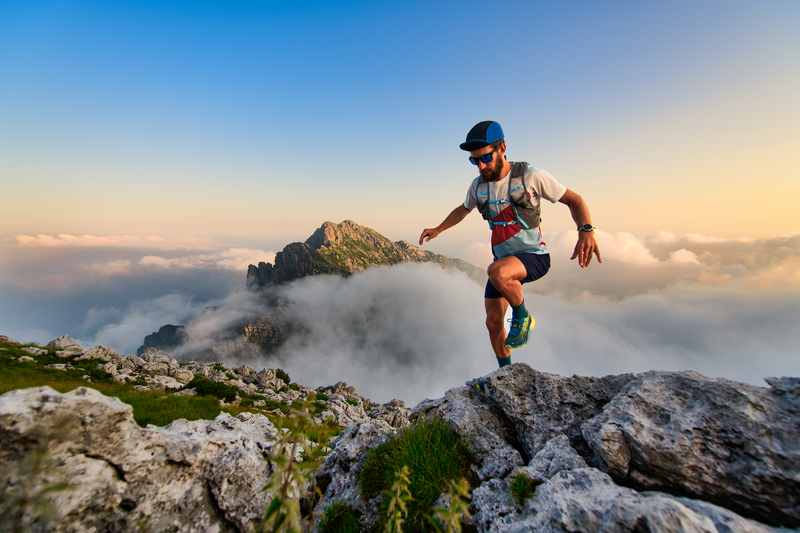
95% of researchers rate our articles as excellent or good
Learn more about the work of our research integrity team to safeguard the quality of each article we publish.
Find out more
ORIGINAL RESEARCH article
Front. Bioeng. Biotechnol. , 14 September 2021
Sec. Synthetic Biology
Volume 9 - 2021 | https://doi.org/10.3389/fbioe.2021.692901
This article is part of the Research Topic Mining, Deciphering and Engineering of Bacterial Natural Products View all 6 articles
Erythromycins produced by Saccharopolyspora erythraea have broad-spectrum antibacterial activities. Recently, several TetR-family transcriptional regulators (TFRs) were identified to control erythromycin production by multiplex control modes; however, their regulatory network remains poorly understood. In this study, we report a novel TFR, SACE_0303, positively correlated with erythromycin production in Sac. erythraea. It directly represses its adjacent gene SACE_0304 encoding a MarR-family regulator and indirectly stimulates the erythromycin biosynthetic gene eryAI and resistance gene ermE. SACE_0304 negatively regulates erythromycin biosynthesis by directly inhibiting SACE_0303 as well as eryAI and indirectly repressing ermE. Then, the SACE_0303 binding site within the SACE_0303-SACE_0304 intergenic region was defined. Through genome scanning combined with in vivo and in vitro experiments, three additional SACE_0303 target genes (SACE_2467 encoding cation-transporting ATPase, SACE_3156 encoding a large transcriptional regulator, SACE_5222 encoding α-ketoglutarate permease) were identified and proved to negatively affect erythromycin production. Finally, by coupling CRISPRi-based repression of those three targets with SACE_0304 deletion and SACE_0303 overexpression, we performed stepwise engineering of the SACE_0303-mediated mini-regulatory network in a high-yield strain, resulting in enhanced erythromycin production by 67%. In conclusion, the present study uncovered the regulatory network of a novel TFR for control of erythromycin production and provides a multiplex tactic to facilitate the engineering of industrial actinomycetes for yield improvement of antibiotics.
The high G+C Gram-positive bacterial actinomycetes are well known as one of the most abundant sources of bioactive secondary metabolites (Barka et al., 2016). The biosynthetic gene clusters of antibiotics in actinomycetes are transcriptionally controlled through subtle and delicate regulatory mechanisms, during which cluster-situated regulators (CSRs) or global regulators modulate the expression of their targets by sensing environmental or physiological signals (Niu et al., 2016; van der Heul et al., 2018). Thus far, at least 20 families of transcription factors (TFs) have been found in the antibiotic-producing actinomycetes (Romero-Rodriguez et al., 2015). Engineering of those regulators and their targets that control the biosynthesis of pharmaceutical antibiotics is an effective manner to boost the productivity of these fermentation products (Martín and Liras, 2010).
Saccharopolyspora erythraea, an important industrial actinomycete, is commonly used for the large-scale fermentation manufacturing of the valuable polyketide antibiotic erythromycin A (Er-A). Er-A and its derived macrolide drugs exhibit nice activities of many Gram-positive and some Gram-negative bacteria and have high annual sales in the billions of dollars (Robertsen and Musiol-Kroll, 2019). Hence, titer improvement of industrial erythromycin production is of vital significance. The erythromycin biosynthetic gene (ery) cluster consists of 20 genes arranged in four main polycistronic units in Sac. erythraea (Oliynyk et al., 2007). In general, there is lack of insight into the transcriptional regulation of the ery cluster owing to the absence of a CSR gene (Mironov et al., 2004). This not only implicates the unique mechanism for regulating erythromycin biosynthesis, but also increases the difficulty in regulatory engineering of Sac. erythraea for erythromycin biosynthetic titer improvement.
The genome of Sac. erythraea encodes 1,118 genes with latent regulatory functions, in which numerous types of TFs were discovered (Oliynyk et al., 2007). The developmental regulator BldD was first proved to directly control the synthesis of erythromycin (Chng et al., 2008). Next, several types of TFs were subsequently reported to be involved in erythromycin production in Sac. erythraea, mainly including the families of TetR and Lrp as well as nutrient-sensing regulators (Wu et al., 2014a,b, 2016, 2019; Liu et al., 2017, 2019; Xu et al., 2018, 2019b). This uncovered the complicated mechanism for controlling erythromycin biosynthesis with reciprocal (interactive regulation) or cascaded (hierarchical regulation) modes, which enable us to understand and manipulate the regulatory network governing erythromycin biosynthesis for titer improvement.
As a typical representative of TFs, TetR family transcriptional regulators (TFRs), consisting of an N-terminal DNA-binding domain and a C-terminal ligand-responsive domain, usually participate in the control of antibiotic production in actinomycetes (Cuthbertson and Nodwell, 2013). A total of 97 TFRs were encoded by the Sac. erythraea genome (Wu et al., 2016), and only five of them (SACE_3986, SACE_7301, SACE_3446, PccD, and SACE_5754) have been successively reported to control the biosynthesis of erythromycin so far (Wu et al., 2014a,b, 2016, 2019; Xu et al., 2018). These investigations show varied molecular mechanisms for regulating erythromycin production (negatively or positively) or the ery cluster (directly or indirectly). In particular, little is known about the TFR-mediated regulatory network concerning erythromycin biosynthesis.
