- Department of Chemistry, University of Pittsburgh, Pittsburgh, PA, United States
Microdialysis probes, electrochemical microsensors, and neural prosthetics are often used for in vivo monitoring, but these are invasive devices that are implanted directly into brain tissue. Although the selectivity, sensitivity, and temporal resolution of these devices have been characterized in detail, less attention has been paid to the impact of the trauma they inflict on the tissue or the effect of any such trauma on the outcome of the measurements they are used to perform. Factors affecting brain tissue reaction to the implanted devices include: the mechanical trauma during insertion, the foreign body response, implantation method, and physical properties of the device (size, shape, and surface characteristics. Modulation of the immune response is an important step toward making these devices with reliable long-term performance. Local release of anti-inflammatory agents such as dexamethasone (DEX) are often used to mitigate the foreign body response. In this article microdialysis is used to locally deliver DEX to the surrounding brain tissue. This work discusses the immune response resulting from microdialysis probe implantation. We briefly review the principles of microdialysis and the applications of DEX with microdialysis in (i) neuronal devices, (ii) dopamine and fast scan cyclic voltammetry, (iii) the attenuation of microglial cells, (iv) macrophage polarization states, and (v) spreading depolarizations. The difficulties and complexities in these applications are herein discussed.
Introduction
Microdialysis is a powerful technique for near real-time intracranial chemical monitoring in both animal models and human patients (Roberts and Anderson, 1979; Ungerstedt, 1984, 1991; Benveniste et al., 1987, 1989; Benveniste, 1989; Santiago and Westerink, 1990; Carneheim and Stahle, 1991; Parsons et al., 1991; Dykstra et al., 1992; Stenken, 1999; Hutchinson et al., 2000; Chefer et al., 2001; Stenken et al., 2001, 2010; Bosche et al., 2003, 2010; Schuck et al., 2004; Ungerstedt and Rostami, 2004; Parkin et al., 2005; Shou et al., 2006; Mitala et al., 2008; Hashemi et al., 2009; Jaquins-Gerstl et al., 2011; Wang and Michael, 2012; Zhang et al., 2013; Nesbitt et al., 2015; Dreier et al., 2016). A key advantage of microdialysis is its broad scope: it has been used to monitor neurotransmitters, peptides, amino acids, metabolites, and hormones, etc. This is possible because the only requirement is that the molecule of interest be smaller than the molecular weight cutoff of the chosen membrane material. As probes are perfused with, typically, an artificial cerebrospinal fluid solution (aCSF), small molecules enter the probe by passive diffusion and are swept to the probe outlet. The outlet is either interfaced directly to an analytical system, such as liquid chromatography, capillary electrophoresis, or mass spectrometry for on-line analysis, or discrete dialysate samples are collected and stored for later off-line analysis. Because microdialysis is highly compatible with awake-behaving animals, numerous studies have examined the relationships between chemical events in the extracellular space of the brain and animal behaviors (Becker and Cha, 1989; Robinson and Justice, 1991; Castner et al., 1993; Borjigin and Liu, 2008). Microdialysis also finds use in anesthetized or sedated patients receiving intensive care, after traumatic brain injury for example (Globus et al., 1995; Strong et al., 2002, 2005, 2007; Ungerstedt and Rostami, 2004; Westerink and Cremers, 2007; Haddad and Arabi, 2012; Sanchez et al., 2013; Rogers et al., 2017; Booth et al., 2018; Pagkalos et al., 2018; Gowers et al., 2019).
Slow temporal resolution is often mentioned as a limitation of microdialysis. It is important, however, to consider the factors that limit temporal resolution. Often, extended sample collection times are necessary to assure that a detectable quantity of target analyte is available. Thus, over time, various workers have improved the temporal resolution of microdialysis by developing refined analytical methods with lower detection limits. For example, the Kennedy group has developed numerous rapid dialysate analyses by on-line capillary liquid chromatography or on-line capillary electrophoresis (Watson et al., 2006; Li et al., 2009; Perry et al., 2009; Wang et al., 2010; Lee et al., 2013; Kennedy et al., 2018; Ngernsutivorakul et al., 2018). The Andrews and Weber groups have used capillary liquid chromatography to analyze dopamine and serotonin in brain dialysates with minute and sub-minute temporal resolution (Liu et al., 2010; Yang et al., 2013; Zhang et al., 2013; Gu et al., 2015; Wilson and Michael, 2017).
Past observations from our laboratory have suggested, further, that an additional limitation of the temporal resolution of microdialysis-based monitoring stems from the so-called penetration trauma of the tissue surrounding the track of the microdialysis probe itself. Early experiments in the Michael lab attempted to monitor electrically evoked dopamine release in the rat striatum using a dopamine-sensitive microelectrode interfaced to the outlet line of a microdialysis probe (Lu et al., 1998; Yang and Michael, 2007; Nesbitt et al., 2013; Varner et al., 2016). In vitro characterization of the probe-microelectrode combination suggested a temporal resolution near 30 s, sufficiently rapid to monitor evoked dopamine responses recorded at microelectrodes implanted directly in brain tissue. However, evoked responses were not observable at the probe outlet without the aid of a dopamine uptake inhibitor, nomifensine (Borland et al., 2005). Eventually, we performed experiments with microelectrodes implanted side-by-side with microdialysis probes: without the aid of the uptake inhibitor there was, unexpectedly, no evoked response in the tissue surrounding the probe. Thus, our failure to detect evoked dopamine release at the probe outlet was not a matter of temporal resolution but rather was a matter of tissue disruption: there was no evoked response taking place in the surrounding tissue (Borland et al., 2005). This finding raised our initial concerns that the tissue surrounding the probe was in an abnormal, most likely traumatized, state and prompted our subsequent focus on the issue of penetration trauma and, eventually, strategies to mitigate it. These strategies are the focus of this review article.
A key, and somewhat unique, strategy available to the mitigation of penetration trauma induced by the implantation of microdialysis probes is so-called “retrodialysis” (Stenken, 1999; Shippenberg and Thompson, 2001; Cano-Cebrian et al., 2005). Retrodialysis is the term coined for the delivery of substances to brain tissues via the microdialysis probe itself. The focus of this review is our experience with dexamethasone (DEX)-enhanced microdialysis, which involves the retrodialysis of DEX to the probe track (Schuck et al., 2004; Nesbitt, 2015; Nesbitt et al., 2015; Kozai et al., 2016). DEX is a very well-known anti-inflammatory agent that has proven, thus far, highly effective at mitigating probe-induced penetration trauma during intracranial microdialysis in both the rat striatum and the rat cortex.
Dexamethasone-enhanced microdialysis offers key and specific benefits. First, it has facilitated the detection of evoked dopamine release at the probe outlet, resolving the technical difficulty described above. Second, we have found that DEX-enhanced microdialysis offers stabilized monitoring performance in brain tissue for at least 10 days following probe insertion. We hope that this improvement in longitudinal monitoring capability will be a benefit especially to patients who require neuromonitoring after traumatic brain injury.
Of course, DEX is not just an anti-inflammatory agent: it is steroid with its own potential neurochemical effects, raising the possibility that it might alter the outcome of neurochemical investigations. This, of course, must be acknowledged and addressed. As will be discussed later in this review, we have found that it is not necessary to deliver DEX continuously throughout an extended period of microdialysis monitoring. Preliminary evidence suggests that DEX retrodialysis is mainly needed at the time of probe insertion and that its benefits last beyond the termination of retrodialysis (Nesbitt, 2015; Nesbitt et al., 2015; Kozai et al., 2016; Varner et al., 2016). This is a matter that potential adopters of DEX-enhanced microdialysis will need to evaluate in the future in the context of their own particular intended applications.
Principles of Microdialysis
The underlying process driving microdialysis sampling is the passive diffusion of substances across a semi-permeable hollow-fiber dialysis membrane (Stenken et al., 1997, 2001, 2010; Stenken, 1999; Chefer et al., 2001; Bungay et al., 2007; Chaurasia et al., 2007; Huang et al., 2008; Darvesh et al., 2011). Various polymeric materials, with molecular weight cutoff values between 10 and 100 kDa, are available for probe construction (Stenken, 1999; Chefer et al., 2001; Bungay et al., 2007; Darvesh et al., 2011; Hammarlund-Udenaes, 2017). The overall sampling efficiency, however, is influenced by the membrane and the tortuosity and volume fraction of the brain tissue, the tendency of analyte molecules to stick to the membrane or connecting tubing, the stability of the analyte molecule, etc. (Torto et al., 1999). The probes are commonly between 200 and 500 μm in external diameter and several millimeters in length, chosen to match the target brain structure. The dialysate concentration of a target analyte is proportional, but not quantitatively equal, to its concentration in the surrounding extracellular space (Ungerstedt, 1991). The analyte concentration at the probe outlet (COUT) reflects two contributions, one derived from the external medium (CEXT), and one derived from the probe inlet (CIN) if analyte retrodialysis is ongoing. The relationship between these quantities is:
where E is the extraction fraction and R is the so-called relative recovery: the term CEXT,∞ denotes the concentration of analyte in the external medium sufficiently far from the probe so as not to be disturbed by the probe per se. “Conventional microdialysis” refers to the case that CIN = 0. A common rearrangement of Eq. 1 leads to the concentration differences plot:
Equation 2 shows that a plot of CIN–COUT (the concentration differences) against CIN is expected to be a straight line, with a slope of E and an x-intercept of RCEXT,∞. While such a plot provides the value of the extraction fraction, the relative recovery cannot generally be measured without independent knowledge of the external concentration. Thus, while in vitro probe calibration is straightforward, generally the value of R is an uncertain quantity during in vivo measurements. It is now generally recognized that R values determined during in vitro calibration are not reliably applicable to in vivo conditions: investigators need to keep this in mind. Our preference, and recommendation, is to report “dialysate concentrations” of analytes of interest, without attempting to convert to in vivo concentrations. A number of mathematical models have been developed in an effort to theoretically predict in vivo R values (Amberg and Lindefors, 1989; Benveniste, 1989; Lindefors et al., 1989; Bungay et al., 1990, 2003, 2007) but such work is beyond the scope of this review.
Inflammation
Penetration Trauma
The average spacing of the microvessels in brain tissues is around 50 μm and a number of larger blood vessels are also present. Inevitably, therefore, insertion of microdialysis probes and other neural devices such as microelectrode arrays with dimensions greater than 50 μm induces penetration trauma (Benveniste and Diemer, 1987; Zhou et al., 2001). In one of our first studies using immunohistochemistry we labeled blood vessels with dye-laden polystyrene nanobeads (100 nm in diameter) delivered to the brain by transcardial perfusion and the antibody platelet endothelial cell adhesion molecule (PECAM), a histochemical marker for endothelial cells. In healthy tissue the blood vessels were double-labeled with nanobeads and PECAM. However, tissue near the microdialysis probe tracks exhibited ischemia (diminished blood flow), in the form of PECAM immunoreactivity and blood vessels devoid of nanobeads, Figure 1A (Mitala et al., 2008). Probe tracks were surrounded by endothelial cell debris, which appeared as a diffuse halo of PECAM immunoreactivity and there was a large region that was devoid of the nanobeads indicating a lack of blood flow (Jaquins-Gerstl and Michael, 2009).
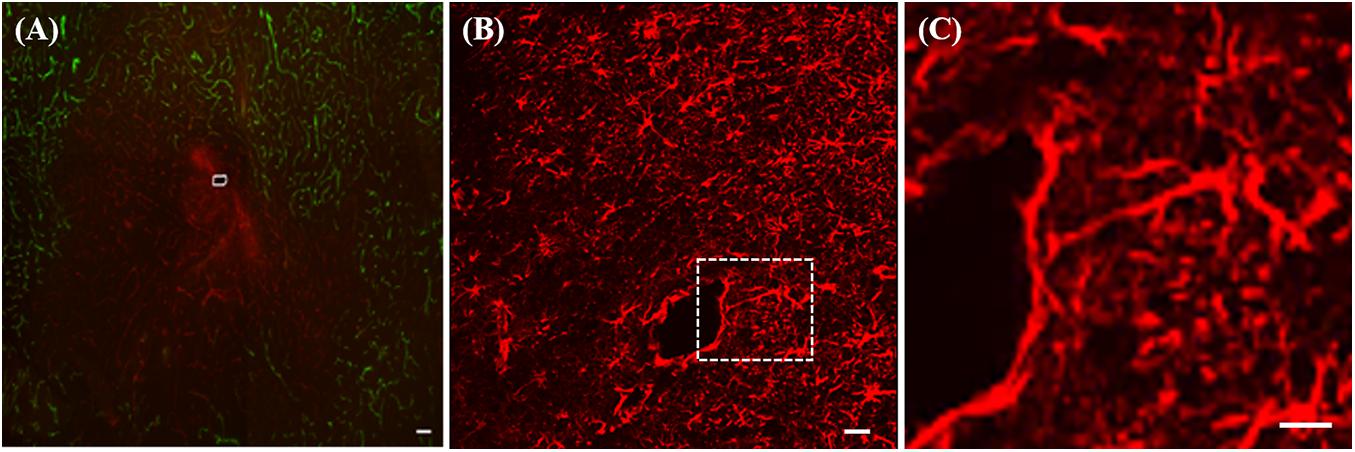
Figure 1. (A) Fluorescence image of ischemia (green-blood vessels) and a PECAM (red) halo at a microdialysis probe track (white marking) in rat striatum. (B) GFAP immunoreactivity after 24-h probe implant, (C) enlargement of the area in the white box in B showing a glial cell extending a process ∼300 μm toward the track. All scale bars = 100 μM. Adopted from references (Mitala et al., 2008; Jaquins-Gerstl and Michael, 2009).
