- 1Clostridia Research Group, BBSRC/EPSRC Synthetic Biology Research Centre (SBRC), Biodiscovery Institute, School of Life Sciences, University of Nottingham, Nottingham, United Kingdom
- 2CHAIN Biotechnology Ltd., MediCity, Nottingham, United Kingdom
Chirally pure (R)-1,3-butanediol ((R)-1,3-BDO) is a valuable intermediate for the production of fragrances, pheromones, insecticides and antibiotics. Biotechnological production results in superior enantiomeric excess over chemical production and is therefore the preferred production route. In this study (R)-1,3-BDO was produced in the industrially important whole cell biocatalyst Clostridium saccharoperbutylacetonicum through expression of the enantio-specific phaB gene from Cupriavidus necator. The heterologous pathway was optimised in three ways: at the transcriptional level choosing strongly expressed promoters and comparing plasmid borne with chromosomal gene expression, at the translational level by optimising the codon usage of the gene to fit the inherent codon adaptation index of C. saccharoperbutylacetonicum, and at the enzyme level by introducing point mutations which led to increased enzymatic activity. The resulting whole cell catalyst produced up to 20 mM (1.8 g/l) (R)-1,3-BDO in non-optimised batch fermentation which is a promising starting position for economical production of this chiral chemical.
Introduction
Chirally active compounds are much sought after by the chemical and pharmaceutical industry. The R-form of 1,3-butanediol (1,3-BDO), for example, is used for fragrances, pheromones, insecticides and as starting material for the production of azetidinone derivatives. The latter are intermediates of penem and carbapenem antibiotic synthesis (Nakatsuka et al., 1991; Matsuyama et al., 2001), a major area of development. Old β-lactam antibiotics lose their efficacy against bacteria due to emergent resistances which makes new discoveries crucial (Llarrull et al., 2010; Qin et al., 2014). This makes the cost-effective production of precursor molecules an important field of study.
Chemical production of (R)-1,3-BDO from threonine via asymmetric hydrogenation of 4-hydroxy-2-butanone (4H2B) has been studied, but satisfactory enantiomeric purity was not achieved (Larchevêque et al., 1991; Boaz et al., 2006). Enzymatic production of (R)-1,3-BDO has the potential to be cheaper than chemical production from petroleum-based substrates and its stereo-specificity is superior with up to 100% of the product yield having the desired conformation. Different whole cell catalysis approaches have been adopted with mixed results. Reduction through yeast fermentation of 4H2B led to low product yields when the desired stereo-specificity was reached (Matsuyama et al., 2001). However, later research showed promising results when reducing 4H2B using either newly isolated yeast strains, with up to 100% enantiomeric excess and a titre of 38.2 g/l (Zheng et al., 2012; Yang et al., 2014), or genetically engineered E. coli, where a 99% enantiomeric excess and 99% substrate yield were obtained (Itoh et al., 2007). Another approach using genetically modified E. coli based on the enantio-selective oxidation of (S)-1,3-BDO was initially promising (Matsuyama et al., 2001) but proved difficult to scale-up (Yamamoto et al., 2002).
As all of the above studies were reliant on relatively expensive substrates, the use of cheaper alternatives would benefit process economics. Two notable alternative routes to (R)-1,3-BDO have been explored. Nemr et al. (2018) assembled an elaborate metabolic pathway in E. coli that mediated the condensation of two pyruvate-derived molecules of acetaldehyde to form (R)-3-hydroxybutyrate ((R)-3-HB) and further to (R)-1,3-BDO. (R)-1,3-BDO yield equivalent to 13% of the theoretical maximum from glucose was achieved. In another approach, Kataoka et al. (2013) designed a synthetic pathway in E. coli encoding the acetyl-CoA acetyltransferase (phaA) and the acetoacetyl-CoA reductase (phaB) derived from Cupriavidus necator, combined with the Clostridium saccharoperbutylacetonicum-derived butyraldehyde dehydrogenase (bld) to produce 100.4 mM (9.05 g/l) (R)-1,3-BDO with 98.5% enantiomeric excess in batch fermentation. Fed-batch operation subsequently increased the product titre to 174.8 mM (15.8 g/l) (Kataoka et al., 2014).
C. saccharoperbutylacetonicum DSM 14923 (Hongo et al., 1968) is a widely used industrial acetone-butanol-ethanol- (ABE-) fermenting organism which is renowned for its particularly high n-butanol yields (Shaheen et al., 2000; Herman et al., 2017) and industrially favourable low sporulation capability (Keis et al., 2001). It has been found to grow on a wide range of industrially relevant first- and second-generation feedstocks, such as glucose, cellobiose, xylose, arabinose, mannose, galactose, pentose, starch and molasses (Shaheen et al., 2000; Yoshida et al., 2012; Yao et al., 2017). These characteristics make C. saccharoperbutylacetonicum an optimal whole cell biocatalyst for industrial applications. Since C. saccharoperbutylacetonicum possesses the necessary alcohol dehydrogenases and an inherently strong acetyl-CoA acetyltransferase (thl), introduction of the enantioselective PhaB from C. necator should be sufficient to produce (R)-1,3-BDO in this organism, obviating the need for multiple engineering steps. Green et al. (2017) expressed a phaB gene codon optimised for generic Clostridium spp. in C. saccharoperbutylacetonicum and observed 0.25 mM of (R)-1,3-BDO (plasmid expression) and 6 mM (R)-1,3-BDO (chromosomal integration) with no production of (S)-1,3-BDO. This study improves on earlier work engineering C. saccharoperbutylacetonicum for production of chirally pure (R)-1,3-BDO, through optimisation of PhaB-mediated catalysis at transcriptional, translational, enzyme and population levels.
Materials and Methods
Bacterial Strains, Growth, and Maintenance Conditions
E. coli HB101 (NEB, United Kingdom) was used as a host strain for plasmid propagation. E. coli was grown at 30°C or 37°C in Luria-Bertani (LB) broth or on LB-agar with 500 μg/ml erythromycin or 100 μg/ml spectinomycin. Unless stated otherwise, all chemicals were purchased from Sigma Aldrich, United Kingdom. The parent wild type strain C. saccharoperbutylacetonicum N1-4 (HMT) DSM14923 (Hongo et al., 1968) was purchased from the German collection of microorganisms and cell cultures GMBH (DSMZ). All C. saccharoperbutylacetonicum strains were grown in an anaerobic cabinet (Don Whitley, Yorkshire, United Kingdom) or tent (Coy Lab Products, United States) with artificial atmosphere (80:10:10 volume% nitrogen: carbon dioxide: hydrogen) at 37°C on solid RCM agar or on solid CBM (O’Brien and Morris, 1971). Standard liquid cultures were grown unshaken in TYA (Tashiro et al., 2004) with, per litre in g: yeast extract, 2; tryptone, 6; ammonium acetate, 3; MgSO4.7H2O, 0.3; KH2PO4, 0.5; FeSO4.7H2O, 0.01. Growth and solvent production assays of C. saccharoperbutylacetonicum were performed in CGM, 2xYTG, CBM, P2 and Biebl medium, with the following compositions per litre in g: CGM was prepared as described by Hartmanis and Gatenbeck with some modification (Hartmanis and Gatenbeck, 1984) yeast extract, 5; KH2PO4, 0.75; K2HPO4, 0.75; MgSO4.7H2O, 0.4; MnSO4.H2O, 0.01; FeSO4.7H2O, 0.01; NaCl, 1; asparagine, 2; (NH4)2SO4, 2; glucose, 50; CaCO3, 20. 2xYTG contained: yeast ectract, 10; tryptone, 16; NaCl, 5. CBM similar to O’Brien and Morris (1971), MgSO4.7H2O, 0.2; MnSO4.H2O, 0.00758; FeSO4.7H2O, 0.01; p-aminobenzoic acid, 0.001; biotin, 2 × 10–6; thiamine HCl, 0.001; casein hydrolysate, 4; K2HPO4, 0.5; KH2PO4, 0.5 (O’Brien and Morris, 1971). P2 similar to Baer et al. (1987), K2HPO4, 0.5; KH2PO4, 0.5; ammonium acetate, 2.2; MgSO4.7H2O, 2; MnSO4.H2O, 0.1; NaCl, 0.1; FeSO4.7H2O, 0.1; p-aminobenzoic acid, 0.1; thiamine, 0.1; biotine, 0.01 (Baer et al., 1987). Biebl media composition similar to Biebl, 2001: KH2PO4, 0.5; K2HPO4, 0.5; (NH4)2SO4, 5; MgSO4.7H2O, 0.2; CaCl2.2H2O, 0.02; FeSO4.7H2O, 0.00914; biotin, 25 × 10–6; 2 ml trace element solution made up of, per litre in mg: MnCl2.4H2O, 100; ZnCl2, 70; H3BO3, 60; NaMoO4.2H2O, 40; CoCl2.6H2O, 28.7; CuCl2.2H2O, 20; NiCl2.6H2O, 20; 1 ml 25% HCl (Biebl, 2001). All media were prepared with 50 g/l glucose and 20 g/l CaCO3 and adjusted to pH 6.2. For antibiotic selection in C. saccharoperbutylacetonicum, cultures were supplemented with 50 μg/ml erythromycin or 250 μg/ml spectinomycin.
Growth curves were done by growing C. saccharoperbutylacetonicum strains until mid-exponential phase for inoculation of triplicate 35 ml CGM broths to an OD600 of 0.05 in 250 ml serum bottles. The bottles were capped with rubber-butyl stoppers inside a cabinet and transferred outside the cabinet for crimping and static incubation at 37°C. Glucose, acids and solvents including (R)-3-HB and (R)-1,3-BDO were quantified with HPLC as previously described (Schwarz et al., 2017). Data was analysed with Graphpad PRISM 8.1 (Graphpad Software Inc., United States). The chirality of 3-HB and 1,3-BDO is determined using LC/MS analysis following DATAN (Diacetyl-L-Tartaric Anhydride) derivatisation (Struys et al., 2004).
Clostridium saccharoperbutylacetonicum Electroporation
Electro-competent Clostridium cells were prepared based on the method described by Little et al. (2018). Culture in exponential phase was used to inoculate 300 ml TYA to OD600 0.05 and grown to mid-exponential phase (OD600 0.4–0.6). Cells were centrifuged at 7,500 rpm for 10 min and washed twice with ice-cold, reduced EPB buffer (270 mM Sucrose, 5 mM Na2HPO4, 5 mM NaH2PO4, pH 7.4), resuspended in 9 ml ice-cold EPB buffer containing 10% dimethyl sulphoxide and aliquots frozen at −80°C. For electroporation 580 μl of competent cells and typically 6 μg plasmid were added to a 4 mm electroporation cuvette inside an anaerobic cabinet. The mixture was kept on ice for 5 min before being electroporated with pulse settings of 2 kV, 25 μF and ∞Ω. Cells were immediately mixed with 10 ml warm TYA to recover for 6 h. Finally, cells were plated out on appropriate medium with appropriate antibiotics for selection.
Plasmid Stability Assay
Plasmid segregational stability was determined as described previously (Heap et al., 2009). Briefly, C. saccharoperbutylacetonicum transformants were cultured for 12 h in TYA medium supplemented with the antibiotic erythromycin to select for the plasmid. The culture was washed twice in PBS to remove the antibiotic and then used to inoculate fresh, unsupplemented TYA medium at 1% (vol/vol). This marked the start (i.e., 0 h) of the stability experiment. The unsupplemented culture was then diluted 1% (vol/vol) into fresh medium every 12 h. At 12, 24, 36, 48, 60, 72, 84, and 96 h the culture was plated on both selective and non-selective agar to enumerate erythromycin-resistant colony-forming units and total colony-forming units.