Although manipulation of those TFRs and (or) their targets resulted in yield improvement of erythromycin, traditional genetic engineering in Sac. erythraea remained limited owing to much time and effort regarding multigene engineering. In particular, the low efficiency of the homologous recombination-based gene knockout in Sac. erythraea has always restricted genetic engineering of the industrial actinomycetes. In the past 3 years, clustered regularly interspaced short palindromic repeats interference (CRISPRi) mediated multiplex gene repression has been developed in the model actinomycetes Streptomyces coelicolor (Zhao et al., 2018) and was subsequently utilized in the industrial Streptomyces rapamycinicus and Streptomyces bingchenggensis to improve the titers of rapamycin and milbemycin, respectively (Tian et al., 2020; Liu et al., 2021b).
In this study, we report a novel TFR, SACE_0303, which indirectly triggered the erythromycin structure gene eryAI and resistance gene ermE, but directly suppressed its adjacent gene SACE_0304, encoding a MarR-family regulator (MFR). SACE_0304 was shown to directly repress SACE_0303 and eryAI but indirectly inhibit ermE. Three new SACE_0303’ target genes, SACE_2467, SACE_5222, and SACE_3156, were discovered and validated to negatively affect erythromycin production. Further, we performed stepwise engineering of the SACE_0303-mediated mini-regulatory network in a high-yield strain by coupling CRISPRi-based repression of those three targets with SACE_0304 deletion and SACE_0303 overexpression, resulting in obvious titer improvement of erythromycin.
All strains, plasmids, and primers used in this study are listed in Supplementary Tables 1–3. Escherichia coli were cultured in Luria-Bertani (LB) broth medium or on LB agar plate at 37°C. E. coli DH5α were used to construct plasmid. E. coli BL21 (DE3) was used for protein expression. Sac. erythraea A226, WB, and their derivative mutants were grown on the R3M agar plate medium for sporulation, protoplast regeneration, and phenotypic observation and in tryptone soya broth (TSB) medium for seed stock culture, genomic DNA extraction, and protoplast preparation at 30°C.
With the genome DNA of A226 as a template, two 1.5-kb DNA fragments flanking the SACE_0303 gene were amplified via PCR with the primer pairs SACE_0303-up-F/R and SACE_0303-down-F/R, respectively (Supplementary Table 3). The amplified fragments were successively treated with EcoRI/KpnI and XbaI/HindIII and ligated into the corresponding sites of pUCTSR (Han et al., 2011), generating pUCTSR-Δ0303 (Supplementary Table 2). Plasmid pUCTSR-Δ0303 was introduced into Sac. erythraea A226 by PEG-mediated protoplast transformation. A 424-bp DNA fragment of SACE_0303 was replaced by the thiostrepton resistance gene (tsr) by the method of chromosomic homologous recombination. The ΔSACE_0303 mutant with thiostrepton resistance was confirmed by PCR with the primers SACE_0303-C1/C2 (Supplementary Table 3). A full-length SACE_0303 gene of 564-bp were amplified by PCR using the primers SACE_0303-C1/C2 (Supplementary Table 3) from the genomic DNA of A226. The amplified fragment and pIB139 were cleaved with NdeI/Xbal and ligated to generate pIB139-0303 (Supplementary Table 2). Plasmid pIB139-0303 was introduced into ΔSACE_0303 and A226, respectively, by PEG-mediated protoplast transformation. The complemented strains ΔSACE_0303/pIB-0303 and overexpression strain A226/pIB-0303 were obtained by apramycin resistance screening and confirmed by PCR analysis with primers Apr-test-F/R (Supplementary Table 3). The same genetic manipulation methods were applied to construct SACE_0304 relevant mutants in A226 with corresponding primers (Supplementary Table 3). Likewise, three additional SACE_0303 target genes were individually overexpressed in A226 with their respective primers, and corresponding strains A226/pIB-2467, A226/pIB-3156, and A226/pIB-5222 were obtained (Supplementary Tables 1–3).
Moreover, SACE_0303 overexpression and SACE_0304 deletion in the high-yield Sac. erythraea WB were also manipulated, subsequently generating the mutants WB/pIB-0303 and WBΔ0304 (Supplementary Tables 1, 2).
With the plasmid DNA of pSET-dCas9-actII4-NT-S1 as a template, the ermE∗ promoter was obtained by PCR amplification with the primers P-PermE∗-F/R (Supplementary Table 3). With the genomic DNA of A226 as a template, the SACE_0303 gene was amplified with the primers P0303-F/R (Supplementary Table 3). Then, the two fragments were, respectively, digested with EcoRV/XbaI and XbaI/KpnI and ligated to the EcoRV/KpnI sites of pSET-dCas9-actII4-NT-S1 to generate pSETdCas9-0303 (Supplementary Table 2). The 20 nt sequences of the genes SACE_2467, SACE_3156, and SACE_5222 were obtained by the software sgRNA cas9 (v3.0) (Xie et al., 2014), which is suitable for gene editing with CRISPRi technology operation. A 699 bp sgRNA tandem sequence of the three SACE_0303 target genes synthesized from Sangon Biotech (Supplementary Figure 4) was digested with KpnI/EcoRI and ligated into the corresponding sites of pSETdCas9-0303, generating pSETdCas9-0303-sg2467-3156-5222 (Supplementary Table 2). Finally, pSETdCas9-0303 was successively introduced into the WB and WBΔ0304 to obtain WB/pSETdCas9-0303 and WBΔ0304/pSETdCas9-0303, respectively (Supplementary Table 1), and pSETdCas9-0303-sg2467-3156-5222 was transformed into WBΔ0304 to generate the strain WBΔ0304/p0303-sg2467-3156-5222 (Supplementary Table 1).
Flask fermentation of A226, WB, and their derived mutants were performed as previously described. Spores of A226 and its derivative strains were inoculated into TSB seed medium and grown for 2 days. Then, 5 mL seed cultures were inoculated into the R5 liquid medium to grow at 220 rpm, 30°C for 6 days. For WB and its derivatives, strains were cultivated in the industrial seed and fermentation media with the same culture conditions as A226 (Wu et al., 2014a). Er-A extracted from those fermentation broths was quantitatively measured by HPLC. An Agilent Extend-C18 column (5 μm; 250 × 4.6 mm) was equipped in the Shimadzu LC-2030 Plus HPLC system equilibrated with 60% solution A (5 mM ammonia acetate, pH 7.0) and 40% solution B (acetonitrile). An isocratic program was performed at a flow rate of 1.0 mL/min at 30°C using a ELSA-LT II ELSD detector (Wu et al., 2014a).
The SACE_0303 gene was amplified using the primers SACE_0303-C5/C6 (Supplementary Table 3) and was cloned into the NdeI/HindIII sites of pET28a to generate pET28a-0303. pET28a-0303 was transformed into E. coli BL21 (DE3), and SACE_0303 expression was induced by 0.5 mM IPTG at 30°C for 8–10 h. Purification of His6-tagged SACE_0303 protein was performed on a Ni2+-NTA spin column (BIO-RAD). BCA protein assay kit (Thermo Fisher Scientific) was used to analyze the concentration of purified protein, and its quality was estimated by SDS-PAGE.
