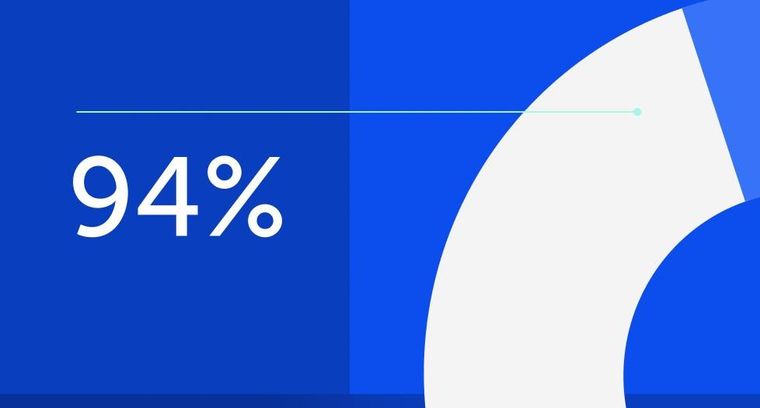
94% of researchers rate our articles as excellent or good
Learn more about the work of our research integrity team to safeguard the quality of each article we publish.
Find out more
MINI REVIEW article
Front. Bioeng. Biotechnol., 03 December 2020
Sec. Biomaterials
Volume 8 - 2020 | https://doi.org/10.3389/fbioe.2020.602009
This article is part of the Research TopicBiomaterials for Engineering Cellular Environments in Tissue EngineeringView all 15 articles
Cell-derived extracellular matrices (CD-ECMs) captured increasing attention since the first studies in the 1980s. The biological resemblance of CD-ECMs to their in vivo counterparts and natural complexity provide them with a prevailing bioactivity. CD-ECMs offer the opportunity to produce microenvironments with costumizable biological and biophysical properties in a controlled setting. As a result, CD-ECMs can improve cellular functions such as stemness or be employed as a platform to study cellular niches in health and disease. Either on their own or integrated with other materials, CD-ECMs can also be utilized as biomaterials to engineer tissues de novo or facilitate endogenous healing and regeneration. This review provides a brief overview over the methodologies used to facilitate CD-ECM deposition and manufacturing. It explores the versatile uses of CD-ECM in fundamental research and therapeutic approaches, while highlighting innovative strategies. Furthermore, current challenges are identified and it is accentuated that advancements in methodologies, as well as innovative interdisciplinary approaches are needed to take CD-ECM-based research to the next level.
The extracellular matrix (ECM) is the non-cellular component present in all connective tissues and has a composition specific for each tissue. It is comprised of a complex and highly organized three-dimensional macromolecular network of biomolecules. These include fibrous proteins (such as collagens) and glycosaminoglycan (GAG)-based components. Fibrous ECM components form the backbone of the polymer network, thereby providing shape/stability and tensile strength to tissues. They also regulate cell adhesion and support cell migration. GAG-based components fill the interstitial space, ensuring hydration and lubrication of tissues, and acting as a reservoir and modulator of cytokine signaling (Theocharis et al., 2016; Yong et al., 2020).
ECM-driven communication arises from a complex combination of biochemical, topological and biomechanical cues, facilitating a reciprocal dialogue with cells, which can respond via remodeling of the ECM. This multi-dimensional signaling enables the ECM to guide intricate cellular and tissue processes such as homeostasis, healing and regeneration (Kaukonen et al., 2017).
The ECM is a biomaterial designed by nature that underwent over 600 million years of material optimization (Ozbek et al., 2010). It serves as a blueprint for many man-made biomimetic biomaterials. Nonetheless, these materials represent oversimplified versions of the ECM that are not able to replicate its complex bioactivity (Kaukonen et al., 2017). As a result, ECM derived from decellularized tissues, remains one of the most successful biomaterials in clinics (Hussey et al., 2018).
Unfortunately, tissue-derived ECM faces various challenges to its clinical application. The limited availability of human cadaveric tissue leads to the use of animal tissue-derived ECM as an alternative source. Especially the incomplete decellularization of tissue carries the risk of disease transmission and immunological rejection. Some ECMs are plainly not available, since some specific tissues are hard to isolate (e.g., stem cell niches). Further, tissue-derived ECM is set in its composition, therefore cannot be customized in its bioactivity toward a specific application (Aamodt and Grainger, 2016).
As cell-derived ECM (CD-ECM) partially recapitulates the complex biological machinery of native tissue (Ahlfors and Billiar, 2007), it can address many of the tissue-derived ECM’s limitations. It can be derived from human cell cultures by gentle decellularization to remove immunogenic components, while preserving its bioactivity. ECM-synthesizing cells can be standardized and pre-screened (Sharma et al., 2020), minimizing the risk of disease transmission. Deriving ECM in vitro provides the opportunity to select appropriate ECM-producing cell types, further modify them (e.g., genetically) and expose them to specific stimuli, thus enabling the creation of ECM with desired properties (Maia et al., 2020). CD-ECM is therefore an incredibly versatile material to be used in physiological studies and therapeutic approaches.
Stromal cell-derived ECMs are rich in collagens (Antebi et al., 2015), while endothelial/epithelial CD-ECMs contain a laminin-rich basement membrane-like ECM (Davis and Senger, 2005). CD-ECM can be generated by culturing cells scaffold-free in 2D and 3D cultures (Serebriiskii et al., 2008; Hoshiba et al., 2016; Sharma et al., 2020). Alternatively, cells can also be seeded within hydrogels or scaffolds, forming hybrid CD-ECM-based materials (Sart et al., 2014; Suhaeri et al., 2018).
Slow ECM assembling kinetics in vitro necessitate long cell culture periods up to several weeks to harvest sufficient CD-ECM amounts for the desired application (Bourget et al., 2012). This can be improved by adjusting culture conditions (Hoshiba, 2017).
The most essential supplement for robust ECM deposition is ascorbate, a cofactor of lysyl hydroxylase and prolyl hydroxylase, essential enzymes in collagen fibrillogenesis (Pinnell, 1985). Collagen type I is the most prominent ECM component and its deposition increases the overall yield of CD-ECM and improves its mechanical properties. Nonetheless, rapid degradation of ascorbate (Grinnell et al., 1989) calls for frequent media changes, thereby discarding the not-yet deposited ECM components. A stable form of ascorbate (2-phospho-L-ascorbate) can reduce the frequency of medium replacements (Chen et al., 2011).
The yield of deposited ECM can be amplified by introducing macromolecules, which emulate the crowded conditions present in vivo. The biophysical principle of macromolecular crowding (MMC) relies on macromolecules occupying space, thereby increasing the effective concentration of other molecules and the thermodynamic activity of the system. This has profound effects on protein folding, molecular interactions and enzyme kinetics (Chen et al., 2011). In particular, under MMC more ECM can be deposited within 1 week than after several weeks under non-crowded conditions. Most commonly used “crowders” are Ficoll, carrageenan, polyvinylpyrrolidone and dextran sulfate (Lareu et al., 2007; Lu et al., 2011; Blocki et al., 2015; Gaspar et al., 2019), albeit dextran sulfate was recently found to act as a precipitating agent, independent of MMC (Assunção et al., 2020).
Culturing cells with low serum concentration (<1% v/v) was also beneficial, as serum carries exogenous matrix metalloproteases that degrade ECM and imbalance the ECM’s natural remodeling rate (Satyam et al., 2014; Kumar et al., 2015). Furthermore, hypoxia was shown to induce synthesis of ECM richer in collagenous proteins and angiogenic factors, as seen in fibroblasts (Distler et al., 2007; Kumar et al., 2018) and mesenchymal stem cells (MSCs) (Cigognini et al., 2016; Du et al., 2017).
