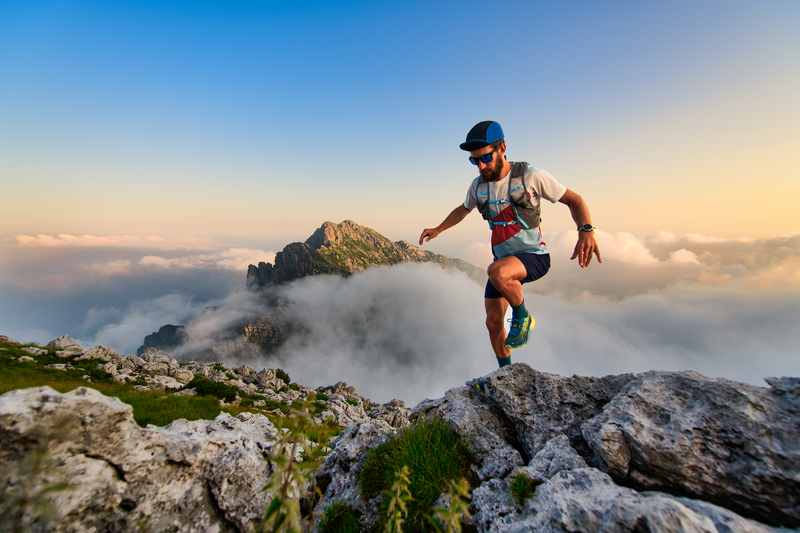
95% of researchers rate our articles as excellent or good
Learn more about the work of our research integrity team to safeguard the quality of each article we publish.
Find out more
REVIEW article
Front. Bioeng. Biotechnol. , 14 January 2021
Sec. Tissue Engineering and Regenerative Medicine
Volume 8 - 2020 | https://doi.org/10.3389/fbioe.2020.619980
This article is part of the Research Topic Perinatal Derivatives and the Road to Clinical Translation, Volume I View all 15 articles
The COVID-19 pandemic has become a priority in the health systems of all nations worldwide. In fact, there are currently no specific drugs or preventive treatments such as vaccines. The numerous therapies available today aim to counteract the symptoms caused by the viral infection that in some subjects can evolve causing acute respiratory distress syndromes (ARDS) with consequent admission to intensive care unit. The exacerbated response of the immune system, through cytokine storm, causes extensive damage to the lung tissue, with the formation of edema, fibrotic tissues and susceptibility to opportunistic infections. The inflammatory picture is also aggravated by disseminated intravascular coagulation which worsens the damage not only to the respiratory system, but also to other organs. In this context, perinatal cells represent a valid strategy thanks to their strong immunomodulatory potential, their safety profile, the ability to reduce fibrosis and stimulate reparative processes. Furthermore, perinatal cells exert antibacterial and antiviral actions. This review therefore provides an overview of the characteristics of perinatal cells with a particular focus on the beneficial effects that they could have in patients with COVID-19, and more specifically for their potential use in the treatment of ARDS and sepsis.
The Severe Acute Respiratory Syndrome CoronaVirus 2 (SARS-CoV-2) pandemic began in Wuhan, China, in December 2019, and rapidly spread worldwide. The disease caused by SARS-CoV-2 is called coronavirus disease 2019 (COVID-19) and by December 2020, the disease has reached more than 61 million cases, with over 1.4 million deaths and the numbers are constantly rising (https://COVID-19.who.int/).
Patients affected by COVID-19 infection report symptoms such as headache, fever, weakness, cough, general malaise, and shortness of breath (Chen et al., 2020). Although in many cases the disease does not lead to the development of symptoms, in around 5% of patients, especially the elderly, in the presence of co-morbidities like hypertension and diabetes, the disease can become critical, giving rise to acute respiratory distress syndrome (ARDS), pneumonia and multiple organ dysfunction requiring intensive care unit treatment (ICU) (Chen et al., 2020; Li et al., 2020). The mortality rate ranges between 1 and 4% (Guan et al., 2020; Rothan and Byrareddy, 2020), with the highest percentage in the aged and most critical patients >80 years old (Chen et al., 2020).
Many of the observed symptoms in ICU patients are attributable to a widespread and uncontrolled inflammatory response that gives rise to cytokine storm, as well as a disseminated intravascular coagulopathy (DIC) and multiorgan failure (Li et al., 2020).
As a matter of fact, screenings conducted on ICU patients showed that severe COVID-19 patients had high serum levels of inflammatory cytokines, such as tumor necrosis factor-alpha (TNF-α), interleukin-1 beta (IL-1β), IL-6, IL-17, interferon-gamma (IFNγ), granulocyte colony-stimulating factors (GM-CSF) and chemokine like the macrophage inflammatory proteins 1-α (MIP-1 α) (De Biasi et al., 2020; Huang et al., 2020; Liu J. et al., 2020).
Moreover, many patients also tend to develop a symptomatic framework that meets the diagnostic criteria for sepsis and septic shock according to the Sepsis-3 International Consensus (Singer et al., 2016). Importantly, following the inflammatory phase due to the dysregulated immune response, many patients develop lymphopenia, and thus are subject to other infections due to lowered immune response (Tan et al., 2020).
At the moment there is no specific treatment for SARS-CoV-2 infection and its consequences. Several clinical trials have been conducted using drugs targeting cytokines responsible for the observed adverse effects such as tocilizumab that targets IL-6 (Guaraldi et al., 2020; Stone et al., 2020), or anakinra to counteract the effects related to IL-1β (NCT04443881, NCT04603742, Huet et al., 2020). Other antiviral drugs such as Remdesivir are still present in therapeutic protocols today, finding an application in limiting viral replication (Beigel et al., 2020).
Mesenchymal stromal cells (MSCs) represent a possible alternative treatment for COVID-19 aimed at counteracting the damage and organ failure due to inflammatory and fibrotic processes induced by SARS-CoV2, as well as responding to many of the symptoms induced by the viral infection. In fact, MSCs have been studied for years in regenerative medicine for the immunomodulatory properties that make them suitable for the treatment of many chronic or degenerative diseases, where the immune response is dysregulated (Shi et al., 2018; Koliaraki et al., 2020). Furthermore, these cells are also characterized by antimicrobial properties (Alcayaga-Miranda et al., 2017) and reduced expression of HLA-II strongly reducing the risk of allograft rejection (Ryan et al., 2005).
Initially isolated from the bone marrow over the years they have been isolated from different tissues such as adipose tissue, dental pulp, and perinatal tissues (Silini et al., 2020). In particular, in this review we will focus our attention on the potential application of perinatal derived cells (PDCs) in the treatment of COVID-19 with a focus on their anti-inflammatory and antifibrotic properties, aimed at counteracting ARDS, and on their antimicrobic and antiviral properties.
The use of cell therapy has attracted much attention in the scenario of regenerative medicine due to its potential therapeutic benefits. Indeed, living cells provide multifaceted mechanisms of action that can tackle a broad spectrum of deregulated physiological processes especially in degenerative and inflammatory diseases.
One of the most intriguing sources for cell therapy that has attracted much attention in the past decade is perinatal tissues. The term “perinatal” refers to birth-associated tissues that are obtained from term placentas (including chorionic villi, chorionic plate) and fetal annexes (that include amniotic and chorionic membrane, amniotic fluid, and umbilical cord). Indeed, perinatal tissues have proven to be precious reservoirs of cells (In 't Anker et al., 2004; Wang et al., 2004; De Coppi et al., 2007; Parolini et al., 2008; Abumaree et al., 2013).
Initially, perinatal tissues drew attention as an interesting cell source due to their early embryological origin suggesting that perinatal cells could possess unique plasticity and differentiation properties (Parolini and Soncini, 2006). In addition, when compared to other cell sources such as bone marrow, practical and logistical reasons made the placenta an attractive cell source. It is easily obtained after birth without invasive procedures, and it is considered biological waste thus bypassing ethical tensions associated to other cell sources.
Nowadays, perinatal-derived cells (PDCs) have obtained significant recognition for their promising applications in regenerative medicine (Silini et al., 2015). As a matter of fact, during this past decade the literature published on PDCs has grown exponentially, substantiating the interest in using cells from these tissues and, most importantly, demonstrating that indeed PDCs are important contenders with stromal cells from other tissues, such as bone marrow- or adipose-derived mesenchymal stromal cells (MSCs). Below we will briefly discuss why perinatal cells represent valid alternatives.
First, there is strong evidence that PDCs from both maternal and fetal tissues do not induce an immune response in vitro (Bailo et al., 2004; Wolbank et al., 2007; Banas et al., 2008; Magatti et al., 2008; Weiss et al., 2008; Prasanna et al., 2010; Tipnis et al., 2010; Papait et al., 2020), making them attractive for allogeneic transplantation. Second, in order to become fully immunosuppressive, several studies indicate that MSCs from bone marrow require “licensing” with inflammatory stimuli such as IFNγ and TNFα (Krampera et al., 2006; Ren et al., 2008; Sheng et al., 2008; Mougiakakos et al., 2011; Shi et al., 2012). Accordingly, bone marrow MSCs cultured in transwell, and the secretome from bone marrow MSCs, are not able to exert suppressive effects if they are not previously exposed to inflammatory stimuli (Krampera et al., 2003, 2006; Groh et al., 2005). In the case of PDCs, “licensing” does not seem to be mandatory for their suppressive effects (Magatti et al., 2008; Rossi et al., 2012; Lange-Consiglio et al., 2020; Papait et al., 2020) although stimulation of PDCs with pro-inflammatory cytokines has been shown to increase secretome potency (Allen et al., 2018). In line with this, the secretome from unstimulated PDCs exerts significant immunomodulatory effects in vitro (Rossi et al., 2012; Pianta et al., 2015; Magatti et al., 2016; Papait et al., 2020) and therapeutic effects in vivo in diseases with a deregulated inflammatory response (Cargnoni et al., 2012, 2014; Roy et al., 2013; Danieli et al., 2015; Chatterjee et al., 2016; Magatti et al., 2016; Van Linthout et al., 2017; Giampa et al., 2019), altogether suggesting that these cells constitutively secrete bioactive factors that promote regeneration.
Third, PDCs and their secretome have robust therapeutic properties when transplanted in animal models of different pathological conditions, such as inflammatory disorders, autoimmune diseases, neurodegenerative diseases, ischemia/reperfusion injuries, diabetes, and liver and lung fibrosis. The controversial ability of PDCs to differentiate in vivo, as well as the low survival and engraftment in host tissues after transplantation, suggest that they do not act via stemness properties to replace injured tissue but rather act via paracrine mechanisms on tissue resident cells or on immune cells located in the injured tissue. PDCs can create a reparative environment through cell-to-cell contact and the secretion of growth factors, cytokines, and the release of extracellular vesicles (containing peptides/proteins, mRNA, and microRNA). These in turn can suppress the activation/function of inflammatory cells; prevent apoptosis and promote the survival of damaged tissue cells, reduce oxidative injury often involved in tissue damage, and favor angiogenesis.
The immunomodulatory activity of PDCs includes inhibition of immune cell (e.g., T and B lymphocyte) proliferation (Magatti et al., 2008, 2020; Weiss et al., 2008; Najar et al., 2010a; Prasanna et al., 2010; Raicevic et al., 2011; Zhou et al., 2011; Roy et al., 2013; Li et al., 2014; Wang et al., 2014; Pianta et al., 2016; Cargnoni et al., 2020; Papait et al., 2020), inhibition of the maturation toward effector immune cells (cells which actively respond to an immunogenic stimuli) (Magatti et al., 2009; Pianta et al., 2016), expansion of regulatory immune cells that can suppress the immune response (Chang et al., 2006; Najar et al., 2010b; Parolini et al., 2014; Amari et al., 2015; Pianta et al., 2015, 2016; Papait et al., 2020), inhibition of the secretion of pro-inflammatory cytokines and chemokines (IL-1β, IL-2, TNF -α, and IFN-γ) (Kronsteiner et al., 2011; Kang et al., 2012; Karlsson et al., 2012; Parolini et al., 2014; Pianta et al., 2015), involved in the aggravation of the inflammatory response, and promotion of the production of anti-inflammatory factors (IL-10, TGF-β), (Pianta et al., 2015; Papait et al., 2020).
The promising findings obtained from preclinical studies, along with the high availability, excellent safety profile, lack of ethical challenges, and low immunogenicity, provide a strong rationale for investigating perinatal cells as a potential innovative therapy in humans.
Over the past decade a large number of clinical trials have emerged worldwide using cell-based therapies to treat various diseases (clinicaltrials.gov), including neurological disorders, muscle repair, cardiovascular diseases, immune related diseases, and lung diseases. Among these studies the use of PDCs is rapidly rising with only 1% of the studies using perinatal tissues as a source of cells, between the years 1994 and 2009, to almost 30% in 2018 (Verter et al., 2018; Moll et al., 2019).