EMSAs were performed as previously published report (Hellman and Fried, 2007). DNA probes were amplified by PCR with their respective primers listed in Supplementary Table 3. The DNA probe was incubated with various concentrations of His6-tagged SACE_0303. The binding reaction system contained 60 mM KCl, 50 mM EDTA, 10 mM Tris-HCl (pH 7.5), 10 mM DTT, 5 mM MgCl2, 10% glycerol, 150 ng DNA probe labeled by 5′-FAM/3′-HEX and purified His6-SACE_0303 protein. Unlabeled DNA fragments or poly-dIdC were used for competitive assays. After incubation at 30°C for 20 min in 20 μL reaction mixtures, the reactants were fractionated on 6% native PAGE gels in 1 × TAE buffer at 40 mA for 35–45 min.
Using the TransZol up plus RNA kit (Transgen), total RNA was isolated from A226 and its derivatives after 24 h fermentation in R5 liquid medium or WB derivatives after 12 h culture in industrial fermentation medium. The RNA concentration was measured with the microplate reader (BioTek). RNA was treated with DNase I (MBI Fermentas), and reverse transcription was achieved using a cDNA synthesis kit (MBI Fermentas). The relative transcriptional levels of genes were examined with QuantStudioTM 6 Flex (Thermo Fisher Scientific) using the primers listed in Supplementary Table 3. The hrdB (SACE_1801) gene in Sac. erythraea was served as an internal control to normalize samples.
DNA fragments of four promoters containing P0303, P0304, PeryAI, and PermE regions were successively amplified using the primer pairs in Supplementary Table 3 with A226 as the template and digested with HindIII/XbaI. The enhanced green fluorescent protein gene (egfp) fragment obtained by XbaI/BamHI digestion of pKC-DE (Liu et al., 2017) and the above PCR products were ligated into HindIII/BamHI sites of pKC1139 (Wilkinson et al., 2002) to obtain the control plasmids pKC-ME, pKC-TE, pKC-AE, and pKC-EE (Supplementary Table 2). Next, the Papr with EcoRV/NdeI was obtained from pIB139 using the primers P-Papr-F/R (Supplementary Table 3), and the SACE_0304 gene with NdeI/EcoRI was amplified using the primers SACE_0304-F-F/R (Supplementary Table 3) with A226 genomic DNA as the template. Then, the two fragments were jointly ligated into EcoRV/EcoRI sites of the above four control plasmids, generating the reporter plasmids pKC-MR-ME, pKC-MR-TE, pKC-MR-AE, and pKC-MR-EE, respectively (Supplementary Table 2).
The above plasmids were separately transformed into DH5α for detection of green fluorescence (excitation at 485 nm; emission at 510 nm, Molecular Devices). All fluorescence values were normalized to growth rates (OD600).
The DNase I footprinting assay was performed as previously described (Xu et al., 2019a). To precisely determine the DNA binding site of SACE_0303, 100 ng FAM/HEX-labeled P0303–0304 was successively incubated with 0, 70, and 490 nM His6-SACE_0303 in a total 50 μL of binding buffer at 20°C for 20 min, and then 2 μL DNase I (1 U/μg; Promega) was performed at 20°C for 30 s, 10 μL DNase I stop solution was added to the mixture and reacted at 65°C for 10 min. The ethanol precipitation method was used to purify and recover DNA samples. Purified DNA was sequenced with a 3730XL DNA genetic analyzer (Applied Biosystems), and GeneMarker software program v2.2 for data analysis.
All data in this study were stated as means ± standard error of the mean (SD), and analyzed by Student’s t-test, with ∗p <0.05, ∗∗ p < 0.01, and ∗∗∗p < 0.001, ns, not significant.
The information on SACE_0303 and its adjacent genes of the Sac. erythraea chromosome is shown in Figure 1A. SACE_0304, the neighboring gene of SACE_0303, encodes an MFR. To clarify the function of SACE_0303, the fragment homologous recombination method was performed in Sac. erythraea A226 to obtain the SACE_0303-deleted mutant strain ΔSACE_0303 (Figure 1B), which was confirmed by PCR analyses (Figure 1C). The Er-A production of ΔSACE_0303 was significantly reduced by 33% compared with A226 fermented for 6 days in the R5 liquid medium. No obvious change in cellular growth and morphological differentiation was observed between the two strains (Supplementary Figure 1). To determine that the yield decrease in ΔSACE_0303 was only caused by inactivation of SACE_0303, we constructed the complement strains ΔSACE_0303/pIB-0303 and overexpressing strains A226/pIB-0303 based on the pIB139 vector. Results showed that pIB139 had no effect on the yield of erythromycin by testing the fermentation extracts of A226/pIB139 and ΔSACE_0303/pIB139 (Figure 1D) although Er-A yield of ΔSACE_0303/pIB-0303 was nearly recovered to the parental level, and overexpression of SACE_0303 in A226 increased the Er-A yield by ∼31% (Figure 1D). Furthermore, we overexpressed SACE_0303 in the high-yield Sac. erythraea WB and confirmed that the Er-A yield of WB/pIB-0303 was ∼25% higher than that in WB (Figure 1E). These findings indicate that SACE_0303 has a positive effect on the biosynthesis of erythromycin.
Figure 1. Inactivation of SACE_0303 in Sac. erythraea. (A) SACE_0303 genome location information. (B) Schematic deletion of SACE_0303 by linearized fragment homologous recombination in A226. (C) PCR confirmation of the SACE_0303 deletion mutant by the primers SACE_0303-C1 and SACE_0303-C2. The size of gene 564 bp amplified by PCR bands observed in A226, 1,540 bp was detected from pUCTSR-Δ0303 and ΔSACE_0303. (D) Er- A yields in A226, ΔSACE_0303, ΔSACE_0303/pIB139, ΔSACE_0303/pIB-0303, A226/pIB139, and A226/pIB-0303. (E) Er- A yields in WB and WB/pIB-0303. Error bars (D): SD from triplicate experiments. Statistical notations (D): ns, not significant; ∗p < 0.05.
It has been documented that actinomycete TFRs affect the biosynthesis of antibiotics by directly regulating the expression of adjacent genes (Cuthbertson and Nodwell, 2013). Thereby, EMSA was herein utilized to examine if SACE_0303 also exhibits a similar regulatory mode. The FAM-labeled probe P0303–0304 covering the entire promoter regions of SACE_0303 and SACE_0304 was mixed with purified His6-SACE_0303. After the addition of purified SACE_0303, a mobility shift was obviously observed (Figure 2A). External addition of a 50-fold excess unlabeled probe can notably compete with the labeled probe to bind to SACE_0303, while an excess 50-fold nonspecific probe, poly-dIdC, cannot abolish the shift band (Figure 2A), together providing the solid evidence that SACE_0303 bound specifically to P0303–0304. RT-qPCR analyses showed that SACE_0303 was transcriptionally decreased by 93% upon its activation, but transcript of SACE_0304 in ΔSACE_0303 was increased by 120% (Figure 2B). These results indicate that SACE_0303 transcriptionally activates its own gene and represses SACE_0304 via direct regulatory pattern.
