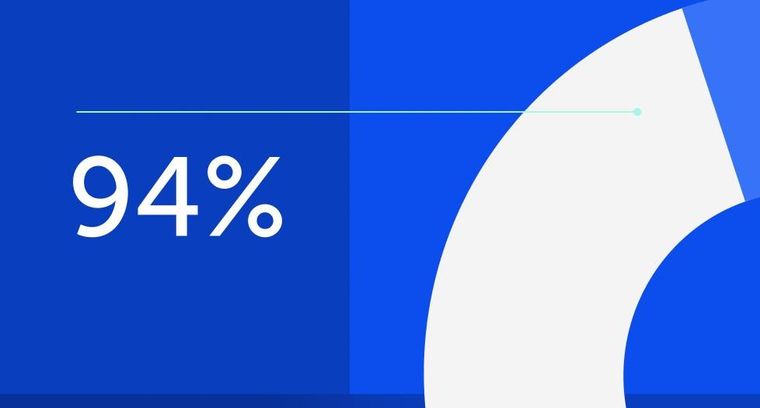
94% of researchers rate our articles as excellent or good
Learn more about the work of our research integrity team to safeguard the quality of each article we publish.
Find out more
ORIGINAL RESEARCH article
Front. Bioeng. Biotechnol., 19 August 2021
Sec. Nanobiotechnology
Volume 9 - 2021 | https://doi.org/10.3389/fbioe.2021.714922
This article is part of the Research TopicOptical Technologies for Disease Diagnosis and Therapy in Deep TissuesView all 8 articles
Quantum dots (QDs) as a promising optical probe have been widely used for in vivo biomedical imaging; especially enormous efforts recently have focused on the potential toxicity of QDs to the human body. The toxicological effects of the representative InP/ZnS QDs as a cadmium-free emitter are still in the early stage and have not been fully unveiled. In this study, the DPPC/DPPG mixed monolayer was used to simulate the lung surfactant monolayer. The InP/ZnS-COOH QDs and InP/ZnS-NH2 QDs were introduced to simulate the lung surfactant membrane’s environment in the presence of InP/ZnS QDs. The effects of InP/ZnS QDs on the surface behavior, elastic modulus, and stability of DPPC/DPPG mixed monolayer were explored by the surface pressure-mean molecular area isotherms and surface pressure-time curves. The images observed by Brewster angle microscope and atomic force microscope showed that the InP/ZnS QDs affected the morphology of the monolayer. The results further demonstrated that the InP/ZnS QDs coated with different surface groups can obviously adjust the mean molecular area, elastic modulus, stability, and microstructure of DPPC/DPPG mixed monolayer. Overall, this work provided useful information for in-depth understanding of the effects of the −COOH or −NH2 group coated InP/ZnS QDs on the surface of lung surfactant membrane, which will help scientists to further study the physiological toxicity of InP/ZnS QDs to lung health.
Quantum dots (QDs) are one kind of the most classical quasi-zero dimensional and semiconductor nanocrystals, which have special optical properties: fluorescence, phosphorescence, and electro-chemiluminescence (Bruchez et al., 1998; Namdari et al., 2017; Pohanka, 2017). They have been used in various areas of human life (Pelley et al., 2009; Matea et al., 2017; Zhao et al., 2018), such as electronic and quantum computing applications, in vitro diagnostics, and in vivo biomedical imaging (Hong et al., 2012; Wang and Yan, 2013; Chen et al., 2014; Zhang et al., 2018). Typically, the cadmium-containing quantum dots as the foremost probe were rapidly developed, but the released cadmium ions can cause dramatic damage to cells and organs (Li et al., 2009; Wang et al., 2016). The InP/ZnS QDs as a core/shell type cadmium-free nanoparticle can effectively replace cadmium-containing quantum dots because the former has relatively lower toxicity (Chibli et al., 2011). In fact, the potential toxicity of the InP/ZnS QDs has not been fully explored since it is still in the early stage.
