- 1Department of Cell and Systems Biology, University of Toronto, Toronto, ON, Canada
- 2Theranostics and Drug Discovery Research Group, Faculty of Pharmaceutical Sciences, University of Nigeria, Nsukka, Nigeria
- 3Department of Pharmaceutics, Faculty of Pharmaceutical Sciences, University of Nigeria, Nsukka, Nigeria
- 4Department of Genetics, University of Karachi, Karachi, Pakistan
Plant virus nanoparticles (VNPs) are inexpensive to produce, safe, biodegradable and efficacious as treatments. The applications of r plant virus nanoparticles range from epitope carriers for vaccines to agents in cancer immunotherapy. Both VNPs and virus-like particles (VLPs) are highly immunogenic and are readily phagocytosed by antigen presenting cells (APCs), which in turn elicit antigen processing and display of pathogenic epitopes on their surfaces. Since the VLPs are composed of multiple copies of their respective capsid proteins, they present repetitive multivalent scaffolds which aid in antigen presentation. Therefore, the VLPs prove to be highly suitable platforms for delivery and presentation of antigenic epitopes, resulting in induction of more robust immune response compared to those of their soluble counterparts. Since the tumor microenvironment poses the challenge of self-antigen tolerance, VLPs are preferrable platforms for delivery and display of self-antigens as well as otherwise weakly immunogenic antigens. These properties, in addition to their diminutive size, enable the VLPs to deliver vaccines to the draining lymph nodes in addition to promoting APC interactions. Furthermore, many plant viral VLPs possess inherent adjuvant properties dispensing with the requirement of additional adjuvants to stimulate immune activity. Some of the highly immunogenic VLPs elicit innate immune activity, which in turn instigate adaptive immunity in tumor micro-environments. Plant viral VLPs are nontoxic, inherently stable, and capable of being mass-produced as well as being modified with antigens and drugs, therefore providing an attractive option for eliciting anti-tumor immunity. The following review explores the use of plant viruses as epitope carrying nanoparticles and as a novel tools in cancer immunotherapy.
Introduction
Nanomedicine is an emerging area of multidisciplinary research that has already shown promise of transforming into a disruptive innovative development (Farokhzad et al., 2008). Already, there are dozens of products in clinical trials and even some on the shelf in some pharmacies across the world though users are relatively few because of the rather prohibitive price tags of these innovative products (Park, 2019). It is pertinent to state that while a lot has been proposed in terms of the anticipated efficacy of nanomedicines, opinions tend to vary when it comes to the stage of a critical cost-benefit- analysis for the availability of nanomedicines for use in the treatment of cancer and other ailments (FDA Drug Reports, 2017).
Nanomedicines includes a wide array of nanomaterials with particle size ranging from 1nm to more than 400 nm and are a remarkably diverse group of materials (Zhang et al., 2008; Zhou et al., 2012). They may be made up of entirely of a metal as in the case of Gold and Silver nanoparticles (Paviolo and Stoddart, 2017), or a combination of liquids or a ternary system composed of an assortment of several compatible materials giving rise in most cases to a multifunctional entity often possessing stimuli responsive attributes enabling it to respond to minute changes in factors such as pH and temperature variations (Moreira et al., 2016). Additionally, nanoparticles can be prepared with simple polymeric materials such as cellulose and chitosan (Steinmetz and Manchester, 2009).
Immunotherapy in cancer treatment simply refers to a strategy with the objective of galvanizing the immune system of the patient to resist the implanting of cancerous cells. There are several approaches to achieve the desired end. One approach involves the use of drugs known as “Immune checkpoint inhibitors, to block immune checkpoints (Byun et al., 2017). The checkpoints are a typical part of the immune system and serve to modulate the immune response so that it does not come as too strong. The net effect of this treatment modality is that the blocking of these checkpoints makes it possible for the immune cells to respond more strongly to cancer.
Plant virus-based nanoparticles (VNPs) have been explored as a unique class of nanocarriers for biomedical applications (Pitek et al., 2016). In addition to their ease of production and quality control maintenance, plant virus VNPs offer a logical alternative to synthetic nanoparticles as they are inexpensive to produce, nontoxic and biodegradable (Rybickie, 2020). Plant virus nanoparticles have been further improved for their performance in terms of stimuli-responsivity (Brun, Gomez, and Suh 2017).
Plant virus nanoparticles tend to be either rod shaped, such as Tobacco mosaic virus (TMV) and Potato virus X (PVX), or icosahedral shaped, such as Cowpea mosaic virus (CPMV). Different shaped viruses respond differently as nanoparticles in vivo. Tobacco mosaic virus can assemble into VLPs without requiring its RNA genome carry a drug payload on the surface or to a limited extent, within the inner channel of the nanoparticle. Potato virus X, cannot self-assemble in the absence of its RNA genome, and thus can only carry a payload on the outer surface. Cowpea mosaic virus can be made to self-assemble into empty virus like particles in the absence of its RNA genome and can thus carry a payload both inside and outside of its protein shell (Sainsbury et al., 2010). In this review, we provide a series of examples to discuss how plant virus architecture contributes to their applications in cancer diagnostics and therapy (Wen et al., 2015a; Wen et al., 2015b). We discuss the architecture of plant viruses, how they came to be used as nanoparticles in various medical applications, and how they may be employed in the future as novel cancer immunotherapies (Shahgolzari et al., 2021).
Architecture of Plant Virus Nanoparticles
Viruses are composed of outer protein shells which encapsulate the genomic material. The multiple copies of coat proteins that form the virus outer shell of viruses are collectively known as the capsid (Liu et al., 2016). Primarily, the capsid occurs in different shapes and sizes and is meant to protect the genomic material to keep viruses safe under extreme environments (Pokorski and Steinmetz 2011). The immense diversity with respect to the shape and size of plant viruses enables them to be tailored for specific applications. The structural integrity of viruses remains intact even when surface properties have been altered through chemical and genetic modification; this allows control over targeting ligands, drugs and contrast agents for imaging (Rong et al., 2011). Various chemical and genetic approaches are reported to control the virus surface properties without affecting structural integrity, and allow control on the attachment sites of drug molecules or contrast agents on the virus surface (Rong et al., 2011). Plant-virus capsid pores are also reported to be employed to encapsulate small therapeutic molecules (Zeng et al., 2013).
Plant viruses have been used as virus like particles (VLPs) and virus nanoparticles (VNPs) as epitope display systems for vaccine production. VLPs are a subset of the VNPs but lack any nucleic acid genome, thus making them non-infectious. VNPs and VLPs based on plant viruses are both non-pathogenic to humans and biodegradable (Steinmetz, 2010). VNPs and VLPs are advantageous due to their ability to be generated quickly while serving as highly versatile molecular scaffolds (Young et al., 2008; Steinmetz and Evans, 2007]. Examples of plant viruses utilized as VLPs include Cowpea mosaic virus (CPMV) and Tobacco mosaic virus (TMV). An example of a plant virus utilized as a VNP is Potato virus X (PVX). These are listed in Figure 1.