According to a large analysis of 914 MSC trials reported through 2018 (Kabat et al., 2020), the three most common conditions for MSC trials are neurological, joint, and cardiovascular diseases which account for 42% of all trials. Pulmonary conditions account for only about 6% of all trials (Marquez-Curtis et al., 2015; Kabat et al., 2020). However, since the emergence of the novel coronavirus disease (COVID-19), that features pathological changes that are similar to acute lung injury with serious pulmonary inflammation and inflammatory cytokine storm, numerous clinical trials with MSCs as a new treatment for COVID-19 were registered (Liu S. et al., 2020). Indeed, as of July 2020 there were 83 clinical trials (clinicaltrials.gov) using MSCs for various immune/inflammatory pulmonary conditions (Figure 1A) and 57% of these (47 out of 83) were specifically for COVID-19. With respect to the source of the MSCs, perinatal tissues account for 40% of all pulmonary studies and 49% of the COVID-19 trials (Figure 1B). Results obtained from clinical trials which transplanted PDCs intravenously (IV) in patients with lung fibrosis have shown that these cells are safe with null/mild side effects. Specifically, in a phase 1 clinical trial, PDCs (PDA-001) infused in four patients with refractory pulmonary sarcoidosis produced a transient and mild increase in mean pulmonary artery pressure. In the year following treatment two of the patients showed improvement in their chest x-ray and had prednisone withdrawn (Baughman et al., 2015). Another phase 1b study using placental stromal cells to treat eight patients with mild to moderate idiopathic pulmonary fibrosis (IPF) demonstrated that 6 months after placental MSC infusion, lung function and CT fibrosis scores were stable from baseline, proving feasibility and short-term safety of placental MSCs (Chambers et al., 2014). Patients tolerated cell administration with only a transient fall of oxygen saturation but no changes in hemodynamics showing satisfactory short-term safety profile in IPF patients (Chambers et al., 2014).
Figure 1. Clinical trials using cell-based therapy for pulmonary disorders. (A) Clinical trials as reported on clinicaltrials.gov (as of July 1, 2020) with stromal cells for pulmonary conditions. Colors represent the different cells used in the studies. ARDS, acute respiratory distress syndrome; IPF, idiopathic pulmonary fibrosis; ILD, interstitial lung disease; COPD, chronic obstructive pulmonary disease. (B) Percentage of MSCs from different tissues used in COVID-19 clinical trials.
In addition, a case report at the Siping Hospital of China Medical University described that, at the end of 12 month follow-up, a 56-year-old patient with IPF that was IV-injected with human umbilical cord MSCs showed a relevant reduction of oxygen therapy requirement, an improvement of physical performance and respiratory parameters (lung function and CT scores). No serious or clinically relevant side effects were observed during the entire study period (Zhang et al., 2017). Finally, a completed phase 1 clinical trial (NCT02277145), without published results, investigated the effects of clinical grade umbilical cord MSCs, injected directly into lung lesion by fiberoptic bronchoscopy in patients with radiation-induced pulmonary fibrosis. Six months from injection, the safety and effectiveness of the treatment will be evaluated through CT density histogram and the patients' self-evaluation.
Numerous clinical trials are currently evaluating the efficacy and safety of PDCs applied as treatment for acute respiratory distress syndrome (ARDS) induced by COVID-19 infection. Many countries promoted these studies including China, US, Europe (France, Spain, UK, Ukraine), and South America (Colombia). These studies, registered at www.clinicaltrials.gov, combine standard of care with the infusion of human umbilical cord or Wharton's jelly derived MSCs (hUC-MSCs and hWJ-MSCs, respectively), or with the intramuscular (IM) injection of placental expanded adherent stromal cells (NCT04614025, NCT04389450) to evaluate the ability of cell therapies to potentiate conventional treatment efficacy for COVID19. Out of these studies one study will use placenta-derived multipotent mesenchymal stromal cells (NCT04461925) and another, the REALIST study, will apply a selected cell population and specifically CD362 enriched hUC-MSCs to treat patients with moderate to severe ARDS (NCT03042143).
Most of these studies plan to implement multiple cell infusions with cell doses ranging from 1 × 106 cells/kg (NCT04313322, NCT04429763, NCT03608592, NCT04339660, NCT04457609, NCT04461925, NCT04390139, NCT04333368), to 5 × 106 cells/kg (NCT04273646) or independent from body weight, doses ranging from 4 to 5 × 107 cells (NCT04288102, NCT04390152) and up to 1–6 × 108 cells (NCT04456361, NCT04355728, NCT04389450). One of these studies is a Phase 1/2a dose-escalating study (NCT04452097) assessing the safety and the maximum tolerated dose of IV infused hUC-MSCs. In the phase 1 three doses will be infused 0.5 × 106, 1.0 × 106, or 1.5 × 106 cells/kg/body weight with three patients/group; at day 3 the incidence of infusion-related adverse events will be evaluated and at day 28 the incidence of any treatment-emergent serious adverse events. In the phase 2a, a total of 30 subjects will be randomized into the treatment and control groups.
Almost all studies are aimed to assess the efficacy of cell therapy by evaluating intergroup (cell treated group vs. placebo group) differences for clinical parameters such as mortality rate, length of hospitalization, oxygenation index (PaO2/FiO2); one of these studies (NCT04339660) has the primary endpoint to assess the improvement of inflammatory parameters/factors such as plasma levels of TNF-α, IL-1β, IL-6, TGF-β, IL-8 and C-reactive protein (CRP).
Although most are Phase I or Phase I/II clinical trials, few of these (NCT04269525, NCT03042143, NCT04288102, NCT04429763, NCT04389450, NCT04614025) are Phase II trials that will collect preliminary data on cell therapy efficacy, in addition to data about safety and short-term adverse events.
In general, however, all these studies will involve a small numbers of patients, from a few participants (five to nine patients) to a maximum of 140 (NCT04389450), to have conclusive indications on PDCs' safety and efficacy in COVID-19 patients, cells will be administered in large, randomized, controlled, double-blinded trials with long-term follow-up.
Considering these important limitations, it cannot be ignored that some published reports of early phase studies performed in China show promising results from PDC therapy in severe cases of COVID-19. In a Phase I study (Meng et al., 2020), 18 patients with moderate-severe COVID-19 were enrolled and received standard COVID-treatment regimens, nine of them (the treated group), also received three IV infusions of hUC-MSCs (1 × 106cells/each infusion) on days 0, 3, and 6. Two patients from the treated group suffered from transient facial flushing and fever, and another from transient hypoxia at 12 h post infusion. A reduced number of patients in treated group with respect to control group (1 vs. 4), required mechanical ventilation and experienced dyspnea (1 treated vs. 5 controls). Furthermore, hUC-MSC-treated patients showed a trend in reduction of serum IL-6. On the basis of these outcomes, the authors started a Phase 2 randomized placebo-controlled trial which will recruit 90 patients with severe COVID-19 (see NCT04288102).
From January to April 2020, another clinical study enrolled 31 patients with severe COVID-19, all treated with one to three doses of hUC-MSCs (1 × 106cells/kg each dose) suspended in 100 ml saline (Guo et al., 2020). The authors observed no adverse events attributable to IV cell infusion. Four patients died, 27 were discharged with amelioration of clinical and hematologic parameters, but lack of the control group did not allow conclusions to be drawn on potential treatment efficacy.
Another study, published by Shu et al. (2020), enrolled 41 patients with severe COVID-19 who did not respond to a 7- to 10-day standard treatment. All patients received conventional treatment (ventilation, antiviral agents, antibiotic agents if needed, glucocorticoid therapy) and, in addition, a group of these patients (n = 12), received one IV infusion of hUC-MSCs (2 × 106cells/kg in 100 ml saline). During 2 week-period of observation, patients who received hUC-MSCs, had no adverse reactions. In comparison with control group (n = 29), treated patients showed a shorter time to clinical improvement (9.0 vs. 14.0 days, p = 0.006), a higher percentage with significant remission of dyspnea and absorption of imaging (91.67 vs. 51.72%), a better oxygenation index, and a significant amelioration of CT scores, ground-glass opacity and consolidation, paralleled with reduced plasmatic levels of CRP and IL-6; all are parameters that indicate reduced lung inflammation. The authors speculate that hUC-MSCs can reduce lung inflammation by reducing the release of inflammatory cytokines through an immunomodulatory action.
In most of the completed and ongoing studies, COVID-19 patients received/will receive PDCs through IV infusion, however it is not yet clear whether the IV route is the best choice. COVID-19 can lead to a form of disseminated intravascular coagulation (DIC) and many of the critically ill COVID-19 patients with poor prognosis are in a systemic procoagulant state (Arachchillage and Laffan, 2020; Connors and Levy, 2020; Klok et al., 2020; Magro et al., 2020; Oxley et al., 2020; Spyropoulos et al., 2020; Tang et al., 2020; Wang T. et al., 2020; Zhang et al., 2020; Zhou et al., 2020), and MSC products are known to express variable levels of a highly procoagulant tissue factor (TF/CD142) (Morrissey, 2004). Therefore, alternative routes of cell administration such as the IM injection are increasingly explored. The IM route holds great advantages over other administration routes, such as the possibility to administer a higher number of cells and thus potentially increase efficacy (Braid et al., 2018; Caplan et al., 2019), and the vascularized muscle support provides a channel for systemic release of paracrine mediators. In addition, the large muscle tissue allows for multiple injection sites (Caplan et al., 2019; Hamidian Jahromi and Davies, 2019). IM delivery has been shown to be safe (reviewed in Caplan et al., 2019; Hamidian Jahromi and Davies, 2019) in several clinical studies (Winkler et al., 2018; Norgren et al., 2019) with placenta-derived mesenchymal-like cells [PLacental eXpanded (PLX)-PAD] and is now being tested in a double-blind, multicenter study to evaluate the efficacy of PLX-PAD for the treatment of COVID-19 (NCT04389450). PLX-PAD cells are adherent stromal cells isolated from the placenta of healthy women following a cesarean section. While PLX-PAD cells express membrane markers typical of classical MSCs, they have a minimal ability to differentiate in vitro into cells of the mesodermal lineage and are thus termed MSC-like cells. In addition to the previously mentioned phase II study, PLX-PAD are also being used (as of July 2020) to treat 20 COVID-19 patients in Israel under emergency/compassionate use, and in the USA under Single Patient IND for Compassionate or Emergency Use. Patients received either one or two treatments of 300 million cells administered via IM injections. Data published on the first eight patients describe an overall good safety profile and clinical improvement in several parameters such as CRP, PEEP, and P/F following PLX-PAD treatment. Specifically, levels of CRP in all patients were elevated prior to PLX-PAD treatment with levels ranged from 82 to 394 mg/L at time of treatment. Starting as early as 24 h post treatment, CRP levels decreased and have decreased by 45 and 77% by day 3 and by day 5, respectively (Barkama et al., 2020).
Recent evidence has suggested a link between lung fibrosis and the SARS-CoV-2 virus infection. Indeed, COVID-19 patients experience a large spectrum of pulmonary fibrotic diseases from fibrosis associated with pneumonia to major, widespread fibrotic changes of the lung (George et al., 2020). A longitudinal study conducted by Wang Y. et al. (2020) on computed tomography (CT) manifestations during the course of COVID-19 pneumonia reported that, in the early stage of disease (first 11 days), ~85% of enrolled patients showed pulmonary ground-glass opacity and tissue consolidation. Later, between days 12 and 17, evolving from ground-glass opacity, a mixed pattern with lung architectural distortion appeared, characterized by perilobular abnormalities, suggesting the presence of secondary organizing pneumonia. Given that organizing pneumonia possibly evolves to fibrosis with progressive and permanent lung damage, this may indicate that some patients, although negative for COVID-19 at their discharge, can develop progressive, fibrotic irreversible interstitial lung disease (Figure 2A) (George et al., 2020; Wang Y. et al., 2020).
Figure 2. Etiopathology of the Sars-CoV2 viral infection and therapeutic effect of PDCs injection. (A) Sars-CoV2 is responsible for acute respiratory distress syndrome (ARDS) resulting in thousands of patients requiring intensive care unit (ICU) treatment. Importantly, the virus responsible of trigger an exacerbated immune response, resulting in a cytokine storm, and consequently to the damage of the epithelial airway, followed by lung edema, dysfunction of air exchange and the formation of interstitial fibrosis, thus creating an environment conducive to opportunistic bacterial infections or superinfection. Furthermore, COVID-19 is characterized also by the formation of vascular microthrombi that are often identified in areas of diffuse alveolar damage and are associated with diffuse endothelial damage. (B) PDCs treatment can directly affect the immune response through the release of immunomodulatory cytokines, such as IL-10, or anti-inflammatory molecules such as Prostaglandin (PG) E2, thus immunomodulating the exacerbated inflammatory response. Furthermore, MSCs can exert beneficial properties through the release of anti-microbial peptides such as LL-37. Moreover, PDCs can degrade or inhibit ARDS-induced fibrotic tissue by remodeling the extracellular matrix through the release of metalloprotease and TIMP, and modulation of the immune response can consequently reduce the formation of microthrombi affecting the alveolar capillaries. Created with Biorender.com.