Soenen et al. (2014) has investigated the toxicity of InP/ZnS QDs on the cells in vitro using three different cell types: primary human umbilical vein endothelial cells (HUVEC), murine neural progenitor cells (C17.2), and rat pheochromocytoma cells (PC12). They found that the InP/ZnS QDs can be ingested efficiently by the three cell types and the uptake of InP/ZnS QDs was mainly dependent on their concentration and surface modification (Soenen et al., 2014). Moreover, the InP/ZnS QDs can be ingested by the epithelial cell line A549 (human lung carcinoma) and the neuronal cell line SH SY5Y (human neuroblastoma) (Brunetti et al., 2013). Chen et al. have investigated the in vitro toxicity of InP/ZnS terminated with different surface groups (−COOH, −NH2, and −OH, respectively) on two lung-derived cell lines, human lung cancer cell HCC-15, and Alveolar epithelial type II (AEII) cell RLE-6TN (Chen et al., 2018). They found that the intake of InP/ZnS-OH QDs in the two types of cells was relatively lower than that of the InP/ZnS-COOH QDs and InP/ZnS-NH2 QDs. However, the uptake mechanism of the InP/ZnS QDs toward cell membrane has been not researched so far.
Lung inhalation is a potential pathway for human exposure to quantum dots, and the lung is the first exposure target for inhaled nanoparticles. When the nanoparticles are inhaled by the human body, they are first exposed to the surface of the alveolar. The uptake of quantum dots by alveolar cells is related to the interaction between quantum dots and cell membrane (Soenen et al., 2014). The study of the effect of quantum dots on the surface behavior of the alveolar membrane is important to understand the mechanism of the uptake of quantum dots by alveolar cells and its effect on lung health.
The alveolar surface is covered with a layer of lipid secretion, called pulmonary surfactants, which is composed of phospholipids (80%), neutral lipid (8–10%), and surfactant associated proteins (10%) (Wang et al., 2018). Phosphatidylcholine (PC) comprises about 80% of total surfactant phospholipid, and dipalmitoylphosphatidylcholine (DPPC) is the most prevalent single compound (40–50% of total PC). Hydroxylated anionic phospholipids, such as phosphatidylglycerol (PG) and phosphatidylinositol (PI), constitute around 10–15% of the total lung surfactant mass (Notter et al., 2020). The pulmonary surfactant monolayer on the alveolar surface is relative to respiration, which can regulate the surface tension of the alveolar surface and promote gas exchange during respiration. The DPPC molecules play a significant role in reducing the surface tension (Veldhuizen and Haagsman, 2000). However, the spreadability of DPPC is poor, which limits the fluidity of the monolayer. The negatively charged phosphatidylglycerols-dipalmitoylphosphatidylglycerol (DPPG), another component of pulmonary surfactant, can enhance the fluidity of pulmonary surfactant and facilitate the interfacial adsorption of phospholipids and the rapid spreading of monolayer (Hallman et al., 1977). The mixed monolayer composed of DPPC and DPPG in the ratio of 4:1 (mol:mol) was widely adopted to mimic the real pulmonary surfactant monolayer (Harishchandra et al., 2009; Sachan et al., 2012; Hu et al., 2020) according to the compositional analysis of mammalian lung surfactant extracts.
In this work, the DPPC/DPPG monolayers at the air-water surface were adopted to mimic the lung surfactant monolayers and the InP/ZnS (−COOH, −NH2) QDs were selected as the target to study the effects of InP/ZnS QDs on the surface behavior of DPPC/DPPG monolayers by a variety of techniques, including surface pressure-mean molecular area and elastic modulus-surface pressure isotherms and surface pressure-time isotherms, as well as Brewster angle microscope and atomic force microscope observations. The effect of InP/ZnS QDs modified by different groups on surface behavior of DPPC/DPPG monolayers was explored. The results will not only give in-depth understanding of the effect of InP/ZnS QDs on surface behavior of DPPC/DPPG monolayers but also be helpful to study the harm of InP/ZnS QDs to lung health.
1,2-Dipalmitoyl-sn-glycero-3-phosphocholine (DPPC: purity ≥99%) and 1,2-dipalmitoyl-sn-glycero-3-phosphoglycerol sodium (DPPG: purity≥99%) were purchased from Avanti Polar Lipids (Alabaster, AL). High purity water was produced by a Milli-Q plus water purification system (18.2 MΩ/cm, Millipore, United States).