CD-ECMs are usually generated in a small format, permitting gentle decellularization methods with focus on maintaining architecture and bioactivity. Most methods use detergents, enzymes, chelating agents, mechanical approaches and combinations thereof (Figure 1A; Woods and Gratzer, 2005; Faulk et al., 2014; Levorson et al., 2014; Gilpin and Yang, 2017). Complete decellularization is further achieved by removing genetic material with nucleases to prevent host immune reaction, as can be observed in tissue-derived ECMs (Crapo et al., 2011).
Figure 1. Methodologies to generate CD-ECM in different formats. (A) CD-ECMs are synthesized by different cell types (i.e., MSCs and fibroblasts). Culture conditions are adjusted to facilitate ECM deposition by e.g., introducing MMC or hypoxia into cell culture. The assembled ECM is then gently decellularized, while preserving the ECM’s integrity as much as possible. The resulting CD-ECM can be used in its original format or further processed. (B) The arrangement in which the ECM producing cells are cultured determines the format of the CD-ECM material. Which type of presentation is most advantageous depends on the desired application. Easiest decellularization can be achieved in 2D cultures. The resulting CD-ECM is suitable for coating of culture dishes and biomaterial surfaces or can be further processed and integrated with other biomaterials, such as hydrogels. Integration of CD-ECM with other materials provides the opportunity to combine the bioactivity of the ECM with desired geometries and mechanical properties. CD-ECM assembled in 3D recapitulates native cellular niches more closely. It can thus be utilized to engineer improved tissue models and ECM 3D scaffolds with desired geometries. Various techniques exist that enable the construction of 3D scaffolds based on CD-ECM.
Decellularized CD-ECMs can then be used in their original format, fragmented (Carvalho et al., 2019b), grinded (Wei et al., 2015) or solubilized (Decaris et al., 2012). These formats give rise to 2D ECM layers or more complex 3D structures comprising 3D scaffolds (McAllister et al., 2009), spheroids (Cheng et al., 2009), fibers (Roberts et al., 2017), and sheets (Sharma et al., 2020; Figure 1B).
Numerous applications have been explored for CD-ECMs including the improvement of cellular functions, seen in tailored cellular niches, the study of ECM in a physiological and pathophysiological context, and the application in tissue engineering and regenerative medicine (TERM) (Figure 2).
Figure 2. CD-ECM applications in fundamental research, pathophysiological studies and renegerative medicine. The ease with which CD-ECM can be modified, makes it the ideal platform to study detailed ECM mechanisms or the role of cellular niches under physiological and pathophysiological conditions. Specialized engineered cellular niches can be further utilized to improve cellular functions in vitro, such as stemness. In TERM, CD-ECMs can be created with specific mechanical and biological properties to be used on their own (i.e., as vascular grafts) or to enhance the performance of (semi-) synthetic biomaterials.
The emulation of the native cellular microenvironment in culture is a prerequisite to maintain the cells’ phenotype and function. This is especially true for sensitive cell types, such as stem cells, which are known to undergo senescence and lose their stemness ex vivo (Hoshiba et al., 2016).
Various studies demonstrated that MSC-derived ECM can recapitulate the stem cell niche sufficiently to protect reseeded MSCs from oxidative stress, promote their proliferation, and conserve their stemness (Chen et al., 2007; Lai et al., 2010; Liu et al., 2016; Xing et al., 2020). CD-ECMs were also shown to maintain the native phenotype of neural progenitor cells (Yang et al., 2017; Hoshiba et al., 2018), embryonic stem cells (ESCs) (Klimanskaya et al., 2005), periodontal ligament stem cells (Xiong et al., 2019) and hematopoietic stem cells (Prewitz et al., 2013). Furthermore, ECMs derived from younger MSCs were shown to rejuvenate in vitro-aged and chronologically-aged MSCs (Pei et al., 2011; Sun et al., 2011; Lin et al., 2012). These effects were tightly linked to the biological profile of the ECM (reviewed in Sart et al., 2020).
Similar to MSC-derived ECM supporting stemness, ECMs derived from adipogenically or osteogenically induced MSCs promoted the respective lineage commitment of reseeded MSCs via integrated structural and regulatory proteins (Ang et al., 2014; Jeon et al., 2018; Carvalho et al., 2019a). Chondrogenic differentiation was best supported by chondrogenic ECM deposited in 3D (Cheng et al., 2009; Lu et al., 2011). Synovial MSC-derived ECM also protected chondrocytes from pro-inflammatory stimuli (Yan et al., 2020).
CD-ECMs from stromal, endothelial and epithelial cells could improve the function of specialized cell types, such as podocytes (Satyam et al., 2020), chondrocytes (Wei et al., 2015; Yang et al., 2018; Zhang et al., 2019), hepatocytes (Grant et al., 2017; Guo et al., 2017), Schwann cells (Xiao et al., 2016), as well as promote natural killer cell differentiation (Lee et al., 2020).
Similarly to adult stem cells, CD-ECMs synthesized by differentiating ESCs were able to promote early differentiation of ESCs, even without external factors (Goh et al., 2013). ECM produced by an endoderm-inducing cell line and ECM from liver progenitor cells promoted differentiation of pluripotent cells into insulin-expressing pancreatic β-cells (Higuchi et al., 2010) and hepatic cells (Kanninen et al., 2016), respectively.
Hence, CD-ECMs can be utilized to tailor cell and tissue-specific niches to promote cellular functions and study cell-niche interactions in detail.
The ECM has a long-implicated role in disease development and progression, although the exact mechanisms often remain elusive. While the CD-ECM platform provides the opportunity to manipulate ECM and study it in detail, few studies utilized CD-ECM to study ECM mechanisms in disease (Raghunathan et al., 2018), most of them related to cancer.
It is currently well accepted that the tumor microenvironment plays a pivotal role in cancer cell behavior, including proliferation, invasiveness, metastasis and drug resistance (Serebriiskii et al., 2008). CD-ECMs provide the prospect to improve cancer models by recreating the cancer microenvironment using standard 2D, 3D cultures or more complex, organ-on-a-chip strategies (Gioiella et al., 2016; Kaukonen et al., 2017; Hoshiba, 2018). Indeed, culture of cancer cells on tumor CD-ECMs led to more physiologically relevant cancer cell phenotypes, as observed in various carcinoma (Serebriiskii et al., 2008; Eberle et al., 2011; Kaukonen et al., 2017), breast (Castelló-Cros et al., 2009; Hoshiba and Tanaka, 2013), and colon (Hoshiba, 2018) cancer models. Increased malignancy and drug resistance of cells was observed on invasive cancer CD-ECMs, in comparison to non-invasive cancer CD-ECMs (Hoshiba and Tanaka, 2013; Hoshiba, 2018). In contrast, upon culture on MSC-derived ECM, cancer cells proliferated less (Marinkovic et al., 2016) and showed reduced tumorgenecity upon implantation (Sun et al., 2010). Differences in cancer cell behavior were attributed not only to the biochemical composition of the tumor-associated ECM, but also to changes in stiffness (Kaukonen et al., 2016; Hoshiba, 2018) and a decreased cell adhesion (Hoshiba and Tanaka, 2013).
The ease of manipulating CD-ECM in vitro provides the opportunity to examine the reciprocal relationship between cells and their ECM.
Biochemical ECM re-engineering could be achieved through direct addition of functional groups (Xing et al., 2015) or exogenous factors (Sart et al., 2020), genetic modification (Higuchi et al., 2010) or growth factor stimulation of ECM-synthesizing cells (Wolchok and Tresco, 2012). Other changes in culture conditions, such as hypoxic cultures, were also shown to affect ECM properties and bioactivity (Hielscher, 2013).
