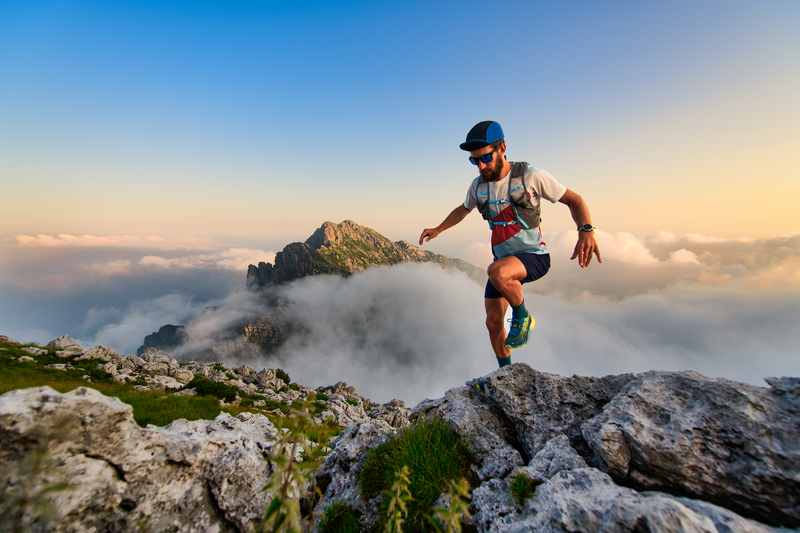
95% of researchers rate our articles as excellent or good
Learn more about the work of our research integrity team to safeguard the quality of each article we publish.
Find out more
REVIEW article
Front. Bioeng. Biotechnol. , 06 August 2021
Sec. Tissue Engineering and Regenerative Medicine
Volume 9 - 2021 | https://doi.org/10.3389/fbioe.2021.704048
This article is part of the Research Topic Advances in Additive Manufacturing Technologies for the Production of Tissue-Engineered Bone Scaffolds for Dental Applications View all 13 articles
Additive manufacturing (AM) is the automated production of three-dimensional (3D) structures through successive layer-by-layer deposition of materials directed by computer-aided-design (CAD) software. While current clinical procedures that aim to reconstruct hard and soft tissue defects resulting from periodontal disease, congenital or acquired pathology, and maxillofacial trauma often utilize mass-produced biomaterials created for a variety of surgical indications, AM represents a paradigm shift in manufacturing at the individual patient level. Computer-aided systems employ algorithms to design customized, image-based scaffolds with high external shape complexity and spatial patterning of internal architecture guided by topology optimization. 3D bioprinting and surface modification techniques further enhance scaffold functionalization and osteogenic potential through the incorporation of viable cells, bioactive molecules, biomimetic materials and vectors for transgene expression within the layered architecture. These computational design features enable fabrication of tissue engineering constructs with highly tailored mechanical, structural, and biochemical properties for bone. This review examines key properties of scaffold design, bioresorbable bone scaffolds produced by AM processes, and clinical applications of these regenerative technologies. AM is transforming the field of personalized dental medicine and has great potential to improve regenerative outcomes in patient care.
Hard tissue deficiencies in the maxillofacial region are the result of numerous diseases, disorder and injuries, and appropriate rehabilitative therapies are necessary to restore quality-of-life for affected individuals. The Global Burden of Diseases, Injuries, and Risk Factors Study 2017 (GBD 2017) revealed that oral disorders had the greatest age-standardized prevalence and incidence in the world (Spencer et al., 2018). Periodontal disease is a significant contributor to oral disease burden; in 2017, the reported global prevalence was 796 million and the percentage change in age-standardized rates for this high impact disease has continued to increase (Spencer et al., 2018). Periodontitis is a chronic, multifactorial inflammatory disease associated with host-microbiome dysbiosis (Papapanou et al., 2018). The disease pathogenesis involves a complex, immunoinflammatory response, modulated by individual microbial, environmental, and genetic factors (Kornman, 2008). Further, periodontal disease is strongly interrelated with overall health, as evidenced by the vast number of oral manifestations in systemic diseases (Kornman et al., 2017; Albandar et al., 2018). Consequences of periodontitis include progressive deterioration of the periodontal attachment apparatus and alveolar bone, ultimately resulting in tooth loss and oral dysfunction (Page and Kornman, 1997; Pihlstrom et al., 2005). The disease may be further characterized by continuous progression, intermittent periods of disease activity (Goodson et al., 1982), or an “asynchronous multiple burst” model (Socransky et al., 1984), to which older adults are more susceptible (Page and Kornman, 1997; Marcenes et al., 2013).
Similar to trends for periodontal disease, incidence rates for cancers of the lip and oral cavity are also increasing (Spencer et al., 2018). Squamous cell carcinoma (SCC) is the leading form of head and neck cancer and the recent surge in prevalence is primarily attributed to oncogenic types of human papillomavirus (HPV) infection (Gillison et al., 2015; Menezes et al., 2021). High level evidence implicates HPV in a quarter of oral cavity cancers and well over half of cases in the oropharynx (Abogunrin et al., 2014; Rodrigo et al., 2014). Malignant tumors involving the oral cavity are often treated by surgical resection, accompanied by other treatment modalities such as radiotherapy, chemotherapy, or immunotherapy. Remission may be attained at the expense of a substantial loss of tissue and large residual bone defects in the maxillofacial region (Muzaffar et al., 2021). Another common cause of hard tissue deficiencies include craniomaxillofacial trauma resulting from motor vehicular collisions, falls, and other accidents (Manodh et al., 2016). According to the GBD 2017, head injuries had a global prevalence and incidence of 47 and 21.6 million, respectively (Spencer et al., 2018). Ultimately, craniomaxillofacial bone defects have a wide range of etiologies including infection, periodontal disease, oral cancer, tooth extraction or tooth loss, and trauma (Bodic et al., 2005). These bone deficiencies can detrimentally affect facial esthetics and important oral functions such as mastication, speech, and nutrition, thereby significantly impairing patient quality-of-life.
The regeneration of periodontal defects in humans is case-sensitive due to the involvement of multiple tissue types and variability in defect morphology (Kao et al., 2015; Yu et al., 2019). For instance, a single defect in the periodontium may consist of all four of its major anatomical components: the gingiva, cementum, periodontal ligament (PDL), and alveolar bone (Smith et al., 2015). The regeneration of these tissues and their unique interfaces is necessary to restore full function as a supportive structure for the teeth (Melcher, 1976). Generally, vertical intrabony defects progress more rapidly than horizontal defects and are at an increased risk for tooth loss (Papapanou and Wennstrom, 1991). Moreover, a residual probing pocket depth (PPD) ≥ 7 mm after periodontal treatment represents risk for tooth loss at a 64.2 odds ratio compared to a PPD of ≤3 mm (Matuliene et al., 2008). In turn, tooth loss initiates anatomic remodeling processes which precede the formation of localized deficiencies in alveolar bone (Araujo et al., 2005). Considering the dramatic decrease in prognosis associated with defect progression and imminent ridge resorption after tooth loss, periodontal defects require timely intervention in order to maintain teeth and their associated bone volume.
The prognosis of regenerative periodontal therapy is dictated by the defect morphology, which primarily considers the number of remaining bone walls and the defect angle (Klein et al., 2001; Reynolds et al., 2015). 3-wall intrabony defects and class II furcations are well-contained spaces that offer the most predictable indications for periodontal regeneration. Defects with fewer bony walls or wider angles tend to be more difficult to treat and the results are often unpredictable (Klein et al., 2001; Reynolds et al., 2015). Other factors that decrease prognosis include an unfavorable vertical sub-classification of furcation involvement, root proximity and root concavities (Aichelmann-Reidy et al., 2015; Tonetti et al., 2017). Following complete debridement to reduce bacterial load and remove granulomatous tissue, periodontal regeneration can be achieved with or without biologics. In dental regenerative medicine, the most commonly used biologics are enamel matrix derivative (EMD) (Hammarstrom et al., 1997; Tsai et al., 2020) and recombinant human platelet-derived growth factor (rhPDGF-BB) (Nevins et al., 2003). rhPDGF-BB has demonstrated acceleration of clinical attachment level (CAL) gain and improved bone fill in the reconstruction of periodontal defects (Nevins et al., 2005; Nevins et al., 2013; Tavelli et al., 2021b). Recently, the second-generation platelet concentrate, platelet-rich fibrin (PRF), has attracted widespread attention for its regenerative potential in soft tissues, however, influence on bone healing and periodontal regeneration is not well established (Tsai et al., 2020). Also of note, clinical trials using fibroblast growth factor-2 (FGF2) have demonstrated promising results for the regeneration of periodontal defects (Cochran et al., 2016; Kitamura et al., 2016) and this biologic currently has approval for use in Japan.
The key elements of periodontal regeneration are cells, scaffolds, growth factors, and blood supply (Larsson et al., 2016). Improved knowledge of how these components interact to promote periodontal tissue formation (Figure 1), accompanied by the advancement of microsurgical techniques and modern biomaterials, has led to the development of minimally invasive treatment approaches with improved clinical outcomes (Cortellini and Tonetti, 2011; Cortellini, 2012; Schincaglia et al., 2015; Moreno Rodriguez et al., 2019; Aslan et al., 2020; Barbato et al., 2020). While clinical standards for regeneration are usually well-achieved, true, histologic periodontal regeneration, involving formation of new cementum, PDL, and alveolar bone, remains elusive and instead, periodontal repair is often observed (Sculean et al., 2008). Animal studies have revealed that conventional guided tissue regeneration (GTR) results in long junctional epithelium and connective tissue (Sculean et al., 2015a), rather than an anatomic, periodontal attachment apparatus. In humans, EMD application paired with the coronally advanced flap (CAF) technique promoted new bone and cementum formation in the apical region of Miller class I and II (Miller, 1985) gingival recession defects (McGuire et al., 2016). Overall, periodontal regeneration requires technical surgeries and judicious, decision-making strategies to adapt a broad range of biomaterials, either in combination or alone, to achieve desired biologic and clinical results (Tavelli et al., 2020).
Figure 1. Principles and current endeavors for periodontal regeneration with tissue bioengineering. (A) Key components of periodontal regeneration with tissue engineering. Cells, growth factors, scaffold, mechanical loading, pathogen control, and ideal blood supply are the key for periodontal regeneration. (B) Examples of micropatterned scaffold, which enhances the orientation of fiber in periodontal regeneration. Left panel: SEM image of a micropatterned scaffold with grooves. Center: Viral Gene delivery (Ad-BMP-7) with chemical vapor deposition. Right: human PDL cells aligned along with the grooves of micropattern. (C) Left: prospective sources of stem cells in dental and maxillofacial region. BMSCs, bone marrow-derived mesenchymal stem cells from orofacial bone; DPSCs, dental pulp stem cells; SHED, stem cells from human exfoliated deciduous teeth; PDLSCs, periodontal ligament stem cells; DFSCs, dental follicle stem cells; TGPCs, tooth germ progenitor cells; SCAP, stem cells from the apical papilla; OESCs, oral epithelial progenitor/stem cells; GMSCs, gingiva-derived MSCs; PSCs, periosteum-derived stem cells; SGSCs, salivary gland-derived stem cells. Right: autologous PDL-derived a three-layered cell sheet with woven PGA. Adapted with permission from Egusa et al. (2012), Iwata et al. (2018), Pilipchuk et al. (2018), and Yu et al. (2019).
Dental implant therapy is often the treatment of choice to replace missing teeth, offering patients high satisfaction and improved oral health-related quality-of-life following treatment (Feine et al., 2018). The suitability of an edentulous site for implant placement is contingent on a sufficient, available bone volume (Avila-Ortiz et al., 2014). With advanced computer-aided design (CAD), virtual planning of the restorative position can accurately guide preoperative assessments of the residual ridge. Alveolar ridge augmentation with hard and soft tissue is frequently required to support a functional and esthetic result. In larger defects, guided bone regeneration (GBR) using barrier membranes and bone grafts may be performed, followed by implant placement and peri-implant soft tissue phenotype modification, if indicated (Tavelli et al., 2021a). Mounting evidence supports the augmentation of the peri-implant soft tissue volume and keratinized mucosa width to promote peri-implant health and stability of the marginal bone level (Giannobile et al., 2018; Longoni et al., 2019; Tavelli et al., 2021a). Autologous grafts remain the most effective treatment for soft tissue augmentation (Zucchelli et al., 2020). However, xenografts may offer similar clinical results with improved patient-reported outcomes in terms of pain and satisfaction (McGuire et al., 2020). With regards to hard tissue augmentation, autogenous bone grafts impart osteogenic influence and are often considered as the “Gold Standard” for regeneration (Al-Moraissi et al., 2020). Their disadvantage is that large quantities of graft material necessitate a secondary surgical site, such as the mandibular ramus or symphysis, in which donor-site morbidity and limited available bone volume for harvest are important considerations (Sculean et al., 2015b). As GBR requires substantial amounts of bone graft material compared to periodontal defects, a mixture of autograft and xenograft is commonly used (Urban et al., 2011, 2013).
Alveolar ridge deficiencies are categorized by their severity and defect type, generally described as horizontal, vertical, or combined (Seibert, 1983; Allen et al., 1985; Seibert and Salama, 1996; Wang and Al-Shammari, 2002). More severe and combined defects may require multiple surgical procedures for augmentation and are difficult to regenerate. Reported survival rates of dental implants placed in resultant bone from GBR procedures is comparable to rates in native bone (Jensen and Terheyden, 2009; Clementini et al., 2012). According to a recent systematic review, weighted means of clinical vertical bone gain were 8.04 mm for distraction osteogenesis, 4.18 mm for GBR, and 3.46 mm for bone block grafts, and post-operative complication rates were 47.3, 12.1, and 23.9%, respectively (Urban et al., 2019). GBR is technique-sensitive as surgical success relies upon adequate flap release to achieve primary closure and proper membrane application to prevent ingrowth of connective tissues into the bone compartment (Eskan et al., 2017; Soldatos et al., 2017). Non-resorbable membrane exposure, which is the predominant post-operative complication, occurs at rates of 13.8% in horizontal augmentation and 18% in vertical augmentation (Jensen and Terheyden, 2009). The development of dense, polytetrafluoroethylene (PTFE) membranes has enabled ridge preservation without primary closure, facilitating comparable results to GBR using e-PTFE membranes while reducing complications (Urban et al., 2019).
The clinical limitations, implications of invasive reconstructive surgical procedures, and prognostic uncertainty are current challenges in regenerative dental medicine. Clinical scenarios in which predictable treatments have yet to be achieved include ridge defects with severe horizontal or vertical components of alveolar bone loss, class III furcations, papilla deficiencies, and advanced peri-implant defects (McGuire and Scheyer, 2007; Reynolds et al., 2015; Monje et al., 2019). Additionally, few clinical strategies emphasize bone regeneration in the craniofacial complex. Defects in the calvaria, facial bones, and temporomandibular joint (TMJ) are often reconstructed with customized metal plates and implants with varying degrees of success. However, anatomic regeneration of functional craniomaxillofacial bone structures has yet to be achieved (Zhang and Yelick, 2018). Current regenerative biomaterials for bone commonly present issues related to early resorption or persistence, and limited capacity to reconstruct large or uncontained defects (Giannobile et al., 2019; Tao et al., 2019). Since 2000, regenerative medicine research, mainly in the field of bioengineering, has made significant progress. A broad range of research has been conducted using stem cells (Kaigler et al., 2013, 2015; Iwata et al., 2018; Xuan et al., 2018; Park J. Y. et al., 2020; Sanchez et al., 2020), gene delivery (Jin et al., 2003; Dunn et al., 2005; Chang et al., 2009, 2010; Sugano et al., 2014; Zhang Y. et al., 2015), surface modification with microstructures (Pilipchuk et al., 2016; Zhang Z. et al., 2016; Pilipchuk et al., 2018), three-dimensional (3D) bioprinting (Rasperini et al., 2015; Raveendran et al., 2019), and whole tooth regeneration (Kim et al., 2010; Oshima et al., 2011, 2017; Oshima and Tsuji, 2015). Additionally, clinical trials of microstructure-applied scaffolds (Rasperini et al., 2015; Raveendran et al., 2019) and PDL-derived cell sheets or PDL-derived mesenchymal stem cells (MSCs) have been conducted in humans (Iwata et al., 2018; Sanchez et al., 2020). The clinical regeneration of oral, dental, and craniofacial structures has advanced tremendously in recent years but there are still considerable needs for improving the customization of scaffolds to complex architectures to gain more predictable outcomes. Usage of modern scaffold fabrication techniques in coordination with biologic agents and novel cellular and molecular therapies are expected to develop the next generation of biomaterials in bone tissue engineering.
Material selection is critical in the design of scaffolds produced by additive manufacturing (AM) techniques. Suitable biomaterials must demonstrate process compatibility with the specific AM technique applied, as well as appropriate biochemical and physical characteristics to function successfully in vivo (Bourell et al., 2017). Although optimal processing parameters vary between the different forms of AM, typical features of a suitable material include buildability of the incrementally deposited layers, adequate densification after chemical or thermal treatments, and structural tolerance of other post-processing steps (Gu, 2015). Certain combinations of materials and AM processes may not facilitate adequate process accuracy, thus detrimentally affecting the consistency of a scaffold’s internal architecture, overall part quality, and reproducibility (Leong et al., 2003). Additionally, techniques involving processing steps that employ high temperatures (Han et al., 2017; Ligon et al., 2017), ultraviolet light irradiation (Bagheri and Jin, 2019), or organic solvents (Mikos and Temenoff, 2000) may preclude the simultaneous incorporation of cells and other biological factors.
AM technology enables scaffold production with a diverse array of materials, including polymers, metals, ceramics, hydrogels, and carbon-based nanomaterials (Guvendiren et al., 2016). Thermoplastic polymers are often used in extrusion-based technologies whereas ceramic, metal, or polymer powders are typically processed at higher temperatures in laser-based methods (Zhang S. et al., 2015; Yang et al., 2019). Recently, biodegradable metal alloys containing magnesium (Mg) or Zinc (Zn) are of increasing interest due to their improved corrosion resistance and biomimicry (Li et al., 2018; Wen et al., 2018; Hernández-Escobar et al., 2019). However, these materials present unique challenges such as high melting points, flammability, and generation of metallic vapors that compromise process stability (Grasso et al., 2018). Numerous synthetic polymers are practical material choices for AM fabrication of biomedical implants due to their high biocompatibility, biodegradability, bioresorption, and processability (Puppi et al., 2010). Polycaprolactone (PCL) is the most commonly used biomaterial in AM due to its excellent mechanical properties, low cost, ease of processability, and low melting point.
