherent Organic Compounds in Biochar-Their Content, Composition and Potential Toxic Effects. J. Environ. Manage. 156, 150–157. doi:10.1016/j.jenvman.2015.03.035
Cárdenas-Fernández, M., Neto, W., López, C., Álvaro, G., Tufvesson, P., and Woodley, J. M. (2012). Immobilization of Escherichia coli Containing ω-transaminase Activity in LentiKats®. Biotechnol. Prog. 28, 693–698. doi:10.1002/btpr.1538
Cabana, H., Jones, J. P., and Agathos, S. N. (2007). Preparation and Characterization of Cross-Linked Laccase Aggregates and Their Application to the Elimination of Endocrine Disrupting Chemicals. J. Biotechnol. 132, 23–31. doi:10.1016/j.jbiotec.2007.07.948
Catania, F., Marras, E., Giorcelli, M., Jagdale, P., Lavagna, L., Tagliaferro, A., et al. (2021). A Review on Recent Advancements of Graphene and Graphene-Related Materials in Biological Applications. Appl. Sci. 11, 614–621. doi:10.3390/app11020614
Cha, J. S., Park, S. H., Jung, S.-C., Ryu, C., Jeon, J.-K., Shin, M.-C., et al. (2016). Production and Utilization of Biochar: A Review. J. Ind. Eng. Chem. 40, 1–15. doi:10.1016/j.jiec.2016.06.002
Chan-Cupul, W., Heredia-Abarca, G., and Rodríguez-Vázquez, R. (2016). Atrazine Degradation by Fungal Co-culture Enzyme Extracts Under Different Soil Conditions. J. Environ. Sci. Health B 51, 298–308. doi:10.1080/03601234.2015.1128742
Chang, B.-V., and Chang, Y.-M. (2016). Biodegradation of Toxic Chemicals by Pleurotus Eryngii in Submerged Fermentation and Solid-State Fermentation. J. Microbiol. Immunol. Infect. 49, 175–181. doi:10.1016/j.jmii.2014.04.012
Chauhan, R. (2019). Nitrogen Sources and Trace Elements Influence Laccase and Peroxidase Enzymes Activity of Grammothele Fuligo. Vegetos 32, 316–323. doi:10.1007/s42535-019-00049-w
Chen, J., Leng, J., Yang, X., Liao, L., Liu, L., and Xiao, A. (2017). Enhanced Performance of Magnetic Graphene Oxide-Immobilized Laccase and its Application for the Decolorization of Dyes. Molecules 22, 221. doi:10.3390/molecules22020221
Chen, X., Zhou, Q., Liu, F., Peng, Q., and Bian, Y. (2021). Performance and Kinetic of Pesticide Residues Removal by Microporous Starch Immobilized Laccase in a Combined Adsorption and Biotransformation Process. Environ. Tech. Innovation 21, 101235. doi:10.1016/j.eti.2020.101235
Chen, X., Zhou, Q., Liu, F., Peng, Q., and Teng, P. (2019). Removal of Nine Pesticide Residues from Water and Soil by Biosorption Coupled with Degradation on Biosorbent Immobilized Laccase. Chemosphere 233, 49–56. doi:10.1016/j.chemosphere.2019.05.144
Cheng, N., Wang, B., Wu, P., Lee, X., Xing, Y., Chen, M., et al. (2021). Adsorption of Emerging Contaminants from Water and Wastewater by Modified Biochar: A Review. Environ. Pollut. 273, 116448. doi:10.1016/j.envpol.2021.116448
Chenthamarakshan, A., Parambayil, N., Miziriya, N., Soumya, P. S., Lakshmi, M. S. K., Ramgopal, A., et al. (2017). Optimization of Laccase Production from Marasmiellus Palmivorus LA1 by Taguchi Method of Design of Experiments. BMC Biotechnol. 17, 12. doi:10.1186/s12896-017-0333-x
Costa, J. B., Lima, M. J., Sampaio, M. J., Neves, M. C., Faria, J. L., Morales-Torres, S., et al. (2019). Enhanced Biocatalytic Sustainability of Laccase by Immobilization on Functionalized Carbon Nanotubes/polysulfone Membranes. Chem. Eng. J. 355, 974–985. doi:10.1016/j.cej.2018.08.178
Dai, Y., Yao, J., Song, Y., Liu, X., Wang, S., and Yuan, Y. (2016). Enhanced Performance of Immobilized Laccase in Electrospun Fibrous Membranes by Carbon Nanotubes Modification and its Application for Bisphenol A Removal from Water. J. Hazard. Mater. 317, 485–493. doi:10.1016/j.jhazmat.2016.06.017
Daneshmandi, L., Barajaa, M., Tahmasbi Rad, A., Sydlik, S. A., and Laurencin, C. T. (2021). Graphene‐Based Biomaterials for Bone Regenerative Engineering: A Comprehensive Review of the Field and Considerations Regarding Biocompatibility and Biodegradation. Adv. Healthc. Mater. 10, 2001414–2001425. doi:10.1002/adhm.202001414
Dao, A. T. N., Vonck, J., Janssens, T. K. S., Dang, H. T. C., Brouwer, A., and de Boer, T. E. (2019). Screening White-rot Fungi for Bioremediation Potential of 2,3,7,8-Tetrachlorodibenzo-P-Dioxin. Ind. Crops Prod. 128, 153–161. doi:10.1016/j.indcrop.2018.10.059
Daoud, F. B.-O., Kaddour, S., and Sadoun, T. (2010). Adsorption of Cellulase Aspergillus niger on a Commercial Activated Carbon: Kinetics and Equilibrium Studies. Colloids Surf. B: Biointerfaces 75, 93–99. doi:10.1016/j.colsurfb.2009.08.019
Daronch, N. A., Kelbert, M., Pereira, C. S., de Araújo, P. H. H., and de Oliveira, D. (2020). Elucidating the Choice for a Precise Matrix for Laccase Immobilization: A Review. Chem. Eng. J. 397, 125506. doi:10.1016/j.cej.2020.125506
Datta, S., Christena, L. R., and Rajaram, Y. R. S. (2013). Enzyme Immobilization: An Overview on Techniques and Support Materials. 3 Biotech. 3, 1–9. doi:10.1007/s13205-012-0071-7
Dayaram, P., and Dasgupta, D. (2008). Decolorisation of Synthetic Dyes and Textile Wastewater Using Polyporus Rubidus. J. Environ. Biol. 29, 831–836.
DiCosimo, R., McAuliffe, J., Poulose, A. J., and Bohlmann, G. (2013). Industrial Use of Immobilized Enzymes. Chem. Soc. Rev. 42, 6437–6474. doi:10.1039/c3cs35506c
Ding, Y., Liu, Y., Liu, S., Li, Z., Tan, X., Huang, X., et al. (2016). Biochar to Improve Soil Fertility. A Review. Agron. Sustain. Dev. 36, 36. doi:10.1007/s13593-016-0372-z
Domingos, M., Souza-Cruz, P. B. d., Ferraz, A., and Prata, A. M. R. (2017). A New Bioreactor Design for Culturing Basidiomycetes: Mycelial Biomass Production in Submerged Cultures of Ceriporiopsis Subvermispora. Chem. Eng. Sci. 170, 670–676. doi:10.1016/j.ces.2017.04.004
Egea-Corbacho Lopera, A., Gutiérrez Ruiz, S., and Quiroga Alonso, J. M. (2019). Removal of Emerging Contaminants from Wastewater Using Reverse Osmosis for its Subsequent Reuse: Pilot Plant. J. Water Process Eng. 29, 100800. doi:10.1016/j.jwpe.2019.100800
Elisashvili, V., Kachlishvili, E., Asatiani, M., Darlington, R., and Kucharzyk, K. (2017). Physiological Peculiarities of Lignin-Modifying Enzyme Production by the White-Rot Basidiomycete Coriolopsis Gallica Strain BCC 142. Microorganisms 5, 73. doi:10.3390/microorganisms5040073
Elisashvili, V., Kachlishvili, E., and Asatiani, M. D. (2018). Efficient Production of Lignin-Modifying Enzymes and Phenolics Removal in Submerged Fermentation of Olive Mill By-Products by White-rot Basidiomycetes. Int. Biodeterioration Biodegradation 134, 39–47. doi:10.1016/j.ibiod.2018.08.003
Elisashvili, V., Kachlishvili, E., Khardziani, T., and Agathos, S. N. (2010). Effect of Aromatic Compounds on the Production of Laccase and Manganese Peroxidase by White-rot Basidiomycetes. J. Ind. Microbiol. Biotechnol. 37, 1091–1096. doi:10.1007/s10295-010-0757-y
Englande, A. J., Krenkel, P., and Shamas, J. (2015). Wastewater Treatment and Water Reclamation (Amsterdam: Elsevier). doi:10.1016/b978-0-12-409548-9.09508-7
Fernández-Fernández, M., Sanromán, M. Á., and Moldes, D. (2013). Recent Developments and Applications of Immobilized Laccase. Biotechnol. Adv. 31, 1808–1825. doi:10.1016/j.biotechadv.2012.02.013
Fernandez-Sanroman, A., Acevedo-García, V., Pazos, M., Sanromán, M. A., and Rosales, E. (2020). Removal of Sulfamethoxazole and Methylparaben Using Hydrocolloid and Fiber Industry Wastes: Comparison with Biochar and Laccase-Biocomposite. J. Clean. Prod. 271, 122436. doi:10.1016/j.jclepro.2020.122436
Figueiredo, P., Lintinen, K., Hirvonen, J. T., Kostiainen, M. A., and Santos, H. A. (2018). Properties and Chemical Modifications of Lignin: Towards Lignin-Based Nanomaterials for Biomedical Applications. Prog. Mater. Sci. 93, 233–269. doi:10.1016/j.pmatsci.2017.12.001
