- 1Joint International Research Laboratory of Metabolic & Developmental Sciences, Key Laboratory of Urban Agriculture (South) Ministry of Agriculture, Plant Biotechnology Research Center, Fudan-Shanghai Jiaotong University (SJTU)-Nottingham Plant Biotechnology R&D Center, School of Agriculture and Biology, Shanghai Jiao Tong University, Shanghai, China
- 2Southwest University-Tibet Agriculture and Animal Husbandry College (SWU-TAAHC) Medicinal Plant Joint R&D Centre, School of Life Sciences, Southwest University, Chongqing, China
- 3Corporate R&D Division, Firmenich Aromatics (China) Co. Ltd., Shanghai, China
Terpenes constitute the largest class of secondary metabolites in plants. Some terpenes are essential for plant growth and development, membrane components, and photosynthesis. Terpenes are also economically useful for industry, agriculture, and pharmaceuticals. However, there is very low content of most terpenes in microbes and plants. Chemical or microbial synthesis of terpenes are often costly. Plants have the elaborate and economic biosynthetic way of producing high-value terpenes through photosynthesis. Here we engineered the heterogenous sesquiterpenoid patchoulol production in A. annua. When using a strong promoter such as 35S to over express the avian farnesyl diphosphate synthase gene and patchoulol synthase gene, the highest content of patchoulol was 52.58 μg/g DW in transgenic plants. When altering the subcellular location of the introduced sesquiterpene synthetase via a signal peptide, the accumulation of patchoulol was observably increased to 273 μg/g DW. This case demonstrates that A. annua plant with glandular trichomes is a useful platform for synthetic biology studies.
Introduction
Plants synthesize and secrete a good deal of secondary metabolites, some of which are considerable, economically in industry, agriculture, and pharmaceuticals (Balandrin et al., 1985; Pichersky and Gershenzon, 2002). Terpenes comprise the largest class of secondary metabolites in plants (Kappers et al., 2008). Many of them, such as phytohormones (abscisic acid, brassinosteroid, and gibberellin), sterols and carotenoid pigments, play critical roles in plant growth, development, membrane components, and photosynthesis (Bohlmann and Keeling, 2008). In addition, the majority of plant terpenes are involved in the interaction of plant with the environment and other organisms (Gershenzon and Dudareva, 2007). For instance, some terpenes bear antibacterial and antifungal activity (Rastogi et al., 1998; Lunde and Kubo, 2000). They can also hold the protective role in plants defense system against insects, mollusks, fish and nematodes (Lorimer et al., 1996; Ito et al., 1997; Laurent et al., 2003; Quintana et al., 2003). Terpenes can sometimes act a tool of communication among organisms. For instance, when the predators attack aphids, they normally release a kind of terpenoid, (E)-β-farnesene, as an alarm pheromone, to disperse and leave the host. Besides, (E)-β-farnesene is also released to attract natural enemies of aphids at the same time in plants (Hardie and Minks, 1999; Kunert et al., 2005).
On the basis of the number of five-carbon (isoprene) units, terpenoids are classified into hemiterpenes (half-terpenes), monoterpenes (C10), sesquiterpenes (C15), diterpenes (C20), triterpenes (C30), tetraterpenes (C40), polyterpenes, and meroterpenes (Croteau, 2000). In plants, terpenoids are synthesized from two precursors, dimethylallyl diphosphate (DMADP) and isopentenyl diphosphate provided by MVA (mevalonate) and MEP (2-C-methyl-D-erythritol-4-phosphate) pathway (Rohmer et al., 1996; Rohmer, 1999; Lange et al., 2000). In plants, terpenoids are synthesized from two precursors, dimethylallyl diphosphate (DMADP) and isopentenyl diphosphate provided by MVA (mevalonate) and MEP (2-C-methyl-D-erythritol-4-phosphate) pathway (Daviet and Schalk, 2010). Afterwards terpene synthases catalyze the cyclization of GPP (geranyl diphosphate), FPP (farnesyl diphosphate) and GGPP (geranylgeranyl diphosphate) to generate the carbon skeletons of terpenoids. Finally, the enzymes further modify the terpene backbone to synthesize plenty of natural terpene derivatives, for example, cytochrome P450 monooxygenases (P450) (Cheng et al., 2007) (Figure 1).
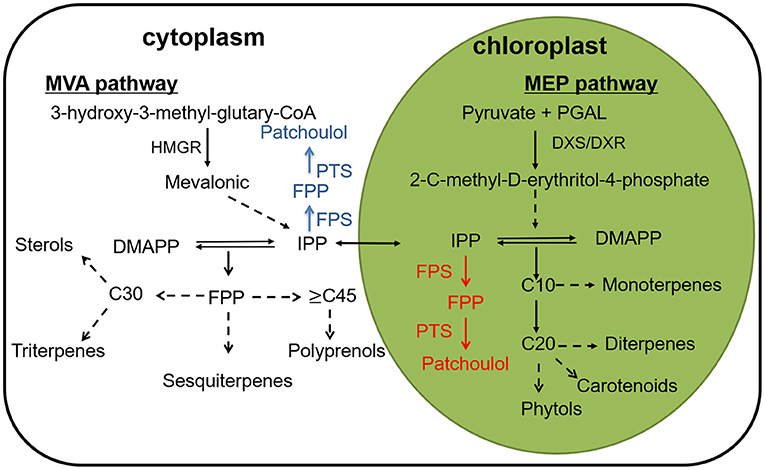
Figure 1. A depiction of the terpene biosynthesis, along with a conceptualization for patchoulol biosynthesis to the cytoplasm (blue) and to the chloroplast (red) compartments. HMGR, 3-hydroxy-3- methylglutaryl coenzyme A reductase; DXS, 1-deoxy-D-xylulose-5-phosphate synthase; DXR, 1-deoxy-D-xylulose5-phosphate reductase; FPS, farnesyl diphosphate synthase; FPP, farnesyl pyrophosphate; PTS, patchoulol synthase.
Overexploitation and wasteful consumption of natural resources for high-value terpenes compounds, may drive the species to extinction and alter the environment. For instance, Taxus chinensis is famous for Taxol, an effective anti-cancer drug (Ru et al., 2006). However, T. chinensis is facing extinction because of deforestation (Zhang and Ru, 2010). To date, many efforts have been made to manipulate terpene metabolism in microorganism (Carter et al., 2003; Martin et al., 2003), fungus (Jackson et al., 2003; Ro et al., 2006; Westfall et al., 2012; Paddon et al., 2013), and plants (Wu et al., 2006; Farhi et al., 2011; Zhan et al., 2014; Wang et al., 2016) to synthetically produce more high-value chemicals.