Furthermore, early analysis from patients with COVID-19 at hospital discharge suggests a high rate of lung fibrotic abnormalities (George et al., 2020). However, the relation between the presence of fibrotic lesions and prognosis is under debate. Some researchers indeed suggest that the presence of fibrosis indicates a resolution status of the disease and a good prognosis (Pan et al., 2020). Others instead suggest that fibrosis may progress resulting in pulmonary interstitial fibrosis disease indicating a poor prognosis (Kong and Agarwal, 2020). Similar fibrotic lesions were previously observed in patients surviving from respiratory diseases caused by coronaviruses (SARS-CoV and MERS-CoV) similar to the SARS-CoV-2 virus responsible of COVID-19. Long-term studies indicated that a third of SARS patients who recovered from severe infection were left with permanent lung damage (Cox, 2020).
Similarly, long-term studies will be required to establish the real prevalence of post-COVID-19 fibrosis. However, due to pandemic nature of COVID-19, some researchers underline the prompt need to identify early biomarkers in the disease course to identify patients who potentially progress to pulmonary fibrosis (George et al., 2020; Spagnolo et al., 2020), and suggest that the delivery of antifibrotic therapy, combined with anti-inflammatory treatment to mitigate the cytokine storm that seems to be responsible of severe ARDS thought to trigger the development of lung fibrosis, might have a role in preventing fibrosis progression after SARS-CoV-2 infection.
The development of anti-fibrotic drugs able to restore lung function in IPF patients remains an open challenge. Current antifibrotic therapy applied to patients with IPF, mainly represented by nintedanib and pirfenidone, can slow the progression of the disease and improve the rate of mortality, however they do not reverse the fibrotic process, and the only approach to effectively resolve pulmonary damage remains lung transplantation (Chase and Gallicchio, 2019). Therefore, there is a need for new therapeutics with anti-fibrotic effects on the injured lungs.
PDCs are widely considered as possible treatment for inflammatory-based fibrotic diseases. These cells, and their derivatives such as cell-derived exosomes and soluble factors, possess anti-inflammatory, anti-fibrotic, anti-apoptotic, and regenerative properties which altogether may synergistically contribute to stop and reverse fibrotic disease.
The ability of PDCs and their derivatives to slow and/or reverse pulmonary fibrosis has been mostly investigated in mouse models of bleomycin-induced lung fibrosis that represents the most used to study pulmonary fibrosis and to test possible anti-fibrotic drugs (Peng et al., 2013). In this model, bleomycin induces oxidative stress specifically to alveolar epithelial cells leading to acute alveolar injury, and a consequent inflammatory response with immune cell infiltration in lung parenchyma and secretion of inflammatory and pro-fibrotic cytokines. Lung inflammation triggers the fibrotic response characterized by fibroblast activation to myofibroblasts, cells with high proliferation and responsible of excessive production of extracellular matrix that disrupts the normal lung architecture with loss of respiratory capacity (Peng et al., 2013). Given the involvement of an inflammatory trigger, it has been hypothesized that therapeutic approaches with a positive outcome in the bleomycin model might be beneficial also in COVID-19, both during the acute inflammatory phase and in preventing long-term fibrotic complications (George et al., 2020).
PDCs, including those from amniotic membrane, Wharton's jelly, and related derivatives, including cell secretome and secretome-derived exosomes, have been administered in animals with pulmonary fibrosis to evaluate their ability to prevent disease (delivery concomitant to fibrosis induction) (Cargnoni et al., 2009; Moodley et al., 2010; Murphy et al., 2011) and to evaluate their potential to cure the disease (delivery after fibrosis establishment) (Moodley et al., 2013; Vosdoganes et al., 2013).
hAEC and their derivatives are the most studied perinatal cells in experimental models of lung fibrosis. The antifibrotic effect of these cells have been investigated when delivered within 24 h from fibrosis induction by bleomycin (Moodley et al., 2010; Murphy et al., 2011) and when delivered at days 7 or 14 post bleomycin induction when lung injury was established (Moodley et al., 2013; Vosdoganes et al., 2013; Tan et al., 2018). However, globally these studies suggest that hAEC can not only slow down fibrosis progression, but also partly reverse the established fibrotic lesion. Furthermore, the lower levels of pro-inflammatory and pro-fibrotic cytokines (MCP-1, TNF-α, IL-1, IL-6, TGF-β), suggest that hAEC may exert their reparative effects by modulating the host inflammatory response.
Other studies demonstrate the ability of hAEC to interfere with specific immune cells involved in the bleomycin-induced inflammatory response. Murphy et al. (2012) observed that the therapeutic effect of hAEC depends upon the presence of functional macrophages and Tan and collaborators (Tan et al., 2015) demonstrated that hAEC treatment promotes the switch from M1 (pro-inflammatory activity), to M2 macrophages (associated with fibrosis resolution) characterized by lipoxin A4-dependent upregulated phagocytosis (Tan et al., 2017). The same group showed that hAEC can partly counteract T cell-mediated inflammation by increasing pulmonary levels of regulatory T cells (Treg) (Tan et al., 2015). Furthermore, they found that exosomes from hAEC, when delivered early after bleomycin-challenge, exerted an anti-inflammatory effect on macrophages and neutrophils, while a delayed delivery (7 days after bleomycin) was able to improve tissue-to-airspace ratio and reduce fibrosis (Tan et al., 2018), indicating that a cell-free treatment derived from hAEC (i.e., exosomes) can counteract both the pulmonary inflammatory phase and the following fibrotic phase induced by bleomycin challenge.
In a model of pulmonary fibrosis induced by a dual bleomycin doses to better reflect the repeated injuries that underlie most lung diseases, Moodley et al. (2013) compared the antifibrotic potency of hAMSC, hAEC, and BM-MSC. All reduced infiltration of inflammatory cells in lung parenchyma and reduced lung levels of the profibrotic cytokine TGF-β. Interestingly, only hAMSC effectively reduced collagen deposition. In another study, hAMSC mixed with hAEC and human chorionic MSCs reduced the severity and extension of lung fibrosis and decreased neutrophil infiltration when delivered 15 min after bleomycin challenge (Cargnoni et al., 2009). Interestingly similar effects, together with improved blood gas parameters, were found when the hAMSC secretome was used (Cargnoni et al., 2012, 2014), indicating that hAMSC secrete active mediators crucial for tissue repair. More recently, hAMSC were found able to reduce collagen deposition, T cell lung infiltration, and inflammatory cytokine expressions also in rats with paraquat-induced lung fibrosis (He et al., 2020). Another recent study reported that administration of human placental MSC of fetal origin (hfPMSC), isolated from an unspecified region of placenta, in mice with bleomycin-induced lung fibrosis reduced collagen deposition and the production of pro-fibrotic cytokines by attenuating the dysregulation of MyD88/TGF-β signaling axis that is considered involved in the pathogenesis of pulmonary fibrosis in mice (Li et al., 2017). Finally, a very recent study demonstrates that hAMSC delivery in bleomycin-challenged mice reduces pulmonary B cell recruitment, retention and maturation, and counteract the formation and expansion of intrapulmonary lymphoid aggregates, thus dampening chronicization of lung inflammatory processes with a consequent reduction in fibrosis progression (Cargnoni et al., 2020).
Mesenchymal cells derived from the human umbilical cord (including Wharton's jelly, herein collectively referred to as hUC-MSC), have also been extensively investigated in experimental models of lung fibrosis. The first study performed with these cells reported that IV injection in immunodeficient mice (SCID) 24 h post-bleomycin challenge decreased lung inflammatory cell infiltrate and the expression of pro-inflammatory (MIF, TNF-α and INF-⋎) and pro-fibrotic cytokines (TGF-β). hUC-MSC also reduced collagen interstitial levels which could be correlated to the pro-degradative milieu created by increased MMP-2 levels and reduced their endogenous inhibitors (Moodley et al., 2009). Similar findings related to hUC-MSC ability to reduce collagen deposition were reported in a more recent study where hUC-MSC were administered twice (24 h and 7 days post-bleomycin) (Moroncini et al., 2018). In addition, hUC-MSC reduced the expression of IL-6 and levels of M2 macrophages.
hUC-MSC have been also used in the effort to revert pulmonary fibrosis induced by bleomycin in lung left lobe of rats. In this case, cells were administered at day 21 after bleomycin challenge. hUC-MSC transplantation reduced fibroblast activation and collagen accumulation associated with preserved alveolar structure and gas exchange (Chu et al., 2019). Furthermore, in contrast to Moroncini et al. (2018), hUC-MSC increased levels of pulmonary M2 macrophages producing matrix metallopeptidase 9 (MMP-9) involved in collagen degradation. Hyaluronan, released from hUC-MSC, appeared to be involved in macrophage polarization to an M2-like phenotype (Chu et al., 2019).
Recently, to improve the migration and homing of transplanted cells toward injured tissues, CXCR4 over-expressing hUC-MSC were IV injected in a murine model of radiation-induced lung injury (Zhang et al., 2019). A higher number of CXCR4-overexpressing hUC-MSC reached the injured lung tissues with respect to “normal” hUC-MSC and were more effective in decreasing the radiation-induced lung injury. Indeed, a marked reduction in the expression of the pro-fibrotic cytokine TGF-β1 and of α-SMA and collagen I was reported. Furthermore, CXCR4-overexpressing hUC-MSC preserved the expression of E-cadherin, suggesting that cell treatment can inhibit the radiation induced epithelial-mesenchymal transition (EMT) process and fibroblast activation into myofibroblasts. The same study reported that transplanted CXCR4-overexpressing hUC-MSC could express pro-Surfactant Protein (SP)-C, a marker of alveolar epithelial II cells, suggesting a possible differentiation of hUC-MSC into alveolar epithelium when transplanted in vivo one day after radiation (Zhang et al., 2019). Finally, MSCs derived from the umbilical cord vein (hUCV-MSC), not from the stromal tissue of umbilical cord, were transplanted in bleomycin challenged mice after previous exposure to oxygen peroxide (H2O2) and, similar to hUC-MSC, preserved alveolar spaces, reduced TGF-β and α-SMA expressions and myeloperoxidase activity, indicative of reduced lung inflammation (Mahmoudi et al., 2020).
It is known that MSCs and other stromal cells present antimicrobial activities, as well as resistance or partial resistance to viral infection (Li et al., 2016; Khoury et al., 2020). Importantly, MSCs and other stromal cells including those of perinatal origin can exert antimicrobial effects either by limiting viruses/bacteria cell colonization and replication, or by limiting complications due to a dysregulated host response to infection such as sepsis. At the moment there are only a few studies reporting the antiviral and antimicrobial effects of perinatal stromal cells. Indeed, the most part of the effects herein reported were observed using other sources of MSCs, such as bone marrow, so we have highlighted those that resulted from studies conducted with perinatal MSCs. Thus, in the following sections we will provide an overview of the applications of MSCs for the treatment of viral infections and sepsis.
Antiviral properties of MSCs have been studied in animal models of respiratory virus infections with controversial findings (Khoury et al., 2020). On one hand many studies report the ability of MSCs to counteract the damage caused by the virus exerting a protective action, putatively also through their immunomodulatory activity that is able to counteract an altered immune response. On the other hand, it should be emphasized that, other studies report how the susceptibility of MSCs to viral infection can alter their immunomodulatory properties while others report limited or negligible results upon MSCs treatment.
Indeed, in an immune-competent mouse model of H9N2 AIV-induced acute lung injury, (Li et al., 2016) reported how a single IV injection of BM-MSCs 30 min after the administration of the virus significantly improved physiological parameters with reduction in mortality, lung edema, and histologic injury. Furthermore, they reported reduced levels of inflammatory cytokines and chemokines (Li et al., 2016). On the other hand, systemic administration of BM-MSC in an immune-competent mouse model of lung injury by H1N1 infection, supplemented or not with the antiviral oseltamivir, did not improve overall survival or lung injury (Darwish et al., 2013). These findings where confirmed by another group that reported how BM-MSCs injection failed to prevent the damage induced by PR8 infection.