Mechano-physical re-engineering could be achieved by culturing ECM-secreting cells in 3D sacrificial hydrogels (Yuan et al., 2018), on micro-molds (Schell et al., 2016), and micro- and nano-grooves (Ozguldez et al., 2018; Almici et al., 2020; Yang et al., 2020), forcing cell reorganization and leading to ECM assemblies with unique architectures (i.e., parallel fiber alignment). ECM postprocessing, such as cross-linking, could further alter ECM stiffness (Subbiah et al., 2016) or the overall presentation of CD-ECM. In particular, cross-linking of pepsin-solubilized CD-ECM with genipin resulted in the formation of hydrogels (Nyambat et al., 2020), Changes in biochemical and mechano-physical properties of the ECM let to changes in gene expression and behavior of reseeded cells (Kim et al., 2015; Ozguldez et al., 2018; Sart et al., 2020).
CD-ECM characterization and correlation with specific bioactivities can contribute to the mechanistic understanding of the ECM. ECM ultrastructure can be generally studied by scanning electron microscopy or atomic force microscopy (Kaukonen et al., 2016; Raghunathan et al., 2018). The latter method can also be used for biomechanical characterization (Prewitz et al., 2013; Assunção et al., 2020). Identification of proteins of interest is best performed by antibody-based assays such as immunocytochemistry or western blotting (Sart et al., 2020). Proteomic analysis based on mass spectroscopy enables the simultaneous identification of many components, however also faces challenges based on the insolubility and high complexity of the ECM (Ragelle et al., 2017; Senthebane et al., 2018; Silva et al., 2019). Furthermore, additional methods, such as Raman microscopy, can be used for biochemical characterization (Brauchle and Schenke-Layland, 2013).
CD-ECM uses for TERM have been increasingly explored, either with CD-ECM alone or integrated in biomaterials. 3D scaffolds purely composed of CD-ECM were produced by decellularizing stacked cell sheets (McAllister et al., 2009) and pellets (Zwolinski et al., 2011), or depositing ECM in sacrificial materials, such as hollow tubes (ECM fibers) (Roberts et al., 2017) and foams (ECM porous scaffolds) (Wolchok and Tresco, 2010; Figure 1B).
For applications that require specific mechanical properties of the biomaterials, CD-ECM was integrated with synthetic materials, forming hybrid scaffolds (Schenke-Layland et al., 2009; Carvalho et al., 2019b; Sart et al., 2020). Hybrid materials met mechanical requirements, while providing adequate biochemical stimuli, thus facilitating implant integration and functionality (Silva et al., 2020). Commonly, CD-ECM was utilized as a coating by simply decellularizing cells on the biomaterial surface (Kumar et al., 2016; Junka et al., 2020), although solubilized CD-ECM was also used as a coating (Decaris et al., 2012). A more sophisticated approach introduced azide-modified monosaccharides into culture media, which subsequently were incorporated into the ECM. The CD-ECM could then be covalently “clicked” to material surfaces (Ruff et al., 2017). Alternative approaches directly incorporated CD-ECM into the biomaterial during synthesis (e.g., electro-spinning) (Schenke-Layland et al., 2009; Carvalho et al., 2019b).
CD-ECMs based biomaterials were mainly investigated for skeletal and cardiovascular repair, although other applications such as in skin (Suhaeri et al., 2018) and peripheral nerve repair (Gu et al., 2017) were also explored.
Most approaches to engineer CD-ECM-carrying bone implants utilized inorganic materials (reviewed in Zhang et al., 2016), such as meshes and scaffolds (Kang et al., 2012; Antebi et al., 2015; Kim et al., 2015; Jeon et al., 2016; Kumar et al., 2016; Noh et al., 2016; Junka et al., 2020; Silva et al., 2020). These were coated with ECM assembled by collagen I-overexpressing epithelial cells (Noh et al., 2016), fibroblasts (Kim et al., 2015), MSCs (Kang et al., 2011; Silva et al., 2020), endothelial cells (Kang et al., 2012), osteoblasts (Jeon et al., 2016; Kumar et al., 2016) and combinations thereof (Junka et al., 2020). CD-ECM coated scaffolds promoted attachment, proliferation, and bone-like tissue formation in vitro. In a more advanced approach, Kim et al. (2015) enhanced an PLGA/PLA-based mesh scaffold coated with CD-ECM, by covalently conjugating heparin to the ECM. The heparin then acted as a growth factor reservoir for bone morphogenic protein-2 (BMP2), thereby promoting bone healing in vivo (Kim et al., 2015). CD-ECM was also used to increase retention of osteogenically precommitted MSCs on biomaterial surfaces after implantation. This revitalized ECM successfully repaired mouse calvaria defects (Zeitouni et al., 2012).
Therapeutic approaches targeting cartilage repair mainly utilized 3D scaffolds purely composed of CD-ECM (Jin et al., 2007; Tang et al., 2013, 2014) or CD-ECM-loaded hydrogels (Yuan et al., 2013). Indeed, 3D scaffolds of chondrocyte- and MSC-derived ECM reseeded with chondrocytes induced ectopic hyaline-like cartilage formation in vivo (Jin et al., 2007; Tang et al., 2013). When applied to an osteochondral defect together with bone marrow stimulation, autologous MSC-derived ECM could enhance cartilage repair (Tang et al., 2014). In another study, a protective effect on the degenerating cartilage could be demonstrated, when collagen I microspheres containing nucleus pulposus CD-ECM and MSCs were injected into a rabbit degenerative disc model (Yuan et al., 2013).
CD-ECMs have been explored as cardiac patches for cell-delivery (Schmuck et al., 2014; Kim et al., 2019), as well as to engineer heart valves replacements (Weber et al., 2013) and blood vessel grafts (McAllister et al., 2009; Syedain et al., 2014; Xing et al., 2017).
Cardiac patches were composed of fibroblast ECM alone (Schmuck et al., 2014) or combined with a polyvinyl alcohol sheet, resulting in a stretchable scaffold for cell delivery. Application of the latter in a rat myocardial infarct model resulted in improved cardiac remodeling (Kim et al., 2019).
A cardiac valve prototype containing vein-derived fibroblast ECM was implanted in a non-human primate. Albeit valve functionality was reduced, there was a significant improvement in repopulation by host cells, when compared to decellularized human heart valves (Weber et al., 2013).
McAllister et al. (2009) utilized partially devitalized autologous fibroblast/endothelial CD-ECM sheets to form vascular access grafts for dialysis patients. Complete remodeling and repopulation of CD-ECM occurred, although diffuse dilation of the graft was observed (McAllister et al., 2009).
In order to improve this low graft resistance, Syedain et al. (2014) stimulated tubular fibroblast cultures in a pulsed-flow-stretch bioreactor. Upon implantation of the decellularized graft into the femoral artery of sheep, no dilation was observed. Once completely recellularized, the grafts resembled native vessels in terms of cellular composition, ECM architecture and mechanical properties (Syedain et al., 2014).
Although CD-ECM was continuously explored for over three decades and many safety concerns associated with tissue-derived products can be addressed, relatively slow advancements were made over the years. This can be partially attributed to the low amounts of CD-ECM that can be harvested in vitro, indicating that strategies for upscaling processes as well as manufacturing of larger 3D constructs need to be developed.