Plasmid Cloning
All restriction enzymes used were fast digest enzymes (ThermoFisher Scientific, United Kingdom), the ligase used throughout this work was the Rapid DNA Ligation Kit (ThermoFisher Scientific, United Kingdom). Plasmids were transformed into chemically competent E. coli HB101 according to supplier’s instructions (New England Biolabs, United Kingdom). Plasmids are listed in Supplementary Table 1 and primers and synthetic DNA fragments are listed in Supplementary Table 2.
Plasmid pMTL83353::phaB and Derivatives
Plasmid pMTL83353::phaB (p_phaB) was cloned by digesting pMTL83251-pfdx_PhaB (Green et al., 2017) (featuring the phaB gene from C. necator N-1 codon optimised for Clostridium spp.) and pMTL83353 (Heap et al., 2009) with NdeI and NheI, ligating the fragment containing phaB into the pMTL83353 backbone. The point-mutated p_phaB derivatives were produced using the Quikchange site-directed mutagenesis kit according to supplier’s instruction (Agilent, United Kingdom) using primers Q47L_F and Q47L_R to produce pMTL83353::phaB_Q47L (p_phaB_L) and primers T173S_F and T173_R to produce pMTL83353::phaB_T173S (p_phaB_S). To produce the plasmid pMTL83353::phaB_Q47L_T173S (p_phaB_LS) carrying the phaB double-mutant, plasmid p_phaB_L was point-mutated using the Quikchange site directed mutagenesis kit as above with primers T173S_F and T173S_R.
The C. necator phaB gene was codon optimised to match the C. saccharoperbutylacetonicum codon adaptation index using the codon usage table calculated by Nakamura et al. at https://www.kazusa.or.jp/codon/(accessed 3/9/2019) (Nakamura et al., 2000). The codon optimised phaB fragment (phaB-opt) and the point mutated derivatives phaB-opt_Q47L, phaB-opt_T173S and phaB-opt_Q47L_T173S were ordered as synthetic DNA fragments (Twist Bioscience, United States) flanked by NotI and NdeI restriction sites upstream of the gene and HindIII and NheI downstream of the gene. The fragments were cloned into pMTL83353 (Heap et al., 2009) by digesting the plasmid and the fragments with NdeI and NheI and ligating each fragment separately into the digested backbone to obtain plasmids pMTL83353::phaB-opt_Q47L (p_phaB-opt_L), pMTL83353::phaB-opt_T173S (p_phaB-opt_S) and pMTL83353::phaB-opt_Q47L_T173S (p_phaB-opt_LS).
ACE Plasmids
The Allele Coupled Exchange (ACE) (Heap et al., 2012) pyrE truncation plasmid pMTL-AGH21 (p_AGH21) was cloned by amplifying the following with Phusion High-Fidelity DNA Polymerase (New England Biolabs, United Kingdom): (i) a 300 bp fragment of the DSM14923 pyrE gene with primers pE_upstream_F and SOE_pE_trunc_up_R; (ii) the 282 bp multiple cloning site (MCS) from pMTL-ME6C (Heap et al., 2012)with primers SOE_pE_trunc_up_F and SOE_pE_trunc_dwn_R; (iii) the 1,200 bp downstream region of the DSM14923 pyrE locus with primers SOE_pE_trunc_dwn_F and pE_downstream_R. All three fragments were spliced together by splicing by overlap extension PCR (SOE-PCR) with primers pE_upstream_F and pE_downstream_R for ligation into pMTL85241 (Heap et al., 2009) at SbfI and AscI sites.
The cargo delivery ACE plasmid pMTL-AGH23 (p_AGH23) was cloned by amplifying the following: (i) a 626 bp fragment of pyrE with primers pE_upstream_F and SOE_pE_KI_up_R; (ii) a 323 bp fragment of the MCS from pMTL-ME6C (Heap et al., 2012) with primers SOE_pE_KI_up_F and SOE_pE_KI_dwn_R; (iii) a 1,200 bp fragment downstream of the DSM14923 pyrE locus with primers SOE_pE_KI_dwn_F and pE_downstream_R. The three fragments were used in a SOE-PCR as above with primers pE_upstream_F and pE_downstream_R, for ligation into pMTL85241.
Plasmid pMTL-AGH23::cspo_fdx-phaB was cloned by digesting p_phaB and p_AGH23 with enzymes NotI and NheI and ligating the resulting phaB fragment into the p_AGH23 backbone. The same process led to production of pMTL-AGH23::cspo_fdx-phaB-opt using plasmids p_AGH23 and p_phaB-opt and pMTL-AGH23::cspo_fdx-phaB-opt_Q47L_T173S using p_AGH23 and p_phaB-opt_LS. Plasmids pMTL-AGH23::cac_ptb-phaB-opt and pMTL-AGH23::cac_ptb-phaB-opt_Q47L_T173S were produced by exchanging the promoter Pcspo_fdx with promoter Pcac_ptb from plasmid pMTL8225::cac_ptb_gusA (p_ptb-gusA) through digestion of pMTL-AGH23::cspo_fdx-phaB or pMTL-AGH23::cac_ptb-phaB-opt and p_ptb-gusA with NotI and NdeI cloning the Pcac_ptb promoter into the 5,215 bp backbone.
gusA Reporter Plasmids
Reporter plasmids to measure promoter strength were cloned fusing promoters from a promoter library (Willson, 2014) with a gusA (β-glucuronidase) gene. The latter was based on plasmid pMTL-JL1 (pMTL8514::fdx-gusA) which consisted of the Streptococcus agalactiae gusA gene (Wallace et al., 2015) under control of the C. acetobutylicum ferredoxin gene promoter in the modular shuttle vector pMTL85141. Plasmids pMTL8225::cac_araE-gusA (p_araE-gusA), pMTL8225::cac_adhE-gusA (p_adhE-gusA), pMTL8225::cac_ptb-gusA (p_ptb-gusA) and pMTL8225:: pj23119-gusA (p_pj-gusA) were constructed by digesting both pMTL8514::fdx-gusA and pMTL82251 (Heap et al., 2009) with NotI and NheI and ligating the fdx-gusA fragment into the pMTL82251 backbone. Plasmids pMTL8225::cbe_fdx-gusA (p_cbe_fdx-gusA), pMTL8225::cte_fdx-gusA (p_cte_fdx-gusA), pMTL8225::sac_fdx-gusA (p_sac_fdx-gusA), pMTL8225::clk_ fdx-gusA (p_clk_fdx-gusA), pMTL8225::cpf_fdx-gusA (p_cpf_fdx-gusA), pMTL8225::cbe_thl-gusA (p_cbe_thl-gusA), pMTL8225::ccv_thl-gusA (p_ccv_thl-gusA) and pMTL8225:: cby_thl-gusA (p_cby_thl-gusA) were cloned by digesting the corresponding pMTL82254 based plasmids (Willson, 2014; Supplementary Table 1) and p_ptb-gusA with enzymes NotI and NdeI and cloning the [promoter] fragment excised from pMTL82254::[promoter] into the backbone of p_ptb-gusA. The same process led to production of pMTL8225::cspo_fdx-gusA (p_cspo_fdx-gusA) by digestion of pMTL83353 (Heap et al., 2009) and p_ptb-gusA with NotI and NdeI and ligating the cspo_fdx fragment into the backbone.
Allelic Exchange Mutant Generation
C. saccharoperbutylacetonicumΔpyrE truncation and gene insertions downstream of pyrE were made as described by Heap et al. (2012). C. saccharoperbutylacetonicum DSM14923 was transformed with plasmid p_AGH21 followed by selection on RCM plates containing erythromycin. Faster growing colonies (potential single crossover integrants) were restreaked three times on CBM agar supplemented with 400 μg/ml 5-fluoroorotic acid and 20 μg/ml uracil and tested by colony PCR and subsequent Sanger sequencing (Eurofins Genomics, United Kingdom) for pyrE truncation and absence of plasmid. Colony PCR was performed as previously described for Clostridium pasteurianum (Pyne et al., 2013) with primers pE_genome_F and pE_genome_R. PCR products were sequenced with primer ACE_insert_seq_F. Plasmid loss was confirmed by colony PCR with primers 85XXX-RF and 8X2XX-RR.
Cargo integrated strains C. saccharoperbutylacetonicum Ω Cspo_fdx-phaB (Ωfdx-phaB), ΩCspo_fdx-phaB-opt (Ωfdx- phaB-opt), ΩCac_ptb-phaB-opt (Ωptb-phaB-opt), ΩCspo_ fdx-phaB-opt_Q47L_T173S (Ωfdx-phaB-opt_LS) and ΩCac_ptb-phaB-opt_Q47L_T173S (Ωptb-phaB-opt_LS) were produced by transforming the corresponding pMTL-AGH23 cargo plasmid into C. saccharoperbutylacetonicumΔpyrE followed by direct selection for double-crossover integration on CBM plates without uracil. Integration was tested by PCR with primers pE_genome_F and pE_genome_R leading to a 2,836 bp fragment for Ωfdx-phaB, a 2,892 bp fragment for both Pcspo_fdx promoter based gene insertions and a 2,895 bp fragment for both Pcac_ptb promoter-based insertions if integration was successful, compared to 2,213 bp in C. saccharoperbutylacetonicumΔpyrE. PCR products were sequenced with primers ACE_insert_seq_F and ACE_insert_seq_R. Plasmid loss was confirmed by PCR with primers 85XXX-RF and 8X2XX-RR.
GusA Reporter Assay
Promoter strength in C. saccharoperbutylacetonicum was tested by measuring GusA activity when gusA was expressed from different promoters according to protocols by Miller (1972). Cell pellets from 1 ml cultures were defrosted and resuspended in up to 500 μl of Z-buffer (60 mM Na2HPO4, 45 mM NaH2PO4, 10 mM KCl, 1 mM MgSO2.7H2O, 50 mM β-mercaptoethanol, pH 8.5) to normalise OD600 across all samples. A 75 μl volume of the sample was transferred to a Corning 96 Well Black Polystyrene Microplate with clear bottom (Merck, United Kingdom) and OD600 measured in a Clariostar Plus plate reader (BMG Labtech, United Kingdom). Reaction was started by addition of 25 μl of 40 μg/ml 4-methylumbelliferyl-β-D-glucuronide dissolved in Z-buffer to each sample. Fluorescence was measured for 30 min at 450 nm after excitation at 360 nm at a distance of 7 mm and with a gain of 750. The resulting data was analysed in Excel (Microsoft Corporation, United States) by measuring slope of the regression line in the linear phase and the data was further analysed in GraphPad PRISM 8.1 (GraphPad Software Inc., United States).
Results
Based on previous studies in E. coli (Kataoka et al., 2013), a pathway to chirally pure (R)-1,3-BDO in C. saccharoperbutylacetonicum was formulated based on the conversion of acetoacetyl-CoA to (R)-3-hydroxybutyryl-CoA using the C. necator enzyme acetoacetyl-CoA reductase encoded by phaB. In E. coli the condensation of two acetyl-CoA to one acetoacetyl-CoA was reliant on the over-expression of the C. necator phaA gene (coding for 3-ketothiolase). This was not necessary in C. saccharoperbutylacetonicum since the endogenous thiolase gene (thlA) is highly expressed (Wiesenborn et al., 1988). Furthermore, the endogenous enzymes alcohol- and aldehyde-alcohol dehydrogenases (bdh and adh) should catalyse the reactions from (R)-3-hydroxybutyryl-CoA via (R)-3-hydroxybutyraldehyde to (R)-1,3-BDO analogous to reduction of butyryl-CoA to butanol (Figure 1). The side product (R)-3-HB was expected to be produced predominantly through conversion of (R)-3-hydroxybutyryl-CoA via the endogenous phosphotransbutyrylase (ptb) to (R)-3-hydroxybutyryl-P and further via butyrate kinase (buk) to (R)-3-HB in analogy to the natural conversion of butyryl-CoA to butyrate. Additionally, some (R)-3-HB maybe produced by unspecific hydrolysis of the CoA thioester bond by endogenous thioesterases analogous to E. coli (Guevara-Martínez et al., 2019).