Moreover, the penetration trauma triggers a tissue response: sometimes called a foreign body response or a wound-healing response. The tissue response, left unattended, leads to gliosis of the probe track. Within a few days of insertion, microdialysis probes are surrounded by a layer of activated glial cells that do not exhibit the same neurochemical responsiveness of normal, healthy brain tissue, Figures 1B,C (Jaquins-Gerstl et al., 2011). Microdialysis probe tracks are surrounded by glia exhibiting marked hyperplasia and hypertrophia, Figure 1B. A closer view, Figure 1C, shows that the track is encircled around most of its circumference (∼75%) by a barrier of GFAP immunoreactive elements, revealing that glial encapsulation and isolation of the probe is underway 24 h after implantation. The field of view in Figure 1C prominently displays a glial cell extending a process in excess of 300 μm in a linear fashion from the cell body toward the probe track.
We are not the first to report the penetration injury associated with microdialysis probes. Drew’s work examined the tissue surrounding microdialysis probes implanted in the striatum by light microscopy and revealed tissue damage 1.4 mm and neuronal loss 400 μm from the probe track (Clapp-Lilly et al., 1999). Drew also used hibernation as a model of neuroprotection by placing microdialysis probes into the striatum of the Arctic ground squirrel. Activated microglia and astrocytes were dramatically attenuated around the probe tracks in hibernating animals compared to euthermic controls (Zhou et al., 2001). Not only does the large size of the microdialysis probe cause damage, but the tissue response to the probe also contributes to the severity of the injury.
Implantation results in activation of biochemical and cellular mechanisms to heal the injury. The initial response is initiated as soon as a device is implanted. It may last from minutes to weeks depending on the injury (Szarowski et al., 2003). A host of immune processes are set into motion which have been well-documented in current literature. Following penetration injury from the microdialysis probe, the blood brain barrier is compromised due to vascular damage, and blood-borne macrophages enter the brain and become indistinguishable from resident microglia. Microglia/macrophages then transform morphologically and functionally into an active state, home toward the site of injury, and are involved in phagocytosis and debris clearance (Stence et al., 2001; Kozai et al., 2012, 2015, 2016, 2017; Bettinger et al., 2020). In addition, microglia and blood-borne macrophages can release reactive oxygen species, which can damage healthy bystander cells such as neurons.
From our own studies we know that microdialysis probe implantation causes inflammation: it restricts blood flow (ischemia), activates macrophages, reduces neurons, diminished dopamine terminal, and axons and triggers gliosis, shown in Figure 2 (Jaquins-Gerstl and Michael, 2009; Nesbitt et al., 2013). Therefore, the tissue sampled by microdialysis is not in its normal state. Even though the injury associated with microdialysis probe implantation is significant, it is important to note that overall brain function and animal behavior does not change (Jaquins-Gerstl and Michael, 2009; Nesbitt et al., 2013). Efforts to reduce the tissue response associated with neural device implantation have been made and are further discussed in this manuscript.
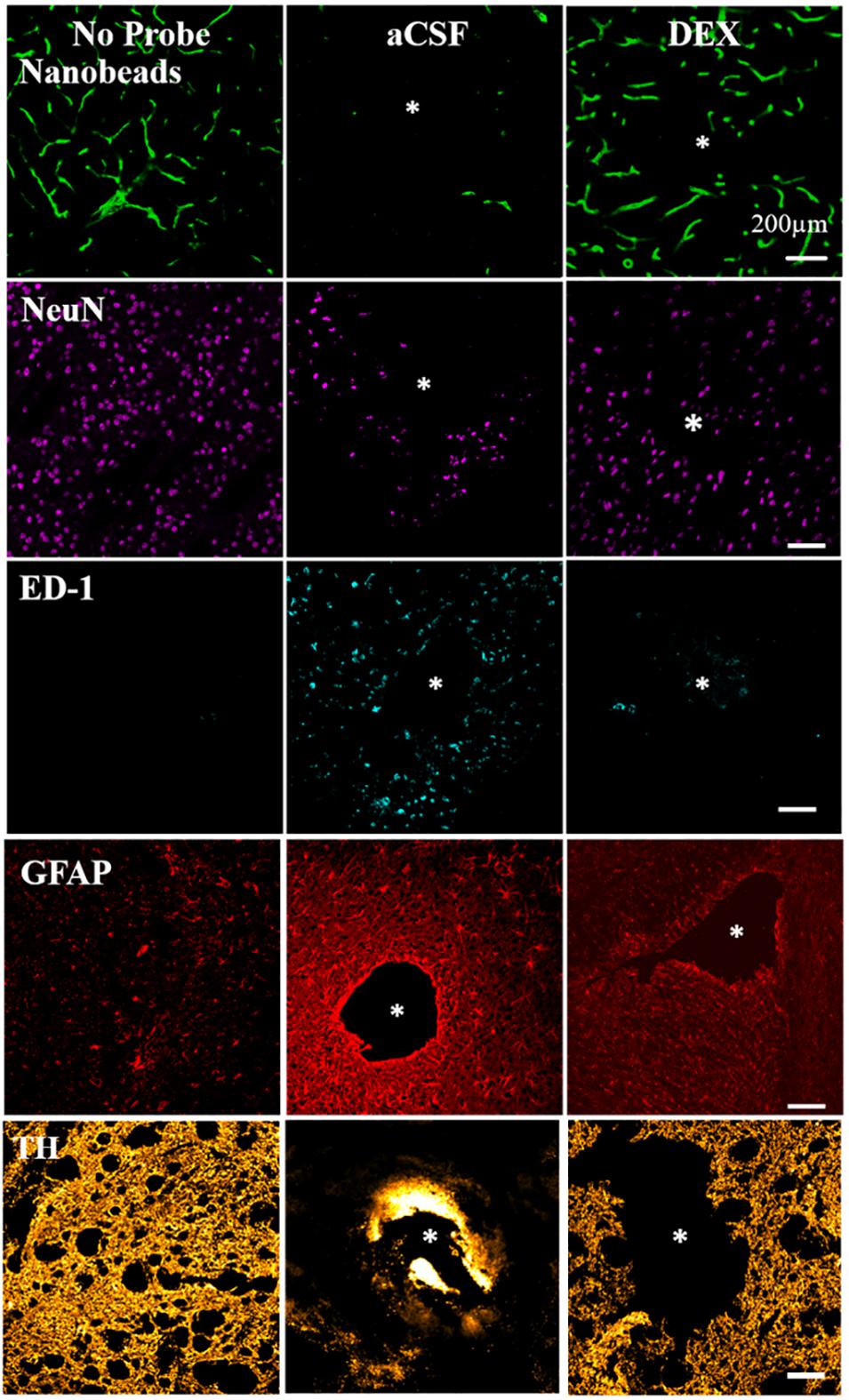
Figure 2. DEX mitigates the histochemical impact of penetration injury. Separate columns provide representative images of tissue after retrodialysis of aCSF, and DEX for 4 h. The left-most column shows images of non-implanted control striatal tissue. Separate rows provide representative images of tissue labeled with markers for blood flow (nanobeads), neuronal nuclei (NeuN), macrophages (ED1), glial fibrillary acidic protein (GFAP), and dopamine axons and terminals (TH). The probe track is in the center of the asterisk. Scale bar = 200 μm. Adopted from reference (Jaquins-Gerstl and Michael, 2009).
Strategies to Reduce Inflammation
Inflammation is a complex reaction involving protein adsorption, leukocyte migration, localization and activation, and secretion of inflammatory mediators (Ghirnikar et al., 1998; Lefkowitz and Lefkowitz, 2001; Zhong and Bellamkonda, 2005; Klueh et al., 2007; Anderson et al., 2008; Winslow and Tresco, 2010; Chao et al., 2012). The degree of intensity of this response is largely influenced by the extent of tissue injury, implantation site, implant shape and size, and chemical and physical properties of the membrane material (Bridges and García, 2008). Strategies to mediate inflammation are a subject of much interest. It is thought that preventing non-specific protein adsorption and subsequent immune cell adhesion onto the biomaterial surface (Wisniewski and Reichert, 2000; Wisniewski et al., 2000; Bridges and García, 2008; Helmy et al., 2009) can reduce leukocyte recruitment and tissue fibrosis (Gerritsen et al., 1999; Gifford et al., 2006). Although this has proven successful in vitro, implementing these in vivo have not translated into decreasing the inflammatory responses (Wisniewski et al., 2001; Polikov et al., 2005).
A more direct and active approach regulating the tissue response has been through the delivery of anti-inflammatory agents (Nakase et al., 2002; Zhong and Bellamkonda, 2005, 2007; Kim et al., 2007; Klueh et al., 2007; Nichols et al., 2012; Wang et al., 2012; Zachman et al., 2012; Koh et al., 2013). One such anti-inflammatory agent used in this area is glucocorticoid steroids. Glucocorticoid steroids are a class of steroids that bind to the glucocorticoid receptor which regulates inflammation; they have been used extensively to treat inflammatory conditions (Chao et al., 2012; Webber et al., 2012).
Dexamethasone is a potent synthetic glucocorticoid associated with diminished migration and activation of immune cells, upregulation of anti-inflammatory cytokines, and decreased collagen production at the implant site (Schmidt et al., 1999; Hickey et al., 2002; Norton et al., 2005; Klueh et al., 2007; Chao et al., 2012). Once DEX crosses the cell membrane it binds to specific cytoplasmic receptors such as the glucocorticoid receptors and then moves into the nucleus (Patacsil et al., 2016). This ability to cross cell membranes results in interference of leukocyte infiltration at the inflammation site along with inhibiting other inflammatory mediators.
Like many glucocorticoids, DEX has limited solubility. To overcome this problem, manufacturers have formulated DEX as water soluble hemisuccinate or phosphate ester pro-drugs. DEX 21-phosphate disodium (Figure 3), a phosphate ester pro-drug of DEX, is converted to active steroid DEX in blood rapidly and completely. DEX is used to treat many different inflammatory conditions such as allergic reactions, skin conditions, ulcerative colitis, arthritis, lupus, psoriasis, and breathing disorders. Most recently, it was used to treat patients hospitalized with COVID-19 (Lammers et al., 2020; Stauffer et al., 2020).
Applications
Dexamethasone has a wide variety of uses in the medical and science field and is widely prescribed. This section discusses applications of DEX used in science as an anti-inflammatory drug, see Table 1 for a summary.
DEX and Neuronal Devices
Several studies have examined the use of DEX with regard to neural prosthetics. In 2003, Shain et al. (2003) published a study using DEX to reduce gliosis near implanted silicone devices in the brain. Peripheral injections of DEX were made by subcutaneous injection. DEX was dissolved in ethanol (0.2 mg/mL) and delivered to produce a dose of 200 mg/kg. This study revealed that DEX reduced gliosis near the implanted device. However, the effects of the drug on the major inflammatory cells at the interface, which were macrophages, were not investigated.
Later in 2005, Spataro et al. (2005) injected DEX (200 mg/kg) for 1 or 6 days subcutaneously and showed the tissue reaction around neural implants was reduced. DEX treatment greatly attenuated astroglia responses. Cohorts with 6-day treatment showed this was more effective than a single injection regime.
Others have used DEX (100 μg) incorporated into a nitrocellulose coating deposited on electrodes for local drug delivery (Zhong and Bellamkonda, 2007). The local delivery of DEX reduced inflammation at 1-week post implantation but not at 4 weeks (Zhong and Bellamkonda, 2007), possibly because the amount of drug incorporated into the coating was not high enough for long-term release at a sufficient dosage.
Dexamethasone has been used to down regulate nitric oxide production which protects neurons (Zha et al., 2011), (Hempen et al., 2002), inhibits astrocyte proliferation and inhibited proliferation of NG2 cells (Kim and Martin, 2006). Because DEX can have serious side effects other studies have incorporated DEX into different probe coatings; poly(ethyl-vinyl) acetate, nitrocellulose, carbon nanotubes, and poly (lactic-co-glycolic acid) nanoparticles within alginate hydrogel matrices (Hempen et al., 2002; Kim and Martin, 2006; Zhong and Bellamkonda, 2007; Mercanzini et al., 2010; Luo et al., 2011). Benefits common to all the studies included decreased astrocytic response, reduced microglial/macrophage activity, mitigated neuronal loss, and minimized chondroitin sulfate proteoglycan expression.
Dopamine Microdialysis and Detection With Fast Scan Cyclic Voltammetry
Dopamine (DA) is one of the analytes commonly sampled with microdialysis. DA is an important neurotransmitter with numerous roles in normal brain function and it is also involved in a variety of disorders including substance abuse, schizophrenia, and Parkinson’s disease (Lotharius and Brundin, 2002; Phillips et al., 2003; Schultz, 2007; Brisch et al., 2014). Microdialysis coupled to fast scan cyclic voltammetry (FSCV) is a method commonly used in our lab and many others for studying DA in vivo (Garris and Wightman, 1995; Robinson et al., 2003; Michael and Borland, 2006).
Previous studies from our lab demonstrate that the penetration trauma associated with microdialysis profoundly changes DAergic activity near the probe site. We placed voltammetric microelectrodes in the tissue adjacent to the probes. We compared DA as measured with microelectrodes placed 1 mm from the probes, 235 μm from the probes, and immediately adjacent to the probes, called a dialytrode. These recording locations produce dramatically different results, revealing a previously unrecognized 1000-fold gradient of DA activity across the traumatized tissue layer. DA levels measured 1 mm from the probe were in the micromolar range, whereas DA levels 235 μm from the probes were in the nanomolar range and the DA response at the dialytrode was completely abolished (Borland et al., 2005; Yang and Michael, 2007). Only after the administration of a dopamine uptake inhibitor nomifensine, (20 mg/kg i.p.) was DA detectable at the dialytrode. We questioned “why does penetration trauma of the microdialysis probe have an extreme impact on in vivo measurements of DA?”