Engineering terpene metabolism in plants is an innovative and attractive strategy to provide high-value terpenes. Plants have the elaborate biosynthetic ability and a cheaper way of using photosynthesis to produce high-value terpenes (Wu et al., 2006). For example, the metabolic engineered tobaccos stably transformed with the deregulated 3-hydroxy-3-methylglutaryl-coenzyme A reductase gene (tHMG), ADS, CYP71AV1, CPR, and artemisinic aldehyde reductase gene (DBR2), produced artemisinin, although the content in the transgenic tobaccos was much lower than that in A. annua plants (Farhi et al., 2011). In another study, the transgenic tobacco accumulated high-levels of terpenes, containing patchoulol, amorpha-4,11-diene, and limonene, via overexpressing farnesyl diphosphate synthase gene from avian and the terpene synthase gene to control the carbon flux (Wu et al., 2006). The results from these researches indicate the necessity of deeper exploring and demonstration of terpenes biosynthesis pathway genes with respect to over-expression strategies (Wu et al., 2006). In recent years, various strategies for engineering of triterpene squalene metabolism in tobacco have been developed. For instance, co-expression of farnesyl diphosphate synthase gene FPS from avian and yeast squalene synthase gene SQS, driven by trichome-specific gene promoter in the chloroplast, resulted in the accumulation of squalene to a high level in transgenic tobacco (Wu et al., 2012). These findings suggested that the accumulation of high-value terpenes could be observably elevated by directing the biosynthesis to other subcellular compartments.
Patchoulol, a volatile sesquiterpenoid isolated from leaves of Pogostemon cablin plants, is an important ingredient in fragrance products like perfumes, soaps and cosmetics. Plant patchouli is the only commercial source of this compound (Srikrishna and Satyanarayana, 2005). Limited natural resources lead to the fluctuation in the price of patchoulol between 30 and 200 US dollar/kg (Zhan et al., 2014). A sesquiterpene cyclase enzyme, patchoulol synthase was identified to catalyze FPP to form patchoulol in patchouli plants (Deguerry et al., 2006). With the development of molecular and synthetic biology, the engineered tobacco could produce 0.030 mg/g fresh weight (FW) patchoulol (Wu et al., 2006). Biotechnological production of patchoulol has been carried out in Physcomitrella patens, and the highest yield of patchoulol was 1.34 mg/g dry weight (Zhan et al., 2014). Besides, Albertsen et al. reported that expression of FPPS of yeast fused with PTS from P. cablin in Saccharomyces cerevisiae increased the production of patchoulol compared with the accumulation produced by PTS (Albertsen et al., 2011). Subsequently, several strategies were adopted to increase patchoulol content in S. cerevisiae. The shaken flask contained 59.2 ± 0.7 mg/L patchoulol, and a final production was 466.8 ± 12.3 mg/L (20.5 ± 0.5 mg/g dry cell weight) after fermentation optimization (Ma et al., 2019).
Here we demonstrated, an engineered heterogenous sesquiterpenoid patchoulol production in A. annua. The highest content of patchoulol was 52.58 μg/g dry weight in the transgenic plants by overexpressing farnesyl diphosphate synthase gene and patchoulol synthase gene. Furthermore, the accumulation of patchoulol was increased to 273 μg/g dry weight by altering the subcellular location of the introduced sesquiterpene synthetase expression.
Result
Patchoulol Was Produced in FPS+PTS-overexpressing Transgenic A. annua Plants
To engineer the heterogenous sesquiterpenoid patchoulol production in A. annua, patchoulol synthase gene (PTS) from P. cablin and farnesyl diphosphate synthase gene (FPS) from an avian were chosen (Tarshis et al., 1994, Deguerry et al., 2006). Despite the cloned and identified FPS from A. annua, the avian FPS was observed not to be operated by the transcriptional or post-translational regulatory mechanisms in plants (Wu et al., 2006). To generate FPS+PTS-overexpressing transgenic A. annua plants, both PTS and FPS were transformed into A. annua plants via A. tumefaciens EHA105. Analysis of PCR showed that 25 independent lines were obtained. We then performed qRT-PCR to test the expression of both FPS and PTS genes in the transgenic lines. Among these, six transgenic lines showed a combination of high levels of FPS and PTS overexpression (Figure 2A). The patchoulol content in leaves was measured by GC-MS and the sesquiterpene alcohol patchoulol was successfully identified in FPS+PTS-overexpressing transgenic lines (Supplementary Figure 1). Quantification of patchoulol in transgenic lines showed that the six lines produced 23.51–52.58 μg patchoulol/g dry weight (Figure 2B), which was twice the transgenic tobaccos (Wu et al., 2006). The expressions of both FPS and PTS were higher than other transgenic lines in FPS+PTS-6 and 10, so they accumulated the highest patchoulol content.
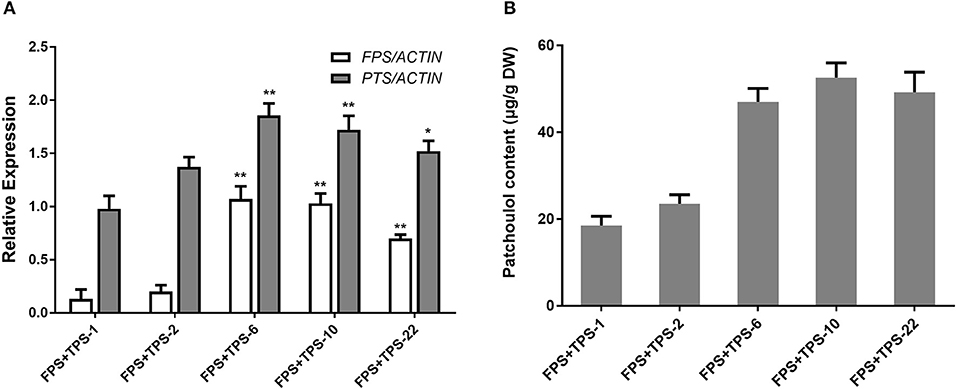
Figure 2. Engineering patchoulol biosynthesis in the cytoplasm of A. annua leaves. (A) Relative expression of FPS and PTS in FPS+PTS transgenic A. annua lines. (B) The patchoulol content in FPS+PTS transgenic A. annua lines. ACTIN was used as internal control. T0 transgenic lines were used for analysis. The error bars represent the means ± SD from three biological replicates. All data represent the means ± SD of three replicates. **P < 0.05, *P < 0.01, student's t-test.