While polymers are excellent materials in their ability to accommodate AM processing parameters, singular material groups are limited in their capacity to mutually satisfy requirements for both AM processing and clinical utility in biomedical applications. For instance, polylactides have high tensile strength accompanied by a slow degradation rate, which may persist longer than desirable in vivo. In contrast, although polyglycolic acid (PGA) and poly lactic-co glycolic acid (PLGA) offer superior mechanical properties, they degrade quickly when used as a bioresorbable scaffold and within 2 weeks, their tensile strengthen is reduced by half (Ikada, 2006). To address this obstacle, ceramics are often combined with polymers to form composite materials with improved mechanical characteristics and biologic properties (Nyberg et al., 2017; Zhang et al., 2018).
Bone itself is a composite tissue by nature, consisting of a mineral phase predominated by nanocrystalline hydroxyapatite (HA) and an organic phase, consisting of extracellular matrix proteins, of which approximately 90% is collagen type 1 (Paschalis et al., 2003). The presence of mineralized collagen fibers affords bone both high elasticity and strength to prevent fracture during weight-bearing activities (Nair et al., 2013). In the periodontium, alveolar bone houses the dentition in fibrous joints classified as gomphoses. The tooth-bone interface is mediated by the PDL, a well-vascularized structure constituted by collagenous sheets of extracellular matrix, extending from alveolar bone and embedding into the root cementum (Naveh et al., 2013). Fibers of the PDL exhibit region-specific orientation that participate in physiologic loading, nutrient transport, and bone remodeling (Connizzo et al., 2021). Due to complex organization and composition required for function, multi-material constructs have superior capability to replicate hybrid tissue structures and promote scaffold performance (Jakus and Shah, 2017; Kim et al., 2018).
By mimicking the physiologic characteristics of native bone, material property tailoring enhances the regenerative capacity of tissue engineered constructs in the presence of biomechanical stresses (Palmer et al., 2008). This is especially relevant for dental applications as the bone that comprises the periodontium and jaws is regularly subject to extrinsic forces (Korioth et al., 1992) that result in a physiologic degree of elastic deformation (Daegling et al., 1992; Korioth and Hannam, 1994). Consequently, alveolar bone is anisotropic in nature, meaning that it demonstrates a non-linear, elastic symmetry (Giesen et al., 2001; Peterson et al., 2006). This regional and directional variation in modulus is imparted by the structural orientation of mineralized collagen fibers and aids proper stress distribution (Lettry et al., 2003; Wang and Ural, 2018). The elastic modulus of trabecular and cortical bone have been reported to be in the ranges of 3.5–125.6 MPa (Misch et al., 1999) and 6.9–16.0 GPa, respectively (Dechow et al., 2010). AM techniques can produce versatile scaffolds with mechanical properties within these physiologic ranges for craniofacial and dentoalveolar reconstruction. This has been demonstrated in degradable polymers, calcium phosphate ceramics, and composite ceramic-polymer scaffolds fabricated with both direct and indirect means of solid free-form fabrication (SFF) (Hollister et al., 2005).
The layered construction process utilized in AM is advantageous for the production of lightweight and porous constructs that can support tissue regeneration in an irregular defect. CAD files can be used to generate scaffold configurations that accurately replicate the overall defect shape and dimensions. Further, customized 3D surface topology can be generated by using standard triangle language (STL) files to topologically subtract defects from a digital scaffold design (Park et al., 2012). This promotes anatomical scaffold adaptation to the defect boundaries, minimizing dead space and micromotion (Grottkau et al., 2002). An effective scaffold should also provide sufficient rigidity to sustain matrix deposition until newly formed tissue has developed the mechanical integrity to withstand normal load bearing conditions. Resistance to deformation is largely dictated by material selection, degradation rate, internal geometry and porosity. Cell seeding can further reinforce scaffolds through enhanced extracellular matrix production while also compensating for the gradual decline in structural integrity that accompanies degradation (Spalazzi et al., 2006a). Finally, modulus matching of the scaffold material to bone is essential to prevent disadvantageous mechanoregulation of anatomic remodeling (Sandino and Lacroix, 2011), as well as other adverse sequelae, such as scaffold fragmentation and stress shielding. Stress shielding occurs when an implanted substrate has a higher modulus than the surrounding host bone, creating areas of differential strain distribution on the adjacent tissue (Orr et al., 2001) and resulting in a localized decrease in density of the surrounding bone (Spector, 1994; Van Lenthe et al., 1997).
Mechanical cues provided by scaffold materials can regulate the fate of stem and progenitor cells (Vining and Mooney, 2017). In 2D culture, mechanical properties of the extracellular matrix (ECM) such as stiffness dictate the differentiation of MSCs derived from bone marrow or adipose tissues (Engler et al., 2006). In 3D systems, Huebsch et al. (2010) showed that the elasticity of the substrate or matrix appears to direct MSCs differentiation to the cell fate that best matches the elasticity of the native physiological ECM; stiffer matrix (11–30 kPa) stimulates osteogenic differentiation while softer matrix (2.5–5 kPa) promotes adipogenic or neuronal differentiation. Furthermore, variations in matrix stiffness can regulate MSC behaviors such as cell fate and migration (Tse and Engler, 2011). MSCs may be more responsive to a gradient of stiffness established by tunable levels of crosslinking along a spatial axis in a hydrogel scaffold (Sunyer et al., 2016).
Viscoelasticity, a key property of living tissues, is another regulator of MSC behavior. Viscoelastic materials exhibit a combination of storage of elastic energy as a solid, and loss of mechanical energy as a fluid. These materials exhibit stress relaxation and hysteresis in the stress-strain relationship during loading and unloading. When a mechanical load is applied then removed, viscoelastic materials can dissipate energy (Chaudhuri et al., 2020). Chaudhuri et al. (2016) demonstrated that MSC cell fate and activity is regulated by tuning the stress relaxation of the alginate hydrogel scaffold, independently of the hydrogel’s initial elastic modulus, degradation and cell-adhesion ligand density. More specifically, MSC cell spreading, proliferation, and osteogenic differentiation, and bone matrix production are enhanced when encapsulated in hydrogels with faster stress relaxation. When implanting alginate hydrogels with tunable stress relaxation to deliver human MSCs into rodent calvaria defects, animals receiving fast-relaxing hydrogels showed significantly enhanced new bone growth, extensive matrix remodeling and hydrogel disappearance compared to the group that received slow relaxing, stiffness-matched hydrogels (Darnell et al., 2017).
Mechanistically, the effect of scaffold mechanics is mediated by adhesion-ligand binding via integrin, actin-myosin contractility and activation of mechanosensing and mechanotransduction pathways. For instance, matrix elasticity directed stem cell lineage specification is non-muscle myosin II dependent (Engler et al., 2006). In addition, when stiffness matched, stress relaxation led to increased nuclear translocation of the YAP transcription factor, a key transcription factor mediating mechanotransduction (Chaudhuri et al., 2016). In tissue engineering and regenerative medicine, synthetic matrices with defined mechanical and biophysical properties are useful to guide stem cells ex vivo prior to transplantation, and to tune stem cell behavior in vivo following transplantation in order to improve their regenerative capacity (Huebsch et al., 2015; Darnell et al., 2017; Vining and Mooney, 2017).
AM can be used to produce sophisticated scaffolds with optimized macroscale architecture, internal geometry, and topographical features that enhance the requisite cellular processes for new tissue formation (Figure 2). The precise control of scaffold design afforded by AM techniques is a valuable feature for dental and craniofacial bone applications, as defects in these regions often involve multiple tissues that require complex spatiotemporal regulation for development (Lee et al., 2016). This presents the unique challenge of guiding the differentiation and maintenance of multiple, cellular phenotypes, as well as achieving synthesis of distinct but continuous tissues in a single construct. Triphasic scaffolds consisting of stratified compartments with unique material compositions mimic the organization of native tissues and enable tri-culture of chondrocytes, fibroblasts, and osteoblasts (Spalazzi et al., 2006b). This scaffold architecture efficaciously mediates phase-specific cellular proliferation and phenotypic matrix production (Spalazzi et al., 2006a, 2008).
Figure 2. Key determinants of cell-scaffold interactions. Resorbable scaffolds for the regeneration of functional dental, oral, and craniofacial tissues require tailored, biomimetic features that consider structural design, internal geometry, and surface topography to promote cell-scaffold interactions. Additive manufacturing facilitates optimization of physical properties of scaffold substrates to promote overall mechanical performance and fine tune biomechanical regulation of cell behavior. Intrinsic material properties such as degradation rate and surface chemistry are key biochemical considerations, and various exogenous agents with bioactive properties may be incorporated for scaffold functionalization to further enhance regenerative outcomes.
Modern AM techniques have driven the evolution of hybrid scaffold systems designed for the regeneration of fibrous articulations within the craniofacial complex (Vaquette et al., 2018). 3D printed wax molds have been used to indirectly fabricate polymeric scaffolds with fiber-guiding microchannels to align fibroblasts and their subsequent connective tissue formation in a novel tooth to PDL interface (Park et al., 2010). Fused deposition modeling (FDM) and electrospinning techniques have been combined to produce biphasic periodontal scaffolds with well-integrated compartments for PDL and bone (Vaquette et al., 2012; Costa et al., 2014). Electrospinning methods have also produced functionally graded scaffolds with seamless transition zones (Erisken et al., 2008) and gradients in scaffold features such as pore size (Abbasi et al., 2019; Zhou et al., 2020).
It has been well established that pore characteristics mediate important cell-scaffold interactions that dictate cell morphology, phenotype maintenance, and biosynthetic activity (Nehrer et al., 1997). The recommended pore size for bone scaffolds ranges from 300 to 800 μm (Ishaug et al., 1997; Tsuruga et al., 1997), with the optimal size depending on the selected biomaterial composition and intended regional application of the scaffold. Larger pores are thought to facilitate vascularization, oxygenation, and direct osteogenesis while smaller pores may favor osteochondral ossification (Karageorgiou and Kaplan, 2005). Although the significance of pore size within this range may be minimal (Roosa et al., 2010), it is universally acknowledged that pores less than 100 μm in size prevent cellular infiltration and result in the formation of undesirable, non-mineralized osteoid or fibrous tissue (Hulbert et al., 1970). Further, small pores <125 μm in diameter prevent differentiation of MSCs (Swanson et al., 2021). As long as the selected pore size permits adequate cell migration for tissue ingrowth and osteogenic cell phenotypes, other features affecting fluid conductance (Hui et al., 1996), such as pore interconnectivity and orientation may be of greater influence. While conventional, porogen-leached scaffolds exhibit variable microarchitecture consisting of random interconnections, indirect SFF allows for controlled introduction of porosity, pore interconnectivity, and surface topography at the microscale (Taboas et al., 2003), resulting in superior distribution and quality of mineralized tissue formation in vivo (Park et al., 2012, 2014).
With increasingly advanced AM processes, high resolution features can be incorporated into the internal microarchitecture of a scaffold. Modern image rendering technology can develop biomimetic surface topographies that positively influence osteoblast behavior and local production of osteogenic factors such as osteocalcin (OCN), vascular endothelial growth factor (VEGF), osteoprotegerin (OPG), and bone morphogenetic protein (BMP)-2 (Cheng et al., 2016). Computer-directed deposition can produce micropores with customized orientation and interconnecting channels (Lim et al., 2010; Park et al., 2017). These features improve nutrient and oxygen diffusion throughout larger defect volumes and may also play a role in cell–cell communication. Microscale features such as patterning and surface roughness further enhance cell migration, adhesion, proliferation, and osteogenic differentiation (Zhu et al., 2020). This topographical influence on cell response is derived from effects on surface energy and protein adsorption, interactions that are recapitulated on the submicron (Wang et al., 2015) and nanoscales (Khang et al., 2012). Additionally, nanotopographical features can upregulate expression of genes known to be important for osteoblast adhesion, such as intercellular adhesion molecule 1 (ICAM1), integrin αM (ITGAM), integrin α1 (ITGA1) (Dalby et al., 2007), integrin α5 (ITGA5) and integrin β1 (ITGB1) (Liu et al., 2017).
Regenerative medicine is based upon the manipulation of known physiologic processes to create a microenvironment that simulates a desired stage of tissue development, thus inducing tissue formation and renewal. Not only must tissue dynamics be replicated at a macroscopic organ or tissue scale, but on the cellular and molecular levels as well. Scaffolds primarily serve to provide an osteoconductive matrix and benefit from the addition of growth factors that exert osteoinductive influence on cellular activity. Biologics such as recombinant human BMP-2 and BMP-7, growth differential factor-5 (GDF-5), EMD, and rhPDGF-BB, have all been well-studied for their capacities to promote osteogenic differentiation and enhance bone formation in regenerative dental medicine (Suárez-López Del Amo et al., 2015). Growth factor delivery strategies frequently take advantage of bioresorbable, polymer-based scaffolds as a carrier (Schliephake, 2010). The simplest method is scaffold immersion in a growth factor solution. However, drawbacks of physical adsorption include an initial burst release in the first 24 h followed by rapid attenuation (Caballé-Serrano et al., 2019). Post-processing, polyelectrolyte nanolayer coatings can deliver physiologically relevant quantities of active biologics with tunable release, however, this modification reduces pore area (Wei et al., 2007; Jin et al., 2008; Shah et al., 2014). Common strategies to simultaneously incorporate growth factors during the AM process include physical entrapment, which involves direct-loading of growth factor solution into structural reservoirs with a multi-head deposition system, or pre-loading, using a growth factor loaded paste as raw material in extrusion-based printing. In a study that compared these approaches, direct-loading exhibited similar issues to adsorption, in that it had diminished capability for sustained growth factor release (Huang et al., 2018).
Limitations in conventional growth factor delivery may be mitigated by the use of gene-activated scaffolds, in which a scaffold is utilized as a gene delivery device to facilitate controlled gene transduction upon implantation (Fang et al., 1996; Jin et al., 2004). Common methods of vector-based gene delivery may utilize peptides, viruses (adenovirus, baculovirus, or lentivirus), or non-viral vectors to deliver genes that induce expression of growth factors (Yan et al., 2019). Gene therapy has been further enhanced with treatments such as chemical vapor deposition (CVD) polymerization (Lahann et al., 2001), in which antibodies conjugated to adenoviral vectors for transgene expression (Hao et al., 2016) are immobilized onto a scaffold surface. This delivery mechanism allows for multi-growth factor gene expression to promote regenerative activities in target cells, as previously demonstrated with rhPDGF-BB and BMP-7 in human PDL fibroblasts (Hao et al., 2016). The same CVD-mediated, adenoviral vector treatment using adPDGF-BB and adBMP-7 was assessed in micropatterned, biphasic PLGA/PCL scaffolds implanted into alveolar bone defects in vivo (Pilipchuk et al., 2018). The results confirmed the ability to control the localization of multiple growth factors within a single scaffold construct to improve the formation and quality of regenerated periodontal tissues. Gene expression may be further altered by leveraging the epigenetic capabilities of microRNA (miRNA). MiRNA are small non-coding RNAs that regulate post-transcriptional modifications of a target messenger RNA and can inhibit translation of multiple genes by sequence-pairing homology (Larsson et al., 2015). Epigenetic functionalization of scaffolds to impart anti-inflammatory, immunomodulatory, or osteogenic influence may be achieved through incorporation of miRNA-transfected cells or direct loading of miRNA into the biomaterials (Asa’ad et al., 2020).
Finally, AM offers great capability to produce bioresorbable, scaffold-based drug delivery systems, incorporating pharmacologic agents that confer antimicrobial properties or other therapeutic effects. New bone formation occurs slowly over a period of several months and the scaffold material must persist for a relatively long duration of time. Biofilm colonization, localized tissue infection, and chronic inflammatory processes pose serious risks to the final regenerative outcome. Scaffolds produced by AM processes may address these concerns through the addition of antibiotics and corticosteroids, while enhancing the regenerative outcome. A 0.5 mg/ml concentration of doxycycline has been demonstrated to promote osteoblast differentiation in vitro (Almazin et al., 2009) and other research has identified anti-osteoclastic (Bettany et al., 2000) and anti-collagenase (Holmes et al., 2004) activity. It should be noted that pre-loading methods involving high temperatures can significantly reduce the efficacy of specific types of antibiotic compounds (Shim et al., 2015). Additionally, controlled release is essential, otherwise excessive dosages of antibiotics will confer cytotoxic effects on osteogenic cell populations (Feng et al., 2010; Park, 2011). Use of nanocoatings or nanofiber delivery mechanisms (Han et al., 2017; Li Z. et al., 2020) to convey antimicrobial properties should be further explored (Kumar et al., 2020). Scaffold modifications with anti-fouling, zwitterionic polymer coatings (Chen et al., 2019) and antimicrobial peptides (Liang et al., 2021) represent promising alternative strategies to discourage biofilm formation or microbial infections.
AM is a layer-by-layer construction process used to create 3D constructs with CAD and computer-aided manufacturing (CAM) technology. It is often used interchangeably with SFF, which also implies the use of a fixture-less platform without part-specific tooling or human intervention, or rapid prototyping (RP), often used in contexts that describe fast fabrication of scale models or parts. AM processes first emerged in the 1980’s and have rapidly evolved to become a powerful tool for biomedical scaffold design, capturing particular interest from the research community in bone tissue engineering. Research involving bone-related applications account for approximately 20% of the existing publications in searches for articles with the terms “additive manufacturing” and “3D printing.”
AM fabrication of personalized biomedical constructs begins with the acquisition of high resolution, 3D datasets, typically with computed tomography (CT) or magnetic resonance imaging (MRI), from an individual patient. The images are then converted to a common medical file format referred to as digital imaging and communication in medicine (DICOM). The DICOM file is then imported into a software package that performs segmentation to produce serial sectional data (slices) and reconstruction of a volumetric model composed of voxels. A voxel is a single unit of volume within a 3D grid as opposed to a 2D pixel. A density threshold is then established and the signal intensity of point data is used to determine which points will be included. Surface polygons are then extracted to reconstruct a tessellated, polyhedral model, also known as a mesh which can be exported as an STL file. Surface refinement is performed with algorithms such as non-uniform rational B-spline (NURBS) functions; this can occur either before the creation of the STL-triangulated surface, known as reverse modeling or after, which is the STL-triangulated model converting approach (Sun et al., 2005). With these steps, a CAD-based solid model is available for further optimization and digital manipulation.