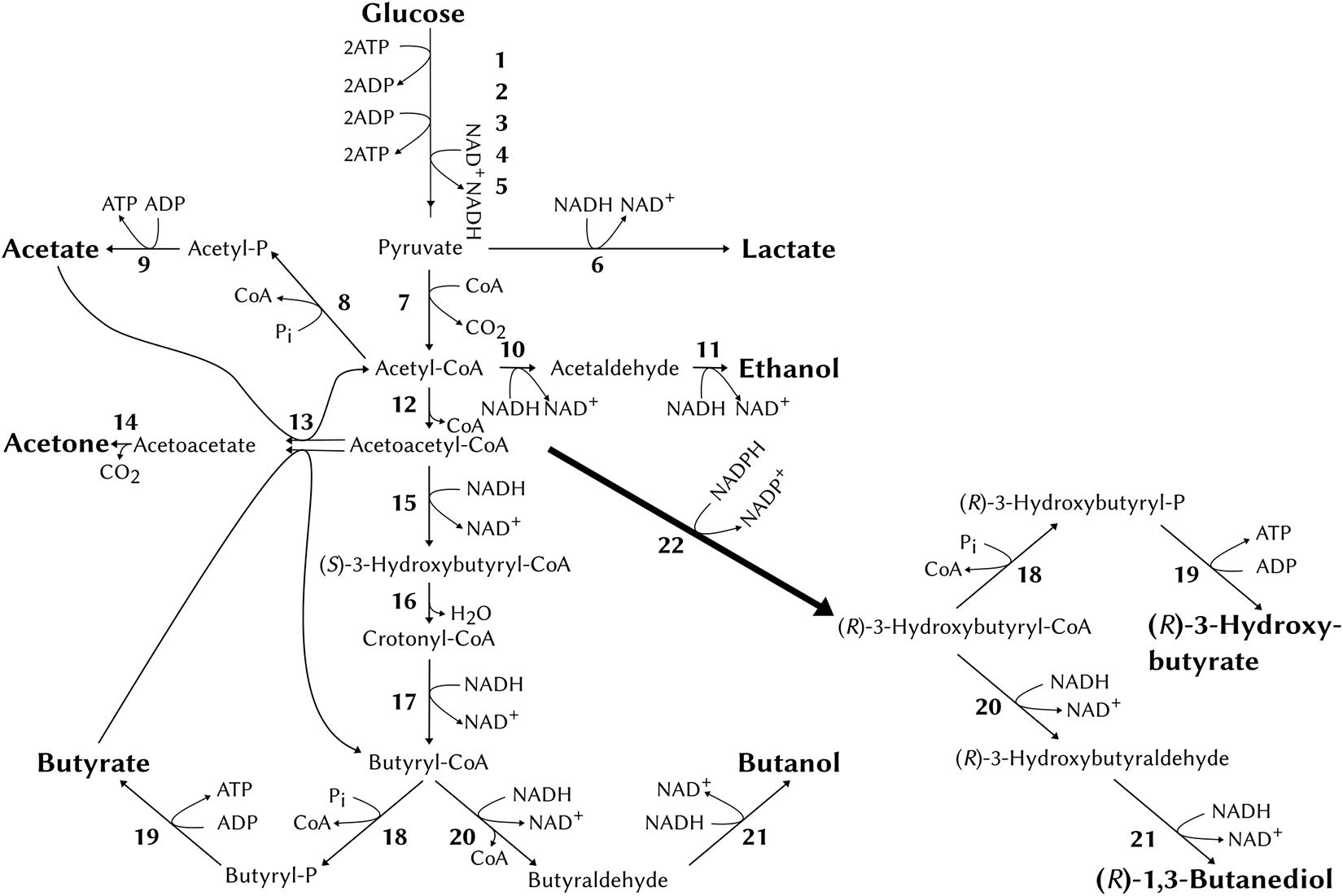
Figure 1. The natural acid- and solvent- producing pathways of C. saccharoperbutylacetonicum and the heterologous pathway from acetoacetyl-CoA via PhaB (acetoacetyl-CoA reductase, 22). Numbers correspond to enzymes as follows: 1, hexokinase; 2, phosphoglucose isomerase; 3, phosphofructokinase; 4, glyceraldehyde-3-phosphate dehydrogenase; 5, pyruvate kinase; 6, lactate dehydrogenase; 7, pyruvate-ferredoxin oxidoreductase; 8, phosphate acetyltransferase; 9, acetate kinase; 10, acetaldehyde dehydrogenase; 11, ethanol dehydrogenase; 12, thiolase; 13, acetoacetyl-CoA:acetate:CoA transferase/acetoacetyl-CoA:butyrate:CoA transferase; 14, acetoacetate decarboxylase; 15, β-hydroxybutyryl-CoA dehydrogenase; 16, crotonase; 17, butyryl-CoA dehydrogenase; 18, phosphotransbutyrylase; 19, butyrate kinase; 20, butyraldehyde dehydrogenase; 21, butanol dehydrogenase; 22, acetoacetyl-CoA reductase (phaB, from Cupriavidus necator).
Growth and Solvent Production
Various growth media were tested for solvent production from C. saccharoperbutylacetonicum DSM 14923 with the expectation that media supporting high solvent production would also promote (R)-1,3-BDO production (Supplementary Figure 1). The strain produced the highest yield of solvents per glucose (g solvents/g glucose) when grown in complex media (CGM and TYA) with 0.28 ± 0.02 g/g and 0.28 ± 0.00 g/g, respectively. In minimal Biebl medium the strain still produced 0.24 ± 0.00 g/g solvents. The highest solvent to acid ratio was found when growing on minimal media P2 or Biebl with 4.4 g/g (g solvents/g acids) and 4.3 g/g, respectively, while the ratio was 3.2 g/g for CGM and 3.5 g/g for TYA. However, since PhaB uses the four-carbon intermediate acetoacetyl-CoA to produce (R)-3-hydroxybutyryl-CoA, the 4-carbon to 2-carbon product ratio is more important for the production of (R)-1,3-BDO. This ratio was 5.1 g/g (g 4-carbon products/g 2-carbon products) for CGM, 3.5 g/g for TYA, 3.9 g/g for P2 and 5.0 g/g for Biebl medium. Thus, as it was expected that the highest yields of (R)-1,3-BDO yield in C. saccharoperbutylacetonicum strain would be obtained when grown in CGM, this medium was used throughout the rest of this work.
Plasmid Stability Assays
Four Gram-positive replicons (Heap et al., 2009) were tested for segregational stability in C. saccharoperbutylacetonicum by following the ratio of antibiotic resistant (plasmid containing) cells to total cell counts in non-selective media over time. The two most stable replicons were pBP1 and pCB102 over 8 generations which corresponded to approximately 96 h (Figure 2). Due to its reduced length of 1,625 bp compared to 2,403 bp of pBP1, the second most stable replicon pCB102 was chosen for plasmid-based expression of the heterologous phaB gene, as increasing plasmid size has been shown to negatively correlate with transformation efficiency (Hanahan, 1983).
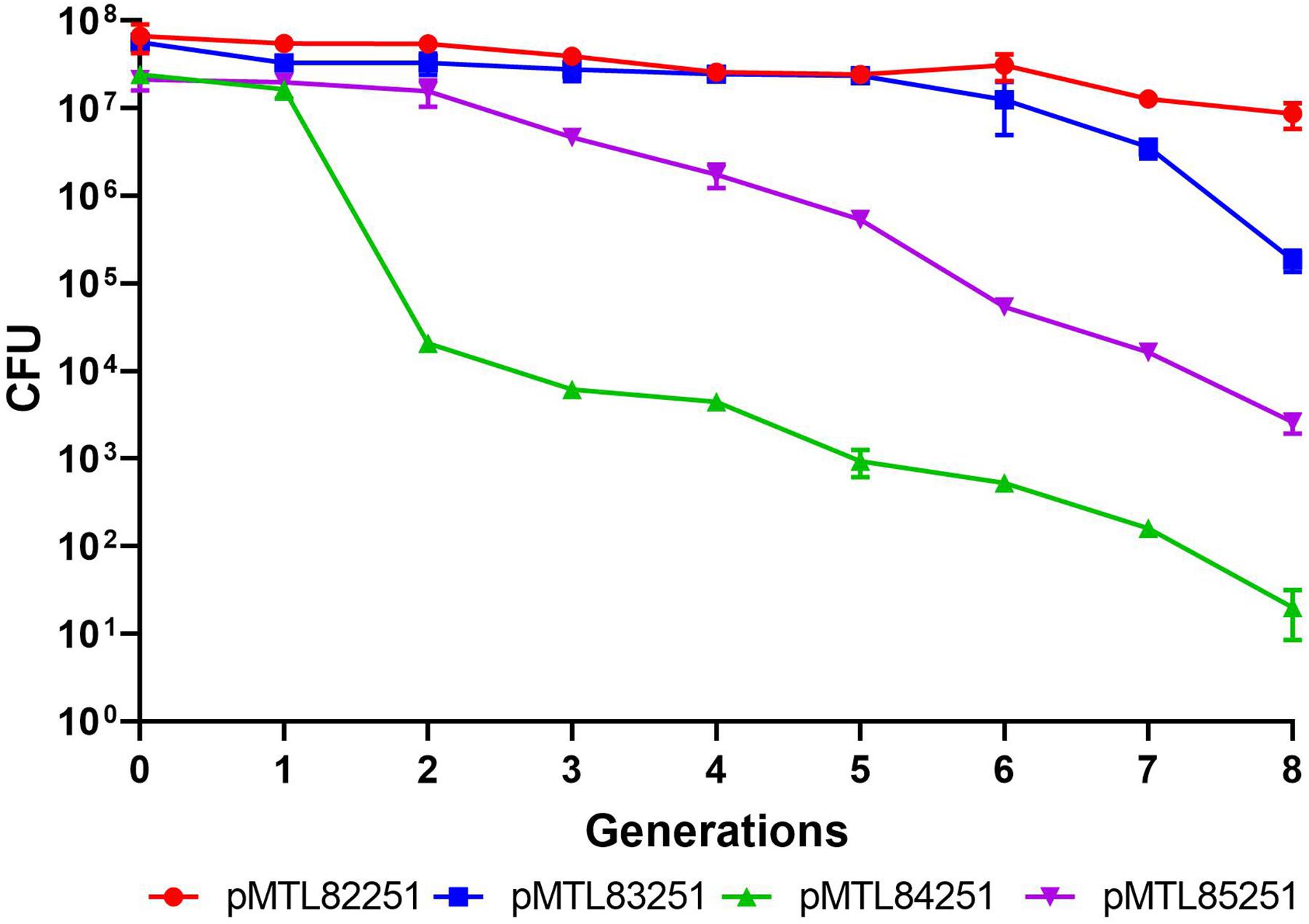
Figure 2. Plasmid stability assay testing four Gram-positive replicons in C. saccharoperbutylacetonicum. Plasmid pMTL82251 with pBP1 replicon in red circles, pMTL83251 with pCB102 replicon in blue squares, pMTL84251 with pCD6 replicon in green upward pointing triangles and pMTL85251 with pIM13 replicon in downward pointing triangles.