Repressing the Artemisinin Biosynthetic Pathway Is Useful for Getting a High Yield of Patchoulol
Blocking the competitive biosynthetic pathways is an effective approach for increasing the sesquiterpene content. Former studies have reported, diverse array of sesquiterpene synthases in A. annua. For sesquiterpene biosynthesis in A. annua, FPSs convert the common precursor FPP into an array of cyclized products, such as amorpha-4,11-diene via the action of ADS (Bouwmeester et al., 1999), β-caryophyllene by β-caryophyllene synthase (CPS) (Cai et al., 2002), β-farnesene by β-farnesene synthase (BFS) (Picaud et al., 2005), germacrene A by germacrene A synthase (GAS) (Bertea et al., 2006) and epi-cedrol by epi-cedrol synthase (ECS) (Mercke et al., 1999) respectively. Artemisinin, an important sesquiterpene isolated from A. annua, is ~0.1–1% of the dry weight in this plant, and artemisinin biosynthesis occurs in the cytosol in A. annua (Wallaart et al., 2001; Abdin et al., 2003). We speculated that silencing ADS gene competing for FPS with TPS by RNAi technology would enhance the patchoulol content in transgenic lines. To assess whether blocking the artemisinin biosynthetic pathway could increase the yield of patchoulol, the FPS+ PTS and ADS-RNAi constructs were co-transformed into A. annua plants resulting in 18 independent transgenic lines for further analysis. In transgenic lines, ADSi +FPS+ PTS −1, −5, −12, −18, and −23 had a combination of high levels of FPS and PTS transcripts (Figure 3C), as well as low ADS transcript level (Figure 3A). Compared with the wild-type, the artemisinin content was reduced to 42–55% in transgenic lines (Figure 3B). The results from patchoulol content GC-MS analysis showed that FPS+PTS+ADSi lines produced 41.13–83.23 μg patchoulol/g DW (Figure 3D). Consistent with the hypothesis, blocking the competing pathway could be an applicable approach for getting higher yield of patchoulol.
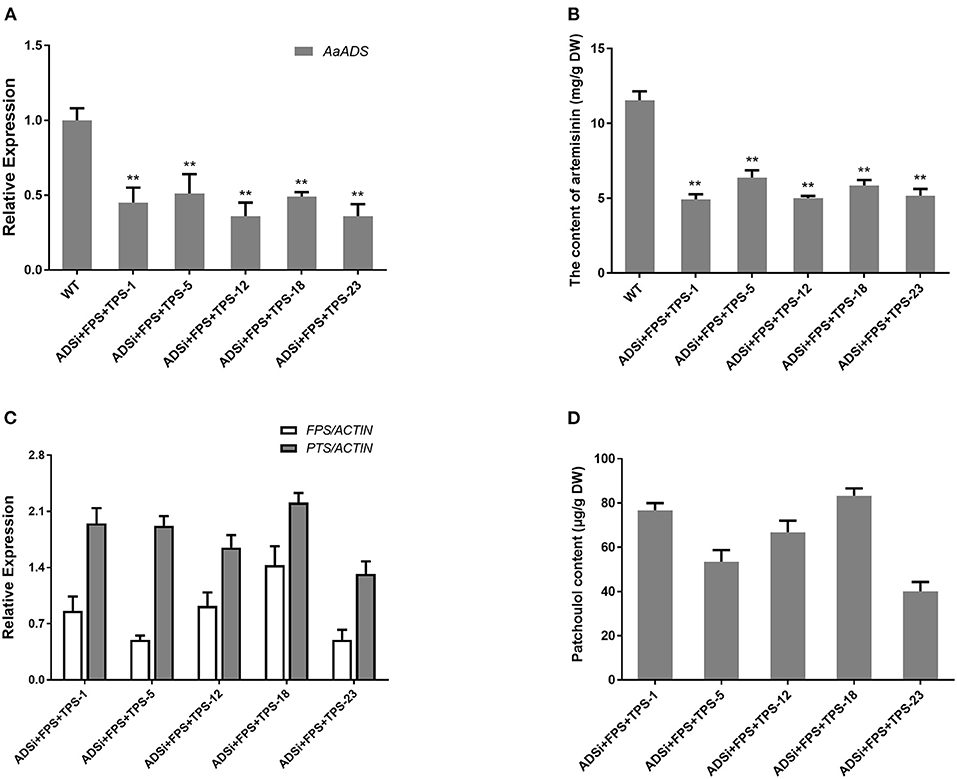
Figure 3. Blocking the artemisinin biosynthesis increased patchoulol content in ADSi+ FPS+PTS transgenic A. annua plants. (A) Relative expression of ADS in ADSi+ FPS+PTS transgenic A. annua lines. (B) The artemisinin content in ADSi+ FPS+PTS transgenic A. annua lines. (C) Relative expression of FPS and PTS in ADSi+ FPS+PTS transgenic A. annua lines. (D) The patchoulol content in ADSi+ FPS+PTS transgenic A. annua lines. T0 transgenic lines were used for analysis. ACTIN was used as internal control. The error bars represent the means ± SD from three biological replicates. All data represent the means ± SD of three replicates. **P < 0.05, *P < 0.01, student's t-test.
The Localization of Heterologous Proteins
Many efforts had been made to introduce the terpene synthases into the cellular compartments, in which the terpene is naturally synthesized, to compete for substrates or overcome prospective rate-limiting steps. For instance, tobacco was used to produce heterologous patchoulol, in which, both FPS and PTS were expressed in tobacco, and the final yield was about 0.3 μg/g FW (Wu et al., 2006). Furthermore, higher level of patchoulol (30 μg/g FW) was observed in transgenic tobacco when chloroplast-targeting signal sequence from the signal peptide of Arabidopsis RUBISCO small unit (tpFPS and tpPTS) was fused with the amino terminus of both FPS and PTS (Lee et al., 2006; Wu et al., 2006). To confirm the localization of FPS and PTS, the full-length of FPS and PTS were fused with GFP (Green Fluorescent Protein), respectively. The recombinant plasmids were transiently expressed in tobacco leaves. The results showed that both FPS-GFP and PTS-GFP fusion proteins were separated from the fluorescence of chloroplasts (Figures 4A,B). When the chloroplast-targeting signal sequence (TP) was targeted to the amino terminus of both FPS and PTS, tpFPS-GFP and tpPTS-GFP fusion proteins were completely matched the fluorescence of chloroplasts (Figures 4A,B).