Dentistry is a field that has embraced AM techniques and frequently uses commercially available equipment to fabricate patient-specific constructs in everyday practice. AM is considered a technologic hallmark of the 4th industrial revolution and has resulted in a paradigm shift from design for manufacturing to manufacturing for design (Figure 3). In the next decade, AM is expected to drastically reduce the utilization of conventional manufacturing techniques and consequently transform employment dynamics in numerous industries (Pérez-Pérez et al., 2018). AM will give rise to new fields and technical occupations, as it has already done with the advent of computer-aided tissue engineering (Sun et al., 2005), and eliminate tasks that can be performed by automated processes. The future of clinical regenerative procedures will possibly involve biomedical laboratories staffed with bioengineers and computer technicians dedicated to the fabrication of personalized bone scaffolds, similar to the current scenario in which dental laboratories produce customized prosthetic components like dentures and crowns. While ongoing research has yet to produce a reliable AM protocol to create custom, bioresorbable scaffolds for bone reconstruction, this pending scientific development signifies untapped potential for unprecedented regenerative outcomes, as well as commercialization and economic growth.
Figure 3. Paradigm shift in scaffold production. Additive manufacturing has introduced a departure from design for conventional manufacturing processes to additive manufacturing driven by design for the individual patient. The generalized design approach utilizes traditional product specification and engineering processes to facilitate large-scale production for distribution to a target population. Disadvantages of conventional manufacturing include limited capacity for complex designs and less customization. Additive manufacturing (AM) utilizes individual patient data processed by computer-aided design (CAD)/computer-aided manufacturing (CAM) software to perform virtual planning, design optimization, and fabrication of highly personalized scaffolds for bone regeneration. This design process begins and ends with direct patient interaction. AM has enormous potential to improve accessibility to personalized regenerative medicine in everyday clinical dentistry.
Depending on the specific tissue and critical defect size, there are numerous options for AM regenerative scaffolds in the oral and craniofacial arena. The predominant methods for non-metallic bone scaffold production can be categorized broadly into extrusion-based, laser-assisted, or binder jetting type processes (Figure 4). The details regarding the primary compatible materials and specific advantages and disadvantages of each AM technique is summarized in Table 1. The main extrusion-based method for non-metallic scaffold production is FDM. First developed in 1988, FDM is commonly used in the oral and craniofacial regeneration research areas. Materials are extruded as a filament through an output (nozzle or syringe) that is directed by CAD files obtained via radiology or similar imaging techniques (Mota et al., 2015). FDM’s main advantages include greater mechanical strengths and simpler processes relative to other AM techniques. When considering some of the complex oral internal structures, such as the intricate geometry of the periodontal ligament, the lack of resolution needed to create detailed features via FDM is a disadvantage when compared to other AM techniques (George et al., 2017). FDM enables high production rate at a low cost, which has positive implications for FDM’s ability to be used widely in the clinical setting. Additionally, FDM may be used in conjunction with other scaffold fabrication techniques such as electrospinning; preclinical research has shown potential of this combined approach for biphasic constructs employed in vertical bone augmentation (Sudheesh Kumar et al., 2018; Vaquette et al., 2021) and regeneration of the periodontal complex and supporting alveolar bone (Vaquette et al., 2012).
Figure 4. Overview of major types of additive manufacturing processes for bone tissue engineering applications. Additive manufacturing (AM) falls into three major categories: laser-based, extrusion-based, or binder jetting processes. Stereolithography apparatus (SLA) and selective laser sintering (SLS) are the predominant forms of laser-assisted techniques for production of non-metallic bone scaffolds. Fused deposition modeling (FDM) is the main extrusion-based method and binder-jetting is the last method. Melt electrospinning or bioprinting are similar, but distinct scaffold fabrication processes that may be used in conjunction with traditional methods of AM.
Stereolithography apparatus (SLA) and selective laser sintering (SLS) are the primary laser-assisted techniques for non-metallic bone scaffold production. First developed in 1983, SLA utilizes photochemical reactions with UV lasers to produce scaffolds out of photosensitive polymers. Because of specific material and post-processing requirements associated with toxicity concerns, SLA is not as commonly used for craniofacial regeneration. The main advantage of SLA is its capability for high accuracy and refined internal resolution relative to other AM techniques (Msallem et al., 2020). First developed and then subsequently commercialized in 1992, SLS is a powder-based technique which utilizes a laser to sinter powder spread across a rolling plate. Scaffolds developed with SLS have strong mechanical properties suitable for bone and can be designed with complex geometries (Sudarmadji et al., 2011). SLS is especially useful for fabricating porous, bioactive bone scaffolds consisting of polymer-ceramic composites, most commonly involving the combination of HA and PCL (Xia et al., 2013; Du et al., 2015).
Melt electrospinning is a distinct processing technique often used in conjunction with AM. In general, this technique allows for the introduction of micro- and nano-scale features into regenerative scaffolds. The technique is similar to FDM, with the main difference being a high-voltage power supply to extrude precise droplets with a refined resolution. Electrospun fibers and scaffolds are particularly advantageous for drug or small molecule loading because of its nanoscale morphological structure (Chew et al., 2006). Biomaterials with antimicrobial properties offer a significant advantage in the regeneration of periodontal structures affected by periodontal disease, in which oral-biofilm is a key component in the dysregulated inflammatory response. An electrospun gelatin and low molecular weight chitosan scaffold demonstrated antimicrobial efficacy against Aggregatibacter actinomycetemcomitans, a facultative anaerobe commonly implicated in periodontal infections (Budai-Szűcs et al., 2021). Further, an ibuprofen-functionalized, nanofibrous PCL scaffold improved CAL and reduced expression of inflammatory mediators COX-2 and IL-8 in seeded human oral epithelial cells and fibroblasts challenged by Porphyromonas gingivalis lipopolysaccharide, a key pathogenic factor in periodontitis (Jain and Darveau, 2010). Alternatively, solution electrospinning involves polymer solutions and solvents to solubilize the solutions into materials in the scaffold design (Xue et al., 2019). This technique is still used with improved alignment, although melt electrospinning offers more detailed control over the architecture and less toxicity concerns for craniofacial regenerative purposes.
Bioresorbable scaffolds are materials that may be degraded into moieties in vivo, undergoing subsequent elimination through natural pathways resulting in total removal of the initial material without adverse biologic effects (Vert et al., 1992). A large variety of bioresorbable materials with unique material properties and degradation rates are available for scaffold fabrication (Figure 5). The mechanism of degradation occurs either through highly specific enzymatic cleavage, as is the case for natural polymers such as collagen, or passive hydrolysis, which induces chain scission of synthetic polymers under physiologic conditions. The degradation rate is influenced by a multitude of factors including but not limited to the molecular weight, chain configuration, comonomer ratio, residual monomer content, and crystallinity, as well as annealing and sterilization procedures and incorporation of drugs or other additives (Yannas, 2015). Bioresorbable materials are advantageous in bone tissue engineering due to their ability to facilitate regeneration while eliminating the need for removal by a secondary surgical procedure. This is an essential feature for periodontal tissue regeneration, in which delicate connective tissue structures and their interfaces must be restored; removal of a non-resorbable material would traumatize the site and disrupt healing. A principal challenge in formulating bioresorbable materials is matching the degradation rate to the intrinsic pace of native tissue remodeling, while maintaining sufficient mechanical properties of the scaffold. Failure to do so poses a high risk of scaffold exposure in periodontal surgery due to inflammatory complications in the thin gingival tissues that overlay alveolar bone, however, this risk is also present in the use of non-resorbable, metallic scaffolds. Careful flap design and suturing technique must also be employed to obtain primary closure and promote normal wound healing.
Figure 5. Biomaterials for bone scaffold fabrication. A variety of candidate materials are available for scaffold fabrication using additive manufacturing or bioprinting processes. Additive manufacturing typically employs polymers, to which ceramic materials may be added to form composites. Bioprinting incorporates all three elements of the tissue engineering triad: cells, scaffold (hydrogel), and growth factors. Exogenous agents are often incorporated either with pre-loading or post-processing methods.
Scaffolds for bone tissue engineering can be typically assigned to one of the following categories: natural biopolymers, synthetic polymers, ceramics, acellular tissue matrices, and composite materials composed of two or more material groups (Akter and Ibanez, 2016). Natural polymers are biologically active and can be further categorized into polypeptides or polysaccharides, which are both frequently used in 3D bioprinting techniques (covered in section “Three Dimensional Bioprinting”). Polypeptide-based materials in particular possess amino acid sequences associated with integrin-binding domains conducive to cell adhesion and growth (Filippi et al., 2020). Another notable advantage is their biodegradability, which facilitates host cell production of extracellular matrix to replace the degrading scaffold (Akter and Ibanez, 2016). Disadvantageous features of some natural materials include risk of immunogenicity, possibility for disease transmission, and relatively low mechanical strength.
Synthetic polymers are the largest group of biodegradable polymers and include poly(α-ester)s, polyurethanes, polyacetals, poly(ester amide)s, polyanhydrides, polyphosphazenes, and pseudo poly(amino acids) (Filippi et al., 2020). Their use are highly prevalent in AM techniques due to characteristic low melting points and versatile physical properties that accommodate a wide range of processing parameters. Due to their high biocompatibility, numerous synthetic polymers are FDA-approved and can be employed in a broad range of biomedical applications. The poly(α-ester) family is the most common bioresorbable material choice compatible with AM production of scaffolds for bone tissue engineering and includes PCL, PLGA, polylactic acid (PLA), and polyglycolic acid (PGA) (Burg, 2014). These polymers are also frequently combined with ceramic biomaterials, which not only enhance mechanical properties and osteoconductivity, but also confer osteoinductive and osteogenic potential due to their similar composition to the inorganic phase of bone (Ducheyne and Qiu, 1999; Chai et al., 2012). Incorporation of other bioactive compounds such as calcium-silicate, can improve polymer surface hydrophilicity (Lin et al., 2017) and provide osteostimulation (Zhai et al., 2017). Representative in vivo studies of bioresorbable polymeric and polymeric-composite scaffolds produced by AM techniques for bone regeneration are featured in Table 2.
Table 2. Representative in vivo studies using additive manufacturing (AM) to produce resorbable scaffolds for dental, oral, and craniofacial-related bone regeneration from 2010 to 2020.
Cell-derived, decellularized extracellular matrix (dECM) may also be combined with synthetic polymeric scaffolds to provide appropriate molecular cues for osteogenic activity. AM-printed constructs have been coated with dECM obtained from bone cells (Wu et al., 2019) or non-bone cells such as human lung fibroblasts (Kim et al., 2018) and MSCs from nasal inferior turbinate tissue (Pati et al., 2015). dECM has also been obtained from dental pulp (Sangkert et al., 2016). Preclinical experiments have demonstrated superior ability of dECM coatings to enhance new bone formation in vivo compared to bare scaffold controls (Pati et al., 2015; Kim et al., 2018; Wu et al., 2019). Further, dECM coatings downregulate expression of pro-inflammatory cytokines tumor necrosis factor α (TNF-α) and interleukin-1 (IL-1) and improve MSC adhesion, proliferation, and osteogenic differentiation through induction of attachment protein expression in vitro (Wu et al., 2019). Application of dECM coatings to scaffolds produced by AM addresses the need for balance between biologic and mechanical properties while overcoming limitations of tissue-derived ECM which consists of decellularized tissues or organs (Zhang W. et al., 2016).
Bioprinting, generally defined as “the use of computer-aided transfer processes for patterning and assembly of living and non-living materials with a prescribed 2D or 3D organization to produce bioengineered structures” (Daly et al., 2021b), is a promising field in regenerative medicine, providing precise and controlled deposition of cells, hormones, drugs, and growth factors, etc. thus directing improved tissue regeneration (Aljohani et al., 2018). Among the broad range of 3D printing techniques, the most common and accessible bioprinting method is extrusion bioprinting, where the pressure-driven extrusion of a bioink from a printer head is used to print filaments following a defined design or pattern (Ozbolat and Hospodiuk, 2016). Inkjet printing falls under the umbrella of extrusion printing but involves the deposition of bioink droplets through the printhead rather than continuous filaments (Li X. et al., 2020). For extrusion-based or extrusion-related bioprinting, the bioink is a unique feature compared with cell-free 3D printing. Bioinks can generally be described as “a formulation of cells that is suitable to be processed by an automated biofabrication technology” (Groll et al., 2018), which usually has a hydrogel formulation as the main component containing cell-suspensions or cell aggregates (Daly et al., 2021b).
The selection of bioinks is one of the most critical steps in the process of bioprinting, mainly relying on two important aspects: biofabrication and biocompatibility. Biofabrication usually refers to the printability of the ink, such as the compatibility with the printer and printing resolution, which is highly related to the rheological properties of the bioink. Viscous and shear-thinning hydrogels, such as gelatin and methylcellulose (Ahlfeld et al., 2020), are often considered suitable for many bioprinting scenarios, as these materials can flow smoothly during extrusion, avoid the formation of clogging within the printhead, and stabilize after deposition. Biocompatibility involves the impact of the bioink on cell behaviors including short-term cell viability and long-term cell proliferation, migration, differentiation and organization. The cellular interactions with the bioink can be influenced by multiple factors simultaneously such as the gelation and deposition processes, as well as the biological and biophysical properties. Of note, a desired bioink might be specifically related to limited cell types and biological scenarios.
Dental, oral and craniofacial tissues are organized with complex 3D architectures involving multiple types of cells and tissues. Mimicking their 3D complexity and multicellular interactions represents one of the main barriers in dental and craniofacial regeneration (Obregon et al., 2015). 3D bioprinting holds great potential for creating 3D defect-specific constructs with multiple cell sources for use in regenerative medicine. 3D bioprinting studies applied to dental and craniofacial regeneration can be divided into three general focuses including the periodontal complex, pulp-dentin complex and craniomaxillofacial bone (Table 3). As periodontal ligament cells (PDLCs) contain stem cells that harbor the potential to generate cementum/PDL-like tissue (Seo et al., 2004), PDLCs are one of the most frequently-employed cell types for periodontal regeneration-oriented bioprinting. A previous study systematically investigated the printability of various concentrations of GelMA hydrogels and the influence of different 3DP parameters such as photoinitiator concentration, UV exposure, pressure and needle diameter on the viability of PDLCs in order to achieve high printing resolution, dimension ability and cell viability simultaneously for periodontal regeneration (Raveendran et al., 2019).
Table 3. Representative studies on 3D bioprinting for dental, oral, and craniofacial-related regeneration from 2016 to 2020.
The regeneration of pulp-dentin complex or the whole tooth has attracted great attention in dentistry. It is known that dental pulp stem cells (DPSCs) can differentiate into several cell types, including odontoblasts, neural progenitors, osteoblasts, chondrocytes, and adipocytes with high proliferative capability (Casagrande et al., 2011). Therefore, various studies have combined DPSCs with modified bioinks to establish 3D-bioprinted dental constructs. In previous research, a dentin matrix was isolated and combined with alginate to fabricate hydrogel blends as the bioink (Athirasala et al., 2018). The printability of the bioinks was greater in the formulations containing higher concentrations of alginate, whereas a higher proportion of dentin matrix proteins significantly improved cell viability and a 1:1 ratio of alginate and dentin was determined to be most suitable. Further, addition of acid-soluble dentin molecules into hydrogels enhanced odontogenic differentiation. Besides naturally derived molecules, synthetic biomolecules such as BMP-mimetic peptide have been incorporated into bioink as well. Park J. H. et al. (2020) developed a novel BMP peptide-tethering bioink formulation and found 50% of the peptides remained in the bioprinted construct after 3 weeks in an in vitro cell culture. The BMP peptide construct group exhibited the highest calcification as compared to the growth medium, osteogenic medium, and control groups with robust expression of osteogenic genes. In addition to pulp-dentin complex, the feasibility of whole tooth bioprinting has been studied by co-printing the hDPSCs-laden bioinks with PCL. The results not only achieved localized differentiation of hDPSCs in the outer region of the 3D cellular construct but also successfully produced 3D patient-specific cellular constructs for tooth tissue engineering in a predefined pattern (Han et al., 2019).
Engineering craniomaxillofacial bone tissue is a unique challenge due to the complex architecture of bone, consisting of organized calcified regions with interpenetrated vasculature (Salgado et al., 2004). In order to support osteogenesis, stem cells with osteogenic potential such as bone marrow-derived MSCs or DPSCs were frequently used. Moreover, various bioactive components have been incorporated into bioinks to enhance the osteogenic ability including amorphous magnesium phosphate (Dubey et al., 2020), bioactive glass (Ojansivu et al., 2019) and silicate nanoplatelets (Byambaa et al., 2017). To further promote vascularization, human umbilical vein endothelial cells (HUVECs) have been encapsulated into GelMA hydrogel bioinks to engineer vasculogenic niches. Moreover, to promote vascular spreading, chemically conjugated VEGF were introduced in the surrounding bone niches (Byambaa et al., 2017).
Although extrusion bioprinting is a common and accessible bioprinting technology compatible with a large variety of bioinks, other bioprinting technologies have been developed to overcome the main limitations of extrusion-based printing including lithography bioprinting and spheroid bioprinting (Daly et al., 2021b). Lithography bioprinting technology can create physical features at the scale of 10–100 μm, which is a significant advantage over extrusion bioprinting in which the minimum resolution is ∼100 μm (Bertlein et al., 2017; Lim et al., 2018). Spheroid bioprinting, which processes self-organized tissues (often cell spheroids) into 3D constructs to scale and direct self-organization, can mimic tissue-like features and achieve high cell densities to promote cell–cell contacts (Skylar-Scott et al., 2019; Daly et al., 2021a). There are numerous interesting and promising applications of lithography and spheroid bioprinting techniques to fabricate complicated in vitro systems that would otherwise be challenging for extrusion-based processes to realize, including a liver lobule model (Ma et al., 2016), alveolar lung model (Grigoryan et al., 2019), and other organ and tissue models (Grigoryan et al., 2019; Daly et al., 2021a). Until now, dental, oral, and craniofacial applications using these novel bioprinting technologies for repair and regeneration have been scarce.
The numerous bones of the craniofacial skeleton exhibit variable anatomical forms and exist in intimate relation to one another, as well as to abundant nerves and vessels. As such, bony reconstruction within this region often entails labor-intensive, multi-step operations with limited surgical access to morphologically complex defects. In the early stage of AM, stereolithographic models were introduced as an adjunct to standard diagnostic imaging and casts. These 3D models improved surgeon visualization of bony defects and their spatial relationship to adjacent structures, thus enhancing accuracy in preoperative evaluation, diagnosis, and treatment planning (D’Urso et al., 1999). With significant advancements in high resolution medical imaging and CAD-CAM software, AM processes are now employed to fabricate personalized constructs for a vast range of applications in all phases of craniomaxillofacial surgery (Levine et al., 2012; Yu et al., 2019).
