- 1Neuro-computing and Neuro-robotics Research Group, Complutense University of Madrid, Madrid, Spain
- 2Innovation Group, Institute for Health Research San Carlos Clinical Hospital, Madrid, Spain
- 3Department of Biomedical Engineering, Tufts University, Medford, MA, United States
- 4Silk Biomed SL, Madrid, Spain
- 5Ophthalmology Service, La Paz University Hospital, Madrid, Spain
- 6Center for Biomedical Technology, Universidad Politécnica de Madrid, Pozuelo de Alarcon, Spain
- 7Department of Material Science, Civil Engineering Superior School, Universidad Politécnica de Madrid, Madrid, Spain
- 8Biomedical Research Networking Center in Bioengineering, Biomaterials and Nanomedicine, Madrid, Spain
Age-related Macular Degeneration (AMD) is an up-to-date untreatable chronic neurodegenerative eye disease of multifactorial origin, and the main causes of blindness in over 65 y.o. people. It is characterized by a slow progression and the presence of a multitude of factors, highlighting those related to diet, genetic heritage and environmental conditions, present throughout each of the stages of the illness. Current therapeutic approaches, mainly consisting on intraocular drug delivery, are only used for symptoms relief and/or to decelerate the progression of the disease. Furthermore, they are overly simplistic and ignore the complexity of the disease and the enormous differences in the symptomatology between patients. Due to the wide impact of the AMD and the up-to-date absence of clinical solutions, Due to the wide impact of the AMD and the up-to-date absence of clinical solutions, different treatment options have to be considered. Cell therapy is a very promising alternative to drug-based approaches for AMD treatment. Cells delivered to the affected tissue as a suspension have shown poor retention and low survival rate. A solution to these inconveniences has been the encapsulation of these cells on biomaterials, which contrive to their protection, gives them support, and favor their retention of the desired area. We offer a two-papers critical review of the available and under development AMD therapeutic approaches, from a biomaterials and biotechnological point of view. We highlight benefits and limitations and we forecast forthcoming alternatives based on novel biomaterials and biotechnology methods. In this second part we review the preclinical and clinical cell-replacement approaches aiming at the development of efficient AMD-therapies, the employed cell types, as well as the cell-encapsulation and cell-implant systems. We discuss their advantages and disadvantages and how they could improve the survival and integration of the implanted cells.
Introduction
Age-Related Macular Degeneration
Age-Related Macular Degeneration (AMD) is a multifactorial degenerative eye disease, estimated to affect nearly 290 million people by 2040 (Wong et al., 2014). It is characterized by the deterioration of the central retinal area in the elderly population leading to vision deterioration and even blindness [see Therapeutic Strategies for Age-Related Macular Degeneration Part I and Part II, also (Hoon et al., 2014; Wong et al., 2014)].
The retina is the inner component of the eyeball (Figure 1). It is a 10-layers complex structure, where a several types of neural cells, photoreceptors, bipolar, horizontal, amacrine and retinal ganglion cells, are tightly interconnected by means of chemical and electrical synapses to form a network. Visual perception starts at photoreceptors, highly specialized retinal neurons which convert light into electric signals. Retina’s outer sheet, thePhotoreceptors, lies over three non-neural layers: choroid, Bruch’s membrane (BrM) and retinal pigment epithelium (RPE), all of them strongly involved in the development of the AMD (Figure 1; Hoon et al., 2014; Wong et al., 2014). Retina’s inner layer is in contact with the vitreous humor, a non-vascularized transparent gelatinous medium that fills the interior of the eyeball, whose function is to maintain the shape of the eye and smooth the retinal surface to form sharp images.
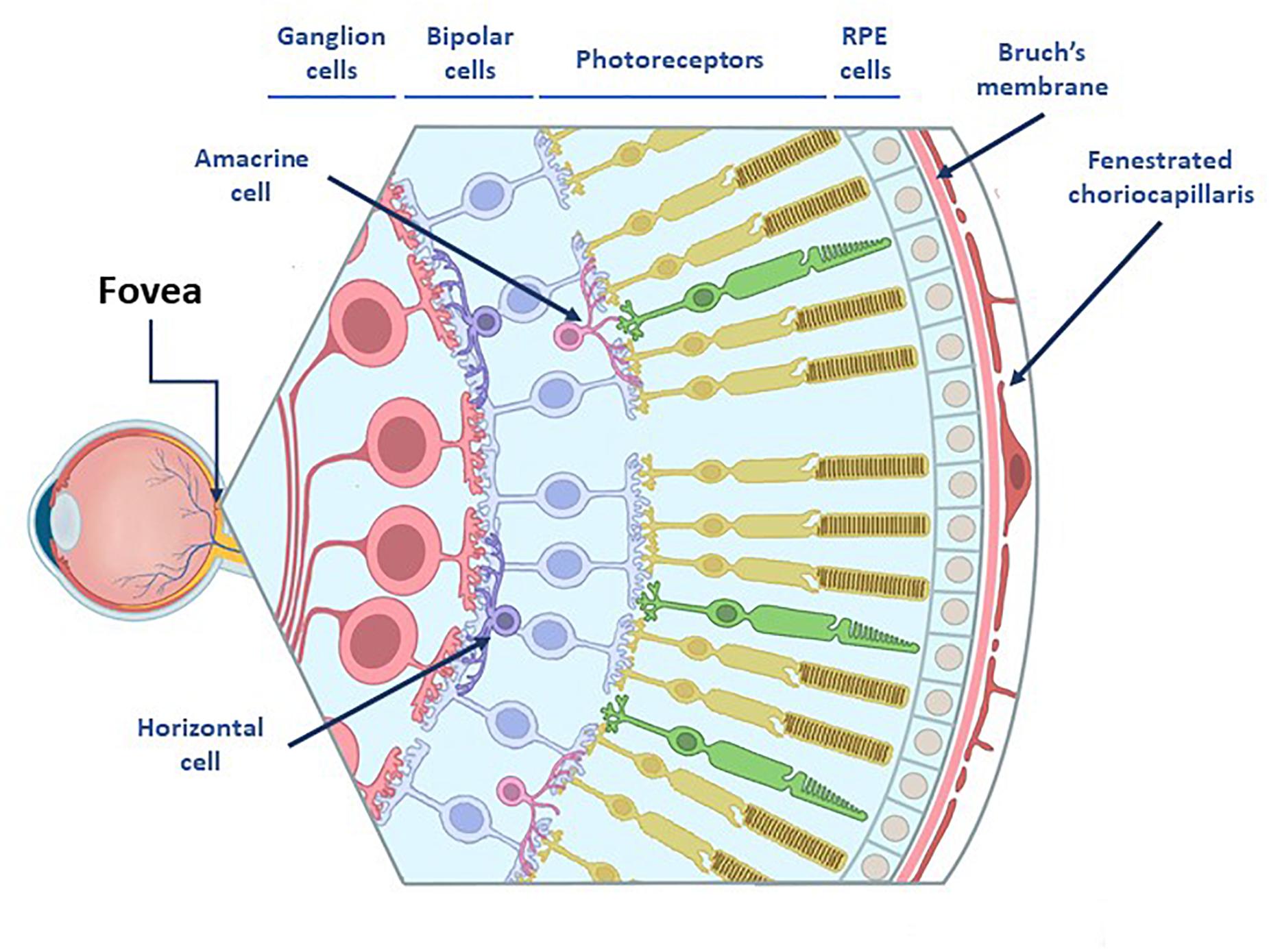
Figure 1. Representation of the eye anatomy. The retina consists on 3 nuclear layers: (I) tanner nuclear layer which consists of ganglion cells bodies whose axons will form the optic nerve (II) the middle nuclear layer which are located the bipolar cell bodies and (III) the outer nuclear layer which includes photoreceptors, those cells lies over three non-neural layers: Choroid, Bruch’s membrane (BrM) and retinal pigment epithelium (RPE) cells. In between the nuclear cell layers are two plexiform layers which contain amacrine and horizontal cells whose function is the modulation of the activity in the bipolar and ganglion cells via lateral inhibition.
The choroid is a vascularized layer that supplies oxygen and nutrients to the whole retina. Additionally, it serves as physical support and it also contributes to the maintenance of the intraocular pressure and the regulation of eye’s temperature (Marmor and Wolfensberger, 1998; Michalska-Małecka et al., 2015). BrM is a 2–5 μm-thick acellular sheet, mainly composed of elastin, collagen I-V, laminin and fibronectin and it is divided into five layers: the choriocapillary basement membrane, the outer collagenous layer, the central band of elastic fibers, the inner collagenous layer and the RPE basement membrane (Figure 2; Hogan et al., 1971; Booij et al., 2010). It gives physical support to the RPE cells and regulates the exchange of molecules, oxygen, nutrients and metabolic residues between the choroid and the RPE (Hogan et al., 1971; Booij et al., 2010). RPE is a monolayer of highly specialized hexagonal cells that are in direct contact with the outer segment of the photoreceptors. It is dedicated to the absorption of scattered light, to the secretion of growth factors, the transport of nutrients from the choroid to the neural cells, the phagocytosis of the outer segment of the photoreceptors and, together with BrM, to the formation of the blood-retinal barrier (Figure 2; Cunha-Vaz, 1997; Campbell and Humphries, 2013). RPE cells play a key role in the maintenance of the visual function and the survival of the photoreceptors (Hoon et al., 2014; Wong et al., 2014) and, similarly to what happens to the neural cells, RPE cells cannot regenerate after birth (Marmor and Wolfensberger, 1998; Bharti et al., 2006; Cunha-Vaz et al., 2011; Jin et al., 2019).
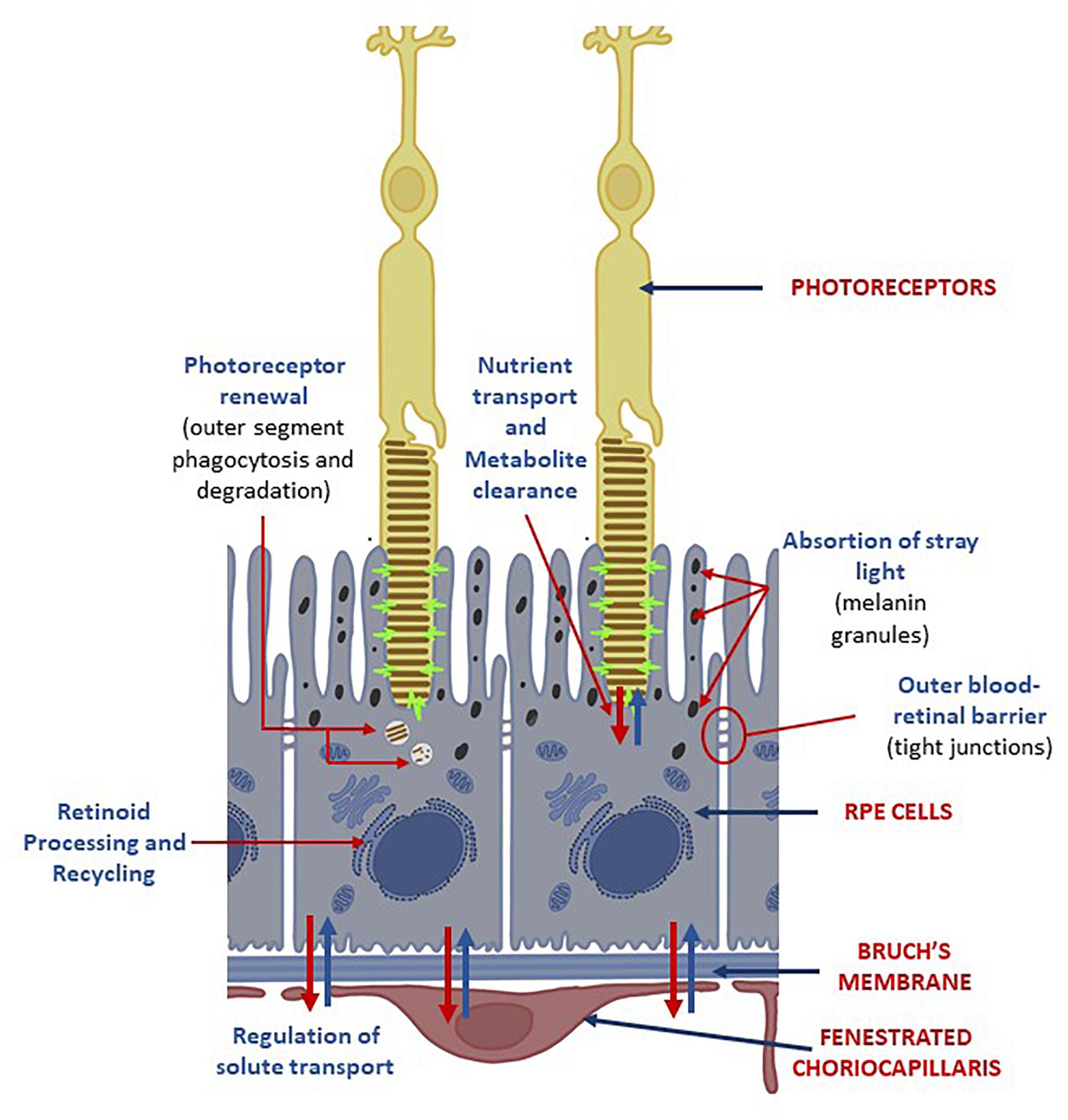
Figure 2. Choroid-RPE-Photoreceptors interactions. RPE cells are located between the light-sensitive outer segments of the photoreceptors and the fenestrated choriocapillaris from the choroid. Its apical membrane faces the subretinal space and it’s specialized to enable interactions between the monolayer of RPE and the outer segments of the photoreceptors. The functions of RPE cells on the neural retina are: (I) absorption of tray light by its abundant melanin granules; (II) retinoid processing and recycling; (III) transport of nutrients and metabolites: (IV) degradation of photoreceptors’ outer segments. Its basolateral membrane is in contact with the Bruch’s membrane. The tight junctions between RPE cells create a selective blood-retinal barrier that regulates the flow of nutrients, metabolic waste products, ions, proteins and water into and out the retina.
Since choroid, BrM and RPE provide physical support for the neural retina, and since they are in charge for both, the interchange of the metabolic molecules and the correct physiological functioning of the photoreceptors, any degenerative pathology affecting one of these layers, would also impair or even kill the photoreceptors with the consequent visual impairment or even blindness. In AMD, BrM weakens and thickens, thus losing its permeability and diffusive properties (Booij et al., 2010). Low permeability and diffusion capability provoke an accumulation of the lipids and the cellular/metabolic residues that are originated in the RPE and the surrounding tissue, giving rise to drusen formation between RPE and BrM. In turn, these changes cause loss of the integrity of the external blood-retinal barrier as well as death of the RPE cells (Marmor and Wolfensberger, 1998; Booij et al., 2010). Due to RPE extensive interactions with the photoreceptors and because RPE cells are unable to regenerate after birth, RPE dysfunction or dead can cause permanent loss of vision capabilities (Marmor and Wolfensberger, 1998; Bharti et al., 2006; Cunha-Vaz et al., 2011; Jin et al., 2019). When alterations occur in the choroid, they give origin to the most aggressive form of AMD, the wet one (W-AMD): choriocapillar alterations provoke uncontrolled angiogenesis, sub-choroidal neovessels penetrate the subretinal space through existing BrM/RPE defects and destroy the morphology and cellular structure of the retinal tissue (Marmor and Wolfensberger, 1998; Michalska-Małecka et al., 2015).
In the human eye, there are approximately 137 million photoreceptors: 7 million cones and 130 million rods. Cones are concentrated in the center of the eye (in the fovea) and their density decreases radially from the center to the periphery. Rods are concentrated at about 20° from the center (approx. 6 mm from the fovea) and their density also decreases toward the periphery but, slower than the cones. Photoreceptors’ degeneration is irreversible because all cellular regeneration and repair processes are inhibited by the unfavorable molecular conditions that prevail throughout the central nervous system. Among them, the absence or inappropriate concentration of neurotrophic and/or neurotropic factors, the appearance of inflammatory processes, astrocytic reactions and inhibitory molecular signals (myelin binding proteins or excess of neurotransmitters and ions), as well as the presence of myelin-associated factors and/or glial scar (Bray et al., 1987; Aguayo et al., 1991; Sauve and Gaillard, 1995; Liu et al., 2006).
Drugs, molecules and factors’ administration are the prevalent therapeutic options today, none of them being a therapy for the disease. They only contribute to slow down the progression of the pathology, with the aggravating factor that they are effective in only a small percentage of patients (Gehrs et al., 2006; Sabel et al., 2011; Irisvision, 2020). For this reason, in the last decades, serious efforts have been done to develop new, more effective treatments based either on the implant of stem cells that are used as micro-factories producing in situ neuroprotective and neuroregenerative biomolecules (Emerich and Thanos, 2008; Augustin et al., 2012; Guerrero-Naranjo et al., 2013; Barar et al., 2016), or on the replacement of damaged cells by autologous or allogeneic cell transplants (Gaillard and Sauvé, 2007; MacLaren and Pearson, 2007; Singh and MacLaren, 2011; Bharti et al., 2014; Singh et al., 2020).
The Immunoprivileged State of the Eye
The inflammatory response in the eye is regulated by its own immunosuppressive microenvironment, a series of complex regulatory systems which include vasoactive peptides (α-melanocyte-stimulating hormone, a regulator of the adaptive immune response, and calcitonin gene-related peptide), macrophage migration-inhibitory factor, and soluble CD95L (which regulates the innate immune response) (Taylor, 2016). Furthermore, the eye complement system plays an important role in the production of inflammatory cytokines (Goslings et al., 1998). Multiple complement factors have been described in the eye of both, human and mice (Anderson et al., 2010; Luo et al., 2011), which can be regulated by the CD46, CD55, CD59, and Crry proteins, expressed by microglia and RPE cells.