Plasmid Borne Expression of phaB With Increased Catalytic Activity
Production of (R)-1,3-BDO was measured in C. saccharoperbutylacetonicum cells containing the plasmid p_phaB expressing the C. necator phaB (coding for acetoacetyl-CoA reductase) gene under the control of the promoter of the Clostridium sporogenes fdx gene (Pcspo_fdx) (Figure 3, also Supplementary Figure 2). A control strain carrying the empty vector pMTL83353 was included for comparative purposes. Expression of phaB slowed growth of the strain considerably and the highest cell density was reached only after 48 h as compared to 24 h in the control. Strain p_phaB produced 6.3 ± 0.1 mM of (R)-1,3-BDO after 48 h while the control did not produce the compound, establishing that the pathway was functioning as intended. The by-product (R)-3-HB was produced at up to 5.1 ± 0.1 mM and is assumed to be reassimilated in the solventogenic phase via acetoacetate-CoA transferase (ctfAB) as it is the case for butyrate and acetate. The chemical chirality of 1,3-BDO and 3-HB were confirmed to be enantio-specific R form in previous study (Green et al., 2017). No S form of either compounds was found in the fermentation broth.
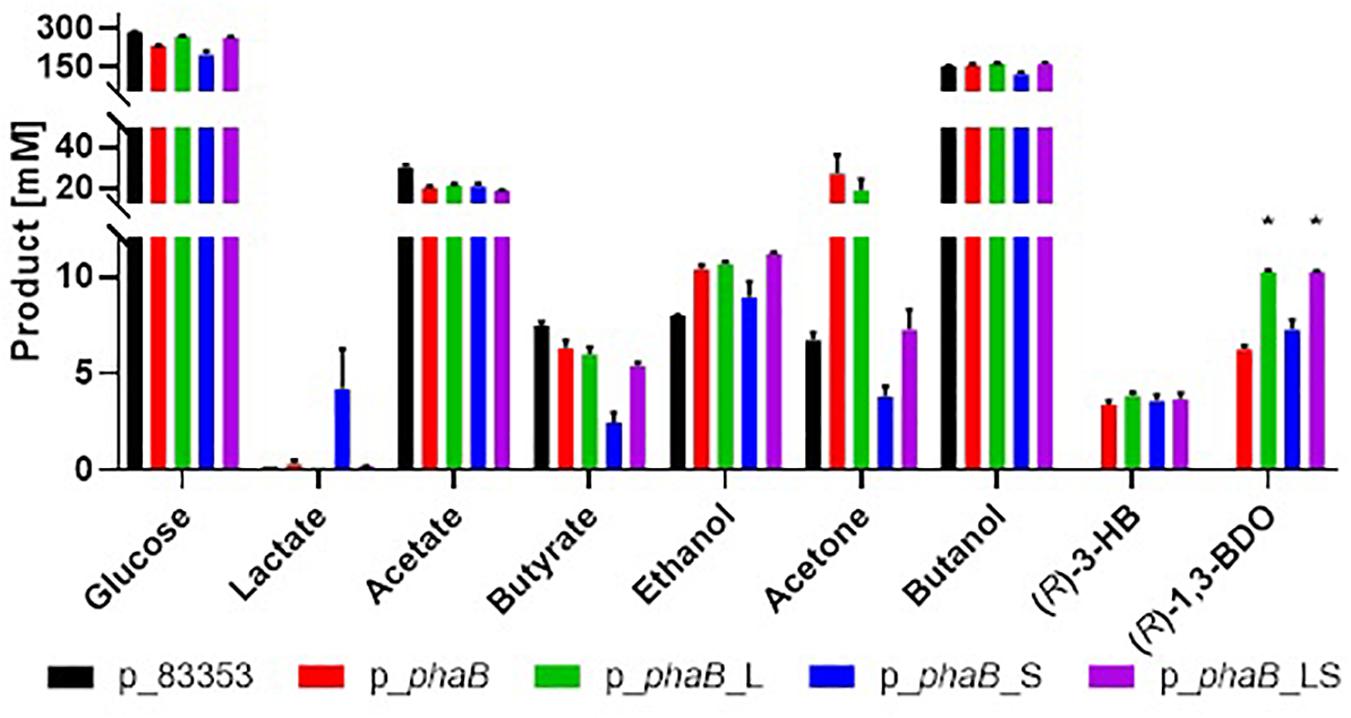
Figure 3. Comparison of product spectrum of Cupriavidus necator wild type phaB and the derivatives with point mutations and negative control without phaB (p_83353) expressed in C. saccharoperbutylacetonicum after 48 h. From left to right for each product p_83353 in black, p_phaB in red, p_phaB_L in green, p_phaB_S in blue, p_phaB_LS in purple. For glucose the consumed amount is shown for all other the produced amount. Error-bars represent SEM and * represents statistical significance against p_phaB (t-test, p < 0.05), n = 3.
Point mutations Q47L and T173S in PhaB have previously been shown to increase activity and poly-3-HB accumulation in Corynebacterium glutamicum (Matsumoto et al., 2013) and in tobacco (Yokoo et al., 2015). Both point mutations were introduced separately into PhaB (plasmids p_phaB_L and p_phaB_S) and a double mutant (plasmid p_phaB_LS) was produced. C. saccharoperbutylacetonicum cells carrying plasmids p_phaB_L, p_phaB_S and p_phaB_LS produced 10.2 ± 0.2, 7.8 ± 0.1 and 10.3 ± 0.1 mM (R)-1,3-BDO, respectively (Figure 3).
Codon Optimisation of Plasmid Expressed Enzyme
The second option chosen to increase product titres after the point mutations, was to translationally optimise the heterologous C. necator gene through the alteration of its codon frequency to match that of C. saccharoperbutylacetonicum. Codon usage frequencies were determined using the database compiled by Nakamura and coworkers (Nakamura et al., 2000). Synonymous substitutions were made selecting codons with the highest frequency of usage in C. saccharoperbutylacetonicum. The resulting gene (phaB-opt), single point mutations (phaB-opt_L & phaB-opt_S) and the double point mutated gene (phaB-opt_LS) were ordered as synthetic constructs. Fermentation performance of the strains carrying codon optimised genes was tested against a plasmid control (Figure 4 and Supplementary Figure 3). Plasmids p_phaB-opt (6.3 ± 0.7 mM) and p_phaB-opt_S (8.8 ± 0.4 mM) produced slightly higher (R)-1,3-BDO concentrations while p_phaB-opt_L (8 ± 0.2 mM) and p_phaB-opt_LS (9.2 ± 0.3 mM) produced slightly less compared to the p_phaB based constructs.
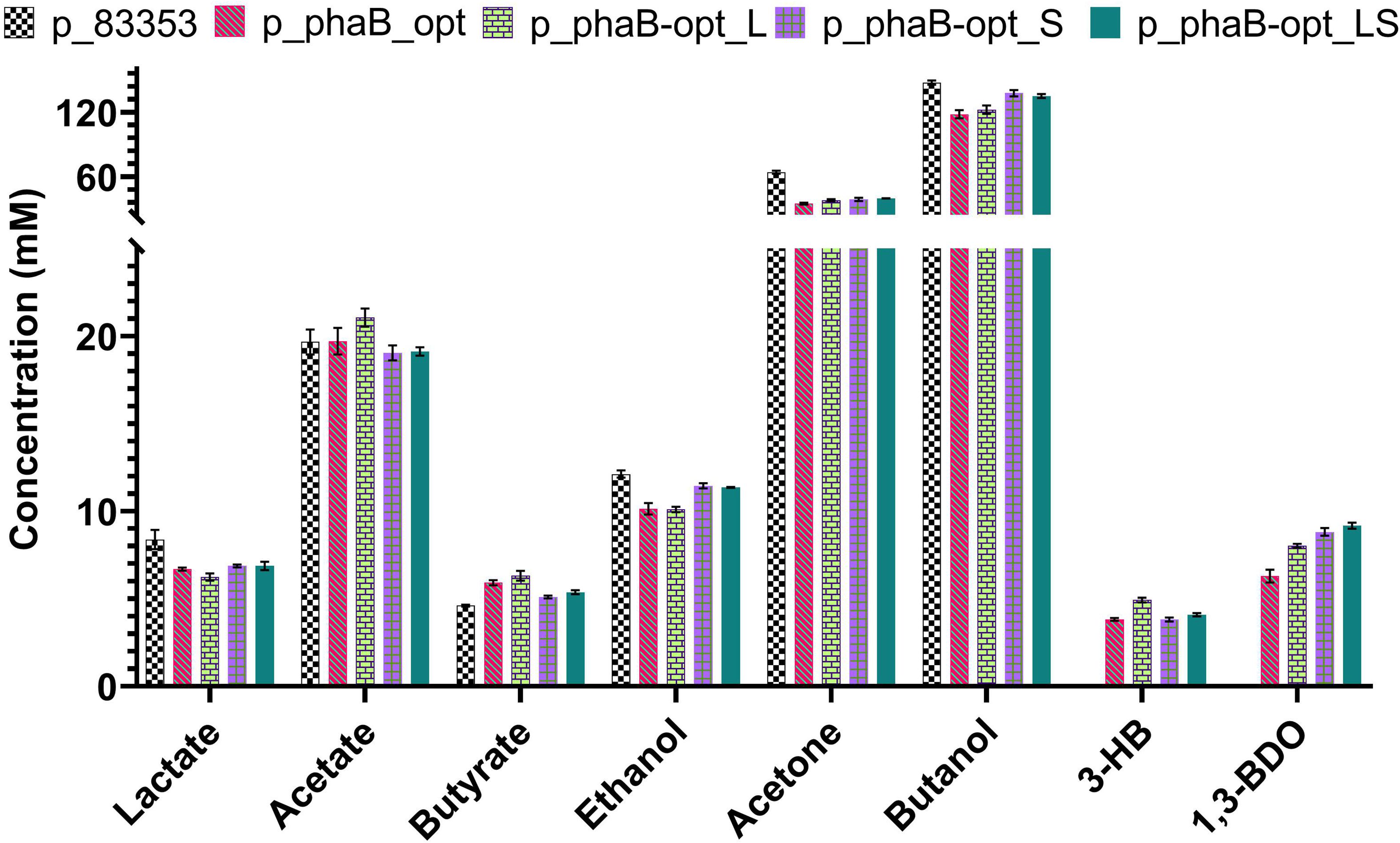
Figure 4. Comparison of product spectrum C. saccharoperbutylacetonicum cells carrying the empty plasmid control p_83353 and codon optimised phaB and its derivatives with point mutations after 48 h. From left to right for each product p_83353 in chequerboard, p_phaB_opt in red diagonal lines, p_phaB-opt_L in green bricks, p_phaB-opt_S in purple squares and p_phaB-opt_LS in green dots. Error-bars represent SEM.
GusA Promoter Strength Assays
Although several native Clostridium and synthetic promoters have been used for enzyme expression in C. saccharoperbutylacetonicum (Nakayama et al., 2008; Zhang et al., 2018) no systematic analysis of promoter strength has been published in this organism so far. A gusA (β-glucuronidase) reporter gene was employed to assess the relative strength of various clostridial promoters, including a library derived from the fdx and thl genes of several different clostridial species (Willson, 2014) as well as a synthetic promoter (iGEM part BBa_J23119). The gusA gene derived from Streptococcus agalactiae DSM 2134 was chosen because of its compatible GC content and codon usage as well as favourable kinetic properties of the encoded enzyme (Wallace et al., 2015), which were similar to those of E. coli GusA previously employed in C. acetobutylicum (Girbal et al., 2003). Relative promoter strength at 24 h post-inoculation was measured and the resulting gusA activities cluster into weak, mid-strength and strong promoters (Figure 5). Although the C. sporogenes fdx promoter (Pcspo_fdx) is known to be a strong promoter for expression of heterologous genes in Clostridium owing to its central position in the ABE-fermentation pathway (Minton et al., 2016), it was ineffective in C. saccharoperbutylacetonicum. The strongest promoter identified was that of the C. acetobutylicum ptb gene (Pcac_ptb) which was nearly 5400-fold stronger than Pcspo_fdx. Since the phosphotransbutyrylase (ptb) in Clostridium is only strongly expressed during exponential (acidogenic) phase in C. acetobutylicum (Girbal et al., 2003) and Clostridium beijerinckii (Ravagnani et al., 2000), GusA activity with expression from Pcac_ptb and Pcspo_fdxpromoters in C. saccharoperbutylacetonicum was measured over 48 h (Figure 5). The activity of Pcac_ptb was high over the whole time-course, whilst gusA expression from the Pcspo_fdx promoter was only marginally higher than that of the empty vector control pMTL82251.