Forde, J., Tully, E., Vakurov, A., Gibson, T. D., Millner, P., and Ó’Fágáin, C. (2010). Chemical Modification and Immobilisation of Laccase from Trametes Hirsuta and from Myceliophthora Thermophila. Enzyme Microb. Tech. 46, 430–437. doi:10.1016/j.enzmictec.2010.01.004
Frišták, V., Pipíška, M., and Soja, G. (2018). Pyrolysis Treatment of Sewage Sludge: A Promising Way to Produce Phosphorus Fertilizer. J. Clean. Prod. 172, 1772–1778. doi:10.1016/j.jclepro.2017.12.015
Furukawa, T., Bello, F. O., and Horsfall, L. (2014). Microbial Enzyme Systems for Lignin Degradation and Their Transcriptional Regulation. Front. Biol. 9, 448–471. doi:10.1007/s11515-014-1336-9
Gahlout, M., Rudakiya, D. M., Gupte, S., and Gupte, A. (2017). Laccase-conjugated Amino-Functionalized Nanosilica for Efficient Degradation of Reactive Violet 1 Dye. Int. Nano Lett. 7, 195–208. doi:10.1007/s40089-017-0215-1
Galhaup, C., and Haltrich, D. (2001). Enhanced Formation of Laccase Activity by the White-rot Fungus Trametes Pubescens in the Presence of Copper. Appl. Microbiol. Biotechnol. 56, 225–232. doi:10.1007/s002530100636
Ganesh Kumar, A., Perinbam, K., Kamatchi, P., Nagesh, N., and Sekaran, G. (2010). In Situ Immobilization of Acid Protease on Mesoporous Activated Carbon Packed Column for the Production of Protein Hydrolysates. Bioresour. Tech. 101, 1377–1379. doi:10.1016/j.biortech.2009.09.014
Garcia-Galan, C., Berenguer-Murcia, Á., Fernandez-Lafuente, R., and Rodrigues, R. C. (2011). Potential of Different Enzyme Immobilization Strategies to Improve Enzyme Performance. Adv. Synth. Catal. 353, 2885–2904. doi:10.1002/adsc.201100534
García-Morales, R., García-García, A., Orona-Navar, C., Osma, J. F., Nigam, K. D. P., and Ornelas-Soto, N. (2018). Biotransformation of Emerging Pollutants in Groundwater by Laccase from P. Sanguineus CS43 Immobilized onto Titania Nanoparticles. J. Environ. Chem. Eng. 6, 710–717. doi:10.1016/j.jece.2017.12.006
Giller, K. E., Witter, E., and Mcgrath, S. P. (1998). Toxicity of Heavy Metals to Microorganisms and Microbial Processes in Agricultural Soils: A Review. Soil Biol. Biochem. 30, 1389–1414. doi:10.1016/S0038-0717(97)00270-8
Girelli, A. M., Quattrocchi, L., and Scuto, F. R. (2020). Silica-chitosan Hybrid Support for Laccase Immobilization. J. Biotechnol. 318, 45–50. doi:10.1016/j.jbiotec.2020.05.004
Glaser, B., Parr, M., Braun, C., and Kopolo, G. (2009). Biochar Is Carbon Negative. Nat. Geosci 2, 2. doi:10.1038/ngeo395
Gomes, I. B., Maillard, J.-Y., Simões, L. C., and Simões, M. (2020). Emerging Contaminants Affect the Microbiome of Water Systems-Strategies for Their Mitigation. Npj Clean. Water 3, 1. doi:10.1038/s41545-020-00086-y
Grazu, V., Betancor, L., Montes, T., Lopez-Gallego, F., Guisan, J. M., and Fernandez-Lafuente, R. (2006). Glyoxyl Agarose as a New Chromatographic Matrix. Enzyme Microb. Tech. 38, 960–966. doi:10.1016/j.enzmictec.2005.08.034
Hachi, M., Chergui, A., Yeddou, A. R., Selatnia, A., and Cabana, H. (2017). Removal of Acetaminophen and Carbamazepine in Single and Binary Systems with Immobilized Laccase from Trametes Hirsuta. Biocatal. Biotransformation 35, 51–62. doi:10.1080/10242422.2017.1280032
Hafid, H. S., Baharuddin, A. S., Mokhtar, M. N., Omar, F. N., Mohammed, M. A. P., and Wakisaka, M. (2021). Enhanced Laccase Production for Oil palm Biomass Delignification Using Biological Pretreatment and its Estimation at Biorefinary Scale. Biomass and Bioenergy 144, 105904. doi:10.1016/j.biombioe.2020.105904
Hanefeld, U., Gardossi, L., and Magner, E. (2009). Understanding Enzyme Immobilisation. Chem. Soc. Rev. 38, 453–468. doi:10.1039/b711564b
Hariharan, S., and Nambisan, P. (2012). Optimization of Lignin Peroxidase, Manganese Peroxidase, and Lac Production from Ganoderma Lucidum Under Solid State Fermentation of Pineapple Leaf. BioResources 8, 250–271. doi:10.15376/biores.8.1.250-271
Harms, H., Schlosser, D., and Wick, L. Y. (2011). Untapped Potential: Exploiting Fungi in Bioremediation of Hazardous Chemicals. Nat. Rev. Microbiol. 9, 177–192. doi:10.1038/nrmicro2519
Hartmann, M., and Kostrov, X. (2013). Immobilization of Enzymes on Porous Silicas - Benefits and Challenges. Chem. Soc. Rev. 42, 6277–6289. doi:10.1039/c3cs60021a
Hermanson, G. T. (2013). Bioconjugate Techniques. Amsterdam, Netherlands: Elsevier. doi:10.1016/C2009-0-64240-9
Hernandez, K., and Fernandez-Lafuente, R. (2011). Control of Protein Immobilization: Coupling Immobilization and Site-Directed Mutagenesis to Improve Biocatalyst or Biosensor Performance. Enzyme Microb. Tech. 48, 107–122. doi:10.1016/j.enzmictec.2010.10.003
Hudson, S., Cooney, J., and Magner, E. (2008). Proteins in Mesoporous Silicates. Angew. Chem. Int. Ed. 47, 8582–8594. doi:10.1002/anie.200705238
Husk, B., Sanchez, J. S., Leduc, R., Takser, L., Savary, O., and Cabana, H. (2019). Pharmaceuticals and Pesticides in Rural Community Drinking Waters of Quebec, Canada - A Regional Study on the Susceptibility to Source Contamination. Water Qual. Res. J. Can. 54 (2), 88–103. doi:10.2166/wqrj.2019.038
Ibrahim, K. S. (2013). Carbon Nanotubes-Properties and Applications: A Review. Carbon Lett. 14, 131–144. doi:10.5714/cl.2013.14.3.131
Imam, A., Suman, S. K., Singh, R., Vempatapu, B. P., Ray, A., and Kanaujia, P. K. (2021). Application of Laccase Immobilized Rice Straw Biochar for Anthracene Degradation. Environ. Pollut. 268, 115827. doi:10.1016/j.envpol.2020.115827
Ippolito, J. A., Berry, C. M., Strawn, D. G., Novak, J. M., Levine, J., and Harley, A. (2017). Biochars Reduce Mine Land Soil Bioavailable Metals. J. Environ. Qual. 46, 411–419. doi:10.2134/jeq2016.10.0388
Ippolito, J. A., Cui, L., Kammann, C., Wrage-Mönnig, N., Estavillo, J. M., Fuertes-Mendizabal, T., et al. (2020). Feedstock Choice, Pyrolysis Temperature and Type Influence Biochar Characteristics: A Comprehensive Meta-Data Analysis Review. Biochar 2, 421–438. doi:10.1007/s42773-020-00067-x
Janu, R., Mrlik, V., Ribitsch, D., Hofman, J., Sedláček, P., Bielská, L., et al. (2021). Biochar Surface Functional Groups as Affected by Biomass Feedstock, Biochar Composition and Pyrolysis Temperature. Carbon Resour. Convers. 4, 36–46. doi:10.1016/j.crcon.2021.01.003
Jasińska, A., Góralczyk-Bińkowska, A., Soboń, A., and Długoński, J. (2019). Lignocellulose Resources for the Myrothecium Roridum Laccase Production and Their Integrated Application for Dyes Removal. Int. J. Environ. Sci. Technol. 16, 4811–4822. doi:10.1007/s13762-019-02290-x
Jéquier, E., and Constant, F. (2010). Water as an Essential Nutrient: The Physiological Basis of Hydration. Eur. J. Clin. Nutr. 64, 115–123. doi:10.1038/ejcn.2009.111
Ji, C., Nguyen, L. N., Hou, J., Hai, F. I., and Chen, V. (2017). Direct Immobilization of Laccase on Titania Nanoparticles from Crude Enzyme Extracts of P. Ostreatus Culture for Micro-pollutant Degradation. Sep. Purif. Tech. 178, 215–223. doi:10.1016/j.seppur.2017.01.043
Jiménez-Barrera, D., Chan-Cupul, W., Fan, Z., and Osuna-Castro, J. A. (2018). Fungal Co-culture Increases Ligninolytic Enzyme Activities: Statistical Optimization Using Response Surface Methodology. Prep. Biochem. Biotechnol. 48, 787–798. doi:10.1080/10826068.2018.1509084
Jimenez-Cordero, D., Heras, F., Alonso-Morales, N., Gilarranz, M. A., and Rodriguez, J. J. (2015). Ozone as Oxidation Agent in Cyclic Activation of Biochar. Fuel Process. Tech. 139, 42–48. doi:10.1016/j.fuproc.2015.08.016
Jin, H., Capareda, S., Chang, Z., Gao, J., Xu, Y., and Zhang, J. (2014). Biochar Pyrolytically Produced from Municipal Solid Wastes for Aqueous As(V) Removal: Adsorption Property and its Improvement with KOH Activation. Bioresour. Tech. 169, 622–629. doi:10.1016/j.biortech.2014.06.103
J. Lehmann, and S. Joseph (Editors) (2009). Biochar for Environmental Management: Science and Technology (London; Sterling, VA: Earthscan).