In a recent systematic review of customized objects used in 3D printing-assisted craniofacial and maxillofacial operations, four major categories of personalized constructs were identified: (1) contour models; (2) guides; (3) splints; and (4) implants (Jacobs and Lin, 2017). Contour models facilitate accurate prebending of reconstruction meshes or plates, eliminating the need for extensive intraoperative manipulation and significantly reducing operating time (Sumida et al., 2015; von Wilmowsky et al., 2020). Guides utilize negative space relative to patient anatomy to provide intraoperative reference for precise osteotomy preparation and controlled positioning of dental (Ersoy et al., 2008) and zygomatic (Wang et al., 2020) implants. Splints are similar to guides; however, they are fabricated to align structures in virtually planned, postoperative positions. Finally, implants are medical devices surgically placed patient tissues. Customized CAD-CAM devices have been employed for human clinical use in the reconstruction of structures such as the temporomandibular joint (Ackland et al., 2018), maxilla and mandible (Lethaus et al., 2012; Ma et al., 2017; Chiapasco et al., 2021), paranasal sinuses, nasal bone (Horn et al., 2012), orbit (Bachelet et al., 2018), and cranial vault (Jardini et al., 2014; Park et al., 2016; Unterhofer et al., 2017). Use of components produced by AM can also minimize discrepancies between planned and actual surgical outcomes. For example, in a case series documenting nine patients undergoing orthognathic surgery or distraction osteogenesis procedures, the use of custom templates and reconstruction microplates enabled accurate repositioning of the maxillary segment within 1 mm of the digitally planned centroid position and 1° orientation in all linear and axial directions, respectively (He et al., 2015).
Additive manufacturing is now widely employed in dentistry for a variety of purposes, including fabrication of dentures, occlusal splints, temporary crowns and bridges, orthodontic appliances, and surgical guides. More recently, customized, non-resorbable titanium metal cages intended for extraosseous alveolar ridge augmentation have become available to clinicians as well. Despite prolific use of commercially available AM equipment to create custom dental devices in every day clinical practice, attempts to utilize bioresorbable bone scaffolds produced by this technology have only recently begun in academic, clinical settings. In 2015, the first dental use of a personalized, bioresorbable scaffold produced with AM in humans was reported. A PCL scaffold fabricated by selective laser sintering was loaded with rhPDGF-BB solution and implanted into a periosseous defect in the periodontium localized to a mandibular canine site (Rasperini et al., 2015). The design incorporated novel, cylindrical-shaped, PDL fiber-guiding architecture previously reported in a rodent model (Park et al., 2012). At 1 year, a modest 3 mm gain in clinical attachment and partial root coverage was achieved but graft exposure culminated in scaffold failure (Rasperini et al., 2015). This complication also occurred in a case series assessing the use of prefabricated, FDM-printed, PCL scaffolds for alveolar ridge preservation (Goh et al., 2015). The scaffolds remained largely intact within the healing extraction sockets for 6 months and 2/13 patients experienced manageable graft exposure, highlighting the challenges posed by the slow resorption rate of PCL.
Customized, bioresorbable bone scaffolds created by AM processes have been tested more extensively in the fields of maxillofacial and craniofacial surgery. In 2016, a clinical case series described 20 patients who received artificial bone constructed with 3D inkjet printing to graft non-weight bearing, maxillofacial bone deformities in 23 sites (Saijo et al., 2009; Kanno et al., 2016). These scaffolds were fabricated in 0.1 mm layers by spraying hardening liquid composed of 5% sodium chondroitin sulfate, 12% disodium succinate, and 83% distilled water onto an α-TCP powder. At 1 year, 18 of 21 remaining grafted sites demonstrated CT values indicative of satisfactory bone union. Bacterial infection necessitated removal in 4 sites within a period of 1 – 5 years postoperatively. Failures tended to occur in grafts spanning larger missing bone volume or in one case, a patient that was a carrier of MRSA. With the longest follow-up period occurring just over 7 years, none of the artificial bones demonstrated complete replacement and only partial new bone formation was observed within the scaffold. Despite the limited success observed in these initial translational studies, these efforts represent the emergence of image-based, bioresorbable scaffold technology in the clinical arenas of dental and craniomaxillofacial surgery.
Bone grafting is a routine procedure in clinical dentistry and may occur in approximately half of all dental implant sites (Cha et al., 2016). Augmentation of the alveolar ridge through procedures such as guided bone regeneration and maxillary sinus lifts, are often necessary to create adequate bone volume prior to implant placement. Due to a high frequency of bone grafting procedures in the healthcare field overall and a limited pool of musculoskeletal tissue donors, increased use of bone graft substitutes relative to autogenous grafts is a precipitating trend (Kinaci et al., 2014). This is reflected in the global market for dental bone graft substitutes, which had an estimated value of $450 million in 2020 and is projected to reach $659 million by 2025 (Markets and Markets, 2020). In the future, AM may yield a new generation of bone graft substitutes that achieve improved regenerative outcomes by uniting the versatility of CAD-CAM technology with modern tissue engineering principles and a personalized medicine treatment approach.
Contemporary biomedical research is steadily approaching a reliable AM strategy to create bioresorbable bone scaffolds for clinical use and the implications for patient care are enormous. Recently, a workflow for AM fabrication of porous, bioresorbable scaffolds consisting of medical grade PCL for the reconstruction of large, posterior mandibular defects was demonstrated (Bartnikowski et al., 2020). The resultant porosity (83.91%) and mean pore size (590 ± 243 μm) were within suitable ranges for bone regeneration and the mean discrepancy between the template implant model and the scanned scaffold was found to be 74 ± 14 μm, representing a level of accuracy adequate for clinical application. Pending further preclinical validation and clinical trials, rapid in-house fabrication and deployment of personalized bone scaffolds with accurate replication of individual patient anatomy could revolutionize trauma care in the fields of maxillofacial and craniofacial surgery. Esthetics, form, and function could also be restored in patients that have undergone massive tumor resection in craniofacial structures. In implant dentistry, substantial augmentation or reconstruction of the alveolar ridge could be accomplished with personalized constructs rather than adapting universal materials to anatomically diverse defects. Invasive procedures, such as autogenous harvesting of large, block grafts from secondary surgical sites or the placement of zygomatic implants, which are reserved for severely atrophic maxillae, may also be avoided. Finally, AM offers new strategies to employ scaffolds as carriers for exogenous agents that not only enhance regeneration but offer therapeutic benefit to the patient as well. This may be especially valuable for modulation of the destructive, biochemical mechanisms inherent to tissues affected by chronic inflammation, such as in periodontal disease.
AM holds tremendous promise for the advancement of regenerative medicine; however, this impressive technology must overcome several obstacles before it can be extensively introduced to clinical settings for the purpose of fabricating personalized bone tissue scaffolds. First, AM has historically been limited by relatively low production speed. In 2017, the average build time to create a personalized object constructed by AM techniques in the field of craniomaxillofacial surgery is approximately 18.9 h but can be as high as 96 h per object (Jacobs and Lin, 2017). Practical utilization requires faster manufacturing processes that maintain adequate print resolution, surface quality, and mechanical integrity, especially to hold relevance for applications in urgent care. Use of modern multi-extrusion printing systems is swiftly rising in tissue engineering for bone and periodontal structures due to its one-step printing approach, improved speeds, and ability to use versatile material formulations (Porta et al., 2020). Second, variations in part quality can occur due to errors introduced during the digital manipulation of virtual models or during the physical construction process. Third, decentralization of bioresorbable scaffold fabrication from commercial biomaterial manufacturing facilities to local centers of production may complicate safety assessments and reporting of adverse events. Last, the use of customized scaffolds with innumerable variations in composition and design may present significant challenges for standardized regulation in the clinical dental practice setting. Development and oversight of appropriate guidelines for post-manufacturing quality assurance and sterilization would be required. In the case of implantable scaffolds, sterilization is especially critical to prevent infection. Scaffolds constructed with certain polymeric materials may not be able to withstand the high temperatures necessary for autoclave sterilization and would require alternative sterilization methods.
Despite these potential limitations, the technologic infrastructure necessary to produce bioresorbable scaffolds for bone regeneration by AM is available for implementation. Successful translation to clinical use now relies upon the ability to manipulate biomaterials and precisely coordinate their architectural and biochemical features with known physiologic mechanisms of tissue formation. This process is further complicated by the addition of exogenous agents, such as viable cells, growth factors, gene vectors, drugs, and other bioactive components, which must be present in appropriate quantities to simulate a suitable microenvironment for regeneration. Simultaneous incorporation of viable cells during scaffold fabrication remains a preeminent challenge. Ensuring cell survival during the AM process and preserving their phenotype and morphology post-processing will require continued development of cell-deposition techniques and cell-carrier systems. Improved control of cellular responses will benefit from the progressive advancement of scaffold-based gene therapy techniques. Finally, innovation in the use of hydrogel bioinks and 3D bioprinting processes will further refine spatiotemporal regulation of biomolecular signaling and progress efforts to regenerate bone tissue with bioresorbable, biomimetic scaffolds in vivo.
A successful, alloplastic bone substitute biomaterial fabricated by AM is a treatment concept just on the horizon of realistic clinical practice and there are many exciting implications for the future. AM has given rise to a new manufacturing concept termed four-dimensional (4D) printing, in which time is the fourth dimension of the printed construct (Saska et al., 2021). 4D printing aims to create scaffolds fabricated with advanced or “smart” materials that react to external stimuli such as pH, humidity, light, and temperature, allowing dynamic responses to in vivo conditions (Valvez et al., 2021). Potential applications of these sensitive materials include utilizing environmental stimuli to induce appropriate release patterns of angiogenic and osteogenic factors during wound healing and tissue formation processes, thus enhancing regenerative capability. Even further, shape-morphing (Gladman et al., 2016) and shape-memory materials (Zhou and Sheiko, 2016; Liu et al., 2020) are setting the stage for scaffold materials capable of controlled self-assembly or even self-repair (Zhang et al., 2019), which may offer pivotal advantages for the regeneration of weight-bearing structures, such as the jaws or temporomandibular joint. Lastly, in situ bioprinting, which entails real-time scaffold fabrication directly within the defect (O’Connell et al., 2016; Di Bella et al., 2018), is an interesting development of AM technology with clinical potential for bone regeneration (Keriquel et al., 2010; Keriquel et al., 2017), especially in defect sites with complex morphologies, significant undercuts, or limited surgical access; examples of potential applications include bone grafting of the maxillary sinuses, intrabony defects, and peri-implant defects.
The treatment of dental, oral, and craniofacial bone defects is currently restricted by available biomaterials, which have limited capacity to facilitate true regeneration of new tissues that exhibit native physiologic form, function and esthetics. Further research efforts are needed to optimize AM for the production of bioresorbable scaffolds that yield safe, predictable, and efficacious clinical outcomes in the reconstruction of bony defects. More preclinical studies are needed to improve the material properties and clinical performance of polymer-ceramic composite scaffolds for bone reconstruction and to refine understanding of the architectural features that promote formation of an anatomic periodontal ligament compartment. Additionally, tremendous opportunity exists to functionalize scaffolds for therapeutic purposes, especially with regards to gene therapy. As the understanding of multifaceted biomaterial interactions and tissue dynamics improves within the scientific community, AM offers a promising future in which a superior generation of sustainable regenerative biomaterials will become accessible for everyday clinical use. Commercialization of custom scaffold technology will dramatically accelerate the trend toward increased usage of synthetic bone substitutes and expand their existing market share within the multibillion dollar industry for biomaterials. Successful adaptation of AM technology for bone tissue engineering will expose a new realm of regenerative possibilities within dental medicine, thus expanding treatment options for patients and significantly improving their oral health related quality-of-life. Eventually, personalized bone constructs for dental regenerative medicine will evolve from state-of-the-art technology to a new standard in patient care.
WG and JL contributed to conception and design. JL led the writing of the manuscript. SM, YY, DW, and MC wrote sections of the manuscript. All authors contributed to manuscript revision. All authors read and approved the submitted version.
This work was supported by U24 DE026915 and U24 DE029462 from the NIDCR (WG) and the Osteology Foundation (SM).
The authors declare that the research was conducted in the absence of any commercial or financial relationships that could be construed as a potential conflict of interest.
All claims expressed in this article are solely those of the authors and do not necessarily represent those of their affiliated organizations, or those of the publisher, the editors and the reviewers. Any product that may be evaluated in this article, or claim that may be made by its manufacturer, is not guaranteed or endorsed by the publisher.
The authors thank James Sugai for critically reviewing the manuscript. All figures were made in BioRender (biorender.com).
Abbasi, N., Abdal-Hay, A., Hamlet, S., Graham, E., and Ivanovski, S. (2019). Effects of gradient and offset architectures on the mechanical and biological properties of 3-D Melt Electrowritten (MEW) scaffolds. ACS Biomater. Sci. Eng. 5, 3448–3461. doi: 10.1021/acsbiomaterials.8b01456
Abogunrin, S., Di Tanna, G. L., Keeping, S., Carroll, S., and Iheanacho, I. (2014). Prevalence of human papillomavirus in head and neck cancers in European populations: a meta-analysis. BMC Cancer 14:968.
Ackland, D., Robinson, D., Lee, P., and Dimitroulis, G. (2018). Design and clinical outcome of a novel 3D-printed prosthetic joint replacement for the human temporomandibular joint. Clin. Biomech. 56, 52–60. doi: 10.1016/j.clinbiomech.2018.05.006
Ahlfeld, T., Guduric, V., Duin, S., Akkineni, A., Schütz, K., Kilian, D., et al. (2020). Methylcellulose–a versatile printing material that enables biofabrication of tissue equivalents with high shape fidelity. Biomater. Sci. 8, 2102–2110. doi: 10.1039/d0bm00027b
Aichelmann-Reidy, M. E., Avila-Ortiz, G., Klokkevold, P. R., Murphy, K. G., Rosen, P. S., Schallhorn, R. G., et al. (2015). Periodontal regeneration - furcation defects: practical applications from the AAP regeneration workshop. Clin. Adv. Periodontics 5, 30–39. doi: 10.1902/cap.2015.140068
Akter, F., and Ibanez, J. (2016). Chapter 8 - bone and cartilage tissue engineering. Tissue Eng. Made Easy 2016, 77–97. doi: 10.1016/b978-0-12-805361-4.00008-4
Albandar, J. M., Susin, C., and Hughes, F. J. (2018). Manifestations of systemic diseases and conditions that affect the periodontal attachment apparatus: case definitions and diagnostic considerations. J. Periodontol. 89(Suppl. 1), S183–S203.
Aljohani, W., Ullah, M. W., Zhang, X., and Yang, G. (2018). Bioprinting and its applications in tissue engineering and regenerative medicine. Int. J. Biol. Macromol. 107, 261–275.
Allen, E. P., Gainza, C. S., Farthing, G. G., and Newbold, D. A. (1985). Improved technique for localized ridge augmentation. a report of 21 cases. J. Periodontol. 56, 195–199. doi: 10.1902/jop.1985.56.4.195
Almazin, S. M., Dziak, R., Andreana, S., and Ciancio, S. G. (2009). The effect of doxycycline hyclate, chlorhexidine gluconate, and minocycline hydrochloride on osteoblastic proliferation and differentiation in vitro. J. Periodontol. 80, 999–1005. doi: 10.1902/jop.2009.080574
Al-Moraissi, E. A., Alkhutari, A. S., Abotaleb, B., Altairi, N. H., and Del Fabbro, M. (2020). Do osteoconductive bone substitutes result in similar bone regeneration for maxillary sinus augmentation when compared to osteogenic and osteoinductive bone grafts? a systematic review and frequentist network meta-analysis. Int. J. Oral. Maxillofac. Surg. 49, 107–120. doi: 10.1016/j.ijom.2019.05.004
Araujo, M. G., Sukekava, F., Wennstrom, J. L., and Lindhe, J. (2005). Ridge alterations following implant placement in fresh extraction sockets: an experimental study in the dog. J. Clin. Periodontol. 32, 645–652. doi: 10.1111/j.1600-051x.2005.00726.x
Asa’ad, F., Pelanyte, G., Philip, J., Dahlin, C., and Larsson, L. (2020). The role of epigenetic functionalization of implants and biomaterials in osseointegration and bone regeneration-a review. Molecules 25:5879. doi: 10.3390/molecules25245879
Aslan, S., Buduneli, N., and Cortellini, P. (2020). Clinical outcomes of the entire papilla preservation technique with and without biomaterials in the treatment of isolated intrabony defects: a randomized controlled clinical trial. J. Clin. Periodontol. 47, 470–478. doi: 10.1111/jcpe.13255
Athirasala, A., Tahayeri, A., Thrivikraman, G., França, C. M., Monteiro, N., Tran, V., et al. (2018). A dentin-derived hydrogel bioink for 3D bioprinting of cell laden scaffolds for regenerative dentistry. Biofabrication 10:024101. doi: 10.1088/1758-5090/aa9b4e
Avila-Ortiz, G., Elangovan, S., Kramer, K. W., Blanchette, D., and Dawson, D. V. (2014). Effect of alveolar ridge preservation after tooth extraction: a systematic review and meta-analysis. J. Dent. Res. 93, 950–958. doi: 10.1177/0022034514541127
Bachelet, J. T., Cordier, G., Porcheray, M., Bourlet, J., Gleizal, A., and Foletti, J. M. (2018). Orbital reconstruction by patient-specific implant printed in porous titanium: a retrospective case series of 12 patients. J. Oral Maxillofac. Surg. 76, 2161–2167. doi: 10.1016/j.joms.2018.04.006
Bagheri, A., and Jin, J. (2019). Photopolymerization in 3D Printing. ACS Appl. Polym. Mater. 1, 593–611. doi: 10.1021/acsapm.8b00165
Barbato, L., Selvaggi, F., Kalemaj, Z., Buti, J., Bendinelli, E., Marca, M., et al. (2020). Clinical efficacy of minimally invasive surgical (MIS) and non-surgical (MINST) treatments of periodontal intra-bony defect. A systematic review and network meta-analysis of RCT’s. Clin. Oral Investig. 24, 1125–1135. doi: 10.1007/s00784-020-03229-0
Bartnikowski, M., Vaquette, C., and Ivanovski, S. (2020). Workflow for highly porous resorbable custom 3D printed scaffolds using medical grade polymer for large volume alveolar bone regeneration. Clin. Oral Implants Res. 31, 431–441. doi: 10.1111/clr.13579
Bertlein, S., Brown, G., Lim, K. S., Jungst, T., Boeck, T., Blunk, T., et al. (2017). Thiol–ene clickable gelatin: a platform bioink for multiple 3D biofabrication technologies. Adv. Mater. 29:1703404. doi: 10.1002/adma.201703404
Bettany, J. T., Peet, N. M., Wolowacz, R. G., Skerry, T. M., and Grabowski, P. S. (2000). Tetracyclines induce apoptosis in osteoclasts. Bone 27, 75–80. doi: 10.1016/s8756-3282(00)00297-0
Bodic, F., Hamel, L., Lerouxel, E., Baslé, M. F., and Chappard, D. (2005). Bone loss and teeth. Joint Bone Spine 72, 215–221. doi: 10.1016/j.jbspin.2004.03.007
Bourell, D., Kruth, J., Leu, M., Levy, G., Rosen, D., Beese, A., et al. (2017). Materials for additive manufacturing. CIRP Ann. 66, 659–681.