However, at the same time, the eye is one of the few immunoprivileged tissues, characterized by the presence of negative regulators which prevent the activation of local inflammatory processes (Medawar, 1948) and where implants can survive for an extended period of time (Streilein, 2003). The biological role of this immunological privilege is to avoid vision deterioration in case of overreaction of the immune system (Sandhu et al., 2019).
Immunoprivileged conditions are the result of a synergy of physical, molecular and cellular barriers (Taylor, 2016) implemented by inflammation suppressors and down-regulators of the immune system, located either on cell membrane or the extracellular tissue (Taylor and Ng, 2018).
Blood-retina barrier and the indirect draining of the ocular microenvironment by the lymphatic system constitute the physical barriers. Blood-retina barrier is formed by the endothelial junctions of RPE cells which impede immune system cells to leak into the eye (Niederkorn et al., 1981). The indirect draining of the eye eliminates the necessity of a channel to the interior of the eye, which increases the difficulty for the immune system to reach the ocular tissue and enhances the efficiency of the blood-retina barrier. TGF-β2 and other soluble immunomodulatory molecules present in the aqueous humor constitute the molecular barrier, whose role is to attack and neutralize the cells of the immune system and to control inflammation (Taylor, 2016). Three antigen-presenting cell types, microglia, perivascular macrophages and dendritic cells, are believed to form the cellular barrier (Forrester and Xu, 2012): macrophages participate in the maintenance of vascular homeostasis in the retina and are believed to be capable of antigen presentation, although microglia is the primary antigen-presenting cell type of the eye.
Age-related macular degeneration-originated damages of the RPE alter this state of the eye. Cellular and tissue engineering AMD therapy procedures, either injections or surgical implants, also contribute to the worsening of the situation.
Cell and Tissue Engineering Therapies
The retina, the tissue most severely by AMD is an easy target for cell and tissue engineering therapeutical approaches, because of its location and its small size, which allow easy surgical accessibility and transplantation of smaller amounts of replacement cells compared to other organs. Besides, the availability of a large number of clinical variables for the assessment of the visual function, facilitated the precise evaluation of the effectiveness of novel AMD therapies (Bennett et al., 2019) and fostered the development of several regenerative approaches based on BrM, RPE and photoreceptor cells replacement (Chichagova et al., 2018).
Biomolecules can be used for therapeutic reasons on a neural tissue, through two, non-mutually exclusive mechanisms. On one side, through the application of neuroprotective and neuroregenerative factors (directly injected to the neural tissue or secreted from implanted stem cells) which diffuse into the host tissue and rescue the degenerating cells. On the other, by implanting healthy cells into the host tissue and replacing the damaged/degenerated cells by the new ones. In the first case, to avoid molecules/cells dispersion into the host tissue, encapsulation can be employed. Porous materials permits the signals of the damaged environment to reach the implanted cells and stimulate the production of neuroprotective biomolecules, as well as to allow the diffusion of the secreted molecules to the damaged tissue (Battler and Leor, 2006; Weiner, 2008; Seiler and Aramant, 2012; Lin et al., 2017; Fernández-García et al., 2018; González-Nieto et al., 2018). However, although neuroprotective factors could protect retinal cells before apoptosis occurrence, their application is not a viable solution for many patients with advanced retinal pathologies. In the second case, cell replacement strategies get advantage of the encapsulation of therapeutic cells in biomaterial formats with an optimal mesh size to obtain a functional connection of donor cells with host tissue; however, this strategy faces the risk of a higher exposition to inflammatory responses that can be detrimental for the grafted cells as well as the risk of rejection of the allogenic material by the host tissue (Figure 3).
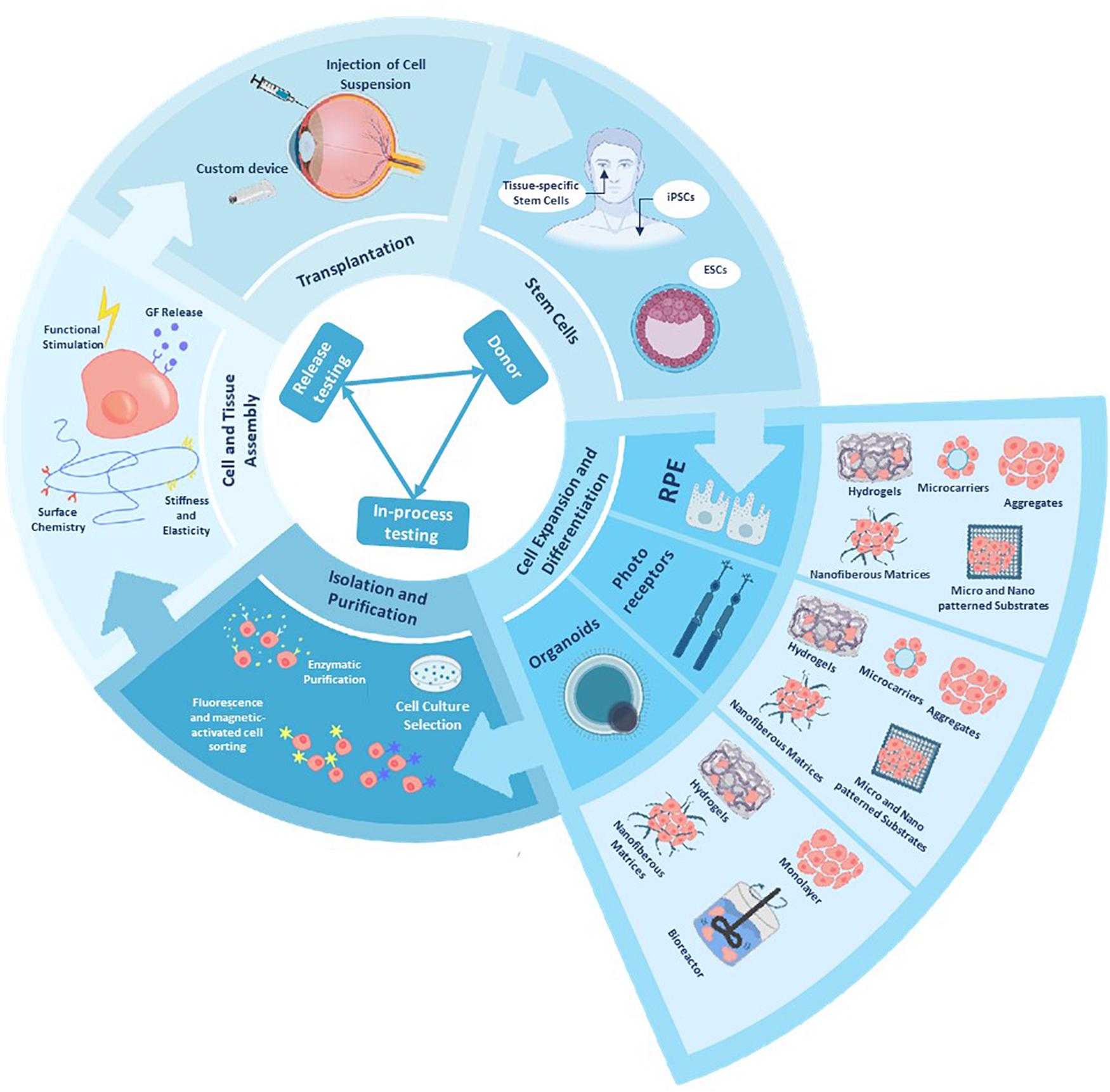
Figure 3. Schematic representation of a tissue bioengineering model, applied to cell and tissue manufacture and delivery. Manipulating and differentiating stem cells into different retinal cells is a challenging with common goals as achieving manufacturing standard and testing for each clinical stage as well achieving an efficient manufacturing and banking. Bioengineering approaches can provide us with scalable 3D cell culture systems that allow differentiation into different retinal cells, biomanufacturer and bioassembly of functional eye tissues and delivery vehicles for an in vivo model [after (Stern et al., 2018)].
Cell Sources for Retinal Implants
Cells source is a key component to the success of any cell therapy. We can identify three main sources for retinal implants:
Embryonic or Fetal-Derived Stem Cells
They represent an attractive source due to their ability to self-renew and differentiate into any type of cell in the body (pluripotent). However, they also present serious disadvantages, e.g., difficult and expensive cell differentiation and cell expansion protocols, complex bioengineering processes, tumor formation risks, rejection risks, possibility of harboring donor’s genetic defects, possible ethical challenges, etc. (Arnhold et al., 2004; Aoki et al., 2009; Lu et al., 2009; Falkner-Radler et al., 2011; Schwartz et al., 2012; Carr et al., 2013; Gonzalez-Cordero et al., 2013; Jayakody et al., 2015; Lu and Barnstable, 2017).
Adult Tissue-Derived Stem Cells
They are a source of autologous multipotent cells. However, these cells could be hard to obtain and they could harbor the genetic cause of the disease (West et al., 2009; Ballios and van der Kooy, 2010; Mead et al., 2013, 2015; Hertz et al., 2014; Park et al., 2017).
Induced Pluripotent Stem Cells
They are a relatively new source of stem cells developed by directly reprogramming adult somatic cells to transit to a pluripotent state. The use of iPSCs is exciting because iPSCs can be derived from the patient’s own tissue and are associated with fewer ethical concerns than ESCs. Using a combination of soluble factors, iPSCs can be expanded and differentiated into various types of retinal cells, including rods, cones, and retinal ganglion cells. They present a very low rejection risk, however, they could conserve epigenetic characteristics of the original cells, harbor the disease genes from the donor and lead to tumor formation (Rowland et al., 2012; Kamao et al., 2014; Clegg et al., 2015; Mead et al., 2015; Fields et al., 2016; Rajala and Gardner, 2016; Bhattacharya et al., 2017; Bracha et al., 2017). The positive outcomes of early-phase trials postulate iPSCs as the most promising choice (Table 1; Chichagova et al., 2018).
Encapsulation of Biomolecule-Producing Stem Cells
At the present, cell encapsulation represents the preferred therapeutic approach since direct injection of cells has been proven to be suboptimal, due to cell losses from the rapid dispersion into the host tissue, along with glia and host immune system attacks.
In vitro Studies
In their attempt to create artificial BrM, (McCormick et al., 2020) generated a poly (ethylene terephthalate) (PET) scaffold with poly (lactic acid-co-glycolic acid) (PLGA) or poly (glycolic acid) (PGA) degradable nanoparticles that exhibited a continuous release of a fluorescent dye from PLGA particles for 2 weeks and from PGA particles for 1 day.
For cell encapsulation Bhatt et al. (2019) focused on composites by encapsulating retinal pigment epithelial cell line-19 model (ARPE-19) in PLGA nanoparticles of sunitinib malate, which in turn were included in thermosensitive hydrogels (from methoxy poly (ethylene glycol)-b-copolymers of polycaprolactone (mPEG-PCL)), to increase the residence time of the particles in the vitreous humor. In an in vitro study they showed that their device had better drug absorption, increased antiangiogenic potential, and prolonged inhibition of vascular endothelial growth factor (VEGF) activity compared to the free drug solution (Bhatt et al., 2019).
Clinical Trials
NT-501, NT-503 and iTrack275 Microcatheter, three products developed for the encapsulation of biomolecule-producing stem cells, are aiming at obtaining Food and Drug Administration (FDA) approval for AMD treatment (Table 2):
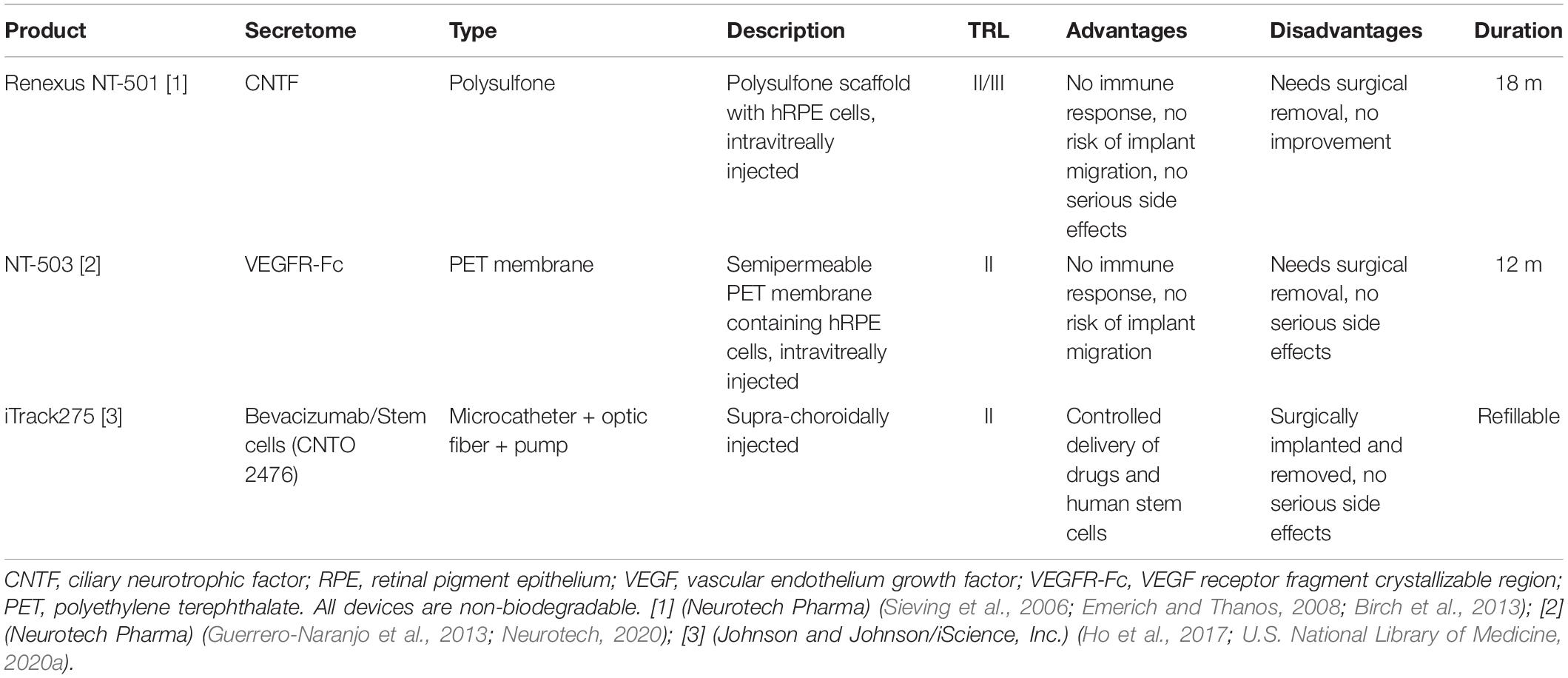
Table 2. Cell encapsulation technologies for solid implantable devices integrating stem cells for in situ production of therapeutic biomolecules.
NT-501 (Renexus, Neurotech Pharma) is a non-biodegradable polysulfone scaffold to encapsulate genetically engineered human RPE cells. The device is injected intravitreally and provides a sustained delivery of ciliary neurotrophic factor for months. The device is sutured into the sclera by a titanium loop to avoid migration risks and requires surgery to get removed. The device is in Phase III clinical trials and it has been effective for up to 18 months providing photoreceptor protection (Tao et al., 2002; Bush et al., 2004; Sieving et al., 2006; Emerich and Thanos, 2008; Kuno et al., 2011; Birch et al., 2013; Bisht et al., 2017; U.S. National Library of Medicine, 2020b, g).
NT-503 (Neurotech Pharma) is a non-biodegradable polyethylene terephthalate semipermeable hollow fiber membrane that encapsulates genetically engineered human RPE cells that synthesize and secrete a VEGF receptor fragment crystallizable region. This device is implanted intravitreally through a peritomy and subsequent 3 mm sclerotomy and it has been shown to maintain therapeutic effects for 12 months in W-AMD and was 20 times more efficient than injections of Ranibizumab. As with NT-501, the device gets fixed to the sclera to avoid migration and also requires surgery to be removed. This device is in Phase II clinical trials (Sieving et al., 2006; Guerrero-Naranjo et al., 2013; Rupenthal, 2017; U.S. National Library of Medicine, 2020u).
iTrack275 Microcatheter (iScience Interventional) is a micro-calibrated pump for supra-choroidal drug/cell delivery, surgically inserted through a non-biodegradable microcatheter that includes an optical fiber illuminator to guide the insertion of the device. This suprachoroidal pump allows an improved supply of drugs, a longer duration and a greater penetration into the tissue due to the proximity to the choroid and the BrM, with the consequent avoidance of blurred vision, changes in the lens and in the vitreous (traction exerted), compared to pharmacological delivery by intravitreal or periocular injection. The device has been used in combination with Bevacizumab in advanced AMD and with cells derived from human umbilical tissue (CNTO 2476) for dry-AMD (D-AMD), showing good safety and efficacy with no serious complications (Kuno et al., 2011; Augustin et al., 2012; Pearce et al., 2015; Bansal et al., 2016; Rupenthal, 2017). Phase I/IIa trials were performed with CNTO 2476 using iTrack275, for the administration of a single subretinal dose. Although, it was associated with a high rate of retinal perforations and retinal detachments, when the cells were “sequestered” by the subretinal space, it was well tolerated and was associated with some improvement in visual acuity. Larger studies are required to confirm these results (Ho et al., 2017; U.S. National Library of Medicine, 2020a).