Joffres, B., Laurenti, D., Charon, N., Daudin, A., Quignard, A., and Geantet, C. (2013). Thermochemical Conversion of Lignin for Fuels and Chemicals: A Review. Oil Gas Sci. Technol. - Rev. IFP Energies Nouvelles 68, 753–763. doi:10.2516/ogst/2013132
Jung, S.-H., and Kim, J.-S. (2014). Production of Biochars by Intermediate Pyrolysis and Activated Carbons from Oak by Three Activation Methods Using CO2. J. Anal. Appl. Pyrolysis 107, 116–122. doi:10.1016/j.jaap.2014.02.011
Kaetzl, K., Lübken, M., Nettmann, E., Krimmler, S., and Wichern, M. (2020). Slow Sand Filtration of Raw Wastewater Using Biochar as an Alternative Filtration Media. Sci. Rep. 10, 1229. doi:10.1038/s41598-020-57981-0
Kameshwar, A. K. S., and Qin, W. (2017). Qualitative and Quantitative Methods for Isolation and Characterization of Lignin-Modifying Enzymes Secreted by Microorganisms. Bioenerg. Res. 10, 248–266. doi:10.1007/s12155-016-9784-5
Karim, A. A., Kumar, M., Mohapatra, S., and Singh, S. K. (2019). Nutrient Rich Biomass and Effluent Sludge Wastes Co-utilization for Production of Biochar Fertilizer Through Different Thermal Treatments. J. Clean. Prod. 228, 570–579. doi:10.1016/j.jclepro.2019.04.330
Karp, S. G., Faraco, V., Amore, A., Letti, L. A. J., Thomaz Soccol, V., and Soccol, C. R. (2015). Statistical Optimization of Laccase Production and Delignification of Sugarcane Bagasse byPleurotus Ostreatusin Solid-State Fermentation. Biomed. Res. Int. 2015, 1–8. doi:10.1155/2015/181204
Karthik, V., Senthil Kumar, P., Vo, D.-V. N., Selvakumar, P., Gokulakrishnan, M., Keerthana, P., et al. (2021). Enzyme-loaded Nanoparticles for the Degradation of Wastewater Contaminants: A Review. Environ. Chem. Lett. 19, 2331–2350. doi:10.1007/s10311-020-01158-8
Kashefi, S., Borghei, S. M., and Mahmoodi, N. M. (2019). Covalently Immobilized Laccase onto Graphene Oxide Nanosheets: Preparation, Characterization, and Biodegradation of Azo Dyes in Colored Wastewater. J. Mol. Liquids 276, 153–162. doi:10.1016/j.molliq.2018.11.156
Kim, M. I., Kim, J., Lee, J., Shin, S., Na, H. B., Hyeon, T., et al. (2008). One-dimensional Crosslinked Enzyme Aggregates in SBA-15: Superior Catalytic Behavior to Conventional Enzyme Immobilization. Microporous Mesoporous Mater. 111, 18–23. doi:10.1016/j.micromeso.2007.07.009
Kloss, S., Zehetner, F., Dellantonio, A., Hamid, R., Ottner, F., Liedtke, V., et al. (2012). Characterization of Slow Pyrolysis Biochars: Effects of Feedstocks and Pyrolysis Temperature on Biochar Properties. J. Environ. Qual. 41, 990–1000. doi:10.2134/jeq2011.0070
Komkiene, J., and Baltrenaite, E. (2016). Biochar as Adsorbent for Removal of Heavy Metal Ions [Cadmium(II), Copper(II), Lead(II), Zinc(II)] from Aqueous Phase. Int. J. Environ. Sci. Technol. 13, 471–482. doi:10.1007/s13762-015-0873-3
Kong, X., Liu, Y., Pi, J., Li, W., Liao, Q., and Shang, J. (2017). Low-cost Magnetic Herbal Biochar: Characterization and Application for Antibiotic Removal. Environ. Sci. Pollut. Res. 24, 6679–6687. doi:10.1007/s11356-017-8376-z
Koumaki, E., Noutsopoulos, C., Mamais, D., Fragkiskatos, G., and Andreadakis, A. (2021). Fate of Emerging Contaminants in High-Rate Activated Sludge Systems. Ijerph 18, 400. doi:10.3390/ijerph18020400
Krull, R., Wucherpfennig, T., Esfandabadi, M. E., Walisko, R., Melzer, G., Hempel, D. C., et al. (2013). Characterization and Control of Fungal Morphology for Improved Production Performance in Biotechnology. J. Biotechnol. 163, 112–123. doi:10.1016/j.jbiotec.2012.06.024
Kumar, A., and Chandra, R. (2020). Ligninolytic Enzymes and its Mechanisms for Degradation of Lignocellulosic Waste in Environment. Heliyon 6, e03170. doi:10.1016/j.heliyon.2020.e03170
Kumar, R., Kaur, J., Jain, S., and Kumar, A. (2016). Optimization of Laccase Production from Aspergillus flavus by Design of Experiment Technique: Partial Purification and Characterization. J. Genet. Eng. Biotechnol. 14, 125–131. doi:10.1016/j.jgeb.2016.05.006
Kuzyakov, Y., Subbotina, I., Chen, H., Bogomolova, I., and Xu, X. (2009). Black Carbon Decomposition and Incorporation into Soil Microbial Biomass Estimated by 14C Labeling. Soil Biol. Biochem. 41, 210–219. doi:10.1016/j.soilbio.2008.10.016
Lai, Y., Wang, F., Zhang, Y., Ou, P., Wu, P., Fang, Q., et al. (2019). Effective Removal of Methylene Blue and Orange II by Subsequent Immobilized Laccase Decolorization on Crosslinked Polymethacrylate/carbon Nanotubes. Mater. Res. Express 6, 085541. doi:10.1088/2053-1591/ab26a5
Lallawmsanga,, , Leo, V. V., Passari, A. K., Muniraj, I. K., Uthandi, S., Hashem, A., et al. (2019). Elevated Levels of Laccase Synthesis by Pleurotus Pulmonarius BPSM10 and its Potential as a Dye Decolorizing Agent. Saudi J. Biol. Sci. 26, 464–468. doi:10.1016/j.sjbs.2018.10.006
Lam, E., Male, K. B., Chong, J. H., Leung, A. C. W., and Luong, J. H. T. (2012). Applications of Functionalized and Nanoparticle-Modified Nanocrystalline Cellulose. Trends Biotechnol. 30, 283–290. doi:10.1016/j.tibtech.2012.02.001
Lavin-Lopez, M. P., Paton-Carrero, A., Sanchez-Silva, L., Valverde, J. L., and Romero, A. (2017). Influence of the Reduction Strategy in the Synthesis of Reduced Graphene Oxide. Adv. Powder Tech. 28, 3195–3203. doi:10.1016/j.apt.2017.09.032
Lei, M., Zhang, L., Lei, J., Zong, L., Li, J., Wu, Z., et al. (20152015). Overview of Emerging Contaminants and Associated Human Health Effects. Biomed. Res. Int. 2015, 1–12. doi:10.1155/2015/404796
Lei, X.-W., Ni, Q.-Q., Shi, J.-X., and Natsuki, T. (2011). Radial Breathing Mode of Carbon Nanotubes Subjected to Axial Pressure. Nanoscale Res. Lett. 6, 492. doi:10.1186/1556-276X-6-492
Leng, L., Xu, S., Liu, R., Yu, T., Zhuo, X., Leng, S., et al. (2020). Nitrogen Containing Functional Groups of Biochar: An Overview. Bioresour. Tech. 298, 122286. doi:10.1016/j.biortech.2019.122286
Li, N., Xia, Q., Niu, M., Ping, Q., and Xiao, H. (2018). Immobilizing Laccase on Different Species Wood Biochar to Remove the Chlorinated Biphenyl in Wastewater. Sci. Rep. 8, 13947. doi:10.1038/s41598-018-32013-0
Li, X., Shen, Q., Zhang, D., Mei, X., Ran, W., Xu, Y., et al. (2013a). Functional Groups Determine Biochar Properties (pH and EC) as Studied by Two-Dimensional 13C NMR Correlation Spectroscopy. PLoS ONE 8, e65949. doi:10.1371/journal.pone.0065949
Li, X., Xu, Q.-M., Cheng, J.-S., and Yuan, Y.-J. (2016). Improving the Bioremoval of Sulfamethoxazole and Alleviating Cytotoxicity of its Biotransformation by Laccase Producing System Under Coculture of Pycnoporus Sanguineus and Alcaligenes Faecalis. Bioresour. Tech. 220, 333–340. doi:10.1016/j.biortech.2016.08.088
Li, Y., Huang, X., and Qu, Y. (2013b). A Strategy for Efficient Immobilization of Laccase and Horseradish Peroxidase on Single-Walled Carbon Nanotubes. J. Chem. Technol. Biotechnol. 88, 2227–2232. doi:10.1002/jctb.4091