Budai-Szűcs, M., Ruggeri, M., Faccendini, A., Léber, A., Rossi, S., Varga, G., et al. (2021). Electrospun scaffolds in periodontal wound healing. Polymers 13:307. doi: 10.3390/polym13020307
Burg, K. (2014). “Poly(α-ester)s,” in Natural and Synthetic Biomedical Polymers, ed. S. G. Kum (London: Newnes).
Byambaa, B., Annabi, N., Yue, K., Trujillo-de Santiago, G., Alvarez, M. M., Jia, W., et al. (2017). Bioprinted osteogenic and vasculogenic patterns for engineering 3D bone tissue. Adv. Healthc. Mater. 6:1700015. doi: 10.1002/adhm.201700015
Caballé-Serrano, J., Abdeslam-Mohamed, Y., Munar-Frau, A., Fujioka-Kobayashi, M., Hernández-Alfaro, F., and Miron, R. (2019). Adsorption and release kinetics of growth factors on barrier membranes for guided tissue/bone regeneration: a systematic review. Arch. Oral Biol. 100, 57–68. doi: 10.1016/j.archoralbio.2019.02.006
Casagrande, L., Cordeiro, M. M., Nör, S. A., and Nör, J. E. (2011). Dental pulp stem cells in regenerative dentistry. Odontology 99, 1–7. doi: 10.1007/978-3-319-55645-1_1
Cha, H. S., Kim, J. W., Hwang, J. H., and Ahn, K. M. (2016). Frequency of bone graft in implant surgery. Maxillofac. Plast. Reconstr. Surg. 38:19. doi: 10.1007/978-1-4471-1934-0_4
Chai, Y. C., Carlier, A., Bolander, J., Roberts, S. J., Geris, L., Schrooten, J., et al. (2012). Current views on calcium phosphate osteogenicity and the translation into effective bone regeneration strategies. Acta Biomater. 8, 3876–3887. doi: 10.1016/j.actbio.2012.07.002
Chang, P. C., Cirelli, J. A., Jin, Q., Seol, Y. J., Sugai, J. V., D’Silva, N. J., et al. (2009). Adenovirus encoding human platelet-derived growth factor-B delivered to alveolar bone defects exhibits safety and biodistribution profiles favorable for clinical use. Hum. Gene Ther. 20, 486–496. doi: 10.1089/hum.2008.114
Chang, P. C., Seol, Y. J., Cirelli, J. A., Pellegrini, G., Jin, Q., Franco, L. M., et al. (2010). PDGF-B gene therapy accelerates bone engineering and oral implant osseointegration. Gene Ther. 17, 95–104. doi: 10.1038/gt.2009.117
Chaudhuri, O., Cooper-White, J., Janmey, P. A., Mooney, D. J., and Shenoy, V. B. (2020). Effects of extracellular matrix viscoelasticity on cellular behaviour. Nature 584, 535–546. doi: 10.1038/s41586-020-2612-2
Chaudhuri, O., Gu, L., Klumpers, D., Darnell, M., Bencherif, S. A., Weaver, J. C., et al. (2016). Hydrogels with tunable stress relaxation regulate stem cell fate and activity. Nat. Mater. 15, 326–334. doi: 10.1038/nmat4489
Chen, X., Lin, Z., Feng, Y., Tan, H., Xu, X., Luo, J., et al. (2019). Zwitterionic PMCP-Modified polycaprolactone surface for tissue engineering: antifouling, cell adhesion promotion, and osteogenic differentiation properties. Small 15:e1903784. doi: 10.1002/smll.201903784
Cheng, A., Humayun, A., Boyan, B. D., and Schwartz, Z. (2016). Enhanced osteoblast response to porosity and resolution of additively manufactured Ti-6Al-4V constructs with trabeculae-inspired porosity. 3D Print. Addit. Manuf. 3, 10–21. doi: 10.1089/3dp.2015.0038
Chew, S. Y., Wen, Y., Dzenis, Y., and Leong, K. W. (2006). The role of electrospinning in the emerging field of nanomedicine. Curr. Pharm. Des. 12, 4751–4770. doi: 10.2174/138161206779026326
Chiapasco, M., Casentini, P., Tommasato, G., Dellavia, C., and Del Fabbro, M. (2021). Customized CAD/CAM Titanium meshes for the guided bone regeneration of severe alveolar ridge defects: preliminary results of a retrospective clinical study in humans. Clin. Oral Implants Res. 32, 498–510. doi: 10.1111/clr.13720
Clementini, M., Morlupi, A., Canullo, L., Agrestini, C., and Barlattani, A. (2012). Success rate of dental implants inserted in horizontal and vertical guided bone regenerated areas: a systematic review. Int. J. Oral Maxillofac. Surg. 41, 847–852. doi: 10.1016/j.ijom.2012.03.016
Cochran, D. L., Oh, T. J., Mills, M. P., Clem, D. S., McClain, P. K., Schallhorn, R. A., et al. (2016). A randomized clinical trial evaluating rh-FGF-2/beta-TCP in periodontal defects. J. Dent. Res. 95, 523–530. doi: 10.1177/0022034516632497
Connizzo, B. K., Sun, L., Lacin, N., Gendelman, A., Solomonov, I., Sagi, I., et al. (2021). Nonuniformity in periodontal ligament: mechanics and matrix composition. J. Dent. Res. 100, 179–186. doi: 10.1177/0022034520962455
Cortellini, P. (2012). Minimally invasive surgical techniques in periodontal regeneration. J. Evid. Based Dent. Pract. 12, 89–100. doi: 10.1016/s1532-3382(12)70021-0
Cortellini, P., and Tonetti, M. S. (2011). Clinical and radiographic outcomes of the modified minimally invasive surgical technique with and without regenerative materials: a randomized-controlled trial in intra-bony defects. J. Clin. Periodontol. 38, 365–373. doi: 10.1111/j.1600-051x.2011.01705.x
Costa, P. F., Vaquette, C., Zhang, Q., Reis, R. L., Ivanovski, S., and Hutmacher, D. W. (2014). Advanced tissue engineering scaffold design for regeneration of the complex hierarchical periodontal structure. J. Clin. Periodontol. 41, 283–294. doi: 10.1111/jcpe.12214
Daegling, D. J., Ravosa, M. J., Johnson, K. R., and Hylander, W. L. (1992). Influence of teeth, alveoli, and periodontal ligaments on torsional rigidity in human mandibles. Am. J. Phys. Anthropol. 89, 59–72. doi: 10.1002/ajpa.1330890106
Dalby, M. J., Gadegaard, N., Tare, R., Andar, A., Riehle, M. O., Herzyk, P., et al. (2007). The control of human mesenchymal cell differentiation using nanoscale symmetry and disorder. Nat. Mater. 6, 997–1003. doi: 10.1038/nmat2013
Daly, A. C., Davidson, M. D., and Burdick, J. A. (2021a). 3D bioprinting of high cell-density heterogeneous tissue models through spheroid fusion within self-healing hydrogels. Nat. Commun. 12:753
Daly, A. C., Prendergast, M. E., Hughes, A. J., and Burdick, J. A. (2021b). Bioprinting for the biologist. Cell 184, 18–32. doi: 10.1016/j.cell.2020.12.002
Darnell, M., Young, S., Gu, L., Shah, N., Lippens, E., Weaver, J., et al. (2017). Substrate stress-relaxation regulates scaffold remodeling and bone formation in vivo. Adv. Healthc. Mater. 6:1601185.
Dechow, P. C., Wang, Q., and Peterson, J. (2010). Edentulation alters material properties of cortical bone in the human craniofacial skeleton: functional implications for craniofacial structure in primate evolution. Anat. Rec. 293, 618–629. doi: 10.1002/ar.21124
Di Bella, C., Duchi, S., O’Connell, C. D., Blanchard, R., Augustine, C., Yue, Z., et al. (2018). In situ handheld three-dimensional bioprinting for cartilage regeneration. J. Tissue Eng. Regen. Med. 12, 611–621. doi: 10.1002/term.2476
Du, Y., Liu, H., Shuang, J., Wang, J., Ma, J., and Zhang, S. (2015). Microsphere-based selective laser sintering for building macroporous bone scaffolds with controlled microstructure and excellent biocompatibility. Colloids Surf. B Biointerfaces 135, 81–89. doi: 10.1016/j.colsurfb.2015.06.074
Duarte Campos, D. F., Blaeser, A., Buellesbach, K., Sen, K. S., Xun, W., Tillmann, W., et al. (2016). Bioprinting organotypic hydrogels with improved mesenchymal stem cell remodeling and mineralization properties for bone tissue engineering. Adv. Healthc. Mater. 5, 1336–1345. doi: 10.1002/adhm.201501033
Dubey, N., Ferreira, J. A., Malda, J., Bhaduri, S. B., and Bottino, M. C. (2020). Extracellular matrix/amorphous magnesium phosphate bioink for 3D bioprinting of Craniomaxillofacial bone tissue. ACS Appl. Mater. Interfaces 12, 23752–23763. doi: 10.1021/acsami.0c05311
Ducheyne, P., and Qiu, Q. (1999). Bioactive ceramics: the effect of surface reactivity on bone formation and bone cell function. Biomaterials 20, 2287–2303. doi: 10.1016/s0142-9612(99)00181-7
Dunn, C. A., Jin, Q., Taba, M., Franceschi, R. T., Bruce Rutherford, R., et al. (2005). BMP gene delivery for alveolar bone engineering at dental implant defects. Mol. Ther. 11, 294–299. doi: 10.1016/j.ymthe.2004.10.005
D’Urso, P. S., Barker, T. M., Earwaker, W. J., Bruce, L. J., Atkinson, R. L., Lanigan, M. W., et al. (1999). Stereolithographic biomodelling in cranio-maxillofacial surgery: a prospective trial. J. Craniomaxillofac. Surg. 27, 30–37. doi: 10.1016/s1010-5182(99)80007-9
Egusa, H., Sonoyama, W., Nishimura, M., Atsuta, I., and Akiyama, K. (2012). Stem cells in dentistry–part I: stem cell sources. J. Prosthodont. Res. 56, 151–165. doi: 10.1016/j.jpor.2012.06.001
Engler, A. J., Sen, S., Sweeney, H. L., and Discher, D. E. (2006). Matrix elasticity directs stem cell lineage specification. Cell 126, 677–689. doi: 10.1016/j.cell.2006.06.044
Erisken, C., Kalyon, D. M., and Wang, H. (2008). Functionally graded electrospun polycaprolactone and beta-tricalcium phosphate nanocomposites for tissue engineering applications. Biomaterials 29, 4065–4073. doi: 10.1016/j.biomaterials.2008.06.022
Ersoy, A. E., Turkyilmaz, I., Ozan, O., and McGlumphy, E. A. (2008). Reliability of implant placement with stereolithographic surgical guides generated from computed tomography: clinical data from 94 implants. J. Periodontol. 79, 1339–1345. doi: 10.1902/jop.2008.080059
Eskan, M. A., Girouard, M. E., Morton, D., and Greenwell, H. (2017). The effect of membrane exposure on lateral ridge augmentation: a case-controlled study. Int. J. Implant Dent. 3:26.
Fang, J., Zhu, Y. Y., Smiley, E., Bonadio, J., Rouleau, J. P., Goldstein, S. A., et al. (1996). Stimulation of new bone formation by direct transfer of osteogenic plasmid genes. Proc. Natl. Acad. Sci. U. S. A. 93, 5753–5758. doi: 10.1073/pnas.93.12.5753
Feine, J., Abou-Ayash, S., Al Mardini, M., de Santana, R. B., Bjelke-Holtermann, T., Bornstein, M. M., et al. (2018). Group 3 ITI consensus report: patient-reported outcome measures associated with implant dentistry. Clin. Oral Implants Res. 29(Suppl. 16), 270–275. doi: 10.1111/clr.13299
Feng, K., Sun, H., Bradley, M. A., Dupler, E. J., Giannobile, W. V., and Ma, P. X. (2010). Novel antibacterial nanofibrous PLLA scaffolds. J. Control. Release 146, 363–369. doi: 10.1016/j.jconrel.2010.05.035
Filippi, M., Born, G., Chaaban, M., and Scherberich, A. (2020). Natural polymeric scaffolds in bone regeneration. Front. Bioeng. Biotechnol. 8:474.
George, E., Liacouras, P., Rybicki, F. J., and Mitsouras, D. (2017). Measuring and establishing the accuracy and reproducibility of 3D printed medical models. Radiographics 37, 1424–1450. doi: 10.1148/rg.2017160165
Giannobile, W. V., Berglundh, T., Al-Nawas, B., Araujo, M., Bartold, P. M., Bouchard, P., et al. (2019). Biological factors involved in alveolar bone regeneration: consensus report of Working Group 1 of the 15(th) european workshop on periodontology on bone regeneration. J. Clin. Periodontol. 46(Suppl. 21), 6–11. doi: 10.1111/jcpe.13130
Giannobile, W. V., Jung, R. E., Schwarz, F., and Groups of the 2nd Osteology Foundation Consensus Meeting. (2018). Evidence-based knowledge on the aesthetics and maintenance of peri-implant soft tissues: osteology foundation consensus report Part 1-effects of soft tissue augmentation procedures on the maintenance of peri-implant soft tissue health. Clin. Oral Implants Res. 29, 7–10. doi: 10.1111/clr.13110
Giesen, E. B. W., Ding, M., Dalstra, M., and van Eijden, T. M. G. J. (2001). Mechanical properties of cancellous bone in the human mandibular condyle are anisotropic. J. Biomech. 34, 799–803. doi: 10.1016/s0021-9290(01)00030-6
Gillison, M. L., Chaturvedi, A. K., Anderson, W. F., and Fakhry, C. (2015). Epidemiology of human papillomavirus-positive head and neck squamous cell carcinoma. J. Clin. Oncol. 33, 3235–3242. doi: 10.1200/jco.2015.61.6995
Gladman, A. S., Matsumoto, E. A., Nuzzo, R. G., Mahadevan, L., and Lewis, J. A. (2016). Biomimetic 4D printing. Nat. Mater. 15, 413–418.
Goh, B. T., Teh, L. Y., Tan, D. B., Zhang, Z., and Teoh, S. H. (2015). Novel 3D polycaprolactone scaffold for ridge preservation–a pilot randomised controlled clinical trial. Clin. Oral Implants Res. 26, 271–277. doi: 10.1111/clr.12486
Goodson, J. M., Tanner, A. C., Haffajee, A. D., Sornberger, G. C., and Socransky, S. S. (1982). Patterns of progression and regression of advanced destructive periodontal disease. J. Clin. Periodontol. 9, 472–481. doi: 10.1111/j.1600-051x.1982.tb02108.x
Grasso, M., Demir, A. G., Previtali, B., and Colosimo, B. M. (2018). In situ monitoring of selective laser melting of zinc powder via infrared imaging of the process plume. Robot. Comput. Integr. Manuf. 49, 229–239. doi: 10.1016/j.rcim.2017.07.001
Grigoryan, B., Paulsen, S. J., Corbett, D. C., Sazer, D. W., Fortin, C. L., Zaita, A. J., et al. (2019). Multivascular networks and functional intravascular topologies within biocompatible hydrogels. Science 364, 458–464. doi: 10.1126/science.aav9750
Groll, J., Burdick, J. A., Cho, D.-W., Derby, B., Gelinsky, M., Heilshorn, S. C., et al. (2018). A definition of bioinks and their distinction from biomaterial inks. Biofabrication 11:013001. doi: 10.1088/1758-5090/aaec52
Grottkau, B. E., Noordin, S., Shortkroff, S., Schaffer, J. L., Thornhill, T. S., and Spector, M. (2002). Effect of mechanical perturbation on the release of PGE(2) by macrophages in vitro. J. Biomed. Mater. Res. 59, 288–293. doi: 10.1002/jbm.1244
Guvendiren, M., Molde, J., Soares, R. M., and Kohn, J. (2016). Designing biomaterials for 3D printing. ACS Biomater. Sci. Eng. 2, 1679–1693. doi: 10.1021/acsbiomaterials.6b00121
Hammarstrom, L., Heijl, L., and Gestrelius, S. (1997). Periodontal regeneration in a buccal dehiscence model in monkeys after application of enamel matrix proteins. J. Clin. Periodontol. 24, 669–677. doi: 10.1034/j.1600-051x.1997.00669.x
Han, C., Yao, Y., Cheng, X., Luo, J., Luo, P., Wang, Q., et al. (2017). Electrophoretic deposition of gentamicin-loaded silk fibroin coatings on 3D-printed porous cobalt–chromium–molybdenum bone substitutes to prevent orthopedic implant infections. Biomacromolecules 18, 3776–3787. doi: 10.1021/acs.biomac.7b01091
Han, J., Kim, D. S., Jang, H., Kim, H.-R., and Kang, H.-W. (2019). Bioprinting of three-dimensional dentin–pulp complex with local differentiation of human dental pulp stem cells. J. Tissue Eng. 10:2041731419845849.
Hao, J., Cheng, K. C., Kruger, L. G., Larsson, L., Sugai, J. V., Lahann, J., et al. (2016). Multigrowth factor delivery via immobilization of gene therapy vectors. Adv. Mater. 28, 3145–3151. doi: 10.1002/adma.201600027
He, W., Tian, K., Xie, X., Wang, X., Li, Y., and Li, Z. (2015). Individualized surgical templates and titanium microplates for Le Fort I osteotomy by computer-aided design and computer-aided manufacturing. J. Craniofac. Surg. 26, 1877–1881. doi: 10.1097/scs.0000000000001938
Hernández-Escobar, D., Champagne, S., Yilmazer, H., Dikici, B., Boehlert, C. J., and Hermawan, H. (2019). Current status and perspectives of zinc-based absorbable alloys for biomedical applications. Acta Biomater. 97, 1–22. doi: 10.1016/j.actbio.2019.07.034
Hollister, S. J., Lin, C. Y., Saito, E., Schek, R. D., Taboas, J. M., Williams, J. M., et al. (2005). Engineering craniofacial scaffolds. Orthod. Craniofac. Res. 8, 162–173.