Figure 2. Regulatory mode of SACE_0303 in SACE_0303 and SACE_0304. (A) EMSAs of the interaction of probe P0303–0304 with purified His6-SACE_0303 protein. (B) Effects of SACE_0303 disruption on transcripts of SACE_0303, SACE_0304, eryAI, and ermE. RT-qPCR was used to quantify the amounts of transcripts in A226 and ΔSACE_0303 cultured for 24 h in the liquid R5 medium. Error bars (B): SD from triplicate experiments. Statistical notations (B): ∗p < 0.05, ∗∗p < 0.01, ∗∗∗p < 0.001.
Furthermore, we chose the promoter regions of erythromycin biosynthetic gene eryAI encoding polyketide synthase I (PeryAI) and the resistance gene ermE encoding rRNA methyltransferase (PermE) for binding to His6-SACE_0303 to uncover its potential action mode in the ery cluster. Results found from the gel-shift assays that SACE_0303 could not bind to PeryAI and PermE (Supplementary Figure 2). The transcripts of eryAI and ermE in ΔSACE_0303 were successively reduced by 68% and 69% over those in A226 (Figure 2B). Seemingly, these data suggest that SACE_0303 exerts an indirect mode in transcriptional control of ery cluster.
Because SACE_0304 was a target gene of SACE_0303, we next examined whether it also affects erythromycin production. The ΔSACE_0304 mutant was constructed by disrupting the SACE_0304 gene in A226 and confirmed by PCR analyses (Figures 3A,B). Further, we performed the overexpression of SACE_0304 under PermE∗ in A226 to obtain the desired strain A226/pIB-0304. By HPLC analyses of cultures from those strain, we found that, compared with A226, ΔSACE_0304 had a ∼22% production increase in Er-A, and A226/pIB-0304 exhibited a decreased yield by about 18% (Figures 3B,C). Deletion of SACE_0304 did not affect the cell growth and morphological differentiation (Supplementary Figure 3). When SACE_0304 was also deleted in the industrial strain WB, Er-A yield was ∼25% higher in WBΔ0304 than of WB (Figure 3D). Thus, these results indicate that SACE_0304 has a negative effect on erythromycin production.
Figure 3. Inactivation of SACE_0304 in Sac. erythraea. (A) Schematic deletion of SACE_0304 by linearized fragment homologous recombination in A226. (B) PCR confirmation of the SACE_0304 deletion mutant by the primers SACE_0304-C1 and SACE_0304-C2. The size of gene 504 bp amplified by PCR bands observed in A226, 1,519 bp was detected from pUCTSR-Δ0304 and ΔSACE_0304. (C) Er-A yields in A226, ΔSACE_0304 and A226/pIB-0304. (D) Er-A yields in WB and WBΔ0304. Error bars (C,D): SD from triplicate experiments. Statistical notations (C,D): ∗p < 0.05.
As MFRs have a similar regulatory mode as TFRs in control of their adjacent genes (Romero-Rodriguez et al., 2015), we first performed EMSA with purified SACE_0304 protein for binding to the probe P0303–0304, but this protein seemed inactive with considerable repeats. Then, we designed the eGFP reporter system in E. coli to verify the interaction of SACE_0304 with the promoter regions of SACE_0303 (P0303) and SACE_0304 (P0304) (Figure 4A). Plasmids pKC-TE and pKC-ME containing egfp under control of P0303 and P0304 were successively constructed and transformed into E. coli DH5α, and obtained strains exhibited obvious fluorescent signals. When SACE_0304 under the promoter of apramycin resistance gene (Papr) was individually ligated into pKC-TE and pKC-ME and transformed into DH5α, the green fluorescence of pKC-MR-TE and pKC-MR-ME was, respectively, decreased by 81% and increased twofold compared with the absence of SACE_0304 (Figure 4B), indicating that SACE_0304 directly repressed P0304 and activated P0303 in the heterologous E. coli host. Further, we used RT-qPCR to compare the transcriptional levels of SACE_0303 and SACE_0304 between A226 and ΔSACE_0304. Compared with A226, ΔSACE_0304 exhibited upregulated transcription of SACE_0303 by 2.5-fold but downregulated transcription of SACE_0304 by 25% (Figure 4C). Therefore, these in vivo and in vitro results demonstrate that SACE_0304 is directly self-activated and represses the expression of SACE_0303.
Figure 4. Regulatory mode of SACE_0304. (A) An illustration of the reporter plasmids. (B) Detection of the relative fluorescence units (RFUs) after different promoters in E. coli DH5α/pKC-EGFP and DH5α/pKC-MR-EGFP. (C) Effects of SACE_0304 disruption on transcripts of SACE_0303, SACE_0304, eryAI, and ermE. Error bars (B,C): SD from triplicate experiments. Statistical notations (B,C): ns, not significant. ∗p < 0.05, ∗∗p < 0.01, ∗∗∗p < 0.001.
To further investigate the regulatory mode of SACE_0304 in the ery cluster, we likewise constructed eGFP reporter plasmids (Figure 4A), which were introduced to DH5α. As shown in Figure 4B, the fluorescence of egfp initiated by PeryAI was reduced by 50% after the addition of SACE_0304. When SACE_0304 under Papr was inserted into the pKC-EE, the fluorescence almost had no statistical difference compared with that without SACE_0304 (Figure 4B). RT-qPCR analyses showed that the expressional levels of eryAI and ermE in ΔSACE_0304 was onefold higher than those in A226 (Figure 4C). These findings indicate that SACE_0304 directly inhibits eryAI but indirectly represses ermE.
To determine the DNA binding site of SACE_0303, a DNase I footprinting assay was manipulated using purified His6-SACE_0303 and the 100 bp probe P0303–0304 labeled via FAM/HEX, and results showed that a 23-nt sequence (GTACTGAAACGACTGTTTCAGGA) was protected by SACE_0303, in which an 18 bp palindrome sequence (CTGAAACGACTGTTTCAG, underlined) was obviously detected (Figures 5A,B). To verify the indispensable palindrome sequence for binding SACE_0303, we introduced mutations to repeat motifs in P0303–0304-56 bp to obtain the probe P0303–0304-56 bpM for EMSAs (Figure 5B). Results found that SACE_0303 only bound to P0303–0304-56 bp (Figure 5C). With individual prediction of the -10/-35 regions and transcriptional start sites (TSSs) of SACE_0303 and SACE_0304 by online software phiSITE1 and BDGB2, the repeat motifs were found to both overlap the putative −10 regions and TSSs of SACE_0303 and SACE_0304 (Figure 5D). Possibly, SACE_0303 represses SACE_0304 by impeding the recruitment of RNA polymerase. The mechanism concerning self-activation of SACE_0303 needs to be further explored due to the unusualness of binding of transcriptional activator to −10 region.