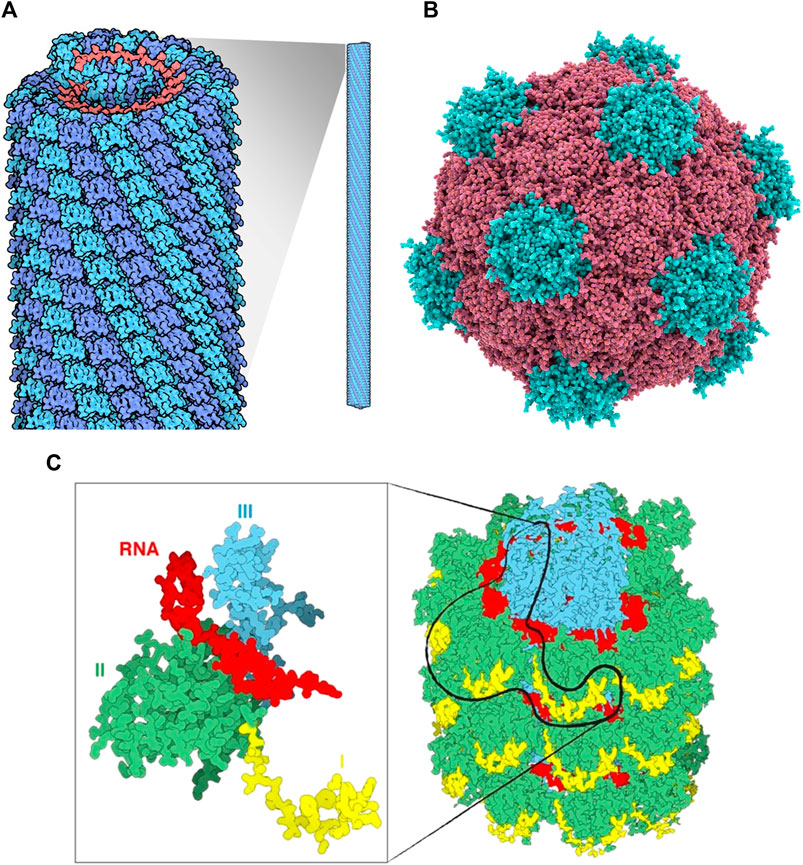
FIGURE 1. (A) Tobacco mosaic virus structure, RNA is in red, protein subunits in blue Source: https://pdb101.rcsb.org/motm/109. (B) Cowpea mosaic virus structure, Protein subunits in red and blue Source: fineartamerica. com. (C) An overview of a portion of the PVX virus (right). The three domains of the protein are shin in yellow, green and cyan, the RNA in red. The magnification on the left displays only one single CP with a fragment of RNA.
Tobacco mosaic virus (TMV) is the most well-studied plant virus and was initially characterized in the 19th century. TMV can be easily produced and purified in bulk amounts and can be manipulated genetically due to its relatively simple particle structure and genome organization. The rod-shaped virus particle measures 300 nm in length and 18 nm in diameter and contains a 6.7 kb viral RNA genome that is encapsulated by 2,130 identical copies of the capsid protein assembled in a helical arrangement. At neutral pH and in the absence of RNA, the CP assembles itself into an 18 nm double disk, a 20S aggregate or nano-ring containing two layers of 17 CP molecules which can serve as a nanoscale scaffold.
Potato virus X (PVX) is a member of the family Alphaflexiviridae, genus Potexvirus, an important plant pathogen of the family Solanaceae, and specifically infects potato, tomato and tobacco (Adams et al., 2004) (Massumi et al., 2014). It has a 6.4-kb positive-stranded RNA genome (Park et al., 2008). Multiple copies of CP assemble around the genomic RNA to form the capsid. PVX can carry large payloads due to its flexible and filamentous structure, making it possible to use for pharmaceutical and imaging applications (Roder, Dickmeis, and Commandeur 2019).
The PVX particle is 515 × 14.5 nm in dimension and comprised of 1,270 subunits of CP, (Parker et al., 2002). The C-terminus of each CP subunit is located internally and the N-terminus projected externally to the assembled particle, which provides a suitable site for modification (Nemykh et al., 2008). Unlike other reported viruses, the assembly of PVX CP subunits into filamentous VLP, in vivo or in vitro, is not possible in the absence of genomic RNA. This reflects the unique connection between virus RNA and CP (Kwon et al., 2005).
The plant pathogen Cowpea mosaic virus (CPMV) belongs to the Comovirus genus. CPMV is an icosahedral shaped virus with a diameter of approximately 27 nm. It is composed of RNA-1 and RNA-2 of 6 and 3.5 kb, respectively packed in 60 copies each of Large and Small coat protein (Singh et al., 2007). CPMV is one of the most developed VNPs for biomedical and nanotechnology applications due to its ability to target specific tissues and act as an efficient drug delivery system. It is also reported to be well-adapted for the attachment of a variety of molecules to the coat protein. Five reactive lysine residues of CPMV coat protein provide sites to chemically conjugate to various compounds such as fluorescent dyes (Steinmetz, 2010). CPMV can be produced as empty virus like particles (eVLPs) Meshcheriakova et al. (2017). eVLPs are non-infectious and could be loaded with heterologous material, has increased the number of possible applications for CPMV-based particles.
Biomedical Applications
VLPs can act as strong vaccine candidates as they simulate the conformations of native viruses, utilizing their intrinsic immunogenicity without compromising their safety. VLPs evoke effectual immune responses as they are readily internalized by antigen presenting cells (APCs) and are ideal platforms for antigen processing and epitope presentation to immune cells. VLPs are composed of multiple copies of their capsid (coat) proteins, which when assembled appear as repetitive, multivalent molecular scaffolds. As a result, the multiple copies of coat protein can facilitate the multivalent presentation of antigens fused to their surface. Therefore, VLP vaccines afford superior immunogenicity compared to antigens in their soluble states. Additionally, plant viral VLPs and VNPs possess inherent adjuvant properties dispensing with the use of additional adjuvants to evoke strong immune responses (Table 1).
TMV VLPs have been utilized as epitope display systems in a variety of settings, with a first example being a polio vaccine by Haynes et al. (1986). Later, TMV was used as an epitope display vehicle for a malaria vaccine and others, including foot and mouth disease virus, human papillomavirus, norovirus, hepatitis B virus, influenza virus and human immunodeficiency virus (Turpen et al., 1995; Nooraei et al., 2021). Röder et al. (2017), were able to fuse a fluorescent protein to the C-terminus of the Tobacco mosaic virus (TMV) coat protein (CP) and also carried an N-terminal Foot-and-mouth disease virus (FMDV) 2A sequence. This enables the fusion protein to be cleaved from TMV.
Potato virus X, in contrast to TMV, has a flexuous rod shape and requires its genomic RNA to self-assemble. PVX has been used extensively as an epitope display system for vaccine research. For example, Uhde-Holzem et al. (2016), reported genetically altered PVX which displayed Staphylococcus aureus protein A fragments on its surface, and proved to be easily functionalized with IgG to be used in biosensing plant viruses. VLPs of Papaya mosaic virus (PapMV), of the Potexvirius family, has been engineered for use as a seasonal flu trivalent vaccine (Carignan et al., 2015; Balke and Zeltins, 2020).
CPMV has also been developed as an autonomously replicating virus vector for the expression of either peptides or polypeptides in plants (Shahgolzari et al., 2020). Examples of CPMV used as an epitope presentation system include epitopes from the outer membrane (OM) protein F of Pseudomonas aeruginosa which were shown to protect mice against bacterial challenge, and an epitope expressing the 30 amino acid D2 domain of the fibronectin-binding protein (FnBP) from Staphylococcus aureus, which has been shown to be able to protect rats against endocarditis (Liu et al., 2005).
Recently, Albakri et al. (2020), explored how CPMV particles can activate human monocytes, dendritic cells (DCs) and macrophages. Monocytes, upon incubation with CPMV in vitro, released the chemokines CXCL10, MIP-1α and MIP-1β into cell culture supernatants. Dendritic cells and monocyte-derived macrophages were also activated after incubation with CPMV, this activation is part of SYK signaling. Shukla et al. (2020) were able to demonstrate that CPMV outperformed many other types of VLPs to be a particularly strong immune stimulant.