Holmes, S. G., Still, K., Buttle, D. J., Bishop, N. J., and Grabowski, P. S. (2004). Chemically modified tetracyclines act through multiple mechanisms directly on osteoclast precursors. Bone 35, 471–478. doi: 10.1016/j.bone.2004.02.028
Horn, D., Engel, M., Bodem, J. P., Hoffmann, J., and Freudlsperger, C. (2012). Reconstruction of a near-total nasal defect using a precontoured titanium mesh with a converse scalping flap. J. Craniofac. Surg. 23, e410–e412.
Huang, K. H., Lin, Y. H., Shie, M. Y., and Lin, C. P. (2018). Effects of bone morphogenic protein-2 loaded on the 3D-printed MesoCS scaffolds. J. Formos. Med. Assoc. 117, 879–887. doi: 10.1016/j.jfma.2018.07.010
Huebsch, N., Arany, P. R., Mao, A. S., Shvartsman, D., Ali, O. A., Bencherif, S. A., et al. (2010). Harnessing traction-mediated manipulation of the cell/matrix interface to control stem-cell fate. Nat. Mater. 9, 518–526. doi: 10.1038/nmat2732
Huebsch, N., Lippens, E., Lee, K., Mehta, M., Koshy, S. T., Darnell, M. C., et al. (2015). Matrix elasticity of void-forming hydrogels controls transplanted-stem-cell-mediated bone formation. Nat. Mater. 14, 1269–1277. doi: 10.1038/nmat4407
Hui, P. W., Leung, P. C., and Sher, A. (1996). Fluid conductance of cancellous bone graft as a predictor for graft-host interface healing. J. Biomech. 29, 123–132. doi: 10.1016/0021-9290(95)00010-0
Hulbert, S. F., Young, F. A., Mathews, R. S., Klawitter, J. J., Talbert, C. D., and Stelling, F. H. (1970). Potential of ceramic materials as permanently implantable skeletal prostheses. J. Biomed. Mater. Res. 4, 433–456. doi: 10.1002/jbm.820040309
Ishaug, S. L., Crane, G. M., Miller, M. J., Yasko, A. W., Yaszemski, M. J., and Mikos, A. G. (1997). Bone formation by three-dimensional stromal osteoblast culture in biodegradable polymer scaffolds. J. Biomed. Mater. Res. 36, 17–28. doi: 10.1002/(sici)1097-4636(199707)36:1<17::aid-jbm3>3.0.co;2-o
Iwata, T., Yamato, M., Washio, K., Yoshida, T., Tsumanuma, Y., Yamada, A., et al. (2018). Periodontal regeneration with autologous periodontal ligament-derived cell sheets - a safety and efficacy study in ten patients. Regen. Ther. 9, 38–44. doi: 10.1016/j.reth.2018.07.002
Jacobs, C. A., and Lin, A. Y. (2017). A new classification of three-dimensional printing technologies: systematic review of three-dimensional printing for patient-specific craniomaxillofacial surgery. Plast. Reconstr. Surg. 139, 1211–1220. doi: 10.1097/prs.0000000000003232
Jain, S., and Darveau, R. P. (2010). Contribution of Porphyromonas gingivalis lipopolysaccharide to periodontitis. Periodontol 2000 54, 53–70. doi: 10.1111/j.1600-0757.2009.00333.x
Jakus, A. E., and Shah, R. N. (2017). Multi and mixed 3D-printing of graphene-hydroxyapatite hybrid materials for complex tissue engineering. J. Biomed. Mater. Res. A 105, 274–283. doi: 10.1002/jbm.a.35684
Jardini, A. L., Larosa, M. A., Maciel Filho, R., Zavaglia, C. A., Bernardes, L. F., Lambert, C. S., et al. (2014). Cranial reconstruction: 3D biomodel and custom-built implant created using additive manufacturing. J. Craniomaxillofac. Surg. 42, 1877–1884. doi: 10.1016/j.jcms.2014.07.006
Jensen, S. S., and Terheyden, H. (2009). Bone augmentation procedures in localized defects in the alveolar ridge: clinical results with different bone grafts and bone-substitute materials. Int. J. Oral Maxillofac. Implants 24(Suppl.), 218–236.
Jin, Q. M., Anusaksathien, O., Webb, S. A., Rutherford, R. B., and Giannobile, W. V. (2003). Gene therapy of bone morphogenetic protein for periodontal tissue engineering. J. Periodontol. 74, 202–213. doi: 10.1902/jop.2003.74.2.202
Jin, Q., Anusaksathien, O., Webb, S. A., Printz, M. A., and Giannobile, W. V. (2004). Engineering of tooth-supporting structures by delivery of PDGF gene therapy vectors. Mol. Ther. 9, 519–526. doi: 10.1016/j.ymthe.2004.01.016
Jin, Q., Wei, G., Lin, Z., Sugai, J. V., Lynch, S. E., Ma, P. X., et al. (2008). Nanofibrous scaffolds incorporating PDGF-BB microspheres induce chemokine expression and tissue neogenesis in vivo. PLoS One 3:e1729. doi: 10.1371/journal.pone.0001729
Kaigler, D., Avila-Ortiz, G., Travan, S., Taut, A. D., Padial-Molina, M., Rudek, I., et al. (2015). Bone engineering of maxillary sinus bone deficiencies using Enriched CD90+ Stem cell therapy: a randomized clinical trial. J. Bone Miner. Res. 30, 1206–1216. doi: 10.1002/jbmr.2464
Kaigler, D., Pagni, G., Park, C. H., Braun, T. M., Holman, L. A., Yi, E., et al. (2013). Stem cell therapy for craniofacial bone regeneration: a randomized, controlled feasibility trial. Cell Transplant. 22, 767–777. doi: 10.3727/096368912x652968
Kanno, Y., Nakatsuka, T., Saijo, H., Fujihara, Y., Atsuhiko, H., Chung, U., et al. (2016). Computed tomographic evaluation of novel custom-made artificial bones, “CT-bone”, applied for maxillofacial reconstruction. Regen. Ther. 5, 1–8. doi: 10.1016/j.reth.2016.05.002
Kao, R. T., Nares, S., and Reynolds, M. A. (2015). Periodontal regeneration - intrabony defects: a systematic review from the AAP regeneration Workshop. J. Periodontol. 86, S77–S104.
Karageorgiou, V., and Kaplan, D. (2005). Porosity of 3D biomaterial scaffolds and osteogenesis. Biomaterials 26, 5474–5491. doi: 10.1016/j.biomaterials.2005.02.002
Keriquel, V., Guillemot, F., Arnault, I., Guillotin, B., Miraux, S., Amédée, J., et al. (2010). In vivo bioprinting for computer- and robotic-assisted medical intervention: preliminary study in mice. Biofabrication 2:014101. doi: 10.1088/1758-5082/2/1/014101
Keriquel, V., Oliveira, H., Rémy, M., Ziane, S., Delmond, S., Rousseau, B., et al. (2017). In situ printing of mesenchymal stromal cells, by laser-assisted bioprinting, for in vivo bone regeneration applications. Sci. Rep. 7:1778.
Khang, D., Choi, J., Im, Y. M., Kim, Y. J., Jang, J. H., Kang, S. S., et al. (2012). Role of subnano-, nano- and submicron-surface features on osteoblast differentiation of bone marrow mesenchymal stem cells. Biomaterials 33, 5997–6007. doi: 10.1016/j.biomaterials.2012.05.005
Kim, J. Y., Ahn, G., Kim, C., Lee, J. S., Lee, I. G., An, S. H., et al. (2018). Synergistic effects of beta tri-calcium phosphate and porcine-derived decellularized bone extracellular matrix in 3D-printed polycaprolactone scaffold on bone regeneration. Macromol. Biosci. 18:e1800025.
Kim, K., Lee, C. H., Kim, B. K., and Mao, J. J. (2010). Anatomically shaped tooth and periodontal regeneration by cell homing. J. Dent. Res. 89, 842–847. doi: 10.1177/0022034510370803
Kinaci, A., Neuhaus, V., and Ring, D. C. (2014). Trends in bone graft use in the United States. Orthopedics 37, e783–e788.
Kitamura, M., Akamatsu, M., Kawanami, M., Furuichi, Y., Fujii, T., Mori, M., et al. (2016). Randomized placebo-controlled and controlled non-inferiority Phase III trials comparing trafermin, a recombinant human fibroblast growth Factor 2, and enamel matrix derivative in periodontal regeneration in intrabony defects. J. Bone Miner. Res. 31, 806–814. doi: 10.1002/jbmr.2738
Klein, F., Kim, T. S., Hassfeld, S., Staehle, H. J., Reitmeir, P., Holle, R., et al. (2001). Radiographic defect depth and width for prognosis and description of periodontal healing of infrabony defects. J. Periodontol. 72, 1639–1646. doi: 10.1902/jop.2001.72.12.1639
Korioth, T. W., and Hannam, A. G. (1994). Deformation of the human mandible during simulated tooth clenching. J. Dent. Res. 73, 56–66. doi: 10.1177/00220345940730010801
Korioth, T. W., Romilly, D. P., and Hannam, A. G. (1992). Three-dimensional finite element stress analysis of the dentate human mandible. Am. J. Phys. Anthropol. 88, 69–96. doi: 10.1002/ajpa.1330880107
Kornman, K. S. (2008). Mapping the pathogenesis of periodontitis: a new look. J. Periodontol. 79, 1560–1568. doi: 10.1902/jop.2008.080213
Kornman, K. S., Giannobile, W. V., and Duff, G. W. (2017). Quo vadis: what is the future of periodontics? how will we get there? Periodontol. 2000 75, 353–371. doi: 10.1111/prd.12217
Kumar, S., Nehra, M., Kedia, D., Dilbaghi, N., Tankeshwar, K., and Kim, K. H. (2020). Nanotechnology-based biomaterials for orthopaedic applications: recent advances and future prospects. Mater. Sci. Eng. C Mater. Biol. Appl. 106:110154. doi: 10.1016/j.msec.2019.110154
Lahann, J., Klee, D., Pluester, W., and Hoecker, H. (2001). Bioactive immobilization of r-hirudin on CVD-coated metallic implant devices. Biomaterials 22, 817–826. doi: 10.1016/s0142-9612(00)00244-1
Larsson, L., Castilho, R. M., and Giannobile, W. V. (2015). Epigenetics and its role in periodontal diseases: a state-of-the-art review. J. Periodontol. 86, 556–568. doi: 10.1902/jop.2014.140559
Larsson, L., Decker, A. M., Nibali, L., Pilipchuk, S. P., Berglundh, T., and Giannobile, W. V. (2016). Regenerative medicine for periodontal and peri-implant diseases. J. Dent. Res. 95, 255–266. doi: 10.1177/0022034515618887
Lee, N., Robinson, J., and Lu, H. (2016). Biomimetic strategies for engineering composite tissues. Curr. Opin. Biotechnol. 40, 64–74. doi: 10.1016/j.copbio.2016.03.006
Leong, K. F., Cheah, C. M., and Chua, C. K. (2003). Solid freeform fabrication of three-dimensional scaffolds for engineering replacement tissues and organs. Biomaterials 24, 2363–2378. doi: 10.1016/s0142-9612(03)00030-9
Lethaus, B., Poort, L., Böckmann, R., Smeets, R., Tolba, R., and Kessler, P. (2012). Additive manufacturing for microvascular reconstruction of the mandible in 20 patients. J. Craniomaxillofac. Surg. 40, 43–46. doi: 10.1016/j.jcms.2011.01.007
Lettry, S., Seedhom, B., Berry, E., and Cuppone, M. (2003). Quality assessment of the cortical bone of the human mandible. Bone 32, 35–44. doi: 10.1016/s8756-3282(02)00921-3
Levine, J. P., Patel, A., Saadeh, P. B., and Hirsch, D. L. (2012). Computer-aided design and manufacturing in craniomaxillofacial surgery: the new state of the art. J. Craniofac. Surg. 23, 288–293. doi: 10.1097/scs.0b013e318241ba92
Li, X., Liu, B., Pei, B., Chen, J., Zhou, D., Peng, J., et al. (2020). Inkjet bioprinting of biomaterials. Chem. Rev. 120, 10793–10833. doi: 10.1021/acs.chemrev.0c00008
Li, Y., Zhou, J., Pavanram, P., Leeflang, M. A., Fockaert, L. I., Pouran, B., et al. (2018). Additively manufactured biodegradable porous magnesium. Acta Biomater. 67, 378–392.
Li, Z., Mei, S., Dong, Y., She, F., Li, Y., Li, P., et al. (2020). Functional nanofibrous biomaterials of tailored structures for drug delivery-a critical review. Pharmaceutics 12:522. doi: 10.3390/pharmaceutics12060522
Liang, C., Chen, J., Zhang, Y., Wei, F., Ling, Y., and Li, X. (2021). Construction of novel antimicrobial peptide-modified extracellular matrix biologic scaffold material. Biochem. Biophys. Res. Commun. 546, 162–168. doi: 10.1016/j.bbrc.2021.02.002
Ligon, S. C., Liska, R., Stampfl, J., Gurr, M., and Mülhaupt, R. (2017). Polymers for 3D printing and customized additive manufacturing. Chem. Rev. 117, 10212–10290.
Lim, K. S., Levato, R., Costa, P. F., Castilho, M. D., Alcala-Orozco, C. R., Van Dorenmalen, K. M., et al. (2018). Bio-resin for high resolution lithography-based biofabrication of complex cell-laden constructs. Biofabrication 10:034101. doi: 10.1088/1758-5090/aac00c
Lim, T. C., Chian, K. S., and Leong, K. F. (2010). Cryogenic prototyping of chitosan scaffolds with controlled micro and macro architecture and their effect on in vivo neo-vascularization and cellular infiltration. J. Biomed. Mater. Res. A 94, 1303–1311.
Lin, Y. H., Chiu, Y. C., Shen, Y. F., Wu, Y. A., and Shie, M. Y. (2017). Bioactive calcium silicate/poly-ε-caprolactone composite scaffolds 3D printed under mild conditions for bone tissue engineering. J. Mater. Sci. Mater. Med. 29:11.
Liu, T., Liu, L., Zeng, C., Liu, Y., and Leng, J. (2020). 4D printed anisotropic structures with tailored mechanical behaviors and shape memory effects. Compos. Sci. Technol. 186:107935. doi: 10.1016/j.compscitech.2019.107935
Liu, Y., Ma, Y., Zhang, J., Xie, Q., Wang, Z., Yu, S., et al. (2017). MBG-modified β-TCP scaffold promotes mesenchymal stem cells adhesion and osteogenic differentiation via a FAK/MAPK signaling pathway. ACS Appl. Mater. Interfaces 9, 30283–30296. doi: 10.1021/acsami.7b02466
Longoni, S., Tinto, M., Pacifico, C., Sartori, M., and Andreano, A. (2019). Effect of peri-implant keratinized tissue width on tissue health and stability: systematic review and meta-analysis. Int. J. Oral Maxillofac. Implants 34, 1307–1317. doi: 10.11607/jomi.7622
Ma, J., Ma, L., Wang, Z., Zhu, X., and Wang, W. (2017). The use of 3D-printed titanium mesh tray in treating complex comminuted mandibular fractures: a case report. Medicine 96:e7250. doi: 10.1097/md.0000000000007250
Ma, X., Qu, X., Zhu, W., Li, Y.-S., Yuan, S., Zhang, H., et al. (2016). Deterministically patterned biomimetic human iPSC-derived hepatic model via rapid 3D bioprinting. Proc. Natl. Acad. Sci. U. S. A. 113, 2206–2211. doi: 10.1073/pnas.1524510113
Manodh, P., Prabhu Shankar, D., Pradeep, D., Santhosh, R., and Murugan, A. (2016). Incidence and patterns of maxillofacial trauma-a retrospective analysis of 3611 patients-an update. Oral Maxillofac. Surg. 20, 377–383. doi: 10.1007/s10006-016-0576-z
Marcenes, W., Kassebaum, N. J., Bernabe, E., Flaxman, A., Naghavi, M., Lopez, A., et al. (2013). Global burden of oral conditions in 1990-2010: a systematic analysis. J. Dent. Res. 92, 592–597. doi: 10.1177/0022034513490168
Markets and Markets (2020). Dental Bone Graft Substitute Market by Type (Synthetic Bone Grafts, Xenograft, Allograft, Alloplast), Application (Sinus Lift, Ridge Augmentation, Socket Preservation), Product (Bio-OSS, OsteoGraf, Grafton), End User (Hospital)- Global Forecast to 2025 [Online]. Available online at: https://www.marketsandmarkets.com/Market-Reports/dental-bone-graft-substitutes-market-159678690.html (accessed March 25, 2021).
Matuliene, G., Pjetursson, B. E., Salvi, G. E., Schmidlin, K., Bragger, U., Zwahlen, M., et al. (2008). Influence of residual pockets on progression of periodontitis and tooth loss: results after 11 years of maintenance. J. Clin. Periodontol. 35, 685–695. doi: 10.1111/j.1600-051x.2008.01245.x
McGuire, M. K., and Scheyer, E. T. (2007). A randomized, double-blind, placebo-controlled study to determine the safety and efficacy of cultured and expanded autologous fibroblast injections for the treatment of interdental papillary insufficiency associated with the papilla priming procedure. J. Periodontol. 78, 4–17. doi: 10.1902/jop.2007.060105
McGuire, M. K., Scheyer, E. T., and Schupbach, P. (2016). A Prospective, case-controlled study evaluating the use of enamel matrix derivative on human buccal recession defects: a human histologic examination. J. Periodontol. 87, 645–653. doi: 10.1902/jop.2016.150459
McGuire, M. K., Scheyer, E. T., Lipton, D. I., and Gunsolley, J. C. (2020). Randomized, controlled clinical trial to evaluate a xenogeneic collagen matrix as an alternative to free gingival grafting for oral soft tissue augmentation: 6 to 8 year follow-up. J. Periodontol. doi: 10.1002/JPER.20-0627 [Online ahead of print].
Melcher, A. H. (1976). On the repair potential of periodontal tissues. J. Periodontol. 47, 256–260. doi: 10.1902/jop.1976.47.5.256
Menezes, F. D. S., Fernandes, G. A., Antunes, J. L. F., Villa, L. L., and Toporcov, T. N. (2021). Global incidence trends in head and neck cancer for HPV-related and -unrelated subsites: a systematic review of population-based studies. Oral Oncol. 115:105177. doi: 10.1016/j.oraloncology.2020.105177
Mikos, A. G. and Temenoff, J. S. (2000). Formation of highly porous biodegradable scaffolds for tissue engineering. Electronic J. Biotechnol. 3, 114–119.