The aqueous InP/ZnS-COOH and InP/ZnS-NH2 QDs were obtained from Xi’an Qiyue Biological Technology Co., Ltd. (China). The successful functionalization of the two surface groups was analyzed by FT-IR spectra (IR Spirit, Shimadzu, Japan). The morphology images of the two InP/ZnS QDs were obtained with a transmission electron microscope (TEM) (HT7820, Hitachi, Japan) operating at an accelerating voltage of 100 kV at room temperature. The absorption spectra of InP/ZnS QDs were measured by a UV-Vis spectrophotometer (Cary 5,000, Agilent, United States). The photoluminescence emission spectra were determined by a Fluorescence spectrophotometer (FLS1000, Edinburgh, Britain) with an excitation wavelength of 378 nm.
A Langmuir trough (KSV-Minitrough, Finland) and Wilhelmy-type tensiometer were respectively used to detect the surface behavior of the monolayer at the air-water interface. A filter paper (10 mm × 30 mm × 0.15 mm) as a pressure sensor was used in this work, and the sensor accuracy was 0.01 mN/m. A monolayer at the air-water interface of the trough subphase could be compressed or expanded symmetrically at the desired rate by using two Teflon barriers. All experiments were maintained at a controlled temperature of 35.0 ± 0.5°C which is closer to the physiological temperature of the lung surface.
The DPPC and DPPG with a molar ratio of 4:1 were fully dissolved in chloroform/methanol (9:1, v/v) mixture and the final concentration was about 0.5
To study the effect of InP/ZnS QDs modified by different groups on lipid monolayers, DPPC/DPPG was deposited dropwise on the surface of InP/ZnS QDs aqueous dispersion with the same concentration of 0.2 µg/ml. After 30 min, the monolayers were compressed with a constant rate of 5.25 cm2/min, and each measurement was repeated three times.
The monolayers on pure water or 0.2 µg/ml InP/ZnS QDs aqueous solution were compressed to the target surface pressure (10 and 30 mN/m) with a rate of 5.25 cm2/min; then, the area of the monolayer was kept constant. The surface pressure-time (
Brewster angle microscope (KSV NIMA, Finland) was used to detect the real-time morphology of the monolayer in situ visual, which is equipped with a 50 mW laser emitting p-polarized light at a wavelength of 659 nm. The image resolution is 12 μm. The maximum field of view is 3,000 μm × 4,500 μm.
The Langmuir monolayers were transferred onto the new micas at 10 mN/m and 30 mN/m, forming the Langmuir-Blodgett (LB) films. The dipping rate for transfer was 7.5 mm/s. Their microstructure characterization was observed by atomic force microscope (Shimadzu, Japan) in the contacting mode using a silicon nitride pyramidal tip mounted on a 100 μm long cantilever with a force constant of 0.1 N/m.
The infrared spectra analysis of the InP/ZnS QDs modified with carboxyl and amino groups were performed, indicating successful functionalization of the two surface groups (Figure 1). The appearance of the band at 1,655 cm−1 corresponded to the stretching vibration of C=O for the coated QDs. The strong band at 3,263 cm−1 was assigned to the OH stretching vibration of COOH (Figure A). The characteristic band at 3,405 cm−1 corresponded to the stretching vibration of N-H present in NH2 (Figure B). From Figure 2, the two QDs exhibited consistent absorption spectra with the same absorption peak around 378 nm. Under exciting by 378 nm light source, the two QDs all exhibited relatively symmetrical photoluminescence spectra with the emission peak around 690 nm. The TEM images of the two QDs are shown in Figure 3. It demonstrated a relatively monodispersed size distribution with an average size of ~5 nm.
FIGURE 2. Absorption spectra and photoluminescence spectra of InP/ZnS QDs coated with carboxyl (A) and amino (B) groups.
The
FIGURE 4. Surface pressure-mean molecular area (
According to the data of
where
The elastic modulus-surface pressure (
Compressing the DPPC/DPPG mixed monolayer to the specific surface pressure (10 mN/m and 30 mN/m) on the surface of water without or with the InP/ZnS QDs and keeping the area of the monolayer being constant, the changes of surface pressure with time were recorded for 30 min, which are shown in Figure 5. The surface pressure decreased or increased until the equilibrium value (
FIGURE 5. Surface pressure-time (
At the surface pressure of 10 mN/m (Table 1), the presence of InP/ZnS-COOH QDs caused the
TABLE 1. Relevant characteristic parameters of the DPPC/DPPG mixed monolayer spread on the surface of different subphases.