In situ Cell Replacement
BrM-RPE Transplantation
Early attempts
Early attempts of AMD tissue reconstruction consisted of either autologous (translocated explants) or allogenic (fetal origin) RPE transplants, to replace the damaged retinal tissue (Steindl and Binder, 2008) by substituting the whole choroid-BrM-RPE complex (Ruysch’s complex) via an RPE-choroid explant or by an RPE layer alone. Despite some promising results on the autologous strategy in a small portion of patients (van Zeeburg et al., 2012) aiming at slowing the progression of the pathology and/or improving visual capability, these therapeutic approaches were abandoned due to a number of serious drawbacks. The most significant problems were the limited availability of autologous tissue and the maintenance of genetic risks to develop the disease. Even though allogenic transplants showed better results, today, RPE transplants with natural membranes have been totally replaced by implants based on biomaterials and eye/stem cells. However, the beneficial effects of these autologous and allogenic transplants served as a proof-of-principle for the subsequent cell therapy strategies (Hynes and Lavik, 2010; Yao et al., 2011; Kim et al., 2012; Tam et al., 2014).
In vitro and pre-clinical trials
Retinal pigment epithelium degeneration can lead to the development of more advanced AMD stages so, transplants of healthy RPE cells have been considered as candidates for the deceleration of the disease (Ambati and Fowler, 2012). Vision improvement of AMD patients subject to RPE cell transplantation supported this approach (van Zeeburg et al., 2012). The specific requirements for improving physiological functions and fostering the survival of RPE cells, are thickness, flexibility, permeability and biodegradability of the implant, as well as easiness to handle during surgical intervention (Hynes and Lavik, 2010). Artificial BrM needs to be biocompatible while promoting and maintaining a proper RPE phenotype. Good integration with the choroid is also sought. In addition, artificial membranes must be porous, thin (less than 10 μm, mimicking BrM) and mechanically competent to withstand manipulation during surgery. Since damaged BrM can also compromise the success of cell transplantation, several researchers dedicated their efforts to the development of artificial membranes allowing successful transplantation of RPE cells (Srivastava et al., 2011; Hu et al., 2012; Warnke et al., 2013; McHugh et al., 2014; Stanzel et al., 2014; Ilmarinen et al., 2015; Peng et al., 2016; Calejo et al., 2017; Harris et al., 2019; McCormick et al., 2020).
A number of artificial scaffolds seeded with RPE cells, mimicking a healthy BrM-RPE complex, have been investigated. Collagen has been one of the most experimented materials because it is one of the fundamental components of BrM: (Lu et al., 2007) cultured human ARPE-19 cells in 2.4 μm-thick collagen films confirming cell attachment and viability at 25 days and a suitable cell phenotype. Other polymeric substrates have been tested in vitro and/or in vivo with comparable results: gelatin (Del Priore et al., 2004; Lai, 2013; Rose et al., 2014), poly-methyl-methacrylate (PMMA) (Tao et al., 2007), modified polytetrafluoroethylene (PTFE) (Maminishkis et al., 2016), polyethylene terephthalate (PET) (Kamao et al., 2014; Liu et al., 2014, 2018; Jin et al., 2018), polyester matrix membranes (Liu et al., 2014; Stanzel et al., 2014), poly-caprolactone (PCL) (Redenti et al., 2008; McHugh et al., 2014; Tam et al., 2014), poly(L-lactic acid) blends (PLLA/PLGA) (Giordano et al., 1997; Lu et al., 1998; Hadlock et al., 1999; Warnke et al., 2013), polyethylene glycol di-methacrylate (PEGDMA) (Singh et al., 2001), polydimethylsiloxane (PDMS) (Peng et al., 2016). Silk fibroin was also used as a versatile material for scaffolds since it has a number of functional groups that can react with biomolecules of interest like arginine–glycine–aspartic acid (RGD) (Chirila et al., 2008, 2015; Wenk et al., 2011; Kundu et al., 2013; Xiang et al., 2014; Zhang et al., 2015), all of them in experimental phase.
Synthetic BrMs consisting of PET scaffolds and degradable nanoparticles were developed by McCormick et al. (2020). ARPE-19 cells cultured on these scaffolds were able to form monolayers and were maintained for up to 3 months without any cytotoxic effect. This proof-of-concept showed the potential of this nanoparticle-scaffold system for future RPE transplantation. In another synthetic BrM, composed of collagen type I and PLGA, cultured human RPE (hRPE) showed polarity, phagocytic activity, and native RPE characteristic morphology and they were maintained alive for at least 11 days (Warnke et al., 2013). Calejo et al. (2017) developed microporous films comprised of copolymer 96/4 L-lactide/D-lactide with deposited layers of collagen type I and IV. These films simulated BrM characteristics and acted as a support for cultured human embryonic stem cell-derived RPE (hESCs-RPE) cells. These cells were maintained alive for 8 weeks and revealed good adhesion, morphology, and expressed RPE markers. In a different study, fetal human RPE (fhRPE) cultured on porous PCL scaffolds for 8 weeks showed positive results regarding survival, mature and functional RPE markers expression, barrier function, etc. (McHugh et al., 2014). Plasma-modified PDMS with laminin (PDMS-PmL) scaffolds were also used as an artificial BrM. Pluripotent differentiated RPE (dRPE) cells cultured on these scaffolds revealed good adhesion, proliferation, polarization, maturation, and functionality. Also, PDMS-PmL could sustain a multilayer of dRPE cells and precursors of photoreceptors. Furthermore, the PDMS-PmL-RPE subretinal implant in vivo in porcine eyes confirmed the biocompatibility of the material for a 2-year period (Peng et al., 2016). In another study, films made of elastin-like recombinamers (ELRs) were investigated: ARPE-19 cells cultured with a bioactive sequence (RGD), maintained their phenotype for up to 5 days and no cytotoxicity was detected (Srivastava et al., 2011).
Stanzel et al. (2014) tested the viability of a subretinal transplant of hESCs-derived RPE cells on a polyester matrix in rabbits. 1-month post-implantation, hESCs-derived RPE remained viable, maintaining their polarization and monolayer structure. hESCs-derived RPE cultured on ultrafine porous polyimide (PI) membranes survived for 6 weeks after subretinal injection in rats, which also showed some functional rescue of electroretinography signal (Ilmarinen et al., 2015). hESCs-RPE on PI transplanted subretinally into rabbit’s eyes showed good membrane tolerability but low RPE cells survival (Ilmarinen et al., 2015).
Spider silk, a very promising biomaterial for artificial BrM, was also tested in vitro. This silk fibroin-based membrane allowed ARPE-19 cells to develop a morphology and physiology very similar to those of native RPE cells, as it was shown by the morphological studies and the analysis of their protein expression profiles. This native-like phenotype remained in the silk fibroin films for up to 7 days (Harris et al., 2019). In a very recent study (Jemni Damer et al., 2020) built a multilayer 3D biohybrid retina with RPE, Müller and retinal neural cells on silkworm silk fibroin biofilms, glued with silk fibroin hydrogels. Both, RPE and neural retinal cells survived in vitro for the 7 days of the experiment.
In addition to the above substrates, expanded PTFE (ePTFE)-modified membranes were developed to serve as a substrate for RPE growth (Krishna et al., 2011). The surface of the membranes was modified by an ammonia gas plasma treatment, which resulted in ARPE-19 cells attachment and enhanced proliferation. ARPE-19 cells grew in a monolayer and displayed phagocytic capacity. Parylene polymer has also been used as support for the growth and subretinal implantation of hESCs-RPE cells in rats, allowing post-implant cell survival (Hu et al., 2012).
Hsiung et al. (2015) showed that RPE cells derived from hESCs, implanted in the subretinal space within a supporting biomaterial, had higher survival rates than injections of cell suspensions. Previous studies testing artificial RPE strata built with hESCs growing on thin polymeric sheets obtained good results (Redenti et al., 2008; Liu et al., 2012, 2013). Functional integration into the host system is not easy, as it requires migration to the target nuclei, differentiation into the correct cell type for integration into the existing circuitry, and restoration of long-term function. All these steps have to be done by the stem cells while exposed to the hostile conditions of the degenerating retina. Almost all current tissue reconstruction approaches are in the experimental phase, either in vitro or in vivo, with only a few of these are in clinical trials (see Tables 3, 4).
Clinical trials
Clinical trials of RPE transplantation include both, injections of suspensions of RPE cells to the posterior segment of the eye and implants of RPE monolayers (alone or in a substrate) between the retina and the native RPE cells.
Safety and tolerability of subretinally transplanted hESC-RPE cells in suspension in patients with advanced D-AMD has been assessed in a Phase I/II trial (U.S. National Library of Medicine, 2020m). The transplanted cells were well tolerated up to 4 months post-injection (Schwartz et al., 2012) and no adverse effects were detected in the patients in the next 22 months (Schwartz et al., 2015). Another Phase I/II Trial with the same objective is currently recruiting patients (U.S. National Library of Medicine, 2020k).
Regarding RPE monolayers transplantation, a Phase I Trial tested the safety of an ESCs-RPE patch on a vitronectin-coated polyester membrane subretinally implanted in two patients affected by W-AMD (U.S. National Library of Medicine, 2020c). Implants were well tolerated and resulted in a gain in visual acuity in both patients (da Cruz et al., 2018). A Phase I/II Trial to assess the safety and tolerability of subretinally implanted hESCs-RPE on a non-degradable Parylene membrane in D-AMD patients is underway (U.S. National Library of Medicine, 2020m).
Parylene and PLGA membranes are in Phases I/II and Phase I/II Clinical Trials, respectively (Liu et al., 2012; Lu et al., 2012; Diniz et al., 2013; U.S. National Library of Medicine, 2020m). A Phase I/II Trial to assess the safety and tolerability of subretinally implanted hESCs-RPE on a non-degradable Parylene membrane in D-AMD patients is underway (U.S. National Library of Medicine, 2020m). Also, Phase I/II Trial for the subretinal implant of a PLGA biodegradable scaffold, carrying autologous iPSC-derived RPEs in patients affected by AMD-associated geographic atrophy is currently recruiting patients (U.S. National Library of Medicine, 2020d).
It is worthy to state that a sheet of iPSC-derived RPE implanted in a W-AMD patient after removal of the neovascular membrane did not show visual acuity improvement and provoked a macular edema 1 year after surgery (Mandai et al., 2017a), while a Japanese Phase I Trial of RPE monolayers of iPSC-derived RPE cells, implanted as strips with no substrate, began in early 2014 was suspended in that same year.
Resuming, up to now, both, RPE cells injected in suspension and implanted as monolayers on artificial BrMs showed good preliminary results in terms of safety and tolerability. RPE cells injected in suspension have several drawbacks such as the necessity to migrate from the injection site and the tendency of suspended RPE to de-differentiate, problems not present in implanted scaffolded RPE monolayers. No positive results are available in terms of AMD therapy and visual acuity recovery.
Photoreceptors’ Transplantation
General considerations
Different types of cells have been used for neural retinal cell replacement, including photoreceptor precursor cells (PPCs), photoreceptors, and retinal stem cells (RSCs) (see (Lakowski et al., 2011; Tucker et al., 2011; Eberle et al., 2012, 2014; Pearson et al., 2012; Barnea-Cramer et al., 2016) for the former, (Yang et al., 2010; Gust and Reh, 2011; Pearson et al., 2012; Singh et al., 2013; Gagliardi et al., 2018; Garita-Hernandez et al., 2019; Lorach et al., 2019) for the second and (Singh et al., 2020) for the latter. The sources of RSCs can be either endogenous, including neuronal stem cells, or exogenous, including ESCs and iPSCs (Tu et al., 2019).
Similarly to other types of cells, also photoreceptors can be transplanted, either to secret immunomodulatory and/or neuroprotective factors that foster the protection and recovery of the damaged cells or to replace the damaged photoreceptors by new ones (Singh et al., 2020). Photoreceptors’ replacement can be a good therapeutic strategy when, in advanced AMD stages, this type of cells become dysfunctional and eventually die as a result of RPE cells’ deterioration and death (Bhutto and Lutty, 2012). Fortunately, there is a wide time-window for therapeutic interventions, because inner retinal layers’ neurons can survive for prolonged periods of time after photoreceptors degeneration, offering the possibility for cell transplantation to be successful (Medeiros and Curcio, 2001; Jones et al., 2003). However, to make synapses with the bipolar cells and to reverse the synaptic remodeling that takes place after photoreceptors degeneration, implanted photoreceptors need to be functional, a very hard to achieve condition (Jones et al., 2012; Mandai et al., 2017a).
Although post-mitotic transplanted photoreceptors have been proved to be able to regain relative vision functionality (Pearson et al., 2012; Barber et al., 2013), to date no one has achieved a significant restoration of visual function.
In vitro and pre-clinical trials
Photoreceptor transplantation without biomaterials
Similarly to RPE cells, photoreceptors transplantation to the diseased eye can be done either by injection of dissociated cells suspension (see Table 5) (Gust and Reh, 2011; Pearson et al., 2012; Barber et al., 2013; Gagliardi et al., 2018; Garita-Hernandez et al., 2019) or by implanting them as a sheet of tissue, cultivated on a biomaterial substrate (Yang et al., 2010). If the outer nuclear layer, the layer of the retina formed by the bodies of rods and cones, has already degenerated, the latter strategy would be preferable due to the difficulty of the injected cells to get integrated to the damaged tissue and reconstruct a new layer.
Subretinal injection of a suspension of photoreceptors from embryonic-postnatal mice showed that donor cells integrated well in the host retina and the morphology and marker expression of donor cells were similar to those of the photoreceptors of the host retina (Gust and Reh, 2011).
Early postnatal rat photoreceptors implanted as a sheet to a model of dominant retinitis pigmentosa slowed cone loss 6 months after surgery (Yang et al., 2010). Rod precursors from postnatal mice injected as dissociated cells formed synaptic connections with bipolar and horizontal cells in the retina of Gnat12/2 mice (congenital stationary night blindness model) and responded to light stimuli (Pearson et al., 2012). Also, early postnatal mice rod-photoreceptors, transplanted as a cell suspension into 6 different mice models of retinal degeneration, got integrated into the host tissue with better results in the Prph2+/Δ307 model (retinitis pigmentosa model) (Barber et al., 2013). hiPSC-derived CD73+ photoreceptors isolated and injected in clusters in the eyes of immunosuppressed rats survived, and matured for 10 weeks (Gagliardi et al., 2018). Rat RPE/photoreceptors’ grafts transplanted in a retinal degeneration rat model protected the host photoreceptor layer from degeneration 6 months after surgery. The graft integrated well with the host tissue and created synapses with the bipolar cells (Lorach et al., 2019).
An interesting approach was taken by Garita-Hernandez et al. (2019). They introduced a hyperpolarizing microbial opsin in photoreceptors extracted from P4 mice and transplant them as a cell suspension into transgenic blind mice lacking the whole layer of photoreceptors (Cpfl1/Rho-/- and C3H rd/rd mice) who recovered their visual function. The same authors obtained hiPSCs-derived cones expressing NpHR (Natronomonas pharaonis halorhodopsin); blind mice that received them also as cell suspension, displayed signs of retinal repair 4 weeks post-injection (Garita-Hernandez et al., 2019).
Photoreceptor transplantation with biomaterials
Both synthetic polymers and biological materials can be used in the fabrication of scaffolding structures for photoreceptors implants: natural materials like alginate, collagen, etc., are suitable for imitating natural architectures (Ramakrishna et al., 2001); synthetic polymers, such as poly-caprolactone (PCL) and poly (lactic-co-glycolic) acid (PLGA), offer higher mechanical strength and controllable degradation rates (Chen et al., 2011) although the latter usually cause less biological activity (Yang et al., 2017).
Scaffolds for retinal progenitor cells (RPCs) transplants can be cylindrical, mimicking the vertical disposition of cells in the retina, fibrous, mimicking the microstructure of the extracellular matrix or made by hydrogels, to mimic the mechanical properties of the retina (Kador and Goldberg, 2012). The employment of 2D–3D scaffolds has been proved to be an efficient strategy to overcome the limitations of free cell injections, like low survival, low cell integration at the injection site and difficulty to maintain the injected cells in the target areas (Behtaj et al., 2020).
A 3D biodegradable poly-caprolactone (PCL)-microfabricated scaffold promoted good RPCs retention in vitro (Sodha et al., 2011). Another biodegradable PCL scaffold, in this case subretinally implanted in porcine eyes, showed good tolerability without retinal neither choroid inflammation (Christiansen et al., 2012). PCL scaffolds with varying surface topographies enhanced mice RPCs differentiation toward photoreceptor phenotype in vitro. Transplanted RPCs-PCL integrated well into the host retina and expressed specific markers of photoreceptors (Yao et al., 2015). And (Lawley et al., 2015) found that vitronectin-simulating peptides into a PCL film increased hRPCs adhesion, inhibited hRPCs proliferation and induced differentiation to photoreceptors phenotypes. Transplanted cells into mice degenerating retina, migrated to the outer nuclear layer and survive for 3 weeks.
In a multiple substrates study, RPCs showed good adhesion on poly-l-lysine, fibronectin, laminin, hyaluronic acid, and matrigel substrates in vitro (Thakur et al., 2018).
The fabrication of hybrids has also been explored. Poly-caprolactone (PCL)-retinal extracellular matrix hybrids allowed adhesion of the hRPCs cells and helped their differentiation toward the photoreceptor phenotype in vitro (Baranov et al., 2014). Interphotoreceptor matrix employed as a scaffolding material for hRPCs showed good cellular attachment absence of cytotoxicity and hRPCs differentiation toward the photoreceptor phenotype in vitro (Kundu et al., 2018). Tucker et al. (2010) tested a biodegradable cell delivery platform made of pre-activated MMP2 into a poly(lactic-co-glycolic) acid polymer in vitro and in vivo. Active MMP2 was released in a controlled manner and allowed RPCs to migrate into the outer nuclear layer and adopt a photoreceptor phenotype.
Jung et al. (2018) developed a 3D cup-shaped scaffold with μm-sized structures in ultrathin biocompatible elastomer films. These films were composed of a non-biodegradable part plasma-modified polydimethylsiloxane (PDMS) and a biodegradable part poly (glycerol-sebacate) (PGS) and were designed to deliver photoreceptors in a polarized manner. hPSCs-PRs showed polarization and robust survival in vitro 3 months post-seed in the scaffold.