Miller, P. D. (1985). A classification of marginal tissue recession. Int. J. Periodontics Restorative Dent. 5, 8–13.
Misch, C. E., Qu, Z., and Bidez, M. W. (1999). Mechanical properties of trabecular bone in the human mandible: implications for dental implant treatment planning and surgical placement. J. Oral Maxillofac. Surg. 57, 700–706. doi: 10.1016/s0278-2391(99)90437-8
Monje, A., Pons, R., Insua, A., Nart, J., Wang, H. L., and Schwarz, F. (2019). Morphology and severity of peri-implantitis bone defects. Clin. Implant. Dent. Relat. Res. 21, 635–643.
Moreno Rodriguez, J. A., Ortiz Ruiz, A. J., and Caffesse, R. G. (2019). Supra-alveolar attachment gain in the treatment of combined intra-suprabony periodontal defects by non-incised papillae surgical approach. J. Clin. Periodontol. 46, 927–936. doi: 10.1111/jcpe.13158
Mota, C., Puppi, D., Chiellini, F., and Chiellini, E. (2015). Additive manufacturing techniques for the production of tissue engineering constructs. J. Tissue Eng. Regen. Med. 9, 174–190. doi: 10.1002/term.1635
Msallem, B., Sharma, N., Cao, S., Halbeisen, F. S., Zeilhofer, H. F., and Thieringer, F. M. (2020). Evaluation of the dimensional accuracy of 3D-printed anatomical mandibular models using FFF, SLA, SLS, MJ, and BJ printing technology. J. Clin. Med. 9:817. doi: 10.3390/jcm9030817
Muzaffar, J., Bari, S., Kirtane, K., and Chung, C. H. (2021). Recent advances and future directions in clinical management of head and neck squamous cell carcinoma. Cancers 13:338. doi: 10.3390/cancers13020338
Nair, A. K., Gautieri, A., Chang, S. W., and Buehler, M. J. (2013). Molecular mechanics of mineralized collagen fibrils in bone. Nat. Commun. 4:1724.
Naveh, G., Brumfeld, V., Shahar, R., and Weiner, S. (2013). Tooth periodontal ligament: direct 3D microCT visualization of the collagen network and how the network changes when the tooth is loaded. J. Struct. Biol. 181, 108–115. doi: 10.1016/j.jsb.2012.10.008
Nehrer, S., Breinan, A., Ramappa, A., Young, G., Shortkroff, S., Louie, L., et al. (1997). Matrix collagen type and pore size influence behaviour of seeded canine chondrocytes. Biomaterials 18, 769–776. doi: 10.1016/s0142-9612(97)00001-x
Nevins, M., Camelo, M., Nevins, M. L., Schenk, R. K., and Lynch, S. E. (2003). Periodontal regeneration in humans using recombinant human platelet-derived growth factor-BB (rhPDGF-BB) and allogenic bone. J. Periodontol. 74, 1282–1292. doi: 10.1902/jop.2003.74.9.1282
Nevins, M., Giannobile, W. V., McGuire, M. K., Kao, R. T., Mellonig, J. T., Hinrichs, J. E., et al. (2005). Platelet-derived growth factor stimulates bone fill and rate of attachment level gain: results of a large multicenter randomized controlled trial. J. Periodontol. 76, 2205–2215. doi: 10.1902/jop.2005.76.12.2205
Nevins, M., Kao, R. T., McGuire, M. K., McClain, P. K., Hinrichs, J. E., McAllister, B. S., et al. (2013). Platelet-derived growth factor promotes periodontal regeneration in localized osseous defects: 36-month extension results from a randomized, controlled, double-masked clinical trial. J. Periodontol. 84, 456–464. doi: 10.1902/jop.2012.120141
Nyberg, E., Rindone, A., Dorafshar, A., and Grayson, W. L. (2017). Comparison of 3D-Printed Poly-ϵ-caprolactone scaffolds functionalized with tricalcium phosphate, hydroxyapatite, Bio-Oss, or decellularized bone matrix. Tissue Eng. Part A 23, 503–514. doi: 10.1089/ten.tea.2016.0418
Obregon, F., Vaquette, C., Ivanovski, S., Hutmacher, D., and Bertassoni, L. (2015). Three-dimensional bioprinting for regenerative dentistry and craniofacial tissue engineering. J. Dent. Res. 94, 143S–152S.
O’Connell, C. D., Di Bella, C., Thompson, F., Augustine, C., Beirne, S., Cornock, R., et al. (2016). Development of the Biopen: a handheld device for surgical printing of adipose stem cells at a chondral wound site. Biofabrication 8:015019. doi: 10.1088/1758-5090/8/1/015019
Ojansivu, M., Rashad, A., Ahlinder, A., Massera, J., Mishra, A., Syverud, K., et al. (2019). Wood-based nanocellulose and bioactive glass modified gelatin–alginate bioinks for 3D bioprinting of bone cells. Biofabrication 11:035010. doi: 10.1088/1758-5090/ab0692
Orr, T. E., Villars, P. A., Mitchell, S. L., Hsu, H. P., and Spector, M. (2001). Compressive properties of cancellous bone defects in a rabbit model treated with particles of natural bone mineral and synthetic hydroxyapatite. Biomaterials 22, 1953–1959. doi: 10.1016/s0142-9612(00)00370-7
Oshima, M., and Tsuji, T. (2015). Whole tooth regeneration as a future dental treatment. Adv. Exp. Med. Biol. 881, 255–269. doi: 10.1007/978-3-319-22345-2_14
Oshima, M., Mizuno, M., Imamura, A., Ogawa, M., Yasukawa, M., Yamazaki, H., et al. (2011). Functional tooth regeneration using a bioengineered tooth unit as a mature organ replacement regenerative therapy. PLoS One 6:e21531. doi: 10.1371/journal.pone.0021531
Oshima, M., Ogawa, M., and Tsuji, T. (2017). Functional tooth regeneration. Methods Mol. Biol. 1597, 97–116. doi: 10.1007/978-1-4939-6949-4_8
Ozbolat, I. T., and Hospodiuk, M. (2016). Current advances and future perspectives in extrusion-based bioprinting. Biomaterials 76, 321–343. doi: 10.1016/j.biomaterials.2015.10.076
Page, R. C., and Kornman, K. S. (1997). The pathogenesis of human periodontitis: an introduction. Periodontol. 2000 14, 9–11. doi: 10.1111/j.1600-0757.1997.tb00189.x
Palmer, L., Newcomb, C., Kaltz, S., Spoerke, E., and Stupp, S. (2008). Biomimetic systems for hydroxyapatite mineralization inspired by bone and enamel. Chem. Rev. 108, 4754–4783. doi: 10.1021/cr8004422
Papapanou, P. N., and Wennstrom, J. L. (1991). The angular bony defect as indicator of further alveolar bone loss. J. Clin. Periodontol. 18, 317–322. doi: 10.1111/j.1600-051x.1991.tb00435.x
Papapanou, P. N., Sanz, M., Buduneli, N., Dietrich, T., Feres, M., Fine, D. H., et al. (2018). Periodontitis: consensus report of workgroup 2 of the 2017 world workshop on the classification of periodontal and peri-implant diseases and conditions. J. Periodontol. 89(Suppl. 1), S173–S182.
Park, C. H., Kim, K. H., Lee, Y. M., Giannobile, W. V., and Seol, Y. J. (2017). 3D printed, microgroove pattern-driven generation of oriented ligamentous architectures. Int. J. Mol. Sci. 18:1927. doi: 10.3390/ijms18091927
Park, C. H., Rios, H. F., Jin, Q., Bland, M. E., Flanagan, C. L., Hollister, S. J., et al. (2010). Biomimetic hybrid scaffolds for engineering human tooth-ligament interfaces. Biomaterials 31, 5945–5952. doi: 10.1016/j.biomaterials.2010.04.027
Park, C. H., Rios, H. F., Jin, Q., Sugai, J. V., Padial-Molina, M., Taut, A. D., et al. (2012). Tissue engineering bone-ligament complexes using fiber-guiding scaffolds. Biomaterials 33, 137–145. doi: 10.1016/j.biomaterials.2011.09.057
Park, C. H., Rios, H. F., Taut, A. D., Padial-Molina, M., Flanagan, C. L., Pilipchuk, S. P., et al. (2014). Image-based, fiber guiding scaffolds: a platform for regenerating tissue interfaces. Tissue Eng. Part C Methods 20, 533–542. doi: 10.1089/ten.tec.2013.0619
Park, E. K., Lim, J. Y., Yun, I. S., Kim, J. S., Woo, S. H., Kim, D. S., et al. (2016). Cranioplasty enhanced by three-dimensional printing: custom-made three-dimensional-printed titanium implants for skull defects. J. Craniofac. Surg. 27, 943–949. doi: 10.1097/scs.0000000000002656
Park, J. B. (2011). Effects of doxycycline, minocycline, and tetracycline on cell proliferation, differentiation, and protein expression in osteoprecursor cells. J. Craniofac. Surg. 22, 1839–1842. doi: 10.1097/SCS.0b013e31822e8216
Park, J. H., Gillispie, G. J., Copus, J. S., Zhang, W., Atala, A., Yoo, J. J., et al. (2020). The effect of BMP-mimetic peptide tethering bioinks on the differentiation of dental pulp stem cells (DPSCs) in 3D bioprinted dental constructs. Biofabrication 12:035029. doi: 10.1088/1758-5090/ab9492
Park, J. Y., Park, C. H., Yi, T., Kim, S. N., Iwata, T., and Yun, J. H. (2020). rhBMP-2 pre-treated human periodontal ligament stem cell sheets regenerate a mineralized layer mimicking dental cementum. Int. J. Mol. Sci. 21:3767. doi: 10.3390/ijms21113767
Paschalis, E. P., Recker, R., DiCarlo, E., Doty, S. B., Atti, E., and Boskey, A. L. (2003). Distribution of collagen cross-links in normal human trabecular bone. J. Bone Miner. Res. 18, 1942–1946. doi: 10.1359/jbmr.2003.18.11.1942
Pati, F., Song, T. H., Rijal, G., Jang, J., Kim, S. W., and Cho, D. W. (2015). Ornamenting 3D printed scaffolds with cell-laid extracellular matrix for bone tissue regeneration. Biomaterials 37, 230–241. doi: 10.1016/j.biomaterials.2014.10.012
Pérez-Pérez, M. P., Gómez, E., and Sebastián, M. A. (2018). Delphi prospection on additive manufacturing in 2030: implications for education and employment in Spain. Materials 11:1500. doi: 10.3390/ma11091500
Peterson, J., Wang, Q., and Dechow, P. C. (2006). Material properties of the dentate maxilla. Anat. Rec. A Discov. Mol. Cell Evol. Biol. 288, 962–972. doi: 10.1002/ar.a.20358
Pihlstrom, B. L., Michalowicz, B. S., and Johnson, N. W. (2005). Periodontal diseases. Lancet 366, 1809–1820.
Pilipchuk, S. P., Fretwurst, T., Yu, N., Larsson, L., Kavanagh, N. M., Asa’ad, F., et al. (2018). Micropatterned scaffolds with immobilized growth factor genes regenerate bone and periodontal ligament-like tissues. Adv. Healthc. Mater. 7:e1800750.
Pilipchuk, S. P., Monje, A., Jiao, Y., Hao, J., Kruger, L., Flanagan, C. L., et al. (2016). Integration of 3D printed and micropatterned polycaprolactone scaffolds for guidance of oriented collagenous tissue formation in vivo. Adv. Healthc. Mater. 5, 676–687. doi: 10.1002/adhm.201500758
Porta, M., Tonda-Turo, C., Pierantozzi, D., Ciardelli, G., and Mancuso, E. (2020). Towards 3D multi-layer scaffolds for periodontal tissue engineering applications: addressing manufacturing and architectural challenges. Polymers 12:2233. doi: 10.3390/polym12102233
Puppi, D., Chiellini, F., PIras, A. M., and Chiellini, E. (2010). Polymeric materials for bone and cartilage repair. Prog. Polym. Sci. 35, 403–440. doi: 10.1016/j.progpolymsci.2010.01.006
Rasperini, G., Pilipchuk, S. P., Flanagan, C. L., Park, C. H., Pagni, G., Hollister, S. J., et al. (2015). 3D-printed bioresorbable scaffold for periodontal repair. J. Dent. Res. 94, 153S–157S.
Raveendran, N. T., Vaquette, C., Meinert, C., Ipe, D. S., and Ivanovski, S. (2019). Optimization of 3D bioprinting of periodontal ligament cells. Dent. Mater. 35, 1683–1694. doi: 10.1016/j.dental.2019.08.114
Reynolds, M. A., Kao, R. T., Nares, S., Camargo, P. M., Caton, J. G., Clem, D. S., et al. (2015). Periodontal regeneration - intrabony defects: practical applications from the AAP regeneration workshop. Clin. Adv. Periodontics 5, 21–29. doi: 10.1902/cap.2015.140062
Rodrigo, J. P., Heideman, D. A., García-Pedrero, J. M., Fresno, M. F., Brakenhoff, R. H., Díaz Molina, J. P., et al. (2014). Time trends in the prevalence of HPV in oropharyngeal squamous cell carcinomas in northern Spain (1990-2009). Int. J. Cancer 134, 487–492. doi: 10.1002/ijc.28355
Roosa, S. M., Kemppainen, J. M., Moffitt, E. N., Krebsbach, P. H., and Hollister, S. J. (2010). The pore size of polycaprolactone scaffolds has limited influence on bone regeneration in an in vivo model. J. Biomed. Mater. Res. A 92, 359–368. doi: 10.1002/jbm.a.32381
Saijo, H., Igawa, K., Kanno, Y., Mori, Y., Kondo, K., Shimizu, K., et al. (2009). Maxillofacial reconstruction using custom-made artificial bones fabricated by inkjet printing technology. J. Artif. Organs 12, 200–205. doi: 10.1007/s10047-009-0462-7
Salgado, A. J., Coutinho, O. P., and Reis, R. L. (2004). Bone tissue engineering: state of the art and future trends. Macromol. Biosci. 4, 743–765. doi: 10.1002/mabi.200400026
Sanchez, N., Fierravanti, L., Nunez, J., Vignoletti, F., Gonzalez-Zamora, M., Santamaria, S., et al. (2020). Periodontal regeneration using a xenogeneic bone substitute seeded with autologous periodontal ligament-derived mesenchymal stem cells: a 12-month quasi-randomized controlled pilot clinical trial. J. Clin. Periodontol. 47, 1391–1402. doi: 10.1111/jcpe.13368
Sandino, C., and Lacroix, D. (2011). A dynamical study of the mechanical stimuli and tissue differentiation within a CaP scaffold based on micro-CT finite element models. Biomech. Model. Mechanobiol. 10, 565–576. doi: 10.1007/s10237-010-0256-0
Sangkert, S., Meesane, J., Kamonmattayakul, S., and Chai, W. (2016). Modified silk fibroin scaffolds with collagen/decellularized pulp for bone tissue engineering in cleft palate: morphological structures and biofunctionalities. Mater. Sci. Eng. C 58, 1138–1149. doi: 10.1016/j.msec.2015.09.031
Saska, S., Pilatti, L., Blay, A., and Shibli, J. A. (2021). Bioresorbable polymers: advanced materials and 4d printing for tissue engineering. Polymers 13:563. doi: 10.3390/polym13040563
Schincaglia, G. P., Hebert, E., Farina, R., Simonelli, A., and Trombelli, L. (2015). Single versus double flap approach in periodontal regenerative treatment. J. Clin. Periodontol. 42, 557–566. doi: 10.1111/jcpe.12409
Schliephake, H. (2010). Application of bone growth factors–the potential of different carrier systems. Oral Maxillofac. Surg. 14, 17–22. doi: 10.1007/s10006-009-0185-1
Sculean, A., Chapple, I. L., and Giannobile, W. V. (2015a). Wound models for periodontal and bone regeneration: the role of biologic research. Periodontol. 2000 68, 7–20. doi: 10.1111/prd.12091
Sculean, A., Nikolidakis, D., and Schwarz, F. (2008). Regeneration of periodontal tissues: combinations of barrier membranes and grafting materials - biological foundation and preclinical evidence: a systematic review. J. Clin. Periodontol. 35, 106–116. doi: 10.1111/j.1600-051x.2008.01263.x
Sculean, A., Nikolidakis, D., Nikou, G., Ivanovic, A., Chapple, I. L., and Stavropoulos, A. (2015b). Biomaterials for promoting periodontal regeneration in human intrabony defects: a systematic review. Periodontol. 2000 68, 182–216. doi: 10.1111/prd.12086
Seibert, J. S. (1983). Reconstruction of deformed, partially edentulous ridges, using full thickness onlay grafts. Part I. technique and wound healing. Compend. Contin. Educ. Dent. 4, 437–453.
Seibert, J. S., and Salama, H. (1996). Alveolar ridge preservation and reconstruction. Periodontol 2000 11, 69–84. doi: 10.1111/j.1600-0757.1996.tb00185.x
Seo, B.-M., Miura, M., Gronthos, S., Bartold, P. M., Batouli, S., Brahim, J., et al. (2004). Investigation of multipotent postnatal stem cells from human periodontal ligament. Lancet 364, 149–155. doi: 10.1016/s0140-6736(04)16627-0
Shah, N. J., Hyder, M. N., Quadir, M. A., Dorval Courchesne, N.-M., Seeherman, H. J., Nevins, M., et al. (2014). Adaptive growth factor delivery from a polyelectrolyte coating promotes synergistic bone tissue repair and reconstruction. Proc. Natl. Acad. Sci. U. S. A. 111, 12847–12852. doi: 10.1073/pnas.1408035111
Shim, J.-H., Kim, M.-J., Park, J. Y., Pati, R. G., Yun, Y.-P., Kim, S. E., et al. (2015). Three-dimensional printing of antibiotics-loaded poly-ε-caprolactone/poly(lactic-co-glycolic acid) scaffolds for treatment of chronic osteomyelitis. Tissue Eng. Regen. Med. 12, 283–293. doi: 10.1007/s13770-015-0014-6
Skylar-Scott, M. A., Uzel, S. G., Nam, L. L., Ahrens, J. H., Truby, R. L., Damaraju, S., et al. (2019). Biomanufacturing of organ-specific tissues with high cellular density and embedded vascular channels. Sci. Adv. 5:eaaw2459. doi: 10.1126/sciadv.aaw2459
Smith, P. C., Martinez, C., Caceres, M., and Martinez, J. (2015). Research on growth factors in periodontology. Periodontol. 2000 67, 234–250. doi: 10.1111/prd.12068
Socransky, S. S., Haffajee, A. D., Goodson, J. M., and Lindhe, J. (1984). New concepts of destructive periodontal disease. J. Clin. Periodontol. 11, 21–32. doi: 10.1111/j.1600-051x.1984.tb01305.x
Soldatos, N. K., Stylianou, P., Koidou, V. P., Angelov, N., Yukna, R., and Romanos, G. E. (2017). Limitations and options using resorbable versus nonresorbable membranes for successful guided bone regeneration. Quintessence Int. 48, 131–147.