Furthermore, another study conducted on lung MSC isolated from 1 to 2 week-old specific pathogen free chickens lungs susceptible to influenza virus infection. The authors showed that MSCs infected with two avian influenza viruses, H1N1 and H9N5, became reservoirs and replication sites for the virus itself. Moreover, the lung MSCs changed their secretome profile with increased release of inflammatory cytokines such as IL-6 and IL-8 and became apoptotic (Khatri et al., 2010). Similar findings were obtained by Cheung et al. (2016) who reported how the respiratory syncytial virus (RSV) was able to infect both human BM-MSCs as well as UC-MSCs modifying their immunomodulatory properties. Indeed RSV-infected MSCs had increased expression of IL-1β, IL-6, IL-8, and SDF-1, and also showed an altered capacity to affect PBMC proliferation (Cheung et al., 2016).
However, other groups have reported that MSCs prevented influenza-induced thrombocytosis and partially reduced the viral load (Gotts et al., 2014). The protective effect exerted by the MSCs on preserving vascular endothelial integrity was also reported in in vivo model of lung hemorrhagic shock (Pati et al., 2011).
In addition, several studies reported the efficacy of MSC treatment in improving the survival of animals with viral infection. Chan et al. (2016) reported how MSC injection strongly improved the dysregulated alveolar fluid clearance induced by the avian virus H5N1. Furthermore, the authors reported how the treatment with MSCs improved alveolar fluid clearance by upregulating the expression of the sodium chloride channel that is usually downregulated by the viral infection (Chan et al., 2016). Importantly, substantial differences in the beneficial effect exerted by MSCs according to the age of the animal were observed. Indeed, MSC injection induced a general improvement in old mice (8–12 months old) by reducing the mortality, lung edema, the total area of lung lesion. In parallel, researchers observed a reduction in the amount of total infiltrating lymphocytes. These effects were not appreciable in young mice (6–8 weeks old) (Chan et al., 2016). This age dependent sensitivity has also been observed in COVID-19 patients. In fact, the severity of the disease increases in aged people while children often develop mild symptoms (Liu K. et al., 2020). However, it still remains to be understood whether this effect is related to reduced levels of Angiotensin-converting enzyme 2 (ACE2) receptor expressed by children, to the immune-senescence appreciable in elderly people, or to other phenomena (Liu K. et al., 2020; Nikolich-Zugich et al., 2020). Conversely, Li et al. (2016) demonstrated how MSC treatment was effective in reducing the lung injury in young (8 weeks old) immunocompetent mouse model of viral H9N2-AIV infection, in parallel reducing the amount of inflammatory cytokines present at serum level.
In a comparison paper, hUC-MSCs were more effective than BM-MSCs in improving the overall survival of H5N1-infected mice. hUC-MSCs were shown to release angiogenin-1 and hepatocyte growth factors that were able to enhance the alveolar fluid clearance, thus reducing lung edema and inflammation (Loy et al., 2019). However, despite these results, MSC treatment was not found to be associated with a general reduction of the viral load (Khoury et al., 2020). The ability of MSCs to act positively in counteracting the damage caused by viral infection was not limited to the cell therapy per se, but as aforementioned positive results were obtained also by the use of the cell secretome (Krasnodembskaya et al., 2010) as well as with the extracellular vesicles (EVs) (Börger et al., 2020). Indeed, MSC-EVs were able not only to suppress the viral replication in lung epithelial cells (Khatri et al., 2018), but also to exert anti-inflammatory effects (Willis et al., 2018; Park et al., 2019; Varkouhi et al., 2019). Conversely, in an in vitro study, the immunomodulatory effect of hUC-MSCs was reported to be responsible for the reduced cytotoxic activity of a subset of T lymphocytes, Vγ9Vδ2 T lymphocytes, against H1N1-infected A549 cells (Liu et al., 2015). On the other hand, it has also been reported that MSCs did not affect the viral-specific T lymphocyte activity (Karlsson et al., 2008). Indeed, Karlsson et al. (2008) found that MSCs co-cultured with virus specific EBV or CMV cytotoxic T lymphocytes maintained their cytotoxic activity. These results were also confirmed by a clinical report highlighting how patients who received MSCs for graft vs. host disease (GVDH) treatment still maintained the capacity to responded to CMV viral infection (Karlsson et al., 2008).
For many aspects COVID-19 pathogenesis is comparable to sepsis. Indeed, sepsis is defined as a life-threatening organ dysfunction caused by a overreacted host response to infection (Singer et al., 2016). As a matter of fact, COVID-19 patients are often hospitalized for severe pneumonia that rapidly deteriorates to multi organ failure as a consequence of infection spread and cytokine storm (Jose and Manuel, 2020).
The pathogenesis of both sepsis and COVID-19 are indeed characterized by the massive infiltration of immune cells to the target organ or tissue, increased levels of cytokines and signaling molecules released which attract more immune cells that triggers a feedback loop that exacerbates inflammatory response causing the formation of tissue damage and potentially leading to multi-organ failure and death (Singer et al., 2016; Jose and Manuel, 2020). In line with this, one study demonstrated that SARS-CoV2 infection was reported to trigger acute kidney failure in 20% of cases analyzed (Arentz et al., 2020). Another case report highlighted that over 30% patients with COVID-19 developed liver injury (Bhatraju et al., 2020). In both studies the authors report that patients undergo septic shock.
MSCs have been reported to improve survival in different preclinical models of sepsis (Lombardo et al., 2015). They have also been shown to reduce the deleterious effects determined by the cytokine storm typical in sepsis model. These findings were confirmed by the reduction of plasma inflammatory cytokines after administration of BM-MSCs in different mouse models of sepsis, such as intraperitoneal injection of LPS (Xu et al., 2007; Saeedi et al., 2019) or the caecal ligation and puncture (Mei et al., 2010; Luo et al., 2014). A study using hAMSCs also reported that treatment of mice with cecum-puncture-induced sepsis protected mice against death caused by diffuse peritonitis (Parolini et al., 2014).
These effects were also confirmed in a mouse model of ARDS and sepsis due to Escherichia coli (E. coli)-induced pneumonia sepsis (Horie et al., 2020). Treatment with a subpopulation of hUC-MSCs, more specifically CD362+hUC-MSCs, resulted in the reduction of bacterial infiltration and injury, thus ameliorating inflammatory marker levels (Horie et al., 2020). Similar findings were also obtained in rat models of ARDS or ARDS combined with severe sepsis (ARDS-SS) syndrome obtained upon caecal-ligation and puncture. In both models, hUC-MSC administration reduced the serum levels of inflammatory cytokines, and slightly reduced the percentages of inflammatory cells in ascites and kidney parenchyma thus improving the overall survival (Lee et al., 2017). Another study reported that the beneficial effect exerted by hUC-MSC transplantation was due to the release of the anti-microbial peptide LL-37 (Zhu, 2017).
The anti-microbial effect of MSCs is not limited to the MSC per se. Indeed, beneficial results have also been obtained after injection of the secretome derived from BM-MSC culture. These effects were strictly related to the increased expression of the antimicrobial peptide LL-37 by MSCs primed with E. coli (Krasnodembskaya et al., 2010). Other bioactive molecules released by MSCs are lipocalin-2, that has been shown to regulate the activation of neutrophils and thus improve bacteria clearance (Gupta et al., 2012), and beta-defensin-2. Importantly, the injection of hUC-MSCs in a mouse model of E. coli-induced acute lung injury improved the bacteria clearance by the release of beta-defensin-2 upon the direct triggering of the TLR4 (Sung et al., 2016).
The beneficial effects obtained after MSC injection to treat sepsis have been shown to be related to their strong immunomodulatory properties. Indeed, MSCs were able to reduce the plasma levels of different inflammatory cytokines thus improving the lung histology (Gonzalez-Rey et al., 2009; Shin et al., 2013; Sepúlveda et al., 2014), as well as cardiac tissue quality (Weil et al., 2010; Manukyan et al., 2011), in different in vivo models of sepsis. Importantly, MSC treatment can reduce the infiltration of inflammatory cells like neutrophils, macrophages, and T lymphocytes to the target organs in animal model of sepsis (Mei et al., 2010; Anderson et al., 2013; Hall et al., 2013; Zhao et al., 2014). Moreover, reduced IL-6 and IL-1b, two relevant inflammatory cytokines in sepsis that it have been reported to play a relevant role in COVID-19 [as highlighted by the different clinical trials using different monoclonal antibodies targeting IL-6 (Tocilizumab) (De Luna et al., 2020; Toniati et al., 2020) as well as IL-1b (Anakinra) (Huet et al., 2020)], was also observed (Wilson et al., 2015; McIntyre et al., 2018). Also, IL-10 plays a relevant role in modulating the immune response. Indeed, COVID-19 patients often present high plasma concentration of this cytokine (Chiappelli et al., 2020; Huang et al., 2020), and MSCs have been shown to modulate the serum levels of IL-10 by stimulating its production by immune system cells. Indeed, it has been reported that IL-10 is not directly produced by MSCs but is a consequence of the macrophage skewing toward anti-inflammatory M2 macrophages; an effect that seems to be triggered by the prostaglandin E2 (PGE2) released by MSCs (Nemeth et al., 2009). Furthermore, this effect was also confirmed in in vivo studies. Indeed, the intraperitoneal injection of M2 macrophages, induced by the in vitro co-culture with BM-MSCs, in septic mice were able to improve the overall survival (Anderson et al., 2013). The effects were due to a general systemic reduction of immune cell infiltration in different organs (Anderson et al., 2013). Noteworthy, when septic mice depleted for monocytes or neutrophils were treated with MSCs, no beneficial effects were reported, thus highlighting the crucial role of the interactions of MSCs on immune cells in order to exert a therapeutic effect (Nemeth et al., 2009; Hall et al., 2013).
Overall, there are numerous experimental evidences that support the application of PDCs in the treatment of COVID-19. The intrinsic properties of PDCs, notorius for their immunomodulatory properties, seem to perfectly mitigate the etiopathological characteristics of COVID-19 (Figure 2B).
AP, AC, MS, and AS: manuscript writing. AS and GK: manuscript writing and editing. RO and OP: conception and design, financial support. All authors contributed to the article and approved the submitted version.
This work was supported by the Fondazione Poliambulanza-Istituto Ospedaliero, Brescia, Italy, Contributo MIUR 5x1000 (2017), Intramural funds from the Università Cattolica del Sacro Cuore (Linea D1-2018 and Linea D1-2019), and PRIN 2017 program of Italian Ministry of Research and University (MIUR, Grant No. 2017RSAFK7).
MS, GK, and RO are employed by the company Pluristem Ltd.
The remaining authors declare that the research was conducted in the absence of any commercial or financial relationships that could be construed as a potential conflict of interest.
This work contributes to the COST Action CA17116 International Network for Translating Research on Perinatal Derivatives into Therapeutic Approaches (SPRINT), supported by COST (European Cooperation in Science and Technology).
Abumaree, M. H., Al Jumah, M. A., Kalionis, B., Jawdat, D., Al Khaldi, A., AlTalabani, A. A., et al. (2013). Phenotypic and functional characterization of mesenchymal stem cells from chorionic villi of human term placenta. Stem Cell Rev. 9, 16–31. doi: 10.1007/s12015-012-9385-4
Alcayaga-Miranda, F., Cuenca, J., and Khoury, M. (2017). Antimicrobial activity of mesenchymal stem cells: current status and new perspectives of antimicrobial peptide-based therapies. Front. Immunol. 8:339. doi: 10.3389/fimmu.2017.00339
Allen, H., Shraga-Heled, N., Blumenfeld, M., Dego-Ashto, T., Fuchs-Telem, D., Gilert, A., et al. (2018). Human placental-derived adherent stromal cells co-induced with TNF-alpha and IFN-gamma inhibit triple-negative breast cancer in nude mouse xenograft models. Sci. Rep. 8:670. doi: 10.1038/s41598-017-18428-1
Amari, A., Ebtekar, M., Moazzeni, S. M., Soleimani, M., Amirabad, L. M., Tahoori, M. T., et al. (2015). Investigation of immunomodulatory properties of human Wharton's Jelly-derived mesenchymal stem cells after lentiviral transduction. Cell. Immunol. 293, 59–66. doi: 10.1016/j.cellimm.2014.12.003
Anderson, P., Souza-Moreira, L., Morell, M., Caro, M., O'Valle, F., Gonzalez-Rey, E., et al. (2013). Adipose-derived mesenchymal stromal cells induce immunomodulatory macrophages which protect from experimental colitis and sepsis. Gut 62, 1131–1141. doi: 10.1136/gutjnl-2012-302152
Arachchillage, D. R. J., and Laffan, M. (2020). Abnormal coagulation parameters are associated with poor prognosis in patients with novel coronavirus pneumonia. J. Thromb. Haemost. 18, 1233–1234. doi: 10.1111/jth.14820
Arentz, M., Yim, E., Klaff, L., Lokhandwala, S., Riedo, F. X., Chong, M., et al. (2020). Characteristics and outcomes of 21 critically Ill patients with COVID-19 in Washington State. JAMA 323, 1612–1614. doi: 10.1001/jama.2020.4326
Bailo, M., Soncini, M., Vertua, E., Signoroni, P. B., Sanzone, S., Lombardi, G., et al. (2004). Engraftment potential of human amnion and chorion cells derived from term placenta. Transplantation 78, 1439–1448. doi: 10.1097/01.TP.0000144606.84234.49
Banas, R. A., Trumpower, C., Bentlejewski, C., Marshall, V., Sing, G., and Zeevi, A. (2008). Immunogenicity and immunomodulatory effects of amnion-derived multipotent progenitor cells. Hum. Immunol. 69, 321–328. doi: 10.1016/j.humimm.2008.04.007
Barkama, R., Mayo, A., Paz, A., Solopov, A., Mann, T., Vadasz, Z., et al. (2020). Placenta-derived cell therapy to treat patients with respiratory failure due to coronavirus disease 2019. Crit. Care Explor. 2:e0207. doi: 10.1097/CCE.0000000000000207
Baughman, R. P., Culver, D. A., Jankovi, V., Fischkoff, S., Brockway, G., and Lower, E. E. (2015). Placenta-derived mesenchymal-like cells (PDA-001) as therapy for chronic pulmonary sarcoidosis: a phase 1 study. Sarcoidosis Vasc. Diffuse Lung Dis. 32, 106–114.