Liu, Y., Ma, H., Huang, J., Li, Z., Pan, Y., and Du, Y. (2019). Carbonaceous Nanomaterials Stimulate Extracellular Enzyme Release by the Fungus Cladosporium Sp. And Enhance Extracellular Electron Transfer to Facilitate Lignin Biodegradation. Sci. Total Environ. 696, 134072. doi:10.1016/j.scitotenv.2019.134072
Liu, Y., Nie, C., Liu, X., Xu, X., Sun, Z., and Pan, L. (2015). Review on Carbon-Based Composite Materials for Capacitive Deionization. RSC Adv. 5, 15205–15225. doi:10.1039/c4ra14447c
Lonappan, L., Liu, Y., Rouissi, T., Brar, S. K., Verma, M., and Surampalli, R. Y. (2018a). Adsorptive Immobilization of Agro-Industrially Produced Crude Laccase on Various Micro-biochars and Degradation of Diclofenac. Sci. Total Environ. 640-641, 1251–1258. doi:10.1016/j.scitotenv.2018.06.005
Lonappan, L., Liu, Y., Rouissi, T., Pourcel, F., Brar, S. K., Verma, M., et al. (2018b). Covalent Immobilization of Laccase on Citric Acid Functionalized Micro-biochars Derived from Different Feedstock and Removal of Diclofenac. Chem. Eng. J. 351, 985–994. doi:10.1016/j.cej.2018.06.157
Lortie, R., and André, G. (1991). On the Use of Apparent Kinetic Parameters for Immobilized Enzyme with Noncompetitive Substrate Inhibition. Enzyme Microb. Tech. 13, 960–963. doi:10.1016/0141-0229(91)90117-S
Lucas, C., Déniel, F., and Dantigny, P. (2017). Ethanol as an Antifungal Treatment for Silver Gelatin Prints: Implementation Methods Evaluation. Restaur. Int. J. Preserv. Libr. Arch. Mater. 38, 235–248. doi:10.1515/res-2017-0003
Ma, W., Cao, B.-K., Xu, S.-N., Chen, D.-M., and Chen, Y. (2017). “Activated Carbon/Polyvinyl Formal Sponge as a Carrier for Laccase Immobilization for Dyes Decolourization,” in Proceedings of the 2nd Annual 2016 International Workshop on Materials Science and Engineering (IWMSE 2016) (IEEE), 339–346. doi:10.1142/9789813226517_0049
Madadi, R., and Bester, K. (2021). Fungi and Biochar Applications in Bioremediation of Organic Micropollutants from Aquatic Media. Mar. Pollut. Bull. 166, 112247. doi:10.1016/j.marpolbul.2021.112247
Maglangit, F., Fang, Q., Kyeremeh, K., Sternberg, J. M., Ebel, R., and Deng, H. (2020). A Co-culturing Approach Enables Discovery and Biosynthesis of a Bioactive Indole Alkaloid Metabolite. Molecules 25, 256. doi:10.3390/molecules25020256
Mahmoodi, N. M., and Saffar-Dastgerdi, M. H. (2020). Clean Laccase Immobilized Nanobiocatalysts (Graphene Oxide - Zeolite Nanocomposites): From Production to Detailed Biocatalytic Degradation of Organic Pollutant. Appl. Catal. B: Environ. 268, 118443. doi:10.1016/j.apcatb.2019.118443
Manavalan, T., Manavalan, A., Thangavelu, K. P., and Heese, K. (2013). Characterization of Optimized Production, Purification and Application of Laccase from Ganoderma Lucidum. Biochem. Eng. J. 70, 106–114. doi:10.1016/j.bej.2012.10.007
Mann, J., Markham, J. L., Peiris, P., Spooner-Hart, R. N., Holford, P., and Nair, N. G. (2015). Use of Olive Mill Wastewater as a Suitable Substrate for the Production of Laccase by Cerrena Consors. Int. Biodeterioration Biodegradation 99, 138–145. doi:10.1016/j.ibiod.2015.01.010
Marin, C. B., Kuroshima, K. N., Santos, A. P. S., and da Silva, M. A. C. (2020). “Evaluation Techniques of the Chemical and Microbiological Water Quality in the Coastal Environment,” in Microbial Enzymes and Biotechniques. Editor P. Shukla (Singapore: Springer Singapore), 207–233. doi:10.1007/978-981-15-6895-4_11
Martani, F., Beltrametti, F., Porro, D., Branduardi, P., and Lotti, M. (2017). The Importance of Fermentative Conditions for the Biotechnological Production of Lignin Modifying Enzymes from white-rot Fungi. FEMS Microbiol. Lett. 364, 1. doi:10.1093/femsle/fnx134
Masjoudi, M., Golgoli, M., Ghobadi Nejad, Z., Sadeghzadeh, S., and Borghei, S. M. (2021). Pharmaceuticals Removal by Immobilized Laccase on Polyvinylidene Fluoride Nanocomposite with Multi-Walled Carbon Nanotubes. Chemosphere 263, 128043. doi:10.1016/j.chemosphere.2020.128043
Mate, D. M., and Alcalde, M. (2017). Laccase: A Multi‐purpose Biocatalyst at the Forefront of Biotechnology. Microb. Biotechnol. 10, 1457–1467. doi:10.1111/1751-7915.12422
Mateo, C., Abian, O., Bernedo, M., Cuenca, E., Fuentes, M., Fernandez-Lorente, G., et al. (2005). Some Special Features of Glyoxyl Supports to Immobilize Proteins. Enzyme Microb. Tech. 37, 456–462. doi:10.1016/j.enzmictec.2005.03.020
Mateo, C., Palomo, J. M., van Langen, L. M., van Rantwijk, F., and Sheldon, R. A. (2004). A New, Mild Cross-Linking Methodology to Prepare Cross-Linked Enzyme Aggregates. Biotechnol. Bioeng. 86, 273–276. doi:10.1002/bit.20033
Matijošytė, I., Arends, I. W. C. E., de Vries, S., and Sheldon, R. A. (2010). Preparation and Use of Cross-Linked Enzyme Aggregates (CLEAs) of Laccases. J. Mol. Catal. B: Enzymatic 62, 142–148. doi:10.1016/j.molcatb.2009.09.019
Mcallister, M. J., Li, J.-L., Adamson, D. H., Schniepp, H. C., Abdala, A. A., Liu, J., et al. (2007). Single Sheet Functionalized Graphene by Oxidation and Thermal Expansion of Graphite. Chem. Mater. 19, 4396–4404. doi:10.1021/cm0630800
Mery-Araya, C., Lear, G., Perez-Garcia, O., Astudillo-Garcia, C., and Singhal, N. (2019). Using Carbon Substrate as a Selection Pressure to Enhance the Potential of Aerobic Granular Sludge Microbial Communities for Removing Contaminants of Emerging Concern. Bioresour. Tech. 290, 121705. doi:10.1016/j.biortech.2019.121705
Meza, J. C., Lomascolo, A., Casalot, L., Sigoillot, J.-C., and Auria, R. (2005). Laccase Production by Pycnoporus Cinnabarinus Grown on Sugar-Cane Bagasse: Influence of Ethanol Vapours as Inducer. Process Biochem. 40, 3365–3371. doi:10.1016/j.procbio.2005.03.004
Migneault, I., Dartiguenave, C., Bertrand, M. J., and Waldron, K. C. (2004). Glutaraldehyde: Behavior in Aqueous Solution, Reaction with Proteins, and Application to Enzyme Crosslinking. BioTechniques 37, 790–802. doi:10.2144/04375RV01
Mikiashvili, N., Wasser, S. P., Nevo, E., and Elisashvili, V. (2006). Effects of Carbon and Nitrogen Sources on Pleurotus Ostreatus Ligninolytic Enzyme Activity. World J. Microbiol. Biotechnol. 22, 999–1002. doi:10.1007/s11274-006-9132-6
Mishra, V., Jana, A. K., Jana, M. M., and Gupta, A. (2017). Enhancement in Multiple Lignolytic Enzymes Production for Optimized Lignin Degradation and Selectivity in Fungal Pretreatment of Sweet Sorghum Bagasse. Bioresour. Tech. 236, 49–59. doi:10.1016/j.biortech.2017.03.148
Mohapatra, D. P., and Kirpalani, D. M. (2019). Advancement in Treatment of Wastewater: Fate of Emerging Contaminants. Can. J. Chem. Eng. 97, 2621–2631. doi:10.1002/cjce.23533
Morales-Caselles, C., Gao, W., Ross, P. S., and Fanning, L. (2016). Emerging Contaminants of Concern in Canadian Harbours: A Case Study of Halifax Harbour. (Halifax: Marine Affairs Program Technical Report), 15. Available at: http://www.dal.ca/faculty/science/marine-affairs-program/research/research-news/map-technical-series-reports.html.