Table 6 shows the most relevant studies for biomaterials-encapsulated progenitor cells/photoreceptors.
Clinical trials
Although preclinical studies have shown that all, RPCs, photoreceptor precursors and mature photoreceptors could potentially replace damaged retinal cells, clinical trials for these approaches are limited.
A Phase II study in which human fetal neural retinal tissue and RPE were transplanted together in a group of AMD patients, showed promising results (Radtke et al., 2008; U.S. National Library of Medicine, 2020o). Interestingly, the vision improvement observed in treated patients began 6 months after transplantation, the time predicted for the fetal cells to differentiate into mature photoreceptors. Another phase I/II study used HuCNS-SC® (human central nervous system stem cells) injected subretinally in AMD patients (U.S. National Library of Medicine, 2020r). This study was completed in 2015 but no results have been posted yet. Besides, a long-term follow-up safety study from the same sponsor has terminated based on, as posted, a business decision unrelated to safety concerns (U.S. National Library of Medicine, 2020i). Another clinical trial using HuCNS-SC® was terminated due to the same reason (U.S. National Library of Medicine, 2020q).
Bone marrow-derived stem cells have also got promising results in a Phase I/II Trial: NCT01920867 (Weiss et al., 2015; U.S. National Library of Medicine, 2020p). Another Phase I/II Trial testing bone marrow-derived stem cells, proved their safety: NCT01518127 (Cotrim et al., 2017; U.S. National Library of Medicine, 2020h).
Three ongoing clinical trials are using fetal-derived RPC for photoreceptor’s replacement in retinitis pigmentosa patients. ReNeuron is carrying a phase I/II trial to evaluate the safety, tolerability and preliminary efficacy of hRPCs, administered as a suspension by a single subretinal injection (U.S. National Library of Medicine, 2020l). An already completed phase I/II study testing hRPCs injected intravitreally, showed that hRPCs were safe and well-tolerated at doses up to 3 million cells (U.S. National Library of Medicine, 2020n). A phase IIb clinical trial designed to assess efficacy of hRPCs is currently active (U.S. National Library of Medicine, 2020j). A phase I study to determine the safety of CD34+ marrow-derived stem cells injected intravitreally into the eye as a treatment for patients with various retinal conditions started in 2012 (U.S. National Library of Medicine, 2020f). Not results have been posted regarding the enrolled AMD patients. Future results from these studies may be extrapolated to AMD patients.
Retinal Organoids and 3D Structures Transplantation
Retinal cells’ transplantation low success rates are due to the above mentioned adverse retinal environmental conditions, but also to the complexity of the interactions between photoreceptors and other retinal cell types as well as to the structural complexity of the photoreceptors themselves (Llonch et al., 2018). Particularly critical are the interactions RPE-photoreceptors since RPE cells are the responsible for the recycling of the visual pigment, for the phagocytosis of photoreceptors’ external segment as well as for the secretion of growth factors (Strauss, 2005). Indeed, co-culture studies, attempting at de-differentiating mesenchymal stem cells, showed that RPE cells determine both, cell differentiation and connectivity of the differentiated cells (Salero et al., 2012). Unfortunately, during culture passages, RPE cells easily undergo an epithelial-mesenchymal transition (EMT), losing their characteristic cell polarity and cell-cell adhesion, necessary for the survival and functional integration of the photoreceptors (Sonoi et al., 2017). Furthermore, RPE cell cultures should be prepared exclusively with macular RPE cells because of phenotypes’ differences between cells located in the peripheral part and cells located in the posterior part -macula- of the eye (cell shape and size, proliferation capacity, maturation time, granule content, growth potential, timing for the formation of tight junctions, etc. (Burke and Hjelmeland, 2005; Haderspeck et al., 2019). Non-homogeneous cultures would lead to retinal-type tissues, with structure vulnerable to degenerative retinal diseases (Burke and Hjelmeland, 2005).
Due to the above-mentioned complications and to the biological limitations of the available cells, researchers started to build artificial retinal tissues, with structural complexity and functionality mimicking the natural ones. As a consequence, retinal organoids have been built from either ESCs or iPSCs, by differentiating retinal cells through complex experimental protocols in 3D cell cultures (Eiraku et al., 2011; Nakano et al., 2012; Llonch et al., 2018).
The main benefits of 3D cultures are a high diversity of cell types, better tissue survival, functional interaction between the different types of cells, and the achievement of retinal layers thanks to the self-organizing capabilities of the PSCs. In addition to their use for therapeutic purposes, 3D cultures represent a model of great interest for the study of retinogenesis, a non-animal model of retinal diseases and a perfect tissue for drug screening. The main limitations of these types of models are the lack of vascularization, which leads to long-term necrosis in the innermost areas of the organoid, the lack of interaction between the RPE and photoreceptors’ layer, the maturation of the different cell types which does not reach an adult state and the absence of some types of important cells, like microglia (Achberger et al., 2019). Furthermore, all organoids have a characteristic folded structure that not only makes implantation difficult but also causes greater cell death in the internal layers of this artificial tissue. The use of biomaterials could help in developing flat vascularized organoid retinas that would favor the interaction with the host tissue as well as the acceptance and survival of the transplanted cells (Singh D. et al., 2018). Advanced biomaterials could also facilitate artificial tissues manipulation and implant in a specific place in the host retina (Singh R. et al., 2018).
Tables 7, 8 resume up-to-day research in retinal organoids.
Discussion
Functional Integration of the Implanted Cells
Cell therapy, and in particular stem cell-based therapy, is gaining strength as a potential therapeutic approach for untreatable retinal degeneration diseases (Bharti, 2018; Singh et al., 2020). It consists either in the use of ocular multipotent or RPCs, administered in a non-polarized way (cell suspension), to provide non-selective neuroprotective and/or neuromodulator factors, or in the implant of layered constructs of cells aiming at replacing the damaged or degenerated retinal tissue. In both cases, therapeutic effects are conditioned by the limited cell survival within the harmful ocular environment (Singh et al., 2020).
In the majority of the preclinical trials with dissociated cells suspensions for photoreceptor regeneration, the transplanted cells fail to survive or to functionally integrate with the host tissue; despite some positive results none of the attempted approaches achieved a significant restoration of the visual function (Gamm and Wong, 2015; Canto-Soler et al., 2016; Zarbin, 2016). Furthermore, it is not known to which extent the observed improvements are due to the capability of the grafted cells of replacing the lost retinal tissue; to the secretion of protective and regenerative factors that protect the retinal neurons, or by both of them (Liu et al., 2020).
Regarding RPE, there are several investigations on the inoculation of suspensions of this type of cells. However, up-to-now evidence suggests that suspension-injected RPEs do not consistently form a monolayer and do not survive in the long term (Hu et al., 2012; Diniz et al., 2013). Since RPE cells’ therapeutic effects are conditioned by the polarization of the implanted cells as well as by their spatial arrangement in a monolayer settled in the subretinal space, there is a great challenge for injections of suspended cells (Miyagishima et al., 2016, 2017). Injected in suspension RPE cells loose most of their morphological and functional properties as proved by the low efficiency of this therapy in both, preclinical and clinical trials (Singh et al., 2020). The most promising studies focus on tissue transplantation or patches of RPE.
Promising results in both, feasibility and functionality, have been obtained in preclinical and clinical trials using functional RPE derived from both iPSCs (Bracha et al., 2017; Mandai et al., 2017b) and ESC cells (Klimanskaya et al., 2004; Bharti et al., 2006; Idelson et al., 2009; Miyagishima et al., 2016; May-Simera et al., 2018), as well as RPESC (Salero et al., 2012; Blenkinsop et al., 2013; Miyagishima et al., 2016; Mandai et al., 2017b). However, the use of iPSCs and ESC cells can lead to abnormal cell growth in the retina. Moreover, the differentiation toward a specific cell type (RPE) may not be complete or efficient, thus hindering their integration in the host retina. On their side, RPESC, although being fully committed to the RPE lineage, they are extracted from cadavers, which can lead to the retention of endophenotypes of aging and disease. Unlike cell suspensions, where the characteristics of RPE are lost, cell patches are capable of maintaining many of the typical cell functions (Miyagishima et al., 2016, 2017) which makes autologous choroid-RPE transplant surgical procedures a good candidate for AMD treatment (Joussen et al., 2006; Maaijwee K. et al., 2007; Maaijwee K.J.M. et al., 2007; Maaijwee et al., 2008).
Recent studies on subretinal injection of a suspension of photoreceptor precursors derived from mouse or human ESC/iPSC in rodent models of photoreceptor degeneration, suggested that the implanted cells are capable of getting integrated into the host retina, of achieving a morphological and functional differentiation similar to the native photoreceptors as well as of restoring some visual abilities (Lamba et al., 2009; Gonzalez-Cordero et al., 2013; Homma et al., 2013; Decembrini et al., 2014; Barnea-Cramer et al., 2016; Santos-Ferreira et al., 2016b). However, only a low proportion of transplanted cells can get integrated into the retina. What really happens is an exchange of intercellular materials between the immense majority of the native photoreceptors and the transplanted cells (Pearson et al., 2016; Santos-Ferreira et al., 2016b; Singh et al., 2016). This material transfer is bidirectional and, interestingly, it is independent of the source of implanted stem cells (Singh et al., 2016; Ortin-Martinez et al., 2017; Waldron et al., 2018). It seems that the therapeutic effects are due more to neurotrophic and neuroprotective function, rather than functional integration in the diseased retina (Liu et al., 2020). These facts raise the need to reevaluate all up-to-day photoreceptor transplant studies, characterizing the relative contribution to vision restoration based on material transfer and donor integration.
The recent development of 3D technology for the culture and differentiation of ESC/iPSC in retinal tissue has allowed several groups to test the viability of transplanting retinal sheets derived from this type of stem cell (Assawachananont et al., 2014; Shirai et al., 2016; Mandai et al., 2017a; Iraha et al., 2018). These ESC/iPSC-derived retinal sheets, derived from both mice and humans, are capable of surviving in the host retina for up to 6 months after transplantation in the subretinal space, in animal models of end-stage photoreceptor degeneration. In addition, they manage to generate all types of neural cells in the retina, i.e., photoreceptors, bipolar, amacrine, and retinal ganglion cells. In particular, the photoreceptors achieved a high degree of maturity, expressing opsins, synaptic proteins and formation of inner and outer segments (Assawachananont et al., 2014; Iraha et al., 2018). However, it has been revealed that in these grafts it is not possible to maintain an adequate laminar organization; instead, they generate a disorganized histoarchitecture (Assawachananont et al., 2014; Shirai et al., 2016; Mandai et al., 2017a; Iraha et al., 2018). 3D-culture techniques and implantation of retinal sheets suppose an expectation of providing photoreceptors in the form of tissue, providing them with an improved physical and physiological microenvironment, increasing their survival and functional integration capabilities. However, this area of cell therapy research has only just begun, so there are many emerging challenges and limitations that need to be addressed.
Regarding retinal organoids, despite the great advances achieved in this field, a number of serious limitations impede the employment of this technology for real AMD therapies: (1) lack of vascularization, which leads to long-term necrosis in the innermost areas of the organoid (2) lack of interaction between RPE and photoreceptor layer, essential for the proper functioning of the neurons (3) impossibility of the different cell types to reach an adult state, despite the complex differentiation protocols that are carried out and (4) lack of some essential cell types, such as microglia, despite presenting a high number of cell types (Achberger et al., 2019).
The lack of certain cell types during the development of the 3D culture may be due, among other causes, to the lack of sufficient physical and environmental signals, also causing high variability between the various retinal organoid models. A possible solution to some of the limitations mentioned above in relation to this type of culture could be the use of biomaterials as a support for the organoid’s growth and differentiation and their biofunctionalization to make them able to generate the adequate physical and chemical signals (molecules of the extracellular matrix) to stimulate the correct differentiation of the cells (Hynes and Lavik, 2010). Biomaterials could also allow interaction between the various layers of the retina, increasing connectivity and synaptogenesis, favoring obtaining a functional and complete retina (Singh R. et al., 2018).
Summarizing, despite the promising results obtained in several studies, long-term viability of cell implants has not yet been achieved, neither significant functional integration in the host retina nor improvement of long-term visual capabilities. Up-to-day photoreceptors integration in the host retina is clearly insufficient and/or inadequate. AMD cellular therapy has three main unanswered questions: (1) RPE plays a critical role in the maintenance and function of photoreceptors but very few studies consider the interdependence between RPE cells and photoreceptors (Bhutto and Lutty, 2012; Handa, 2012; Handa et al., 2017) which could be one of the reasons long-term maintenance of RPE and photoreceptor transplantation has not yet been achieved. In this case, a possible solution would be the joint transplantation of the two sheets of cell, either sequentially or at the same time. (2) The mechanisms involved in the development and integration of photoreceptors in the retinal circuit remain unknown (Gamm and Wong, 2015). It has been observed expression of specific synaptic proteins in the terminal bar of the implanted photoreceptors; it has also been observed expression of postsynaptic proteins of the host bipolar cells in contact with the donor cells; and it has been observed a certain improvement of visual function. However, evaluation of the neural function of these transplants are very few and clearly insufficient (Singh et al., 2013). (3) Detailed mechanisms for material exchange between donor and host cells are almost unknown (Lluis and Cosma, 2010; Sanges et al., 2016). Most of the cells identified as integrated donor photoreceptors in the most recent studies could actually be host photoreceptors with exchanged material (Pearson et al., 2016; Santos-Ferreira et al., 2016b; Singh et al., 2016; Ortin-Martinez et al., 2017; Waldron et al., 2018).
Addressing these problems could allow us to identify those factors that could be used to promote cellular viability, synaptogenesis and integration of the donor cells to the host tissue. Further questions regard the safety of the transplants (depending on their origin), possibility of transferring of the pathology to the implanted healthy cells, etc. It should be noticed that the most promising results have been obtained in tissue transplantation of retinal sheets and RPE patches.
Maintenance of the Immunoprivileged State of the Eye
Several subfoveal RPE implantation techniques have been employed in AMD patients. Most often, excision at sub-macular level is necessary for transplantation of the monolayer of fetal or adult RPE. This procedure can lead to acute tissue inflammation and a consequent eye damage that can impair the visual route, or even a higher immune reaction if the implant comes from another organism. Therefore, knowledge of the immunosuppression mechanisms is necessary to prevent rejection of RPE layers (Tezel et al., 2007), and it has been postulated that the low success rate of RPE monolayers transplants is due to alterations of the immunoprivileged state of the eye (indeed, rejection rate of retina transplants is higher than of other tissues, such as heart or skin (Streilein, 2003; Niederkorn and Kaplan, 2007; Sandhu et al., 2019)). Clinical studies of RPE transplants in patients undergoing immunosuppressive therapy, suggest that the immune system could destroy the transplanted cells without involving any inflammatory process (Schwartz et al., 2015). On the other hand, a higher survival of photoreceptor transplants has been observed in preclinical studies when some type of immune suppression was used after the surgery (Sandhu et al., 2019). Combined RPE-photoreceptors transplants showed that the use of immunosuppressants could improve the cellular integrity of the graft (Radtke et al., 2002, 2008). However, these results have not yet been replicated. In other preclinical studies, it was observed that only 0.5% of photoreceptors got integrated into the retina (Pearson et al., 2016; Santos-Ferreira et al., 2016a). It is not clear if the effects of photoreceptors transplantation in mice are the result of cell integration and synapse formation, rather than cytoplasmic exchange between donor and host cells. However, we can conclude that the use of immunosuppressants in human retinal transplants may lead to a higher success rate.
Biomaterials and Improvement of Cell Survival
2D – 3D scaffolding approaches have been shown to be efficient for overcoming limitations like low cell survival, lack of cell integration at the transplant site or keeping the injected cells in the target area (Behtaj et al., 2020). However, it is important to mention that the majority of the biomaterials developed for retinal regeneration has not been tested in vivo.
Biodegradable polymer scaffolds for the transplantation of retinal stem cells increase survival cell rate (Schubert et al., 2014). On the other hand, the dimensional conformation or porosity of the scaffold seems to promote the union and subsequent differentiation and orientation of the RPCs (Lee Ventola, 2014). Other studies defend the idea that scaffold topography influences orientation, because of its positive influence on differentiation, morphology, proliferation, migration and adhesion of the cells (Ramakrishna et al., 2001; Kador et al., 2016; Yang et al., 2017).
Scaffolds can actively interact with different cellular components. Furthermore, biomaterials can include biological cues (e.g., cellular ligands) to promote cell adhesion or physical clues (e.g., scaffold topography) to favor cell alignment and morphology. Scaffolds can also serve as supplier or repository for excitatory growth signals to accelerate tissue regeneration (Chan and Leong, 2008). Biomaterials with a sophisticated chemical and morphological structure to allow the survival, proliferation and differentiation of RPE cells and the consequent successful transplantation into the degenerated retina have also been pursued (Chichagova et al., 2018). For the encapsulation of iPSC and hESC, optimal and standardized culture media and good bioengineering processes are needed to achieve the desired expansion and differentiation, processes that are currently under investigation (Bracha et al., 2017; Mandai et al., 2017b; U.S. National Library of Medicine, 2020c).
Biomaterials should be biocompatible, biologically inert and sterilizable, not triggering cytotoxic processes neither inflammatory responses while promoting the proliferation, survival and migration of the implanted cells. Significant challenges are the risk of immune reactions at the implant site as well as the long-term survival and functionality of the transplanted cells.
A very promising biomaterial is silkworm silk fibroin, because it fulfills all the above requirements. The encapsulation of mesenchymal stem cells in silk fibroin hydrogels promotes a substantial release of anti-inflammatory and anti-oxidant molecules with marked neuroregenerative and neuroprotective properties that could be used in AMD treatment (Martín-Martín et al., 2019; Jemni Damer et al., 2020).