Further characterization of the effect of InP/ZnS quantum dots on the morphology of the DPPC/DPPG mixed monolayers was performed in real time by using BAM. Figure 6 shows the BAM images for the mixed monolayers at 10 mN/m and 30 mN/m in the absence and presence of InP/ZnS QDs modified by −COOH or −NH2 group. In the BAM images, the bright areas are the regions of monolayer and the dark areas are the surface of subphase water. At 10 mN/m, dark areas were observed in the regions of DPPC/DPPG mixed monolayer spread on the surface of pure water, which corresponded to the LE phase. The larger the dark areas, the less compact the monolayers. When the density of the mixed monolayer regions is small, the mean molecular area is small. In the presence of InP/ZnS QDs modified by the −COOH group, the dark areas were larger, which was consistent with the larger molecular area (122.72
FIGURE 6. The BAM images (2000 μm × 2000 μm) of the mixed DPPC/DPPG (4:1, mol ratio) monolayers spread on the pure water and in the presence of InP/ZnS QDs modified by −COOH or −NH2 group at 10 mN/m and 30 mN/m.
The microstructure information of mixed monolayers was not available by the above BAM images due to the image resolution of 12 μm. So, we transferred the monolayers onto the surface of micas to be films. The microstructure of the monolayer films was observed by an atomic force microscope (Figure 7), and the root mean square roughness (RMS-Rq) and roughness average (Ra) of AFM images were shown in Table 2. At 10 mN/m, when the monolayer was compressed on the pure water, the shape of the microregions in the film looked like “islands” and the island regions were not very tightly distributed. When the monolayer was spread on the water in the presence of InP/ZnS-COOH QDs, the shape of the microregions in the film were shaped like “narrow discontinuous chains” and the “chains” regions were far apart from each other. It can be suggested that the InP/ZnS-COOH QDs decreased the density of the films, which was in agreement with the larger mean molecular area (75.15
FIGURE 7. The AFM images (2 μm × 2 μm) and height analysis of the DPPC/DPPG (4:1, mol ratio) mixed monolayer films transferred onto the micas, which were spread on the pure water and in the presence of InP/ZnS QDs modified with −COOH or −NH2 group at 10 mN/m and 30 mN/m.
FIGURE 8. Schematic diagram of the DPPC/DPPG mixed monolayer at 10 mN/m and 30 mN/m in the absence and presence of InP/ZnS-COOH and InP/ZnS−NH2 QDs.
At 30 mN/m, the structure of DPPC/DPPG mixed monolayer films was more orderly and compact than that at 10 mN/m. When the monolayer was spread on pure water, the microregions of the film formed a “network.” The shape of its mesh was irregular and there was a small nearly circular “island” region inside each mesh. The height of the lipid monolayer was larger than that at 10 mN/m, which is because the hydrophobic tail chain of phospholipid molecules was straightened at 30 mN/m. In the presence of InP/ZnS-COOH QDs, the shape of microregions was irregular and looked like some “islands” that were close to each other. In the presence of InP/ZnS-NH2 QDs, the films were very dense and uniform with some irregular dark pores faintly observed. The effect of these two QDs on the height of the lipid monolayer was similar to that at10 mN/m.
According to the mean molecular areas and AFM analysis of the DPPC/DPPG mixed monolayer, the schematic diagram at the air-water interface, corresponding to the morphology of the monolayer films in AFM images, is in Figure 8, which may help us to understand the effect of InP/ZnS QDs on the distribution of DPPC/DPPG mixed monolayer. In conclusion, it can be suggested that the effect of InP/ZnS QDs on the microstructure of DPPC/DPPG mixed monolayer is different due to the different modified groups and the different surface pressure (or different phase state of the monolayer).