The capacity for multifunctionality and multivalency makes plant nanoparticle platforms an ideal choice for theranostic applications (Beatty and Lewis 2019; Wang, 2019). Plant nanoparticles are capable of precise molecular imaging to achieve accurate cancer diagnosis and therapy (Ma et al., 2017). Delivery of imaging probes through nanostructures can improve the chances of early-stage cancer diagnosis through the use of multiple modalities to improve resolution, sensitivity, penetration, time, cost and on the top of all clinical relevance compared to the single imaging modalities (Key and Leary, 2014; Shahgolzari et al., 2020). Drug conjugated nanoparticles administered intravenously target tumors, via the process of enhanced permeability and retention (EPR) effect depending on the type of tumor (Hansen et al., 2015).
Molecular imaging is an emerging biomedical field which facilitates the visualization, of biological mechanisms in vivo. Imaging technologies can include magnetic resonance imaging (MRI), computed tomography (CT), positron emission tomography (PET) and optical imaging, which enable the monitoring of molecular and cellular processes in normal and diseased conditions in living subjects. (Chung et al., 2020). Plant VLPs can be beneficial for molecular imaging technologies than synthetic nanoparticles, due to their short half-life in circulation and their lack of side effects (Steinmetz, 2010). Furthermore, plant VLPs can be developed to carry a wide array of contrast agents and fluorescent labels, as they can be modified with antibodies, peptides and aptamers to enable enhanced targeting to specific tissues and cells.
Magnetic resonance imaging (MRI) is a promising technology for the diagnosis of disease due to its high resolution and deep contrast, however, virus-based nanoparticles have been used to increase sensitivity (Pokorski et al., 2011). TMV can act as a carrier to deliver high payloads of MRI contrast imaging agents to diseased tissues (Michael A. Bruckman et al., 2013) and fluorescent dyes for biosensing and bioimaging (Wen et al., 2015). TMV’s biological compatibility and multi-valency enables it to be a suitable carrier of in vivo imaging agents. For example, TMV rods have been conjugated to “BF3,” a multi-photon absorbing fluorophore which permitted mouse brain imaging over an extended duration without crossing the blood-brain barrier (Niehl et al., 2016). A bimodal contrast agent has been prepared to target integrin α2β1by loading the internal cavity of TMV nanoparticles with the complex of dysprosium (Dy3+) and the near-infrared fluorescence (NIRF) dye Cy7.5, as well as the externally conjugated with an Asp-Gly-Glu-Ala (DGEA) peptide through a linker polyethylene glycol. This nanoparticle (Dy-Cy7.5-TMV-DGEA) was stable, displayed a low cytotoxicity and achieved a high resolution when targeted to PC-3 prostate cancer cells (Hu et al., 2017).
Tobacco Mosaic Virus has been used successfully for CD imaging, targeting atherosclerosis and thrombosis by using an NIR dye as well as a targeting peptide conjugated to TMV [96]. These targeted TMV particles were able to identify atherosclerotic lesions in ApoE−/− mice upon intravenous injection, showing that TMV can be used as a platform to detect at-risk lesions.
VNPs based on PVX have been conjugated to fluorescent reporters as well [135]. As mentioned earlier, the small fluorescent iLOV protein was expressed on the surface of PVX through genetic engineering and served as a fluorescent probe which could be of potential use in vivo imaging. Shukla et al. (2018), reportedly produced PVX VNPs that displayed mCherry or GFP on their N-termini in N. benthamiana plants. Significantly, fluorescent PVX could successfully be used for in vivo particle tracking in an HT-29 murine model, for in vitro imaging of HT-29 cells, and for tracing viral infection within plants.
CPMV can also be engineered for intravital imaging (imaging living cells while they are in a multicellular organism) and improved permeability with a retention effect that improves tumor penetration (Beatty and Lewis, 2019). For in vivo imaging of tumors, CPMV-based VNPs have been successfully engineered to target specific tissues (Cho et al., 2014). These tumor targeting VNPs also provide biocompatible platforms for cancer therapy and intravital imaging (Beatty and Lewis, 2019).
Clinical treatment for cancer has been routinely addressed by chemotherapy (Hu et al., 2017). Regardless, the high recurrence of cancers as well as the fast clearance of anti-cancer drugs and non-targeted drug delivery necessitate the administration of maximum tolerable doses of drugs in cancer therapy, leading to increased toxicity and lower performance (Cano-Garrido et al., 2021). Therefore, drug delivery technologies that are highly targeted and promote active drug accumulation in tumors, in concert with reductions of dose requirements, could alleviate these concerns and augment treatment outcomes.
Plant virus VLPs have several attractive features that make them appropriate for targeted administration of therapeutic molecules. The anti-cancer drug doxorubicin (DOX), has been successfully delivered using VNPs and VLPs. TMV- and PVX-derived VLPs and VNPs have been successfully used to deliver DOX (Finbloom et al., 2018). In this context, helical plant VNPs such as TMV and PVX, with high aspect ratios, have proven to be of great use in effective drug delivery. VNPs have shown great promise since their cargo-RNA functions as a ruler establishing the length of the virus particle and simple adsorption of DOX on their surface was shown to be effective for reducing tumor growth (Bruckman et al., 2013; Pitek et al., 2016).
TMV can be used as a carrier of peptides with therapeutic or targeting activity against various cancers. Trastuzumab is a cancer cell inhibiting monoclonal antibody that uses the binding sites of human epidermal growth factor receptor 2 (HER2). Trastuzumab-binding peptides (TBP) are immunogenic in nature and capable of initiating production of HER2-inhibiting antibodies to seize the growth of HER2-carrying cancer cells. TMV particles displaying TBP have been created to activate this immunogenicity (Tyulkina et al., 2011). Similarly, a delivery system reported as PhenPt-TMV, with anticancer drug phenanthriplatin loaded into a hollow TMV carrier, serves as an example of stimuli responsive system, as the release of drug is induced in the presence of acidic environment (Czapar et al., 2016). Along these lines, Tian et al. (2018) demonstrated that the Transacting Activation Transduction (TAT) peptide, conjugated to the external surface of TMV, augmented internalization along with an increased ability to escape endo/lysosomal compartments. Most of these VLPS exhibited uptake by dendritic cells and macrophages and proved to be highly immunogenic. Thus, therapeutic nucleic acids can be easily delivered to immune cells during cancer treatments.
Plant virus VNPs have been used for targeted administration of platinum-based drugs against cancer. This is important as 50% of chemotherapy treatments involve the use of these platinum-derived drugs. TMV has been demonstrated to efficiently deliver Cisplatin (Franke et al., 2018) and Phenanthriplatin (Vernekar et al., 2018), both of which are platinum-based drugs. The drugs were loaded into the TMV VNP cavity using charge-driven interactions or by synthesizing stable covalent adducts. Such a TMV-based drug delivery system was proven to enable superior, targeted cytotoxicity as well as increased ease of uptake by cancer cells in in vitro systems using HepG2 and MCF-7 cancer cell lines (Liu et al., 2016).
Another anti-cancer drug, mitoxanthrone (MTO), is a topoisomerase II inhibitor and has been shown to be encapsulated by TMV (Lin and Steinmetz, 2018). VNPs exhibited superior tumor-reduction in mouse cancer models, while precluding severe cardiac outcomes that sometimes accompany direct delivery of MTO. Yet another anti-neoplastic and antimitotic drug, valine-citrulline monomethyl auristatin E (vcMMAE), was bound to the exterior of TMV VNPs which targeted non-Hodgkin’s lymphoma. Internalization into endolysosomal compartments was reported (Kernan et al., 2017), most likely accompanied by the protease-mediated release of the drug. This system was efficient in terms of cytotoxicity towards the in vitro Karpas 299 non-Hodgkin’s lymphoma cell line with an IC50 of 250 nM.