In addition, most TERM approaches used unmodified ECM from MSCs or tissue-specific cell types to induce cellular responses in vitro and in vivo. And although various approaches on how to re-engineer the CD-ECM are proposed, relatively few are applied to address scientific questions or to manufacture biomaterials with enhanced desired bioactivities. The reason for the limited progress can be partially attributed to our restricted fundamental understanding of the ECM. Hence, functional studies in combination with CD-ECM characterization will have to be adopted. Another reason is that re-engineering approaches are mainly focused on biological manipulation. Research at the interface to other disciplines such as materials science is indeed required to enable further evolvement of the CD-ECM research field. Future applications could focus on bio-inks with tailor-made bioactivities for 3D bioprinting or improved biomimetic cell niches in organ-on-a-chip approaches.
In conclusion, CD-ECM based research is far from its full potential. Advancements in methodologies as well as innovative interdisciplinary approaches are needed to pave the way for an exciting next generation of CD-ECMs for basic research and therapeutic approaches.
All authors contributed to the elaboration of this review.
This study was financially supported by a laboratory start-up grant (8508266) from the Chinese University of Hong Kong (CUHK) and by the Innovation and Technology Commission—Hong Kong (Innovation and Technology Commission of Hong Kong Special Administrative Government) (ITS/116/19) and the Postdoctoral Hub for ITF projects (PiH/007/20).
The authors declare that the research was conducted in the absence of any commercial or financial relationships that could be construed as a potential conflict of interest.
MA would like to acknowledge School of Biomedical Sciences (The Chinese University of Hong Kong) for her post-graduate scholarship.
Aamodt, J. M., and Grainger, D. W. (2016). Extracellular matrix-based biomaterial scaffolds and the host response. Biomaterials 86, 68–82. doi: 10.1016/j.biomaterials.2016.02.003
Ahlfors, J.-E. W., and Billiar, K. L. (2007). Biomechanical and biochemical characteristics of a human fibroblast-produced and remodeled matrix. Biomaterials 28, 2183–2191. doi: 10.1016/j.biomaterials.2006.12.030
Almici, E., Caballero, D., Montero Boronat, J., and Samitier Martí, J. (2020). Engineering cell-derived matrices with controlled 3D architectures for pathophysiological studies. Methods Cell Biol. 156, 161–183. doi: 10.1016/bs.mcb.2019.11.022
Ang, X. M., Lee, M. H. C., Blocki, A., Chen, C., Ong, L. L. S., Asada, H. H., et al. (2014). Macromolecular crowding amplifies adipogenesis of human bone marrow-derived mesenchymal stem cells by enhancing the pro-adipogenic microenvironment. Tissue Eng. Part A 20, 966–981. doi: 10.1089/ten.tea.2013.0337
Antebi, B., Zhang, Z., Wang, Y., Lu, Z., Chen, X.-D., and Ling, J. (2015). Stromal-cell-derived extracellular matrix promotes the proliferation and retains the osteogenic differentiation capacity of mesenchymal stem cells on three-dimensional scaffolds. Tissue Eng. Part C. Methods 21, 171–181. doi: 10.1089/ten.TEC.2014.0092
Assunção, M., Wong, C. W., Richardson, J. J., Tsang, R., Beyer, S., Raghunath, M., et al. (2020). Macromolecular dextran sulfate facilitates extracellular matrix deposition by electrostatic interaction independent from a macromolecular crowding effect. Mater. Sci. Eng. C 106:110280. doi: 10.1016/j.msec.2019.110280
Blocki, A., Wang, Y., Koch, M., Goralczyk, A., Beyer, S., Agarwal, N., et al. (2015). Sourcing of an alternative pericyte-like cell type from peripheral blood in clinically relevant numbers for therapeutic angiogenic applications. Mol. Ther. 23, 510–522. doi: 10.1038/mt.2014.232
Bourget, J.-M., Gauvin, R., Larouche, D., Lavoie, A., Labbé, R., Auger, F. A., et al. (2012). Human fibroblast-derived ECM as a scaffold for vascular tissue engineering. Biomaterials 33, 9205–9213. doi: 10.1016/j.biomaterials.2012.09.015
Brauchle, E., and Schenke-Layland, K. (2013). Raman spectroscopy in biomedicine - non-invasive in vitro analysis of cells and extracellular matrix components in tissues. Biotechnol. J. 8, 288–297. doi: 10.1002/biot.201200163
Carvalho, M. S., Silva, J. C., Cabral, J. M. S. S., da Silva, C. L., Vashishth, D., Silva, C. L., et al. (2019a). Cultured cell-derived extracellular matrices to enhance the osteogenic differentiation and angiogenic properties of human mesenchymal stem/stromal cells. J. Tissue Eng. Regen. Med. 13, 1544–1558. doi: 10.1002/term.2907
Carvalho, M. S., Silva, J. C., Udangawa, R. N., Cabral, J. M. S., Ferreira, F. C., da Silva, C. L., et al. (2019b). Co-culture cell-derived extracellular matrix loaded electrospun microfibrous scaffolds for bone tissue engineering. Mater. Sci. Eng. C 99, 479–490. doi: 10.1016/j.msec.2019.01.127
Castelló-Cros, R., Khan, D. R., Simons, J., Valianou, M., and Cukierman, E. (2009). Staged stromal extracellular 3D matrices differentially regulate breast cancer cell responses through PI3K and beta1-integrins. BMC Cancer 9:94. doi: 10.1186/1471-2407-9-94
Chen, C., Loe, F., Blocki, A., Peng, Y., and Raghunath, M. (2011). Applying macromolecular crowding to enhance extracellular matrix deposition and its remodeling in vitro for tissue engineering and cell-based therapies. Adv. Drug Deliv. Rev. 63, 277–290. doi: 10.1016/j.addr.2011.03.003
Chen, X.-D., Dusevich, V., Feng, J. Q., Manolagas, S. C., and Jilka, R. L. (2007). Extracellular matrix made by bone marrow cells facilitates expansion of marrow-derived mesenchymal progenitor cells and prevents their differentiation into osteoblasts. J. Bone Miner. Res. 22, 1943–1956. doi: 10.1359/jbmr.070725
Cheng, H.-W., Tsui, Y.-K., Cheung, K. M. C., Chan, D., and Chan, B. P. (2009). Decellularization of chondrocyte-encapsulated collagen microspheres: a three-dimensional model to study the effects of acellular matrix on stem cell fate. Tissue Eng. Part C Methods 15, 697–706. doi: 10.1089/ten.tec.2008.0635
Cigognini, D., Gaspar, D., Kumar, P., Satyam, A., Alagesan, S., Sanz-Nogués, C., et al. (2016). Macromolecular crowding meets oxygen tension in human mesenchymal stem cell culture - A step closer to physiologically relevant in vitro organogenesis. Sci. Rep. 6:30746. doi: 10.1038/srep30746