One of our group’s objectives was to reduce, if not eliminate, the penetration injury and its deleterious effects on neurochemical monitoring. Motivated by the findings of Shain and others (Turner et al., 1999; Shain et al., 2003), we investigated the retrodialysis of DEX in the rat striatum. We showed that combining a 5-day post-implantation wait period with continuous retrodialysis of a low-micromolar concentration of DEX vastly reduces both the voltammetric and histological signs of the penetration injury, Figure 4 (Varner et al., 2016). DA measurements were taken at the outlet of the probe. At 5-days after probe implantation, retrodialysis of DEX: (1) reinstates normal evoked DA release activity in the tissue adjacent to the probe, (2) facilitates robust detection of evoked DA release, (3) establishes quantitative agreement between evoked DA measured simultaneously at the probe outlet and in the tissue next to the probe, (4) reinstates normal immunoreactivity for tyrosine hydroxylase and the dopamine transporter near the probe, and (5) prevents glial scarring at the probe track (Varner et al., 2016). Our findings support that the beneficial effects of DEX in this application may be attributed to its actions as an anti-inflammatory agent.
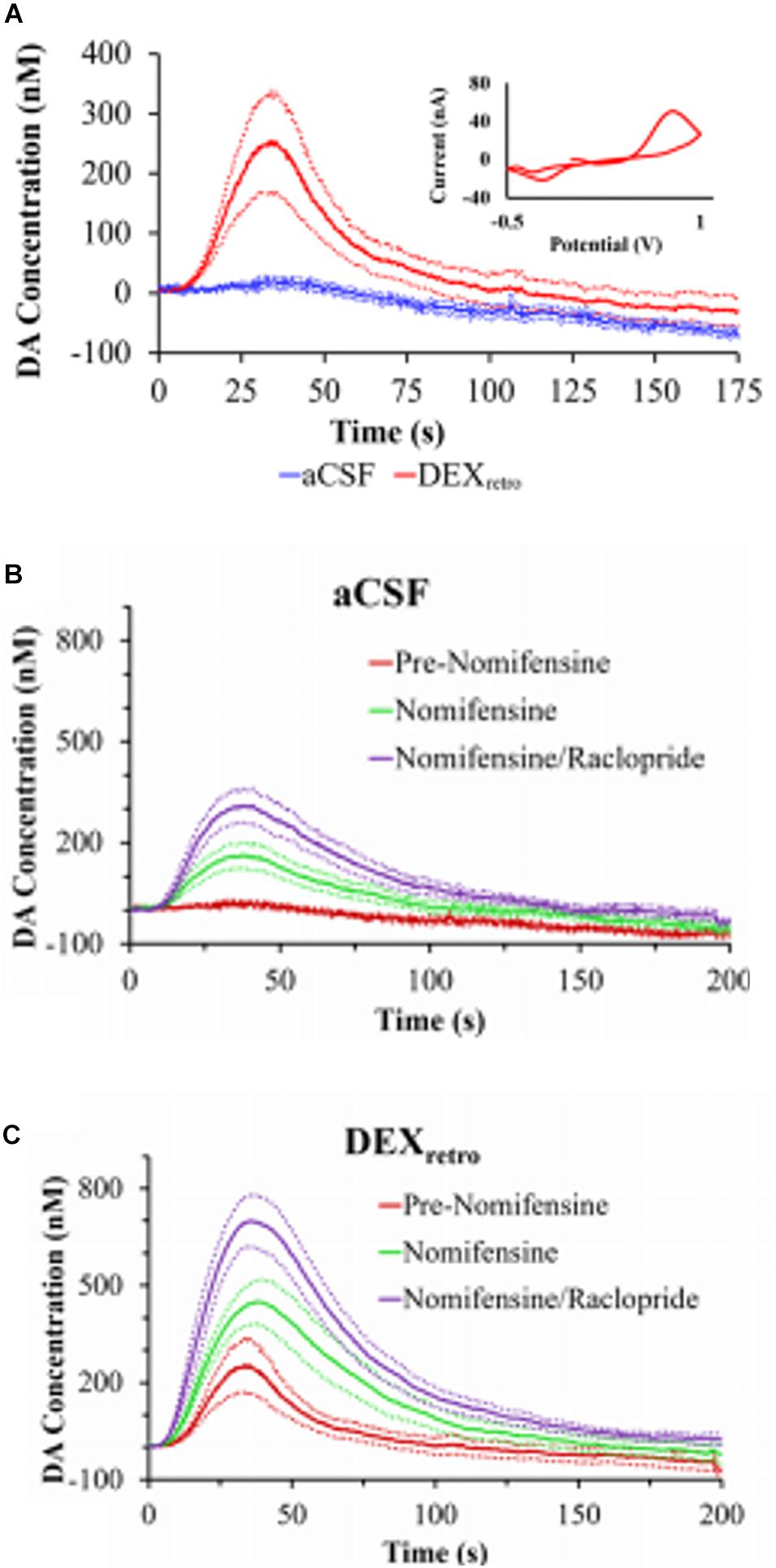
Figure 4. (A) Evoked DA responses (mean ± SEM) recorded at the outlet of microdialysis probes 5 days after implantation. Without DEX (blue) the stimulus evoked no response. With DEX (red), the stimulus evoked clear and reproducible responses. Inset: the average background-subtracted cyclic voltammogram obtained with DEX, showing the expected DA oxidation and reduction peaks. (B) Evoked DA responses (mean ± SEM) measured at the outlet of microdialysis probes perfused with aCSF or (C) DEX. DA was measured before nomifensine (red), after nomifensine (green), and then again after raclopride (purple). Adopted from reference (Varner et al., 2016).
We also used voltammetry next to microdialysis probes to record electrically evoked DA release during the retrodialysis of DEX (Nesbitt et al., 2013). In this study a carbon fiber voltammetric electrode was inserted into the striatum of an anesthetized rat and a stimulating electrode was lowered into the medial forebrain bundle (MFB), Figure 5 (Nesbitt et al., 2013). Electrically evoked release was recorded by FSCV during electrical stimulation of the MFB. The final position of the probe was such that the distance between the tip of the microelectrode and the surface of the probe was 70 μm and the distance between the top of the electrode and the probe was 100 μm. This investigation focused on acute implants only 4 h in duration. We observed that microdialysis probes disrupt evoked DA release. If microdialysis probes were perfused with no DEX, all the electrically evoked DA responses were abolished (Nesbitt et al., 2013). DA was not detected during any of the electrical stimuli applied after implanting the probe. Next, a dose of nomifensine (20 mg/kg i.p.) was given to the rats which caused stimulated DA release. In the case of microdialysis probes perfused with DEX, implanting the probe next to the microelectrode diminished, but did not abolish, electrically evoked DA release. Again, these results confirm that DEX preserved DA activity in the tissue next to the microdialysis probes.
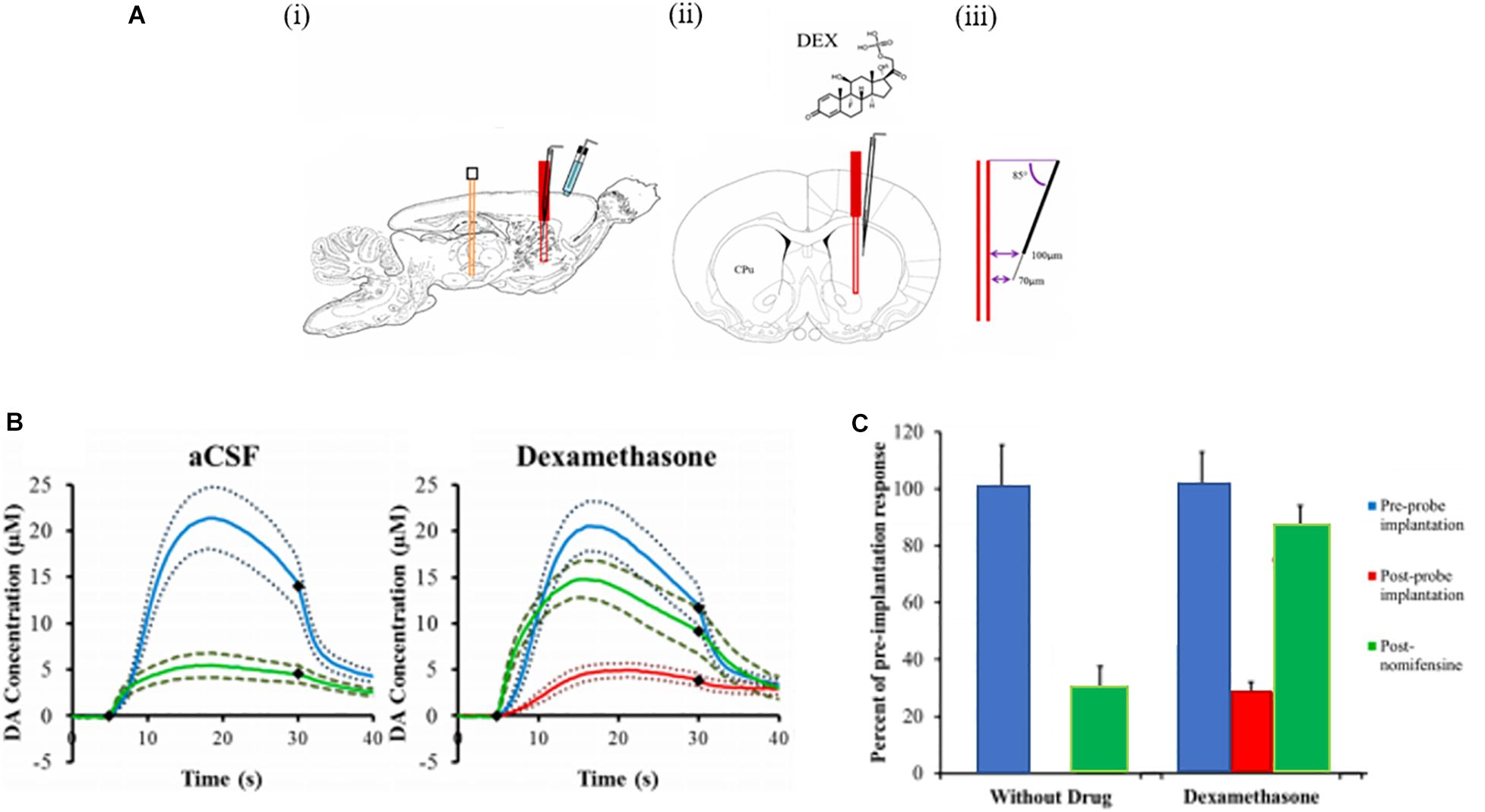
Figure 5. (A) A schematic of the placement of the devices in the rat brain. (i) A sagittal view of the stimulating electrode (orange) in the MFB, the microelectrode (black) and the microdialysis probe (red) in the striatum (CPu), and the Ag/AgCl reference electrode (blue) in contact with the brain surface. (ii) A coronal view showing the microelectrode at a 5° angle from the probe. (iii) The tip of the carbon fiber is 70 μm from the probe and the top of the fiber is 100 μm from the probe. (B) The effect of aCSF and DEX on electrically evoked DA responses measured before implanting the probe (blue lines), 2 h and 40 min after implanting the probe (red lines, the response was non-detectable in aCSF, and 25 min after nomifensine (green lines). The solid lines are the average responses in each group of rats and the broken lines are confidence intervals based on the standard error of the mean of each data point. The black diamonds show when the stimulus begins and ends. (C) The amplitude of evoked DA responses in the rat striatum in the presence of probes perfused with aCSF and DEX. The response amplitudes observed after implanting the probes (red) and after nomifensine (green) are normalized with respect to the amplitude observed before implanting the probes (blue = 100%). The bars show the mean ± SEM of the normalized results. DEX significantly increased evoked DA after implanting the probe. Adapted from reference (Nesbitt et al., 2013).
Immunohistochemistry was performed on brain tissue containing the probe using markers for ischemia, neuronal nuclei, macrophages, and DA axons and terminals (tyrosine hydroxylase). Probes perfused with no DEX caused profound ischemia, a loss of striatal neurons near the probes, activation of macrophages and a loss of tyrosine hydroxylase meaning profoundly disrupted DA axons and terminals (Nesbitt, 2015). Tissue with DEX perfusion showed a decrease in ischemia, neurons near the probe and few activated macrophages, indicating that DEX protected the brain tissue near the probe. Tyrosine hydroxylase was preserved in tissue surrounding the probe with DEX. We conclude that retrodialysis of DEX mitigates penetration injury during brain microdialysis (Nesbitt, 2015).
Using a longer time frame, 4 and 24 h, we implanted microdialysis probes in the rat striatum. We used with and without DEX in the perfusion fluid and measured evoked DA release at the outlet of the probes with FSCV. Responses at the probe outlet were below the detection limits of FSCV unless animals were treated with nomifensine, which increases the microdialysis recovery of evoked dopamine transients (Church et al., 1987; Pontieri et al., 1995). When probes were perfused without DEX, post-nomifensine responses at the probe outlet exhibited a significant decline in amplitude between 4- and 24-h post-implantation. However, DEX abolished this instability, both in animals treated first with nomifensine and then with raclopride, a D2 dopamine receptor antagonist. This study demonstrated that DEX stabilizes, but does not alter, evoked DA responses at the outlet of microdialysis probes.