Figure 5. Determination of the SACE_0303-binding sites. (A) DNase I footprinting assay of SACE_0303-binding site within P0303–0304. Upper fluorogram: control reaction without protein. Protection regions were acquired with increasing concentrations (70 and 490 nM) of His6- SACE_0303. (B) Different probes designed for determining DNA binding site of SACE_0303. (C) Binding assays of SACE_0303 to P0303–0304-56 bp and P0303–0304-56 bpM. (D) Nucleotide sequences of promoter regions of SACE_0303 and SACE_0304. Transcriptional start site, TSS.
Uncovering the regulatory network of SACE_0303 requires the identification of additional SACE_0303 target genes. To this end, we used the above 18 bp SACE_0303-binding sequences to scan the whole genome sequence of Sac. erythraea by an online virtual footprint software suite3. Totally, the upstream regions of 83 genes containing P0303–0304 were identified (cutoff score ≥20) (data not shown), during which 10 predicted high-score sites flanked by well-annotated genes were chosen for EMSAs (Supplementary Table 4). Results confirm that SACE_0303 bound specifically to the promoter regions of SACE_2467 encoding cation-transporting ATPase (P2467), SACE_3156 encoding a large transcriptional regulator (P3156), and SACE_5222 encoding alpha-ketoglutarate permease (P5222) (Figure 6A). Further RT-qPCR analyses showed that the transcripts of these three genes were increased in ΔSACE_0303 to varying degrees compared with those in A226 (Figure 6B). These results demonstrate that SACE_0303 directly controls the transcription of SACE_2467, SACE_3156, and SACE_5222.
Figure 6. Effects of SACE_0303 on its three targets SACE_2467, SACE_3156, and SACE_5222. (A) Binding assays of SACE_0303 to P2467, P3156, and P5222. (B) Transcriptional analyses of SACE_2467, SACE_3156, and SACE_5222 in A226 and ΔSACE_0303. (C) Er-A yields in A226, A226/pIB-2467, A226/pIB-3156, and A226/pIB-5222. Error bars (B,C): SD from triplicate experiments. Statistical notations (B,C): *p < 0.05, **p < 0.01, ***p < 0.001.
To uncover the effects of the three genes on the erythromycin production, SACE_2467, SACE_3156, and SACE_5222 were individually overexpressed in A226. By fermentation and HPLC analyses, Er-A yields in A226/pIB-2467, A226/pIB-3156, and A226/pIB-5222 were, respectively, reduced by 26.6, 37.6, and 26.1% relative to those in A226 (Figure 6C), indicating that these three target genes of SACE_0303 were negatively correlated with erythromycin production.
Given that SACE_0303 overexpression or SACE_0304 deletion in WB both enhanced the Er-A yield (Figures 1E, 3D), we further performed multiplex engineering of the two TFs as well as three new SACE_0303 target genes to estimate their practical potential in the high-yield strain of Sac. erythraea.
To use the CRISPRi system for multigene repression, pSET-dCas9 (Figure 7A), a pSET152-derived integrative plasmid (Zhao et al., 2018) was transformed into WB to evaluate its effect on erythromycin production. Results showed that the Er-A yield of WB/pSETdCas9 was not significantly different from that of WB (Figure 7C). In pSET-dCas9-actII4-NT-S1 with available digestion sites to ligate sgRNA-containing cassette, SACE_0303 under PermE∗ was ligated into the CRISPRi vector to generate recombinant plasmid pSETdCas9-0303 (Figure 7A), which was then introduced into WB and WBΔ0304, respectively. These resulting strains WB/pSETdCas9-0303 (884 mg/L) and WBΔ0304/pSETdCas9-0303 (928 mg/L) exhibited a stepwise increase in Er-A yield over WB (685 mg/L) (Figure 7C). Furthermore, a synthetic cassette containing the sgRNAs of SACE_2467, SACE_3156, and SACE_5222 was ligated into pSETdCas9-0303 (Figure 7A and Supplementary Figure 4), and the desired plasmid pSET-dCas9-0303/sg2467-3156-5222 was transferred into WBΔ0304 to generate the strain WBΔ0304/p0303-sg2467-3156-5222. As expected, the transcriptional levels of SACE_2467, SACE_3156, and SACE_5222 in WBΔ0304/p0303-sg2467-3156-5222 were inhibited to different degrees via RT-qPCR analyses (Figure 7B). Correspondingly, WBΔ0304/p0303-sg2467-3156-5222 (1,142 mg/L), respectively, exhibited ∼23% and ∼67% increase in Er-A production compared with WBΔ0304/pSETdCas9-0303 and WB (Figure 7C).
Figure 7. Multiplex engineering of SACE_0303-mediated regulatory system in WB. (A) Schematic construction of pSET-dCas9-0303/sg2467-3156-5222. (B) Transcriptional assays of SACE_2467, SACE_3156, and SACE_5222 in WBΔ0304/pSETdCas9-0303 and WBΔ0304/p0303-sg2467-3156-5222. (C) Er-A yield in WB and its derived mutants. Error bars (B,C): SD from triplicate experiments. Statistical notations (B,C): ns, not significant; ∗p < 0.05, ∗∗p < 0.01, ∗∗∗p < 0.001.
Up to now, five TFRs from Sac. erythraea were successively shown to be involved in the repression or activation of erythromycin biosynthesis, in which SACE_7301 and SACE_3446 exerted a direct interaction to the promoters of the ery cluster (Wu et al., 2014a, 2016), and SACE_3986 and SACE_5754 indirectly controlled the transcription of the ery cluster (Wu et al., 2014b, 2019). However, the regulatory network regarding erythromycin biosynthesis has been seldom reported; in particular, hierarchical regulations between TFRs and other TFs have not been investigated yet. In this study, we report a novel TFR, SACE_0303, and reveal reciprocal regulation between this TFR and its adjoining MFR SACE_0304. With the DNA-binding site of SACE_0303 defined, three new SACE_0303 target genes were identified and confirmed to repress the erythromycin production. By integrating CRISPRi-mediated repression of the three targets with SACE_0304 deletion and SACE_0303 overexpression, combinatorial engineering of this mini-regulatory network was performed in a high-yield strain, resulting in dramatic titer improvement of erythromycin.
TFRs generally serve as repressors to transcriptionally regulate their upstream targets for the control of antibiotic production (Deng et al., 2013; Xu et al., 2019a). A similar phenomenon was also found in Sac. erythraea, in which four of the hitherto reported TFRs played the negative role in regulation of erythromycin biosynthesis (Wu et al., 2014b, 2016, 2019; Xu et al., 2018). Only the TFR, SACE_7301, was shown to trigger the transcription of eryAI and ermE by interacting with their promoter regions for positively regulating the erythromycin production (Wu et al., 2014a). In this study, SACE_0303 was likewise affirmed to positively correlate with erythromycin production (Figure 1); however, it indirectly stimulated the transcription of eryAI and ermE (Figure 2), implicating a SACE_0303-mediated regulation for the erythromycin biosynthesis via hierarchical control pattern. Then, SACE_0303 was demonstrated to directly inhibit SACE_0304 (Figure 2). SACE_0304, negatively affecting erythromycin production (Figure 3), was proved, in turn, to directly repress SACE_0303 as well as eryAI but indirectly inhibit ermE (Figure 4). Accordingly, our findings exhibit a very complicated mechanism for cascaded control of erythromycin production by the two types of TFs.