Helical plant virus nanoparticles (VLPs) have also been used as combination therapies to augment their immune efficacy. The PVX-DOX (doxorubicin) (Lee et al., 2017) combination was shown to be highly effective in stimulating cytokine/chemokine levels while prolonging the survival of mice in melanoma models compared to that obtained through the administration of either PVX or DOX alone.
PVX displaying TNF related apoptosis inducing ligand (TRAIL) was used to promote the recruitment and activation of death receptors in vitro in HCC-38 primary ductal carcinoma, BT-549 ductal carcinoma and the MDA-MB-231 breast cancer cell lines (Le et al., 2017; Röder et al., 2018). In vivo mouse models also demonstrated that the PVX-TRAIL formulation potently inhibited tumor growth. PVX has also been used by displaying tumor necrosis factor (TNF)-related apoptosis inducing ligand (TRAIL) on the surface of VNPs. Multivalent display of TRAIL enabled increased recruitment and stimulation of death receptors expressed on cancer cell lines (Le et al., 2019). Similarly, this formulation was shown to successfully suppress tumor growth in mice breast cancer models.
An efficient and new drug delivery system has been reported for Non-Hodgkin’s B cell lymphomas (NHL) based on PVX binding affinity towards malignant B cells. PVX loaded with monomethyl auristatin (MMAE) and administered to tissues harboring malignant B cells lead to inhibition of NHL growth in a mouse model (Shukla et al., 2020). Jobsri et al., 2015 reported a study in which PVX was conjugated to an idiotypic (Id) tumor-associated antigen (TAA) recombinant through a biotin/streptavidin linker, that elicited a 7 times higher anti-Id IgG response compared to Id alone in a mouse B-cell lymphoma model. Cytokine profiling in these mice revealed that the induction of IFN-α and IL-12, also that TLR7 was essential for viral RNA recognition.
PVX nanoparticles are increasingly being used for immunotherapy of tumor microenvironments. The monoclonal antibodies of Herceptin or Trastuzumab can be loaded onto PVX nanofilaments, which successfully induced apoptosis in breast cancer cell lines (Esfandiari et al., 2016). PVX was used as an expression vector for a mutant form of the HPV16 E7 oncoprotein, by fusing it with lichenase. This elicited protection against tumor progression in mice by inducing a robust cytotoxic T-cell response (Demurtas et al., 2013).
Steinmetz et al. (2009), found that CPMV nanoparticles could bind to vimentin, a protein found on the surface of most cells. Vimentin is upregulated during tumor progression, making it an attractive target for cancer therapy. The fact that surface vimentin expression correlated with CPMV uptake in this study demonstrated the ability of CPMV to detect invasive cancer cells.
The tumor microenvironment poses a great challenge to immune clearance by virtue of being immunosuppressive and favoring immune escape of the tumors through the inhibition of anti-tumor T-cells (Chung et al., 2020). CPMV VLP nanoparticles were shown to decrease tumor growth in murine models of lung melanomas, ovarian, colon and breast tumors (Lizotte et al., 2016; Wang et al., 2019). Mechanistically, CPMV has been shown to reprogram the tumor microenvironment by recruitment of natural killer cells and neutrophils, while enabling the transition of M2 to M1 anti-tumor macrophages. This innate immune cell population subsequently combats the tumor leading to cell lysis. Most recently, Mao et al. (2021) have deduced which TLRs are responsible for these properties.
The icosahedral shape of CPMV capsid can be loaded with precise drug cargos to target tumor and cancer cells. CPMV VNPs have also been formulated as slow-release aggregates along with polyamidoamine generation 4 dendrimers (CPMV-G4) (Czapar et al., 2018), where they were shown to be effective in combating ovarian cancer in murine models, even when provided as a single dosage.
CPMV VLPs have been attached to TAAs (tumor associated antigens) using chemical conjugation, genetic fusion and enzyme-mediated ligation techniques. For example, the human epidermal growth factor receptor 2 (HER2) epitope, when conjugated to the icosahedral CPMV, was successfully delivered to the lymphatic system with enhanced uptake and activation of APCs that led to an augmented anti-HER2 immune response. The CPMV HER2 candidate vaccine slowed tumor progression and metastasis in mouse models, enhancing survival (Shukla et al., 2013). Importantly, CPMV-HER2 stimulated a predominantly Th1 immune response while Sesbania Mosaic Virus-HER2 and CCMV-HER2 induced mostly a Th2 response in mouse models, thus proving that the nature of the epitope carrier itself plays an essential role in regulating the Th1/Th2 bias. This could be due to differences in epitope display on the surface of the VNPs as well as the capsid.
Cancer vaccines against carbohydrate antigens associated with tumors (TACAs) could be useful for diminishing tumor progression. Nevertheless, carbohydrates are weakly immunogenic and therefore, plant viruses used as carriers of these molecules could enhance the immune response to TACAs. CPMV-TACA conjugates targeting the Tn antigen (GalNAc-α-O-Ser/Thr) (Yin et al., 2012) were demonstrated to induce enhanced IgG titers, implicating heightened T-cell mediated immunity and antibody isotype switching in mouse models. IgG binding to the Tn antigens were observed in experiments wherein mice sera were added to breast cancer cell lines.
The chemotherapeutic cyclophosphamide, when used in combination with CPMV VNPs, profoundly elicited tumor cell death, releasing extracellular TAAs and stimulating immune cell invasion in addition to augmenting TAA recognition and antigen presentation (Cai et al., 2019) in mouse tumor models. CPMV VNPs have also been administered in combination with CD47-blocking antibodies (Wang and Steinmetz, 2019) which proved to have synergistic effects in combating tumor growth in murine ovarian tumor models, where it activated phagocytes, leading to stimulation of the adaptive immune response. Similar synergistic effects were observed when CPMV VNPs were used in combination with the anti-programmed cell death-1 checkpoint inhibitor (Lam et al., 2018). In addition to this, CPMV has been used successfully in promoting anti-tumor effects, when combined with radiation therapy. In this instance, CPMV was shown to enhance the recruitment of APCs, which in turn targeted the extracellular TAAs and phagocytosed them to induce a prolonged effectual immune response (Patel et al., 2018) in mice and canine models.
The CPMV-DOX conjugate was developed using eighty molecules of the chemotherapeutic drug doxorubicin (DOX), covalently bound to carboxylates at the external surface of the CPMV nanoparticle. This drug delivery vehicle was found to be more cytotoxic than free DOX when used in low concentration, however, CPMV-DOX cytotoxicity is time-delayed at higher concentrations (Aljabali et al., 2013). Cancer cells manage to resist immunotherapies owing to the immunosuppressive nature of tumors. CPMV nanoparticles have been reported as an in situ vaccine to stimulate an anti-tumor response and overcome local immunosuppression (Shukla et al., 2020). CPMV is also shown to be effective for ovarian cancer. The strategy for immunotherapy resulting antitumor efficacy is promising and involved the formation of aggregates of CPMV and polyamidoamine generation 4 dendrimers (CPMV-G4). Administration of CPMV-G4 effectively reduced ovarian cancer (Czapar et al., 2018). CPMV nanoparticles thus provide a therapeutic application for tumor targeting, intravital imaging and cancer therapy (Yildiz et al., 2013). Further exploration into the pharmacology of CPMV nanoparticles will further elucidate its roles in the immune response (Nkanga et al., 2021).
Patel et al. (2018), used CPMV nanoparticles in conjunction with radiotherapy to delay ovarian tumor growth in a mouse model. The treatment was able to result in an increase in tumor infiltrating lymphocytes (TILs), suggesting that this combined treatment could act as a future in situ tumor vaccine. Further studies by Wang and Steinmetz (2019) found that a protein known as CD47, that is widely expressed on tumor cells, prevents the action of T cells and phagocytic cells. The authors used a combination therapy of CD47-blocking antibodies and CPMV nanoparticles to act synergistically and elicit an anti-tumor immune response. The same research group also used low doses of cyclophosphamide (CPA) and CPMV nanoparticles as a combination therapy to successfully reduce mouse tumors in vivo (Wang and Steinmetz, 2019).