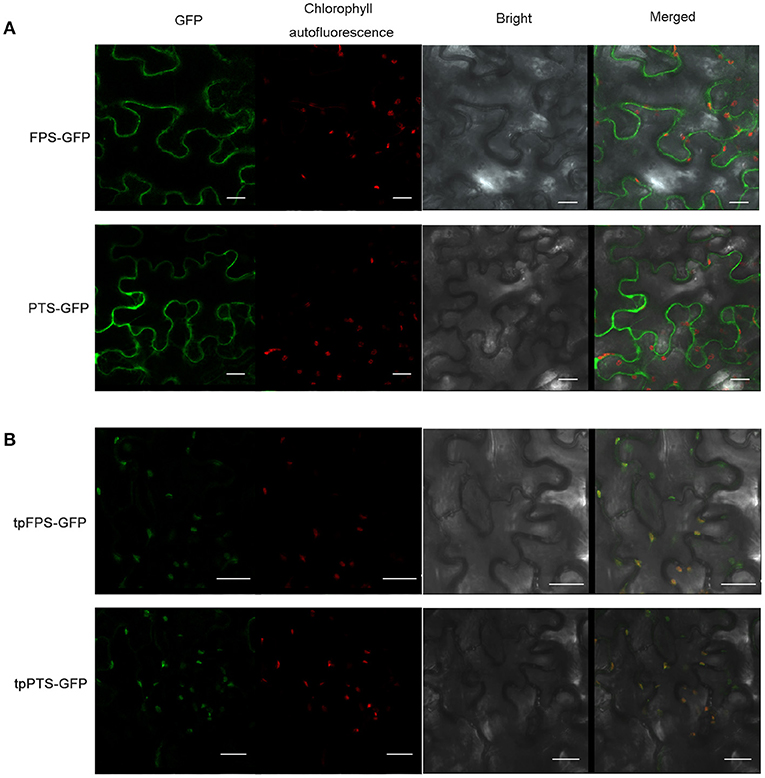
Figure 4. The subcellular localization of heterologous proteins. (A) Subcellular localization of FPS-GFP and PTS-GFP in tobacco leaf epidermal cells. (B) Subcellular localization of tpFPS-GFP and tpPTS-GFP in tobacco leaf epidermal cells. GFP: green fluorescent protein, Bars = 20 μm.
Engineering the Patchoulol Biosynthesis in the Chloroplast Compartment Enhanced the Accumulation of Patchoulol
When a particular terpene was produced in a certain compartment that this biosynthesis could not normally occur by diverting carbon flux at earlier intermediates, the high level of target product was obtained. For example, engineering of six genes encoding cytoplasmic MVA pathway, to chloroplast increased the levels of mevalonate, carotenoids, sterols, and squalene, suggesting the possible enhancement of overall terpene biosynthesis, despite of its organelles where it takes place (Kumar et al., 2011). Therefore, both FPS and PTS were further targeted into chloroplast via TP (tpFPS and tpPTS) (Lee et al., 2006) resulting in the more than 25 independent transgenic lines, which were further found to have higher expression levels of tpFPS and tpPTS (Figure 5A). The results from patchoulol content measurement by GC-MS revealed that, co-expression of tpFPS and tpTPS targeting the chloroplast compartment, could significantly enhance the patchoulol accumulation up to 273 μg/g DW (91 μg/g FW) (Figure 5B), which was 5–11-folds higher than those levels synthesized in the cytosol. The transgenic lines exhibited normal growth characteristics (Supplementary Figure 2).
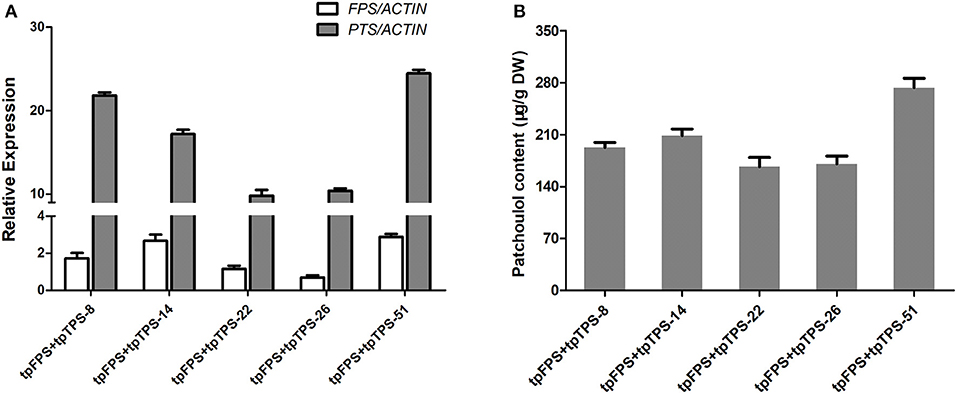
Figure 5. Engineering the patchoulol biosynthesis in the chloroplast compartment increased the patchoulol content in tpFPS+tpPTS transgenic A. annua plants. (A) Relative expression of FPS and PTS in tpFPS+tpPTS transgenic A. annua lines. (B) The patchoulol content in tpFPS+tpPTS transgenic A. annua lines. ACTIN was used as internal control. The error bars represent the means ± SD from three biological replicates.
Discussion
Recently great efforts have been made to engineer terpene metabolism in plant (Lewinsohn et al., 2001; Wu et al., 2006, 2012; Zhan et al., 2014). A. annua is a traditional Chinese medicinal plant and is famous for artemisinin. A stable and efficient Agrobacterium mediated transformation system of A. annua has been established. Besides, there are a large amount of trichomes (glandular trichomes and T-shaped trichomes) on the leaves in A. annua, where large quantities of terpenes are synthetized and stored to protect plants against insects, pathogens, and herbivores (Wagner, 1991; Duke and Paul, 1993; Pichersky and Gershenzon, 2002). Recently the biochemistry of trichomes has been studied in A. annua. Numerous information from former studies on trichome development, provides a great opportunity for engineering of terpene biosynthesis in the specific target cellular compartment.
The Advantage of Using A. annua as Platform for Synthetic Botany
In this work, we could apply and develop a novel approach and technique to engineer a cultivar of A. annua, with 273 μg/g DW patchoulol production with no any alteration of artemisinin biosynthesis. This strategy enables us to improve the economic value of medicinal plants. With the development of synthetic botany, many approaches have been made to produce valuable secondary metabolites. For instance, biosynthesis of β-carotene and anthocyanin in rice and the production of artemisinin in tobacco. However, due to the lack of specific storage cells in target plants, the heterogeneous biosynthesis of volatility chemicals, including mono- or sesquiterpene, still remain a big challenge in synthetic biology (Houshyani et al., 2013).