Beigel, J. H., Tomashek, K. M., Dodd, L. E., Mehta, A. K., Zingman, B. S., Kalil, A. C., et al. (2020). Remdesivir for the treatment of Covid-19 - final report. N. Engl. J. Med. 383, 1813–1826. doi: 10.1056/NEJMoa2007764
Bhatraju, P. K., Ghassemieh, B. J., Nichols, M., Kim, R., Jerome, K. R., Nalla, A. K., et al. (2020). Covid-19 in critically Ill patients in the Seattle Region - Case Series. N. Engl. J. Med. 382, 2012–2022. doi: 10.1056/NEJMoa2004500
Börger, V., Weiss, D. J., Anderson, J. D., Borràs, F. E., Bussolati, B., Carter, D. R. F., et al. (2020). ISEV and ISCT statement on EVs from MSCs and other cells: considerations for potential therapeutic agents to suppress COVID-19. Cytotherapy 22, 482–485. doi: 10.1016/j.jcyt.2020.05.002
Braid, L. R., Wood, C. A., Wiese, D. M., and Ford, B. N. (2018). Intramuscular administration potentiates extended dwell time of mesenchymal stromal cells compared to other routes. Cytotherapy 20, 232–244. doi: 10.1016/j.jcyt.2017.09.013
Caplan, H., Olson, S. D., Kumar, A., George, M., Prabhakara, K. S., Wenzel, P., et al. (2019). Mesenchymal stromal cell therapeutic delivery: translational challenges to clinical application. Front. Immunol. 10:1645. doi: 10.3389/fimmu.2019.01645
Cargnoni, A., Gibelli, L., Tosini, A., Signoroni, P. B., Nassuato, C., Arienti, D., et al. (2009). Transplantation of allogeneic and xenogeneic placenta-derived cells reduces bleomycin-induced lung fibrosis. Cell Transplant. 18, 405–422. doi: 10.3727/096368909788809857
Cargnoni, A., Piccinelli, E. C., Ressel, L., Rossi, D., Magatti, M., Toschi, I., et al. (2014). Conditioned medium from amniotic membrane-derived cells prevents lung fibrosis and preserves blood gas exchanges in bleomycin-injured mice-specificity of the effects and insights into possible mechanisms. Cytotherapy 16, 17–32. doi: 10.1016/j.jcyt.2013.07.002
Cargnoni, A., Ressel, L., Rossi, D., Poli, A., Arienti, D., Lombardi, G., et al. (2012). Conditioned medium from amniotic mesenchymal tissue cells reduces progression of bleomycin-induced lung fibrosis. Cytotherapy 14, 153–161. doi: 10.3109/14653249.2011.613930
Cargnoni, A., Romele, P., Bonassi Signoroni, P., Farigu, S., Magatti, M., Vertua, E., et al. (2020). Amniotic MSCs reduce pulmonary fibrosis by hampering lung B-cell recruitment, retention, and maturation. Stem Cells Transl. Med. 9, 1023–1035. doi: 10.1002/sctm.20-0068
Chambers, D. C., Enever, D., Ilic, N., Sparks, L., Whitelaw, K., Ayres, J., et al. (2014). A phase 1b study of placenta-derived mesenchymal stromal cells in patients with idiopathic pulmonary fibrosis. Respirology 19, 1013–1018. doi: 10.1111/resp.12343
Chan, M. C., Kuok, D. I., Leung, C. Y., Hui, K. P., Valkenburg, S. A., Lau, E. H., et al. (2016). Human mesenchymal stromal cells reduce influenza A H5N1-associated acute lung injury in vitro and in vivo. Proc. Natl. Acad. Sci. U. S. A. 113, 3621–3626. doi: 10.1073/pnas.1601911113
Chang, C. J., Yen, M. L., Chen, Y. C., Chien, C. C., Huang, H. I., Bai, C. H., et al. (2006). Placenta-derived multipotent cells exhibit immunosuppressive properties that are enhanced in the presence of interferon-gamma. Stem Cells 24, 2466–2477. doi: 10.1634/stemcells.2006-0071
Chase, D. M., and Gallicchio, V. S. (2019). The effect of mesenchymal stem cells and exosomes to treat idiopathic pulmonary fibrosis. J. Stem Cell Res. Ther. 5, 48–59. Available online at: https://medcraveonline.com/JSRT/JSRT-05-00134.pdf
Chatterjee, P., Chiasson, V. L., Pinzur, L., Raveh, S., Abraham, E., Jones, K. A., et al. (2016). Human placenta-derived stromal cells decrease inflammation, placental injury and blood pressure in hypertensive pregnant mice. Clin. Sci. 130, 513–523. doi: 10.1042/CS20150555
Chen, N., Zhou, M., Dong, X., Qu, J., Gong, F., Han, Y., et al. (2020). Epidemiological and clinical characteristics of 99 cases of 2019 novel coronavirus pneumonia in Wuhan, China: a descriptive study. Lancet 395, 507–513. doi: 10.1016/S0140-6736(20)30211-7
Cheung, M. B., Sampayo-Escobar, V., Green, R., Moore, M. L., Mohapatra, S., and Mohapatra, S. S. (2016). Respiratory syncytial virus-infected mesenchymal stem cells regulate immunity via interferon beta and indoleamine-2,3-dioxygenase. PLoS ONE 11:e0163709. doi: 10.1371/journal.pone.0163709
Chiappelli, F., Khakshooy, A., and Greenberg, G. (2020). CoViD-19 Immunopathology and Immunotherapy. Bioinformation 16, 219–222. doi: 10.6026/97320630016219
Chu, K. A., Wang, S. Y., Yeh, C. C., Fu, T. W., Fu, Y. Y., Ko, T. L., et al. (2019). Reversal of bleomycin-induced rat pulmonary fibrosis by a xenograft of human umbilical mesenchymal stem cells from Wharton's jelly. Theranostics 9, 6646–6664. doi: 10.7150/thno.33741
Connors, J. M., and Levy, J. H. (2020). COVID-19 and its implications for thrombosis and anticoagulation. Blood 135, 2033–2040. doi: 10.1182/blood.2020006000
Cox, D. (2020). Some Patients Who Survive COVID-19 May Suffer Lasting Lung Damage. Available from: https://www.sciencenews.org/article/coronavirus-covid-19-some-patients-may-suffer-lasting-lung-damage (accessed July, 2020).
Danieli, P., Malpasso, G., Ciuffreda, M. C., Cervio, E., Calvillo, L., Copes, F., et al. (2015). Conditioned medium from human amniotic mesenchymal stromal cells limits infarct size and enhances angiogenesis. Stem Cells Transl. Med. 4, 448–458. doi: 10.5966/sctm.2014-0253
Darwish, I., Banner, D., Mubareka, S., Kim, H., Besla, R., Kelvin, D. J., et al. (2013). Mesenchymal stromal (stem) cell therapy fails to improve outcomes in experimental severe influenza. PLoS ONE 8:e71761. doi: 10.1371/journal.pone.0071761
De Biasi, S., Meschiari, M., Gibellini, L., Bellinazzi, C., Borella, R., Fidanza, L., et al. (2020). Marked T cell activation, senescence, exhaustion and skewing towards TH17 in patients with COVID-19 pneumonia. Nat. Comm. 11:3434. doi: 10.1038/s41467-020-17292-4
De Coppi, P., Bartsch, G. Jr., Siddiqui, M. M., Xu, T., Santos, C. C., Perin, L., et al. (2007). Isolation of amniotic stem cell lines with potential for therapy. Nat. Biotechnol. 25, 100–106. doi: 10.1038/nbt1274
De Luna, G., Habibi, A., Deux, J. F., Colard, M., d'Orengiani, P. H. D. A., Schlemmer, F., et al. (2020). Rapid and severe Covid-19 pneumonia with severe acute chest syndrome in a sickle cell patient successfully treated with tocilizumab. Am. J. Hematol. 95, 876–878. doi: 10.1002/ajh.25833
George, P. M., Wells, A. U., and Jenkins, R. G. (2020). Pulmonary fibrosis and COVID-19: the potential role for antifibrotic therapy. Lancet Respir Med 8, 807–815. doi: 10.1016/S2213-2600(20)30225-3
Giampa, C., Alvino, A., Magatti, M., Silini, A. R., Cardinale, A., Paldino, E., et al. (2019). Conditioned medium from amniotic cells protects striatal degeneration and ameliorates motor deficits in the R6/2 mouse model of Huntington's disease. J. Cell. Mol. Med. 23, 1581–1592. doi: 10.1111/jcmm.14113
Gonzalez-Rey, E., Anderson, P., Gonzalez, M. A., Rico, L., Buscher, D., and Delgado, M. (2009). Human adult stem cells derived from adipose tissue protect against experimental colitis and sepsis. Gut 58, 929–939. doi: 10.1136/gut.2008.168534
Gotts, J. E., Abbott, J., and Matthay, M. A. (2014). Influenza causes prolonged disruption of the alveolar-capillary barrier in mice unresponsive to mesenchymal stem cell therapy. Am. J. Physiol. Lung Cell. Mol. Physiol. 307, L395–406. doi: 10.1152/ajplung.00110.2014
Groh, M. E., Maitra, B., Szekely, E., and Koc, O. N. (2005). Human mesenchymal stem cells require monocyte-mediated activation to suppress alloreactive T cells. Exp. Hematol. 33, 928–934. doi: 10.1016/j.exphem.2005.05.002
Guan, W. J., Ni, Z. Y., Hu, Y., Liang, W. H., Ou, C. Q., He, J. X., et al. (2020). Clinical Characteristics of Coronavirus Disease 2019 in China. New Engl. J. Med. 382, 1708–1720. doi: 10.1056/NEJMoa2002032
Guaraldi, G., Meschiari, M., Cozzi-Lepri, A., Milic, J., Tonelli, R., Menozzi, M., et al. (2020). Tocilizumab in patients with severe COVID-19: a retrospective cohort study. Lancet Rheumatol. 2, e474–e484. doi: 10.1016/S2665-9913(20)30173-9
Guo, Z., Chen, Y., Luo, X., He, X., Zhang, Y., and Wang, J. (2020). Administration of umbilical cord mesenchymal stem cells in patients with severe COVID-19 pneumonia. Crit Care 24:420. doi: 10.1186/s13054-020-03142-8
Gupta, N., Krasnodembskaya, A., Kapetanaki, M., Mouded, M., Tan, X., Serikov, V., et al. (2012). Mesenchymal stem cells enhance survival and bacterial clearance in murine Escherichia coli pneumonia. Thorax 67, 533–539. doi: 10.1136/thoraxjnl-2011-201176
Hall, S. R., Tsoyi, K., Ith, B., Padera, R. F. Jr., Lederer, J. A., Wang, Z., et al. (2013). Mesenchymal stromal cells improve survival during sepsis in the absence of heme oxygenase-1: the importance of neutrophils. Stem Cells 31, 397–407. doi: 10.1002/stem.1270
Hamidian Jahromi, S., and Davies, J. E. (2019). Concise review: skeletal muscle as a delivery route for mesenchymal stromal cells. Stem Cells Transl. Med. 8, 456–465. doi: 10.1002/sctm.18-0208
He, F., Wang, Y., Li, Y., and Yu, L. (2020). Human amniotic mesenchymal stem cells alleviate paraquat-induced pulmonary fibrosis in rats by inhibiting the inflammatory response. Life Sci. 243:117290. doi: 10.1016/j.lfs.2020.117290
Horie, S., Masterson, C., Brady, J., Loftus, P., Horan, E., O'Flynn, L., et al. (2020). Umbilical cord-derived CD362(+) mesenchymal stromal cells for E. coli pneumonia: impact of dose regimen, passage, cryopreservation, and antibiotic therapy. Stem Cell Res. Ther. 11:116. doi: 10.1186/s13287-020-01624-8
Huang, C., Wang, Y., Li, X., Ren, L., Zhao, J., Hu, Y., et al. (2020). Clinical features of patients infected with 2019 novel coronavirus in Wuhan, China. Lancet 395, 497–506. doi: 10.1016/S0140-6736(20)30183-5
Huet, T., Beaussier, H., Voisin, O., Jouveshomme, S., Dauriat, G., Lazareth, I., et al. (2020). Anakinra for severe forms of COVID-19: a cohort study. Lancet Rheumatol 2, e393–e400. doi: 10.1016/S2665-9913(20)30164-8
In 't Anker, P. S., Scherjon, S. A., Kleijburg-van der Keur, C., de Groot-Swings, G. M., Claas, F. H., Fibbe, W. E., et al. (2004). Isolation of mesenchymal stem cells of fetal or maternal origin from human placenta. Stem Cells 22, 1338–1345. doi: 10.1634/stemcells.2004-0058