Conclusion and Future Directions
The use of plant virus nanoparticles (VNPs) as drug delivery carriers for the treatment of infectious and chronic diseases including cancer are advantageous when compared with naked drugs (Shoeb and Hefferon, 2019; Hefferon, 2018). The most promising nanoparticle systems have been adopted from naturally occurring plant viruses. Plant viruses are ideal for drug delivery as they are safe, non-infectious and nontoxic to humans (Beatty and Lewis, 2019). Cancer cells exhibit specific antigens on the surface of tumor cells which can be identified and targeted by plant-virus based nanoparticles, thus providing a clinical application of diagnosis and therapeutics for cancer. The most promising nano-scale systems have been adopted from naturally occurring plant viruses such as Tobacco mosaic virus (TMV), Cowpea mosaic virus (CPMV), Potato virus X (PVX) and many more. Currently, these new strategies are only applied in small scale production. As these approaches undergo further development, we will witness a spectrum of possible applications in the fields of medicine and biomedical engineering.
In the future, plant virus nanoparticles will need to be developed for high throughput manufacturing. This will require the dedication of facilities that can produce many grams of plant virus nanoparticles using tens of thousands of plants (McNulty et al., 2021). Today, manufacturing facilities have been generated for plant molecular farming, and adaptations could be tailored for nanoparticles (Fausther-Bovendo and Kobiger, 2021). The regulatory pathway will require more exploration to speed the process. More research regarding how plant virus nanoparticles act upon the immune system is underway and will be needed (Mao et al., 2021). Finally, the use of plant virus chimeras or semi-synthetic plant virus nanoparticles with novel properties must be explored, as well as novel modes of administration, such as microneedle patches (Boone et al., 2020).
Author Contributions
All authors listed have made a substantial, direct, and intellectual contribution to the work and approved it for publication.
Conflict of Interest
The authors declare that the research was conducted in the absence of any commercial or financial relationships that could be construed as a potential conflict of interest.
Publisher’s Note
All claims expressed in this article are solely those of the authors and do not necessarily represent those of their affiliated organizations, or those of the publisher, the editors and the reviewers. Any product that may be evaluated in this article, or claim that may be made by its manufacturer, is not guaranteed or endorsed by the publisher.
References
Adams, M. J., Antoniw, J. F., Bar-Joseph, M., Brunt, A. A., Candresse, T., Foster, G. D., et al. (2004). Virology Division News: The New Plant Virus Family Flexiviridae and Assessment of Molecular Criteria for Species Demarcation. Arch. Virol. 149 (5), 1045–1060. doi:10.1007/s00705-004-0304-0
Albakri, M. M., Veliz, F. A., Fiering, S. N., Steinmetz, N. F., and Sieg, S. F. (2020). Endosomal Toll‐like Receptors Play a Key Role in Activation of Primary Human Monocytes by Cowpea Mosaic Virus. Immunology 159 (2), 183–192. doi:10.1111/imm.13135
Aljabali, A. A. A., Shukla, S., Lomonossoff, G. P., Lomonossoff, N. F., and Steinmetz, D. J. (2013). CPMV-DOX Delivers. Mol. Pharmaceutics 10 (1), 3–10. doi:10.1021/mp3002057
Balke, I., and Zeltins, A. (2020). Recent Advances in the Use of Plant Virus-like Particles as Vaccines. Viruses 12 (3), 270. doi:10.3390/v12030270
Beatty, P. H., and Lewis, J. D. (2019). Cowpea Mosaic Virus Nanoparticles for Cancer Imaging and Therapy. Adv. Drug Deliv. Rev. 145, 130–144. doi:10.1016/j.addr.2019.04.005
Boone, C. E., Wang, C., Lopez-Ramirez, M. A., Beiss, V., Shukla, S., Chariou, P. L., et al. (2020). Active Microneedle Administration of Plant Virus Nanoparticles for Cancer In Situ Vaccination Improves Immunotherapeutic Efficacy. ACS Appl. Nano Mater. 3 (8), 8037–8051. doi:10.1021/acsanm.0c01506
Bruckman, M. A., Hern, S., Jiang, K., Flask, C. A., Yu, X., and Steinmetz, N. F. (2013). Tobacco Mosaic Virus Rods and Spheres as Supramolecular High-Relaxivity MRI Contrast Agents. J. Mater. Chem. B 1 (10), 1482–1490. doi:10.1039/C3TB00461A
Brun, M. J., Gomez, E. J., and Suh, J. (2017). Stimulus-responsive Viral Vectors for Controlled Delivery of Therapeutics. J. Controlled Release 267, 80–89. doi:10.1016/j.jconrel.2017.08.021
Byun, D. J., Wolchok, J. D., Rosenberg, L. M., and Girotra, M. (2017). Cancer Immunotherapy - Immune Checkpoint Blockade and Associated Endocrinopathies. Nat. Rev. Endocrinol. 13 (4), 195–207. doi:10.1038/nrendo.2016.205
Cai, H., Wang, C., Shukla, S., and Steinmetz, N. F. (2019). Cowpea Mosaic Virus Immunotherapy Combined with Cyclophosphamide Reduces Breast Cancer Tumor Burden and Inhibits Lung Metastasis. Adv. Sci. 6 (16), 1802281. doi:10.1002/advs.201802281
Cano-Garrido, O., Álamo, P., Sánchez-García, L., Falgàs, A., Sánchez-Chardi, A., Serna, N., et al. (2021). Biparatopic Protein Nanoparticles for the Precision Therapy of CXCR4+ Cancers. Cancers 13 (12), 2929. doi:10.3390/cancers13122929
Carignan, D., Thérien, A., Rioux, G., Paquet, G., Gagné, M.-È. L., Bolduc, M., et al. (2015). Engineering of the PapMV vaccine platform with a shortened M2e peptide leads to an effective one dose influenza vaccine. Vaccine 33 (51), 7245–7253. doi:10.1016/j.vaccine.2015.10.123
Cho, C.-F., Shukla, S., Simpson, E. J., Steinmetz, N. F., Luyt, L. G., and Lewis, J. D. (2014). Molecular Targeted Viral Nanoparticles as Tools for Imaging Cancer. Methods Mol. Biol. (Clifton, N.J.) 1108, 211–230. doi:10.1007/978-1-62703-751-8_16
Chung, Y. H., Cai, H., and Steinmetz, N. F. (2020). Viral Nanoparticles for Drug Delivery, Imaging, Immunotherapy, and Theranostic Applications. Adv. Drug Deliv. Rev. 156, 214–235. doi:10.1016/j.addr.2020.06.024
Czapar, A. E., Tiu, B. D. B., Veliz, F. A., Pokorski, J. K., and Steinmetz., N. F. (2018). Slow-Release Formulation of Cowpea Mosaic Virus for In Situ Vaccine Delivery to Treat Ovarian Cancer. Adv. Sci. 5 (5), 1700991. doi:10.1002/advs.20170099110.1002/advs.201700991
Czapar, A. E., Zheng, Y.-R., Riddell, I. A., Shukla, S., Awuah, S. G., Lippard, S. J., et al. (2016). Tobacco Mosaic Virus Delivery of Phenanthriplatin for Cancer Therapy. ACS Nano 10 (4), 4119–4126. doi:10.1021/acsnano.5b07360
Demurtas, O. C., Massa, S., Ferrante, P., Venuti, A., Franconi, R., Giuliano, G. A., et al. (2013). A Chlamydomonas-derived Human Papillomavirus 16 E7 vaccine induces specific tumor protection. PLoS One 23 (8), e61473. doi:10.1371/journal.pone.0061473
Esfandiari, N., Arzanani, M. K., Soleimani, M., Kohi-Habibi, M., and Svendsen, W. E. (2016). A New Application of Plant Virus Nanoparticles as Drug Delivery in Breast Cancer. Tumor Biol. 37, 1229–1236. doi:10.1007/s13277-015-3867-3
Farokhzad, O. C. (2008). Nanotechnology for Drug Delivery: the Perfect Partnership. Expert Opin Drug Deliv. 5 (9), 927–929. doi:10.1517/17425247.5.9.927
Fausther-Bovendo, H., and Kobinger, G. (2021). Plant-made Vaccines and Therapeutics. Science 13, 740–741. doi:10.1126/science.abf5375
FDA Drug Products (2017). Including Biological Productsd, that Contain Nanomaterials-Guidance for Industry. Silver Spring, Maryland: FDA Drug Products.