Dexamethasone and Macrophage Polarization States
Understanding the biochemistry that occurs at the site of an implanted biomaterial is important in a wide range of clinical contexts. Julie Stenken’s group from the University of Arkansas has used microdialysis sampling with DEX to understand the inflammatory response caused by macrophages. Although DEX has widely been used as a releasing agent in biomaterials, Stenken and others have identified DEX as a modulator which produces a phenotype that has characteristics of the M2c macrophage (Van Coillie et al., 1999; Martinez, 2011; Keeler et al., 2015b). Macrophages play roles in opposing processes such as inflammation vs anti-inflammation and tissue destruction vs tissue remodeling. Macrophages are driven by micro-environmental chemical signals present within the extracellular matrix which result in different macrophage polarization states (Gordon and Taylor, 2005; Mantovani et al., 2013; Keeler et al., 2015b). While different materials have been used to elicit a desired macrophage activation state, it is unknown whether modulators can be used to shift the macrophage activation state. Stenken et al. was the first to use modulators to attempt regulating the macrophage activation state at an implant site. The primary hypothesis was that by producing a predominantly M2c activation state, improved healing would be seen at the implant site (Keeler et al., 2015b). In this study, DEX was delivered through the microdialysis probe to alter the activation state of macrophages in awake Sprague-Dawley rats. Two probes were implanted in the subcutaneous space on either side of the spine with ∼1-inch separation between the probes. The delivery of DEX (20 μg/ml) resulted in an increase in the percentage of M2c macrophages seen in the tissue surrounding the microdialysis probe. Remarkably, differences were seen when DEX was delivered immediately following probe implantation as compared to a delayed delivery. In tissue where DEX was immediately delivered, fewer macrophages were present compared to the delayed delivery.
The gene expression profiles of the chemokine CCL2 and the inflammatory cytokine IL-6 were also examined; these too were different. CCL2 is known to be one of the primary chemokines responsible for the migration and infiltration of monocytes to a wound site. Once at the wound site, monocytes differentiate to macrophages. While both CCL2 and IL-6 were significantly down-regulated in the tissue from immediate DEX delivery, IL-6 was seen to be significantly up-regulated in response to a delayed DEX infusion. These results showed that it is possible to use modulators to shift macrophages in vivo to a desired activation state at an implant site while also characterizing a predominantly M2c environment (Keeler et al., 2015b).
The time course required for altering macrophages was also examined by Stenken et al. by delivering DEX through an implanted microdialysis probe in the subcutaneous space of male rats (Keeler et al., 2015a, b). They investigated a 3-day post-implantation time period for initiating DEX infusion. They sought to determine if the start of DEX infusion is delayed, allowing the initial inflammatory response to begin, would be more optimal in terms of converting macrophages to an M(Dex) state. The resulting foreign body response to the implanted microdialysis probes was examined by immunohistochemical and molecular means at the gene and protein level. The delayed delivery of DEX resulted in an upregulation of IL-6 gene transcripts as well as a moderate decrease in CCL2 concentrations (Keeler et al., 2015a, b). The delayed DEX treatment resulted in an increase in cellular density in the tissue surrounding the microdialysis probe. More importantly the delayed delivery of DEX shifted the macrophages to an M(Dex) activation state. Most studies involved in the use of modulators to shift the activation state of macrophages has primarily been in vitro. Stenken’ s work is cutting edge as it demonstrates the use of microdialysis sampling to deliver DEX to alter macrophage polarization in vivo, which improves tissue remodeling.
DEX Retrodialysis Attenuates Microglial Activity
Microglia play a critical role in living brain tissue. They perform a wide range of tasks while in the native ramified state; they are constantly scavenging the CNS for plaques, damaged neurons, and synapses. They are also involved in experience-dependent synaptic maintenance (Wake et al., 2009; Tremblay et al., 2010), debris clearing (Nimmerjahn et al., 2005), and surveillance against injury and invasion (Nimmerjahn et al., 2005). Microglial cells are extremely sensitive to even small pathological changes in the CNS and are also extremely plastic. They adopt a specific form in response to the local conditions and chemical signals they have detected (Nimmerjahn et al., 2005). When a brain insult is detected by microglial cells, they enter an ameboid form called a transition stage (T-stage); this process is generally referred to as “microglial activation.” During this stage, the resting microglia retract their processes, which become fewer and much thicker, increase the size of their cell bodies, change the expression of various enzymes and receptors, and begin to produce immune response molecules. Acutely, following probe insertion, nearby microglia activate and encapsulate the implant with their processes and lamellipodia sheath (Kozai et al., 2012).
We wanted to understand how DEX affects microglia morphology/motility in real time by characterizing the dynamic microglia response to penetration trauma of microdialysis probes. We employed in vivo two-photon microscopy to quantify the acute microglial response to microdialysis probes in the brain with or without retrodialysis of DEX. We examined the cellular microglial response to microdialysis probe insertion up to 6 h; morphological changes and activation characteristics of microglia around the implants were observed and quantified, Figure 6 (Kozai et al., 2016). We found that implantation of the microdialysis probe with DEX reduced microglial activation. In tissue without DEX (aCSF perfused) the activation state of the microglia were delayed and were significant. DEX had a significant effect on the radius of microglia activation, morphology, T-stage activation, and microglia directionality index. The temporal dynamics of microglial response also showed distinct differences between the control and DEX treated tissue. While many microglia appeared non-polarized with unusually thicker processes in the DEX treated tissue at 6 h, some polarized toward nearby blood brain barrier. In this study the local administration of DEX rendered it a great candidate to reduce the effects of penetration injury by neural probes (Kozai et al., 2016).
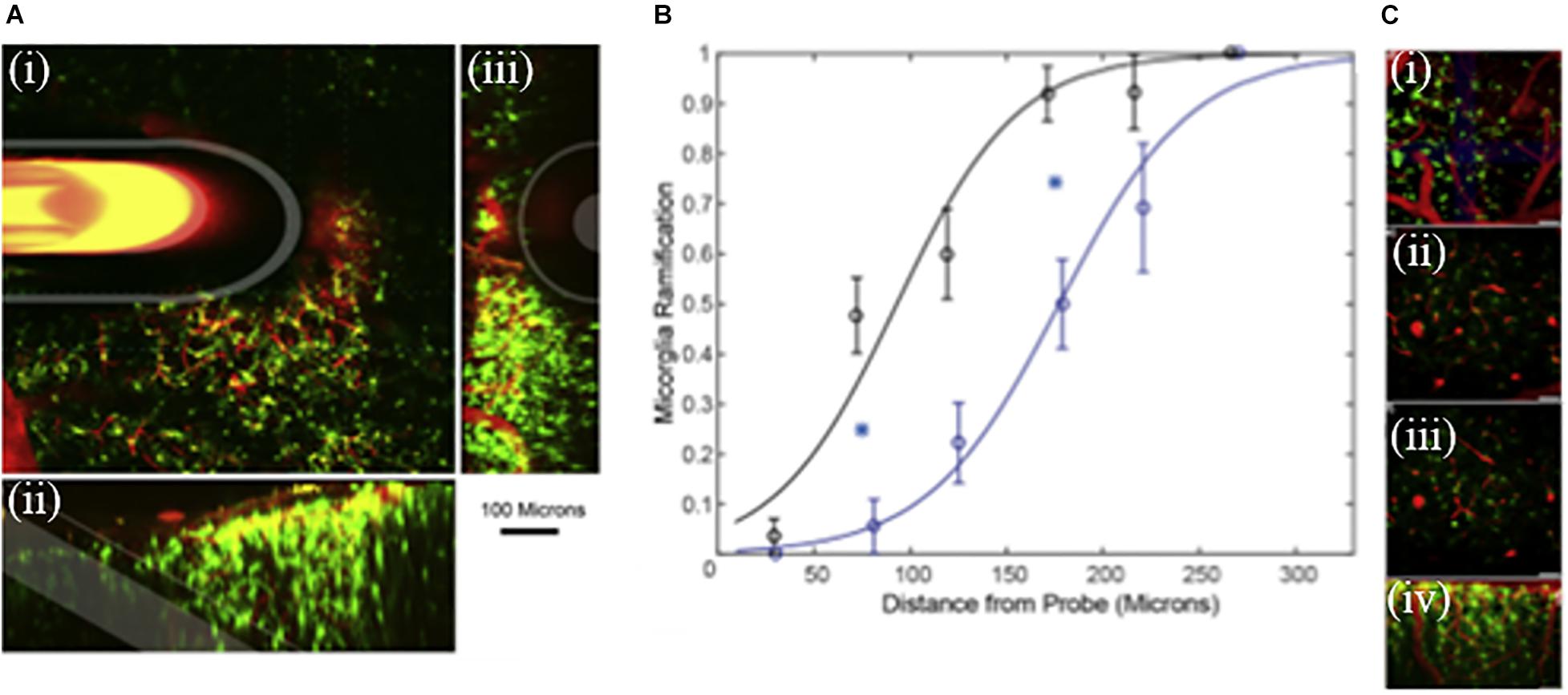
Figure 6. (A) Microdialysis probe in vivo into the cortex. Green represent microglia and red blood vessels. (i) 100 μm thick Z-projection of a microdialysis probe implanted into the tissue. The image is parallel to the skull. Microdialysis, Outer membrane and fused silica tube is highlighted in white. (ii) Side view 3D reconstruction of microdialysis probe. (iii) 3D reconstruction of the tissue rotated; probe is projecting out of the image. Scale = 100 μm. (B) Curve characterizes microglia ramification vs the distance from the probe. Index = 1 represents all microglial cells being ramified, while index = 0 represents all microglial cells being activated around the microdialysis probe perfused with Dex (black, n = 8) and aCSF (blue, n = 8). Line is the best fit logarithmic binomial generalized linear regression curve. Cyan stars indicate significant difference (p < 0.05). (C) Zoomed in images (i–iv) of ramified microglia. Adapted from reference (Kozai et al., 2012).
Spreading Depolarizations and Microdialysis
One application of clinical microdialysis is monitoring brain injured patients in the intensive care unit to identify chemical markers of secondary brain injuries. Our group and others have focused on a phenomenon of secondary injury called spreading depolarization (SD). Incidences of SDs are significantly correlated with poor patient outcomes, including death, vegetative state, and severe disability (Hartings et al., 2009, 2011a, 2011b; Lauritzen et al., 2011). SD is characterized by a wave of near-complete depolarization of neurons and glia resulting in a temporary disruption of the ion homeostasis and silencing of electrical activity (Guiou et al., 2005; Fabricius et al., 2006; Dreier et al., 2006a, b, 2009; Strong et al., 2007; Dreier, 2011; Seule et al., 2015; Rogers et al., 2017). Excess K+ released into the extracellular space during SDs can diffuse to and depolarize neighboring cells, thus creating a wave that propagates across the cortex at a rate of 2–5 mm/min. The brain tissue depends on the vasculature to deliver glucose and oxygen to meet these energy demands. The swelling and restricted blood flow commonly observed after a TBI can hinder this process. Clusters of SDs impose particularly severe energy demands on the injured brain and can result in long-lasting declines in basal glucose (Fabricius et al., 2006; Dreier et al., 2006a; Feuerstein et al., 2010; Nakamura et al., 2010; Lauritzen et al., 2011; Hartings et al., 2011a, b).
The importance of SD monitoring during neurointensive care has been widely recognized and numerous animal studies have been dedicated to improving SD monitoring (Dreier, 2011; Ayata and Lauritzen, 2015; Dreier et al., 2016; Osier and Dixon, 2016). Methods for SD monitoring include electrocortiograph (ECoG), blood flow analysis, and microdialysis. The Boutelle lab has developed a rapid sampling microdialysis (rsMD) system (Figure 7) that monitors SD-associated changes in glucose and lactate at the patient’s bedside (Jones et al., 2002). Combining the rsMD system with an online K+ ion-selective electrode and ECoG provides a multimodal analysis system that can detect episodes of SDs in the days following the patient’s primary injury (Rogers et al., 2013, 2017; Papadimitriou et al., 2016).