Crapo, P. M., Gilbert, T. W., and Badylak, S. F. (2011). An overview of tissue and whole organ decellularization processes. Biomaterials 32, 3233–3243. doi: 10.1016/j.biomaterials.2011.01.057
Davis, G. E., and Senger, D. R. (2005). Endothelial extracellular matrix: biosynthesis, remodeling, and functions during vascular morphogenesis and neovessel stabilization. Circ. Res. 97, 1093-1107. doi: 10.1161/01.RES.0000191547.64391.e3
Decaris, M. L., Binder, B. Y., Soicher, M. A., Bhat, A., and Leach, J. K. (2012). Cell-derived matrix coatings for polymeric scaffolds. Tissue Eng. Part A 18, 2148–2157. doi: 10.1089/ten.tea.2011.0677
Distler, J. H. W., Jüngel, A., Pileckyte, M., Zwerina, J., Michel, B. A., Gay, R. E., et al. (2007). Hypoxia-induced increase in the production of extracellular matrix proteins in systemic sclerosis. Arthritis Rheum. 56, 4203–4215. doi: 10.1002/art.23074
Du, H.-C., Jiang, L., Geng, W.-X., Li, J., Zhang, R., Dang, J.-G., et al. (2017). Growth factor-reinforced ecm fabricated from chemically hypoxic msc sheet with improved in vivo wound repair activity. Biomed. Res. Int. 2017, 1–11. doi: 10.1155/2017/2578017
Eberle, K. E., Sansing, H. A., Szaniszlo, P., Resto, V. A., and Berrier, A. L. (2011). Carcinoma matrix controls resistance to cisplatin through talin regulation of NF-kB. PLoS One 6:e21496. doi: 10.1371/journal.pone.0021496
Faulk, D. M. M., Carruthers, C. A. A., Warner, H. J. J., Kramer, C. R. R., Reing, J. E. E., Zhang, L., et al. (2014). The effect of detergents on the basement membrane complex of a biologic scaffold material. Acta Biomater. 10, 183–193. doi: 10.1016/j.actbio.2013.09.006
Gaspar, D., Fuller, K. P., and Zeugolis, D. I. (2019). Polydispersity and negative charge are key modulators of extracellular matrix deposition under macromolecular crowding conditions. Acta Biomater. 88, 197–210. doi: 10.1016/j.actbio.2019.02.050
Gilpin, A., and Yang, Y. (2017). Decellularization strategies for regenerative medicine: from processing techniques to applications. Biomed. Res. Int. 2017, 1–13. doi: 10.1155/2017/9831534
Gioiella, F., Urciuolo, F., Imparato, G., Brancato, V., and Netti, P. A. (2016). An engineered breast cancer model on a chip to replicate ECM-activation in vitro during tumor progression. Adv. Healthc. Mater. 5, 3074–3084. doi: 10.1002/adhm.201600772
Goh, S.-K., Olsen, P., and Banerjee, I. (2013). Extracellular matrix aggregates from differentiating embryoid bodies as a scaffold to support ESC proliferation and differentiation. PLoS One 8:e61856. doi: 10.1371/journal.pone.0061856
Grant, R., Hay, D. C., and Callanan, A. (2017). A drug-induced hybrid electrospun poly-capro-lactone: cell-derived extracellular matrix scaffold for liver tissue engineering. Tissue Eng. Part A 23, 650–662. doi: 10.1089/ten.tea.2016.0419
Grinnell, F., Fukamizu, H., Pawelek, P., and Nakagawa, S. (1989). Collagen processing, crosslinking, and fibril bundle assembly in matrix produced by fibroblasts in long-term cultures supplemented with ascorbic acid. Exp. Cell Res. 181, 483–491. doi: 10.1016/0014-4827(89)90105-5
Gu, Y., Li, Z., Huang, J., Wang, H., Gu, X., and Gu, J. (2017). Application of marrow mesenchymal stem cell- derived extracellular matrix in peripheral nerve tissue engineering. J. Tissue Eng. Regen. Med. 11, 2250–2260. doi: 10.1002/term
Guo, X., Li, W., Ma, M., Lu, X., and Zhang, H. (2017). Endothelial cell-derived matrix promotes the metabolic functional maturation of hepatocyte via integrin-Src signalling. J. Cell. Mol. Med. 21, 2809–2822. doi: 10.1111/jcmm.13195
Hielscher, A. (2013). Hypoxia affects the structure of breast cancer cell-derived matrix to support angiogenic responses of endothelial cells. J. Carcinog. Mutagen Suppl. 13:005. doi: 10.4172/2157-2518.s13-005
Higuchi, Y., Shiraki, N., Yamane, K., Qin, Z., Mochitate, K., Araki, K., et al. (2010). Synthesized basement membranes direct the differentiation of mouse embryonic stem cells into pancreatic lineages. J. Cell Sci. 123, 2733–2742. doi: 10.1242/jcs.066886
Hoshiba, T. (2017). Cultured cell-derived decellularized matrices: a review towards the next decade. Royal Soc. Chem. 5, 4322-4331. doi: 10.1039/c7tb00074j
Hoshiba, T. (2018). An extracellular matrix (ECM) model at high malignant colorectal tumor increases chondroitin sulfate chains to promote epithelial-mesenchymal transition and chemoresistance acquisition. Exp. Cell Res. 370, 571–578. doi: 10.1016/j.yexcr.2018.07.022
Hoshiba, T., Chen, G., Endo, C., Maruyama, H., Wakui, M., Nemoto, E., et al. (2016). Decellularized Extracellular Matrix As an in Vitro Model to Study the Comprehensive Roles of the ECM in Stem Cell Differentiation. New York, NY: Hindawi Publishing Corporation, doi: 10.1155/2016/6397820
Hoshiba, T., Sugano, Y., and Yokoyama, N. (2018). Murine neural stem cell (NSC) line, MEB5-derived decellularized matrix as an in vitro extracellular matrix model in NSC Niche. Chem. Lett. 47, 1498–1501. doi: 10.1246/cl.180788
Hoshiba, T., and Tanaka, M. (2013). Breast cancer cell behaviors on staged tumorigenesis-mimicking matrices derived from tumor cells at various malignant stages. Biochem. Biophys. Res. Commun. 439, 291–296. doi: 10.1016/j.bbrc.2013.08.038
Hussey, G. S., Dziki, J. L., and Badylak, S. F. (2018). Extracellular matrix-based materials for regenerative medicine. Nat. Rev. Mater. 3, 159–173. doi: 10.1038/s41578-018-0023-x
Jeon, H., Lee, J., Lee, H., and Kim, G. H. (2016). Nanostructured surface of electrospun PCL/dECM fibres treated with oxygen plasma for tissue engineering. RSC Adv. 6, 32887–32896. doi: 10.1039/C6RA03840A
Jeon, J., Lee, M. S., and Yang, H. S. (2018). Differentiated osteoblasts derived decellularized extracellular matrix to promote osteogenic differentiation. Biomater. Res. 22:4. doi: 10.1186/s40824-018-0115-0
Jin, C. Z., Park, S. R., Choi, B. H., Park, K., and Min, B.-H. (2007). In vivo cartilage tissue engineering using a cell-derived extracellular matrix scaffold. Artif. Organs 31, 183–192. doi: 10.1111/j.1525-1594.2007.00363.x
Junka, R., Quevada, K., and Yu, X. (2020). Acellular polycaprolactone scaffolds laden with fibroblast/endothelial cell-derived extracellular matrix for bone regeneration. J. Biomed. Mater. Res. Part A 108, 351–364. doi: 10.1002/jbm.a.36821
Kang, Y., Kim, S., Bishop, J., Khademhosseini, A., and Yang, Y. (2012). The osteogenic differentiation of human bone marrow MSCs on HUVEC-derived ECM and β-TCP scaffold. Biomaterials 33, 6998–7007. doi: 10.1016/j.biomaterials.2012.06.061