Finbloom, J., Aanei, I., Bernard, J., Klass, S., Elledge, S., Han, K., et al. (2018). Evaluation of Three Morphologically Distinct Virus-like Particles as Nanocarriers for Convection-Enhanced Drug Delivery to Glioblastoma. Nanomaterials 8 (12), 1007. doi:10.3390/nano8121007
Franke, C. E., Czapar, A. E., Patel, R. B., and Steinmetz, N. F. (2018). Tobacco Mosaic Virus-Delivered Cisplatin Restores Efficacy in Platinum-Resistant Ovarian Cancer Cells. Mol. Pharmaceutics 15, 2922–2931. doi:10.1021/acs.molpharmaceut.7b00466
Hansen, A. E., Petersen, A. L., Henriksen, J. R., Boerresen, B., Rasmussen, P., Elema, D. R., et al. (2015). Positron Emission Tomography Based Elucidation of the Enhanced Permeability and Retention Effect in Dogs with Cancer Using Copper-64 Liposomes. ACS Nano 9 (7), 6985–6995. doi:10.1021/acsnano.5b01324
Haynes, J. R., Cunningham, J., von Seefried, A., Lennick, M., Garvin, R. T., and Shen, S.-H. (1986). Development of a Genetically-Engineered, Candidate Polio Vaccine Employing the Self-Assembling Properties of the Tobacco Mosaic Virus Coat Protein. Nat. Biotechnol. 4 (7), 637–641. doi:10.1038/nbt0786-637
Hefferon, K. (2018). Repurposing Plant Virus Nanoparticles. Vaccines 6 (1), 11. doi:10.3390/vaccines6010011
Hu, H., Zhang, Y., Shukla, S., Gu, Y., Yu, X., and Steinmetz, N. F. (2017). Dysprosium-Modified Tobacco Mosaic Virus Nanoparticles for Ultra-high-field Magnetic Resonance and Near-Infrared Fluorescence Imaging of Prostate Cancer. ACS nano 11 (9), 9249–9258. doi:10.1021/acsnano.7b04472
Jobsri, J., Allen, A., Rajagopal, D., Shipton, M., Kanyuka, K., Lomonossoff, G. P., et al. (2015). Plant Virus Particles Carrying Tumour Antigen Activate TLR7 and Induce High Levels of Protective Antibody. PLoS One 10 (2), e0118096. doi:10.1371/journal.pone.0118096
Kernan, D. L., Wen, A. M., Pitek, A. S., and Steinmetz, N. F. (2017). Featured Article: Delivery of Chemotherapeutic vcMMAE Using Tobacco Mosaic Virus Nanoparticles. Exp. Biol. Med. (Maywood) 242, 1405–1411. doi:10.1177/1535370217719222
Kwon, S.-J., Park, M.-R., Kim, K.-W., Plante, C. A., Hemenway, C. L., and Kim, K.-H. (2005). cis-Acting Sequences Required for Coat Protein Binding and In Vitro Assembly of Potato Virus X. Virology 334 (1), 83–97. doi:10.1016/j.virol.2005.01.018
Lam, P., Lin, R. D., and Steinmetz, N. F. (2018). Delivery of Mitoxantrone Using a Plant Virus-Based Nanoparticle for the Treatment of Glioblastomas. J. Mater. Chem. B 6 (37), 5888–5895. doi:10.1039/C8TB01191E
Le, D. H. T., Commandeur, U., and Steinmetz, N. F. (2019). Presentation and Delivery of Tumor Necrosis Factor-Related Apoptosis-Inducing Ligand via Elongated Plant Viral Nanoparticle Enhances Antitumor Efficacy. ACS Nano 13, 2501–2510. doi:10.1021/acsnano.8b09462
Le, D. H. T., Lee, K. L., Shukla, S., Commandeur, U., and Steinmetz, N. F. (2017). Potato Virus X, a Filamentous Plant Viral Nanoparticle for Doxorubicin Delivery in Cancer Therapy. Nanoscale 9 (6), 2348–2357. doi:10.1039/c6nr09099k
Leary, J., and Key, J. (2014). Nanoparticles for Multimodal In Vivo Imaging in Nanomedicine. Int. J. Nanomedicine 9, 711–726. doi:10.2147/ijn.s53717
Lee, K. L., Murray, A. A., Le, D. H. T., Sheen, M. R., Shukla, S., Commandeur, U., et al. (2017). Combination of Plant Virus Nanoparticle-Based In Situ Vaccination with Chemotherapy Potentiates Antitumor Response. Nano Lett. 17, 4019–4028. doi:10.1021/acs.nanolett.7b00107
Lin, R. D., and Steinmetz., N. F. (2018). Tobacco Mosaic Virus Delivery of Mitoxantrone for Cancer Therapy. Nanoscale 10 (34), 16307–16313. doi:10.1039/c8nr04142c
Liu, L., Cañizares, M. C., Monger, W., Perrin, Y., Tsakiris, E., Porta, C., et al. (2005). Cowpea Mosaic Virus-Based Systems for the Production of Antigens and Antibodies in Plants. Vaccine 23 (15), 1788–1792. doi:10.1016/j.vaccine.2004.11.006
Liu, X., Wu, F., Tian, Y., Wu, M., Zhou, Q., Jiang, S., et al. (2016). Size Dependent Cellular Uptake of Rod-like Bionanoparticles with Different Aspect Ratios. Sci. Rep. 6 (1), 24567. doi:10.1038/srep24567
Lizotte, P. H., Wen, A. M., Sheen, M. R., Fields, J., Rojanasopondist, P., Steinmetz, N. F., et al. (2016). In Situ vaccination with Cowpea Mosaic Virus Nanoparticles Suppresses Metastatic Cancer. Nat. Nanotech 11 (3), 295–303. doi:10.1038/nnano.2015.292
Ma, Y.-Y., Jin, K.-T., Wang, S.-B., Wang, H.-J., Tong, X.-M., Huang, D.-S., et al. (2017). Molecular Imaging of Cancer with Nanoparticle-Based Theranostic Probes. Contrast Media Mol. Imaging 2017, 1–11. doi:10.1155/2017/1026270
Mao, C., Beiss, V., Fields, J., Steinmetz, N. F., and Fiering, S. (2021). Cowpea Mosaic Virus Stimulates Antitumor Immunity through Recognition by Multiple MYD88-Dependent Toll-like Receptors. Biomaterials 275, 120914. doi:10.1016/j.biomaterials.2021.120914
Massumi, H., Poormohammadi, S., Pishyar, S., Maddahian, M., Heydarnejad, J., Hosseini-Pour, A., et al. (2014). Molecular Characterization and Field Survey of Iranian Potato Virus X Isolates. Virus Dis. 25 (3), 338–344. doi:10.1007/s13337-014-0222-z
McNulty, M. J., Kelada, K., Paul, D., Nandi, S., and McDonald, K. (2021). Introducing Uncertainty Quantification to Techno-Economic Models of Manufacturing Field-Grown Plant-Made Products. doi:10.31224/osf.io/m8gvx
Meshcheriakova, Y., Durrant, A., Hesketh, E. L., Ranson, N. A., and Lomonossoff, G. P. (2017). Combining High-Resolution Cryo-Electron Microscopy and Mutagenesis to Develop Cowpea Mosaic Virus for Bionanotechnology. Biochem. Soc. Trans. 45 (6), 1263–1269. doi:10.1042/bst20160312