For instance, Wu et al. have constructed the patchoulol biosynthesis pathway in the tobacco plastid with the same strategy used in this study. However, the patchoulol content in the best-performing transgenic tobacco line was reported to be 30 μg/g FW, while the highest patchoulol content in transgenic A. annua in our study, reached to the level of 273 μg/g DW (91 μg/g FW) which could be possibly resulted from the possession of numerous glandular trichomes on the epidermal cells of A. annua leaves, where the accumulation and storage of artemisinin and lots of other mono- or sesquiterpene takes place. Furthermore, there is no significant difference in the artemisinin contents between patchoulol produced in transgenic and wild type A. annua (Supplementary Figure 3), suggesting the transgenic A. annua to be a reliable sources of anti-malaria agent, artemisinin and flavor component patchoulol production. Beside the above evidences, the establishment of an efficient Agrobacterium mediated transformation system in A. annu, gives a noticeable credit to it for being a worthy candidate in biosynthetic biology.
The Enzyme Targeted Cellular Compartment Is Crucial for Patchoulol Biosynthesis in A. annua
It has been well-studied that the terpenoids are biosynthesized from two independent compartmentally pathways: the MVA and MEP pathways. The MEP pathway, located in plastid, is dominantly responsible for the biosynthesis of mono- and diterpenes. The cytoplasm located MVA pathway is mainly responsible for the biosynthesis of sesquiterpenes. In A. annua, the artemisinin biosynthesis depends on cytoplasm directed MVA pathway (Newman and Chappell, 1999; Weathers et al., 2006). However, only about 0.001% dry weight of dihydroartemisinic alcohol (the artemisinin precursor) is reported to be produced in tobacco when expressing the artemisinin biosynthetic genes in the cytoplasm. On the contrast, the artemisinic acid accumulation reached to a maximum of about 0.004% when transferring the entire artemisinin biosynthetic genes into the chloroplast (Saxena et al., 2014). Wu et al. also reported that targeting the PTS to the plastid increased the patchoulol accumulation as well. In this study, targeting PTS into A. annua plastid produced higher patchoulol compared to its expression in cytoplasm. These results suggested that targeting or expressing the enzymes in plastid might be a powerful tool for synthetic botany.
Pathway Block Is a Useful Method for Improving Patchoulol Accumulation
Besides higher production and accumulation of patchoulol through targeting the PTS into A. annua plastid, we also investigated the influence of pathway blockage toward the patchoulol biosynthesis in cytoplasm. Obviously, the risk will be risen when the metabolic pathway is composed of more than two genes. For improving the patchoulol yield in cytoplasm, we blocked the artemisinin biosynthesis through RNA interference for ADS gene. The results showed that the patchoulol content had significantly increased in the ADSi lines affirming the positive role of pathway blockage in elevation of patchoulol biosynthesis. However, the patchoulol content in ADSi+PTS+FPS lines was still lower than that in transgenic plastid targeted PTS in A. annua, which could be the result of incomplete blockage of artemisinin biosynthesis by RNA interference. In addition, the presence of other competitive pathway along with artemisinin biosynthesis could lead to the deficiency of RNAi for higher production of patchoulol.
Materials and Methods
Plant Material and Growth Conditions
A. annua seeds originated from Chongqing province, were developed in Shanghai. A. annua plants were cultured in the greenhouse (16/8 h day/night, 25°C). Tobacco (Nicotiana benthamiana) was grown under the same conditions as A. annua.
Vectors Construction and the Transformation of A. annua
The patchoulol synthase gene (PTS) from P. cablin and farnesyl diphosphate synthase (FPS) from an avian were, respectively, inserted into the AscI/XhoI and SpeI/KpnI sites of the helper pTDUA vector. For the plastid-targeted expression, the transit peptide signal sequence of the RUBISCO protein in Arabidopsis (GenBank accession NM23202) was added to the 5' end of FPS and PTS, respectively. PTS driven by the cassava mosaic promoter and FPS driven by the 35S promoter were subsequently transferred to the pDONR vector through Gateway recombination reaction. The expression vectors were provided by Firmenich.
The 300 bp fragment of AaADS (GenBank accession AF138959) was cloned into the intermediate cloning vector pDONR, and transferred to the pHELLSGATE12 vector by LR recombination reaction (Invitrogen, Carlsbad, CA, USA). The information about the primers used are listed in Supplementary Table 1. The recombinant plasmids were transferred into Agrobacterium tumefaciens strain EHA105, then used to introduced into A. annua plants (Zhang et al., 2009). After 3–4 months, the regenerated plants were obtained.
Transcript Analysis of Terpene Synthase Genes
The first leaves were collected from 3 month-old A. annua plants for RNA extraction. Total RNA was extracted using the RNAprep Pure Plant Kit (Tiangen, Beijing, China). The first-strand cDNA for qRT-PCR was synthesized using the PrimeScript II first Strand cDNA Synthesis Kit (Takara, Shiga, Japan). RT-qPCR was performed using SuperReal PreMix Plus (Tiangen, Beijing, China). β-ACTIN was used as the reference gene. RT-qPCR was performed in a Roche LightCycler96 (Roche). According to the manufacturer's instructions, amplification was carried out using SYBR Green qPCR MasterMix (Takara, Shiga, Japan). The profile for SYBR Green qPCR was 95°C for 10 min, followed by 40 cycles of 95°C for 20 s, 55°C for 20 s, and 72°C for 20 s. The primers are represented in Supplementary Table 1. Three biological repeats were measured for each sample. The transcripts level was calculated using the 2−ΔCt method. ADS expression was analyzed using 2−ΔΔCt (Kilambi et al., 2013).
Subcellular Localization
To determine the subcellular localization of FPS and PTS proteins, the green fluorescent protein (GFP) was fused to the C-terminal domain of FPS, PTS, tpFPS, and tpPTS under the control of the CaMV35S promoter. The recombinant plasmids (pHB-FPS-GFP, pHB-PTS-GFP, pHB-tpFPS-GFP, and pHB-tpPTS-GFP) were, respectively, transferred into GV3101. The strains GV3101 harboring the recombinant plasmids were, respectively, co-transformed into N. benthamiana leaves with the strain containing p19 plasmid (Voinnet et al., 2003). GFP signals were observed by confocal laser microscopy (Leica TCS SP5-II) after 48 h incubation.
Quantification of Artemisinin by HPLC-ELSD
Quantification of artemisinin was performed as described previously (Zhang et al., 2009). Leaves from 3 month-old A. annua were collected, dried for 48 h at 50°C and pulverized into powder. 0.1 g dried-leaf powder was used for the ultrasonic extraction with 1 mL methanol for 30 min. Then the mixture was centrifuged at 12, 000 rpm for 5 min. The supernatants were filtered using filters (0.22 μm). The samples were analyzed by the HITACHI 2695 HPLC system coupled with a SANCO ELSD180 detector. The conditions were as follows: mobile phase, water/methanol (20:80, v/v); column, YMC-Pack ODS-A C18; flowrate, 1 ml/min. Artemisinin was set at 5.577 for artemisinin. The artemisinin standard was purchased from Sigma. Three biological repeats were measured for each sample.