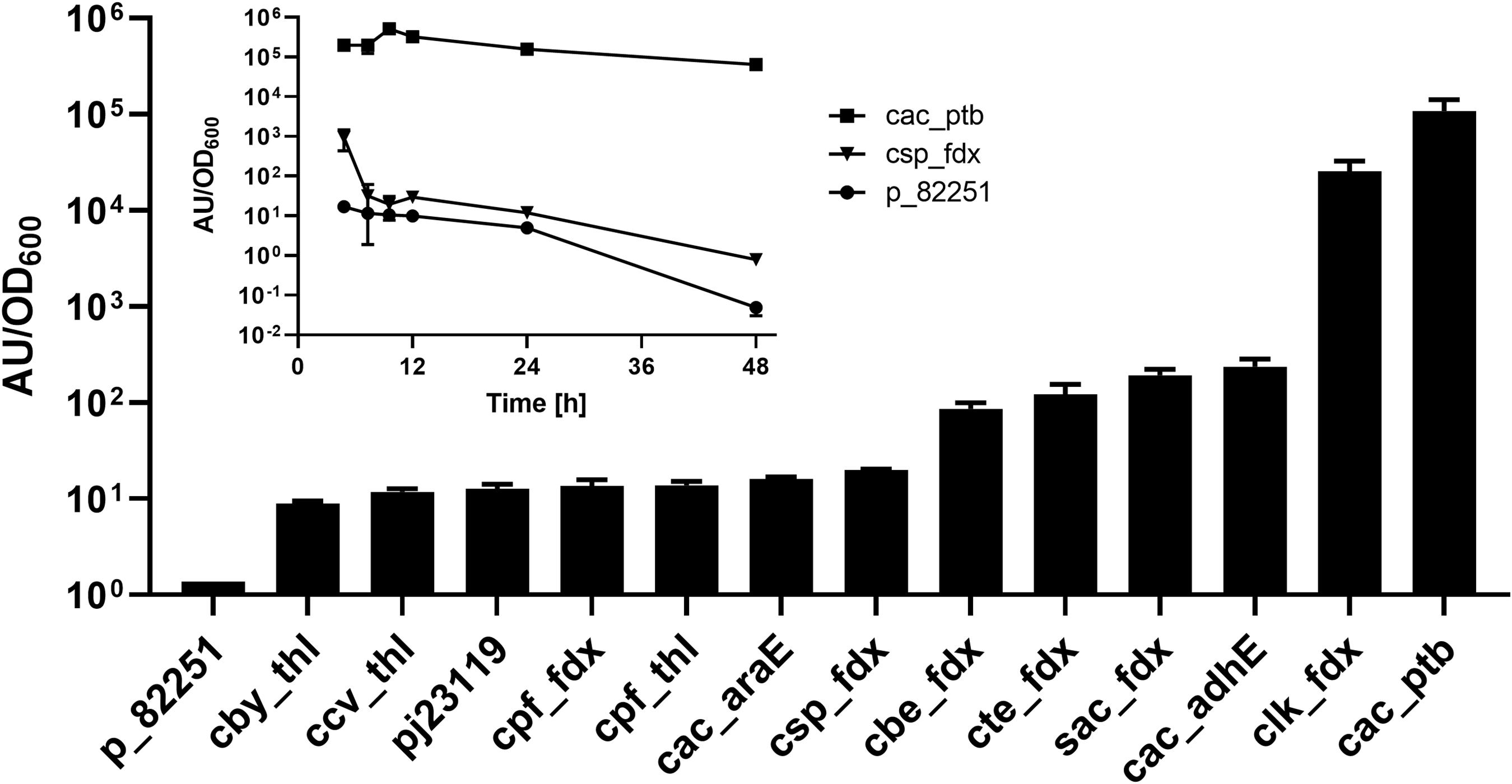
Figure 5. Promoter assay based on GusA production measured by accumulation of fluorescent product. A library of promoters derived from fdx and thl genes as well as a library of C. acetobutylicum promoters were tested for expression strength in C. saccharoperbutylacetonicum. Expression profiles over time of the strongest promoter Pcac_ptb and the standard promoter Pcspo_fdx were further assayed (inset graph). Error-bars represent SEM with n = 6 for all samples in the bar chart except p_82251 and Pcspo_fdx (n = 3) and n = 3 for all samples in the inset diagram.
phaB Chromosomally Integrated at the pyrE Locus
The C. necator phaB gene was integrated into the C. saccharoperbutylacetonicum chromosome downstream of the pyrE gene using the ACE method (Heap et al., 2012). Selection for reversion to uracil prototrophy by restoration of the pyrE gene was used as a marker for successful cargo integration. A 3-fold higher (R)-1,3-BDO concentration of 19 ± 0.7 mM was produced after 48 h when phaB was expressed from the chromosome (Ωcspo_fdx-phaB) (Figure 6), than when expressed on a plasmid (p_phaB). A two-fold increase in product titre was obtained compared to the best performing plasmid-based codon optimised mutant (p_phaB-opt_LS).
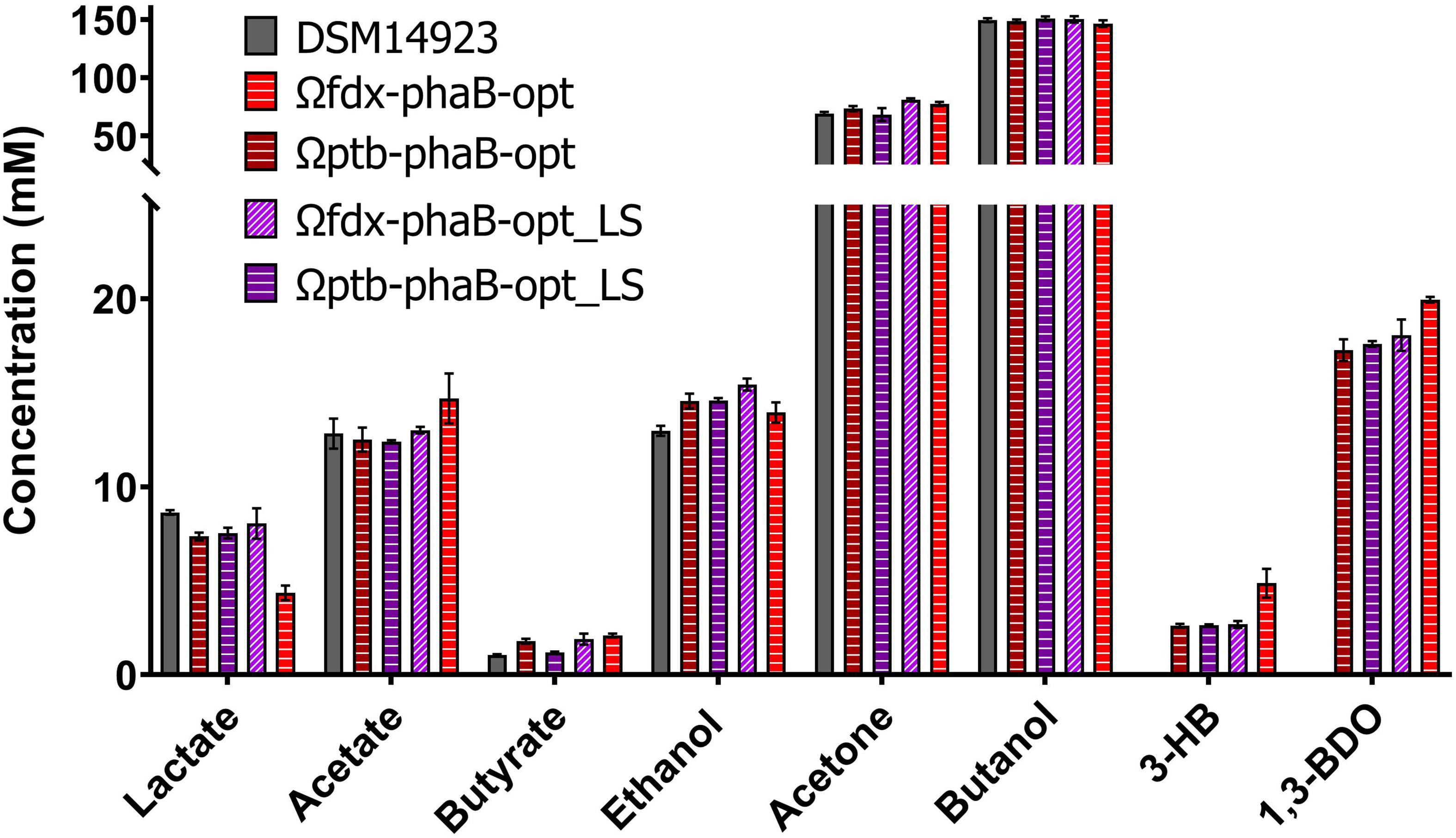
Figure 6. Comparison of product spectrum of the codon optimised phaB version and its derivatives encoding PhaB point mutations expressed in C. saccharoperbutylacetonicum by either weak promoter Pcspo_fdx or strong promoter Pcac_ptb from the chromosomal pyrE locus after 48 h. From left to right for each product DSM14923 in black, Ωfdx-phaB-opt in red horizontal lines, Ωptb-phaB-opt in burgundy horizontal lines, Ωfdx-phaB-opt_LS in light purple diagonal lines and Ωptb-phaB-opt_LS in purple horizontal lines. Error-bars represent SEM (n = 3).
Production of (R)-1,3-BDO by chromosomal expression of codon optimised phaB (phaB-opt) and the codon optimised double mutant (phaB-opt_LS) were measured from the differing strength Pcac_ptb and Pcspo_fdx promoters. All the codon optimised genes produced increased (R)-1,3-BDO concentrations compared to the p_phaB sequence. The weak Pcspo_fdx promoter (Ωfdx-phaB-opt: 20 ± 0.1 mM; Ωfdx-phaB-opt_LS: 18 ± 1.6 mM) performed marginally better than the strong Pcac_ptb promoter (Ωptb-phaB-opt 17.3 ± 1.1 mM; Ωptb-phaB-opt_LS 17.6 ± 0.3 mM) (Figure 6).
Discussion
Different growth conditions were tested for maximal solvent production in C. saccharoperbutylacetonicum and a stable plasmid selected for expression of heterologous phaB. Having shown that expression of phaB leads to (R)-1,3-BDO production in C. saccharoperbutylacetonicum, options to increase the production titre were analysed. Three possibilities on different levels of protein expression were considered. Firstly, at an enzyme level, the catalytic activity of PhaB can be increased through point mutations in sites which were identified previously (Matsumoto et al., 2013). Secondly, translational efficiency can be increased by codon optimisation of the gene to match it to the natural codon adaptation of C. saccharoperbutylacetonicum (Quax et al., 2015). And lastly, increasing mRNA levels through boosting of transcription efficiency can be achieved through the use of strong promoters (Deuschle et al., 1986).