In conclusion, the effects of −COOH and −NH2 groups modified InP/ZnS QDs on the surface behavior and morphology of the DPPC/DPPG (4:1, mol/mol) mixed monolayer at the air-water interface has been systematically studied. The Langmuir experiments suggested the InP/ZnS QDs with different groups had different effects on the mean molecular areas, elastic modulus, phase transition point, and stability of the DPPC/DPPG mixed monolayer. The results from BAM and AFM images indicated the different influences of InP/ZmS QDs on the morphology of mixed monolayer due to QDs’ groups and monolayers’ phase states. The effect of InP/ZnS-NH2 QDs on the
The results of this work help us to understand the effects of fluorescent quantum dots on pulmonary surfactant monolayer on the alveolar surface during respiration. If the fluorescent quantum dots are inhaled into the lungs, especially when they are used in living lung imaging or detection, the expansion behavior and morphology of pulmonary surfactant monolayer may be affected, which may damage the health of the lung. This work has shown that the effects of InP/ZnS QDs modified -carboxyl or -amino on the pulmonary surfactant monolayer are significantly different. The relationship between these different effects and the functional changes of pulmonary surfactant monolayer on the alveolar surface is not clear now, which needs to be further explored. In addition, the adsorption of quantum dots on the pulmonary surfactant monolayer is also worthy of careful study to indicate the adsorption behavior and mechanism of quantum dots on the alveolar surface. Our work in the future will involve the following two points: 1) the adsorption kinetics of quantum dots on the pulmonary surfactant monolayer; 2) the effect of quantum dots on phase behavior and morphology of the pulmonary surfactant monolayer at different temperatures (35~45°C) and pH conditions. More detailed information on the interaction of quantum dots with a pulmonary surfactant monolayer on the alveolar surface during respiration will be obtained, helping us understand the more details close to the real situation.
The original contributions presented in the study are included in the article/supplementary material; further inquiries can be directed to the corresponding authors.
JW: methodology, writing-original draft, writing-review, and editing; JL: data curation; SF: data curation; R-LL: review and editing.
This project was supported by the Scientific research project of the Shaanxi Education Department (no. 20JK0961) from the Shaanxi Provincial Department of Education (the grantee is JW) and the Special Doctoral Fund of Xijing University (XJ17T02) in China.
The authors declare that the research was conducted in the absence of any commercial or financial relationships that could be construed as a potential conflict of interest.
All claims expressed in this article are solely those of the authors and do not necessarily represent those of their affiliated organizations, or those of the publisher, the editors, and the reviewers. Any product that may be evaluated in this article, or claim that may be made by its manufacturer, is not guaranteed or endorsed by the publisher.
Bruchez, M., Moronne, M., Gin, P., Weiss, S., and Alivisatos, A. P. (1998). Semiconductor Nanocrystals as Fluorescent Biological Labels. Science 281, 2013–2016. doi:10.1126/science.281.5385.2013
Brunetti, V., Chibli, H., Fiammengo, R., Galeone, A., Malvindi, M. A., Vecchio, G., et al. (2013). InP/ZnS as a Safer Alternative to CdSe/ZnS Core/shell Quantum Dots: In Vitro and In Vivo Toxicity Assessment. Nanoscale 5, 307–317. doi:10.1039/c2nr33024e
Chen, H., Li, B., Zhang, M., Sun, K., Wang, Y., Peng, K., et al. (2014). Characterization of Tumor-Targeting Ag2S Quantum Dots for Cancer Imaging and Therapy In Vivo. Nanoscale 6, 12580–12590. doi:10.1039/c4nr03613a
Chen, T., Li, L., Xu, G., Wang, X., Wang, J., Chen, Y., et al. (2018). Cytotoxicity of InP/ZnS Quantum Dots with Different Surface Functional Groups toward Two Lung-Derived Cell Lines. Front. Pharmacol. 9, 763. doi:10.3389/fphar.2018.00763
Chibli, H., Carlini, L., Park, S., Dimitrijevic, N. M., and Nadeau, J. L. (2011). Cytotoxicity of InP/ZnS Quantum Dots Related to Reactive Oxygen Species Generation. Nanoscale 3, 2552–2559. doi:10.1039/c1nr10131e
Davies, J. T., and Rideal, E. K. (1963). Interfacial Phenomena. 2nd edition. New York: Academic Press.