Moreira, A. F., Dias, D. R., and Correia, I. J. (2016). Stimuli-responsive Mesoporous Silica Nanoparticles for Cancer Therapy: A Review. Microporous Mesoporous Mater. 236, 141–157. doi:10.1016/j.micromeso.2016.08.038
Nemykh, M. A., Efimov, A. V., Novikov, V. K., Orlov, V. N., Arutyunyan, A. M., Drachev, V. A., et al. (2008). One More Probable Structural Transition in Potato Virus X Virions and a Revised Model of the Virus Coat Protein Structure. Virology 373 (1), 61–71. doi:10.1016/j.virol.2007.11.024
Niehl, A., Appaix, F., Boscá, S., van der Sanden, B., Nicoud, J.-F., Bolze, F., et al. (2016). Fluorescent Tobacco Mosaic Virus-Derived Bio-Nanoparticles for Intravital Two-Photon Imaging. Front. Plant Sci. 6, 1244. doi:10.3389/fpls.2015.01244
Nkanga, C. I., and Steinmetz, N. F. (2021). The Pharmacology of Plant Virus Nanoparticles. Virology 556, 39–61. doi:10.1016/j.virol.2021.01.012
Nooraei, S., Bahrulolum, H., Hoseini, Z. S., Katalani, C., Hajizade, A., Easton, A. J., et al. (2021). Virus-like Particles: Preparation, Immunogenicity and Their Roles as Nanovaccines and Drug Nanocarriers. J. Nanobiotechnol 19 (1), 59. doi:10.1186/s12951-021-00806-7
Park, K. (2019). The Beginning of the End of the Nanomedicine Hype. J.Control. Release 305. doi:10.1016/j.jconrel.2019.05.044
Park, M.-R., Kwon, S.-J., Choi, H.-S., Hemenway, C. L., and Kim, K.-H. (2008). Mutations that Alter a Repeated ACCA Element Located at the 5′ End of the Potato Virus X Genome Affect RNA Accumulation. Virology 378 (1), 133–141. doi:10.1016/j.virol.2008.05.004
Parker, L., Kendall, A., and Stubbs, G. (2002). Surface Features of Potato Virus X from Fiber Diffraction. Virology 300 (2), 291–295. doi:10.1006/viro.2002.1483
Patel, R., Czapar, A. E., Fiering, S., Oleinick, N. L., and Steinmetz, N. F. (2018). Radiation Therapy Combined with Cowpea Mosaic Virus Nanoparticle In Situ Vaccination Initiates Immune-Mediated Tumor Regression. ACS Omega 3 (4), 3702–3707. doi:10.1021/acsomega.8b00227
Paviolo, C., and Stoddart, P. (2017). Gold Nanoparticles for Modulating Neuronal Behavior. Nanomaterials 7, 92. doi:10.3390/nano7040092
Pitek, A. S., Jameson, S. A., Veliz, F. A., Shukla, S., and Steinmetz, N. F. (2016). Serum Albumin 'camouflage' of Plant Virus Based Nanoparticles Prevents Their Antibody Recognition and Enhances Pharmacokinetics. Biomaterials 89, 89–97. doi:10.1016/j.biomaterials.2016.02.032
Pokorski, J. K., Breitenkamp, K., Liepold, L. O., Qazi, S., and Finn, M. G. (2011). Functional Virus-Based Polymer-Protein Nanoparticles by Atom Transfer Radical Polymerization. J. Am. Chem. Soc. 133 (24), 9242–9245. doi:10.1021/ja203286n
Pokorski, J. K., and Steinmetz, N. F. (2011). The Art of Engineering Viral Nanoparticles. Mol. Pharmaceutics 8 (1), 29–43. doi:10.1021/mp100225y
Röder, J., Dickmeis, C., and Commandeur, U. (2019). Small, Smaller, Nano: New Applications for Potato Virus X in Nanotechnology. Front. Plant Sci. 10, 158. doi:10.3389/fpls.2019.00158
Röder, J., Dickmeis, C., Fischer, R., and Commandeur, U. (2018). Systemic Infection of Nicotiana Benthamianawith Potato Virus X Nanoparticles Presenting a Fluorescent iLOV Polypeptide Fused Directly to the Coat Protein. Biomed. Res. Int. 2018, e9328671. doi:10.1155/2018/9328671
Röder, J., Fischer, R., and Commandeur, U. (2017). Adoption of the 2A Ribosomal Skip Principle to Tobacco Mosaic Virus for Peptide Display. Front. Plant Sci. 8, 1125. doi:10.3389/fpls.2017.01125
Rong, J., Niu, Z., Lee, L. A., and Wang, Q. (2011). Self-assembly of Viral Particles. Curr. Opin. Colloid Interf. Sci. 16 (6): 441–450. doi:10.1016/j.cocis.2011.09.001
Rybicki, E. P. (2020). Plant Molecular Farming of Virus‐like Nanoparticles as Vaccines and Reagents. WIREs Nanomed Nanobiotechnol 12 (2), e1587. doi:10.1002/wnan.1587
Sainsbury, F., Cañizares, M. C., and Lomonossoff, G. P. (2010). Cowpea mosaicVirus: The Plant Virus-Based Biotechnology Workhorse. Annu. Rev. Phytopathol. 48, 437–455. doi:10.1146/annurev-phyto-073009-114242
Shahgolzari, M., Pazhouhandeh, M., Milani, M., Yari Khosroushahi, A., and Fiering, S. (2020). Plant Viral Nanoparticles for Packaging and In Vivo Delivery of Bioactive Cargos. Wiley Interdiscip Rev Nanomed Nanobiotechnol. 12 (5), e1629. doi:10.1002/wnan.1629
Shahgolzari, M., Dianat-Moghadam, H., and Fiering, S. (2021). Multifunctional Plant Virus Nanoparticles in the Next Generation of Cancer Immunotherapies. Semin. Cancer Biol. 2021, S1044. doi:10.1016/j.semcancer.2021.07.018
Shoeb, E., and Hefferon, K. (2019). Future of Cancer Immunotherapy Using Plant Virus-Based Nanoparticles. Future Sci. OA 5 (7), FSO401. doi:10.2144/fsoa-2019-0001
Shukla, S., Ablack, A. L., Wen, A. M., Lee, K. L., Lewis, J. D., and Steinmetz, N. F. (2013). Increased Tumor Homing and Tissue Penetration of the Filamentous Plant Viral Nanoparticle Potato Virus X. Mol. Pharmaceutics 10 (1), 33–42. doi:10.1021/mp300240m
Shukla, S., Dickmeis, C., Fischer, R., Commandeur, U., and Steinmetz, N. F. (2018). In Planta Production of Fluorescent Filamentous Plant Virus-Based Nanoparticles. Methods Mol. Biol. 1776, 61–84. doi:10.1007/978-1-4939-7808-3_5
Shukla, S., Roe, A. J., Liu, R., Veliz, F. A., Commandeur, U., Wald, D. N., et al. (2020). Affinity of Plant Viral Nanoparticle Potato Virus X (PVX) towards Malignant B Cells Enables Cancer Drug Delivery. Biomater. Sci. 8, 3935–3943. doi:10.1039/D0BM00683A
Shukla, S., Wang, C., Beiss, V., Cai, H., Washington, T., Murray, A. A., et al. (2020). The Unique Potency of Cowpea Mosaic Virus (CPMV) In Situ Cancer Vaccine. Biomater. Sci. 8 (19), 5489–5503. doi:10.1039/D0BM01219J10.1039/D0BM01219J