Jose, R. J., and Manuel, A. (2020). COVID-19 cytokine storm: the interplay between inflammation and coagulation. Lancet Respir Med 8, e46–e47. doi: 10.1016/S2213-2600(20)30216-2
Kabat, M., Bobkov, I., Kumar, S., and Grumet, M. (2020). Trends in mesenchymal stem cell clinical trials 2004-2018: is efficacy optimal in a narrow dose range? Stem Cells Transl. Med. 9, 17–27. doi: 10.1002/sctm.19-0202
Kang, J. W., Koo, H. C., Hwang, S. Y., Kang, S. K., Ra, J. C., Lee, M. H., et al. (2012). Immunomodulatory effects of human amniotic membrane-derived mesenchymal stem cells. J. Vet. Sci. 13, 23–31. doi: 10.4142/jvs.2012.13.1.23
Karlsson, H., Erkers, T., Nava, S., Ruhm, S., Westgren, M., and Ringden, O. (2012). Stromal cells from term fetal membrane are highly suppressive in allogeneic settings in vitro. Clin. Exp. Immunol. 167, 543–555. doi: 10.1111/j.1365-2249.2011.04540.x
Karlsson, H., Samarasinghe, S., Ball, L. M., Sundberg, B., Lankester, A. C., Dazzi, F., et al. (2008). Mesenchymal stem cells exert differential effects on alloantigen and virus-specific T-cell responses. Blood 112, 532–541. doi: 10.1182/blood-2007-10-119370
Khatri, M., O'Brien, T. D., Goyal, S. M., and Sharma, J. M. (2010). Isolation and characterization of chicken lung mesenchymal stromal cells and their susceptibility to avian influenza virus. Dev. Comp. Immunol. 34, 474–479. doi: 10.1016/j.dci.2009.12.008
Khatri, M., Richardson, L. A., and Meulia, T. (2018). Mesenchymal stem cell-derived extracellular vesicles attenuate influenza virus-induced acute lung injury in a pig model. Stem Cell Res. Ther. 9:17. doi: 10.1186/s13287-018-0774-8
Khoury, M., Cuenca, J., Cruz, F. F., and Figueroa, F. E. (2020). Current status of cell-based therapies for respiratory virus infections: applicability to COVID-19. Eur. Respir. J. 55:2000858. doi: 10.1183/13993003.00858-2020
Klok, F. A., Kruip, M., van der Meer, N. J. M., Arbous, M. S., Gommers, D., Kant, K. M., et al. (2020). Incidence of thrombotic complications in critically ill ICU patients with COVID-19. Thromb. Res. 191, 145–147. doi: 10.1016/j.thromres.2020.04.013
Koliaraki, V., Prados, A., Armaka, M., and Kollias, G. (2020). The mesenchymal context in inflammation, immunity and cancer. Nat. Immunol. 21, 974–982. doi: 10.1038/s41590-020-0741-2
Kong, W., and Agarwal, P. P. (2020). Chest imaging appearance of COVID-19 infection. Radiol. Cardiothorac. Imaging 2:e200028. doi: 10.1148/ryct.2020200028
Krampera, M., Cosmi, L., Angeli, R., Pasini, A., Liotta, F., Andreini, A., et al. (2006). Role for interferon-gamma in the immunomodulatory activity of human bone marrow mesenchymal stem cells. Stem Cells 24, 386–398. doi: 10.1634/stemcells.2005-0008
Krampera, M., Glennie, S., Dyson, J., Scott, D., Laylor, R., Simpson, E., et al. (2003). Bone marrow mesenchymal stem cells inhibit the response of naive and memory antigen-specific T cells to their cognate peptide. Blood 101, 3722–3729. doi: 10.1182/blood-2002-07-2104
Krasnodembskaya, A., Song, Y., Fang, X., Gupta, N., Serikov, V., Lee, J. W., et al. (2010). Antibacterial effect of human mesenchymal stem cells is mediated in part from secretion of the antimicrobial peptide LL-37. Stem Cells 28, 2229–2238. doi: 10.1002/stem.544
Kronsteiner, B., Wolbank, S., Peterbauer, A., Hackl, C., Redl, H., Griensven, M. V., et al. (2011). Human mesenchymal stem cells from adipose tissue and amnion influence T-cells depending on stimulation method and presence of other immune cells. Stem Cells Dev. 20, 2115–2126. doi: 10.1089/scd.2011.0031
Lange-Consiglio, A., Romele, P., Magatti, M., Silini, A., Idda, A., Martino, N. A., et al. (2020). Priming with inflammatory cytokines is not a prerequisite to increase immune-suppressive effects and responsiveness of equine amniotic mesenchymal stromal cells. Stem Cell Res. Ther. 11:99. doi: 10.1186/s13287-020-01611-z
Lee, F. Y., Chen, K. H., Wallace, C. G., Sung, P. H., Sheu, J. J., Chung, S. Y., et al. (2017). Xenogeneic human umbilical cord-derived mesenchymal stem cells reduce mortality in rats with acute respiratory distress syndrome complicated by sepsis. Oncotarget 8, 45626–45642. doi: 10.18632/oncotarget.17320
Li, F., Han, F., Li, H., Zhang, J., Qiao, X., Shi, J., et al. (2017). Human placental mesenchymal stem cells of fetal origins-alleviated inflammation and fibrosis by attenuating MyD88 signaling in bleomycin-induced pulmonary fibrosis mice. Mol. Immunol. 90, 11–21. doi: 10.1016/j.molimm.2017.06.032
Li, H., Liu, L., Zhang, D., Xu, J., Dai, H., Tang, N., et al. (2020). SARS-CoV-2 and viral sepsis: observations and hypotheses. Lancet 395, 1517–1520. doi: 10.1016/S0140-6736(20)30920-X
Li, X., Bai, J., Ji, X., Li, R., Xuan, Y., and Wang, Y. (2014). Comprehensive characterization of four different populations of human mesenchymal stem cells as regards their immune properties, proliferation and differentiation. Int. J. Mol. Med. 34, 695–704. doi: 10.3892/ijmm.2014.1821
Li, Y., Xu, J., Shi, W., Chen, C., Shao, Y., Zhu, L., et al. (2016). Mesenchymal stromal cell treatment prevents H9N2 avian influenza virus-induced acute lung injury in mice. Stem Cell Res. Ther. 7:159. doi: 10.1186/s13287-016-0395-z
Liu, J., Li, S., Liu, J., Liang, B., Wang, X., Wang, H., et al. (2020). Longitudinal characteristics of lymphocyte responses and cytokine profiles in the peripheral blood of SARS-CoV-2 infected patients. EBioMedicine 55:102763. doi: 10.1016/j.ebiom.2020.102763
Liu, K., Chen, Y., Lin, R., and Han, K. (2020). Clinical features of COVID-19 in elderly patients: A comparison with young and middle-aged patients. J. Infect. 80, e14–e18. doi: 10.1016/j.jinf.2020.03.005
Liu, S., Peng, D., Qiu, H., Yang, K., Fu, Z., and Zou, L. (2020). Mesenchymal stem cells as a potential therapy for COVID-19. Stem Cell Res. Ther. 11:169. doi: 10.1186/s13287-020-01678-8
Liu, X., Feng, T., Gong, T., Shen, C., Zhu, T., Wu, Q., et al. (2015). Human umbilical cord mesenchymal stem cells inhibit the function of allogeneic activated Vγ9Vδ2 T lymphocytes in vitro. BioMed Res. Int. 2015:317801. doi: 10.1155/2015/317801
Lombardo, E., van der Poll, T., DelaRosa, O., and Dalemans, W. (2015). Mesenchymal stem cells as a therapeutic tool to treat sepsis. World J. Stem Cells 7, 368–379. doi: 10.4252/wjsc.v7.i2.368
Loy, H., Kuok, D. I., Hui, K. P., Choi, M. H., Yuen, W., Nicholls, J. M., et al. (2019). Therapeutic implications of human umbilical cord mesenchymal stromal cells in attenuating influenza A(H5N1) virus-associated acute lung injury. J. Infect. Dis. 219, 186–196. doi: 10.1093/infdis/jiy478
Luo, C. J., Zhang, F. J., Zhang, L., Geng, Y. Q., Li, Q. G., Hong, Q., et al. (2014). Mesenchymal stem cells ameliorate sepsis-associated acute kidney injury in mice. Shock 41, 123–129. doi: 10.1097/SHK.0000000000000080
Magatti, M., De Munari, S., Vertua, E., Gibelli, L., Wengler, G. S., and Parolini, O. (2008). Human amnion mesenchyme harbors cells with allogeneic T-cell suppression and stimulation capabilities. Stem Cells 26, 182–192. doi: 10.1634/stemcells.2007-0491
Magatti, M., De Munari, S., Vertua, E., Nassauto, C., Albertini, A., Wengler, G. S., et al. (2009). Amniotic mesenchymal tissue cells inhibit dendritic cell differentiation of peripheral blood and amnion resident monocytes. Cell Transplant. 18, 899–914. doi: 10.3727/096368909X471314
Magatti, M., Masserdotti, A., Bonassi Signoroni, P., Vertua, E., Stefani, F. R., Silini, A. R., et al. (2020). B Lymphocytes as targets of the immunomodulatory properties of human amniotic mesenchymal stromal cells. Front. Immunol. 11:1156. doi: 10.3389/fimmu.2020.01156
Magatti, M., Vertua, E., De Munari, S., Caro, M., Caruso, M., Silini, A., et al. (2016). Human amnion favours tissue repair by inducing the M1-to-M2 switch and enhancing M2 macrophage features. J. Tissue Eng. Regen. Med. 11, 2895–2911. doi: 10.1002/term.2193
Magro, C., Mulvey, J. J., Berlin, D., Nuovo, G., Salvatore, S., Harp, J., et al. (2020). Complement associated microvascular injury and thrombosis in the pathogenesis of severe COVID-19 infection: a report of five cases. Transl. Res. doi: 10.1016/j.trsl.2020.04.007
Mahmoudi, T., Abdolmohammadi, K., Bashiri, H., Mohammadi, M., Rezaie, M. J., Fathi, F., et al. (2020). Hydrogen peroxide preconditioning promotes protective effects of umbilical cord vein mesenchymal stem cells in experimental pulmonary fibrosis. Adv. Pharm. Bull. 10, 72–80. doi: 10.15171/apb.2020.009
Manukyan, M. C., Weil, B. R., Wang, Y., Abarbanell, A. M., Herrmann, J. L., Poynter, J. A., et al. (2011). Female stem cells are superior to males in preserving myocardial function following endotoxemia. Am. J. Physiol. Regul. Integr. Comp. Physiol. 300, R1506–R1514. doi: 10.1152/ajpregu.00518.2010
Marquez-Curtis, L. A., Janowska-Wieczorek, A., McGann, L. E., and Elliott, J. A. (2015). Mesenchymal stromal cells derived from various tissues: Biological, clinical and cryopreservation aspects. Cryobiology 71, 181–197. doi: 10.1016/j.cryobiol.2015.07.003
McIntyre, L. A., Stewart, D. J., Mei, S. H. J., Courtman, D., Watpool, I., et al. (2018). Cellular immunotherapy for septic shock. A phase I clinical trial. Am. J. Respir. Crit. Care Med. 197, 337–347. doi: 10.1164/rccm.201705-1006OC
Mei, S. H., Haitsma, J. J., Dos Santos, C. C., Deng, Y., Lai, P. F., Slutsky, A. S., et al. (2010). Mesenchymal stem cells reduce inflammation while enhancing bacterial clearance and improving survival in sepsis. Am. J. Respir. Crit. Care Med. 182, 1047–1057. doi: 10.1164/rccm.201001-0010OC
Meng, F., Xu, R., Wang, S., Xu, Z., Zhang, C., Li, Y., et al. (2020). Human umbilical cord-derived mesenchymal stem cell therapy in patients with COVID-19: a phase 1 clinical trial. Signal Transd. Target. Ther. 5:172. doi: 10.1038/s41392-020-00286-5
Moll, G., Ankrum, J. A., Kamhieh-Milz, J., Bieback, K., Ringdén, O., Volk, H. D., et al. (2019). Intravascular mesenchymal stromal/stem cell therapy product diversification: time for new clinical guidelines. Trends Mol. Med. 25, 149–163. doi: 10.1016/j.molmed.2018.12.006