Myasoedova, N. M., Gasanov, N. B., Chernykh, A. M., Kolomytseva, M. P., and Golovleva, L. A. (2015). Selective Regulation of Laccase Isoform Production by theLentinus Strigosus1566 Fungus. Прикл. Биохимия И Микробиол. 51, 221–228. doi:10.7868/S0555109915020130
Naghdi, M., Taheran, M., Brar, S. K., Kermanshahi-pour, A., Verma, M., and Surampalli, R. Y. (2017). Immobilized Laccase on Oxygen Functionalized Nanobiochars Through Mineral Acids Treatment for Removal of Carbamazepine. Sci. Total Environ. 584-585, 393–401. doi:10.1016/j.scitotenv.2017.01.021
Naghdi, M., Taheran, M., Brar, S. K., Kermanshahi-pour, A., Verma, M., and Surampalli, R. Y. (2018). Pinewood Nanobiochar: A Unique Carrier for the Immobilization of Crude Laccase by Covalent Bonding. Int. J. Biol. Macromolecules 115, 563–571. doi:10.1016/j.ijbiomac.2018.04.105
Nair, R. R., Demarche, P., and Agathos, S. N. (2013). Formulation and Characterization of an Immobilized Laccase Biocatalyst and its Application to Eliminate Organic Micropollutants in Wastewater. New Biotechnol. 30, 814–823. doi:10.1016/j.nbt.2012.12.004
Nguyen, L. N., Hai, F. I., Dosseto, A., Richardson, C., Price, W. E., and Nghiem, L. D. (2016). Continuous Adsorption and Biotransformation of Micropollutants by Granular Activated Carbon-Bound Laccase in a Packed-Bed Enzyme Reactor. Bioresour. Tech. 210, 108–116. doi:10.1016/j.biortech.2016.01.014
Nguyen, L. N., Hai, F. I., Price, W. E., Leusch, F. D. L., Roddick, F., Ngo, H. H., et al. (2014). The Effects of Mediator and Granular Activated Carbon Addition on Degradation of Trace Organic Contaminants by an Enzymatic Membrane Reactor. Bioresour. Tech. 167, 169–177. doi:10.1016/j.biortech.2014.05.125
Nguyen, L. T., Seow, N., and Yang, K.-L. (2017). Hollow Cross-Linked Enzyme Aggregates (H-CLEA) of Laccase with High Uniformity and Activity. Colloids Surf. B: Biointerfaces 151, 88–94. doi:10.1016/j.colsurfb.2016.12.006
Novak, J. M., Sigua, G. C., Ducey, T. F., Watts, D. W., and Stone, K. C. (2019). Designer Biochars Impact on Corn Grain Yields, Biomass Production, and Fertility Properties of a Highly-Weathered Ultisol. Environments 6, 64. doi:10.3390/environments6060064
Olabi, A. G., Abdelkareem, M. A., Wilberforce, T., and Sayed, E. T. (2021). Application of Graphene in Energy Storage Device - A Review. Renew. Sust. Energ. Rev. 135, 110026. doi:10.1016/j.rser.2020.110026
Olivieri, G., Marzocchella, A., Salatino, P., Giardina, P., Cennamo, G., and Sannia, G. (2006). Olive Mill Wastewater Remediation by Means of Pleurotus Ostreatus. Biochem. Eng. J. 31, 180–187. doi:10.1016/j.bej.2006.07.005
Ormategui, N., Veloso, A., Leal, G. P., Rodriguez-Couto, S., and Tomovska, R. (2015). Design of Stable and Powerful Nanobiocatalysts, Based on Enzyme Laccase Immobilized on Self-Assembled 3D Graphene/Polymer Composite Hydrogels. ACS Appl. Mater. Inter. 7, 14104–14112. doi:10.1021/acsami.5b03325
Othman, A. M., González-Domínguez, E., Sanromán, Á., Correa-Duarte, M., and Moldes, D. (2016). Immobilization of Laccase on Functionalized Multiwalled Carbon Nanotube Membranes and Application for Dye Decolorization. RSC Adv. 6, 114690–114697. doi:10.1039/c6ra18283f
Ozcirak Ergun, S., and Ozturk Urek, R. (2017). Production of Ligninolytic Enzymes by Solid State Fermentation Using Pleurotus Ostreatus. Ann. Agrarian Sci. 15, 273–277. doi:10.1016/j.aasci.2017.04.003
Palma, C., Lloret, L., Sepúlveda, L., and Contreras, E. (2016). Production of Versatile Peroxidase from Pleurotus Eryngii by Solid-State Fermentation Using Agricultural Residues and Evaluation of its Catalytic Properties. Prep. Biochem. Biotechnol. 46, 200–207. doi:10.1080/10826068.2015.1084513
Pandey, D., Daverey, A., and Arunachalam, K. (2020). Biochar: Production, Properties and Emerging Role as a Support for Enzyme Immobilization. J. Clean. Prod. 255, 120267. doi:10.1016/j.jclepro.2020.120267
Pang, R., Li, M., and Zhang, C. (2015). Degradation of Phenolic Compounds by Laccase Immobilized on Carbon Nanomaterials: Diffusional Limitation Investigation. Talanta 131, 38–45. doi:10.1016/j.talanta.2014.07.045
Park, J. H., Xue, H., Jung, J. S., and Ryu, K. (2012). Immobilization of Laccase on Carbon Nanomaterials. Korean J. Chem. Eng. 29, 1409–1412. doi:10.1007/s11814-012-0024-1
Park, S. H., Cho, H. J., Ryu, C., and Park, Y.-K. (2016). Removal of Copper(II) in Aqueous Solution Using Pyrolytic Biochars Derived from Red Macroalga Porphyra Tenera. J. Ind. Eng. Chem. 36, 314–319. doi:10.1016/j.jiec.2016.02.021
Patel, S. K. S., Anwar, M. Z., Kumar, A., Otari, S. V., Pagolu, R. T., Kim, S.-Y., et al. (2018). Fe2O3 Yolk-Shell Particle-Based Laccase Biosensor for Efficient Detection of 2,6-dimethoxyphenol. Biochem. Eng. J. 132, 1–8. doi:10.1016/j.bej.2017.12.013
Patel, S. K. S., Choi, H., and Lee, J.-K. (2019). Multimetal-Based Inorganic-Protein Hybrid System for Enzyme Immobilization. ACS Sust. Chem. Eng. 7, 13633–13638. doi:10.1021/acssuschemeng.9b02583
Patel, S. K. S., Choi, S. H., Kang, Y. C., and Lee, J.-K. (2017). Eco-friendly Composite of Fe3O4-Reduced Graphene Oxide Particles for Efficient Enzyme Immobilization. ACS Appl. Mater. Inter. 9, 2213–2222. doi:10.1021/acsami.6b05165
Patel, S. K. S., Choi, S. H., Kang, Y. C., and Lee, J.-K. (2016). Large-scale Aerosol-Assisted Synthesis of Biofriendly Fe2O3yolk-Shell Particles: A Promising Support for Enzyme Immobilization. Nanoscale 8, 6728–6738. doi:10.1039/C6NR00346J
Pedroche, J., del Mar Yust, M., Mateo, C., Fernández-Lafuente, R., Girón-Calle, J., Alaiz, M., et al. (2007). Effect of the Support and Experimental Conditions in the Intensity of the Multipoint Covalent Attachment of Proteins on Glyoxyl-Agarose Supports: Correlation Between Enzyme-Support Linkages and Thermal Stability. Enzyme Microb. Tech. 40, 1160–1166. doi:10.1016/j.enzmictec.2006.08.023
Pei, S., and Cheng, H.-M. (2012). The Reduction of Graphene Oxide. Carbon 50, 3210–3228. doi:10.1016/j.carbon.2011.11.010
Peters, B. M., Ward, R. M., Rane, H. S., Lee, S. A., and Noverr, M. C. (2013). Efficacy of Ethanol Against Candida Albicans and Staphylococcus aureus Polymicrobial Biofilms. Antimicrob. Agents Chemother. 57, 74–82. doi:10.1128/AAC.01599-12
Petrie, B., Barden, R., and Kasprzyk-Hordern, B. (2015). A Review on Emerging Contaminants in Wastewaters and the Environment: Current Knowledge, Understudied Areas and Recommendations for Future Monitoring. Water Res. 72, 3–27. doi:10.1016/j.watres.2014.08.053
Phetsom, J., Khammuang, S., Suwannawon, P., and Sarnthima, R. (2009). Copper-alginate Encapsulation of Crude Laccase from Lentinus Polychrous Lev. And Their Effectiveness in Synthetic Dyes Decolorizations. J. Biol. Sci. 9 (6), 573–583. doi:10.3923/jbs.2009.573.583
Pollegioni, L., Tonin, F., and Rosini, E. (2015). Lignin-degrading Enzymes. FEBS J. 282, 1190–1213. doi:10.1111/febs.13224
Qambrani, N. A., Rahman, M. M., Won, S., Shim, S., and Ra, C. (2017). Biochar Properties and Eco-Friendly Applications for Climate Change Mitigation, Waste Management, and Wastewater Treatment: A Review. Renew. Sust. Energ. Rev. 79, 255–273. doi:10.1016/j.rser.2017.05.057
Qian, X., Chen, L., Sui, Y., Chen, C., Zhang, W., Zhou, J., et al. (2020). Biotechnological Potential and Applications of Microbial Consortia. Biotechnol. Adv. 40, 107500. doi:10.1016/j.biotechadv.2019.107500
Qiu, H., Xu, C., Huang, X., Ding, Y., Qu, Y., and Gao, P. (2009). Immobilization of Laccase on Nanoporous Gold: Comparative Studies on the Immobilization Strategies and the Particle Size Effects. J. Phys. Chem. C 113, 2521–2525. doi:10.1021/jp8090304
Qiu, X., Wang, Y., Xue, Y., Li, W., and Hu, Y. (2020). Laccase Immobilized on Magnetic Nanoparticles Modified by Amino-Functionalized Ionic Liquid via Dialdehyde Starch for Phenolic Compounds Biodegradation. Chem. Eng. J. 391, 123564. doi:10.1016/j.cej.2019.123564
Rai, B., and Shrivastav, A. (2022). “Removal of Emerging Contaminants in Water Treatment by Nanofiltration and Reverse Osmosis,” in Development in Wastewater Treatment Research and Processes. Editors M. Shah, S. Rodriguez-Couto, and J. Biswas (Elsevier), 605–628. doi:10.1016/B978-0-323-85583-9.00026-0
Rajapaksha, A. U., Chen, S. S., Tsang, D. C. W., Zhang, M., Vithanage, M., Mandal, S., et al. (2016). Engineered/designer Biochar for Contaminant Removal/immobilization from Soil and Water: Potential and Implication of Biochar Modification. Chemosphere 148, 276–291. doi:10.1016/j.chemosphere.2016.01.043
Ramesha, G. K., and Sampath, S. (2009). Electrochemical Reduction of Oriented Graphene Oxide Films: An In Situ Raman Spectroelectrochemical Study. J. Phys. Chem. C 113, 7985–7989. doi:10.1021/jp811377n