Quantification of Patchoulol by GC-MS
Quantification of patchoulol was identified and quantified using gas chromatography and mass spectrometry (GC-MS). Five hundred milligram leaves were collected from 3 month-old A. annua and rapidly ground into powder in liquid nitrogen. The powder was used for the ultrasonic extraction with 3 ml ethyl acetate for 20 min. Then the samples were centrifuged at 5,000 g for 10 min. And the supernatants were filtered using filters (0.22 μm). Meanwhile, 500 mg leaves of A. annua were collected and dried at 60°C overnight. The weights were accurately measured for the calculation of the dry weight. Quantification was achieved based on the standard patchoulol (Aladdin, China). GC-MS analysis was performed on a GC-MS 7890/5975C (Agilent) according to the methods described previously (Zhan et al., 2014). Dodecane was used as the internal standards.
Data Availability Statement
The raw data supporting the conclusions of this article will be made available by the authors, without undue reservation.
Author Contributions
XF and KT designed the research. XF and FZ performed the experiments and wrote the first draft of the manuscript. YM, BP, QP, ZD, WL, JZhang, JZhao, and XS analyzed the data. XF, KT, and DH drafted the manuscript. YZ, DC, and LL revised the manuscript. All authors approved the manuscript.
Funding
This work was funded by National Key R&D Program of China (2018YFA0900600), the National Science Foundation of China (18Z103150043), and SJTU Trans-med Awards Research (20190104).
Conflict of Interest
YZ, ZD, WL, JZhang, LH, and DC were employed by company Firmenich Aromatics (China) Co. Ltd.
The remaining author declares that the research was conducted in the absence of any commercial or financial relationships that could be construed as a potential conflict of interest.
Acknowledgments
We thank Dr. Zhang Y. H. from Firmenich for providing the patchoulol and farnesyl diphosphate synthase genes and the pBDON vector. We also thank the Instrumental Analysis Center of the Shanghai Jiao Tong University for assistance with GC-MS.
Supplementary Material
The Supplementary Material for this article can be found online at: https://www.frontiersin.org/articles/10.3389/fbioe.2020.621127/full#supplementary-material
References
Abdin, M. Z., Israr, M., Rehman, R. U., and Jain, S. K. (2003). Artemisinin, a novel antimalarial drug: biochemical and molecular approaches for enhanced production. Planta Med. 69, 289–299. doi: 10.1055/s-2003-38871
Albertsen, L., Chen, Y., Bach, L. S., Rattleff, S., Maury, J., Brix, S., et al. (2011). Diversion of flux toward sesquiterpene production in Saccharomyces cerevisiae by fusion of host and heterologous enzymes. Appl. Environ. Microbiol. 77, 1033–1040. doi: 10.1128/AEM.01361-10
Balandrin, M. F., Klocke, J. A., Wurtele, E. S., and Bollinger, W. H. (1985). Natural plant chemicals: sources of industrial and medicinal materials. Science 228,1154–1160. doi: 10.1126/science.3890182
Bertea, C. M., Voster, A., Verstappen, F. W., Maffei, M., Beekwilder, J., and Bouwmeester, H. J. (2006). Isoprenoid biosynthesis in Artemisia annua: cloning and heterologous expression of a germacrene A synthase from a glandular trichome cDNA library. Arch. Biochem. Biophys. 448, 3–12. doi: 10.1016/j.abb.2006.02.026
Bohlmann, J., and Keeling, C. I. (2008). Terpenoid biomaterials. Plant J. 54, 656–669. doi: 10.1111/j.1365-313X.2008.03449.x
Bouwmeester, H. J., Wallaart, T. E., Janssen, M. H., van Loo, B., Jansen, B. J., Posthumus, M. A., et al. (1999). Amorpha-4, 11-diene synthase catalyses the first probable step in artemisinin biosynthesis. Phytochemistry 52, 843–854. doi: 10.1016/S0031-9422(99)00206-X
Cai, Y., Jia, J. W., Crock, J., Lin, Z. X., Chen, X. Y., and Croteau, R. (2002). A cDNA clone for β-caryophyllene synthase from Artemisia annua. Phytochemistry 61, 523–529. doi: 10.1016/S0031-9422(02)00265-0
Carter, O. A., Peters, R. J., and Croteau, R. (2003). Monoterpene biosynthesis pathway construction in Escherichia coli. Phytochemistry 64, 425–433. doi: 10.1016/S0031-9422(03)00204-8
Cheng, A. X., Lou, Y. G., Mao, Y. B., Lu, S., Wang, L. J., and Chen, X. Y. (2007). Plant terpenoids: biosynthesis and ecological functions. J. Integr. Plant Biol. 49,179–186. doi: 10.1111/j.1744-7909.2007.00395.x
Croteau, R. (2000). “Natural products (secondary metabolites),” in Biochemistry and Molecular Biology of Plants, eds B. B. Bob, G. Wilhelm, and L. J. Russell (New York, NY; Hoboken, NJ: Wiley), 1250–1381.
Daviet, L., and Schalk, M. (2010). Biotechnology in plant essential oil production: progress and perspective in metabolic engineering of the terpene pathway. Flavour Frag. J. 25,123–127. doi: 10.1002/ffj.1981
Deguerry, F., Pastore, L., Wu, S. Q., Clark, A., Chappell, J., and Schalk, M. (2006). The diverse sesquiterpene profile of patchouli, Pogostemon cablin, is correlated with a limited number of sesquiterpene synthases. Arch. Biochem. Biophys. 454,123–136. doi: 10.1016/j.abb.2006.08.006
Duke, S. O., and Paul, R. N. (1993). Development and fine structure of the glandular trichomes of Artemisia annua L. Int. J. Plant Sci. 154, 107–118.