Moodley, Y., Atienza, D., Manuelpillai, U., Samuel, C. S., Tchongue, J., Ilancheran, S., et al. (2009). Human umbilical cord mesenchymal stem cells reduce fibrosis of bleomycin-induced lung injury. Am. J. Pathol. 175, 303–313. doi: 10.2353/ajpath.2009.080629
Moodley, Y., Ilancheran, S., Samuel, C., Vaghjiani, V., Atienza, D., Williams, E. D., et al. (2010). Human amnion epithelial cell transplantation abrogates lung fibrosis and augments repair. Am. J. Respir. Crit. Care Med. 182, 643–651. doi: 10.1164/rccm.201001-0014OC
Moodley, Y., Vaghjiani, V., Chan, J., Baltic, S., Ryan, M., Tchongue, J., et al. (2013). Anti-inflammatory effects of adult stem cells in sustained lung injury: a comparative study. PLoS ONE 8:e69299. doi: 10.1371/journal.pone.0069299
Moroncini, G., Paolini, C., Orlando, F., Capelli, C., Grieco, A., Tonnini, C., et al. (2018). Mesenchymal stromal cells from human umbilical cord prevent the development of lung fibrosis in immunocompetent mice. PLoS ONE 13:e0196048. doi: 10.1371/journal.pone.0196048
Morrissey, J. H. (2004). Tissue factor: a key molecule in hemostatic and nonhemostatic systems. Int. J. Hematol. 79, 103–108. doi: 10.1532/IJH97.03167
Mougiakakos, D., Jitschin, R., Johansson, C. C., Okita, R., Kiessling, R., and Le Blanc, K. (2011). The impact of inflammatory licensing on heme oxygenase-1-mediated induction of regulatory T cells by human mesenchymal stem cells. Blood 117, 4826–4835. doi: 10.1182/blood-2010-12-324038
Murphy, S., Lim, R., Dickinson, H., Acharya, R., Rosli, S., Jenkin, G., et al. (2011). Human amnion epithelial cells prevent bleomycin-induced lung injury and preserve lung function. Cell Transplant. 20, 909–923. doi: 10.3727/096368910X543385
Murphy, S. V., Shiyun, S. C., Tan, J. L., Chan, S., Jenkin, G., Wallace, E. M., et al. (2012). Human amnion epithelial cells do not abrogate pulmonary fibrosis in mice with impaired macrophage function. Cell Transplant. 21, 1477–1492. doi: 10.3727/096368911X601028
Najar, M., Raicevic, G., Boufker, H. I., Fayyad Kazan, H., De Bruyn, C., Meuleman, N., et al. (2010a). Mesenchymal stromal cells use PGE2 to modulate activation and proliferation of lymphocyte subsets: Combined comparison of adipose tissue, Wharton's Jelly and bone marrow sources. Cell Immunol. 264, 171–179. doi: 10.1016/j.cellimm.2010.06.006
Najar, M., Raicevic, G., Boufker, H. I., Fayyad-Kazan, H., De Bruyn, C., Meuleman, N., et al. (2010b). Adipose-tissue-derived and Wharton's jelly-derived mesenchymal stromal cells suppress lymphocyte responses by secreting leukemia inhibitory factor. Tissue Eng. Part A 16, 3537–3546. doi: 10.1089/ten.tea.2010.0159
Nemeth, K., Leelahavanichkul, A., Yuen, P. S., Mayer, B., Parmelee, A., Doi, K., et al. (2009). Bone marrow stromal cells attenuate sepsis via prostaglandin E(2)-dependent reprogramming of host macrophages to increase their interleukin-10 production. Nat. Med. 15, 42–49. doi: 10.1038/nm.1905
Nikolich-Zugich, J., Knox, K. S., Rios, C. T., Natt, B., Bhattacharya, D., and Fain, M. J. (2020). SARS-CoV-2 and COVID-19 in older adults: what we may expect regarding pathogenesis, immune responses, and outcomes. GeroScience 42, 505–514. doi: 10.1007/s11357-020-00186-0
Norgren, L., Weiss, N., Nikol, S., Hinchliffe, R. J., Lantis, J. C., Patel, M. R., et al. (2019). PLX-PAD cell treatment of critical limb ischaemia: rationale and design of the PACE trial. Eur. J. Vasc. Endovasc. Surg. 57, 538–545. doi: 10.1016/j.ejvs.2018.11.008
Oxley, T. J., Mocco, J., Majidi, S., Kellner, C. P., Shoirah, H., Singh, I. P., et al. (2020). Large-vessel stroke as a presenting feature of Covid-19 in the Young. N. Engl. J. Med. 382:e60. doi: 10.1056/NEJMc2009787
Pan, Y., Guan, H., Zhou, S., Wang, Y., Li, Q., Zhu, T., et al. (2020). Initial CT findings and temporal changes in patients with the novel coronavirus pneumonia (2019-nCoV): a study of 63 patients in Wuhan, China. Eur. Radiol. 30, 3306–3309. doi: 10.1007/s00330-020-06731-x
Papait, A., Vertua, E., Magatti, M., Ceccariglia, S., De Munari, S., Silini, A. R., et al. (2020). Mesenchymal stromal cells from fetal and maternal placenta possess key similarities and differences: potential implications for their applications in regenerative medicine. Cells 9:127. doi: 10.3390/cells9010127
Park, K. S., Svennerholm, K., Shelke, G. V., Bandeira, E., Lässer, C., Jang, S. C., et al. (2019). Mesenchymal stromal cell-derived nanovesicles ameliorate bacterial outer membrane vesicle-induced sepsis via IL-10. Stem Cell Res. Ther. 10:231. doi: 10.1186/s13287-019-1352-4
Parolini, O., Alviano, F., Bagnara, G. P., Bilic, G., Buhring, H. J., Evangelista, M., et al. (2008). Concise review: isolation and characterization of cells from human term placenta: outcome of the first international Workshop on Placenta Derived Stem Cells. Stem Cells 26, 300–311. doi: 10.1634/stemcells.2007-0594
Parolini, O., and Soncini, M. (2006). Human placenta: a source of progenitor/stem cells? J. Reprod. Endokrinol. 3, 117–126.
Parolini, O., Souza-Moreira, L., O'Valle, F., Magatti, M., Hernandez-Cortes, P., Gonzalez-Rey, E., et al. (2014). Therapeutic effect of human amniotic membrane-derived cells on experimental arthritis and other inflammatory disorders. Arthritis Rheumatol. 66, 327–339. doi: 10.1002/art.38206
Pati, S., Gerber, M. H., Menge, T. D., Wataha, K. A., Zhao, Y., Baumgartner, J. A., et al. (2011). Bone marrow derived mesenchymal stem cells inhibit inflammation and preserve vascular endothelial integrity in the lungs after hemorrhagic shock. PLoS ONE 6:e25171. doi: 10.1371/journal.pone.0025171
Peng, R., Sridhar, S., Tyagi, G., Phillips, J. E., Garrido, R., Harris, P., et al. (2013). Bleomycin induces molecular changes directly relevant to idiopathic pulmonary fibrosis: a model for “active” disease. PLoS ONE 8:e59348. doi: 10.1371/journal.pone.0059348
Pianta, S., Bonassi Signoroni, P., Muradore, I., Rodrigues, M. F., Rossi, D., Silini, A., et al. (2015). Amniotic membrane mesenchymal cells-derived factors skew T cell polarization toward Treg and downregulate Th1 and Th17 cells subsets. Stem Cell Rev. 11, 394–407. doi: 10.1007/s12015-014-9558-4
Pianta, S., Magatti, M., Vertua, E., Bonassi Signoroni, P., Muradore, I., Nuzzo, A. M., et al. (2016). Amniotic mesenchymal cells from pre-eclamptic placentae maintain immunomodulatory features as healthy controls. J. Cell. Mol. Med. 20, 157–169. doi: 10.1111/jcmm.12715
Prasanna, S. J., Gopalakrishnan, D., Shankar, S. R., and Vasandan, A. B. (2010). Pro-inflammatory cytokines, IFNgamma and TNFalpha, influence immune properties of human bone marrow and Wharton jelly mesenchymal stem cells differentially. PLoS ONE 5:e9016. doi: 10.1371/journal.pone.0009016
Raicevic, G., Najar, M., Stamatopoulos, B., De Bruyn, C., Meuleman, N., Bron, D., et al. (2011). The source of human mesenchymal stromal cells influences their TLR profile as well as their functional properties. Cell Immunol. 270, 207–216. doi: 10.1016/j.cellimm.2011.05.010
Ren, G., Zhang, L., Zhao, X., Xu, G., Zhang, Y., Roberts, A. I., et al. (2008). Mesenchymal stem cell-mediated immunosuppression occurs via concerted action of chemokines and nitric oxide. Cell Stem Cell 2, 141–150. doi: 10.1016/j.stem.2007.11.014
Rossi, D., Pianta, S., Magatti, M., Sedlmayr, P., and Parolini, O. (2012). Characterization of the conditioned medium from amniotic membrane cells: prostaglandins as key effectors of its immunomodulatory activity. PLoS ONE 7:e46956. doi: 10.1371/journal.pone.0046956
Rothan, H. A., and Byrareddy, S. N. (2020). The epidemiology and pathogenesis of coronavirus disease (COVID-19) outbreak. J. Autoimmun. 109:102433. doi: 10.1016/j.jaut.2020.102433
Roy, R., Brodarac, A., Kukucka, M., Kurtz, A., Becher, P. M., Julke, K., et al. (2013). Cardioprotection by placenta-derived stromal cells in a murine myocardial infarction model. J. Surg. Res. 185, 70–83. doi: 10.1016/j.jss.2013.05.084
Ryan, J. M., Barry, F. P., Murphy, J. M., and Mahon, B. P. (2005). Mesenchymal stem cells avoid allogeneic rejection. J. Inflamm. 2:8. doi: 10.1186/1476-9255-2-8
Saeedi, P., Halabian, R., and Fooladi, A. A. I. (2019). Mesenchymal stem cells preconditioned by staphylococcal enterotoxin B enhance survival and bacterial clearance in murine sepsis model. Cytotherapy 21, 41–53. doi: 10.1016/j.jcyt.2018.11.002
Sepúlveda, J. C., Tomé, M., Fernández, M. E., Delgado, M., Campisi, J., Bernad, A., et al. (2014). Cell senescence abrogates the therapeutic potential of human mesenchymal stem cells in the lethal endotoxemia model. Stem Cells 32, 1865–1877. doi: 10.1002/stem.1654
Sheng, H., Wang, Y., Jin, Y., Zhang, Q., Zhang, Y., Wang, L., et al. (2008). A critical role of IFNgamma in priming MSC-mediated suppression of T cell proliferation through up-regulation of B7-H1. Cell Res. 18, 846–857. doi: 10.1038/cr.2008.80
Shi, Y., Su, J., Roberts, A. I., Shou, P., Rabson, A. B., and Ren, G. (2012). How mesenchymal stem cells interact with tissue immune responses. Trends Immunol. 33, 136–143. doi: 10.1016/j.it.2011.11.004
Shi, Y., Wang, Y., Li, Q., Liu, K., Hou, J., Shao, C., et al. (2018). Immunoregulatory mechanisms of mesenchymal stem and stromal cells in inflammatory diseases. Nat. Rev. Nephrol. 14, 493–507. doi: 10.1038/s41581-018-0023-5
Shin, S., Kim, Y., Jeong, S., Hong, S., Kim, I., Lee, W., et al. (2013). The therapeutic effect of human adult stem cells derived from adipose tissue in endotoxemic rat model. Int. J. Med. Sci. 10, 8–18. doi: 10.7150/ijms.5385
Shu, L., Niu, C., Li, R., Huang, T., Wang, Y., Huang, M., et al. (2020). Treatment of severe COVID-19 with human umbilical cord mesenchymal stem cells. Stem Cell Res. Ther. 11:361. doi: 10.1186/s13287-020-01875-5
Silini, A. R., Cargnoni, A., Magatti, M., Pianta, S., and Parolini, O. (2015). The long path of human placenta, and its derivatives, in regenerative medicine. Front. Bioeng. Biotechnol. 3:162. doi: 10.3389/fbioe.2015.00162
Silini, A. R., Di Pietro, R., Lang-Olip, I., Alviano, F., Banerjee, A., Basile, M., et al. (2020). Perinatal derivatives: where do we stand? A roadmap of the human placenta and consensus for tissue and cell nomenclature. Front. Bioeng. Biotechnol. 8:1438. Available online at: https://www.frontiersin.org/articles/10.3389/fbioe.2020.610544/full