Ramírez-Montoya, L. A., Hernández-Montoya, V., Montes-Morán, M. A., and Cervantes, F. J. (2015). Correlation Between Mesopore Volume of Carbon Supports and the Immobilization of Laccase from Trametes versicolor for the Decolorization of Acid Orange 7. J. Environ. Manage. 162, 206–214. doi:10.1016/j.jenvman.2015.07.035
Rathi, B. S., Kumar, P. S., and Show, P.-L. (2021). A Review on Effective Removal of Emerging Contaminants from Aquatic Systems: Current Trends and Scope for Further Research. J. Hazard. Mater. 409, 124413. doi:10.1016/j.jhazmat.2020.124413
Rekuć, A., Kruczkiewicz, P., Jastrzembska, B., Liesiene, J., Peczyńska-Czoch, W., and Bryjak, J. (2008). Laccase Immobilization on the Tailored Cellulose-Based Granocel Carriers. Int. J. Biol. Macromolecules 42, 208–215. doi:10.1016/j.ijbiomac.2007.09.014
Rivera-Hoyos, C. M., Morales-Álvarez, E. D., Poutou-Piñales, R. A., Pedroza-Rodríguez, A. M., RodrÍguez-Vázquez, R., and Delgado-Boada, J. M. (2013). Fungal Laccases. Fungal Biol. Rev. 27, 67–82. doi:10.1016/j.fbr.2013.07.001
Rodriguez-Couto, S., Arzac, A., Leal, G. P., and Tomovska, R. (2014). Reduced Graphene Oxide Hydrogels and Xerogels Provide Efficient Platforms for Immobilization and Laccase Production byTrametes Pubescens. Biotechnol. J. 9, 578–584. doi:10.1002/biot.201300474
Rombolà, A. G., Fabbri, D., Meredith, W., Snape, C. E., and Dieguez-Alonso, A. (2016). Molecular Characterization of the Thermally Labile Fraction of Biochar by Hydropyrolysis and Pyrolysis-GC/MS. J. Anal. Appl. Pyrolysis 121, 230–239. doi:10.1016/j.jaap.2016.08.003
Rouhani, S., Azizi, S., Maaza, M., Mamba, B., and Msagati, T. A. M. (2021). Covalent Immobilization of Laccase on Fe3O4-Graphene Oxide Nanocomposite for Biodegradation of Phenolic Compounds. Environ. Protect. Eng. 47, 1. doi:10.37190/epe210107
Rouhani, S., Rostami, A., Salimi, A., and Pourshiani, O. (2018). Graphene oxide/CuFe2O4 Nanocomposite as a Novel Scaffold for the Immobilization of Laccase and its Application as a Recyclable Nanobiocatalyst for the green Synthesis of Arylsulfonyl Benzenediols. Biochem. Eng. J. 133, 1–11. doi:10.1016/j.bej.2018.01.004
Rout, P. R., Zhang, T. C., Bhunia, P., and Surampalli, R. Y. (2021). Treatment Technologies for Emerging Contaminants in Wastewater Treatment Plants: A Review. Sci. Total Environ. 753, 141990. doi:10.1016/j.scitotenv.2020.141990
Rueda-Marquez, J. J., Levchuk, I., Fernández Ibañez, P., and Sillanpää, M. (2020). A Critical Review on Application of Photocatalysis for Toxicity Reduction of Real Wastewaters. J. Clean. Prod. 258, 120694. doi:10.1016/j.jclepro.2020.120694
Ruelle, B., Bittencourt, C., and Dubois, P. (2011). “Surface Treatment of Carbon Nanotubes via Plasma Technology,” in Polymer–Carbon Nanotube Composites Woodhead Publishing Series in Composites Science and Engineering. Editors T. McNally, and P. Pötschke (Sawston, Cambridge: Woodhead Publishing), 25–54. doi:10.1533/9780857091390.1.25
Sabzehmeidani, M. M., Mahnaee, S., Ghaedi, M., Heidari, H., and Roy, V. A. L. (2021). Carbon Based Materials: A Review of Adsorbents for Inorganic and Organic Compounds. Mater. Adv. 2, 598–627. doi:10.1039/d0ma00087f
Samak, N. A., Tan, Y., Sui, K., Xia, T.-T., Wang, K., Guo, C., et al. (2018). CotA Laccase Immobilized on Functionalized Magnetic Graphene Oxide Nano-Sheets for Efficient Biocatalysis. Mol. Catal. 445, 269–278. doi:10.1016/j.mcat.2017.12.004
Santos, J. C. S. D., Barbosa, O., Ortiz, C., Berenguer-Murcia, A., Rodrigues, R. C., and Fernandez-Lafuente, R. (2015). Importance of the Support Properties for Immobilization or Purification of Enzymes. ChemCatChem 7, 2413–2432. doi:10.1002/cctc.201500310
Scaria, J., Gopinath, A., and Nidheesh, P. V. (2021). A Versatile Strategy to Eliminate Emerging Contaminants from the Aqueous Environment: Heterogeneous Fenton Process. J. Clean. Prod. 278, 124014. doi:10.1016/j.jclepro.2020.124014
Schmidt, T. C. (2018). Recent Trends in Water Analysis Triggering Future Monitoring of Organic Micropollutants. Anal. Bioanal. Chem. 410, 3933–3941. doi:10.1007/s00216-018-1015-9
Schneider, W. D. H., Costa, P. C., Fontana, R. C., de Siqueira, F. G., Pinheiro Dillon, A. J., and Camassola, M. (2019). Upscale and Characterization of Lignin-Modifying Enzymes from Marasmiellus Palmivorus VE111 in a Bioreactor under Parameter Optimization and the Effect of Inducers. J. Biotechnol. 295, 1–8. doi:10.1016/j.jbiotec.2019.03.002
Schneider, W. D. H., Fontana, R. C., Baudel, H. M., de Siqueira, F. G., Rencoret, J., Gutiérrez, A., et al. (2020). Lignin Degradation and Detoxification of eucalyptus Wastes by On-Site Manufacturing Fungal Enzymes to Enhance Second-Generation Ethanol Yield. Appl. Energ. 262, 114493. doi:10.1016/j.apenergy.2020.114493
Schoevaart, R., Wolbers, M. W., Golubovic, M., Ottens, M., Kieboom, A. P. G., van Rantwijk, F., et al. (2004). Preparation, Optimization, and Structures of Cross-Linked Enzyme Aggregates (CLEAs). Biotechnol. Bioeng. 87, 754–762. doi:10.1002/bit.20184
Senthivelan, T., Kanagaraj, J., and Panda, R. C. (2016). Recent Trends in Fungal Laccase for Various Industrial Applications: An Eco-Friendly Approach - A Review. Biotechnol. Bioproc. E 21, 19–38. doi:10.1007/s12257-015-0278-7
Shakerian, F., Zhao, J., and Li, S.-P. (2020). Recent Development in the Application of Immobilized Oxidative Enzymes for Bioremediation of Hazardous Micropollutants - A Review. Chemosphere 239, 124716. doi:10.1016/j.chemosphere.2019.124716
Shao, B., Liu, Z., Zeng, G., Liu, Y., Yang, X., Zhou, C., et al. (2019). Immobilization of Laccase on Hollow Mesoporous Carbon Nanospheres: Noteworthy Immobilization, Excellent Stability and Efficacious for Antibiotic Contaminants Removal. J. Hazard. Mater. 362, 318–326. doi:10.1016/j.jhazmat.2018.08.069
Sharifi-Bonab, M., Arjomandi Rad, F., and Talat Mehrabad, J. (2016). Preparation of Laccase-Graphene Oxide Nanosheet/alginate Composite: Application for the Removal of Cetirizine from Aqueous Solution. J. Environ. Chem. Eng. 4, 3013–3020. doi:10.1016/j.jece.2016.06.012
Shen, B., Chen, J., Yue, S., and Li, G. (2015). A Comparative Study of Modified Cotton Biochar and Activated Carbon Based Catalysts in Low Temperature SCR. Fuel 156, 47–53. doi:10.1016/j.fuel.2015.04.027
Shim, T., Yoo, J., Ryu, C., Park, Y.-K., and Jung, J. (2015). Effect of Steam Activation of Biochar Produced from a Giant Miscanthus on Copper Sorption and Toxicity. Bioresour. Tech. 197, 85–90. doi:10.1016/j.biortech.2015.08.055
Shoukat, R., and Khan, M. I. (2021). Carbon Nanotubes: A Review on Properties, Synthesis Methods and Applications in Micro and Nanotechnology. Microsyst. Technol. 27, 4183–4192. doi:10.1007/s00542-021-05211-6
Silva, C. G., Tavares, A. P. M., Dražić, G., Silva, A. M. T., Loureiro, J. M., and Faria, J. L. (2014). Controlling the Surface Chemistry of Multiwalled Carbon Nanotubes for the Production of Highly Efficient and Stable Laccase-Based Biocatalysts. ChemPlusChem 79, 1116–1122. doi:10.1002/cplu.201402054
Silvério, S. C., Moreira, S., Milagres, A. M. F., Macedo, E. A., Teixeira, J. A., and Mussatto, S. I. (2013). Laccase Production by Free and Immobilized Mycelia of Peniophora Cinerea and Trametes versicolor: A Comparative Study. Bioproc. Biosyst. Eng. 36, 365–373. doi:10.1007/s00449-012-0793-2
Singh, B., Singh, B. P., and Cowie, A. L. (2010). Characterisation and Evaluation of Biochars for Their Application as a Soil Amendment. Soil Res. 48, 516. doi:10.1071/SR10058
Sirisha, V. L., Jain, A., and Jain, A. (2016). Enzyme Immobilization: An Overview on Methods, Support Material, and Applications of Immobilized Enzymes. 1st Edn. Elsevier, 179–211. doi:10.1016/bs.afnr.2016.07.004
Skoronski, E., Souza, D. H., Ely, C., Broilo, F., Fernandes, M., Fúrigo, A., et al. (2017). Immobilization of Laccase from Aspergillus oryzae on Graphene Nanosheets. Int. J. Biol. Macromolecules 99, 121–127. doi:10.1016/j.ijbiomac.2017.02.076
Soccol, C. R., Costa, E. S. F. d., Letti, L. A. J., Karp, S. G., Woiciechowski, A. L., and Vandenberghe, L. P. d. S. (2017). Recent Developments and Innovations in Solid State Fermentation. Biotechnol. Res. Innovation 1, 52–71. doi:10.1016/j.biori.2017.01.002
Sohi, S., Lopez-Capel, E., Krull, E., and Bol, R. (2009). Biochar’s Roles in Soil and Climate Change: A Review of Research Needs. CSIRO Land Water Sci. Rep. Ser. 05, 64. doi:10.4225/08/58597219a199a
Sophia, A. C., Lima, E. C., et al. (2008). Removal of Emerging Contaminants from the Environment by Adsorption. Ecotoxicology and Environmental Safety 150, 1–17. doi:10.1016/j.ecoenv.2017.12.026
Soumya, P. S., Lakshmi, M. S. K., and Nambisan, P. (2016). Application of Response Surface Methodology for the Optimization of Laccase Production from Pleurotus ostreatus by Solid State Fermentation on Pineapple Leaf Substrate. J. Scientific Ind. Res. 75, 306–314.