Current research of cell and tissue engineering approaches focuses on the preservation of photoreceptors by replacing the damaged retinal pigment epithelium since this technique has been shown to slow the degeneration of photoreceptors and even restore partial visual functions (Aboutaleb Kadkhodaeian et al., 2019; Jin et al., 2019; McGill et al., 2019; Zarbin, 2019). The development of physical substrates for artificial RPE sheets is the main objective for biomaterials engineers. It is worth noting that actual focusing on photoreceptor’s preservation is partly due to the current difficulties to maintain functional photoreceptors alive before they could get transplanted, as well as to integrate the transplanted photoreceptors to the neural retina and functionally replace those already lost. In culture, photoreceptors can be maintained alive for 24–48 h, depending on the age of the donor (Fontaine et al., 1998) and less than 20% survive after transplantation (Sortwell et al., 2000). It is unclear how many of these alive cells are functional photoreceptors. State-of-the-art 3D scaffolds have been used to build a polarized photoreceptor monolayer capable to can get integrated into the neural retina, but also to facilitate the differentiation of progenitors of photoreceptor (Jung et al., 2018).
Good results on biomaterials and RPE cells have been obtained from both, in vitro and in vivo studies (Warnke et al., 2013; McHugh et al., 2014; Stanzel et al., 2014; Ilmarinen et al., 2015; Peng et al., 2016; Calejo et al., 2017; Jemni Damer et al., 2020) although how long the transplanted cells could survive has still to be determined. The scaffolds on which they are placed may be designed to act either as supporting devices or as artificial BrMs, showing the latter the best viability of RPE cells. In clinical trials, RPE cells have been injected in suspension, as a monolayer and also on biomaterials (Schwartz et al., 2015; da Cruz et al., 2018; U.S. National Library of Medicine, 2020s). Unfortunately, these clinical trials are very few and are in very early stages of development, so their results should be analyzed accordingly.
In conclusion, the use of biomaterials to generate implantable biohybrid tissue may be a potential solution to the main problems of cell transplantation, increasing the survival of implanted cells and improving their integration capacity.
Conclusion
Cell replacement, either by using cell suspensions or by integrating cell’s sheets on a scaffolding material to promote the survival of the transplanted cells, is a promising treatment for advanced AMD stages. Pioneering studies have provided a proof-of-concept for RPE and photoreceptors transplantation as a plausible therapeutic strategy.
The effective integration of the transplanted cells into the damaged retina is the principal limitation of cell therapy. The development of advanced biomaterials to support the in vitro development of artificial 3D retinal tissues, as well as the implantation and the functional integration of these constructs into the damaged retina represents the most promising therapeutic approach. Indeed, there is evidence that retinal cells on supporting biomaterials, implanted in the posterior segment of the eye, achieve a better but limited integration than injections of free cells in the same region, and slightly improve vision capabilities in different experimental models.
To obtain clinically relevant therapeutic solutions, a good effectiveness of the proposed treatment must be achieved. And the key factor to increase the effectiveness of retinal implant therapies is the accomplishment of a substantial integration of the implanted cells. In this aspect an immense amount of work is still needed: the discovery of the exchange of genetic material between native and implanted photoreceptors suggests that the integration of these neurons to the host retina, reported in up-to-date studies, is probably not real, putting on the table the need to verify and reevaluate these studies. It is still unknown whether the therapeutic effect of cell transplants is due to an improvement in functionality by integration or due to production of protective factors. The elucidation of the involved mechanisms is essential for the accomplishment of this goal. Furthermore, functionality analysis of the integrated implants has to be performed.
Author Contributions
NJ-D and AG-D: bibliographic research and information synthesis, AMD course, wrote the manuscript, and equal contribution. MF-A, NS-B, and NA-L: bibliographic research and information synthesis. FA-M: clinical aspects. GG, JP-R, FR, and DK: biomaterials aspects. DG-N: biomaterials and cell and tissue therapy. GG, JP-R, DK, DG-N: manuscript revision. FP: manuscript design, information synthesis, supervision, and wrote the manuscript. All authors contributed to the article and approved the submitted version.
Funding
The authors gratefully acknowledge financial support received from the Spanish Ministerio de Economía y Competitividad through grants MAT2016-76847-R, MAT2016-79832-R, and MAT2015-66666-C3-3-R; from the Comunidad de Madrid, Spain through grants Neurocentro-B2017/BrMD-3760 and IND2018/BrMD-9804, and from the National Institutes of Health (P41EB002520); predoctoral FPI grant from the Spanish Ministerio de Economía y Competitividad (AGD) and research contract from the Comunidad de Madrid, Spain (MFA).
Conflict of Interest
The authors declare that the research was conducted in the absence of any commercial or financial relationships that could be construed as a potential conflict of interest.
References
Aboutaleb Kadkhodaeian, H., Tiraihi, T., Ahmadieh, H., Ziaei, H., Daftarian, N., and Taheri, T. (2019). Generation of retinal pigmented epithelium-like cells from pigmented spheres differentiated from bone marrow stromal cell-derived neurospheres. Tissue Eng. Regen. Med. 16, 253–263. doi: 10.1007/s13770-019-00183-1
Achberger, K., Probst, C., Haderspeck, J. C., Bolz, S., Rogal, J., Chuchuy, J., et al. (2019). Merging organoid and organ-on-a-chip technology to generate complex multi-layer tissue models in a human retina-on-a-chip platform. Elife 8:e46188. doi: 10.7554/eLife.46188
Aguayo, A. J., Rasminsky, M., Bray, G. M., Carbonetto, S., and Mckerracher, L. (1991). Degenerative and regenerative responses of injured neurons in the central nervous system of adult mammals. Philos. Trans. R Soc. Lond. B Biol. Sci. 331, 337–343. doi: 10.1098/rstb.1991.0025
Ambati, J., and Fowler, B. J. (2012). Mechanisms of age-related macular degeneration. Neuron 75, 26–39. doi: 10.1016/j.neuron.2012.06.018
Anderson, D. H., Radeke, M. J., Gallo, N. B., Chapin, E. A., Johnson, P. T., Curletti, C. R., et al. (2010). The pivotal role of the complement system in aging and age-related macular degeneration: hypothesis re-visited. Prog. Retin. Eye Res. 29, 95–112. doi: 10.1016/j.preteyeres.2009.11.003
Aoki, H., Hara, A., Niwa, M., Yamada, Y., and Kunisada, T. (2009). In vitro and in vivo differentiation of human embryonic stem cells into retina-like organs and comparison with that from mouse pluripotent epiblast stem cells. Dev. Dyn. 238, 2266–2279. doi: 10.1002/dvdy.22008
Arnhold, S., Klein, H., Semkova, I., Addicks, K., and Schraermeyer, U. (2004). Neurally selected embryonic stem cells induce tumor formation after long-term survival following engraftment into the subretinal space. Invest. Opthalmol. Vis. Sci. 45, 4251–4255. doi: 10.1167/iovs.03-1108
Assawachananont, J., Mandai, M., Okamoto, S., Yamada, C., Eiraku, M., Yonemura, S., et al. (2014). Transplantation of embryonic and induced pluripotent stem cell-derived 3D retinal sheets into retinal degenerative mice. Stem Cell Rep. 2, 662–674. doi: 10.1016/j.stemcr.2014.03.011
Augustin, C., Augustin, A., Tetz, M., and Rizzo, S. (2012). Suprachoroidal drug delivery – a new approach for the treatment of severe macular diseases. Eur. Ophthalmic Rev. 06:25. doi: 10.17925/EOR.2012.06.01.25
Ballios, B. G., Cooke, M. J., van der Kooy, D., and Shoichet, M. S. (2010). A hydrogel-based stem cell delivery system to treat retinal degenerative diseases. Biomaterials 31, 2555–2564. doi: 10.1016/j.biomaterials.2009.12.004
Ballios, B. G., and van der Kooy, D. (2010). Biology and therapeutic potential of adult retinal stem cells. Can. J. Ophthalmol. 45, 342–351. doi: 10.3129/i10-070
Bansal, P., Garg, S., Sharma, Y., and Venkatesh, P. (2016). Posterior segment drug delivery devices: current and novel therapies in development. J. Ocul. Pharmacol. Ther. 32, 135–144. doi: 10.1089/jop.2015.0133
Baranov, P., Michaelson, A., Kundu, J., Carrier, R. L., and Young, M. (2014). Interphotoreceptor matrix-poly(ϵ-caprolactone) composite scaffolds for human photoreceptor differentiation. J. Tissue Eng. 5:2041731414554139. doi: 10.1177/2041731414554139
Barar, J., Aghanejad, A., Fathi, M., and Omidi, Y. (2016). Advanced drug delivery and targeting technologies for the ocular diseases. BioImpacts 6, 49–67. doi: 10.15171/bi.2016.07
Barber, A. C., Hippert, C., Duran, Y., West, E. L., Bainbridge, J. W. B., Warre-Cornish, K., et al. (2013). Repair of the degenerate retina by photoreceptor transplantation. Proc. Natl. Acad. Sci. U.S.A. 110, 354–359. doi: 10.1073/pnas.1212677110
Barnea-Cramer, A. O., Wang, W., Lu, S. J., Singh, M. S., Luo, C., Huo, H., et al. (2016). Function of human pluripotent stem cell-derived photoreceptor progenitors in blind mice. Sci. Rep. 6:29784. doi: 10.1038/srep29784
Battler, A., and Leor, J. (2006). Stem Cell and Gene-Based Therapy: Frontiers in Regenerative Medicine. Berlin: Springer. doi: 10.1007/1-84628-142-3
Behtaj, S., Öchsner, A., Anissimov, Y. G., and Rybachuk, M. (2020). Retinal tissue bioengineering, materials and methods for the treatment of glaucoma. Tissue Eng. Regen. Med. 17, 253–269. doi: 10.1007/s13770-020-00254-8
Bennett, C. R., Bex, P. J., Bauer, C. M., and Merabet, L. B. (2019). The assessment of visual function and functional vision. Semin. Pediatr. Neurol. 31, 30–40. doi: 10.1016/j.spen.2019.05.006
Bharti, K. (2018). Patching the retina with stem cells. Nat. Biotechnol. 36, 311–313. doi: 10.1038/nbt.4118
Bharti, K., Nguyen, M. T. T., Skuntz, S., Bertuzzi, S., and Arnheiter, H. (2006). The other pigment cell: specification and development of the pigmented epithelium of the vertebrate eye. Pigment Cell Res. 19, 380–394. doi: 10.1111/j.1600-0749.2006.00318.x
Bharti, K., Rao, M., Hull, S. C., Stroncek, D., Brooks, B. P., Feigal, E., et al. (2014). Developing cellular therapies for retinal degenerative diseases. Invest. Ophthalmol. Vis. Sci. 55, 1191–1201. doi: 10.1167/iovs.13-13481
Bhatt, N. S., Newsome, D. A., Fenech, T., Hessburg, T. P., Diamond, J. G., Miceli, M. V., et al. (1994). Experimental transplantation of human retinal pigment epithelial cells on collagen substrates. Am. J. Ophthalmol. 117, 214–221. doi: 10.1016/S0002-9394(14)73079-X
Bhatt, P., Narvekar, P., Lalani, R., Chougule, M. B., Pathak, Y., and Sutariya, V. (2019). An in vitro assessment of thermo-reversible gel formulation containing sunitinib nanoparticles for neovascular age-related macular degeneration. AAPS PharmSciTech 20:280. doi: 10.1208/s12249-019-1474-0
Bhattacharya, S., Gangaraju, R., and Chaum, E. (2017). Recent advances in retinal stem cell therapy. Curr. Mol. Biol. Rep. 3, 172–182. doi: 10.1007/s40610-017-0069-3
Bhutto, I., and Lutty, G. (2012). Understanding age-related macular degeneration (AMD): relationships between the photoreceptor/retinal pigment epithelium/Bruch’s membrane/choriocapillaris complex. Mol. Aspects Med. 33, 295–317. doi: 10.1016/j.mam.2012.04.005
Birch, D. G., Weleber, R. G., Duncan, J. L., Jaffe, G. J., Tao, W., and Ciliary Neurotrophic Factor Retinitis Pigmentosa Study Groups (2013). Randomized trial of ciliary neurotrophic factor delivered by encapsulated cell intraocular implants for retinitis pigmentosa. Am. J. Ophthalmol. 156, 283.e1–292.e1. doi: 10.1016/j.ajo.2013.03.021
Bisht, R., Jaiswal, J. K., and Rupenthal, I. D. (2017). Nanoparticle-loaded biodegradable light-responsive in situ forming injectable implants for effective peptide delivery to the posterior segment of the eye. Med. Hypotheses 103, 5–9. doi: 10.1016/j.mehy.2017.03.033
Blenkinsop, T. A., Salero, E., Stern, J. H., and Temple, S. (2013). The culture and maintenance of functional retinal pigment epithelial monolayers from adult human eye. Methods Mol. Biol. 945, 45–65. doi: 10.1007/978-1-62703-125-7_4
Booij, J. C., Baas, D. C., Beisekeeva, J., Gorgels, T. G. M. F., and Bergen, A. A. B. (2010). The dynamic nature of Bruch’s membrane. Prog. Retin. Eye Res. 29, 1–18. doi: 10.1016/j.preteyeres.2009.08.003
Bracha, P., Moore, N. A., and Ciulla, T. A. (2017). Induced pluripotent stem cell-based therapy for age-related macular degeneration. Expert Opin. Biol. Ther. 17, 1113–1126. doi: 10.1080/14712598.2017.1346079
Bray, G. M., Villegas-Pérez, M. P., Vidal-Sanz, M., and Aguayo, A. J. (1987). The use of peripheral nerve grafts to enhance neuronal survival, promote growth and permit terminal reconnections in the central nervous system of adult rats. J. Exp. Biol. 132, 5–19.
Burke, J. M., and Hjelmeland, L. M. (2005). Mosaicism of the retinal pigment epithelium: seeing the small picture. Mol. Interv. 5, 241–249. doi: 10.1124/mi.5.4.7
Bush, R. A., Lei, B., Tao, W., Raz, D., Chan, C.-C., Cox, T. A., et al. (2004). Encapsulated cell-based intraocular delivery of ciliary neurotrophic factor in normal rabbit: dose-dependent effects on ERG and retinal histology. Invest. Ophthalmol. Vis. Sci. 45, 2420–2430. doi: 10.1167/iovs.03-1342
Caffé, A. R., Ahuja, P., Holmqvist, B., Azadi, S., Forsell, J., Holmqvist, I., et al. (2002). Mouse retina explants after long-term culture in serum free medium. J. Chem. Neuroanat. 22, 263–273. doi: 10.1016/S0891-0618(01)00140-5
Calejo, M. T., Ilmarinen, T., Vuorimaa-Laukkanen, E., Talvitie, E., Hakola, H. M., Skottman, H., et al. (2017). Langmuir-Schaefer film deposition onto honeycomb porous films for retinal tissue engineering. Acta Biomater. 54, 138–149. doi: 10.1016/j.actbio.2017.02.035
Campbell, M., and Humphries, P. (2013). The blood-retina barrier tight junctions and barrier modulation introduction: general anatomy of the human eye. Adv. Exp. Med. Biol. 763, 70–84. doi: 10.1007/978-1-4614-4711-5_3
Canto-Soler, V., Flores-Bellver, M., and Vergara, M. N. (2016). Stem cell sources and their potential for the treatment of retinal degenerations. Invest. Ophthalmol. Vis. Sci. 57, ORSFd1–ORSFd9. doi: 10.1167/iovs.16-19127
Carr, A. F., Smart, M. J. K., Ramsden, C. M., Powner, M. B., Cruz, L., and Coffey, P. J. (2013). Development of human embryonic stem cell therapies for age-related macular degeneration. Trends Neurosci. 36, 385–395. doi: 10.1016/j.tins.2013.03.006
Carr, A.-J., Vugler, A. A., Hikita, S. T., Lawrence, J. M., Gias, C., Chen, L. L., et al. (2009). Protective effects of human ips-derived retinal pigment epithelium cell transplantation in the retinal dystrophic rat. PLoS One 4:e8152. doi: 10.1371/journal.pone.0008152
Chan, B. P., and Leong, K. W. (2008). Scaffolding in tissue engineering: general approaches and tissue-specific considerations. Eur. Spine J. 4, (Suppl. 4), 467–479. doi: 10.1007/s00586-008-0745-3
Chen, H. Y., Kaya, K. D., Dong, L., and Swaroop, A. (2016). Three-dimensional retinal organoids from mouse pluripotent stem cells mimic in vivo development with enhanced stratification and rod photoreceptor differentiation. Mol. Vis. 22, 1077–1094.