Gopal, A., and Lee, K. Y. C. (2006). Headgroup Percolation and Collapse of Condensed Langmuir Monolayers†. J. Phys. Chem. B 110, 22079–22087. doi:10.1021/jp061562t
Gzyl-Malcher, B., Handzlik, J., and Klekowska, E. (2012). Interaction of Prazosin with Model Membranes - A Langmuir Monolayer Study. Bioelectrochemistry 87, 96–103. doi:10.1016/j.bioelechem.2011.12.005
Hallman, M., Feldman, B. H., Kirkpatrick, E., and Gluck, L. (1977). Absence of Phosphatidylglycerol (PG) in Respiratory Distress Syndrome in the Newborn. Pediatr. Res. 11, 714–720. doi:10.1203/00006450-197706000-00003
Harishchandra, R. K., Saleem, M., and Galla, H. J. (2009). Nanoparticle Interaction with Model Lung Surfactant Monolayers. J. R. Soc. Interf. 7, S15–S26. doi:10.1098/rsif.2009.0329.focus
Harishchandra, R. K., Wulff, S., Lentzen, G., Neuhaus, T., and Galla, H.-J. (2010). The Effect of Compatible Solute Ectoines on the Structural Organization of Lipid Monolayer and Bilayer Membranes. Biophysical Chem. 150, 37–46. doi:10.1016/j.bpc.2010.02.007
Hong, G., Robinson, J. T., Zhang, Y., Diao, S., Antaris, A. L., Wang, Q., et al. (2012). In Vivo Fluorescence Imaging with Ag2S Quantum Dots in the Second Near-Infrared Region. Angew. Chem. Int. Ed. 51, 9818–9821. doi:10.1002/anie.201206059
Hu, J., Li, X., Li, M., Shang, Y., He, Y., and Liu, H. (2020). Real-Time Monitoring of the Effect of Carbon Nanoparticles on the Surface Behavior of DPPC/DPPG Langmuir Monolayer. Colloids Surf. B: Biointerfaces 190, 110922. doi:10.1016/j.colsurfb.2020.110922
Li, K. G., Chen, J. T., Bai, S. S., Wen, X., Song, S. Y., Yu, Q., et al. (2009). Intracellular Oxidative Stress and Cadmium Ions Release Induce Cytotoxicity of Unmodified Cadmium Sulfide Quantum Dots. Toxicol. Vitro 23, 1007–1013. doi:10.1016/j.tiv.2009.06.020
Matea, C., Mocan, T., Tabaran, F., Pop, T., Mosteanu, O., Puia, C., et al. (2017). Quantum Dots in Imaging, Drug Delivery and Sensor Applications. Int. J. Nanomed. Nanosurg. 12, 5421–5431. doi:10.2147/ijn.s138624
Nakahara, H., Lee, S., Sugihara, G., Chang, C.-H., and Shibata, O. (2008). Langmuir Monolayer of Artificial Pulmonary Surfactant Mixtures with an Amphiphilic Peptide at the Air/Water Interface: Comparison of New Preparations with Surfacten (Surfactant TA). Langmuir 24, 3370–3379. doi:10.1021/la703180x
Namdari, P., Negahdari, B., and Eatemadi, A. (2017). Synthesis, Properties and Biomedical Applications of Carbon-Based Quantum Dots: An Updated Review. Biomed. Pharmacother. 87, 209–222. doi:10.1016/j.biopha.2016.12.108
Notter, R. H., Chess, P. R., and Pryhuber, G. S. (2020). Lung Surfactant: Overview. Reference Module Biomed. Sci. 1–10. doi:10.1016/B978-0-12-801238-3.11692-7
Panda, A. K., Possmayer, F., Petersen, N. O., Nag, K., and Moulik, S. P. (2005). Physico-Chemical Studies on Mixed Oppositely Charged Surfactants: Their Uses in the Preparation of Surfactant Ion Selective Membrane and Monolayer Behavior at the Air Water Interface. Colloids Surf. A: Physicochemical Eng. Aspects 264, 106–113. doi:10.1016/j.colsurfa.2005.02.011
Pelley, J. L., Daar, A. S., and Saner, M. A. (2009). State of Academic Knowledge on Toxicity and Biological Fate of Quantum Dots. Toxicol. Sci. 112, 276–296. doi:10.1093/toxsci/kfp188
Pohanka, M. (2017). Quantum Dots in the Therapy: Current Trends and Perspectives. Mini. Rev. Med. Chem. 17, 650–656. doi:10.2174/1389557517666170120153342
Sachan, A. K., Harishchandra, R. K., Bantz, C., Maskos, M., Reichelt, R., and Galla, H.-J. (2012). High-Resolution Investigation of Nanoparticle Interaction with a Model Pulmonary Surfactant Monolayer. ACS Nano 6, 1677–1687. doi:10.1021/nn204657n