Kang, Y., Kim, S., Khademhosseini, A., and Yang, Y. (2011). Creation of bony microenvironment with CaP and cell-derived ECM to enhance human bone-marrow MSC behavior and delivery of BMP-2. Biomaterials 32, 6119–6130. doi: 10.1016/j.biomaterials.2011.05.015
Kanninen, L. K., Porola, P., Niklander, J., Malinen, M. M., Corlu, A., Guguen-Guillouzo, C., et al. (2016). Hepatic differentiation of human pluripotent stem cells on human liver progenitor HepaRG-derived acellular matrix. Exp. Cell Res. 341, 207–217. doi: 10.1016/J.YEXCR.2016.02.006
Kaukonen, R., Jacquemet, G., Hamidi, H., and Ivaska, J. (2017). Cell-derived matrices for studying cell proliferation and directional migration in a complex 3D microenvironment. Nat. Protoc. 12, 2376–2390. doi: 10.1038/nprot.2017.107
Kaukonen, R., Mai, A., Georgiadou, M., Saari, M., De Franceschi, N., Betz, T., et al. (2016). Normal stroma suppresses cancer cell proliferation via mechanosensitive regulation of JMJD1a-mediated transcription. Nat. Commun. 7:12237. doi: 10.1038/ncomms12237
Kim, I. G., Hwang, M. P., Du, P., Ko, J., Ha, C., Do, S. H., et al. (2015). Bioactive cell-derived matrices combined with polymer mesh scaffold for osteogenesis and bone healing. Biomaterials 50, 75–86. doi: 10.1016/j.biomaterials.2015.01.054
Kim, I. G., Hwang, M. P., Park, J. S., Kim, S. S.-H., Kim, J.-H. J., Kang, H. J., et al. (2019). Stretchable ECM patch enhances stem cell delivery for Post-MI cardiovascular repair. Adv. Healthc. Mater. 8:e1900593. doi: 10.1002/adhm.201900593
Klimanskaya, I., Chung, Y., Meisner, L., Johnson, J., West, M. D., and Lanza, R. (2005). Human embryonic stem cells derived without feeder cells. Lancet 365, 1636–1641. doi: 10.1016/S0140-6736(05)66473-2
Kumar, A., Nune, K. C., and Misra, R. D. K. (2016). Biological functionality and mechanistic contribution of extracellular matrix-ornamented three dimensional Ti-6Al-4V mesh scaffolds. J. Biomed. Mater. Res. Part A 104, 2751–2763. doi: 10.1002/jbm.a.35809
Kumar, P., Satyam, A., Cigognini, D., Pandit, A., and Zeugolis, D. I. (2018). Low oxygen tension and macromolecular crowding accelerate extracellular matrix deposition in human corneal fibroblast culture. J. Tissue Eng. Regen. Med. 12, 6–18. doi: 10.1002/term.2283
Kumar, P., Satyam, A., Fan, X., Collin, E., Rochev, Y., Rodriguez, B. J., et al. (2015). Macromolecularly crowded in vitro microenvironments accelerate the production of extracellular matrix-rich supramolecular assemblies. Sci. Rep. 5:8729. doi: 10.1038/srep08729
Lai, Y., Sun, Y., Skinner, C. M., Son, E. L., Lu, Z., Tuan, R. S., et al. (2010). Reconstitution of marrow-derived extracellular matrix ex vivo: a robust culture system for expanding large-scale highly functional human mesenchymal stem cells. Stem Cells Dev. 19, 1095–1107. doi: 10.1089/scd.2009.0217
Lareu, R. R., Harve, K. S., and Raghunath, M. (2007). Emulating a crowded intracellular environment in vitro dramatically improves RT-PCR performance. Biochem. Biophys. Res. Commun. 363, 171–177. doi: 10.1016/j.bbrc.2007.08.156
Lee, B. J., Hegewisch Solloa, E., Shannon, M. J., and Mace, E. M. (2020). Generation of cell-derived matrices that support human NK cell migration and differentiation. J. Leukoc. Biol 108, MA0420–MA635. doi: 10.1002/JLB.1MA0420-635R
Levorson, E. J., Hu, O., Mountziaris, P. M., Kasper, F. K., and Mikos, A. G. (2014). Cell-derived polymer/extracellular matrix composite scaffolds for cartilage regeneration, part 2: construct devitalization and determination of chondroinductive capacity. Tissue Eng. Part C Methods 20, 358–372. doi: 10.1089/ten.tec.2013.0288
Lin, H., Yang, G., Tan, J., and Tuan, R. S. (2012). Influence of decellularized matrix derived from human mesenchymal stem cells on their proliferation, migration and multi-lineage differentiation potential. Biomaterials 33, 4480–4489. doi: 10.1016/J.BIOMATERIALS.2012.03.012
Liu, X., Zhou, L., Chen, X., Liu, T., Pan, G., Cui, W., et al. (2016). Culturing on decellularized extracellular matrix enhances antioxidant properties of human umbilical cord-derived mesenchymal stem cells. Mater. Sci. Eng. C 61, 437–448. doi: 10.1016/j.msec.2015.12.090
Lu, H., Hoshiba, T., Kawazoe, N., Koda, I., Song, M., and Chen, G. (2011). Cultured cell-derived extracellular matrix scaffolds for tissue engineering. Biomaterials 32, 9658–9666. doi: 10.1016/j.biomaterials.2011.08.091
Maia, F. R., Reis, R. L., and Oliveira, J. M. (2020). Decellularized hASCs-derived matrices as biomaterials for 3D in vitro approaches. Methods Cell Biol. 156, 45–58. doi: 10.1016/bs.mcb.2019.11.019
Marinkovic, M., Block, T. J., Rakian, R., Li, Q., Wang, E., Reilly, M. A., et al. (2016). One size does not fit all: developing a cell-specific niche for in vitro study of cell behavior. Matrix Biol. 5:426. doi: 10.1016/J.MATBIO.2016.01.004
McAllister, T. N., Maruszewski, M., Garrido, S. A., Wystrychowski, W., Dusserre, N., Marini, A., et al. (2009). Effectiveness of haemodialysis access with an autologous tissue-engineered vascular graft: a multicentre cohort study. Lancet 373, 1440–1446. doi: 10.1016/S0140-6736(09)60248-8
Noh, Y. K., Du, P., Kim, I. G., Ko, J., Kim, S. W., and Park, K. (2016). Polymer mesh scaffold combined with cell-derived ECM for osteogenesis of human mesenchymal stem cells. Biomater. Res. 20:6. doi: 10.1186/s40824-016-0055-5
Nyambat, B., Manga, Y. B., Chen, C.-H., Gankhuyag, U., Pratomo, W. P. A., Kumar Satapathy, M., et al. (2020). New insight into natural extracellular matrix: genipin cross-linked adipose-derived stem cell extracellular matrix gel for tissue engineering. Int. J. Mol. Sci. 21:4864. doi: 10.3390/ijms21144864
Ozbek, S., Balasubramanian, P. G., Chiquet-Ehrismann, R., Tucker, R. P., and Adams, J. C. (2010). The evolution of extracellular matrix. Mol. Biol. Cell 21, 4300–4305. doi: 10.1091/mbc.E10-03-0251
Ozguldez, H. O., Cha, J., Hong, Y., Koh, I., and Kim, P. (2018). Nanoengineered, cell-derived extracellular matrix influences ECM-related gene expression of mesenchymal stem cells. Biomater. Res. 22:32. doi: 10.1186/s40824-018-0141-y
Pei, M., He, F., and Kish, V. L. (2011). Expansion on extracellular matrix deposited by human bone marrow stromal cells facilitates stem cell proliferation and tissue-specific lineage potential. Tissue Eng. Part A 17, 3067–3076. doi: 10.1089/ten.TEA.2011.0158
Pinnell, S. R. (1985). Regulation of collagen biosynthesis by ascorbic acid: a review. Yale J. Biol. Med. 58, 553–559.