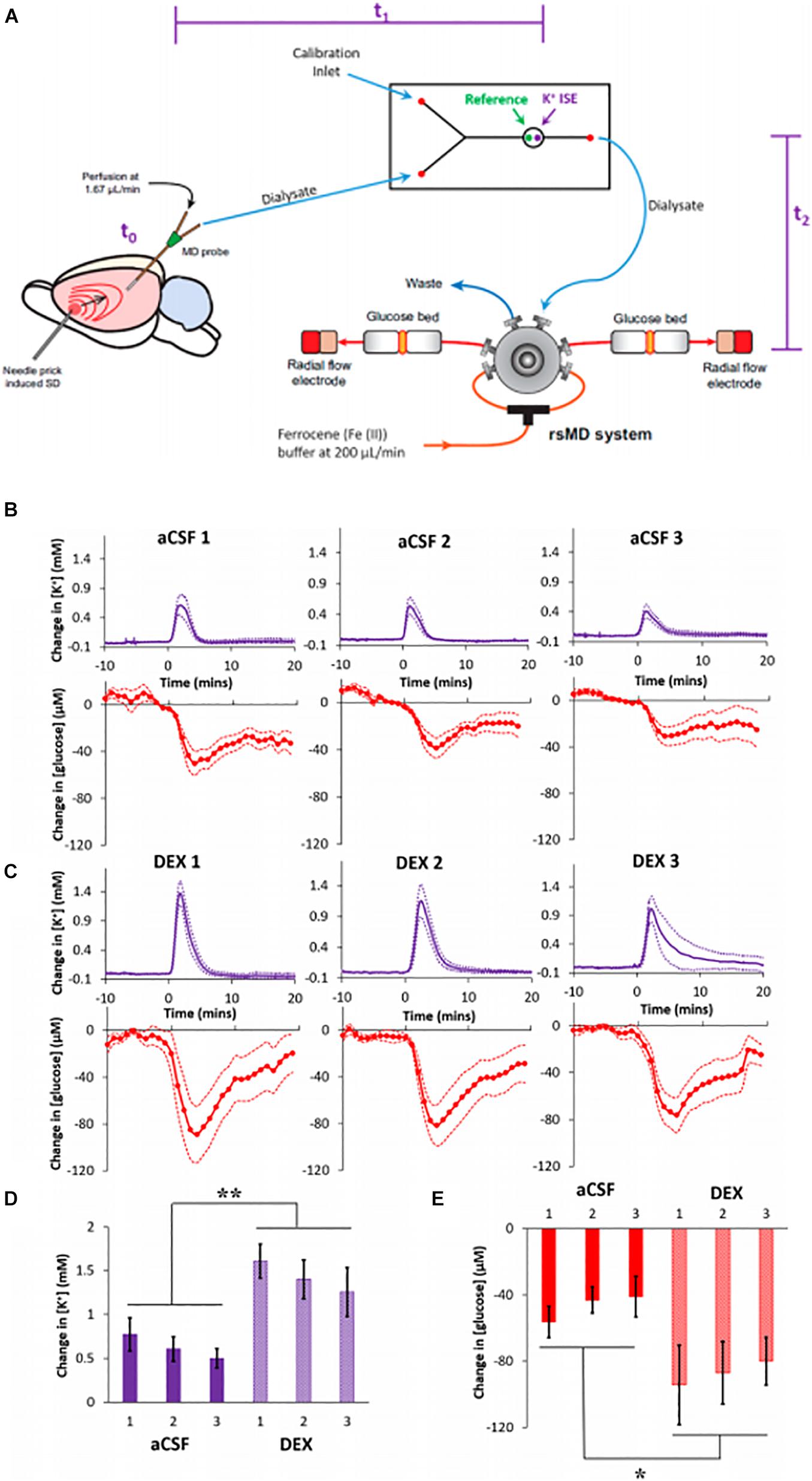
Figure 7. (A) Experimental design of the rsMD. SD was induced by needle pricks in the cortex. The SD arrives at the microdialysis probe at t0: intervals between the needle pricks and t0 were typically less than 1 min. Next, the sample travels to the K + ISE in approximately 4 min, t1. Finally, the sample travels to the glucose detector in approximately 7 min, t2. (B) Cortical responses to three needle pricks recorded 2 h after probe insertion with aCSF or (C) DEX (mean ± SEM). Maximum changes in (D) K + and (E) glucose were analyzed with two-way ANOVAs with group (aCSF, DEX) and needle prick (1, 2, 3; repeated measures) as the factors. The needle prick and interactions were not significant, but group was significant for both K + [F(1,14) = 13.422] and glucose [F(1,14) = 6.253]. **p < 0.005 and *p < 0.05. Adapted from reference (Varner et al., 2017).
Our group reported that DEX conferred profound benefits to the microdialysis monitoring of SDs induced glucose and K+ transients in the rat cortex, Figure 7 (Varner et al., 2017). We inserted microdialysis probes, with and without retrodialysis of DEX, and monitored SD-induced glucose and K+ transients 2 h, 5 days, or 10 days later (Varner et al., 2017). SDs were induced by needle pricks and were performed at 30-min intervals. Retrodialysis of DEX improved the detection of SD-induced transients at all three time points. DEX increased the amplitudes of the SD-induced K+ spikes and glucose dips by 127% and 86% (averages of the three responses), respectively, compared to those observed without DEX. In the presence of DEX, the amplitudes of the K+ spikes were significantly larger at 2 h compared to 5 and 10 days. In contrast, there were no significant differences between the amplitudes of the glucose dips at the three time points. In the presence of DEX, the fraction of K+ spikes accompanied by quantifiable glucose dips was relatively constant across the three time points. In the absence of DEX, glucose dips were essentially non-detectable 5 days after probe insertion.
In our 10-day studies, DEX retrodialysis was performed only during the first 5 days, confirming that continuous DEX delivery for the entire 10-day time window is not required. After retrodialysis of DEX, histochemical inspection of probe tracks found no signs of ischemia or gliosis 10 days after insertion (Varner et al., 2017). Our findings confirmed that DEX enhances the performance of microdialysis for monitoring SD-induced glucose and K+ transients in the rat cortex for at least 10 days after probe insertion.
Our most recent study was to investigate whether the microdialysis with DEX is translatable to the monitoring of spontaneous SD in rats after controlled cortical impact (CCI), a widely studied rodent model of TBI (Dixon et al., 1987; Wagner et al., 2005; Xiong et al., 2013). Microdialysis probes were placed 1 or 3 mm away from the CCI site expecting that the secondary injury would spread over time into the penumbra (Robbins et al., 2019). But this did not happen. There was no difference between 1 and 3 mm location of the probes. We recorded K+ and glucose in dialysates from 10 rats for 10–12 days following CCI and microdialysis probe insertion (Robbins et al., 2019). Overall, recordings from n = 8 rats exhibited 185 spontaneous SDs, hallmarked by a transient rise, and fall in dialysate K+, over the course of 5–7 min. Of the SDs observed while we were also monitoring glucose (n = 126), some were accompanied by negative glucose transients (n = 89), no obvious change in glucose (n = 25), or transient increases in glucose (n = 12). Some rats (n = 2) exhibited no SDs, which seems to be consistent with clinical reports that SDs are detected in some but not all TBI patients who undergo neuromonitoring (Hartings et al., 2011a, b; Dreier et al., 2016).
We also observed a second post-CCI phenomenon consisting of a slow, progressive decline of dialysate glucose from basal concentrations to levels below the detection limit (Robbins et al., 2019). Once this occurred, glucose concentrations did not return to detectable levels. We found a high degree of animal-to-animal variability in the outcome of chemical monitoring after CCI. Individual animals varied widely in the number and frequency of SDs and the onset and duration of progressive glucose decline. We attribute this to the varied extent of the injury induced by CCI. Despite the animal-to-animal variability, this study yielded several consistent observations. Most rats (8 of 10) exhibited spontaneous SDs, either isolated or in clusters. Spontaneous SDs occurred before, during, and after the glucose decline.
Probe tracks were examined by immunohistochemistry and showed no presence of a glial barrier, absence of blood flow, or profound losses of neurons: these observations indicate the anti-inflammatory efficacy of DEX in the presence of CCI. This study adds to a mounting body of evidence that DEX-enhanced microdialysis facilitates extended intracranial microdialysis in the rat brain over the course of at least 10 days.
Outlook
The development of long-term microdialysis is an inspiring yet greatly challenging process. The foreign bodies response toward the penetration trauma associated with the implant is a critical barrier to overcome even with the use of an anti-inflammatory drug such as DEX. We have shown studies where DEX-enhanced microdialysis was used to stabilize the surrounding tissue allowing for better detection of (a) DA, (b) attenuated microglia, (c) reduce gliosis, and (d) enhances the detection of K+ and glucose transients induced by spreading depolarization. DEX improves and stabilizes the tissue surrounding the probe and promotes longevity of the probe.
Current and cutting-edge research is being performed in clinical studies using DEX and microdialysis with the eventual goal of better patient outcomes. There are many types of cells involved in the bodies’ response to the microdialysis implant, although DEX is very successful at damping the immune response. Many questions still arise. To what extent does macrophages traffic across the blood brain barrier following chronic implantation? What is the extent of changes to the local vasculature and the intact blood brain barrier? Are there better strategies for reducing the foreign body response? Are there better suited drugs or probe coating which could be used with microdialysis? Understanding the penetration injury associated with probe implantation and providing protective strategies promotes long-term sampling by microdialysis and plays a lasting role in understanding the neurochemistry of the brain. The future of long term microdialysis is unlimited.
Author Contributions
AJ-G and AM conceived the overall topics of discussion. AJ-G wrote the sections. Both authors read and approved the final manuscript.
Funding
Salary support was provided by the authors through the NIH grants R01NS102725.
Conflict of Interest
The authors declare that the research was conducted in the absence of any commercial or financial relationships that could be construed as a potential conflict of interest.
References
Amberg, G., and Lindefors, N. (1989). Intracerebral microdialysis: II. Mathematical studies of diffusion kinetics. J. Pharmaco.l Methods 22, 157–183. doi: 10.1016/0160-5402(89)90012-0
Anderson, J. M., Rodriguez, A., and Chang, D. T. (2008). Foreign Body Reaction to Biomaterials. In Seminars in Immunology. Amsterdam: Elsevier, 86–100. doi: 10.1016/j.smim.2007.11.004
Ayata, C., and Lauritzen, M. J. P. R. (2015). Spreading depression, spreading depolarizations, and the cerebral vasculature. Cenk Ayata Martin Lauritzen 95, 953–993. doi: 10.1152/physrev.00027.2014
Becker, J. B., and Cha, J. H. (1989). Estrous cycle-dependent variation in amphetamine-induced behaviors and striatal dopamine release assessed with microdialysis. Behav. Brain Res. 35, 117–125. doi: 10.1016/S0166-4328(89)80112-3
Benveniste, H. (1989). Brain microdialysis. J. Neurochem. 52, 1667–1679. doi: 10.1111/j.1471-4159.1989.tb07243.x
Benveniste, H., and Diemer, N. H. (1987). Cellular reactions to implantation of a microdialysis tube in the rat hippocampus. Acta Neuropathol. 74, 234–238. doi: 10.1007/BF00688186
Benveniste, H., Drejer, J., Schousboe, A., and Diemer, N. H. (1987). Regional cerebral glucose phosphorylation and blood-flow after insertion of a microdialysis fiber through the dorsal hippocampus in therRat. J. Neurochem. 49, 729–734. doi: 10.1111/j.1471-4159.1987.tb00954.x
Benveniste, H., Hansen, A. J., and Ottosen, N. S. (1989). Determination of brain interstitial concentrations by microdialysis. J. Neurochem. 52, 1741–1750. doi: 10.1111/j.1471-4159.1989.tb07252.x
Bettinger, C. J., Ecker, M., Kozai, T. D. Y., Malliaras, G. G., Meng, E., Voit, W., et al. (2020). Recent advances in neural interfaces—Materials chemistry to clinical translation. MRS Bull. 45, 655–668. doi: 10.1557/mrs.2020.195
Booth, M. A., Gowers, S. A., Leong, C. L., Rogers, M. L., Samper, I. C., Wickham, A. P., et al. (2018). Chemical monitoring in clinical settings: recent developments toward real-time chemical monitoring of patients. Anal. Chem. 90, 2–18. doi: 10.1021/acs.analchem.7b04224
Borjigin, J., and Liu, T. (2008). Application of long-term microdialysis in circadian rhythm research. Pharmacol. Biochem. Behav. 90, 148–155. doi: 10.1016/j.pbb.2007.10.010
Borland, L. M., Shi, G., Yang, H., and Michael, A. C. (2005). Voltammetric study of extracellular dopamine near microdialysis probes acutely implanted in the striatum of the anesthetized rat. J. Neurosci. Methods 146, 149–158. doi: 10.1016/j.jneumeth.2005.02.002
Bosche, B., Dohmen, C., Graf, R., Neveling, M., Staub, F., Kracht, L., et al. (2003). Extracellular concentrations of non–transmitter amino acids in peri-infarct tissue of patients predict malignant middle cerebral artery infarction. Clin. Trial 34, 2908–2913. doi: 10.1161/01.STR.0000100158.51986.EB
Bosche, B., Graf, R., Ernestus, R. I., Dohmen, C., Reithmeier, T., Brinker, G., et al. (2010). Recurrent spreading depolarizations after subarachnoid hemorrhage decreases oxygen availability in human cerebral cortex. Ann. Neurol. 67, 607–617. doi: 10.1002/ana.21943
Bridges, A. W., and García, A. (2008). Anti-inflammatory polymeric coatings for implantable biomaterials and devices. J. Diabetes Sci. Technol. 2, 984–994. doi: 10.1177/193229680800200628
Brisch, R., Saniotis, A., Wolf, R., Bielau, H., Bernstein, H. G., Steiner, J., et al. (2014). The role of dopamine in schizophrenia from a neurobiological and evolutionary perspective: old fashioned, but still in vogue. Front. Psychiatry 5:47. doi: 10.3389/fpsyt.2014.00047
Bungay, P. M., Morrison, P. F., and Dedrick, R. L. (1990). Steady-state theory for quantitative microdialysis of solutes and water in vivo and in vitro. Life Sci. 46, 105–119. doi: 10.1016/0024-3205(90)90043-Q
Bungay, P. M., Morrison, P. F., Dedrick, R. L., Chefer, V. I., and Zapata, A. (2007). “Principles of quantitative microdialysis,” in Handbook of Microdialysis: Methods, Applications, and Perspectives, eds B. H. C. Westerink and T. Cremers (Amsterdam: Elsevier), 131–167. doi: 10.1016/S1569-7339(06)16008-7
Bungay, P. M., Newton-Vinson, P., Isele, W., Garris, P. A., and Justice, J. B. (2003). Microdialysis of dopamine interpreted with quantitative model incorporating probe implantation trauma. J. Neurochem. 86, 932–946. doi: 10.1046/j.1471-4159.2003.01904.x
Cano-Cebrian, M. J., Zornoza, T., Polache, A., and Granero, L. (2005). Quantitative in vivo microdialysis in pharmacokinetic studies: some reminders. Curr. Drug Metab. 6, 83–90. doi: 10.2174/1389200053586109
Carneheim, C., and Stahle, L. (1991). Microdialysis of lipophilic compounds: a methodological study. Pharmacol. Toxicol. 69, 378–380. doi: 10.1111/j.1600-0773.1991.tb01315.x
Castner, S. A., Xiao, L., and Becker, J. B. (1993). Sex differences in striatal dopamine: in vivo microdialysis and behavioral studies. Brain Res. 610, 127–134. doi: 10.1016/0006-8993(93)91225-H
Chao, J., Viets, Z., Donham, P., Wood, J. G., and Gonzalez, N. C. (2012). Dexamethasone blocks the systemic inflammation of alveolar hypoxia at several sites in the inflammatory cascade. Am. J. Physiol. Heart Circulat. Physiol. 303, H168–H177. doi: 10.1152/ajpheart.00106.2012
Chaurasia, C. S., Müller, M., Bashaw, E. D., Benfeldt, E., Bolinder, J., Bullock, R., et al. (2007). AAPS-FDA workshop white paper: microdialysis principles, application and regulatory perspectives. Pharm. Res. 24, 1014–1025. doi: 10.1007/s11095-006-9206-z
Chefer, V. I., Thompson, A. C., Zapata, A., and Shippenberg, T. S. (2001). Overview of Brain Microdialysis: Current Protocols in Neuroscience. Hoboken, NJ: John Wiley & Sons, Inc.