Spokas, K. A., Novak, J. M., Stewart, C. E., Cantrell, K. B., Uchimiya, M., DuSaire, M. G., et al. (2011). Qualitative Analysis of Volatile Organic Compounds on Biochar. Chemosphere 85, 869–882. doi:10.1016/j.chemosphere.2011.06.108
Stankovich, S., Dikin, D. A., Piner, R. D., Kohlhaas, K. A., Kleinhammes, A., Jia, Y., et al. (2007). Synthesis of Graphene-Based Nanosheets via Chemical Reduction of Exfoliated Graphite Oxide. Carbon 45, 1558–1565. doi:10.1016/j.carbon.2007.02.034
Stella Mary, G., Sugumaran, P., Niveditha, S., Ramalakshmi, B., Ravichandran, P., and Seshadri, S. (2016). Production, Characterization and Evaluation of Biochar from Pod (Pisum sativum), Leaf (Brassica oleracea) and Peel (Citrus sinensis) Wastes. Int. J. Recycl Org. Waste Agricult 5, 43–53. doi:10.1007/s40093-016-0116-8
Taheran, M., Naghdi, M., Brar, S. K., Verma, M., and Surampalli, R. Y. (2018). Emerging Contaminants: Here Today, There Tomorrow!. Environ. Nanotechnology, Monit. Manag. 10, 122–126. doi:10.1016/j.enmm.2018.05.010
Tartaj, P. (2011). Sub-100 Nm TiO2mesocrystalline Assemblies with Mesopores: Preparation, Characterization, Enzyme Immobilization and Photocatalytic Properties. Chem. Commun. 47, 256–258. doi:10.1039/c0cc01540g
Taskin, E., Branà, M. T., Altomare, C., and Loffredo, E. (2019a). Biochar and Hydrochar from Waste Biomass Promote the Growth and Enzyme Activity of Soil-Resident Ligninolytic Fungi. Heliyon 5, e02051. doi:10.1016/j.heliyon.2019.e02051
Taskin, E., de Castro Bueno, C., Allegretta, I., Terzano, R., Rosa, A. H., and Loffredo, E. (2019b). Multianalytical Characterization of Biochar and Hydrochar Produced from Waste Biomasses for Environmental and Agricultural Applications. Chemosphere 233, 422–430. doi:10.1016/j.chemosphere.2019.05.204
Tavares, A. P. M., Silva, C. G., Dražić, G., Silva, A. M. T., Loureiro, J. M., and Faria, J. L. (2015). Laccase Immobilization over Multi-Walled Carbon Nanotubes: Kinetic, Thermodynamic and Stability Studies. J. Colloid Interf. Sci. 454, 52–60. doi:10.1016/j.jcis.2015.04.054
Thiyagarajan, P., Selvam, K., Sudhakar, C., and Selvankumar, T. (2020). Enhancement of Adsorption of Magenta Dye by Immobilized Laccase on Functionalized Biosynthesized Activated Carbon Nanotubes. Water Air Soil Pollut. 231, 1. doi:10.1007/s11270-020-04737-1
Tong, Y., McNamara, P. J., and Mayer, B. K. (2019). Adsorption of Organic Micropollutants onto Biochar: A Review of Relevant Kinetics, Mechanisms and Equilibrium. Environ. Sci. Water Res. Technol. 5, 821–838. doi:10.1039/C8EW00938D
Touahar, I. E., Haroune, L., Ba, S., Bellenger, J.-P., and Cabana, H. (2014). Characterization of Combined Cross-Linked Enzyme Aggregates from Laccase, Versatile Peroxidase and Glucose Oxidase, and Their Utilization for the Elimination of Pharmaceuticals. Sci. Total Environ. 481, 90–99. doi:10.1016/j.scitotenv.2014.01.132
Tran, N. H., Reinhard, M., Khan, E., Chen, H., Nguyen, V. T., Li, Y., et al. (2019). Emerging Contaminants in Wastewater, Stormwater Runoff, and Surface Water: Application as Chemical Markers for Diffuse Sources. Sci. Total Environ. 676, 252–267. doi:10.1016/j.scitotenv.2019.04.160
UNESCO (2019). Emerging Pollutants in Water and Wastewater. Available at: www.en.unesco.org, 1–5.
Unuofin, J. O., Okoh, A. I., and Nwodo, U. U. (2019). Aptitude of Oxidative Enzymes for Treatment of Wastewater Pollutants: A Laccase Perspective. Molecules 24, 2064. doi:10.3390/molecules24112064
Valle, J. S., Vandenberghe, L. P. S., Oliveira, A. C. C., Tavares, M. F., Linde, G. A., Colauto, N. B., et al. (2015). Effect of Different Compounds on the Induction of Laccase Production by Agaricus Blazei. Genet. Mol. Res. 14, 15882–15891. doi:10.4238/2015.December.1.40
Valle, J. S., Vandenberghe, L. P. S., Santana, T. T., Almeida, P. H., Pereira, A. M., Linde, G. A., et al. (2014). Optimum Conditions for Inducing Laccase Production in Lentinus Crinitus. Genet. Mol. Res. 13, 8544–8551. doi:10.4238/2014.October.20.31
Valle-Vigón, P., and Fuertes, A. B. (2011). Magnetically Separable Carbon Capsules Loaded with Laccase and Their Application to Dye Degradation. RSC Adv. 1, 1756–1762. doi:10.1039/c1ra00333j
Varsha, M., Senthil Kumar, P., and Senthil Rathi, B. (2022). A Review on Recent Trends in the Removal of Emerging Contaminants from Aquatic Environment Using Low-Cost Adsorbents. Chemosphere 287, 132270. doi:10.1016/j.chemosphere.2021.132270
Vera, M., Fodor, C., Garcia, Y., Pereira, E., Loos, K., and Rivas, B. L. (2020). Multienzymatic Immobilization of Laccases on Polymeric Microspheres: A Strategy to Expand the Maximum Catalytic Efficiency. J. Appl. Polym. Sci. 137, 49562. doi:10.1002/app.49562
Viancelli, A., Michelon, W., Rogovski, P., Cadamuro, R. D., de Souza, E. B., Fongaro, G., et al. (2020). A Review on Alternative Bioprocesses for Removal of Emerging Contaminants. Bioproc. Biosyst. Eng. 43, 2117–2129. doi:10.1007/s00449-020-02410-9
Vrsanska, M., Voberkova, S., Langer, V., Palovcikova, D., Moulick, A., Adam, V., et al. (2016). Induction of Laccase, Lignin Peroxidase and Manganese Peroxidase Activities in White-Rot Fungi Using Copper Complexes. Molecules 21, 1553. doi:10.3390/molecules21111553
Wahab, R. A., Elias, N., Abdullah, F., and Ghoshal, S. K. (2020). On the Taught New Tricks of Enzymes Immobilization: An All-Inclusive Overview. Reactive Funct. Polym. 152, 104613. doi:10.1016/j.reactfunctpolym.2020.104613
Wang, F., Xu, L., Zhao, L., Ding, Z., Ma, H., and Terry, N. (2019). Fungal Laccase Production from Lignocellulosic Agricultural Wastes by Solid-State Fermentation: A Review. Microorganisms 7, 665. doi:10.3390/microorganisms7120665
Wang, J., Xiong, Z., and Kuzyakov, Y. (2016). Biochar Stability in Soil: Meta‐analysis of Decomposition and Priming Effects. GCB Bioenergy 8, 512–523. doi:10.1111/gcbb.12266
Wang, Z., Ren, D., Jiang, S., Yu, H., Cheng, Y., Zhang, S., et al. (2021a). The Study of Laccase Immobilization Optimization and Stability Improvement on CTAB-KOH Modified Biochar. BMC Biotechnol. 21, 47. doi:10.1186/s12896-021-00709-3
Wang, Z., Ren, D., Zhao, Y., Huang, C., Zhang, S., Zhang, X., et al. (2021b). Remediation and Improvement of 2,4-dichlorophenol Contaminated Soil by Biochar-Immobilized Laccase. Environ. Tech. 42, 1679–1692. doi:10.1080/09593330.2019.1677782
Weber, K., and Quicker, P. (2018). Properties of Biochar. Fuel 217, 240–261. doi:10.1016/j.fuel.2017.12.054
Wen, X., Zeng, Z., Du, C., Huang, D., Zeng, G., Xiao, R., et al. (2019). Immobilized Laccase on Bentonite-Derived Mesoporous Materials for Removal of Tetracycline. Chemosphere 222, 865–871. doi:10.1016/j.chemosphere.2019.02.020
Wiberth, C.-C., Casandra, A.-Z. C., Zhiliang, F., and Gabriela, H. (2019). Oxidative Enzymes Activity and Hydrogen Peroxide Production in White-rot Fungi and Soil-Borne Micromycetes Co-cultures. Ann. Microbiol. 69, 171–181. doi:10.1007/s13213-018-1413-4
Xiang, W., Zhang, X., Chen, J., Zou, W., He, F., Hu, X., et al. (2020). Biochar Technology in Wastewater Treatment: A Critical Review. Chemosphere 252, 126539. doi:10.1016/j.chemosphere.2020.126539
Xiang, X., Suo, H., Xu, C., and Hu, Y. (2018). Covalent Immobilization of Lipase onto Chitosan-Mesoporous Silica Hybrid Nanomaterials by Carboxyl Functionalized Ionic Liquids as the Coupling Agent. Colloids Surf. B: Biointerfaces 165, 262–269. doi:10.1016/j.colsurfb.2018.02.033