Point Mutations in phaB Result in a Small Increase of (R)-1,3-BDO Production
Matsumoto et al. (2013) engineered and tested a library of approximately 20,000 phaB mutants generated by error prone PCR of which two variant genes were isolated that encoded an enzyme that exhibited increased kcat values compared to the wild type enzyme. In one mutant a glutamine at position 47 was changed to leucine (Q47L) and in the other mutant a threonine at position 173 was changed to serine (T173S). Both mutated enzymes showed increased activity and poly-3-hydroxybutyrate accumulation when heterologously expressed in Corynebacterium glutamicum (Matsumoto et al., 2013) and in tobacco (Yokoo et al., 2015). It was hypothesised that introduction of these point mutations in phaB and expression in C. saccharoperbutylacetonicum should analogously lead to higher metabolic flux toward (R)-1,3-BDO. Both point mutations were introduced separately and a double mutant carrying both amino acid changes was created. Both single mutants exhibited the desired effect of increased (R)-1,3-BDO, however, the double mutant did not show an accumulative titre increase (Figure 3).
PhaB Q47L mutant (p_phaB_L) produced the highest amount of (R)-1,3-BDO, 1.6-fold higher than the PhaB wild type, while the T173S mutant (p_phaB_S) only showed a 1.2-fold increase of product titre. The double mutant produced the same amount as p_phaB_L which suggested either product inhibition at this level or the fact that the mutations cannot function in conjunction. Product inhibition can be ruled out by a growth assay in increasing meso-1,3-BDO concentrations which found C. saccharoperbutylacetonicum to grow uninhibited up to 300 mM of meso-1,3-BDO (Supplementary Figure 4). On the other hand, the fact that the effects of the two mutations would not accumulate could be explained based on data collected by Matsumoto et al. (2013). They found that PhaB T173S increases kcat/Km(Acetoacetate–CoA) and decreases kcat/Km(NADPH) while PhaB Q47L enhances kcat/Km(NADPH) and decreases kcat/Km(Acetoacetate–CoA). Therefore, the positive effects on catalytic enzyme activity of each mutation could cancel the effect of the other.
It was interesting to note that both p_phaB and p_phaB_S had a detrimental effect on C. saccharoperbutylacetonicum growth, evident by a slower growth rate and longer fermentation time (Supplementary Figure 2). Both strains also used less glucose than the control. This effect was reversed in p_phaB_L and p_phaB_LS. Suggesting that the Q47L mutation offsets the deleterious effect of phaB expression while increasing product formation. The deleterious effects of phaB on C. saccharoperbutylacetonicum growth were not observed in any of the codon optimised versions of the gene analysed (Supplementary Figures 3, 6). Since the only difference between the native phaB and phaB_L are two nucleotides (138-CAG and 130-CTG, respectively) and the same two nucleotides were altered to 138-CAA in phaB-opt (Supplementary Figure 5) it can be assumed that the deleterious effect stems from translational complications in this region. Correddu et al. (2019) found that co-occurrence of certain amino acid codons in E. coli led to a premature stop in the protein chain potentially through a frameshift. Similar effects could be at play here. The codon adaptation frequency pattern found in the vicinity of the mutation, however, does not suggest codon rarity to be a factor (Supplementary Figure 5), since the point mutated version uses an uncommon (in C. saccharoperbutylacetonicum) CTG codon for lysine (Nakamura et al., 2000). Thus, the ultimate cause of the observed deleterious effect cannot be ascertained.
Codon Optimisation of phaB Has Minimal Impact on Product Concentration
Although codon optimisation was expected to lead to higher product yield, this was not found to be the case here (Figure 4). This was not entirely unexpected as codon optimisation based on the codon adaption index of an organism is relatively random with no significant correlation between the use of frequent codons and protein expression (Kudla et al., 2009). Future work should include codon harmonisation, where the relative native codon frequency per codon location found in C. necator is synchronised in the heterologous host organism. This approach takes into consideration mRNA regions presenting codons with low relative frequency (or codon adaptation index, CAI) which are hypothesised to slow down translation and allowing correct folding of the protein (Quax et al., 2015). Furthermore, it was shown that the 5’ mRNA leading sequence has a big influence on protein expression and surprisingly it was found that these sequences are preferably made up of rare codons, allowing appropriate ribosome distribution on the coding mRNA (Kudla et al., 2009; Quax et al., 2015).
Chromosomal Integration Significantly Increases (R)-1,3-BDO Formation
Concomitant with testing the influence of codon optimisation on product titres the consequence of locating the heterologous phaB to the host chromosome as opposed to an autonomous plasmid was evaluated. Stable chromosomal integration is key to biotechnological exploitation of this technology by abolishing the need for antibiotics in fermentation and overcoming the inherent instability of replicative plasmids (Friehs, 2004; Heap et al., 2012). Expression was expected to be lower when the gene was expressed from the chromosome since there are more copies of the plasmid present than of the chromosome. This higher copy number would hypothetically translate into more mRNA and subsequently more protein, which in turn should be reflected in increased product titres. However, 3-fold higher (R)-1,3-BDO production was found in the mutant expressing phaB from the chromosome (Ωcspo_fdx-phaB) compared with it being expressed from a plasmid (p_phaB) and double the product titres compared to the best performing plasmid-based codon optimised mutant (p_phaB-opt_LS) were found (Figure 7). This effect was also previously reported by our team member (Green et al., 2017).
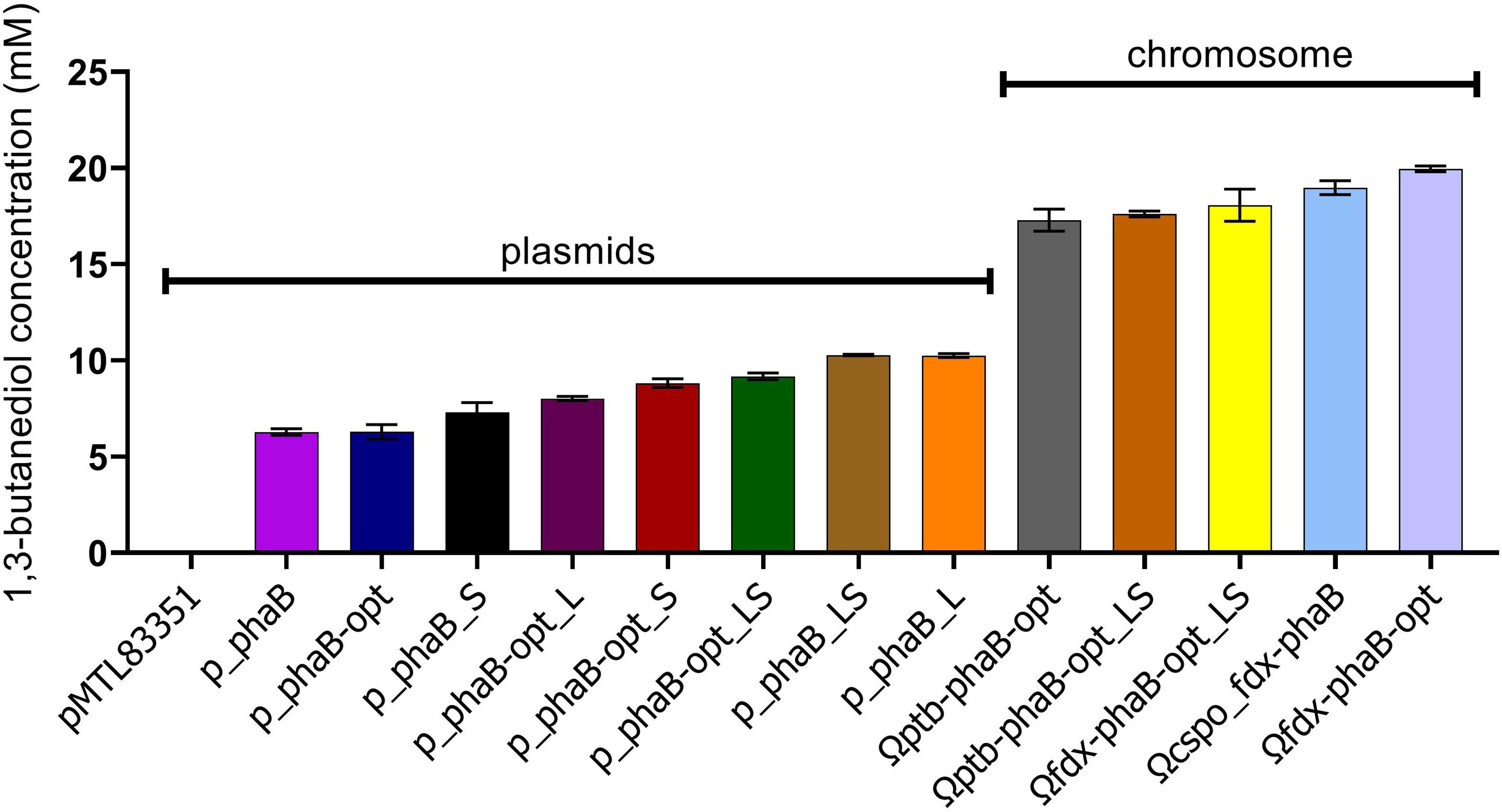
Figure 7. 1,3-butanediol production from all the different constructs tested in this study. Error-bars represent SEM (n = 3).
This finding suggests that overexpression of phaB has a negative impact on the final product titres. This may be due to pathway overloading, protein aggregation or polymerisation, liquid phase separation or general resource exhaustion of the cell (Bolognesi and Lehner, 2018). If any of these overexpression related issues play a role, then further overexpression would be pointless. To test this hypothesis phaB and the double mutant (phaB_LS) and codon optimised versions were expressed from the chromosome under the strong Pcac_ptb promoter and tested against expression from the weak Pcspo_fdx promoter (Ωfdx-phaB-opt). Product titres for (R)-1,3-BDO were lower when phaB-opt was strongly overexpressed with Pcac_ptb (Ωptb-phaB-opt) or when the potentially more active double mutant was used (Ωfdx-phaB-opt_LS and Ωptb-phaB-opt_LS). However, one-way ANOVA revealed that the reduction was not significant [F(3,7) = 0.71, p = 0.57].
These findings suggest that the earlier hypothesis was correct and more active or higher expressed phaB does not lead to increased product titres. Since not only overexpression but also increasing enzyme activity by introduction of point mutations led to decreased product titre (Ωfdx-phaB-opt compared to Ωfdx-phaB-opt_LS), protein burden on the cell (Eguchi et al., 2018), protein underwrapping (Birchler and Veitia, 2012) or protein aggregation (Rosano and Ceccarelli, 2014) as a cause can potentially be excluded. Instead, evidence points toward reactant or product inhibition of the reaction. It is not clear through what mechanism this inhibition arises. However, the unfamiliar R stereo form of the substrates (R)-3-hydroxybutyryl-CoA and (R)-3-hydroxybutyraldehyde may not be reduced efficiently by native enzymes butyraldehyde dehydrogenase and butanol dehydrogenase, leading to intracellular build up that may cause feedback inhibition. Moreover, similar effects have been reported before and were attributed to metabolic imbalances caused by highly overexpressed genes and high enzyme activity of heterologously expressed genes (Atsumi et al., 2008; Kataoka et al., 2013).
Final Remarks
It was shown that (R)-1,3-BDO can be produced up to 20 mM (1.8 g/l) in a non-optimised batch fermentation with C. saccharoperbutylacetonicum. This is the first time (R)-1,3-BDO production from glucose has been shown outside of E. coli in an anaerobic non-optimised batch fermentation. Titres reached are similar to studies using plasmid borne expression of pyruvate decarboxylase from Zymomonas mobilis and deoxyribose-5-phosphate aldolase from Bacillus halodurans (Nemr et al., 2018; Kim et al., 2020) but lower than in studies using plasmid borne expression of PhaA and PhaB from R. eutropha coupled with expression of Bld from C. saccharoperbutylacetonicum (Kataoka et al., 2013, 2014) summarised in Table 1. Higher titres can be reached using 4-hydroxy-2-butanone as substrate (Itoh et al., 2007; Zheng et al., 2012; Yang et al., 2014), however glucose is more widely available and more ecenomical than 4-hydroxy-2-butanone. Finally, future work should aim at optimising the fermentation and at upscaling of the production which is easier with an anaerobic organism such as the one used in this work.