Soenen, S. J., Manshian, B. B., Aubert, T., Himmelreich, U., Demeester, J., De Smedt, S. C., et al. (2014). Cytotoxicity of Cadmium-Free Quantum Dots and Their Use in Cell Bioimaging. Chem. Res. Toxicol. 27, 1050–1059. doi:10.1021/tx5000975
Song, C. S., Ye, R. Q., and Mu, B. Z. (2007). Molecular Behavior of a Microbial Lipopeptide Monolayer at the Air–Water Interface. Colloids Surf. A: Physicochemical Eng. Aspects 302 (1-3), 82–87. doi:10.1016/j.colsurfa.2007.01.055
Veldhuizen, E. J. A., and Haagsman, H. P. (2000). Role of Pulmonary Surfactant Components in Surface Film Formation and Dynamics. Biochim. Biophys. Acta (Bba) - Biomembranes 1467, 255–270. doi:10.1016/s0005-2736(00)00256-x
Wang, J., Ma, Y., and Hou, S. (2020). Effect of Potassium Ions at the Different Concentration on the Interaction Between AmB and the Lipid Monolayer Containing Cholesterol or Ergosterol. Biochem. Biophysical Res. Commun. 521 (3), 699–705. doi:10.1016/j.bbrc.2019.10.166
Wang, M., Wang, J., Sun, H., Han, S., Feng, S., Shi, L., et al. (2016). Time-Dependent Toxicity of Cadmium telluride Quantum Dots on Liver and Kidneys in Mice: Histopathological Changes with Elevated Free Cadmium Ions and Hydroxyl Radicals. Int. J. Nanomedicine 11, 2319–2328. doi:10.2147/IJN.S103489
Wang, R., Guo, Y., Liu, H., Chen, Y., Shang, Y., and Liu, H. (2018). The Effect of Chitin Nanoparticles on Surface Behavior of DPPC/DPPG Langmuir Monolayers. J. Colloid Interf. Sci. 519, 186–193. doi:10.1016/j.jcis.2018.02.021
Wang, Y., and Yan, X.-P. (2013). Fabrication of Vascular Endothelial Growth Factor Antibody Bioconjugated Ultrasmall Near-Infrared Fluorescent Ag2S Quantum Dots for Targeted Cancer Imaging In Vivo. Chem. Commun. 49, 3324–3326. doi:10.1039/c3cc41141a
Zhang, Y., Zhao, N., Qin, Y., Wu, F., Xu, Z., Lan, T., et al. (2018). Affibody-Functionalized Ag2S Quantum Dots for Photoacoustic Imaging of Epidermal Growth Factor Receptor Overexpressed Tumors. Nanoscale 10, 16581–16590. doi:10.1039/c8nr02556h
Keywords: pulmonary surfactant monolayer, elastic modulus, Brewster angle microscope, InP/ZnS QDs, carboxyl and amino groups
Citation: Wang J, Feng S, Liu J and Liu R-L (2021) Effects of Carboxyl or Amino Group Modified InP/ZnS Nanoparticles Toward Simulated Lung Surfactant Membrane. Front. Bioeng. Biotechnol. 9:714922. doi: 10.3389/fbioe.2021.714922
Received: 26 May 2021; Accepted: 19 July 2021;
Published: 19 August 2021.
Edited by:
Sílvia Pujals, Institute for Bioengineering of Catalonia (IBEC), SpainReviewed by:
Ravindra Pratap Singh, Indira Gandhi National Tribal University, IndiaCopyright © 2021 Wang, Feng, Liu and Liu. This is an open-access article distributed under the terms of the Creative Commons Attribution License (CC BY). The use, distribution or reproduction in other forums is permitted, provided the original author(s) and the copyright owner(s) are credited and that the original publication in this journal is cited, in accordance with accepted academic practice. No use, distribution or reproduction is permitted which does not comply with these terms.
*Correspondence: Juan Wang, d2piaW9waHlzaWNzQHllYWgubmV0; Rui-Lin Liu, bHJseGp0dTE5ODdAeGp0dS5lZHUuY24=
Disclaimer: All claims expressed in this article are solely those of the authors and do not necessarily represent those of their affiliated organizations, or those of the publisher, the editors and the reviewers. Any product that may be evaluated in this article or claim that may be made by its manufacturer is not guaranteed or endorsed by the publisher.
Research integrity at Frontiers
Learn more about the work of our research integrity team to safeguard the quality of each article we publish.