Xing, X., Han, Y., Jiang, Q., Sun, Y., Wang, X., Qu, G., et al. (2021). Immobilization of Laccases onto Cellulose Nanocrystals Derived from Waste Newspaper: Relationship between Immobilized Laccase Activity and Dialdehyde Content. Cellulose 28 (8), 4793–4805. doi:10.1007/s10570-021-03867-x
Xu, H.-M., Sun, X.-F., Wang, S.-Y., Song, C., and Wang, S.-G. (2018). Development of Laccase/graphene Oxide Membrane for Enhanced Synthetic Dyes Separation and Degradation. Sep. Purif. Tech. 204, 255–260. doi:10.1016/j.seppur.2018.04.036
Xu, L., Sun, K., Wang, F., Zhao, L., Hu, J., Ma, H., et al. (2020). Laccase Production by Trametes versicolor in Solid-State Fermentation Using Tea Residues as Substrate and its Application in Dye Decolorization. J. Environ. Manage. 270, 110904. doi:10.1016/j.jenvman.2020.110904
Xu, R., Tang, R., Zhou, Q., Li, F., and Zhang, B. (2015). Enhancement of Catalytic Activity of Immobilized Laccase for Diclofenac Biodegradation by Carbon Nanotubes. Chem. Eng. J. 262, 88–95. doi:10.1016/j.cej.2014.09.072
Xu, X., Feng, L., Han, Z., Luo, S., Wu, A. m., and Xie, J. (2016). Selection of High Laccase-Producing Coriolopsis Gallica Strain T906: Mutation Breeding, Strain Characterization, and Features of the Extracellular Laccases. J. Microbiol. Biotechnol. 26, 1570–1578. doi:10.4014/jmb.1604.04011
Xu, X., Lu, P., Zhou, Y., Zhao, Z., and Guo, M. (2009). Laccase Immobilized on Methylene Blue Modified Mesoporous Silica MCM-41/PVA. Mater. Sci. Eng. C 29, 2160–2164. doi:10.1016/j.msec.2009.04.019
Yang, J., Wang, G., Ng, T. B., Lin, J., and Ye, X. (2016). Laccase Production and Differential Transcription of Laccase Genes in Cerrena Sp. In Response to Metal Ions, Aromatic Compounds, and Nutrients. Front. Microbiol. 6, 1. doi:10.3389/fmicb.2015.01558
Yang, X., Chen, Y., Yao, S., Qian, J., Guo, H., and Cai, X. (2019). Preparation of Immobilized Lipase on Magnetic Nanoparticles Dialdehyde Starch. Carbohydr. Polym. 218, 324–332. doi:10.1016/j.carbpol.2019.05.012
Yavaşer, R., and Karagözler, A. A. (2021). Laccase Immobilized Polyacrylamide-Alginate Cryogel: A Candidate for Treatment of Effluents. Process Biochem. 101, 137–146. doi:10.1016/j.procbio.2020.11.021
Zdarta, J., Meyer, A., Jesionowski, T., and Pinelo, M. (2018a). A General Overview of Support Materials for Enzyme Immobilization: Characteristics, Properties, Practical Utility. Catalysts 8, 92. doi:10.3390/catal8020092
Zdarta, J., Meyer, A. S., Jesionowski, T., and Pinelo, M. (2018b). Developments in Support Materials for Immobilization of Oxidoreductases: A Comprehensive Review. Adv. Colloid Interf. Sci. 258, 1–20. doi:10.1016/j.cis.2018.07.004
Zerva, A., Simić, S., Topakas, E., and Nikodinovic-Runic, J. (2019). Applications of Microbial Laccases: Patent Review of the Past Decade (2009-2019). Catalysts 9, 1023. doi:10.3390/catal9121023
Zerva, A., Zervakis, G. I., Christakopoulos, P., and Topakas, E. (2017). Degradation of Olive Mill Wastewater by the Induced Extracellular Ligninolytic Enzymes of Two Wood-rot Fungi. J. Environ. Manage. 203, 791–798. doi:10.1016/j.jenvman.2016.02.042
Zhang, C., Gong, L., Mao, Q., Han, P., Lu, X., and Qu, J. (2018a). Laccase Immobilization and Surface Modification of Activated Carbon Fibers by Bio-Inspired Poly-Dopamine. RSC Adv. 8, 14414–14421. doi:10.1039/c8ra01265b
Zhang, C., You, S., Liu, Y., Wang, C., Yan, Q., Qi, W., et al. (2020a). Construction of luffa Sponge-Based Magnetic Carbon Nanocarriers for Laccase Immobilization and its Application in the Removal of Bisphenol A. Bioresour. Tech. 305, 123085. doi:10.1016/j.biortech.2020.123085
Zhang, G., Guo, X., Zhu, Y., Liu, X., Han, Z., Sun, K., et al. (2018b). The Effects of Different Biochars on Microbial Quantity, Microbial Community Shift, Enzyme Activity, and Biodegradation of Polycyclic Aromatic Hydrocarbons in Soil. Geoderma 328, 100–108. doi:10.1016/j.geoderma.2018.05.009
Zhang, H., and Hay, A. G. (2020). Magnetic Biochar Derived from Biosolids via Hydrothermal Carbonization: Enzyme Immobilization, Immobilized-Enzyme Kinetics, Environmental Toxicity. J. Hazard. Mater. 384, 121272. doi:10.1016/j.jhazmat.2019.121272
Zhang, J., Zhang, F., Yang, H., Huang, X., Liu, H., Zhang, J., et al. (2010a). Graphene Oxide as a Matrix for Enzyme Immobilization. Langmuir 26, 6083–6085. doi:10.1021/la904014z
Zhang, W., Yang, Q., Luo, Q., Shi, L., and Meng, S. (2020b). Laccase-Carbon Nanotube Nanocomposites for Enhancing Dyes Removal. J. Clean. Prod. 242, 118425. doi:10.1016/j.jclepro.2019.118425
Zhang, Y., Guo, L., Wei, S., He, Y., Xia, H., Chen, Q., et al. (2010b). Direct Imprinting of Microcircuits on Graphene Oxides Film by Femtosecond Laser Reduction. Nano Today 5, 15–20. doi:10.1016/j.nantod.2009.12.009
Zhang, Y., Hu, P., Muhammad, Y., Tang, Y., Shao, S., Gao, Z., et al. (2021). High-density Immobilization of Laccase on Hollow Nano-Sphere NH2-MIL88(Fe) Host with Interfacial Defects to Improve Enzyme Activity and Stability for Remazol Brilliant Blue R Decolorization. Chem. Eng. J. 405, 127003. doi:10.1016/j.cej.2020.127003
Zhao, L., Chen, W., Wang, L., Sun, H., and Zhu, Z. (2017a). Improvement of Laccase Production by Pleurotus Ostreatus by Means of Agroindustrial Waste and Fermentation Kinetics. Mycosphere 8 (1), 147–161. doi:10.5943/mycosphere/8/1/14
Zhao, S.-X., Ta, N., and Wang, X.-D. (2017b). Effect of Temperature on the Structural and Physicochemical Properties of Biochar with Apple Tree Branches as Feedstock Material. Energies 10, 1293. doi:10.3390/en10091293
Zhou, W., Zhang, W., and Cai, Y. (2021). Laccase Immobilization for Water Purification: A Comprehensive Review. Chem. Eng. J. 403, 126272. doi:10.1016/j.cej.2020.126272
Zhu, C., Bao, G., and Huang, S. (2016). Optimization of Laccase Production in the White-rot fungusPleurotus Ostreatus(ACCC 52857) Induced Through Yeast Extract and Copper. Biotechnol. Biotechnological Equipment 30, 270–276. doi:10.1080/13102818.2015.1135081
Zhu, Y., Murali, S., Stoller, M. D., Velamakanni, A., Piner, R. D., and Ruoff, R. S. (2010). Microwave Assisted Exfoliation and Reduction of Graphite Oxide for Ultracapacitors. Carbon 48, 2118–2122. doi:10.1016/j.carbon.2010.02.001
Keywords: immobilization, biochar, micropolluants, environmental contaminants, laccase
Citation: Adamian Y, Lonappan L, Alokpa K, Agathos SN and Cabana H (2021) Recent Developments in the Immobilization of Laccase on Carbonaceous Supports for Environmental Applications - A Critical Review. Front. Bioeng. Biotechnol. 9:778239. doi: 10.3389/fbioe.2021.778239
Received: 16 September 2021; Accepted: 11 November 2021;
Published: 06 December 2021.
Edited by:
Susana Rodriguez-Couto, LUT University, FinlandReviewed by:
Hafiz M. N. Iqbal, Monterrey Institute of Technology and Higher Education (ITESM), MexicoSanjay Kumar Singh Patel, Konkuk University, South Korea
Copyright © 2021 Adamian, Lonappan, Alokpa, Agathos and Cabana. This is an open-access article distributed under the terms of the Creative Commons Attribution License (CC BY). The use, distribution or reproduction in other forums is permitted, provided the original author(s) and the copyright owner(s) are credited and that the original publication in this journal is cited, in accordance with accepted academic practice. No use, distribution or reproduction is permitted which does not comply with these terms.
*Correspondence: Spiros N. Agathos, c3Bpcm9zLmFnYXRob3NAdWNsb3V2YWluLmJl; Hubert Cabana, aHViZXJ0LmNhYmFuYUB1c2hlcmJyb29rZS5jYQ==