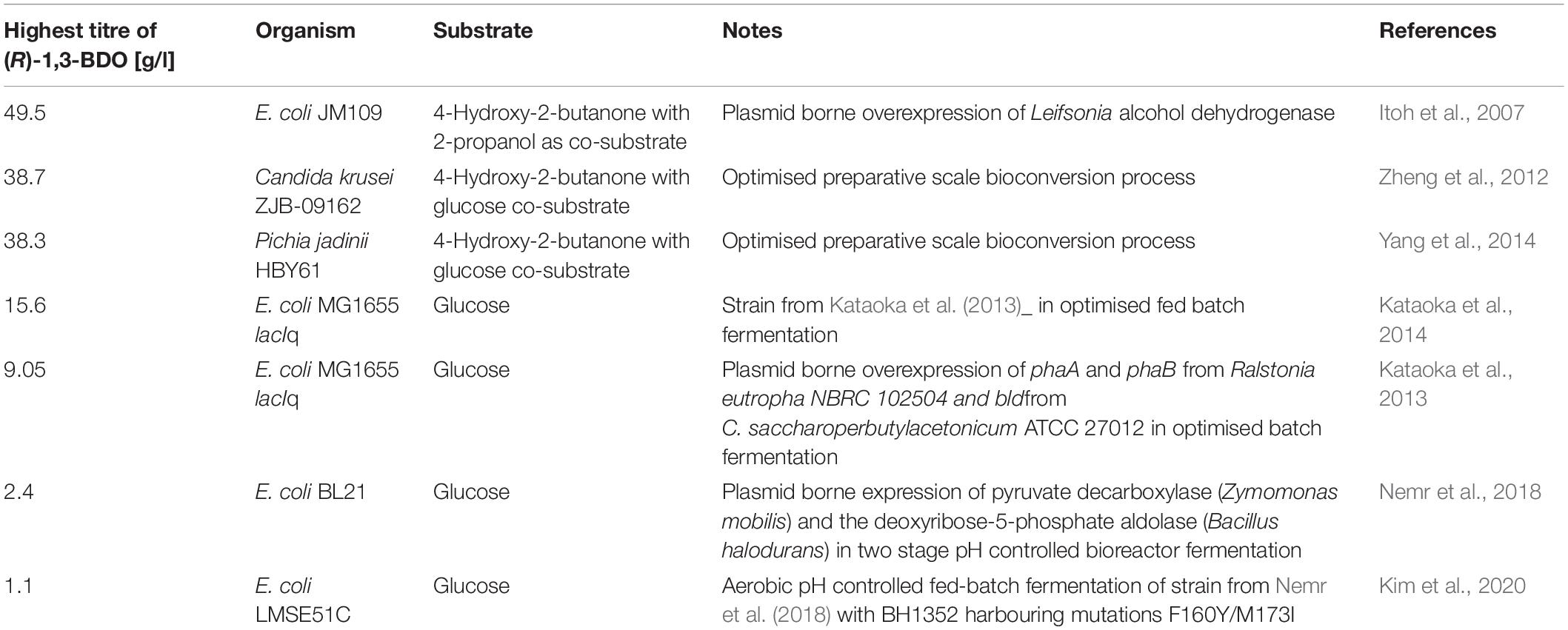
Table 1. Summary of literature to date presenting microbial production of (R)-1,3-BDO from different substrates.
Here, three non-exclusive approaches to increase production of (R)-1,3-BDO were tested and combined. Firstly, point mutations were applied to increase enzyme activity. Secondly, the gene was codon optimised to fit the C. saccharoperbutylacetonicum codon adaptation index. Lastly, the point-mutated and codon-optimised versions of phaB were expressed from the chromosome with differing strength promoters. However, it has been shown that increasing transcription and protein activity did not lead to increased product titres. On the contrary these alterations led to decreased product formation by unknown mechanisms. Future work should aim at investigating these imbalances, debottlenecking the downstream pathway (e.g., by overexpression of endogenous genes of the pathway) and optimising the fermentation to increase product titres further.
Data Availability Statement
The original contributions presented in the study are included in the article/Supplementary Material, further inquiries can be directed to the corresponding author.
Author Contributions
AG-H carried out the laboratory work, data analysis, and drafted the manuscript. ZB and DH provided materials and participated in the laboratory work. EG and NM helped design the study and edited the manuscript. GL and RC coordinated the final part of the study and drafted the manuscript. The manuscript was written through contributions of all authors. YZ conceived the study, oversaw its design and coordination, helped with the data analysis, and revised the manuscript. All authors read and approved the final manuscript.
Funding
This work was supported by the Biotechnology and Biological Sciences Research Council (BBSRC; Grant numbers BB/L013940/1, BB/N022718/1, and BB/S011951/1) and the Engineering and Physical Sciences Research Council (EPSRC; Grant number BB/L013940/1). YZ acknowledges the financial support of BBSRC NIBB LBNet: Lignocellulosic Biorefinery Network.
Conflict of Interest
EG, DH, and ZB work for CHAIN Biotechnology Ltd. which has interest in commercialisation of the present microbial platform. CHAIN Biotechnology Ltd. has filed a patent application encompassing the initial work described in this article, International Application No. PCT/GB2017/050601.
The remaining authors declare that the research was conducted in the absence of any commercial or financial relationships that could be construed as a potential conflict of interest.
Acknowledgments
The authors thank Ben Willson and Katalin Kovacs for the provision of their Clostridium promoter libraries and Jessica Locker for plasmid pMTL-JL1. Matthew Abbott and James Fothergill for their help with the analytics.
Supplementary Material
The Supplementary Material for this article can be found online at: https://www.frontiersin.org/articles/10.3389/fbioe.2021.659895/full#supplementary-material
References
Atsumi, S., Cann, A. F., Connor, M. R., Shen, C. R., Smith, K. M., Brynildsen, M. P., et al. (2008). Metabolic Engineering of Escherichia coli for 1-Butanol Production. Metab. Eng. 10, 305–311. doi: 10.1016/j.ymben.2007.08.003
Baer, S. H., Blaschek, H. P., and Smith, T. L. (1987). Effect of Butanol Challenge and Temperature on Lipid Composition and Membrane Fluidity of Butanol-Tolerant Clostridium acetobutylicum. Appl. Environ. Microbiol. 53, 2854–2861.
Biebl, H. (2001). Fermentation of Glycerol by Clostridium pasteurianum - Batch and Continuous Culture Studies. J. Indust. Microbiol. Biotechnol. 27, 18–26.
Birchler, J. A., and Veitia, R. A. (2012). Gene Balance Hypothesis: connecting Issues of Dosage Sensitivity across Biological Disciplines. Proc. Nat. Acad. Sci. U. S. A. 109, 14746–14753. doi: 10.1073/pnas.1207726109
Boaz, N. W., Ponasik, J. A., and Large, S. E. (2006). Ruthenium Complexes of Phosphine-Aminophosphine Ligands. Tetrahedr. Lett. 47, 4033–4035. doi: 10.1016/j.tetlet.2006.04.009
Bolognesi, B., and Lehner, B. (2018). Protein Overexpression: reaching the Limit. ELife 7:e39804. doi: 10.7554/eLife.39804
Correddu, D., López, J.d.J.M, Angermayr, S. A., Middleditch, M. J., Payne, L. S., and Leung, I. K. H. (2019). Effect of Consecutive Rare Codons on the Recombinant Production of Human Proteins in Escherichia coli. IUBMB Life 72, 266–274. doi: 10.1002/iub.2162
Deuschle, U., Kammerer, W., Gentz, R., and Bujard, H. (1986). Promoters of Escherichia coli: a Hierarchy of in Vivo Strength Indicates Alternate Structures. EMBO J. 5, 2987–2994. doi: 10.1002/j.1460-2075.1986.tb04596.x
Friehs, K. (2004). “Plasmid Copy Number and Plasmid Stability,” in New Trends and Developments in Biochemical Engineering. Advances in Biochemical Engineering, ed. T. Scheper (United States Springer), doi: 10.1007/b12440
Girbal, L., Mortier-Barrière, I., Raynaud, F., Rouanet, C., Croux, C., and Soucaille, P. (2003). Development of a Sensitive Gene Expression Reporter System and an Inducible Promoter-Repressor System for Clostridium acetobutylicum. Appl. Environ. Microbiol 69, 4985–4988. doi: 10.1128/aem.69.8.4985-4988.2003
Green, E., Heldt, D., and Bradley, B. (2017). Method and microbes for the production of chiral compounds. U. S. Patent Appl. 505:18.
Guevara-Martínez, M., Perez-Zabaleta, M., Gustavsson, M., Quillaguamán, J., Larsson, G., and van Maris, A. J. A. (2019). The role of the acyl-CoA thioesterase “YciA” in the production of (R)-3-hydroxybutyrate by recombinant Escherichia coli. Appl. Microbiol. Biotechnol. 103, 3693–3704.
Hanahan, D. (1983). Studies on Transformation of Escherichia coli with Plasmids. J. Mole. Biol. 166, 557–580. doi: 10.1016/S0022-2836(83)80284-8
Hartmanis, M. G., and Gatenbeck, S. (1984). Intermediary Metabolism in Clostridium acetobutylicum: levels of Enzymes Involved in the Formation of Acetate and Butyrate. Appl. Environmental Microbiol. 47, 1277–1283.