Members of the multiple antibiotic resistance regulators (MarR) family of TFs, widely distributing among prokaryotes, could modulate diverse physiological processes, including stress response, antibiotic resistance, and export, etc. (Grove, 2013). In spite of abundant distribution of MFRs in the antibiotic-producing actinomycetes, just a few members have been characterized in Streptomyces (Yang et al., 2010; Davis et al., 2013; Huang and Grove, 2013; Zhang et al., 2015; Guo et al., 2018; Kong et al., 2019; Nag and Mehra, 2021), and the functional probe into MFRs in Sac. erythraea has not been reported. Until lately, we identified an MFR (SACE_6745) to directly inhibit the genes for erythromycin biosynthesis, export, and resistance (Liu et al., 2021a). This study identified an additional MFR (SACE_0304) in Sac. erythraea and proved its direct transcriptional control of erythromycin production. It is believed that, together with SACE_6745, the identification of SACE_0304 provides the starting point to deepen the understanding of MFR-mediated regulation for erythromycin biosynthesis.
TFRs and MFRs, both serving as one-component regulators, could control the expression of upstream targets by responding to specific ligands (Cuthbertson and Nodwell, 2013; Grove, 2013). Typically, they could bind to intergenic regions to autoregulate their own gene and divergently transcribed gene (Romero-Rodriguez et al., 2015). We herein found that SACE_0303 and SACE_0304 indeed abided by the paradigm that they are both self-activated directly and can repress the expression of each other (Figures 2, 4). Whether certain or common ligands exist to affect reciprocal regulation of the two TFs needs to be further explored.
Based on defined DNA binding site of SACE_0303 within P0303–0304 (Figure 5), we utilized genome scanning, EMSAs, and transcriptional assays to identify three new SACE_0303 target genes, SACE_2467, SACE_5222, and SACE_3156, which were next proved to negatively affect erythromycin production (Figure 6). SACE_2467 encodes a P-type ATPase, which could generally utilize energy released by ATP hydrolysis to transport cations across the cell membrane (Sitsel et al., 2015). A previous report shows that deletion of SCO2731 (a P-type ATPase) and its adjacent SCO2730 (a copper chaperon) activated secondary metabolic pathways in S. coelicolor by enabling cytosolic copper to optimal homeostasis (Gonzalez-Quinonez et al., 2019). As the erythromycin biosynthesis was recently shown to positively correlate with the ATP/ADP ratio in Sac. erythraea (Li et al., 2020), we inferred that SACE_2467 overexpression might consume more ATP to transport cations, subsequently decreasing erythromycin production. α-ketoglutarate (α-KG), an intermediate of the tricarboxylic acid (TCA) cycle, intersects between carbon and nitrogen metabolic pathways (Commichau et al., 2006). In S. coelicolor, increased α-KG could promote the TCA cycle to form more NADH for maintaining intracellular redox homeostasis (Xu et al., 2017). We speculate that overexpression of SACE_5222 encoding α-ketoglutarate permease might unbalance intracellular redox status, exhibiting an adverse effect on erythromycin biosynthesis. SACE_3156 encodes a large transcriptional regulator belonging to a LuxR family, and its overexpression likewise decreased the erythromycin yield (Figure 6). However, the regulatory mechanism of SACE_3156 requires further investigation. Building on current results, the SACE_0303-mediated mini-regulatory network was proposed (Supplementary Figure 5), which not only controls different types of TFs to exert the regulatory cascade, but also might affect cofactors hemostasis and metabolism for control of erythromycin production.
Rewiring the regulatory network with engineering of TFs and their targets is an effective approach to boost the productivity of antibiotics in actinomycetes (Martín and Liras, 2010; Xia et al., 2020). For example, certain TFs and (or) their targets have been manipulated in Sac. erythraea WB for enhanced erythromycin production (Wu et al., 2014a,b, 2016, 2019; Liu et al., 2017, 2019). Nevertheless, few genes were jointly manipulated, and two genes at most were hitherto knocked out simultaneously in the high-yield strain (Wu et al., 2016; Liu et al., 2021a). Herein, we manage to exert stepwise engineering of three types of TFs and two metabolic genes in WB. Specifically, SACE_0303 under PermE∗ was ligated into the CRISPRi system and the obtained plasmid was introduced into an existing WB mutant with SACE_0304 deletion for concurrent transcriptional downregulation of three new SACE_0303 targets as well as SACE_0303 overexpression (Figure 7). Expectedly, corresponding mutants displayed stepwise titer improvement, in which a final strain with joint engineering of five genes had ∼67% increase in Er-A yield over WB (Figure 7). The present study provides a new tactic for antibiotic yield improvement by TF-based combinatorial engineering of industrial actinomycetes.
The raw data supporting the conclusions of this article will be made available by the authors, without undue reservation.
HW and BZ conceived and designed the study. YL, SK, PW, BL, LL, JN, HZ, and KC performed the experiments. YL and HW analyzed the data. HW wrote the manuscript. BZ modified the manuscript. All authors have read and approved the manuscript.
This work was supported by the National Natural Science Foundation of China (Grant Nos. 31972930 and 32170073), the University Synergy Innovation Program of Anhui Province (Grant No. GXXT-2019-035) and the Anhui Provincial Program on Key Research and Development Project (Grant No. 201904a07020080).
The authors declare that the research was conducted in the absence of any commercial or financial relationships that could be construed as a potential conflict of interest.
All claims expressed in this article are solely those of the authors and do not necessarily represent those of their affiliated organizations, or those of the publisher, the editors and the reviewers. Any product that may be evaluated in this article, or claim that may be made by its manufacturer, is not guaranteed or endorsed by the publisher.
We thank Prof. Yinhua Lu from Shanghai Normal University for providing these plasmids pSET-dCas9 and pSET-dCas9-actII4-NT-S1.
The Supplementary Material for this article can be found online at: https://www.frontiersin.org/articles/10.3389/fbioe.2021.692901/full#supplementary-material
Supplementary Figure 1 | Morphological differentiation and growth rates of A226 and ΔSACE_0303.
Supplementary Figure 2 | EMSAs of DNA-binding of SACE_0303 to PeryAI and PermE.
Supplementary Figure 3 | Morphological differentiation and growth rates of A226 and ΔSACE_0304.
Supplementary Figure 4 | The synthetic cassette of sg2467-3156-5222.
Supplementary Figure 5 | Possible regulatory pathway of SACE_0303 for control of erythromycin biosynthesis in Sac. erythraea.
Supplementary Table 1 | Strains used in this study.
Supplementary Table 2 | Plasmids used in this study.
Supplementary Table 3 | Primers used in this study.