Church, W. H., Justice, J. B. Jr., and Byrd, L. D. (1987). Extracellular dopamine in rat striatum following uptake inhibition by cocaine, nomifensine and benztropine. Eur. J. Pharmacol. 139, 345–348. doi: 10.1016/0014-2999(87)90592-9
Clapp-Lilly, K. L., Roberts, R. C., Duffy, L. K., Irons, K. P., Hu, Y., and Drew, K. L. (1999). An ultrastructural analysis of tissue surrounding a microdialysis probe. J. Neurosci. Methods 90, 129–142. doi: 10.1016/S0165-0270(99)00064-3
Darvesh, A. S., Carroll, R. T., Geldenhuys, W. J., Gudelsky, G. A., Klein, J., Meshul, C. K., et al. (2011). In vivo brain microdialysis: advances in neuropsychopharmacology and drug discovery. Exp. Opin. Drug Discov. 6, 109–127. doi: 10.1517/17460441.2011.547189
Dixon, C. E., Lyeth, B. G., Povlishock, J. T., Findling, R. L., Hamm, R. J., Marmarou, A., et al. (1987). A fluid percussion model of experimental brain injury in the rat. J. Neurosurg. 67, 110–119. doi: 10.3171/jns.1987.67.1.0110
Dreier, J. P. (2011). The role of spreading depression, spreading depolarization and spreading ischemia in neurological disease. Nat. Med. 17, 439–447. doi: 10.1038/nm.2333
Dreier, J. P., Bhatia, R., Major, S., Drenckhahn, C., Lehmann, T. N., Sarrafzadeh, A., et al. (2006a). Delayed ischaemic neurological deficits after subarachnoid haemorrhage are associated with clusters of spreading depolarizations. Brain 129, 3224–3237.
Dreier, J. P., Fabricius, M., Ayata, C., Sakowitz, O. W., Shuttleworth, C. W., Dohmen, C., et al. (2016). Recording, analysis, and interpretation of spreading depolarizations in neurointensive care: review and recommendations of the COSBID research group. J. Cereb. Blood Flow Metab. 37, 1595-1625.
Dreier, J. P., Major, S., Manning, A., Woitzik, J., Drenckhahn, C., Steinbrink, J., et al. (2009). Cortical spreading ischaemia is a novel process involved in ischaemic damage in patients with aneurysmal subarachnoid haemorrhage. Brain 132, 1866–1881. doi: 10.1093/brain/awp102
Dreier, J. P., Woitzik, J., Fabricius, M., Bhatia, R., Major, S., Drenckhahn, C., et al. (2006b). Delayed ischaemic neurological deficits after subarachnoid haemorrhage are associated with clusters of spreading depolarizations. Brain 129, 3224–3237. doi: 10.1093/brain/awl297
Dykstra, K. H., Hsiao, J. K., Morrison, P. F., Bungay, P. M., Mefford, I. N., Scully, M. M., et al. (1992). Quantitative examination of tissue concentration profiles associated with microdialysis. J. Neurochem. 58, 931–940. doi: 10.1111/j.1471-4159.1992.tb09346.x
Fabricius, M., Fuhr, S., Bhatia, R., Boutelle, M., Hashemi, P., Strong, A. J., et al. (2006). Cortical spreading depression and peri-infarct depolarization in acutely injured human cerebral cortex. Brain 129, 778–790. doi: 10.1093/brain/awh716
Feuerstein, D., Manning, A., Hashemi, P., Bhatia, R., Fabricius, M., Tolias, C., et al. (2010). Dynamic metabolic response to multiple spreading depolarizations in patients with acute brain injury: an online microdialysis study. J. Cereb. Blood Flow Metab. 30, 1343–1355. doi: 10.1038/jcbfm.2010.17
Garris, P. A., and Wightman, R. M. (1995). Regional differences in dopamine release, uptake, and diffusion measured by fast-scan cyclic voltammetry. In Voltammetric methods in brain systems. New York, NY: Springer, 179–220. doi: 10.1385/0-89603-312-0:179
Gerritsen, M., Jansen, J., and Lutterman, J. (1999). Performance of subcutaneously implanted glucose sensors for continuous monitoring. Neth. J. Med 54, 167–179. doi: 10.1016/S0300-2977(99)00006-6
Ghirnikar, R. S., Lee, Y. L., and Eng, L. F. (1998). Inflammation in traumatic brain injury: role of cytokines and chemokines. Neurochem. Res. 23, 329–340. doi: 10.1023/A:1022453332560
Gifford, R., Kehoe, J. J., Barnes, S. L., Kornilayev, B. A., Alterman, M. A., and Wilson, G. S. J. B. (2006). Protein interactions with subcutaneously implanted biosensors. Biomaterials 27, 2587–2598. doi: 10.1016/j.biomaterials.2005.11.033
Globus, M. Y., Alonso, O., Dietrich, W. D., Busto, R., and Ginsberg, M. D. (1995). Glutamate release and free radical production following brain injury: effects of posttraumatic hypothermia. J. Neurochem. 65, 1704–1711. doi: 10.1046/j.1471-4159.1995.65041704.x
Gordon, S., and Taylor, P. R. (2005). Monocyte and macrophage heterogeneity. Nat. Rev. Immunol. 5:953. doi: 10.1038/nri1733
Gowers, S. A., Rogers, M. L., Booth, M. A., Leong, C. L., Samper, I. C., Phairatana, T., et al. (2019). Clinical translation of microfluidic sensor devices: focus on calibration and analytical robustness. Lab. Chip 19, 2537–2548. doi: 10.1039/C9LC00400A
Gu, H., Varner, E. L., Groskreutz, S. R., Michael, A. C., and Weber, S. G. (2015). In vivo monitoring of dopamine by microdialysis with 1 min temporal resolution using online capillary liquid chromatography with electrochemical detection. Anal. Chem. 87, 6088–6094. doi: 10.1021/acs.analchem.5b00633
Guiou, M., Sheth, S., Nemoto, M., Walker, M., Pouratian, N., Ba, A., et al. (2005). Cortical spreading depression produces long-term disruption of activity-related changes in cerebral blood volume and neurovascular coupling. J. Biomed. Opt. 10:0110044–0110047. doi: 10.1117/1.1852556
Haddad, S. H., and Arabi, Y. M. (2012). Critical care management of severe traumatic brain injury in adults. Scand. J. Trauma Resuscit. Emerg. Med. 20:12. doi: 10.1186/1757-7241-20-12
Hammarlund-Udenaes, M. (2017). Microdialysis as an important technique in systems pharmacology—a historical and methodological review. AAPS J 19, 1294–1303. doi: 10.1208/s12248-017-0108-2
Hartings, J. A., Bullock, M. R., Okonkwo, D. O., Murray, L. S., Murray, G. D., Fabricius, M., et al. (2011a). Spreading depolarisations and outcome after traumatic brain injury: a prospective observational study. Lancet Neurol. 10, 1058–1064. doi: 10.1016/S1474-4422(11)70243-5
Hartings, J. A., Strong, A. J., Fabricius, M., Manning, A., Bhatia, R., Dreier, J. P., et al. (2009). Spreading depolarizations and late secondary insults after traumatic brain injury. J. Neurotrauma 26, 1857–1866. doi: 10.1089/neu.2009.0961
Hartings, J. A., Watanabe, T., Bullock, M. R., Okonkwo, D. O., Fabricius, M., Woitzik, J., et al. (2011b). Spreading depolarizations have prolonged direct current shifts and are associated with poor outcome in brain trauma. Brain 134(Pt. 5), 1529-1540. doi: 10.1093/brain/awr048
Hashemi, P., Bhatia, R., Nakamura, H., Dreier, J. P., Graf, R., Strong, A. J., et al. (2009). Persisting depletion of brain glucose following cortical spreading depression, despite apparent hyperaemia: evidence for risk of an adverse effect of Leao’s spreading depression. J. Cereb. Blood Flow Metab. 29, 166–175. doi: 10.1038/jcbfm.2008.108
Helmy, A., Carpenter, K. L., Skepper, J. N., Kirkpatrick, P. J., Pickard, J. D., and Hutchinson, P. J. (2009). Microdialysis of cytokines: methodological considerations, scanning electron microscopy, and determination of relative recovery. J. Neurotrauma 26, 549–561. doi: 10.1089/neu.2008.0719
Hempen, C., Weiss, E., and Hess, C. F. (2002). Dexamethasone treatment in patients with brain metastases and primary brain tumors: do the benefits outweigh the side-effects? Support Care Cancer 10, 322–328. doi: 10.1007/s00520-001-0333-0
Hickey, T., Kreutzer, D., Burgess, D., and Moussy, F. (2002). In vivo evaluation of a dexamethasone/PLGA microsphere system designed to suppress the inflammatory tissue response to implantable medical devices. J. Biomed. Mater. Res. 61, 180–187. doi: 10.1002/jbm.10016
Huang, C.-M., Nakatsuji, T., Liu, Y.-T., and Shi, Y. J. R. (2008). In vivo tumor secretion probing via ultrafiltration and tissue chamber: implication for anti-cancer drugs targeting secretome. Recent Pat. Anticancer Drug Discov. 3, 48–54. doi: 10.2174/157489208783478694
Hutchinson, P. J., O’Connell, M. T., Al-Rawi, P. G., Maskell, L. B., Kett-White, R., Gupta, A. K., et al. (2000). Clinical cerebral microdialysis: a methodological study. J. Neurosurg. 93, 37–43. doi: 10.3171/jns.2000.93.1.0037
Jaquins-Gerstl, A., and Michael, A. C. (2009). Comparison of the brain penetration injury associated with microdialysis and voltammetry. J. Neurosci. Methods 183, 127–135. doi: 10.1016/j.jneumeth.2009.06.023
Jaquins-Gerstl, A., Shu, Z., Zhang, J., Liu, Y., Weber, S. G., and Michael, A. C. (2011). Effect of dexamethasone on gliosis, ischemia, and dopamine extraction during microdialysis sampling in brain tissue. Anal. Chem. 83, 7662–7667. doi: 10.1021/ac200782h
Jones, D., Parkin, M., Langemann, H., Landolt, H., and Hopwood, S. (2002). On-line monitoring in neurointensive care: enzyme-based electrochemical assay for simultaneous, continuous monitoring of glucose and lactate from critical care patients. J. Electroanal. Chem. 538, 243–252. doi: 10.1016/S0022-0728(02)00839-2
Keeler, G. D., Durdik, J. M., and Stenken, J. A. (2015a). Effects of delayed delivery of dexamethasone-21-phosphate via subcutaneous microdialysis implants on macrophage activation in rats. Acta Biomater. 23, 27–37. doi: 10.1016/j.actbio.2015.05.011
Keeler, G. D., Durdik, J. M., and Stenken, J. A. (2015b). Localized delivery of dexamethasone-21-phosphate via microdialysis implants in rat induces M (GC) macrophage polarization and alters CCL2 concentrations. Acta Biomater. 12, 11–20. doi: 10.1016/j.actbio.2014.10.022
Kennedy, R. T., Yoon, H. J., Ngernsutivorakul, T., and Lee, W. H. (2018). Microfabrication of a Microdialysis Probe With Nanoporous Membrane. U.S. Patent No WO2017100028. Washington, DC: U.S. Patent and Trademark Office.
Kim, D. H., and Martin, D. C. J. B. (2006). Sustained release of dexamethasone from hydrophilic matrices using PLGA nanoparticles for neural drug delivery. Biomaterials 27, 3031–3037. doi: 10.1016/j.biomaterials.2005.12.021
Kim, D. H., Smith, J. T., Chilkoti, A., and Reichert, W. M. J. B. (2007). The effect of covalently immobilized rhIL-1ra-ELP fusion protein on the inflammatory profile of LPS-stimulated human monocytes. Biomaterials 28, 3369–3377. doi: 10.1016/j.biomaterials.2007.04.010
Klueh, U., Kaur, M., Montrose, D. C., and Kreutzer, D. L. (2007). Inflammation and glucose sensors: use of dexamethasone to extend glucose sensor function and life span in vivo. J. Diabetes Sci. Technol. 1, 496–504. doi: 10.1177/193229680700100407
Koh, A., Lu, Y., and Schoenfisch, M. H. J. A. C. (2013). Fabrication of nitric oxide-releasing porous polyurethane membranes-coated needle-type implantable glucose biosensors. Anal. Chem. 85, 10488–10494. doi: 10.1021/ac402312b
Kozai, T. D., Jaquins-Gerstl, A. S., Vazquez, A. L., Michael, A. C., and Cui, X. T. (2015). Brain tissue responses to neural implants impact signal sensitivity and intervention strategies. ACS Chem. Neurosci. 6, 48–67. doi: 10.1021/cn500256e
Kozai, T. D. Y., Jaquins-Gerstl, A. S., Vazquez, A. L., Michael, A. C., and Cui, X. T. (2016). Dexamethasone retrodialysis attenuates microglial response to implanted probes in vivo. Biomaterials 87, 157–169. doi: 10.1016/j.biomaterials.2016.02.013
Kozai, T. D. Y., Kipke, D. R., and Subbaroyan, J. (2017). Probe Insertion Device for Implanting a Probe Into Tissue. U.S. Patent No US9814489B2. Washington, DC: U.S. Patent and Trademark Office.