Chen, M., Le, D. Q. S., Baatrup, A., Nygaard, J. V., Hein, S., Bjerre, L., et al. (2011). Self-assembled composite matrix in a hierarchical 3-D scaffold for bone tissue engineering. Acta Biomater. 7, 2244–2255. doi: 10.1016/j.actbio.2010.12.031
Chichagova, V., Hallam, D., Collin, J., Zerti, D., Dorgau, B., Felemban, M., et al. (2018). Cellular regeneration strategies for macular degeneration: past, present and future. Eye 32, 946–971. doi: 10.1038/s41433-018-0061-z
Chirila, T., Barnard, Z., Zainuddin, Harkin, D. G., Schwab, I. R., and Hirst, L. (2008). Bombyx mori silk fibroin membranes as potential substrata for epithelial constructs used in the management of ocular surface disorders. Tissue Eng. A 14, 1203–1211. doi: 10.1089/ten.tea.2007.0224
Chirila, T. V., Shadforth, A. M. A., Richardson, N. A., Suzuki, S., Harkin, D. G., and Theodoropoulos, C. (2015). A Bruch’s membrane substitute fabricated from silk fibroin supports the function of retinal pigment epithelial cells in vitro. J. Tissue Eng. Regen. Med. 11, 1915–1924. doi: 10.1002/term.2089
Christiansen, A. T., Tao, S. L., Smith, M., Wnek, G. E., Prause, J. U., Young, M. J., et al. (2012). Subretinal implantation of electrospun, short nanowire, and smooth poly(ε-caprolactone) Scaffolds to the Subretinal Space of Porcine Eyes. Stem Cells International. 2012:454295. doi: 10.1155/2012/454295
Clegg, D. O., Hinton, D. R., Falabella, P., Rowland, T., Kashani, A. H., Humayun, M. S., et al. (2015). Stem cell based therapies for age-related macular degeneration: the promises and the challenges. Prog. Retin. Eye Res. 48, 1–39. doi: 10.1016/j.preteyeres.2015.06.004
Cotrim, C. C., Toscano, L., Messias, A., Jorge, R., and Siqueira, R. C. (2017). Intravitreal use of bone marrow mononuclear fraction containing CD34+ stem cells in patients with atrophic age-related macular degeneration. Clin. Ophthalmol. 11, 931–938. doi: 10.2147/OPTH.S133502
Crafoord, S., Geng, L., Seregard, S., and Algvere, P. V. (2002). Photoreceptor Survival in Transplantation of Autologous Iris Pigment Epithelial Cells to the Subretinal Space. Available online at: https://onlinelibrary.wiley.com/doi/pdf/10.1034/j.1600-0420.2002.800408.x (accessed May 19, 2019).
Cunha-Vaz, J., Bernardes, R., and Lobo, C. (2011). Blood-retinal barrier. Eur. J. Ophthalmol. 21, 3–9. doi: 10.5301/EJO.2010.6049
Cunha-Vaz, J. G. (1997). The blood-ocular barriers: past, present, and future. Doc. Ophthalmol. 93, 149–157. doi: 10.1007/BF02569055
Curatola, A. M., Moscatelli, D., Norris, A., and Hendricks-Munoz, K. (2005). Retinal blood vessels develop in response to local VEGF-A signals in the absence of blood flow. Exp. Eye Res. 81, 147–158. doi: 10.1016/j.exer.2005.06.001
da Cruz, L., Fynes, K., Georgiadis, O., Kerby, J., Luo, Y. H., Ahmado, A., et al. (2018). Phase 1 clinical study of an embryonic stem cell–derived retinal pigment epithelium patch in age-related macular degeneration. Nat. Biotechnol. 36, 328–337. doi: 10.1038/nbt.4114
Das, T., del Cerro, M., Jalali, S., Rao, V. S., Gullapalli, V. K., Little, C., et al. (1999). The transplantation of human fetal neuroretinal cells in advanced retinitis pigmentosa patients: results of a long-term safety study. Exp. Neurol. 157, 58–68. doi: 10.1006/exnr.1998.6992
Davis, R. J., Alam, N. M., Zhao, C., Müller, C., Saini, J. S., Blenkinsop, T. A., et al. (2017). The developmental stage of adult human stem cell-derived retinal pigment epithelium cells influences transplant efficacy for vision rescue. Stem Cell Rep. 9, 42–49. doi: 10.1016/j.stemcr.2017.05.016
Decembrini, S., Koch, U., Radtke, F., Moulin, A., and Arsenijevic, Y. (2014). Derivation of traceable and transplantable photoreceptors from mouse embryonic stem cells. Stem Cell Rep. 2, 853–865. doi: 10.1016/j.stemcr.2014.04.010
Del Priore, L. V., Tezel, T. H., and Kaplan, H. J. (2004). Survival of allogeneic porcine retinal pigment epithelial sheets after subretinal transplantation. Invest. Opthalmol. Vis. Sci. 45, 985–992. doi: 10.1167/iovs.03-0662
Diniz, B., Thomas, P., Thomas, B., Ribeiro, R., Hu, Y., Brant, R., et al. (2013). Subretinal implantation of retinal pigment epithelial cells derived from human embryonic stem cells: improved survival when implanted as a monolayer. Invest. Opthalmol. Vis. Sci. 54, 5087–5096. doi: 10.1167/iovs.12-11239
Eberle, D., Kurth, T., Santos-Ferreira, T., Wilson, J., Corbeil, D., and Ader, M. (2012). Outer segment formation of transplanted photoreceptor precursor cells. PLoS One 7:e46305. doi: 10.1371/journal.pone.0046305
Eberle, D., Santos-Ferreira, T., Grahl, S., and Ader, M. (2014). Subretinal transplantation of MACS purified photoreceptor precursor cells into the adult mouse retina. J. Vis. Exp. 84:e50932. doi: 10.3791/50932
Eiraku, M., and Sasai, Y. (2012). Mouse embryonic stem cell culture for generation of three-dimensional retinal and cortical tissues. Nat. Protoc. 7, 69–79. doi: 10.1038/nprot.2011.429
Eiraku, M., Takata, N., Ishibashi, H., Kawada, M., Sakakura, E., Okuda, S., et al. (2011). Self-organizing optic-cup morphogenesis in three-dimensional culture. Nature 472, 51–56. doi: 10.1038/nature09941
Emerich, D. F., and Thanos, C. G. (2008). NT-501: an ophthalmic implant of polymer-encapsulated ciliary neurotrophic factor-producing cells. Curr. Opin. Mol. Ther. 10, 506–515.
Engelsberg, K., Ehinger, B., and Ghosh, F. (2008). Early development of retinal subtypes in long-term cultures of human embryonic retina. Curr. Eye Res. 33, 185–191. doi: 10.1080/02713680701843784
Engelsberg, K., Johansson, K., and Ghosh, F. (2005). Development of the embryonic porcine neuroretina in vitro. Ophthalmic Res. 37, 104–111. doi: 10.1159/000084252
Falkner-Radler, C. I., Krebs, I., Glittenberg, C., Povazay, B., Drexler, W., Graf, A., et al. (2011). Human retinal pigment epithelium (RPE) transplantation: outcome after autologous RPE-choroid sheet and RPE cell-suspension in a randomised clinical study. Br J. Ophthalmol. 95, 370–375. doi: 10.1136/bjo.2009.176305
Fernández-García, L., Pérez-Rigueiro, J., Martinez-Murillo, R., Panetsos, F., Ramos, M., Guinea, G. V., et al. (2018). Cortical reshaping and functional recovery induced by silk fibroin hydrogels-encapsulated stem cells implanted in stroke animals. Front. Cell. Neurosci. 12:296. doi: 10.3389/fncel.2018.00296
Fields, M., Cai, H., Gong, J., and Del Priore, L. (2016). Potential of induced pluripotent stem cells (iPSCs) for treating age-related macular degeneration (AMD). Cells 5:44. doi: 10.3390/cells5040044
Fligor, C. M., Langer, K. B., Sridhar, A., Ren, Y., Shields, P. K., Edler, M. C., et al. (2018). Three-dimensional retinal organoids facilitate the investigation of retinal ganglion cell development, organization and neurite outgrowth from human pluripotent stem cells. Sci. Rep. 8:14520. doi: 10.1038/s41598-018-32871-8
Fontaine, V., Kinkl, N., Sahel, J., Dreyfus, H., and Hicks, D. (1998). Survival of purified rat photoreceptors in vitro is stimulated directly by fibroblast growth factor-2. J. Neurosci. 18, 9662–9672. doi: 10.1523/JNEUROSCI.18-23-09662.1998
Forrester, J. V., and Xu, H. (2012). Good news-bad news: the yin and yang of immune privilege in the eye. Front. Immunol. 3:338. doi: 10.3389/fimmu.2012.00338
Gagliardi, G., Ben, M., Barek, K., Chaffiol, A., Slembrouck-Brec, A., Conart, J. B., et al. (2018). Characterization and transplantation of CD73-positive photoreceptors isolated from human iPSC-derived retinal organoids. Stem Cell Rep. 11, 665–680. doi: 10.1016/j.stemcr.2018.07.005
Gaillard, F., and Sauvé, Y. (2007). Cell-based therapy for retina degeneration: the promise of a cure. Vis. Res. 47, 2815–2824. doi: 10.1016/j.visres.2007.06.018
Gamm, D. M., and Wong, R. (2015). Report on the national eye institute audacious goals initiative: photoreceptor regeneration and integration workshop. Transl. Vis. Sci. Technol. 4:2. doi: 10.1167/tvst.4.6.2
Garita-Hernandez, M., Lampič, M., Chaffiol, A., Guibbal, L., Routet, F., Santos-Ferreira, T., et al. (2019). Restoration of visual function by transplantation of optogenetically engineered photoreceptors. Nat. Commun. 10:4524. doi: 10.1038/s41467-019-12330-2
Gehrs, K. M., Anderson, D. H., Johnson, L. V., and Hageman, G. S. (2006). Age-related macular degeneration – Emerging pathogenetic and therapeutic concepts. Ann. Med. 38, 450–471. doi: 10.1080/07853890600946724
Ghosh, F., Arnér, K., and Engelsberg, K. (2009). Isolation of photoreceptors in the cultured full-thickness fetal rat retina. Invest. Ophthalmol. Vis. Sci. 50, 826–835. doi: 10.1167/iovs.08-2389
Giordano, G. G., Thomson, R. C., Ishaug, S. L., Mikos, A. G., Cumber, S., Garcia, C. A., et al. (1997). Retinal pigment epithelium cells cultured on synthetic biodegradable polymers. J. Biomed. Mater. Res. 34, 87–93. doi: 10.1002/(SICI)1097-4636(199701)34:1<87::AID-JBM12>3.0.CO;2-M
Gonzalez-Cordero, A., West, E. L., Pearson, R. A., Duran, Y., Carvalho, L. S., Chu, C. J., et al. (2013). Photoreceptor precursors derived from three-dimensional embryonic stem cell cultures integrate and mature within adult degenerate retina. Nat. Biotechnol. 31, 741–747. doi: 10.1038/nbt.2643
González-Nieto, D., Fernández-García, L., Pérez-Rigueiro, J., Guinea, G. V., and Panetsos, F. (2018). Hydrogels-assisted cell engraftment for repairing the stroke-damaged brain: chimera or reality. Polymers 10:184. doi: 10.3390/polym10020184
Goslings, W. R., Prodeus, A. P., Streilein, J. W., Carroll, M. C., Jager, M. J., and Taylor, A. W. (1998). A small molecular weight factor in aqueous humor acts on C1q to prevent antibody-dependent complement activation. Invest Ophthalmol Vis Sci. 39, 989–995.
Guenther, E., Tröger, B., Schlosshauer, B., and Zrenner, E. (1999). Long-term survival of retinal cell cultures on retinal implant materials. Vis. Res. 39, 3988–3994. doi: 10.1016/S0042-6989(99)00128-5
Guerrero-Naranjo, J. L., Quiroz-Mercado, H., Sanchez-Bermudez, G., Schoonewolff, F., Salinas Longoria, S., Romero Vera, R., et al. (2013). Safety of implantation of the NT-503 device in patients with choroidal neovasculariza- tion secondary to age-related macular degeneration. Assoc. Res. Vis. Ophthalmol. 54:3298.
Gust, J., and Reh, T. A. (2011). Adult donor rod photoreceptors integrate into the mature mouse retina. Invest. Ophthalmol. Vis. Sci. 52, 5266–5272. doi: 10.1167/iovs.10-6329
Haderspeck, J. C., Chuchuy, J., Kustermann, S., Liebau, S., and Loskill, P. (2019). Organ-on-a-chip technologies that can transform ophthalmic drug discovery and disease modeling. Exp. Opin. Drug Discov. 14, 47–57. doi: 10.1080/17460441.2019.1551873
Hadlock, T., Singh, S., Vacanti, J. P., and McLaughlin, B. J. (1999). Ocular cell monolayers cultured on biodegradable substrates. Tissue Eng. 5, 187–196. doi: 10.1089/ten.1999.5.187
Handa, J. T. (2012). How does the macula protect itself from oxidative stress? Mol. Aspects Med. 33, 418–435. doi: 10.1016/j.mam.2012.03.006
Handa, J. T., Cano, M., Wang, L., Datta, S., and Liu, T. (2017). Lipids, oxidized lipids, oxidation-specific epitopes, and Age-related Macular Degeneration. Biochim. Biophys. Acta 1862, 430–440. doi: 10.1016/j.bbalip.2016.07.013
Harris, T. I., Paterson, C. A., Farjood, F., Wadsworth, I. D., Caldwell, L., Lewis, R. V., et al. (2019). Utilizing recombinant spider silk proteins to develop a synthetic bruch’s membrane for modeling the retinal pigment epithelium. ACS Biomater. Sci. Eng. 5, 4023–4036. doi: 10.1021/acsbiomaterials.9b00183
Hasegawa, Y., Takata, N., Okuda, S., Kawada, M., Eiraku, M., and Sasai, Y. (2016). Emergence of dorsal-ventral polarity in ESC-derived retinal tissue. Development 143, 3895–3906. doi: 10.1242/dev.134601
Hertz, J., Qu, B., Hu, Y., Patel, R. D., Valenzuela, D. A., and Goldberg, J. L. (2014). Survival and integration of developing and progenitor-derived retinal ganglion cells following transplantation. Cell Transplant. 23, 855–872. doi: 10.3727/096368913X667024
Hiler, D., Chen, X., Hazen, J., Kupriyanov, S., Carroll, P. A., Qu, C., et al. (2015). Quantification of retinogenesis in 3d cultures reveals epigenetic memory and higher efficiency in iPSCs derived from rod photoreceptors. Cell Stem Cell 17, 101–115. doi: 10.1016/j.stem.2015.05.015
Ho, A. C., Chang, T. S., Samuel, M., Williamson, P., Willenbucher, R. F., and Malone, T. (2017). Experience with a subretinal cell-based therapy in patients with geographic atrophy secondary to age-related macular degeneration. Am. J. Ophthalmol. 179, 67–80. doi: 10.1016/j.ajo.2017.04.006
Hogan, M. J., Alvarado, J. A., and Weddell, J. E. (1971). Histology of the Human Eye; An Atlas and Textbook. Philadelphia, PA: Saunders.
Homma, K., Okamoto, S., Mandai, M., Gotoh, N., Rajasimha, H. K., Chang, Y. S., et al. (2013). Developing rods transplanted into the degenerating retina of Crx-knockout mice exhibit neural activity similar to native photoreceptors. Stem Cells 31, 1149–1159. doi: 10.1002/stem.1372
Hoon, M., Okawa, H., Della Santina, L., and Wong, R. O. L. (2014). Functional architecture of the retina: development and disease. Prog. Retin. Eye Res. 42, 44–84. doi: 10.1016/j.preteyeres.2014.06.003
Hsiung, J., Zhu, D., and Hinton, D. R. (2015). Polarized human embryonic stem cell-derived retinal pigment epithelial cell monolayers have higher resistance to oxidative stress-induced cell death than nonpolarized cultures. Stem Cells Transl. Med. 4, 10–20. doi: 10.5966/sctm.2014-0205
Hu, Y., Liu, L., Lu, B., Zhu, D., Ribeiro, R., Diniz, B., et al. (2012). A novel approach for subretinal implantation of ultrathin substrates containing stem cell-derived retinal pigment epithelium monolayer. Ophthalmic Res. 48, 186–191. doi: 10.1159/000338749
Huang, B. O., Mitchell, C. K., and Redburn-Johnson, D. A. (2000). GABA and GABA a receptor antagonists alter developing cone photoreceptor development in neonatal rabbit retina. Vis. Neurosci. 17, 925–935. doi: 10.1017/S0952523800176126
Hynes, S. R., and Lavik, E. B. (2010). A tissue-engineered approach towards retinal repair: scaffolds for cell transplantation to the subretinal space. Graefes Arch. Clin. Exp. Ophthalmol. 248, 763–778. doi: 10.1007/s00417-009-1263-7
Idelson, M., Alper, R., Obolensky, A., Ben-Shushan, E., Hemo, I., Yachimovich-Cohen, N., et al. (2009). Directed differentiation of human embryonic stem cells into functional retinal pigment epithelium cells. Cell Stem Cell 5, 396–408. doi: 10.1016/j.stem.2009.07.002
Ilmarinen, T., Hiidenmaa, H., Kööbi, P., Nymark, S., Sorkio, A., Wang, J. H., et al. (2015). Ultrathin polyimide membrane as cell carrier for subretinal transplantation of human embryonic stem cell derived retinal pigment epithelium. PLoS One 10:e0143669. doi: 10.1371/journal.pone.0143669
Imai, H., Honda, S., Kondo, N., Ishibashi, K., Tsukahara, Y., and Negi, A. (2007). The upregulation of angiogenic gene expression in cultured retinal pigment epithelial cells grown on type I collagen. Curr. Eye Res. 32, 903–910. doi: 10.1080/02713680701604749
Iraha, S., Tu, H. Y., Yamasaki, S., Kagawa, T., Goto, M., Takahashi, R., et al. (2018). Establishment of immunodeficient retinal degeneration model mice and functional maturation of human ESC-derived retinal sheets after transplantation. Stem Cell Rep. 10, 1059–1074. doi: 10.1016/j.stemcr.2018.01.032
Irisvision (2020). Age Related Macular Degeneration Guidelines – IrisVision. Available online at: https://irisvision.com/age-related-macular-degeneration/ (accessed July 22, 2020).