Supplementary Table 4 | EMSAs for upstream regions of putative SACE_0303 target genes.
Barka, E. A., Vatsa, P., Sanchez, L., Gaveau-Vaillant, N., Jacquard, C., Meier-Kolthoff, J. P., et al. (2016). Taxonomy, physiology, and natural products of actinobacteria. Microbiol. Mol. Biol. Rev. 80, 1–43. doi: 10.1128/MMBR.00019-15
Chng, C., Lum, A. M., Vroom, J. A., and Kao, C. M. (2008). A key developmental regulator controls the synthesis of the antibiotic erythromycin in Saccharopolyspora erythraea. Proc. Natl. Acad. Sci. U.S.A. 105, 11346–11351. doi: 10.1073/pnas.0803622105
Commichau, F. M., Forchhammer, K., and Stulke, J. (2006). Regulatory links between carbon and nitrogen metabolism. Curr. Opin. Microbiol. 9, 167–172. doi: 10.1016/j.mib.2006.01.001
Cuthbertson, L., and Nodwell, J. R. (2013). The TetR family of regulators. Microbiol. Mol. Biol. Rev. 77, 440–475. doi: 10.1128/MMBR.00018-13
Davis, J. R., Brown, B. L., Page, R., and Sello, J. K. (2013). Study of PcaV from Streptomyces coelicolor yields new insights into ligand-responsive MarR family transcription factors. Nucleic Acids Res. 41, 3888–3900. doi: 10.1093/nar/gkt009
Deng, W., Li, C., and Xie, J. (2013). The underling mechanism of bacterial TetR/AcrR family transcriptional repressors. Cell Signal 25, 1608–1613. doi: 10.1016/j.cellsig.2013.04.003
Gonzalez-Quinonez, N., Corte-Rodriguez, M., Alvarez-Fernandez-Garcia, R., Rioseras, B., Lopez-Garcia, M. T., Fernandez-Garcia, G., et al. (2019). Cytosolic copper is a major modulator of germination, development and secondary metabolism in Streptomyces coelicolor. Sci. Rep. 9:4214. doi: 10.1038/s41598-019-40876-0
Grove, A. (2013). MarR family transcription factors. Curr. Biol. 23, R142–R143. doi: 10.1016/j.cub.2013.01.013
Guo, J., Zhang, X., Lu, X., Liu, W., Chen, Z., Li, J., et al. (2018). SAV4189, a MarR-family regulator in Streptomyces avermitilis, activates avermectin biosynthesis. Front. Microbiol. 9:1358. doi: 10.3389/fmicb.2018.01358
Han, S., Song, P., Ren, T., Huang, X., Cao, C., and Zhang, B. (2011). Identification of SACE_7040, a member of TetR family related to the morphological differentiation of Saccharopolyspora erythraea. Curr. Microbiol. 63, 121–125. doi: 10.1007/s00284-011-9943-z
Hellman, L. M., and Fried, M. G. (2007). Electrophoretic mobility shift assay (EMSA) for detecting protein–nucleic acid interactions. Nature Protocols 2, 1849–1861. doi: 10.1038/nprot.2007.249
Huang, H., and Grove, A. (2013). The transcriptional regulator TamR from Streptomyces coelicolor controls a key step in central metabolism during oxidative stress. Mol. Microbiol. 87, 1151–1166. doi: 10.1111/mmi.12156
Kong, L., Liu, J., Zheng, X., Deng, Z., and You, D. (2019). CtcS, a MarR family regulator, regulates chlortetracycline biosynthesis. BMC Microbiol. 19:279. doi: 10.1186/s12866-019-1670-9
Li, X., Chen, J., Andersen, J. M., Chu, J., and Jensen, P. R. (2020). Cofactor engineering redirects secondary metabolism and enhances erythromycin production in Saccharopolyspora erythraea. ACS Synth. Biol. 9, 655–670. doi: 10.1021/acssynbio.9b00528
Liu, J., Chen, Y., Li, L., Yang, E., Wang, Y., Wu, H., et al. (2019). Characterization and engineering of the Lrp/AsnC family regulator SACE_5717 for erythromycin overproduction in Saccharopolyspora erythraea. J. Ind. Microbiol. Biotechnol. 46, 1013–1024. doi: 10.1007/s10295-019-02178-2
Liu, J., Chen, Y., Wang, W., Ren, M., Wu, P., Wang, Y., et al. (2017). Engineering of an Lrp family regulator SACE_Lrp improves erythromycin production in Saccharopolyspora erythraea. Metab. Eng. 39, 29–37. doi: 10.1016/j.ymben.2016.10.012
Liu, J., Li, L., Wang, Y., Li, B., Cai, X., Tang, L., et al. (2021a). Joint engineering of SACE_Lrp and its target MarR enhances the biosynthesis and export of erythromycin in Saccharopolyspora erythraea. Appl. Microbiol. Biotechnol. 105, 2911–2924. doi: 10.1007/s00253-021-11228-8
Liu, Y., Wang, H., Li, S., Zhang, Y., Cheng, X., Xiang, W., et al. (2021b). Engineering of primary metabolic pathways for titer improvement of milbemycins in Streptomyces bingchenggensis. Appl. Microbiol. Biotechnol. 105, 1875–1887. doi: 10.1007/s00253-021-11164-7
Martín, J. F., and Liras, P. (2010). Engineering of regulatory cascades and networks controlling antibiotic biosynthesis in Streptomyces. Curr. Opin. Microbiol. 13, 263–273. doi: 10.1016/j.mib.2010.02.008
Mironov, V. A., Sergienko, O. V., Nastasiak, I. N., and Danilenko, V. N. (2004). Biogenesis and regulation of biosynthesis of erythromycins in Saccharopolyspora erythraea: a review. Prikl. Biokhim. Mikrobiol. 40, 613–624.