Farhi, M., Marhevka, E., Ben-Ari, J., Algamas-Dimantov, A., Liang, Z., Zeevi, V., et al. (2011). Generation of the potent anti-malarial drug artemisinin in tobacco. Nat. Biotechnol. 29, 1072–1074. doi: 10.1038/nbt.2054
Gershenzon, J., and Dudareva, N. (2007). The function of terpene natural products in the natural world. Nat. Chem. Biol. 3, 408–414. doi: 10.1038/nchembio.2007.5
Hardie, J., and Minks, A. K. (1999). Pheromones of non-lepidopteran insects associated with agricultural plants. Austral. Entomol. 39, 97–100. doi: 10.2307/3496420
Houshyani, B., Assareh, M., Busquets, A., Ferrer, A., Bouwmeester, H. J., and Kappers, I. F. (2013). Three-step pathway engineering results in more incidence rate and higher emission of nerolidol and improved attraction of Diadegma semiclausum. Metab. Eng. 15, 88–97. doi: 10.1016/j.ymben.2012.10.002
Ito, H., Muranaka, T., Mori, K., Jin, Z. X., and Yoshida, T. (1997). Dryofragin and aspidin PB, piscicidal components from Dryopteris fragrans. Chem. Pharm. Bull. 45, 1720–1722. doi: 10.1248/cpb.45.1720
Jackson, B. E., Hartwells, E. A., and Matsuda, S. P. (2003). Metabolic engineering to produce sesquiterpenes in yeast. Org. Lett. 5,1629–1632. doi: 10.1021/ol034231x
Kappers, I. F., Dicke, M., and Bouwmeester, H. J. (2008). “Terpenoids in plant signaling, chemical ecology,” in Wiley Encyclopedia of Chemical Biology, eds T. P. Begley (New York, NY; Hoboken, NJ: Wiley, Inc.), 1–8.
Kilambi, H. V., Kumar, R., Sharma, R., and Sreelakshmi, Y. (2013). Chromoplast-specific carotenoid-associated protein appears to be important for enhanced accumulation of carotenoids in hp1 tomato fruits. Plant Physiol. 161:2085. doi: 10.1104/pp.112.212191
Kumar, S., Hahn, F. M., Baidoo, E., Kahlon, T. S., Wood, D. F., Mcmahan, C. M., et al. (2011). Remodeling the isoprenoid pathway in tobacco by expressing the cytoplasmic mevalonate pathway in chloroplasts. Metab. Eng. 14,19–28. doi: 10.1016/j.ymben.2011.11.005
Kunert, G., Otto, S., Röse, U. S. R., Gershenzon, J., and Weisser, W. W. (2005). Alarm pheromone mediates production of winged dispersal morphs in aphids. Ecol. Lett. 8, 596–603. doi: 10.1111/j.1461-0248.2005.00754.x
Lange, B. M., Rujan, T., Martin, W., and Croteau, R. (2000). Isoprenoid biosynthesis: the evolution of two ancient and distinct pathways across genomes. Proc. Natl. Acad. Sci. U.S.A. 97, 13172–13177. doi: 10.1073/pnas.240454797
Laurent, P., Braekman, J. C., Daloze, D., and Pasteels, J. (2003). Biosynthesis of defensive compounds from beetles and ants. Eur. J. Org. Chem. 2003, 2733–2743. doi: 10.1002/ejoc.200300008
Lee, D. W., Lee, S., Lee, G. J., Lee, K. H., Kim, S., Cheong, G. W., et al. (2006). Functional characterization of sequence motifs in the transit peptide of arabidopsis small subunit of Rubisco. Plant Physiol. 140, 466–483. doi: 10.1104/pp.105.074575
Lewinsohn, E., Schalechet, F., Wilkinson, J., Matsui, K., Tadmor, Y., Nam, K., et al. (2001). Enhanced levels of the aroma and flavor compound S-linalool by metabolic engineering of the terpenoid pathway in tomato fruits. Plant Physiol. 127,1256–1265. doi: 10.1104/pp.010293
Lorimer, S. D., Perry, N. B., Foster, L. M., and Burgess, E. J. (1996). A nematode larval motility inhibition assay for screening plant extracts and natural products. J. Agr. Food Chem. 44, 2842–2845. doi: 10.1021/jf9602176
Lunde, C. S., and Kubo, I. (2000). Effect of polygodial on the mitochondrial ATPase of Saccharomyces cerevisiae. Antimicrob. Agents Chemother. 44, 1943–1953. doi: 10.1128/aac.44.7.1943-1953.2000
Ma, B., Liu, M., Li, Z. H., Tao, X., and Wang, F. Q. (2019). Significantly enhanced production of patchoulol in metabolically engineered Saccharomyces cerevisiae. J. Agric. Food Chem. 67, 8590–8598. doi: 10.1021/acs.jafc.9b03456
Martin, V. J., Pitera, D., Withers, S. T., Newman, J. D., and Keasling, J. D. (2003). Engineering a mevalonate pathway in Escherichia coli for production of terpenoids. Nat. Biotechnol. 21, 796–802. doi: 10.1038/nbt833
Mercke, P., Crock, J., Croteau, R., and Brodelius, P. E. (1999). Cloning, expression, and characterization of epi-cedrol synthase, a sesquiterpene cyclase from Artemisia annua L. Arch. Biochem. Biophys. 369, 213–222. doi: 10.1006/abbi.1999.1358
Newman, J. D., and Chappell, J. (1999). Isoprenoid biosynthesis in plants: carbon partitioning within the cytoplasmic pathway. Crit. Rev. Biochem. Mol. 34, 95–106. doi: 10.1080/10409239991209228
Paddon, C. J., Westfall, P. J., Pitera, D. J., Benjamin, K., Fisher, K., Mcphee, D., et al. (2013). High-level semi-synthetic production of the potent antimalarial artemisinin. Nature 496, 528–532. doi: 10.1038/nature12051
Picaud, S., Brodelius, M., and Brodelius, P. E. (2005). Expression, purification and characterization of recombinant (E)-β-farnesene synthase from Artemisia annua. Phytochemistry 66, 961–967. doi: 10.1016/j.phytochem.2005.03.027
Pichersky, E., and Gershenzon, J. (2002). The formation and function of plant volatiles: perfumes for pollinator attraction and defense. Curr. Opin. Plant Biol. 5, 237–243. doi: 10.1016/S1369-5266(02)00251-0
Quintana, A., Reinhard, J., Faure, R., Uva, P., Bagneres, A., Massiot, G., et al. (2003). Interspecific variation in terpenoid composition of defensive secretions of European reticulitermes termites. J. Chem. Ecol. 29, 639–652. doi: 10.1023/A:1022868603108
Rastogi, N., Abaul, J., Goh, K. S., Devallois, A., Philogene, E., and Bourgeois, P. (1998). Antimycobacterial activity of chemically defined natural substances from the caribbean flora in guadeloupe. Fems. Immunol. Med. Mic. 20, 267–273. doi: 10.1111/j.1574-695X.1998.tb01136.x
Ro, D. K., Paradise, E. M., Ouellet, M., Fisher, K. J., Newman, K. L., Ndungu, J. M., et al. (2006). Production of the antimalarial drug precursor artemisinic acid in engineered yeast. Nature 440, 940–943. doi: 10.1038/nature04640
Rohmer, M., Seemann, M., Horbach, S., Bringer-Meyer, S., and Sahm, H. (1996). Glyceraldehyde 3-phosphate and pyruvate as precursors of isoprenic units in an alternative non-mevalonate pathway for terpenoid biosynthesis. J. Am. Chem. Soc. 118, 2564–2566. doi: 10.1021/ja9538344
Rohmer, M. (1999). “Isoprenoids including carotenoids and steroids,” in Comprehensive Natural Products Chemistry, ed D. E. Cane (Oxford), 2.