Prewitz, M. C., Seib, F. P., von Bonin, M., Friedrichs, J., Stißel, A., Niehage, C., et al. (2013). Tightly anchored tissue-mimetic matrices as instructive stem cell microenvironments. Nat. Methods 10, 788–794. doi: 10.1038/nmeth.2523
Ragelle, H., Naba, A., Larson, B. L., Zhou, F., Prijiæ, M., Whittaker, C. A., et al. (2017). Comprehensive proteomic characterization of stem cell-derived extracellular matrices. Biomaterials 128, 147–159. doi: 10.1016/j.biomaterials.2017.03.008
Raghunathan, V. K., Benoit, J., Kasetti, R., Zode, G., Salemi, M., Phinney, B. S., et al. (2018). Glaucomatous cell derived matrices differentially modulate non-glaucomatous trabecular meshwork cellular behavior. Acta Biomater. 71, 444–459. doi: 10.1016/j.actbio.2018.02.037
Roberts, K., Schluns, J., Walker, A., Jones, J. D., Quinn, K. P., Hestekin, J., et al. (2017). Cell derived extracellular matrix fibers synthesized using sacrificial hollow fiber membranes. Biomed. Mater. 13:015023. doi: 10.1088/1748-605X/aa895c
Ruff, S. M. M., Keller, S., Wieland, D. E. E., Wittmann, V., Tovar, G. E. M. E. M., Bach, M., et al. (2017). clickECM: development of a cell-derived extracellular matrix with azide functionalities. Acta Biomater. 52, 159–170. doi: 10.1016/j.actbio.2016.12.022
Sart, S., Jeske, R., Chen, X., Ma, T., and Li, Y. (2020). Engineering stem cell-derived extracellular matrices: decellularization, characterization, and biological function. Tissue Eng. Part B Rev. 26:ten.teb.2019.0349. doi: 10.1089/ten.teb.2019.0349
Sart, S., Ma, T., and Li, Y. (2014). Extracellular matrices decellularized from embryonic stem cells maintained their structure and signaling specificity. Tissue Eng. Part A 20, 54–66. doi: 10.1089/ten.tea.2012.0690
Satyam, A., Kumar, P., Fan, X., Gorelov, A., Rochev, Y., Joshi, L., et al. (2014). Macromolecular crowding meets tissue engineering by self-assembly: a paradigm shift in regenerative medicine. Adv. Mater. 26, 3024–3034. doi: 10.1002/adma.201304428
Satyam, A., Tsokos, M. G., Tresback, J. S., Zeugolis, D. I., and Tsokos, G. C. (2020). Cell-derived extracellular matrix-rich biomimetic substrate supports podocyte proliferation, differentiation, and maintenance of native phenotype. Adv. Funct. Mater. 30:1908752. doi: 10.1002/adfm.201908752
Schell, J. Y., Wilks, B. T., Patel, M., Franck, C., Chalivendra, V., Cao, X., et al. (2016). Harnessing cellular-derived forces in self-assembled microtissues to control the synthesis and alignment of ECM. Biomaterials 77, 120–129. doi: 10.1016/J.BIOMATERIALS.2015.10.080
Schenke-Layland, K., Rofail, F., Heydarkhan, S., Gluck, J. M., Ingle, N. P., Angelis, E., et al. (2009). The use of three-dimensional nanostructures to instruct cells to produce extracellular matrix for regenerative medicine strategies. Biomaterials 30, 4665–4675. doi: 10.1016/j.biomaterials.2009.05.033
Schmuck, E. G., Mulligan, J. D., Ertel, R. L., Kouris, N. A., Ogle, B. M., Raval, A. N., et al. (2014). Cardiac fibroblast-derived 3D extracellular matrix seeded with mesenchymal stem cells as a novel device to transfer cells to the ischemic myocardium. Cardiovasc. Eng. Technol. 5, 119–131. doi: 10.1007/s13239-013-0167-1
Senthebane, D., Jonker, T., Rowe, A., Thomford, N., Munro, D., Dandara, C., et al. (2018). The role of tumor microenvironment in chemoresistance: 3d extracellular matrices as accomplices. Int. J. Mol. Sci. 19:2861. doi: 10.3390/ijms19102861
Serebriiskii, I., Castelló-Cros, R., Lamb, A., Golemis, E. A., and Cukierman, E. (2008). Fibroblast-derived 3D matrix differentially regulates the growth and drug-responsiveness of human cancer cells. Matrix Biol. 27, 573–585. doi: 10.1016/j.matbio.2008.02.008
Sharma, D., Ferguson, M., and Zhao, F. (2020). A step-by-step protocol for generating human fibroblast cell-derived completely biological extracellular matrix scaffolds. Methods Cell Biol. 156, 3–13. doi: 10.1016/BS.MCB.2019.10.010
Silva, J. C., Carvalho, M. S., Han, X., Xia, K., Mikael, P. E., Cabral, J. M. S., et al. (2019). Compositional and structural analysis of glycosaminoglycans in cell-derived extracellular matrices. Glycoconj. J. 36, 141–154. doi: 10.1007/s10719-019-09858-2
Silva, J. C., Carvalho, M. S., Udangawa, R. N., Moura, C. S., Cabral, J. M. S., da Silva, C., et al. (2020). Extracellular matrix decorated polycaprolactone scaffolds for improved mesenchymal stem/stromal cell osteogenesis towards a patient-tailored bone tissue engineering approach. J. Biomed. Mater. Res. Part B Appl. Biomater. 108, 2153–2166. doi: 10.1002/jbm.b.34554
Subbiah, R., Hwang, M. P., Du, P., Suhaeri, M., Hwang, J. H., Hong, J. H., et al. (2016). Tunable crosslinked cell-derived extracellular matrix guides cell fate. Macromol. Biosci. 16, 1723–1734. doi: 10.1002/mabi.201600280
Suhaeri, M., Noh, M. H., Moon, J., Kim, I. G., Oh, S. J., Ha, S. S., et al. (2018). Novel skin patch combining human fibroblast-derived matrix and ciprofloxacin for infected wound healing. Theranostics 8, 5025–5038. doi: 10.7150/thno.26837
Sun, B., Yu, K.-R. R., Bhandari, D. R., Jung, J.-W. W., Kang, S.-K. K., and Kang, K.-S. S. (2010). Human umbilical cord blood mesenchymal stem cell-derived extracellular matrix prohibits metastatic cancer cell MDA-MB-231 proliferation. Cancer Lett. 296, 178–185. doi: 10.1016/j.canlet.2010.04.007
Sun, Y., Li, W., Lu, Z., Chen, R., Ling, J., Ran, Q., et al. (2011). Rescuing replication and osteogenesis of aged mesenchymal stem cells by exposure to a young extracellular matrix. FASEB J. 25, 1474–1485. doi: 10.1096/fj.10-161497
Syedain, Z. H., Meier, L. A., Lahti, M. T., Johnson, S. L., and Tranquillo, R. T. (2014). Implantation of completely biological engineered grafts following decellularization into the sheep femoral artery. Tissue Eng. Part A 20, 1726–1734. doi: 10.1089/ten.TEA.2013.0550
Tang, C., Jin, C., Du, X., Yan, C., Min, B.-H., Xu, Y., et al. (2014). An autologous bone marrow mesenchymal stem cell-derived extracellular matrix scaffold applied with bone marrow stimulation for cartilage repair. Tissue Eng. Part A 20, 2455–2462. doi: 10.1089/ten.TEA.2013.0464
Tang, C., Xu, Y., Jin, C., Min, B.-H., Li, Z., Pei, X., et al. (2013). Feasibility of autologous bone marrow mesenchymal stem cell-derived extracellular matrix scaffold for cartilage tissue engineering. Artif. Organs 37, E179–E190. doi: 10.1111/aor.12130
Theocharis, A. D., Skandalis, S. S., Gialeli, C., and Karamanos, N. K. (2016). Extracellular matrix structure. Amsterdam: Elsevier, doi: 10.1016/j.addr.2015.11.001