Spalazzi, J. P., Dagher, E., Doty, S. B., Guo, X. E., Rodeo, S. A., and Lu, H. H. (2006a). In vivo evaluation of a tri-phasic composite scaffold for anterior cruciate ligament-to-bone integration. Conf. Proc. IEEE Eng. Med. Biol. Soc. 2006, 525–528.
Spalazzi, J. P., Dagher, E., Doty, S. B., Guo, X. E., Rodeo, S. A., and Lu, H. H. (2008). In vivo evaluation of a multiphased scaffold designed for orthopaedic interface tissue engineering and soft tissue-to-bone integration. J. Biomed. Mater. Res. A 86, 1–12. doi: 10.1002/jbm.a.32073
Spalazzi, J. P., Doty, S. B., Moffat, K. L., Levine, W. N., and Lu, H. H. (2006b). Development of controlled matrix heterogeneity on a triphasic scaffold for orthopedic interface tissue engineering. Tissue Eng. 12, 3497–3508. doi: 10.1089/ten.2006.12.3497
Spector, M. (1994). Anorganic bovine bone and ceramic analogs of bone mineral as implants to facilitate bone regeneration. Clin. Plast. Surg. 21, 437–444. doi: 10.1016/s0094-1298(20)31021-x
Spencer, L. J., Degu, A., Kalkidan, H. A., Solomon, M. A., Cristiana, A., Nooshin, A., et al. (2018). Global, regional, and national incidence, prevalence, and years lived with disability for 354 diseases and injuries for 195 countries and territories, 1990-2017: a systematic analysis for the Global Burden of Disease Study 2017. Lancet 392, 1789–1858.
Suárez-López Del, Amo, F., Monje, A., Padial-Molina, M., Tang, Z., and Wang, H. L. (2015). Biologic agents for periodontal regeneration and implant site development. Biomed. Res. Int. 2015:957518.
Sudarmadji, N., Tan, J. Y., Leong, K. F., Chua, C. K., and Loh, Y. T. (2011). Investigation of the mechanical properties and porosity relationships in selective laser-sintered polyhedral for functionally graded scaffolds. Acta Biomater. 7, 530–537. doi: 10.1016/j.actbio.2010.09.024
Sudheesh Kumar, P. T., Hashimi, S., Saifzadeh, S., Ivanovski, S., and Vaquette, C. (2018). Additively manufactured biphasic construct loaded with BMP-2 for vertical bone regeneration: a pilot study in rabbit. Mater. Sci. Eng. C Mater. Biol. Appl. 92, 554–564. doi: 10.1016/j.msec.2018.06.071
Sugano, M., Negishi, Y., Endo-Takahashi, Y., Hamano, N., Usui, M., Suzuki, R., et al. (2014). Gene delivery to periodontal tissue using Bubble liposomes and ultrasound. J. Periodontal. Res. 49, 398–404. doi: 10.1111/jre.12119
Sumida, T., Otawa, N., Kamata, Y. U., Kamakura, S., Mtsushita, T., Kitagaki, H., et al. (2015). Custom-made titanium devices as membranes for bone augmentation in implant treatment: clinical application and the comparison with conventional titanium mesh. J. Craniomaxillofac. Surg. 43, 2183–2188. doi: 10.1016/j.jcms.2015.10.020
Sun, W., Starly, B., Nam, J., and Darling, A. (2005). Bio-CAD modeling and its applications in computer-aided tissue engineering. Comput. Aided Des. 37, 1097–1114. doi: 10.1016/j.cad.2005.02.002
Sunyer, R., Conte, V., Escribano, J., Elosegui-Artola, A., Labernadie, A., Valon, L., et al. (2016). Collective cell durotaxis emerges from long-range intercellular force transmission. Science 353, 1157–1161. doi: 10.1126/science.aaf7119
Swanson, W. B., Omi, M., Zhang, Z., Nam, H., Jung, Y., Wang, G., et al. (2021). Macropore design of tissue engineering scaffolds regulates mesenchymal stem cell differentiation fate. Biomaterials 272:120769. doi: 10.1016/j.biomaterials.2021.120769
Taboas, J. M., Maddox, R. D., Krebsbach, P. H., and Hollister, S. J. (2003). Indirect solid free form fabrication of local and global porous, biomimetic and composite 3D polymer-ceramic scaffolds. Biomaterials 24, 181–194. doi: 10.1016/s0142-9612(02)00276-4
Tao, O., Kort-Mascort, J., Lin, Y., Pham, H. M., Charbonneau, A. M., ElKashty, O. A., et al. (2019). The applications of 3D printing for craniofacial tissue engineering. Micromachines 10:480 doi: 10.3390/mi10070480
Tavelli, L., Barootchi, S., Avila-Ortiz, G., Urban, I. A., Giannobile, W. V., and Wang, H. L. (2021a). Peri-implant soft tissue phenotype modification and its impact on peri-implant health: a systematic review and network meta-analysis. J. Periodontol. 92, 21–44. doi: 10.1002/jper.19-0716
Tavelli, L., McGuire, M. K., Zucchelli, G., Rasperini, G., Feinberg, S. E., Wang, H. L., et al. (2020). Biologics-based regenerative technologies for periodontal soft tissue engineering. J. Periodontol. 91, 147–154. doi: 10.1002/jper.19-0352
Tavelli, L., Ravidà, A., Barootchi, S., Chambrone, L., and Giannobile, W. V. (2021b). Recombinant human platelet-derived growth factor: a systematic review of clinical findings in oral regenerative procedures. JDR Clin. Trans. Res. 6, 161–173. doi: 10.1177/2380084420921353
Tonetti, M. S., Christiansen, A. L., and Cortellini, P. (2017). Vertical subclassification predicts survival of molars with class II furcation involvement during supportive periodontal care. J. Clin. Periodontol. 44, 1140–1144. doi: 10.1111/jcpe.12789
Tsai, S. J., Ding, Y. W., Shih, M. C., and Tu, Y. K. (2020). Systematic review and sequential network meta-analysis on the efficacy of periodontal regenerative therapies. J. Clin. Periodontol. 47, 1108–1120. doi: 10.1111/jcpe.13338
Tse, J. R., and Engler, A. J. (2011). Stiffness gradients mimicking in vivo tissue variation regulate mesenchymal stem cell fate. PLoS One 6:e15978. doi: 10.1371/journal.pone.0015978
Tsuruga, E., Takita, H., Itoh, H., Wakisaka, Y., and Kuboki, Y. (1997). Pore size of porous hydroxyapatite as the cell-substratum controls BMP-induced osteogenesis. J. Biochem. 121, 317–324. doi: 10.1093/oxfordjournals.jbchem.a021589
Unterhofer, C., Wipplinger, C., Verius, M., Recheis, W., Thomé, C., and Ortler, M. (2017). Reconstruction of large cranial defects with poly-methyl-methacrylate (PMMA) using a rapid prototyping model and a new technique for intraoperative implant modeling. Neurol. Neurochir. Pol. 51, 214–220. doi: 10.1016/j.pjnns.2017.02.007
Urban, I. A., Montero, E., Monje, A., and Sanz-Sanchez, I. (2019). Effectiveness of vertical ridge augmentation interventions: a systematic review and meta-analysis. J. Clin. Periodontol. 46(Suppl. 21), 319–339. doi: 10.1111/jcpe.13061
Urban, I. A., Nagursky, H., and Lozada, J. L. (2011). Horizontal ridge augmentation with a resorbable membrane and particulated autogenous bone with or without anorganic bovine bone-derived mineral: a prospective case series in 22 patients. Int. J. Oral Maxillofac. Implants 26, 404–414.
Urban, I. A., Nagursky, H., Lozada, J. L., and Nagy, K. (2013). Horizontal ridge augmentation with a collagen membrane and a combination of particulated autogenous bone and anorganic bovine bone-derived mineral: a prospective case series in 25 patients. Int. J. Periodontics Restorative Dent. 33, 299–307. doi: 10.11607/prd.1407
Valvez, S., Reis, P. N. B., Susmel, L., and Berto, F. (2021). Fused filament fabrication-4D-printed shape memory polymers: a review. Polymers 13:701. doi: 10.3390/polym13050701
Van Lenthe, G. H., de Waal Malefijt, M. C., and Huiskes, R. (1997). Stress shielding after total knee replacement may cause bone resorption in the distal femur. J. Bone. Joint. Surg. Br. 79, 117–122. doi: 10.1302/0301-620x.79b1.0790117
Vaquette, C., Fan, W., Xiao, Y., Hamlet, S., Hutmacher, D. W., and Ivanovski, S. (2012). A biphasic scaffold design combined with cell sheet technology for simultaneous regeneration of alveolar bone/periodontal ligament complex. Biomaterials 33, 5560–5573. doi: 10.1016/j.biomaterials.2012.04.038
Vaquette, C., Mitchell, J., Fernandez-Medina, T., Kumar, S., and Ivanovski, S. (2021). Resorbable additively manufactured scaffold imparts dimensional stability to extraskeletally regenerated bone. Biomaterials 269:120671. doi: 10.1016/j.biomaterials.2021.120671
Vaquette, C., Pilipchuk, S. P., Bartold, P. M., Hutmacher, D. W., Giannobile, W. V., and Ivanovski, S. (2018). Tissue engineered constructs for periodontal regeneration: current status and future perspectives. Adv. Healthc. Mater. 7:e1800457.
Vert, M., Li, S. M., Spenlehauer, G., and Guerin, P. (1992). Bioresorbability and biocompatibility of aliphatic polyesters. J. Mater. Sci. Mater. Med. 3, 432–446. doi: 10.1007/bf00701240
Vining, K. H., and Mooney, D. J. (2017). Mechanical forces direct stem cell behaviour in development and regeneration. Nat. Rev. Mol. Cell Biol. 18, 728–742. doi: 10.1038/nrm.2017.108
von Wilmowsky, C., Schwertner, M. G., Nkenke, E., Moest, T., Adler, W., and Ebker, T. (2020). Use of CAD-based pre-bent implants reduces theatre time in orbital floor reconstruction: results of a prospective study. Br. J. Oral Maxillofac. Surg. 58, 753–758. doi: 10.1016/j.bjoms.2019.11.020
Wang, C. I., Cho, S. H., Cho, D., Ducote, C., Reddy, L. V., and Sinada, N. (2020). A 3D-printed guide to assist in sinus slot preparation for the optimization of zygomatic implant axis trajectory. J. Prosthodontics 29, 179–184. doi: 10.1111/jopr.13139
Wang, H. L., and Al-Shammari, K. (2002). HVC ridge deficiency classification: a therapeutically oriented classification. Int. J. Periodontics Restorative Dent. 22, 335–343.
Wang, X., Schwartz, Z., Gittens, R. A., Cheng, A., Olivares-Navarrete, R., Chen, H., et al. (2015). Role of integrin α2 β1 in mediating osteoblastic differentiation on three-dimensional titanium scaffolds with submicron-scale texture. J. Biomed. Mater. Res. A 103, 1907–1918. doi: 10.1002/jbm.a.35323
Wang, Y., and Ural, A. (2018). Effect of modifications in mineralized collagen fibril and extra-fibrillar matrix material properties on submicroscale mechanical behavior of cortical bone. J. Mech. Behav. Biomed. Mater. 82, 18–26. doi: 10.1016/j.jmbbm.2018.03.013
Wei, G., Jin, Q., Giannobile, W. V., and Ma, P. X. (2007). The enhancement of osteogenesis by nano-fibrous scaffolds incorporating rhBMP-7 nanospheres. Biomaterials 28, 2087–2096. doi: 10.1016/j.biomaterials.2006.12.028
Wen, P., Jauer, L., Voshage, M., Chen, Y., Poprawe, R., and Schleifenbaum, J. H. (2018). Densification behavior of pure Zn metal parts produced by selective laser melting for manufacturing biodegradable implants. J. Mater. Process. Technol. 258, 128–137. doi: 10.1016/j.jmatprotec.2018.03.007
Wu, Y. A., Chiu, Y. C., Lin, Y. H., Ho, C. C., Shie, M. Y., and Chen, Y. W. (2019). 3D-printed bioactive calcium Silicate/Poly-ε-Caprolactone bioscaffolds modified with biomimetic extracellular matrices for bone regeneration. Int. J. Mol. Sci. 20:942. doi: 10.3390/ijms20040942
Xia, Y., Zhou, P., Cheng, X., Xie, Y., Liang, C., Li, C., et al. (2013). Selective laser sintering fabrication of nano-hydroxyapatite/poly-ε-caprolactone scaffolds for bone tissue engineering applications. Int. J. Nanomed. 8, 4197–4213. doi: 10.2147/ijn.s50685
Xuan, K., Li, B., Guo, H., Sun, W., Kou, X., He, X., et al. (2018). Deciduous autologous tooth stem cells regenerate dental pulp after implantation into injured teeth. Sci. Transl. Med. 10:eaaf3227. doi: 10.1126/scitranslmed.aaf3227
Xue, J., Wu, T., Dai, Y., and Xia, Y. (2019). Electrospinning and electrospun nanofibers: methods, materials, and applications. Chem. Rev. 119, 5298–5415.
Yan, X., Chen, Y. R., Song, Y. F., Yang, M., Ye, J., Zhou, G., et al. (2019). Scaffold-based gene therapeutics for osteochondral tissue engineering. Front. Pharmacol. 10:1534.
Yang, Y., Wang, G., Liang, H., Gao, C., Peng, S., Shen, L., et al. (2019). Additive manufacturing of bone scaffolds. Int. J. Bioprint 5:148.
Yu, N., Nguyen, T., Cho, Y. D., Kavanagh, N. M., Ghassib, I., and Giannobile, W. V. (2019). Personalized scaffolding technologies for alveolar bone regenerative medicine. Orthod. Craniofac. Res. 22(Suppl. 1), 69–75. doi: 10.1111/ocr.12275
Zhai, D., Xu, M., Liu, L., Chang, J., and Wu, C. (2017). Silicate-based bioceramics regulating osteoblast differentiation through a BMP2 signalling pathway. J. Mater. Chem. B 5, 7297–7306. doi: 10.1039/c7tb01931a
Zhang, B., Zhang, W., Zhang, Z., Zhang, Y. F., Hingorani, H., Liu, Z., et al. (2019). Self-healing four-dimensional printing with an ultraviolet curable double-network shape memory polymer system. ACS Appl. Mater. Interfaces 11, 10328–10336. doi: 10.1021/acsami.9b00359
Zhang, D., Wu, X., Chen, J., and Lin, K. (2018). The development of collagen based composite scaffolds for bone regeneration. Bioact. Mater. 3, 129–138. doi: 10.1016/j.bioactmat.2017.08.004
Zhang, S., Cheng, X., Yao, Y., Wei, Y., Han, C., Shi, Y., et al. (2015). Porous niobium coatings fabricated with selective laser melting on titanium substrates: preparation, characterization, and cell behavior. Mater. Sci. Eng. C 53, 50–59. doi: 10.1016/j.msec.2015.04.005
Zhang, W., and Yelick, P. C. (2018). Craniofacial tissue engineering. Cold Spring Harb. Perspect. Med. 8:a025775.
Zhang, W., Zhu, Y., Li, J., Guo, Q., Peng, J., Liu, S., et al. (2016). Cell-Derived extracellular matrix: basic characteristics and current applications in orthopedic tissue engineering. Tissue Eng. Part B Rev. 22, 193–207. doi: 10.1089/ten.teb.2015.0290
Zhang, Y., Miron, R. J., Li, S., Shi, B., Sculean, A., and Cheng, X. (2015). Novel MesoPorous BioGlass/silk scaffold containing adPDGF-B and adBMP7 for the repair of periodontal defects in beagle dogs. J. Clin. Periodontol. 42, 262–271. doi: 10.1111/jcpe.12364
Zhang, Z., Cheng, X., Yao, Y., Luo, J., Tang, Q., Wu, H., et al. (2016). Electrophoretic deposition of chitosan/gelatin coatings with controlled porous surface topography to enhance initial osteoblast adhesive responses. J. Mater. Chem. B 4, 7584–7595. doi: 10.1039/c6tb02122k
Zhou, J., and Sheiko, S. S. (2016). Reversible shape-shifting in polymeric materials. J. Polym. Sci. Part B Polym. Phys. 54, 1365–1380. doi: 10.1002/polb.24014
Zhou, X., Jin, Y., and Du, J. (2020). Functionally graded scaffolds with programmable pore size distribution based on triply periodic minimal surface fabricated by selective laser melting. Materials 13:5046. doi: 10.3390/ma13215046
Zhu, L., Luo, D., and Liu, Y. (2020). Effect of the nano/microscale structure of biomaterial scaffolds on bone regeneration. Int. J. Oral Sci. 12:6.
Keywords: bone regeneration, 3D printing, biocompatibility, regenerative medicine, tissue engineering, periodontal diseases/therapy, bioresorbable scaffolds
Citation: Latimer JM, Maekawa S, Yao Y, Wu DT, Chen M and Giannobile WV (2021) Regenerative Medicine Technologies to Treat Dental, Oral, and Craniofacial Defects. Front. Bioeng. Biotechnol. 9:704048. doi: 10.3389/fbioe.2021.704048
Received: 01 May 2021; Accepted: 29 June 2021;
Published: 06 August 2021.
Edited by:
Barbara Zavan, University of Padua, ItalyReviewed by:
Vamsi Yadavalli, Virginia Commonwealth University, United StatesCopyright © 2021 Latimer, Maekawa, Yao, Wu, Chen and Giannobile. This is an open-access article distributed under the terms of the Creative Commons Attribution License (CC BY). The use, distribution or reproduction in other forums is permitted, provided the original author(s) and the copyright owner(s) are credited and that the original publication in this journal is cited, in accordance with accepted academic practice. No use, distribution or reproduction is permitted which does not comply with these terms.
*Correspondence: William V. Giannobile, V2lsbGlhbV9HaWFubm9iaWxlQGhzZG0uaGFydmFyZC5lZHU=
Disclaimer: All claims expressed in this article are solely those of the authors and do not necessarily represent those of their affiliated organizations, or those of the publisher, the editors and the reviewers. Any product that may be evaluated in this article or claim that may be made by its manufacturer is not guaranteed or endorsed by the publisher.
Research integrity at Frontiers
Learn more about the work of our research integrity team to safeguard the quality of each article we publish.