Nag, A., and Mehra, S. (2021). A major facilitator superfamily (MFS) efflux pump, SCO4121, from Streptomyces coelicolor with roles in multidrug resistance and oxidative stress tolerance and its regulation by a MarR regulator. Appl. Environ. Microbiol. 87:e02238-20. doi: 10.1128/AEM.02238-20
Niu, G., Chater, K. F., Tian, Y., Zhang, J., and Tan, H. (2016). Specialised metabolites regulating antibiotic biosynthesis in Streptomyces spp. FEMS Microbiol. Rev. 40, 554–573. doi: 10.1093/femsre/fuw012
Oliynyk, M., Samborskyy, M., Lester, J. B., Mironenko, T., Scott, N., Dickens, S., et al. (2007). Complete genome sequence of the erythromycin-producing bacterium Saccharopolyspora erythraea NRRL23338. Nat. Biotechnol. 25:447. doi: 10.1038/nbt1297
Robertsen, H. L., and Musiol-Kroll, E. M. (2019). Actinomycete-derived polyketides as a source of antibiotics and lead structures for the development of new antimicrobial drugs. Antibiotics (Basel) 8:157. doi: 10.3390/antibiotics8040157
Romero-Rodriguez, A., Robledo-Casados, I., and Sanchez, S. (2015). An overview on transcriptional regulators in Streptomyces. Biochim. Biophys. Acta 1849, 1017–1039. doi: 10.1016/j.bbagrm.2015.06.007
Sitsel, O., Grønberg, C., Autzen, H. E., Wang, K., Meloni, G., Nissen, P., et al. (2015). Structure and function of Cu(I)- and Zn(II)-AT pases. Biochemistry 54, 5673–5683. doi: 10.1021/acs.biochem.5b00512
Tian, J., Yang, G., Gu, Y., Sun, X., Lu, Y., and Jiang, W. (2020). Developing an endogenous quorum-sensing based CRISPRi circuit for autonomous and tunable dynamic regulation of multiple targets in Streptomyces. Nucleic Acids Res. 48, 8188–8202. doi: 10.1093/nar/gkaa602
van der Heul, H. U., Bilyk, B. L., McDowall, K. J., Seipke, R. F., and van Wezel, G. P. (2018). Regulation of antibiotic production in actinobacteria: new perspectives from the post-genomic era. Nat. Prod. Rep. 35, 575–604. doi: 10.1039/c8np00012c
Wilkinson, C. J., Hughes-Thomas, Z. A., Martin, C. J., Bohm, I., Mironenko, T., Deacon, M., et al. (2002). Increasing the efficiency of heterologous promoters in actinomycetes. J. Mol. Microbiol. Biotechnol. 4, 417–426.
Wu, H., Chen, M., Mao, Y., Li, W., Liu, J., Huang, X., et al. (2014a). Dissecting and engineering of the TetR family regulator SACE_7301 for enhanced erythromycin production in Saccharopolyspora erythraea. Microb. Cell Fact. 13, 1–11.
Wu, H., Chu, Z., Zhang, W., Zhang, C., Ni, J., Fang, H., et al. (2019). Transcriptome-guided target identification of the TetR-like regulator SACE_5754 and engineered overproduction of erythromycin in Saccharopolyspora erythraea. J. Biol. Eng. 13:11.
Wu, H., Wang, Y., Yuan, L., Mao, Y., Wang, W., Zhu, L., et al. (2016). Inactivation of SACE_3446, a TetR family transcriptional regulator, stimulates erythromycin production in Saccharopolyspora erythraea. Synth. Syst. Biotechnol. 1, 39–46. doi: 10.1016/j.synbio.2016.01.004
Wu, P., Pan, H., Zhang, C., Wu, H., Yuan, L., Huang, X., et al. (2014b). SACE_3986, a TetR family transcriptional regulator, negatively controls erythromycin biosynthesis in Saccharopolyspora erythraea. J. Ind. Microbiol. Biotechnol. 41, 1159–1167. doi: 10.1007/s10295-014-1449-9
Xia, H., Li, X., Li, Z., Zhan, X., Mao, X., and Li, Y. (2020). The application of regulatory cascades in streptomyces: yield enhancement and metabolite mining. Front. Microbiol. 11:406. doi: 10.3389/fmicb.2020.00406
Xie, S., Shen, B., Zhang, C., Huang, X., and Zhang, Y. (2014). sgRNAcas9: a software package for designing CRISPR sgRNA and evaluating potential off-target cleavage sites. PLoS One 9:e100448. doi: 10.1371/journal.pone.0100448
Xu, F., Wang, J., and Zhao, G. P. (2017). Alpha-ketoglutarate protects Streptomyces coelicolor from visible light-induced phototoxicity. Biochem. Biophys. Rep. 9, 22–28. doi: 10.1016/j.bbrep.2016.11.002
Xu, Y., Ke, M., Li, J., Tang, Y., Wang, N., Tan, G., et al. (2019a). TetR-type regulator SLCG_2919 is a negative regulator of lincomycin biosynthesis in Streptomyces lincolnensis. Appl. Environ. Microbiol. 85:e02091-18. doi: 10.1128/AEM.02091-18
Xu, Y., You, D., Yao, L. L., Chu, X., and Ye, B. C. (2019b). Phosphate regulator PhoP directly and indirectly controls transcription of the erythromycin biosynthesis genes in Saccharopolyspora erythraea. Microb. Cell Fact. 18:206. doi: 10.1186/s12934-019-1258-y
Xu, Z., Liu, Y., and Ye, B.-C. (2018). PccD regulates branched-chain amino acid degradation and exerts a negative effect on erythromycin production in Saccharopolyspora erythraea. Appl. Environ. Microbiol. 84:e00049-18.
Yang, Y. H., Song, E., Lee, B. R., Kim, E. J., Park, S. H., Kim, Y. G., et al. (2010). Rapid functional screening of Streptomyces coelicolor regulators by use of a pH indicator and application to the MarR-like regulator AbsC. Appl. Environ. Microbiol. 76, 3645–3656. doi: 10.1128/AEM.02617-09
Zhang, Q., Chen, Q., Zhuang, S., Chen, Z., Wen, Y., and Li, J. (2015). A MarR family transcriptional regulator, DptR3, activates daptomycin biosynthesis and morphological differentiation in Streptomyces roseosporus. Appl. Environ. Microbiol. 81, 3753–3765. doi: 10.1128/AEM.00057-15
Keywords: TetR-family regulator, erythromycin, MarR-family regulator, Saccharopolyspora erythraea, regulatory network, CRISPRi, system metabolic engineering
Citation: Liu Y, Khan S, Wu P, Li B, Liu L, Ni J, Zhang H, Chen K, Wu H and Zhang B (2021) Uncovering and Engineering a Mini-Regulatory Network of the TetR-Family Regulator SACE_0303 for Yield Improvement of Erythromycin in Saccharopolyspora erythraea. Front. Bioeng. Biotechnol. 9:692901. doi: 10.3389/fbioe.2021.692901
Received: 09 April 2021; Accepted: 14 May 2021;
Published: 14 September 2021.
Edited by:
Jianting Zheng, Shanghai Jiao Tong University, ChinaCopyright © 2021 Liu, Khan, Wu, Li, Liu, Ni, Zhang, Chen, Wu and Zhang. This is an open-access article distributed under the terms of the Creative Commons Attribution License (CC BY). The use, distribution or reproduction in other forums is permitted, provided the original author(s) and the copyright owner(s) are credited and that the original publication in this journal is cited, in accordance with accepted academic practice. No use, distribution or reproduction is permitted which does not comply with these terms.
*Correspondence: Hang Wu, d3VoYW5nQGFodS5lZHUuY24=; Buchang Zhang, emhiY0BhaHUuZWR1LmNu
†These authors have contributed equally to this work
Disclaimer: All claims expressed in this article are solely those of the authors and do not necessarily represent those of their affiliated organizations, or those of the publisher, the editors and the reviewers. Any product that may be evaluated in this article or claim that may be made by its manufacturer is not guaranteed or endorsed by the publisher.
Research integrity at Frontiers
Learn more about the work of our research integrity team to safeguard the quality of each article we publish.