Weber, B., Dijkman, P. E., Scherman, J., Sanders, B., Emmert, M. Y., Grünenfelder, J., et al. (2013). Off-the-shelf human decellularized tissue-engineered heart valves in a non-human primate model. Biomaterials 34, 7269–7280. doi: 10.1016/j.biomaterials.2013.04.059
Wei, B., Guo, Y., Xu, Y., Mao, F., Yao, Q., Jin, C., et al. (2015). Composite scaffolds composed of bone marrow mesenchymal stem cell-derived extracellular matrix and marrow clots promote marrow cell retention and proliferation. J. Biomed. Mater. Res. Part A 103, 2374–2382. doi: 10.1002/jbm.a.35373
Wolchok, J. C., and Tresco, P. A. (2010). The isolation of cell derived extracellular matrix constructs using sacrificial open-cell foams. Biomaterials 31, 9595–9603. doi: 10.1016/J.BIOMATERIALS.2010.08.072
Wolchok, J. C., and Tresco, P. A. (2012). Using growth factor conditioning to modify the properties of human cell derived extracellular matrix. Biotechnol. Prog. 28, 1581–1587. doi: 10.1002/btpr.1625
Woods, T., and Gratzer, P. F. (2005). Effectiveness of three extraction techniques in the development of a decellularized bone-anterior cruciate ligament-bone graft. Biomaterials 26, 7339–7349. doi: 10.1016/j.biomaterials.2005.05.066
Xiao, B., Rao, F., Guo, Z., Sun, X., Wang, Y., Liu, S.-Y., et al. (2016). Extracellular matrix from human umbilical cord-derived mesenchymal stem cells as a scaffold for peripheral nerve regeneration. Neural Regen. Res. 11:1172. doi: 10.4103/1673-5374.187061
Xing, H., Lee, H., Luo, L., and Kyriakides, T. R. (2020). Extracellular matrix-derived biomaterials in engineering cell function. Biotechnol. Adv. 42:107421. doi: 10.1016/J.BIOTECHADV.2019.107421
Xing, Q., Qian, Z., Tahtinen, M., Yap, A. H., Yates, K., and Zhao, F. (2017). Aligned nanofibrous cell-derived extracellular matrix for anisotropic vascular graft construction. Adv. Healthc. Mater. 6:1601333. doi: 10.1002/adhm.201601333
Xing, Q., Zhang, L., Redman, T., Qi, S., and Zhao, F. (2015). Nitric oxide regulates cell behavior on an interactive cell-derived extracellular matrix scaffold. J. Biomed. Mater. Res. - Part A 103, 3807–3814. doi: 10.1002/jbm.a.35524
Xiong, X., Yang, X., Dai, H., Feng, G., Zhang, Y., Zhou, J., et al. (2019). Extracellular matrix derived from human urine-derived stem cells enhances the expansion, adhesion, spreading, and differentiation of human periodontal ligament stem cells. Stem Cell Res. Ther. 10:396. doi: 10.1186/s13287-019-1483-7
Yan, J., Chen, X., Pu, C., Zhao, Y., Liu, X., Liu, T., et al. (2020). Synovium stem cell-derived matrix enhances anti-inflammatory properties of rabbit articular chondrocytes via the SIRT1 pathway. Mater. Sci. Eng. C 106:110286. doi: 10.1016/j.msec.2019.110286
Yang, L., Ge, L., and van Rijn, P. (2020). Synergistic effect of cell-derived extracellular matrices and topography on osteogenesis of mesenchymal stem cells. ACS Appl. Mater. Interfaces 12, 25591-25603. doi: 10.1021/ACSAMI.0C05012
Yang, L., Jiang, Z., Zhou, L., Zhao, K., Ma, X., and Cheng, G. (2017). Hydrophilic cell-derived extracellular matrix as a niche to promote adhesion and differentiation of neural progenitor cells. RSC Adv. 7, 45587–45594. doi: 10.1039/C7RA08273H
Yang, Y., Lin, H., Shen, H., Wang, B., Lei, G., and Tuan, R. S. (2018). Mesenchymal stem cell-derived extracellular matrix enhances chondrogenic phenotype of and cartilage formation by encapsulated chondrocytes in vitro and in vivo. Acta Biomater. 69, 71–82. doi: 10.1016/j.actbio.2017.12.043
Yong, I., Oh, S. W., and Kim, P. (2020). Re-engineered cell-derived extracellular matrix as a new approach to clarify the role of native ECM. Methods Cell Biol. 156, 205–231. doi: 10.1016/BS.MCB.2019.12.007
Yuan, M., Pai, P.-J., Liu, X., Lam, H., and Chan, B. P. (2018). Proteomic analysis of nucleus pulposus cell-derived extracellular matrix niche and its effect on phenotypic alteration of dermal fibroblasts. Sci. Rep. 8:1512. doi: 10.1038/s41598-018-19931-9
Yuan, M., Yeung, C. W., Li, Y. Y., Diao, H., Cheung, K. M. C. M. C., Chan, D., et al. (2013). Effects of nucleus pulposus cell-derived acellular matrix on the differentiation of mesenchymal stem cells. Biomaterials 34, 3948–3961. doi: 10.1016/j.biomaterials.2013.02.004
Zeitouni, S., Krause, U., Clough, B. H., Halderman, H., Falster, A., Blalock, D. T., et al. (2012). Human mesenchymal stem cell-derived matrices for enhanced osteoregeneration. Sci. Transl. Med. 4:132ra55. doi: 10.1126/scitranslmed.3003396
Zhang, W., Yang, J., Zhu, Y., Sun, X., Guo, W., Liu, X., et al. (2019). Extracellular matrix derived by human umbilical cord-deposited mesenchymal stem cells accelerates chondrocyte proliferation and differentiation potential in vitro. Cell Tissue Bank. 20, 351–365. doi: 10.1007/s10561-019-09774-7
Zhang, W., Zhu, Y., Li, J., Guo, Q., Peng, J., Liu, S., et al. (2016). Cell-derived extracellular matrix: basic characteristics and current applications in orthopedic tissue engineering. Tissue Eng. Part B Rev. 22, 193–207. doi: 10.1089/ten.teb.2015.0290
Keywords: cell-derived extracellular matrix, stem cell niche, cell differentiation, tissue engineering, regenerative medicine, skeletal repair, cardiovascular repair, cell-extracellular matrix interactions
Citation: Assunção M, Dehghan-Baniani D, Yiu CHK, Später T, Beyer S and Blocki A (2020) Cell-Derived Extracellular Matrix for Tissue Engineering and Regenerative Medicine. Front. Bioeng. Biotechnol. 8:602009. doi: 10.3389/fbioe.2020.602009
Received: 02 September 2020; Accepted: 10 November 2020;
Published: 03 December 2020.
Edited by:
Xin Zhao, Hong Kong Polytechnic University, Hong KongReviewed by:
Masoud Mozafari, University of Toronto, CanadaCopyright © 2020 Assunção, Dehghan-Baniani, Yiu, Später, Beyer and Blocki. This is an open-access article distributed under the terms of the Creative Commons Attribution License (CC BY). The use, distribution or reproduction in other forums is permitted, provided the original author(s) and the copyright owner(s) are credited and that the original publication in this journal is cited, in accordance with accepted academic practice. No use, distribution or reproduction is permitted which does not comply with these terms.
*Correspondence: Anna Blocki, YW5uYS5ibG9ja2lAY3Voay5lZHUuaGs=
Disclaimer: All claims expressed in this article are solely those of the authors and do not necessarily represent those of their affiliated organizations, or those of the publisher, the editors and the reviewers. Any product that may be evaluated in this article or claim that may be made by its manufacturer is not guaranteed or endorsed by the publisher.
Research integrity at Frontiers
Learn more about the work of our research integrity team to safeguard the quality of each article we publish.