Singer, M., Deutschman, C. S., Seymour, C. W., Shankar-Hari, M., Annane, D., Bauer, M., et al. (2016). The third international consensus definitions for sepsis and septic shock (sepsis-3). JAMA 315, 801–810. doi: 10.1001/jama.2016.0287
Spagnolo, P., Balestro, E., Aliberti, S., Cocconcelli, E., Biondini, D., Casa, G. D., et al., (2020). Pulmonary fibrosis secondary to COVID-19: a call to arms? Lancet Respir Med 8, 750–752. doi: 10.1016/S2213-2600(20)30222-8
Spyropoulos, A. C., Ageno, W., and Barnathan, E. S. (2020). Hospital-based use of thromboprophylaxis in patients with COVID-19. Lancet 395:e75. doi: 10.1016/S0140-6736(20)30926-0
Stone, J. H., Frigault, M. J., Serling-Boyd, N. J., Fernandes, A. D., Harvey, L., Foulkes, A. S., et al. (2020). Efficacy of tocilizumab in patients hospitalized with Covid-19. N. Engl. J. Med 383, 2333–2344. doi: 10.1056/NEJMoa2028836
Sung, D. K., Chang, Y. S., Sung, S. I., Yoo, H. S., Ahn, S. Y., and Park, W. S. (2016). Antibacterial effect of mesenchymal stem cells against Escherichia coli is mediated by secretion of beta- defensin- 2 via toll- like receptor 4 signalling. Cell Microbiol. 18, 424–436. doi: 10.1111/cmi.12522
Tan, J. L., Chan, S. T., Lo, C. Y., Deane, J. A., McDonald, C. A., Bernard, C. C., et al. (2015). Amnion cell mediated immune modulation following bleomycin challenge: controlling the regulatory T cell response. Stem Cell Res. Ther. 6:8. doi: 10.1186/scrt542
Tan, J. L., Lau, S. N., Leaw, B., Nguyen, H. P., Salamonsen, L. A., Saad, M. I., et al. (2018). Amnion epithelial cell-derived exosomes restrict lung injury and enhance endogenous lung repair. Stem Cells Transl. Med. 7, 180–196. doi: 10.1002/sctm.17-0185
Tan, J. L., Tan, Y. Z., Muljadi, R., Chan, S. T., Lau, S. N., Mockler, J. C., et al. (2017). Amnion epithelial cells promote lung repair via lipoxin A(4). Stem Cells Transl. Med. 6, 1085–1095. doi: 10.5966/sctm.2016-0077
Tan, L., Wang, Q., Zhang, D., Ding, J., Huang, Q., Tang, Y.-Q., et al. (2020). Lymphopenia predicts disease severity of COVID-19: a descriptive and predictive study. Sign. Trans. Target. Ther. 5:33. doi: 10.1038/s41392-020-0148-4
Tang, N., Li, D., Wang, X., and Sun, Z. (2020). Abnormal coagulation parameters are associated with poor prognosis in patients with novel coronavirus pneumonia. J. Thromb. Haemost. 18, 844–847. doi: 10.1111/jth.14768
Tipnis, S., Viswanathan, C., and Majumdar, A. S. (2010). Immunosuppressive properties of human umbilical cord-derived mesenchymal stem cells: role of B7-H1 and IDO. Immunol. Cell Biol. 88, 795–806. doi: 10.1038/icb.2010.47
Toniati, P., Piva, S., Cattalini, M., Garrafa, E., Regola, F., Castelli, F., et al. (2020). Tocilizumab for the treatment of severe COVID-19 pneumonia with hyperinflammatory syndrome and acute respiratory failure: A single center study of 100 patients in Brescia, Italy. Autoimmun. Rev. 19:102568. doi: 10.1016/j.autrev.2020.102568
Van Linthout, S., Hamdani, N., Miteva, K., Koschel, A., Muller, I., Pinzur, L., et al. (2017). Placenta-derived adherent stromal cells improve diabetes mellitus-associated left ventricular diastolic performance. Stem Cells Transl. Med. 6, 2135–2145. doi: 10.1002/sctm.17-0130
Varkouhi, A. K., Jerkic, M., Ormesher, L., Gagnon, S., Goyal, S., Rabani, R., et al. (2019). Extracellular vesicles from interferon-γ-primed human umbilical cord mesenchymal stromal cells reduce Escherichia coli-induced acute lung injury in rats. Anesthesiology 130, 778–790. doi: 10.1097/ALN.0000000000002655
Verter, F., Couto, P. S., and Bersenev, A. (2018). A dozen years of clinical trials performing advanced cell therapy with perinatal cells. Future Sci OA. 4:Fso351. doi: 10.4155/fsoa-2018-0085
Vosdoganes, P., Wallace, E. M., Chan, S. T., Acharya, R., Moss, T. J., and Lim, R. (2013). Human amnion epithelial cells repair established lung injury. Cell Transplant. 22, 1337–1349. doi: 10.3727/096368912X657657
Wang, H., Qiu, X., Ni, P., Qiu, X., Lin, X., Wu, W., et al. (2014). Immunological characteristics of human umbilical cord mesenchymal stem cells and the therapeutic effects of their transplantion on hyperglycemia in diabetic rats. Int. J. Mol. Med. 33, 263–270. doi: 10.3892/ijmm.2013.1572
Wang, H. S., Hung, S. C., Peng, S. T., Huang, C. C., Wei, H. M., Guo, Y. J., et al. (2004). Mesenchymal stem cells in the Wharton's jelly of the human umbilical cord. Stem Cells 22, 1330–1337. doi: 10.1634/stemcells.2004-0013
Wang, T., Chen, R., Liu, C., Liang, W., Guan, W., Tang, R., et al. (2020). Attention should be paid to venous thromboembolism prophylaxis in the management of COVID-19. Lancet Haematol. 7, e362–e363. doi: 10.1016/S2352-3026(20)30109-5
Wang, Y., Dong, C., Hu, Y., Li, C., Ren, Q., Zhang, X., et al. (2020). Temporal changes of CT findings in 90 patients with COVID-19 pneumonia: a longitudinal study. Radiology 296:E55–E64. doi: 10.1148/radiol.2020200843
Weil, B. R., Manukyan, M. C., Herrmann, J. L., Wang, Y., Abarbanell, A. M., Poynter, J. A., et al. (2010). Mesenchymal stem cells attenuate myocardial functional depression and reduce systemic and myocardial inflammation during endotoxemia. Surgery 148, 444–452. doi: 10.1016/j.surg.2010.03.010
Weiss, M. L., Anderson, C., Medicetty, S., Seshareddy, K. B., Weiss, R. J., VanderWerff, I., et al. (2008). Immune properties of human umbilical cord Wharton's jelly-derived cells. Stem Cells 26, 2865–2874. doi: 10.1634/stemcells.2007-1028
Willis, G. R., Fernandez-Gonzalez, A., Anastas, J., Vitali, S. H., Liu, X., Ericsson, M., et al. (2018). Mesenchymal stromal cell exosomes ameliorate experimental bronchopulmonary dysplasia and restore lung function through macrophage immunomodulation. Am. J. Respir. Crit. Care Med. 197, 104–116. doi: 10.1164/rccm.201705-0925OC
Wilson, J. G., Liu, K. D., Zhuo, H., Caballero, L., McMillan, M., Fang, X., et al. (2015). Mesenchymal stem (stromal) cells for treatment of ARDS: a phase 1 clinical trial. Lancet Respir. Med. 3, 24–32. doi: 10.1016/S2213-2600(14)70291-7
Winkler, T., Perka, C., von Roth, P., Agres, A. N., Plage, H., Preininger, B., et al. (2018). Immunomodulatory placental-expanded, mesenchymal stromal cells improve muscle function following hip arthroplasty. J. Cachexia Sarcopenia Muscle. 9, 880–897. doi: 10.1101/297739
Wolbank, S., Peterbauer, A., Fahrner, M., Hennerbichler, S., van Griensven, M., Stadler, G., et al. (2007). Dose-dependent immunomodulatory effect of human stem cells from amniotic membrane: a comparison with human mesenchymal stem cells from adipose tissue. Tissue Eng. 13, 1173–1183. doi: 10.1089/ten.2006.0313
Xu, J., Woods, C. R., Mora, A. L., Joodi, R., Brigham, K. L., Iyer, S., et al. (2007). Prevention of endotoxin-induced systemic response by bone marrow-derived mesenchymal stem cells in mice. Am. J. Physiol. Lung Cell. Mol. Physiol. 293, L131–L141. doi: 10.1152/ajplung.00431.2006
Zhang, C., Yin, X., Zhang, J., Ao, Q., Gu, Y., and Liu, Y. (2017). Clinical observation of umbilical cord mesenchymal stem cell treatment of severe idiopathic pulmonary fibrosis: a case report. Exp. Ther. Med. 13, 1922–1926. doi: 10.3892/etm.2017.4222
Zhang, C., Zhu, Y., Wang, J., Hou, L., Li, W., and An, H. (2019). CXCR4-overexpressing umbilical cord mesenchymal stem cells enhance protection against radiation-induced lung injury. Stem Cells Int. 2019:2457082. doi: 10.1155/2019/2457082
Zhang, Y., Xiao, M., Zhang, S., Xia, P., Cao, W., Jiang, W., et al. (2020). Coagulopathy and antiphospholipid antibodies in patients with Covid-19. N. Engl. J. Med. 382:e38. doi: 10.1056/NEJMc2007575
Zhao, X., Liu, D., Gong, W., Zhao, G., Liu, L., Yang, L., et al. (2014). The toll-like receptor 3 ligand, poly(I:C), improves immunosuppressive function and therapeutic effect of mesenchymal stem cells on sepsis via inhibiting MiR-143. Stem Cells 32, 521–533. doi: 10.1002/stem.1543
Zhou, C., Yang, B., Tian, Y., Jiao, H., Zheng, W., Wang, J., et al. (2011). Immunomodulatory effect of human umbilical cord Wharton's jelly-derived mesenchymal stem cells on lymphocytes. Cell Immunol. 272, 33–38. doi: 10.1016/j.cellimm.2011.09.010
Zhou, F., Yu, T., Du, R., Fan, G., Liu, Y., Liu, Z., et al. (2020). Clinical course and risk factors for mortality of adult inpatients with COVID-19 in Wuhan, China: a retrospective cohort study. Lancet 395, 1054–1062. doi: 10.1016/S0140-6736(20)30566-3
Keywords: coronavirus-induced disease 2019, severe acute respiratory distress syndrome coronavirus-2, mesenchymal stromal cells, PLacental eXpanded, perinatal
Citation: Papait A, Cargnoni A, Sheleg M, Silini AR, Kunis G, Ofir R and Parolini O (2021) Perinatal Cells: A Promising COVID-19 Therapy? Front. Bioeng. Biotechnol. 8:619980. doi: 10.3389/fbioe.2020.619980
Received: 21 October 2020; Accepted: 08 December 2020;
Published: 14 January 2021.
Edited by:
Bruce Alan Bunnell, University of North Texas Health Science Center, United StatesReviewed by:
Yuan Shi, Children's Hospital of Chongqing Medical University, ChinaCopyright © 2021 Papait, Cargnoni, Sheleg, Silini, Kunis, Ofir and Parolini. This is an open-access article distributed under the terms of the Creative Commons Attribution License (CC BY). The use, distribution or reproduction in other forums is permitted, provided the original author(s) and the copyright owner(s) are credited and that the original publication in this journal is cited, in accordance with accepted academic practice. No use, distribution or reproduction is permitted which does not comply with these terms.
*Correspondence: Antonietta R. Silini, YW50b25pZXR0YS5zaWxpbmlAcG9saWFtYnVsYW56YS5pdA==
†These authors share first authorship
‡These authors have contributed equally to this work
Disclaimer: All claims expressed in this article are solely those of the authors and do not necessarily represent those of their affiliated organizations, or those of the publisher, the editors and the reviewers. Any product that may be evaluated in this article or claim that may be made by its manufacturer is not guaranteed or endorsed by the publisher.
Research integrity at Frontiers
Learn more about the work of our research integrity team to safeguard the quality of each article we publish.