Kozai, T. D. Y., Vazquez, A. L., Weaver, C. L., Kim, S.-G., and Cui, X. T. (2012). In vivo two-photon microscopy reveals immediate microglial reaction to implantation of microelectrode through extension of processes. J. Neual. Eng. 9:066001. doi: 10.1088/1741-2560/9/6/066001
Lammers, T., Sofias, A. M., van der Meel, R., Schiffelers, R., Storm, G., Tacke, F., et al. (2020). Dexamethasone nanomedicines for COVID-19. Nat. Nanotechnol. 15, 622–624. doi: 10.1038/s41565-020-0752-z
Lauritzen, M., Dreier, J. P., Fabricius, M., Hartings, J. A., Graf, R., and Strong, A. J. (2011). Clinical relevance of cortical spreading depression in neurological disorders: migraine, malignant stroke, subarachnoid and intracranial hemorrhage, and traumatic brain injury. J. Cereb. Blood Flow Metab. 31, 17–35. doi: 10.1038/jcbfm.2010.191
Lee, W. H., Slaney, T. R., Hower, R. W., and Kennedy, R. T. (2013). Microfabricated sampling probes for in vivo monitoring of neurotransmitters. Anal. Chem. 85, 3828–3831. doi: 10.1021/ac400579x
Lefkowitz, D. L., and Lefkowitz, S. S. J. I. (2001). Macrophage–neutrophil interaction: a paradigm for chronic inflammation revisited. Immunol. Cell Biol. 79, 502–506. doi: 10.1046/j.1440-1711.2001.01020.x
Li, Q., Zubieta, J. K., and Kennedy, R. T. (2009). Practical aspects of in vivo detection of neuropeptides by microdialysis coupled off-line to capillary LC with multistage MS. Anal. Chem. 81, 2242–2250. doi: 10.1021/ac802391b
Lindefors, N., Brodin, E., and Ungerstedt, U. (1989). Amphetamine facilitates the in vivo release of neurokinin A in the nucleus accumbens of the rat. Eur. J. Pharmacol. 160, 417–420. doi: 10.1016/0014-2999(89)90100-3
Liu, Y., Zhang, J., Xu, X., Zhao, M., Andrews, A. M., and Weber, S. G. (2010). Capillary ultrahigh performance liquid chromatography with elevated temperature for sub-one minute separations of basal serotonin in submicroliter brain microdialysate samples. Anal. Chem. 82, 9611–9616. doi: 10.1021/ac102200q
Lotharius, J., and Brundin, P. J. N. R. N. (2002). Pathogenesis of Parkinson’s disease: dopamine, vesicles and α-synuclein. Nat. Rev. Neurosci. 3”:932. doi: 10.1038/nrn983
Lu, Y., Peters, J. L., and Michael, A. C. (1998). Direct comparison of the response of voltammetry and microdialysis to electrically evoked release of striatal dopamine. J. Neurochem. 70, 584–593. doi: 10.1046/j.1471-4159.1998.70020584.x
Luo, X., Matranga, C., Tan, S., Alba, N., and Cui, X. T. J. B. (2011). Carbon nanotube nanoreservior for controlled release of anti-inflammatory dexamethasone. Biomaterials 32, 6316–6323. doi: 10.1016/j.biomaterials.2011.05.020
Mantovani, A., Biswas, S. K., Galdiero, M. R., Sica, A., and Locati, M. (2013). Macrophage plasticity and polarization in tissue repair and remodelling. J. Pathol. 229, 176–185. doi: 10.1002/path.4133
Martinez, F. O. (2011). Regulators of macrophage activation. Eur. J. Immunol. 41, 1531–1534. doi: 10.1002/eji.201141670
Mercanzini, A., Reddy, S. T., Velluto, D., Colin, P., Maillard, A., Bensadoun, J.-C., et al. (2010). Controlled release nanoparticle-embedded coatings reduce the tissue reaction to neuroprostheses. J. Contol. Rel. 145, 196–202. doi: 10.1016/j.jconrel.2010.04.025
Michael, A. C., and Borland, L. (2006). Electrochemical Methods for Neuroscience. Boca Raton, FL: CRC press. doi: 10.1201/9781420005868
Mitala, C. M., Wang, Y., Borland, L. M., Jung, M., Shand, S., Watkins, S., et al. (2008). Impact of microdialysis probes on vasculature and dopamine in the rat striatum: a combined fluorescence and voltammetric study. J. Neurosci. Methods 174, 177–185. doi: 10.1016/j.jneumeth.2008.06.034
Nakamura, H., Strong, A. J., Dohmen, C., Sakowitz, O. W., Vollmar, S., Sue, M., et al. (2010). Spreading depolarizations cycle around and enlarge focal ischaemic brain lesions. Brain 133, 1994–2006. doi: 10.1093/brain/awq117
Nakase, H., Okazaki, K., Tabata, Y., Ozeki, M., Watanabe, N., Ohana, M., et al. (2002). New cytokine delivery system using gelatin microspheres containing interleukin-10 for experimental inflammatory bowel disease. J. Pharmacol. Exp. Ther. 301, 59–65. doi: 10.1124/jpet.301.1.59
Nesbitt, K. M. (2015). Retrodialysis of Pharmacological Agents Mitigates Tissue Damage during Brain Microdialysis and Preserves Dopamine Activity in Surrounding Tissue. Pittsburgh, PA: University of Pittsburgh.
Nesbitt, K. M., Jaquins-Gerstl, A., Skoda, E. M., Wipf, P., and Michael, A. C. (2013). Pharmacological Mitigation of Tissue Damage during Brain Microdialysis. Anal. Chem. 85, 8173–8179. doi: 10.1021/ac401201x
Nesbitt, K. M., Varner, E. L., Jaquins-Gerstl, A., and Michael, A. C. (2015). Microdialysis in the rat striatum: effects of 24 h dexamethasone retrodialysis on evoked dopamine release and penetration injury. ACS Chem. Neurosci. 6, 163–173. doi: 10.1021/cn500257x
Ngernsutivorakul, T., Steyer, D. J., Valenta, A. C., and Kennedy, R. T. (2018). In vivo chemical monitoring at high spatiotemporal resolution using microfabricated sampling probes and droplet-based microfluidics coupled to mass spectrometry. Anal. Chem 90, 10943–10950. doi: 10.1021/acs.analchem.8b02468
Nichols, S. P., Koh, A., Brown, N. L., Rose, M. B., Sun, B., Slomberg, D. L., et al. (2012). The effect of nitric oxide surface flux on the foreign body response to subcutaneous implants. Biomaterials 33, 6305–6312. doi: 10.1016/j.biomaterials.2012.05.053
Nimmerjahn, A., Kirchhoff, F., and Helmchen, F. J. S. (2005). Resting microglial cells are highly dynamic surveillants of brain parenchyma in vivo. Science 308, 1314–1318. doi: 10.1126/science.1110647
Norton, L., Tegnell, E., Toporek, S., and Reichert, W. J. B. (2005). In vitro characterization of vascular endothelial growth factor and dexamethasone releasing hydrogels for implantable probe coatings. Biomaterials 26, 3285–3297. doi: 10.1016/j.biomaterials.2004.07.069
Osier, N. D., and Dixon, C. E. (2016). The controlled cortical impact model: applications, considerations for researchers, and future directions. Front. Neurol. 7:134. doi: 10.3389/fneur.2016.00134
Pagkalos, I., Rogers, M. L., Boutelle, M. G., and Drakakis, E. M. J. C. (2018). A high-performance application specific integrated circuit for electrical and neurochemical traumatic brain injury monitoring. Chemphyschem 19, 1215-1225. doi: 10.1002/cphc.201701119
Papadimitriou, K. I., Wang, C., Rogers, M. L., Gowers, S. A., Leong, C. L., Boutelle, M. G., et al. (2016). High-performance bioinstrumentation for real-time neuroelectrochemical traumatic brain injury monitoring. Front. Hum. Neurosci. 10:212. doi: 10.3389/fnhum.2016.00212
Parkin, M., Hopwood, S., Jones, D. A., Hashemi, P., Landolt, H., Fabricius, M., et al. (2005). Dynamic changes in brain glucose and lactate in pericontusional areas of the human cerebral cortex, monitored with rapid sampling on-line microdialysis: relationship with depolarisation-like events. J. Cereb. Blood Flow Metab. 25, 402–413. doi: 10.1038/sj.jcbfm.9600051
Parsons, L., Smith, A., and Justice, J. (1991). The in vivo microdialysis recovery of dopamine is altered independently of basal level by 6-hydroxydopamine lesions to the nucleus accumbens. J. Neurosci. Methods 40, 139–147. doi: 10.1016/0165-0270(91)90063-6
Patacsil, J. A., McAuliffe, M. S., Feyh, L. S., and Sigmon, L. L. (2016). Local anesthetic adjuvants providing the longest duration of analgesia for single-injection peripheral nerve blocks in orthopedic surgery: a literature review. Aana J. 84, 95–103.
Perry, M., Li, Q., and Kennedy, R. T. (2009). Review of recent advances in analytical techniques for the determination of neurotransmitters. Anal. Chim. Acta 653, 1–22. doi: 10.1016/j.aca.2009.08.038
Phillips, P. E., Stuber, G. D., Heien, M. L., Wightman, R. M., and Carelli, R. M. J. N. (2003). Subsecond dopamine release promotes cocaine seeking. Nature 422:614. doi: 10.1038/nature01476
Polikov, V. S., Tresco, P. A., and Reichert, W. M. (2005). Response of brain tissue to chronically implanted neural electrodes. J. Neurosci. Methods 148, 1–18. doi: 10.1016/j.jneumeth.2005.08.015
Pontieri, F. E., Tanda, G., and Di Chiara, G. (1995). Intravenous cocaine, morphine, and amphetamine preferentially increase extracellular dopamine in the “shell” as compared with the “core” of the rat nucleus accumbens. Proc. Natl. Acad. Sci. U.S.A. 92, 12304–12308. doi: 10.1073/pnas.92.26.12304
Robbins, E. M., Jaquins-Gerstl, A., Fine, D. F., Leong, C. L., Dixon, C. E., Wagner, A. K., et al. (2019). Extended (10-Day) real-time monitoring by dexamethasone-enhanced microdialysis in the injured rat cortex. ACS Chem. Neurosci. 10, 3521-3531. doi: 10.1021/acschemneuro.9b00145
Roberts, P. J., and Anderson, S. D. (1979). Stimulatory effect of L-glutamate and related amino acids on [3H]dopamine release from rat striatum: an in vitro model for glutamate actions. J. Neurochem. 32, 1539–1545. doi: 10.1111/j.1471-4159.1979.tb11096.x
Robinson, D. L., Venton, B. J., Heien, M. L., and Wightman, R. M. (2003). Detecting subsecond dopamine release with fast-scan cyclic voltammetry in vivo. Clin. Chem. 49, 1763–1773. doi: 10.1373/49.10.1763
Robinson, T. E., and Justice, J. B. (eds) (1991). Techniques in the Behavioral and Neural Sciences. Amsterdam: Elsevier.
Rogers, M. L., Feuerstein, D., Leong, C. L., Takagaki, M., Niu, X., Graf, R., et al. (2013). Continuous online microdialysis using microfluidic sensors: dynamic neurometabolic changes during spreading depolarization. ACS Chem. Neurosci. 4, 799–807. doi: 10.1021/cn400047x
Rogers, M. L., Leong, C. L., Gowers, S. A., Samper, I. C., Jewell, S. L., Khan, A., et al. (2017). Simultaneous monitoring of potassium, glucose and lactate during spreading depolarization in the injured human brain–proof of principle of a novel real-time neurochemical analysis system, continuous online microdialysis. J. Cereb. Blood Flow Metab. 37, 1883–1895. doi: 10.1177/0271678X16674486
Sanchez, J. J., Bidot, C. J., O’Phelan, K., Gajavelli, S., Yokobori, S., Olvey, S., et al. (2013). Neuromonitoring with microdialysis in severe traumatic brain injury patients. Acta Neurochir. Suppl. 118, 223–227. doi: 10.1007/978-3-7091-1434-6_42
Santiago, M., and Westerink, B. H. (1990). Characterization of the in vivo release of dopamine as recorded by different types of intracerebral microdialysis probes. Naunyn Schmiedebergs Arch. Pharmacol. 342, 407–414. doi: 10.1007/BF00169457
Schmidt, M. H., Pauels, G., Lügering, N., Lügering, A., Domschke, W., and Kucharzik, T. J. (1999). Glucocorticoids induce apoptosis in human monocytes: potential role of IL-1β. J. Immunol. 163, 3484–3490.