Jayakody, S. A., Gonzalez-Cordero, A., Ali, R. R., and Pearson, R. A. (2015). Cellular strategies for retinal repair by photoreceptor replacement. Prog. Retin. Eye Res. 46, 31–66. doi: 10.1016/j.preteyeres.2015.01.003
Jemni Damer, N., Guedan Duran, A., Cichy, J., Lozano-Picazo, P., Gonzalez-Nieto, D., Pérez-Rigueiro, J., et al. (2020). First steps for the development of silk fibroin-based 3D biohybrid retina for age-related macular degeneration (AMD). J. Neural Eng. 17:055003. doi: 10.1088/1741-2552/abb9c0
Jin, Z., Gao, M., Deng, W., Wu, K., Sugita, S., Mandai, M., et al. (2018). Stemming retinal regeneration with pluripotent stem cells. Prog. Retin. Eye Res. 69, 38–56. doi: 10.1016/j.preteyeres.2018.11.003
Jin, Z.-B., Gao, M.-L., Deng, W.-L., Wu, K.-C., Sugita, S., Mandai, M., et al. (2019). Stemming retinal regeneration with pluripotent stem cells. Prog. Retin. Eye Res. 69, 38–56.
Johnson, T. V., and Martin, K. R. (2008). Development and characterization of an adult retinal explant organotypic tissue culture system as an in vitro intraocular stem cell transplantation model. Invest. Ophthalmol. Vis. Sci. 49, 3503–3512. doi: 10.1167/iovs.07-1601
Johnson, T. V., Oglesby, E. N., Steinhart, M. R., Cone-Kimball, E., Jefferys, J., and Quigley, H. A. (2016). Time-lapse retinal ganglion cell dendritic field degeneration imaged in organotypic retinal explant culture. Invest. Opthalmol. Vis. Sci. 57, 253–264. doi: 10.1167/iovs.15-17769
Jones, B. W., Kondo, M., Terasaki, H., Lin, Y., McCall, M., and Marc, R. E. (2012). Retinal remodeling. Jap. J. Ophthalmol. 56, 289–306. doi: 10.1007/s10384-012-0147-2
Jones, B. W., Watt, C. B., Frederick, J. M., Baehr, W., Chen, C. K., Levine, E. M., et al. (2003). Retinal remodeling triggered by photoreceptor degenerations. J. Comp. Neurol. 464, 1–16. doi: 10.1002/cne.10703
Joussen, A. M., Heussen, F. M. A., Joeres, S., Llacer, H., Prinz, B., Rohrschneider, K., et al. (2006). autologous translocation of the choroid and retinal pigment epithelium in age-related macular degeneration. Am. J. Ophthalmol. 142, 17–30. doi: 10.1016/j.ajo.2006.01.090
Julien, S., Peters, T., Ziemssen, F., Arango-Gonzalez, B., Beck, S., Thielecke, H., et al. (2011). Implantation of ultrathin, biofunctionalized polyimide membranes into the subretinal space of rats. Biomaterials 32, 3890–3898. doi: 10.1016/j.biomaterials.2011.02.016
Jung, Y. H., Phillips, M. J., Lee, J., Xie, R., Ludwig, A. L., Chen, G., et al. (2018). 3D Microstructured Scaffolds to Support Photoreceptor Polarization and Maturation. Adv. Mater. 30:e1803550. doi: 10.1002/adma.201803550
Kador, K. E., and Goldberg, J. L. (2012). Scaffolds and stem cells: delivery of cell transplants for retinal degenerations. Exp. Rev. Ophthalmol. 7, 459–470. doi: 10.1586/eop.12.56
Kador, K. E., Grogan, S. P., Dorthé, E. W., Venugopalan, P., Malek, M. F., Goldberg, J. L., et al. (2016). Control of retinal ganglion cell positioning and neurite growth: combining 3D printing with radial electrospun scaffolds. Tissue Eng. A 22, 286–294. doi: 10.1089/ten.tea.2015.0373
Kamao, H., Mandai, M., Okamoto, S., Sakai, N., Suga, A., Sugita, S., et al. (2014). Characterization of human induced pluripotent stem cell-derived retinal pigment epithelium cell sheets aiming for clinical application. Stem Cell Rep. 2, 205–218. doi: 10.1016/j.stemcr.2013.12.007
Kim, H., Cooke, M. J., and Shoichet, M. S. (2012). Creating permissive microenvironments for stem cell transplantation into the central nervous system. Trends Biotechnol. 30, 55–63. doi: 10.1016/j.tibtech.2011.07.002
Klimanskaya, I., Hipp, J., Rezai, K. A., West, M., Atala, A., and Lanza, R. (2004). Derivation and comparative assessment of retinal pigment epithelium from human embryonic stem cells using transcriptomics. Cloning Stem Cells 6, 217–245. doi: 10.1089/clo.2004.6.217
Koizumi, A., Zeck, G., Ben, Y., Masland, R. H., and Jakobs, T. C. (2007). Organotypic culture of physiologically functional adult mammalian retinas. PLoS One 2:e221. doi: 10.1371/journal.pone.0000221
Koss, M. J., Falabella, P., Stefanini, F. R., Pfister, M., Thomas, B. B., Kashani, A. H., et al. (2016). Subretinal implantation of a monolayer of human embryonic stem cell-derived retinal pigment epithelium: a feasibility and safety study in Yucatán minipigs. Graefes Arch. Clin. Exp. Ophthalmol. 254, 1553–1565. doi: 10.1007/s00417-016-3386-y
Krishna, Y., Sheridan, C., Kent, D., Kearns, V., Grierson, I., and Williams, R. (2011). Expanded polytetrafluoroethylene as a substrate for retinal pigment epithelial cell growth and transplantation in age-related macular degeneration. Br. J. Ophthalmol. 95, 569–573. doi: 10.1136/bjo.2009.169953
Kundu, B., Rajkhowa, R., Kundu, S. C., and Wang, X. (2013). Silk fi broin biomaterials for tissue regenerations. Adv. Drug Deliv. Rev. 65, 457–470. doi: 10.1016/j.addr.2012.09.043
Kundu, J., Michaelson, A., Baranov, P., Chiumiento, M., Nigl, T., Young, M. J., et al. (2018). Interphotoreceptor matrix based biomaterial: impact on human retinal progenitor cell attachment and differentiation. J. Biomed. Mater. Res. B Appl. Biomater. 106, 891–899. doi: 10.1002/jbm.b.33901
Kuno, N., Fujii, S., Kuno, N., and Fujii, S. (2011). Recent advances in ocular drug delivery systems. Polymers 3, 193–221. doi: 10.3390/polym3010193
Lai, J.-Y. (2013). Influence of solvent composition on the performance of carbodiimide cross-linked gelatin carriers for retinal sheet delivery. J. Mater. Sci. 24, 2201–2210. doi: 10.1007/s10856-013-4961-y
Lakowski, J., Han, Y. T., Pearson, R. A., Gonzalez-Cordero, A., West, E. L., Gualdoni, S., et al. (2011). Effective transplantation of photoreceptor precursor cells selected via cell surface antigen expression. Stem Cells 29, 1391–1404. doi: 10.1002/stem.694
Lamba, D. A., Gust, J., and Reh, T. A. (2009). Transplantation of human embryonic stem cell-derived photoreceptors restores some visual function in Crx-deficient mice. Cell Stem Cell 4, 73–79. doi: 10.1016/j.stem.2008.10.015
Lamba, D. A., Karl, M. O., Ware, C. B., and Reh, T. A. (2006). Efficient generation of retinal progenitor cells from human embryonic stem cells. Proc. Natl. Acad. Sci. U.S.A. 103, 12769–12774. doi: 10.1073/pnas.0601990103
Lawley, E., Baranov, P., and Young, M. (2015). Hybrid vitronectin-mimicking polycaprolactone scaffolds for human retinal progenitor cell differentiation and transplantation. J. Biomater. Appl. 29, 894–902. doi: 10.1177/0885328214547751
Lee Ventola, C. (2014). Medical applications for 3D printing: current and projected uses. P T 39, 704–711.
Lin, T. C., Seiler, M. J., Zhu, D., Falabella, P., Hinton, D. R., Clegg, D. O., et al. (2017). Assessment of safety and functional efficacy of stem cell-based therapeutic approaches using retinal degenerative animal models. Stem Cells Int. 2017:9428176. doi: 10.1155/2017/9428176
Little, C. W., Cox, C., Wyatt, J., del Cerro, C., and del Cerro, M. (1998). Correlates of Photoreceptor Rescue by Transplantation of Human Fetal RPE in the RCS Rat. Exp. Neurol. 149, 151–160. doi: 10.1006/exnr.1997.6642
Liu, B. P., Cafferty, W. B. J., Budel, S. O., and Strittmatter, S. M. (2006). Extracellular regulators of axonal growth in the adult central nervous system. Philos. Trans. R. Soc. Lond. B Biol. Sci. 361, 1593–1610. doi: 10.1098/rstb.2006.1891
Liu, L., Johnson, L. V., Hikita, S. T., Hinton, D. R., Ribeiro, R., Lu, B., et al. (2012). A novel approach for subretinal implantation of ultrathin substrates containing stem cell-derived retinal pigment epithelium monolayer. Ophthalmic Res. 48, 186–191.
Liu, X., Chen, F., Chen, Y., Lu, H., Lu, X., Peng, X., et al. (2020). Paracrine effects of intraocularly implanted cells on degenerating retinas in mice. Stem Cell Res. Ther. 11:142. doi: 10.1186/s13287-020-01651-5
Liu, Y., Wang, R., Zarembinski, T. I., Doty, N., Jiang, C., Regatieri, C., et al. (2013). The application of hyaluronic acid hydrogels to retinal progenitor cell transplantation. Tissue Eng. A 19, 135–142. doi: 10.1089/ten.tea.2012.0209
Liu, Y., Xu, H. W., Wang, L., Li, S. Y., Zhao, C. J., Hao, J., et al. (2018). Human embryonic stem cell-derived retinal pigment epithelium transplants as a potential treatment for wet age-related macular degeneration. Cell Discov. 4:50. doi: 10.1038/s41421-018-0053-y
Liu, Z., Yu, N., Holz, F. G., Yang, F., and Stanzel, B. V. (2014). Enhancement of retinal pigment epithelial culture characteristics and subretinal space tolerance of scaffolds with 200 nm fiber topography. Biomaterials 35, 2837–2850. doi: 10.1016/j.biomaterials.2013.12.069
Llonch, S., Carido, M., and Ader, M. (2018). Organoid technology for retinal repair. Dev. Biol. 433, 132–143. doi: 10.1016/j.ydbio.2017.09.028
Lluis, F., and Cosma, M. P. (2010). Cell-fusion-mediated somatic-cell reprogramming: a mechanism for tissue regeneration. J. Cell. Physiol. 223, 6–13. doi: 10.1002/jcp.22003
Lorach, H., Kang, S., Bhuckory, M. B., Trouillet, A., Dalal, R., Marmor, M., et al. (2019). Transplantation of mature photoreceptors in rodents with retinal degeneration. Transl. Vis. Sci. Technol. 8:30. doi: 10.1167/tvst.8.3.30
Lowe, A., Harris, R., Bhansali, P., Cvekl, A., and Liu, W. (2016). Intercellular adhesion-dependent cell survival and ROCK-regulated actomyosin-driven forces mediate self-formation of a retinal organoid. Stem Cell Rep. 6, 743–756. doi: 10.1016/j.stemcr.2016.03.011
Lu, A. Q., and Barnstable, C. J. (2017). Generation of photoreceptor precursors from mouse embryonic stem cells. Stem Cell Rev. Rep. 14, 247–261. doi: 10.1007/s12015-017-9773-x
Lu, B., Malcuit, C., Wang, S., Girman, S., Francis, P., Lemieux, L., et al. (2009). Long-term safety and function of RPE from human embryonic stem cells in preclinical models of macular degeneration. Stem Cells 27, 2126–2135. doi: 10.1002/stem.149
Lu, B., Zhu, D., Hinton, D., Humayun, M. S., and Tai, Y.-C. (2012). Mesh-supported submicron parylene-C membranes for culturing retinal pigment epithelial cells. Biomed. Microdevices 14, 659–667. doi: 10.1007/s10544-012-9645-8
Lu, J. T., Lee, C. J., Bent, S. F., Fishman, H. A., and Sabelman, E. E. (2007). Thin collagen film scaffolds for retinal epithelial cell culture. Biomaterials 28, 1486–1494. doi: 10.1016/j.biomaterials.2006.11.023
Lu, L., Garcia, C. A., and Mikos, A. G. (1998). Retinal pigment epithelium cell culture on thin biodegradable poly(DL-lactic-co-glycolic acid) films. J. Biomater. Sci. Polym. Ed. 9, 1187–1205. doi: 10.1163/156856298X00721
Lu, L., Nyalakonda, K., Kam, L., Bizios, R., Göpferich, A., and Mikos, A. G. (2001). Retinal pigment epithelial cell adhesion on novel micropatterned surfaces fabricated from synthetic biodegradable polymers. Biomaterials 22, 291–297. doi: 10.1016/S0142-9612(00)00179-4
Luo, C., Chen, M., and Xu, H. (2011). Complement gene expression and regulation in mouse retina and retinal pigment epithelium/choroid. Mol. Vis. 17, 1588–1597.
Maaijwee, K., Heimann, H., Missotten, T., Mulder, P., Joussen, A., and van Meurs, J. (2007). Retinal pigment epithelium and choroid translocation in patients with exudative age-related macular degeneration: long-term results. Graefes Arch. Clin. Exp. Ophthalmol. 245, 1681–1689. doi: 10.1007/s00417-007-0607-4
Maaijwee, K. J. M., Van Meurs, J. C., Kirchhof, B., Mooij, C. M., Fischer, J. H., Mackiewicz, J., et al. (2007). Histological evidence for revascularisation of an autologous retinal pigment epithelium-choroid graft in the pig. Br. J. Ophthalmol. 91, 546–550. doi: 10.1136/bjo.2006.103259
Maaijwee, K., Joussen, A. M., Kirchhof, B., and Van Meurs, J. C. (2008). Retinal pigment epithelium (RPE)-choroid graft translocation in the treatment of an RPE tear: preliminary results. Br. J. Ophthalmol. 92, 526–529. doi: 10.1136/bjo.2007.131383
MacLaren, R. E., and Pearson, R. A. (2007). Stem cell therapy and the retina. Eye 21, 1352–1359. doi: 10.1038/sj.eye.6702842
Maminishkis, A., Al-Nawaiseh, S., Wolschendorf, M., Liu, Z., Braun, N., Brinken, R., et al. (2016). A step by step protocol for subretinal surgery in rabbits. J. Vis. Exp. 13:53927. doi: 10.3791/53927
Mandai, M., Fujii, M., Hashiguchi, T., Sunagawa, G. A., Ito, S., Sun, J., et al. (2017a). iPSC-derived retina transplants improve vision in rd1 end-stage retinal-degeneration mice. Stem Cell Rep. 8, 69–83. doi: 10.1016/j.stemcr.2016.12.008
Mandai, M., Watanabe, A., Kurimoto, Y., Hirami, Y., Morinaga, C., Daimon, T., et al. (2017b). Autologous induced stem-cell-derived retinal cells for macular degeneration. N. Engl. J. Med. 376, 1038–1046. doi: 10.1056/NEJMoa1608368
Marmor, M. F., and Wolfensberger, T. J. (1998). The Retinal Pigment Epithelium: Function and Disease. Oxford: Oxford University Press. doi: 10.1007/978-94-011-5137-5_1
Martín-Martín, Y., Fernández-García, L., Sanchez-Rebato, M. H., Marí-Buyé, N., Rojo, F. J., Pérez-Rigueiro, J., et al. (2019). Evaluation of neurosecretome from mesenchymal stem cells encapsulated in silk fibroin hydrogels. Sci. Rep. 9:8801. doi: 10.1038/s41598-019-45238-4
May-Simera, H. L., Wan, Q., Jha, B. S., Hartford, J., Khristov, V., Dejene, R., et al. (2018). Primary cilium-mediated retinal pigment epithelium maturation is disrupted in ciliopathy patient cells. Cell Rep. 22, 189–205. doi: 10.1016/j.celrep.2017.12.038
McCormick, R., Pearce, I., Kaye, S., and Haneef, A. (2020). Optimisation of a novel bio-substrate as a treatment for atrophic age-related macular degeneration. Front. Bioeng. Biotechnol. 8:456. doi: 10.3389/fbioe.2020.00456
McGill, T. J., Osborne, L., Lu, B., Stoddard, J., Huhn, S., Tsukamoto, A., et al. (2019). Subretinal transplantation of human central nervous system stem cells stimulates controlled proliferation of endogenous retinal pigment epithelium. Transl. Vis. Sci. Technol. 8:43. doi: 10.1167/tvst.8.3.43
McHugh, K. J., Tao, S. L., and Saint-Geniez, M. (2014). Porous poly(ε-caprolactone) scaffolds for retinal pigment epithelium transplantation. Invest. Ophthalmol. Vis. Sci. 55, 1754–1762. doi: 10.1167/iovs.13-12833
McLelland, B. T., Lin, B., Mathur, A., Aramant, R. B., Thomas, B. B., Nistor, G., et al. (2018). Transplanted hESC-derived retina organoid sheets differentiate, integrate, and improve visual function in retinal degenerate rats. Invest. Ophthalmol. Vis. Sci. 59, 2586–2603. doi: 10.1167/iovs.17-23646
Mead, B., Berry, M., Logan, A., Scott, R. A. H., Leadbeater, W., and Scheven, B. A. (2015). Stem cell treatment of degenerative eye disease. Stem Cell Res. 14, 243–257. doi: 10.1016/j.scr.2015.02.003
Mead, B., Logan, A., Berry, M., Leadbeater, W., and Scheven, B. A. (2013). Intravitreally transplanted dental pulp stem cells promote neuroprotection and axon regeneration of retinal ganglion cells after optic nerve injury. Invest. Opthalmol. Vis. Sci. 54, 7544–7556. doi: 10.1167/iovs.13-13045
Medawar, P. B. (1948). Immunity to homologous grafted skin; the fate of skin homografts transplanted to the brain, to subcutaneous tissue, and to the anterior chamber of the eye. Br. J. Exp. Pathol. 29, 58–69.