Heap, J. T., Ehsaan, M., Cooksley, C. M., Ng, Y. K., Cartman, S. T., Winzer, K., et al. (2012). Integration of DNA into Bacterial Chromosomes from Plasmids without a Counter-Selection Marker. Nucl. Acids Res. 40:e59. doi: 10.1093/nar/gkr1321
Heap, J. T., Pennington, O. J., Cartman, S. T., and Minton, N. P. (2009). A Modular System for Clostridium Shuttle Plasmids. J. Microbiol. Methods 78, 79–85. doi: 10.1016/j.mimet.2009.05.004
Herman, N. A., Li, J., Bedi, R., Turchi, B., Liu, X., Miller, M. J., et al. (2017). Development of a High-Efficiency Transformation Method and Implementation of Rational Metabolic Engineering for the Industrial Butanol Hyperproducer Clostridium saccharoperbutylacetonicum Strain N1-4. Appl. Environ. Microbiol. 83, e2942–e2916. doi: 10.1128/AEM.02942-16
Hongo, M., Murata, A., Kono, K., and Kato, F. (1968). Lysogeny and Bacteriocinogeny in Strains of Clostridium Species. Agric. Biol. Chem. 32, 27–33. doi: 10.1080/00021369.1968.10859021
Itoh, N., Nakamura, M., Inoue, K., and Makino, Y. (2007). Continuous Production of Chiral 1,3-Butanediol Using Immobilized Biocatalysts in a Packed Bed Reactor: promising Biocatalysis Method with an Asymmetric Hydrogen-Transfer Bioreduction. Appl. Microbiol. Biotechnol. 75, 1249–1256. doi: 10.1007/s00253-007-0957-1
Kataoka, N., Vangnai, A. S., Tajima, T., Nakashimada, Y., and Kato, J. (2013). Improvement of (R)-1,3-Butanediol Production by Engineered Escherichia coli. J. Biosci. Bioeng. 115, 475–480. doi: 10.1016/j.jbiosc.2012.11.025
Kataoka, N., Vangnai, A. S., Ueda, H., Tajima, T., Nakashimada, Y., and Kato, J. (2014). Enhancement of (R)-1,3-Butanediol Production by Engineered Escherichia coli Using a Bioreactor System with Strict Regulation of Overall Oxygen Transfer Coefficient and PH. Biosci. Biotechnol., Biochem. 78, 695–700. doi: 10.1080/09168451.2014.891933
Keis, S., Shaheen, R., and Jones, D. T. (2001). Emended Descriptions of Clostridium acetobutylicum and Clostridium beijerinckii, and Descriptions of Clostridium saccharoperbutylacetonicum Sp Nov and Clostridium saccharobutylicum Sp Nov. Int. J. Syst. Evol. Microbiol. 51, 2095–2103. doi: 10.1099/00207713-51-6-2095
Kim, T., Stogios, P. J., Khusnutdinova, A. N., Nemr, K., Skarina, T., Flick, R., et al. (2020). Rational engineering of 2-deoxyribose-5-phosphate aldolases for the biosynthesis of (R)-1, 3-butanediol. J. Biol. Chem. 295, 597–609. doi: 10.1074/jbc.ra119.011363
Kudla, G., Murray, A. W., Tollervey, D., and Plotkin, J. B. (2009). Coding-Sequence Determinants of Gene Expression in Escherichia coli. Science 324, 255–258. doi: 10.1126/science.1170160
Larchevêque, M., Mambu, L., and Petit, Y. (1991). Preparation of Enantiomerically Pure 1,3-Butanediol from Threonine. Syn. Commun. 21, 2295–2300. doi: 10.1080/00397919108021588
Little, G. T., Willson, B. J., Heap, J. T., Winzer, K., and Minton, N. P. (2018). The Butanol Producing Microbe Clostridium beijerinckii NCIMB 14988 Manipulated Using Forward and Reverse Genetic Tools. Biotechnol. J. 13:1700711. doi: 10.1002/biot.201700711
Llarrull, L. I., Testero, S. A., Fisher, J. F., and Mobashery, S. (2010). The Future of the β-Lactams. Curr. Opinion Microbiol. 13, 551–557. doi: 10.1016/j.mib.2010.09.008
Matsumoto, K., Tanaka, Y., Watanabe, T., Motohashi, R., Ikeda, K., Tobitani, K., et al. (2013). Directed Evolution and Structural Analysis of Nadph-Dependent Acetoacetyl Coenzyme A (Acetoacetyl-CoA) Reductase from Ralstonia eutropha Reveals Two Mutations Responsible for Enhanced Kinetics. Appl. Environ. Microbiol. 79, 6134–6139. doi: 10.1128/AEM.01768-13
Matsuyama, A., Yamamoto, H., Kawada, N., and Kobayashi, Y. (2001). Industrial Production of (R)-1,3-Butanediol by New Biocatalysts. J. Mol. Catal. B Enzym. 11, 513–521. doi: 10.1016/S1381-1177(00)00032-1
Minton, N. P., Ehsaan, M., Humphreys, C. M., Little, G. T., Baker, J., Henstra, A. M., et al. (2016). A Roadmap for Gene System Development in Clostridium. Anaerobe 41, 104–112. doi: 10.1016/j.anaerobe.2016.05.011
Nakamura, Y., Gojobori, T., and Ikemura, T. (2000). Codon Usage Tabulated from International DNA Sequence Databases: status for the Year 2000. Nucl. Acids Res 28, 292–292. doi: 10.1093/nar/28.1.292
Nakatsuka, T., Iwata, H., Tanaka, R., Imajo, S., and Ishiguro, M. (1991). A Facile Conversion of the Phenylthio Group to Acetoxy by Copper Reagents for a Practical Synthesis of 4-Acetoxyazetidin-2-One Derivatives from (R)-Butane-1,3-Diol. J. Chem. Soc. Chem. Commun. 9, 662–664. doi: 10.1039/C39910000662
Nakayama, S., Kosaka, T., Hirakawa, H., Matsuura, K., Yoshino, S., and Furukawa, K. (2008). Metabolic Engineering for Solvent Productivity by Downregulation of the Hydrogenase Gene Cluster HupCBA in Clostridium saccharoperbutylacetonicum Strain N1-4. Appl. Microbiol. Biotechnol. 78, 483–493. doi: 10.1007/s00253-007-1323-z
Nemr, K., Müller, J. E. N., Joo, J. C., Gawand, P., Choudhary, R., Mendonca, B., et al. (2018). Engineering a Short, Aldolase-Based Pathway for (R)-1,3-Butanediol Production in Escherichia coli. Metab. Eng. 48, 13–24. doi: 10.1016/j.ymben.2018.04.013
O’Brien, R. W., and Morris, J. G. (1971). Oxygen and Growth and Metabolism of Clostridium acetobutylicum. J. Gen. Microbiol. 68:3.
Pyne, M. E., Moo-Young, M., Chung, D. A., and Chou, C. P. (2013). Development of an Electrotransformation Protocol for Genetic Manipulation of Clostridium pasteurianum. Biotechnol. Biofuels 6:50. doi: 10.1186/1754-6834-6-50
Qin, W., Panunzio, M., and Biondi, S. (2014). β-Lactam Antibiotics Renaissance. Antibiotics 3, 193–215. doi: 10.3390/antibiotics3020193
Quax, T. E. F., Claassens, N. J., Söll, D., and van der Oost, J. (2015). Codon Bias as a Means to Fine-Tune Gene Expression. Mole. Cell 59, 149–161. doi: 10.1016/j.molcel.2015.05.035
Ravagnani, A., Jennert, K. C. B., Steiner, E., Grunberg, R., Jefferies, J. R., Wilkinson, S. R., et al. (2000). Spo0A Directly Controls the Switch from Acid to Solvent Production in Solvent-Forming Clostridia. Mole. Microbiol. 37, 1172–1185. doi: 10.1046/j.1365-2958.2000.02071.x
Rosano, G. L., and Ceccarelli, E. A. (2014). Recombinant Protein Expression in Escherichia coli: advances and Challenges. Front. Microbiol. 5:172. doi: 10.3389/fmicb.2014.00172
Schwarz, K. M., Grosse-Honebrink, A., Derecka, K., Rotta, C., Zhang, Y., and Minton, N. P. (2017). Towards Improved Butanol Production through Targeted Genetic Modification of Clostridium pasteurianum. Metabol. Eng 40, 124–137. doi: 10.1016/j.ymben.2017.01.009
Shaheen, R., Shirley, M., and Jones, D. T. (2000). Comparative Fermentation Studies of Industrial Strains Belonging to Four Species of Solvent-Producing Clostridia. Journal of Mole. Microbiol. Biotechnol. 2, 115–124.
Struys, E. A., Jansen, E. E. W., Verhoeven, N. M., and Jakobs, C. (2004). “Measurement of urinary D- and L-2-hydroxyglutarate enantiomers by stable-isotope-dilution liquid chromatography-tandem mass spectrometry after derivatization with diacetyl-L-tartaric anhydride. Clin. Chem. 50, 1391–1395.
Tashiro, Y., Takeda, K., Kobayashi, G., Sonomoto, K., Ishizaki, A., and Yoshino, S. (2004). High Butanol Production by Clostridium saccharoperbutylacetonicum N1-4 in Fed-Batch Culture with PH-Stat Continuous Butyric Acid and Glucose Feeding Method. J. Biosci. Bioeng. 98, 263–268. doi: 10.1016/S1389-1723(04)00279-8
Wallace, B. D., Roberts, A. B., Pollet, R. M., Ingle, J. D., Biernat, K. A., Pellock, S. J., et al. (2015). Structure and Inhibition of Microbiome β-Glucuronidases Essential to the Alleviation of Cancer Drug Toxicity. Chem. Biol. 22, 1238–1249. doi: 10.1016/j.chembiol.2015.08.005
Wiesenborn, D. P., Rudolph, F. B., and Papoutsakis, E. T. (1988). Thiolase from Clostridium acetobutylicum ATCC 824 and Its Role in the Synthesis of Acids and Solvents. Appl. Environ. Microbiol. 54, 2717–2722.
Willson, B. J. (2014). ). Genetic Modification of Clostridium acetobutylicum for Deconstruction of Lignocellulose. United Kingdom: University of Nottingham. [PhD thesis].
Yamamoto, H., Matsuyama, A., and Kobayashi, Y. (2002). Synthesis of (R)-1,3-Butanediol by Enantioselective Oxidation Using Whole Recombinant Escherichia coli Cells Expressing (S)-Specific Secondary Alcohol Dehydrogenase. Bioscience, Biotechnol. Biochem. 66, 925–927. doi: 10.1271/bbb.66.925
Yang, T., Man, Z., Rao, Z., Xu, M., Zhang, X., and Xu, Z. (2014). Asymmetric Reduction of 4-Hydroxy-2-Butanone to (R)-1,3-Butanediol with Absolute Stereochemical Selectivity by a Newly Isolated Strain of Pichia jadinii. J. Indust. Microbiol. Biotechnol. 41, 1743–1752. doi: 10.1007/s10295-014-1521-5
Yao, D., Dong, S., Wang, P., Chen, T., Wang, J., Yue, Z., et al. (2017). Robustness of Clostridium saccharoperbutylacetonicum for Acetone-Butanol-Ethanol Production: effects of Lignocellulosic Sugars and Inhibitors. Fuel 208, 549–557. doi: 10.1016/J.FUEL.2017.07.004
Yokoo, T., Matsumoto, K., Ooba, T., Morimoto, K., and Taguchi, S. (2015). Enhanced Poly(3-Hydroxybutyrate) Production in Transgenic Tobacco BY-2 Cells Using Engineered Acetoacetyl-CoA Reductase. Biosci., Biotechnol. Biochem 79, 986–988. doi: 10.1080/09168451.2014.1002448
Yoshida, T., Tashiro, Y., and Sonomoto, K. (2012). Novel High Butanol Production from Lactic Acid and Pentose by Clostridium saccharoperbutylacetonicum. J. Biosci. Bioeng. 114, 526–530. doi: 10.1016/J.JBIOSC.2012.06.001
Eguchi, Y., Makanae, K., Hasunuma, T., Ishibashi, Y., Kito, K., and Moriya, H. (2018). Estimating the Protein Burden Limit of Yeast Cells by Measuring the Expression Limits of Glycolytic Proteins. ELife 7:e34595. doi: 10.7554/eLife.34595
Zhang, J., Wang, P., Wang, X., Feng, J., Sandhu, H. S., and Wang, Y. (2018). Enhancement of Sucrose Metabolism in Clostridium saccharoperbutylacetonicum N1-4 through Metabolic Engineering for Improved Acetone–Butanol–Ethanol (ABE) Fermentation. Bioresour. Technol. 270, 430–438. doi: 10.1016/J.BIORTECH.2018.09.059
Keywords: Clostridium saccharoperbutylacetonicum, biotechnology, phaB, Allele Coupled Exchange, (R)-1, 3-butanediol, Clostridium
Citation: Grosse-Honebrink A, Little GT, Bean Z, Heldt D, Cornock RHM, Winzer K, Minton NP, Green E and Zhang Y (2021) Development of Clostridium saccharoperbutylacetonicum as a Whole Cell Biocatalyst for Production of Chirally Pure (R)-1,3-Butanediol. Front. Bioeng. Biotechnol. 9:659895. doi: 10.3389/fbioe.2021.659895
Received: 28 January 2021; Accepted: 12 April 2021;
Published: 13 May 2021.
Edited by:
Petra Patakova, University of Chemistry and Technology in Prague, CzechiaReviewed by:
Yi Wang, Auburn University, United StatesAdam William Westbrook, Genecis Bioindustries Inc., Canada
Copyright © 2021 Grosse-Honebrink, Little, Bean, Heldt, Cornock, Winzer, Minton, Green and Zhang. This is an open-access article distributed under the terms of the Creative Commons Attribution License (CC BY). The use, distribution or reproduction in other forums is permitted, provided the original author(s) and the copyright owner(s) are credited and that the original publication in this journal is cited, in accordance with accepted academic practice. No use, distribution or reproduction is permitted which does not comply with these terms.
*Correspondence: Ying Zhang, Ying.Zhang@nottingham.ac.uk