Singh, P., Prasuhn, D., Yeh, R. M., Destito, G., Rae, C. S., Osborn, K., et al. (2007). Bio-distribution, Toxicity and Pathology of Cowpea Mosaic Virus Nanoparticles In Vivo. J. Controlled Release 120, 41–50. doi:10.1016/j.jconrel.2007.04.003
Steinmetz, N. F., and Evans, D. J. (2007). Utilisation of Plant Viruses in Bionanotechnology. Org. Biomol. Chem. 5 (18), 2891–2902. doi:10.1039/b708175h
Steinmetz, N. F., and Manchester., M. (2009). PEGylated Viral Nanoparticles for Biomedicine: the Impact of PEG Chain Length on VNP Cell Interactions In Vitro and Ex Vivo. Biomacromolecules 10 (4), 784–792. doi:10.1021/bm8012742
Steinmetz, N. F., Shah, S. N., Barclay, J. E., Rallapalli, G., Lomonossoff, G. P., and Evans, D. J. (2009). Virus-templated Silica Nanoparticles. Small 5 (7), 813–816. doi:10.1002/smll.200801348
Steinmetz, N. F. (2010). Viral Nanoparticles as Platforms for Next-Generation Therapeutics and Imaging Devices. Nanomedicine: Nanotechnology, Biol. Med. 6 (5), 634–641. doi:10.1016/j.nano.2010.04.005
Tian, Y., Zhou, M., Shi, H., Gao, S., Xie, G., Zhu, M., et al. (2018). Integration of Cell-Penetrating Peptides with Rod-like Bionanoparticles: Virus-Inspired Gene-Silencing Technology. Nano Lett. 18, 5453–5460. doi:10.1021/acs.nanolett.8b01805
Turpen, T. H., Reinl, S. J., Charoenvit, Y., Hoffman, S. L., Fallarme, V., and Grill, L. K. (1995). Malaria Epitopes Expressed on the Surface of Recombinant Tobacco Mosaic Virus. Nat. Biotechnol. 13 (1), 53–57. doi:10.1038/nbt0195-53
Tyulkina, L. G., Skurat, E. V., Frolova, O. Y., Komarova, T. V., Karger, E. M., and Atabekov, I. G. (2011). New Viral Vector for Superproduction of Epitopes of Vaccine Proteins in Plants. Acta naturae 3 (4), 73. doi:10.32607/20758251-2011-3-4-73-82
Uhde-Holzem, K., McBurney, M., Tiu, B. D., Advincula, R. C., Fischer, R., Commandeur, U., et al. (2016). Production of Immunoabsorbent Nanoparticles by Displaying Single-Domain Protein A on Potato Virus X. Macromol. Biosci. 16, 231–241. doi:10.1002/mabi.201500280
Vernekar, A. A., Berger, G., Czapar, A. E., Veliz, F. A., Wang, D. I., Steinmetz, N. F., et al. (2018). Speciation of Phenanthriplatin and its Analogs in the Core of Tobacco Mosaic Virus. J. Am. Chem. Soc. 140, 4279–4287. doi:10.1021/jacs.7b12697
Wang, C., and Steinmetz, N. F. (2019). CD47 Blockade and Cowpea Mosaic Virus Nanoparticle In Situ Vaccination Triggers Phagocytosis and Tumor Killing. Adv. Healthc. Mater. 8 (8), e1801288. doi:10.1002/adhm.201801288
Wang, C., Beiss, V., and Steinmetz, N. F. (2019). Cowpea Mosaic Virus Nanoparticles and Empty Virus-like Particles Show Distinct but Overlapping Immunostimulatory Properties. J. Virol. 93 (21), e00129–19. doi:10.1128/JVI.00129-19
Wen, A. M., Infusino, M., De Luca, A., Kernan, D. L., Czapar, A. E., Strangi, G., et al. (2015b). Interface of Physics and Biology: Engineering Virus-Based Nanoparticles for Biophotonics. Bioconjug. Chem. 26 (1), 51–62. doi:10.1021/bc500524f
Wen, A. M., Wang, Y., Jiang, K., Hsu, G. C., Gao, H., Lee, K. L., et al. (2015a). Shaping Bio-Inspired Nanotechnologies to Target Thrombosis for Dual Optical-Magnetic Resonance Imaging. J. Mater. Chem. B 3 (29), 6037–6045. doi:10.1039/c5tb00879d
Wen, J., Xu, Y., Li, H., Lu, A., and Sun, S. (2015b). Recent Applications of Carbon Nanomaterials in Fluorescence Biosensing and Bioimaging. Chem. Commun. 51 (57), 11346–11358. doi:10.1039/C5CC02887F
Yildiz, I., Lee, K. L., Chen, K., Shukla, S., and Steinmetz, N. F. (2013). Infusion of imaging and therapeutic molecules into the plant virus-based carrier cowpea mosaic virus: cargo-loading and delivery. J Control Release 172 (2), 568–78. doi:10.1016/j.jconrel.2013.04.023
Yin, Z., Nguyen, H. G., Chowdhury, S., Bentley, P., Bruckman, M. A., Miermont, A., et al. (2012). Tobacco Mosaic Virus as a New Carrier for Tumor Associated Carbohydrate Antigens. Bioconjug. Chem. 23, 1694–1703. doi:10.1021/bc300244a
Young, M., Debbie, W., Uchida, M., and Douglas, T. (2008). Plant Viruses as Biotemplates for Materials and Their Use in Nanotechnology. Annu. Rev. Phytopathol. 46, 361–384. doi:10.1146/annurev.phyto.032508.131939
Zeng, Q., Wen, H., Wen, Q., Chen, X., Wang, Y., Xuan, W., et al. (2013). Cucumber Mosaic Virus as Drug Delivery Vehicle for Doxorubicin. Biomaterials 34 (19), 4632–4642. doi:10.1016/j.biomaterials.2013.03.017
Zhang, L., Gu, F., Chan, J., Wang, A., Langer, R., and Farokhzad, O. (2008). Nanoparticles in Medicine: Therapeutic Applications and Developments. Clin. Pharmacol. Ther. 83, 761–769. doi:10.1038/sj.clpt.6100400
Keywords: nanoparticles, plant virus-like particles, therapeutics, imaging, cancer 2
Citation: Venkataraman S, Apka P, Shoeb E, Badar U and Hefferon K (2021) Plant Virus Nanoparticles for Anti-cancer Therapy. Front. Bioeng. Biotechnol. 9:642794. doi: 10.3389/fbioe.2021.642794
Received: 16 December 2020; Accepted: 27 August 2021;
Published: 15 December 2021.
Edited by:
In-Kyu Park, Chonnam National University, South KoreaCopyright © 2021 Venkataraman, Apka, Shoeb, Badar and Hefferon. This is an open-access article distributed under the terms of the Creative Commons Attribution License (CC BY). The use, distribution or reproduction in other forums is permitted, provided the original author(s) and the copyright owner(s) are credited and that the original publication in this journal is cited, in accordance with accepted academic practice. No use, distribution or reproduction is permitted which does not comply with these terms.
*Correspondence: Paul Apka, cGF1bGFwa2EyNzVAZ21haWwuY29t