Ru, W. M., Zhang, J. T., Zhang, F., and Zhang, G. P. (2006). Eco-environmental characteristics and endangered causes of Taxus mairei, an endemic to China. Bull. Bot. Res. 26, 624–628.
Saxena, B., Subramaniyan, M., Malhotra, K., Bhavesh, N. S., Potlakayala, S. D., and Kumar, S. (2014). Metabolic engineering of chloroplasts for artemisinic acid biosynthesis and impact on plant growth. J. Biosci. 39, 33–41. doi: 10.1007/s12038-013-9402-z
Srikrishna, A., and Satyanarayana, G. (2005). An enantiospecific total synthesis of (–)-patchouli alcohol. Tetrahedron. Asymmetry 16, 3992–3997. doi: 10.1016/j.tetasy.2005.11.012
Tarshis, L. C., Yan, M. J., Poulter, C. D., and Sacchettini, J. C. (1994). Crystal-structure of recombinant farnesyl diphosphate synthase at 2.6-agnstrom resolution. Biochemistry 33, 10871–10877. doi: 10.1021/bi00202a004
Voinnet, O., Rivas, S., Mestre, P., and Baulcombe, D. (2003). An enhanced transient expression system in plants based on suppression of gene silencing by the p19 protein of tomato bushy stunt virus. Plant J. 33, 949–956. doi: 10.1046/j.1365-313X.2003.01676.xs
Wagner, G. J. (1991). Secreting glandular trichomes: more than just hairs. Plant Physiol. 96, 675–679. doi: 10.1104/pp.96.3.675
Wallaart, T. E., Bouwmeester, H. J., Hille, J., Poppinga, L., and Maijers, N. C. (2001). Amorpha-4, 11-diene synthase: cloning and functional expression of a key enzyme in the biosynthetic pathway of the novel antimalarial drug artemisinin. Planta 212, 460–465. doi: 10.1007/s004250000428
Wang, B., Kashkooli, A. B., Sallets, A., Ting, H. M., Ruijter, N. C. A. D., Olofsson, L., et al. (2016). Transient production of artemisinin in Nicotiana benthamiana is boosted by a specific lipid transfer protein from A. annua. Metab. Eng. 38,159–169. doi: 10.1016/j.ymben.2016.07.004
Weathers, P. J., Elkholy, S., and Wobbe, K. K. (2006). Artemisinin: the biosynthetic pathway and its regulation in Artemisia annua, a terpenoid-rich species. In Vitro Cell Dev. Biol. Plant 42, 309–317. doi: 10.1079/IVP2006782
Westfall, P. J., Pitera, D. J., Lenihan, J. R., Eng, D., Woolard, F. X., Regentin, R., et al. (2012). Production of amorphadiene in yeast, and its conversion to dihydroartemisinic acid, precursor to the antimalarial agent artemisinin. Proc. Natl. Acad. Sci. U.S.A. 109, E111–E118. doi: 10.1073/pnas.1110740109
Wu, S. Q., Jiang, Z., Chase, K., Nybo, S. E., Husodo, S., Williams, R., et al. (2012). Engineering triterpene metabolism in tobacco. Planta 236, 867–877. doi: 10.1007/s00425-012-1680-4
Wu, S. Q., Schalk, M., Clark, A., Miles, R. B., Coates, R., and Chappell, J. (2006). Redirection of cytosolic or plastidic isoprenoid precursors elevates terpene production in plants. Nat. Biotechnol. 24, 1441–1447. doi: 10.1038/nbt1251
Zhan, X., Zhang, Y. H., Chen, D. F., and Simonsen, H. T. (2014). Metabolic engineering of the moss Physcomitrella patens to produce the sesquiterpenoids patchoulol and α/β-santalene. Front. Plant Sci. 5:636. doi: 10.3389/fpls.2014.00636
Zhang, J. T., and Ru, W. M. (2010). Population characteristics of endangered species Taxus chinensis var. mairei and its conservation strategy in Shanxi, China. Popul. Ecol. 52, 407–416. doi: 10.1007/s10144-009-0192-y
Zhang, L., Jing, F. Y., Li, F., Li, M. Y., Wang, Y. Y., Wang, G. F., et al. (2009). Development of transgenic Artemisia annua (Chinese wormwood) plants with an enhanced content of artemisinin, an effective anti-malarial drug, by hairpin-RNA-mediated gene silencing. Biotechnol. Appl. Biochem. 52, 199–207. doi: 10.1042/BA20080068
Keywords: patchoulol, Artemisia annua L., synthetic biology, sesquiterpenoids, terpenes
Citation: Fu X, Zhang F, Ma Y, Hassani D, Peng B, Pan Q, Zhang Y, Deng Z, Liu W, Zhang J, Han L, Chen D, Zhao J, Li L, Sun X and Tang K (2021) High-Level Patchoulol Biosynthesis in Artemisia annua L. Front. Bioeng. Biotechnol. 8:621127. doi: 10.3389/fbioe.2020.621127
Received: 25 October 2020; Accepted: 30 December 2020;
Published: 04 February 2021.
Edited by:
Jingwen Zhou, Jiangnan University, ChinaReviewed by:
Xiaoya Chen, Institute of Plant Physiology and Ecology, Shanghai Institutes for Biological Sciences (CAS), ChinaSheng-Hong Li, Kunming Institute of Botany, China
Copyright © 2021 Fu, Zhang, Ma, Hassani, Peng, Pan, Zhang, Deng, Liu, Zhang, Han, Chen, Zhao, Li, Sun and Tang. This is an open-access article distributed under the terms of the Creative Commons Attribution License (CC BY). The use, distribution or reproduction in other forums is permitted, provided the original author(s) and the copyright owner(s) are credited and that the original publication in this journal is cited, in accordance with accepted academic practice. No use, distribution or reproduction is permitted which does not comply with these terms.
*Correspondence: Kexuan Tang, kxtang@sjtu.edu.cn; kxtang1@163.com
†These authors have contributed equally to this work