Schuck, V. J., Rinas, I., and Derendorf, H. (2004). In vitro microdialysis sampling of docetaxel. J. Pharm. Biomed. Anal. 36, 807–813. doi: 10.1016/j.jpba.2004.07.007
Schultz, W. (2007). Multiple dopamine functions at different time courses. Annu. Rev. Neurosci. 30, 259–288. doi: 10.1146/annurev.neuro.28.061604.135722
Seule, M., Keller, E., Unterberg, A., and Sakowitz, O. (2015). The hemodynamic response of spreading depolarization observed by near infrared spectroscopy after aneurysmal subarachnoid hemorrhage. Neurocrit. Care 23, 108–112. doi: 10.1007/s12028-015-0111-3
Shain, W., Spataro, L., Dilgen, J., Haverstick, K., Retterer, S., Isaacson, M., et al. (2003). Controlling cellular reactive responses around neural prosthetic devices using peripheral and local intervention strategies. IEEE Trans. Neural Syst. Rehabil. Eng. 11, 186–188. doi: 10.1109/TNSRE.2003.814800
Shippenberg, T. S., and Thompson, A. C. (2001). Overview of microdialysis. Curr. Protoc. Neurosci. 7:7.1. doi: 10.1002/0471142301.ns0701s00
Shou, M., Ferrario, C. R., Schultz, K. N., Robinson, T. E., and Kennedy, R. T. (2006). Monitoring dopamine in vivo by microdialysis sampling and on-line CE-laser-induced fluorescence. Anal. Chem. 78, 6717–6725. doi: 10.1021/ac0608218
Spataro, L., Dilgen, J., Retterer, S., Spence, A., Isaacson, M., Turner, J., et al. (2005). Dexamethasone treatment reduces astroglia responses to inserted neuroprosthetic devices in rat neocortex. Exp. Neurol. 194, 289–300. doi: 10.1016/j.expneurol.2004.08.037
Stauffer, W. M., Alpern, J. D., and Walker, P. F. (2020). COVID-19 and dexamethasone: a potential strategy to avoid steroid-related strongyloides hyperinfection. JAMA 324, 623–624. doi: 10.1001/jama.2020.13170
Stence, N., Waite, M., and Dailey, M. E. (2001). Dynamics of microglial activation: a confocal time-lapse analysis in hippocampal slices. Glia 33, 256–266. doi: 10.1002/1098-1136(200103)33:3<256::AID-GLIA1024>3.0.CO;2-J
Stenken, J. A. (1999). Methods and issues in microdialysis calibration. Anal. Chim. Acta 379, 337–358. doi: 10.1016/S0003-2670(98)00598-4
Stenken, J. A., Chen, R., and Yuan, X. (2001). Influence of geometry and equilibrium chemistry on relative recovery during enhanced microdialysis. Anal. Chim. Acta 436, 21–29. doi: 10.1016/S0003-2670(01)00885-6
Stenken, J. A., Church, M. K., Gill, C. A., and Clough, G. F. (2010). How minimally invasive is microdialysis sampling? A cautionary note for cytokine collection in human skin and other clinical studies. AAPS J. 12, 73–78. doi: 10.1208/s12248-009-9163-7
Stenken, J. A., Lunte, C. E., Southard, M. Z., and Stahle, L. (1997). Factors that influence microdialysis recovery. Comparison of experimental and theoretical microdialysis recoveries in rat liver. J. Pharm. Sci. 86, 958–966. doi: 10.1021/js960269+
Strong, A. J., Boutelle, M. G., Vespa, P. M., Bullock, M. R., Bhatia, R., and Hashemi, P. (2005). Treatment of critical care patients with substantial acute ischemic or traumatic brain injury. Crit. Care Med. 33, 2147–2149. doi: 10.1097/01.CCM.0000179029.95415.51
Strong, A. J., Fabricius, M., Boutelle, M. G., Hibbins, S. J., Hopwood, S. E., Jones, R., et al. (2002). Spreading and synchronous depressions of cortical activity in acutely injured human brain. Stroke 33, 2738–2743. doi: 10.1161/01.STR.0000043073.69602.09
Strong, A. J., Hartings, J. A., and Dreier, J. P. (2007). Cortical spreading depression: an adverse but treatable factor in intensive care? Curr. Opin. Crit. Care 13, 126–133. doi: 10.1097/MCC.0b013e32807faffb
Szarowski, D. H., Andersen, M. D., Retterer, S., Spence, A. J., Isaacson, M., Craighead, H. G., et al. (2003). Brain responses to mico-machined silicon devices. Brain Res. 983, 23–35. doi: 10.1016/S0006-8993(03)03023-3
Torto, N., Gorton, L., Laurell, T., and Marko-Varga, G. (1999). Technical issues of in vitro microdialysis sampling in bioprocess monitoring. Trends Anal. Chem. 18, 252–260. doi: 10.1016/S0165-9936(98)00115-0
Tremblay, M. È, Lowery, R. L., and Majewska, A. K. (2010). Microglial interactions with synapses are modulated by visual experience. PLoS Biol. 8:e1000527. doi: 10.1371/journal.pbio.1000527
Turner, J. N., Shain, W., Szarowski, D. H., Andersen, M., Martins, S., Isaacson, M., et al. (1999). Cerebral astrocyte response to micromachined silicon implants. Exp. Neurol. 156, 33–49. doi: 10.1006/exnr.1998.6983
Ungerstedt, U. (1984). “Measurement of neurotransmitter release by intracranial dialysis,” in In Measurement of Neurotransmitter Release In Vivo, ed. C. A. Marsden (New York, NY: John Wiley & Sons), 81–105.
Ungerstedt, U. (1991). Microdialysis–principles and applications for studies in animals and man. J. Intern. Med. 230, 365–373. doi: 10.1111/j.1365-2796.1991.tb00459.x
Ungerstedt, U., and Rostami, E. (2004). Microdialysis in neurointensive care. Curr. Pharm. Des. 10, 2145–2152. doi: 10.2174/1381612043384105
Van Coillie, E., Van Damme, J., and Opdenakker, G. J. C. (1999). The MCP/eotaxin subfamily of CC chemokines. Cytokine Growth Factor Rev. 10, 61–86. doi: 10.1016/S1359-6101(99)00005-2
Varner, E. L., Jaquins-Gerstl, A., and Michael, A. C. (2016). Enhanced intracranial microdialysis by reduction of traumatic penetration injury at the probe track. ACS Chem. Neurosci. 7, 728-736. doi: 10.1021/acschemneuro.5b00331
Varner, E. L., Leong, C. L., Jaquins-Gerstl, A., Nesbitt, K. M., Boutelle, M. G., and Michael, A. C. (2017). Enhancing continuous online microdialysis using dexamethasone: measurement of dynamic neurometabolic changes during spreading depolarization. ACS Chem. Neurosci. 8, 1779–1788. doi: 10.1021/acschemneuro.7b00148
Wagner, A. K., Sokoloski, J. E., Ren, D., Chen, X., Khan, A. S., Zafonte, R. D., et al. (2005). Controlled cortical impact injury affects dopaminergic transmission in the rat striatum. J. Neurochem. 95, 457–465. doi: 10.1111/j.1471-4159.2005.03382.x
Wake, H., Moorhouse, A. J., Jinno, S., Kohsaka, S., and Nabekura, J. J. (2009). Resting microglia directly monitor the functional state of synapses in vivo and determine the fate of ischemic terminals. J. Neurosci 29, 3974–3980. doi: 10.1523/JNEUROSCI.4363-08.2009
Wang, M., Slaney, T., Mabrouk, O., and Kennedy, R. T. (2010). Collection of nanoliter microdialysate fractions in plugs for off-line in vivo chemical monitoring with up to 2 s temporal resolution. J. Neurosci. Methods 190, 39–48. doi: 10.1016/j.jneumeth.2010.04.023
Wang, Y., Ma, Y. Y., Song, X. L., Cai, H. Y., Chen, J. C., Song, L. N., et al. (2012). Upregulations of glucocorticoid-induced leucine zipper by hypoxia and glucocorticoid inhibit proinflammatory cytokines under hypoxic conditions in macrophages. J. Immunol. 188:222. doi: 10.4049/jimmunol.1002958
Wang, Y., and Michael, A. C. (2012). Microdialysis probes alter presynaptic regulation of dopamine terminals in rat striatum. J Neurosci Methods 208, 34–39. doi: 10.1016/j.jneumeth.2012.04.009
Watson, C. J., Venton, B. J., and Kennedy, R. T. (2006). In vivo measurements of neurotransmitters by microdialysis sampling. Anal. Chem. 78, 1391–1399. doi: 10.1021/ac0693722
Webber, M. J., Matson, J. B., Tamboli, V. K., and Stupp, S. I. (2012). Controlled release of dexamethasone from peptide nanofiber gels to modulate inflammatory response. Biomaterials 33, 6823–6832. doi: 10.1016/j.biomaterials.2012.06.003
Westerink, B. H., and Cremers, T. I. (2007). Handbook of Microdialysis: Methods, Applications and Perspectives. Cambridge, MA: Academic Press.
Wilson, G. S., and Michael, A. C. (2017). Compendium Of In Vivo Monitoring In Real-time Molecular Neuroscience-Volume 2: Microdialysis And Sensing Of Neural Tissues. Singapore: World Scientific Publishing Company. doi: 10.1142/10462
Winslow, B. D., and Tresco, P. A. (2010). Quantitative analysis of the tissue response to chronically implanted microwire electrodes in rat cortex. Biomaterials 31, 1558–1567. doi: 10.1016/j.biomaterials.2009.11.049
Wisniewski, N., Klitzman, B., Miller, B., and Reichert, W. M. (2001). Decreased analyte transport through implanted membranes: differentiation of biofouling from tissue effects. J. Biomed. Mater. Res. 57, 513–521. doi: 10.1002/1097-4636(20011215)57:4<513::AID-JBM1197>3.0.CO;2-E
Wisniewski, N., Moussy, F., and Reichert, W. J. (2000). Characterization of implantable biosensor membrane biofouling. Fresen. J. Anal. Chem. 366, 611–621. doi: 10.1007/s002160051556
Wisniewski, N., and Reichert, M. J. C. (2000). Methods for reducing biosensor membrane biofouling. Coll. Surf B Biointerface 18, 197–219. doi: 10.1016/S0927-7765(99)00148-4
Xiong, Y., Mahmood, A., and Chopp, M. (2013). Animal models of traumatic brain injury. Nat. Rev. Neurosci. 14, 128–142. doi: 10.1038/nrn3407
Yang, H., and Michael, A. C. (2007). “In vivo fast-scan cyclic voltammetry of dopamine near microdialysis probes,” in Electrochemical Methods for Neuroscience, eds A. C. Michael and L. M. Borland (Boca Raton, FL: CRC Press). doi: 10.1201/9781420005868.ch22
Yang, H., Thompson, A. B., McIntosh, B. J., Altieri, S. C., and Andrews, A. M. (2013). Physiologically relevant changes in serotonin resolved by fast microdialysis. ACS Chem. Neurosci. 4, 790–798. doi: 10.1021/cn400072f
Zachman, A. L., Crowder, S. W., Ortiz, O., Zienkiewicz, K. J., Bronikowski, C. M., Yu, S. S., et al. (2012). Pro-angiogenic and anti-inflammatory regulation by functional peptides loaded in polymeric implants for soft tissue regeneration. Tissue Eng. Part A 19, 437–447. doi: 10.1089/ten.tea.2012.0158
Zha, Q., Wang, Y., Fan, Y., and Zhu, M. Y. (2011). Dexamethasone-induced up-regulation of the human norepinephrine transporter involves the glucocorticoid receptor and increased binding of C/EBP-β to the proximal promoter of norepinephrine transporter. J. Neurochem. 119, 654–663. doi: 10.1111/j.1471-4159.2011.07448.x
Zhang, J., Jaquins-Gerstl, A., Nesbitt, K. M., Rutan, S. C., Michael, A. C., and Weber, S. G. (2013). In vivo monitoring of serotonin in the striatum of freely moving rats with one minute temporal resolution by online microdialysis-capillary high-performance liquid chromatography at elevated temperature and pressure. Anal. Chem. 85, 9889–9897. doi: 10.1021/ac4023605
Zhong, Y., and Bellamkonda, R. V. (2005). Controlled release of anti-inflammatory agent alpha-MSH from neural implants. J. Control. Rel. 106, 309–318. doi: 10.1016/j.jconrel.2005.05.012
Zhong, Y., and Bellamkonda, R. V. (2007). Dexamethasone-coated neural probes elicit attenuated inflammatory response and neuronal loss compared to uncoated neural probes. Brain Res. 1148, 15–27. doi: 10.1016/j.brainres.2007.02.024
Keywords: microdialysis, dexamethasone, penetration injury, brain, mitigate
Citation: Jaquins-Gerstl A and Michael AC (2020) Dexamethasone-Enhanced Microdialysis and Penetration Injury. Front. Bioeng. Biotechnol. 8:602266. doi: 10.3389/fbioe.2020.602266
Received: 02 September 2020; Accepted: 11 November 2020;
Published: 08 December 2020.
Edited by:
Valentina Castagnola, Italian Institute of Technology (IIT), ItalyReviewed by:
Yawen Li, Lawrence Technological University, United StatesCarmen Alvarez-Lorenzo, University of Santiago de Compostela, Spain
Copyright © 2020 Jaquins-Gerstl and Michael. This is an open-access article distributed under the terms of the Creative Commons Attribution License (CC BY). The use, distribution or reproduction in other forums is permitted, provided the original author(s) and the copyright owner(s) are credited and that the original publication in this journal is cited, in accordance with accepted academic practice. No use, distribution or reproduction is permitted which does not comply with these terms.
*Correspondence: Andrea Jaquins-Gerstl, YXNqMTlAcGl0dC5lZHU=