Medeiros, N. E., and Curcio, C. A. (2001). Preservation of ganglion cell layer neurons in age-related macular degeneration. Invest. Ophthalmol. Vis. Sci. 42, 795–803.
Michalska-Małecka, K., Kabiesz, A., Nowak, M., and Śpiewak, D. (2015). Age related macular degeneration – challenge for future: pathogenesis and new perspectives for the treatment. Eur. Geriatr. Med. 6, 69–75. doi: 10.1016/j.eurger.2014.09.007
Miyagishima, K. J., Wan, Q., Corneo, B., Sharma, R., Lotfi, M. R., Boles, N. C., et al. (2016). In pursuit of authenticity: induced pluripotent stem cell-derived retinal pigment epithelium for clinical applications. Stem Cells Transl. Med. 5, 1562–1574. doi: 10.5966/sctm.2016-0037
Miyagishima, K. J., Wan, Q., Miller, S. S., and Bharti, K. (2017). A basis for comparison: sensitive authentication of stem cell derived RPE using physiological responses of intact RPE monolayers. Stem Cell Transl. Investig. 4:e1497.
Nakano, T., Ando, S., Takata, N., Kawada, M., Muguruma, K., Sekiguchi, K., et al. (2012). Self-formation of optic cups and storable stratified neural retina from human ESCs. Cell Stem Cell. 10, 771–785. doi: 10.1016/j.stem.2012.05.009
Neurotech (2020). NC-503 ECT. Available online at: http://www.neurotechusa.com/nc-503-ect.html (accessed July 21, 2020).
Niederkorn, J., Streilein, J. W., and Shadduck, J. A. (1981). Deviant immune responses to allogeneic tumors injected intracamerally and subcutaneously in mice. Invest Ophthalmol Vis Sci. 20, 355–363.
Niederkorn, J. Y., and Kaplan, H. J. (2007). Rationale for immune response and the eye. Chem. Immunol. Allergy 92, 1–3. doi: 10.1159/000099234
Niyadurupola, N., Sidaway, P., Osborne, A., Broadway, D. C., and Sanderson, J. (2011). The development of human organotypic retinal cultures (HORCs) to study retinal neurodegeneration. Br. J. Ophthalmol. 95, 720–726. doi: 10.1136/bjo.2010.181404
Oganesian, A., Gabrielian, K., Ernest, J. T., and Patel, S. C. (1999). A new model of retinal pigment epithelium transplantation with microspheres. Arch. Ophthalmol. 117, 1192–1200. doi: 10.1001/archopht.117.9.1192
Ohlemacher, S. K., Iglesias, C. L., Sridhar, A., Gamm, D. M., and Meyer, J. S. (2015). Generation of highly enriched populations of optic vesicle-like retinal cells from human pluripotent stem cells. Curr. Protoc. Stem Cell Biol. 32, 1H.8.1–1H.8.20. doi: 10.1002/9780470151808.sc01h08s32
Ortin-Martinez, A., Tsai, E. L. S., Nickerson, P. E., Bergeret, M., Lu, Y., Smiley, S., et al. (2017). A reinterpretation of cell transplantation: gFP transfer from donor to host photoreceptors. Stem Cells 35, 932–939. doi: 10.1002/stem.2552
Osakada, F., Ikeda, H., Mandai, M., Wataya, T., Watanabe, K., Yoshimura, N., et al. (2008). Toward the generation of rod and cone photoreceptors from mouse, monkey and human embryonic stem cells. Nat. Biotechnol. 26, 215–224. doi: 10.1038/nbt1384
Osborne, A., Hopes, M., Wright, P., Broadway, D. C., and Sanderson, J. (2016). Human organotypic retinal cultures (HORCs) as a chronic experimental model for investigation of retinal ganglion cell degeneration. Exp. Eye Res. 143, 28–38. doi: 10.1016/j.exer.2015.09.012
Park, S. S., Moisseiev, E., Bauer, G., Anderson, J. D., Grant, M. B., Zam, A., et al. (2017). Advances in Bone Marrow Stem Cell Therapy for Retinal Dysfunction. Amsterdam: Elsevier Ltd, doi: 10.1016/j.preteyeres.2016.10.002
Pearce, W., Hsu, J., and Yeh, S. (2015). Advances in drug delivery to the posterior segment. Curr. Opin. Ophthalmol. 26, 233–239. doi: 10.1097/ICU.0000000000000143
Pearson, R. A., Barber, A. C., Rizzi, M., Hippert, C., Xue, T., West, E. L., et al. (2012). Restoration of vision after transplantation of photoreceptors. Nature 485, 99–103. doi: 10.1038/nature10997
Pearson, R. A., Gonzalez-Cordero, A., West, E. L., Ribeiro, J. R., Aghaizu, N., Goh, D., et al. (2016). Donor and host photoreceptors engage in material transfer following transplantation of post-mitotic photoreceptor precursors. Nat. Commun. 7:13029. doi: 10.1038/ncomms13029
Peng, C. H., Chuang, J. H., Wang, M. L., Jhan, Y. Y., Chien, K. H., Chung, Y. C., et al. (2016). Laminin modification subretinal bio-scaffold remodels retinal pigment epithelium-driven microenvironment in vitro and in vivo. Oncotarget 7, 64631–64648. doi: 10.18632/oncotarget.11502
Radtke, N. D., Aramant, R. B., Petry, H. M., Green, P. T., Pidwell, D. J., and Seiler, M. J. (2008). Vision improvement in retinal degeneration patients by implantation of retina together with retinal pigment epithelium. Am. J. Ophthalmol. 146, 172–182. doi: 10.1016/j.ajo.2008.04.009
Radtke, N. D., Seiler, M. J., Aramant, R. B., Petry, H. M., and Pidwell, D. J. (2002). Transplantation of intact sheets of fetal neural retina with its retinal pigment epithelium in retinitis pigmentosa patients. Am. J. Ophthalmol. 133, 544–550. doi: 10.1016/S0002-9394(02)01322-3
Rajala, R. V. S., and Gardner, T. W. (2016). Burning fat fuels photoreceptors patient iPSCs: a new discovery tool for Smith-Lemli-Opitz syndrome. Nat. Med. 22, 342–343. doi: 10.1038/nm.4080
Ramakrishna, S., Mayer, J., Wintermantel, E., and Leong, K. W. (2001). Biomedical applications of polymer-composite materials: a review. Compos. Sci. Technol. 61, 1189–1224. doi: 10.1016/S0266-3538(00)00241-4
Redenti, S., Neeley, W. L., Rompani, S., Saigal, S., Yang, J., Klassen, H., et al. (2009). Engineering retinal progenitor cell and scrollable poly(glycerol-sebacate) composites for expansion and subretinal transplantation. Biomaterials 30, 3405–3414. doi: 10.1016/j.biomaterials.2009.02.046
Redenti, S., Tao, S., Yang, J., Gu, P., Klassen, H., Saigal, S., et al. (2008). Retinal tissue engineering using mouse retinal progenitor cells and a novel biodegradable, thin-film poly(e-caprolactone) nanowire scaffold. J. Ocul. Biol. Dis. Infor. 1, 19–29. doi: 10.1007/s12177-008-9005-3
Rose, J. B., Pacelli, S., Haj, A. J., El, Dua, H. S., Hopkinson, A., et al. (2014). Gelatin-based materials in ocular tissue engineering. Materials 7, 3106–3135. doi: 10.3390/ma7043106
Rowland, T. J., Buchholz, D. E., and Clegg, D. O. (2012). Pluripotent human stem cells for the treatment of retinal disease. J. Cell. Physiol. 227, 457–466. doi: 10.1002/jcp.22814
Rupenthal, I. D. (2017). Drug-device combination approaches for delivery to the eye. Curr. Opin. Pharmacol. 36, 44–51. doi: 10.1016/j.coph.2017.08.003
Sabel, B. A., Henrich-Noack, P., Fedorov, A., and Gall, C. (2011). Vision restoration after brain and retina damage: the ‘residual vision activation theory. Prog. Brain Res. 192, 199–262. doi: 10.1016/B978-0-444-53355-5.00013-0
Salero, E., Blenkinsop, T. A., Corneo, B., Harris, A., Rabin, D., Stern, J. H., et al. (2012). Adult human RPE can be activated into a multipotent stem cell that produces mesenchymal derivatives. Cell Stem Cell 10, 88–95. doi: 10.1016/j.stem.2011.11.018
Sandhu, H., Adeniran, J. M. F., and Kaplan, H. J. (2019). “Transplantation immunology: retinal cell-based therapy,” in Cell-Based Therapy for Degenerative Retinal Disease. Stem Cell Biology and Regenerative Medicine, eds M. Zarbin, M. Singh, and R. Casaroli-Marano (Cham: Humana Press), 141–156. doi: 10.1007/978-3-030-05222-5_8
Sanges, D., Simonte, G., Di Vicino, U., Romo, N., Pinilla, I., Nicolás, M., et al. (2016). Reprogramming Müller glia via in vivo cell fusion regenerates murine photoreceptors. J. Clin. Invest. 126, 3104–3116. doi: 10.1172/JCI85193
Santos-Ferreira, T., Llonch, S., Borsch, O., Postel, K., Haas, J., and Ader, M. (2016a). Retinal transplantation of photoreceptors results in donor-host cytoplasmic exchange. Nat. Commun. 7:13028. doi: 10.1038/ncomms13028
Santos-Ferreira, T., Völkner, M., Borsch, O., Haas, J., Cimalla, P., Vasudevan, P., et al. (2016b). Stem cell-derived photoreceptor transplants differentially integrate into mouse models of cone-rod dystrophy. Invest. Ophthalmol. Vis. Sci. 57, 3509–3520. doi: 10.1167/iovs.16-19087
Sauve, Y., and Gaillard, F. (1995). Regeneration in the Visual System of Adult Mammals. Available online at: http://www.ncbi.nlm.nih.gov/pubmed/21413374 (accessed July 8, 2020).
Schubert, C., Van Langeveld, M. C., and Donoso, L. A. (2014). Innovations in 3D printing: a 3D overview from optics to organs. Br. J. Ophthalmol. 98, 159–161. doi: 10.1136/bjophthalmol-2013-304446
Schwartz, S. D., Hubschman, J.-P., Heilwell, G., Franco-Cardenas, V., Pan, C. K., Ostrick, R. M., et al. (2012). Embryonic stem cell trials for macular degeneration: a preliminary report. Lancet 379, 713–720. doi: 10.1016/S0140-6736(12)60028-2
Schwartz, S. D., Regillo, C. D., Lam, B. L., Eliott, D., Rosenfeld, P. J., Gregori, N. Z., et al. (2015). Human embryonic stem cell-derived retinal pigment epithelium in patients with age-related macular degeneration and Stargardt’s macular dystrophy: follow-up of two open-label phase 1/2 studies. Lancet 385, 509–516. doi: 10.1016/S0140-6736(14)61376-3
Seaton, A. D., Sheedlo, H. J., and Turner, J. E. (1994). A primary role for RPE transplants in the inhibition and regression of neovascularization in the RCS rat. Invest. Ophthalmol. Vis. Sci. 35, 162–169.
Seiler, M. J., and Aramant, R. B. (2012). Cell replacement and visual restoration by retinal sheet transplants. Prog. Retin. Eye Res. 31, 661–687. doi: 10.1016/j.preteyeres.2012.06.003
Shirai, H., Mandai, M., Matsushita, K., Kuwahara, A., Yonemura, S., Nakano, T., et al. (2016). Transplantation of human embryonic stem cell-derived retinal tissue in two primate models of retinal degeneration. Proc. Natl. Acad. Sci. U.S.A. 113, E81–E90. doi: 10.1073/pnas.1512590113
Shoichet, M. S., van der Kooy, D., Cooke, M. J., Morshead, C. M., Coles, B. L. K., Donaldson, L., et al. (2015). A hyaluronan-based injectable hydrogel improves the survival and integration of stem cell progeny following transplantation. Stem Cell Rep. 4, 1031–1045. doi: 10.1016/j.stemcr.2015.04.008
Sieving, P. A., Caruso, R. C., Tao, W., Coleman, H. R., Thompson, D. J. S., Fullmer, K. R., et al. (2006). Ciliary neurotrophic factor (CNTF) for human retinal degeneration: phase I trial of CNTF delivered by encapsulated cell intraocular implants. Proc. Natl. Acad. Sci. U.S.A. 103, 3896–3901. doi: 10.1073/pnas.0600236103
Singh, D., Wang, S., Bin, Xia, T., Tainsh, L., Ghiassi-Nejad, M., et al. (2018). A biodegradable scaffold enhances differentiation of embryonic stem cells into a thick sheet of retinal cells. Biomaterials 154, 158–168. doi: 10.1016/j.biomaterials.2017.10.052
Singh, R., Cuzzani, O., Binette, F., Sternberg, H., West, M. D., and Nasonkin, I. O. (2018). Pluripotent stem cells for retinal tissue engineering: current status and future prospects. Stem Cell Rev. Rep. 14, 463–483. doi: 10.1007/s12015-018-9802-4
Singh, M. S., Balmer, J., Barnard, A. R., Aslam, S. A., Moralli, D., Green, C. M., et al. (2016). Transplanted photoreceptor precursors transfer proteins to host photoreceptors by a mechanism of cytoplasmic fusion. Nat. Commun. 7:13537. doi: 10.1038/ncomms13537
Singh, M. S., Issa, P. C., Butler, R., Martin, C., Lipinski, D. M., Sekaran, S., et al. (2013). Reversal of end-stage retinal degeneration and restoration of visual function by photoreceptor transplantation. Proc. Natl. Acad. Sci. U.S.A. 110, 1101–1106. doi: 10.1073/pnas.1119416110
Singh, M. S., and MacLaren, R. E. (2011). Stem cells as a therapeutic tool for the blind: biology and future prospects. Proc. R. Soc. B Biol. Sci. 278, 3009–3016. doi: 10.1098/rspb.2011.1028
Singh, M. S., Park, S. S., Albini, T. A., Canto-Soler, M. V., Klassen, H., MacLaren, R. E., et al. (2020). Retinal stem cell transplantation: balancing safety and potential. Prog. Retin. Eye Res. 75:100779. doi: 10.1016/j.preteyeres.2019.100779
Singh, R. K., Occelli, L. M., Binette, F., Petersen-Jones, S. M., and Nasonkin, I. O. (2019). Transplantation of human embryonic stem cell-derived retinal tissue in the subretinal space of the cat eye. Stem Cells Dev. 28, 1151–1166. doi: 10.1089/scd.2019.0090
Singh, S., Woerly, S., and McLaughlin, B. J. (2001). Natural and artificial substrates for retinal pigment epithelial monolayer transplantation. Biomaterials 22, 3337–3343. doi: 10.1016/S0142-9612(01)00171-5
Sodha, S., Wall, K., Redenti, S., Klassen, H., Young, M. J., and Tao, S. L. (2011). Microfabrication of a three-dimensional polycaprolactone thin-film scaffold for retinal progenitor cell encapsulation. J. Biomater. Sci. Polym. Ed. 22, 443–456. doi: 10.1163/092050610X487738
Sonoi, R., Kim, M. H., Yamada, K., and Kino-oka, M. (2017). Phenotypic heterogeneity of human retinal pigment epithelial cells in passaged cell populations. J. Biosci. Bioeng. 124, 227–233. doi: 10.1016/j.jbiosc.2017.03.008
Sortwell, C. E., Pitzer, M. R., and Collier, T. J. (2000). Time course of apoptotic cell death within mesencephalic cell suspension grafts: implications for improving grafted dopamine neuron survival. Exp. Neurol. 165, 268–277. doi: 10.1006/exnr.2000.7476
Srivastava, G. K., Martín, L., Singh, A. K., Fernandez-Bueno, I., Gayoso, M. J., Garcia-Gutierrez, M. T., et al. (2011). Elastin-like recombinamers as substrates for retinal pigment epithelial cell growth. J. Biomed. Mater. Res. A 97, 243–250. doi: 10.1002/jbm.a.33050
Stanzel, B. V., Liu, Z., Somboonthanakij, S., Wongsawad, W., Brinken, R., Eter, N., et al. (2014). Human RPE stem cells grown into polarized RPE monolayers on a polyester matrix are maintained after grafting into rabbit subretinal space. Stem Cell Rep. 2, 64–77. doi: 10.1016/j.stemcr.2013.11.005
Steindl, K., and Binder, S. (2008). Retinal degeneration processes and transplantation of retinal pigment epithelial cells: past, present and future trends. Spektrum der Augenheilkunde 22, 357–361. doi: 10.1007/s00717-008-0292-7
Stern, J. H., Tian, Y., Funderburgh, J., Pellegrini, G., Zhang, K., Goldberg, J. L., et al. (2018). Regenerating eye tissues to preserve and restore vision. Cell Stem Cell 22, 834–849. doi: 10.1016/j.stem.2018.05.013
Strauss, O. (2005). The retinal pigment epithelium in visual function. Physiol. Rev. 85, 845–881. doi: 10.1152/physrev.00021.2004
Streilein, J. W. (2003). Ocular immune privilege: the eye takes a dim but practical view of immunity and inflammation. J. Leukocyte Biol. 74, 179–185. doi: 10.1189/jlb.1102574
Subrizi, A., Hiidenmaa, H., Ilmarinen, T., Nymark, S., Dubruel, P., Uusitalo, H., et al. (2012). Generation of hESC-derived retinal pigment epithelium on biopolymer coated polyimide membranes. Biomaterials 33, 8047–8054. doi: 10.1016/j.biomaterials.2012.07.033
Tam, R. Y., Fuehrmann, T., Mitrousis, N., and Shoichet, M. S. (2014). Regenerative therapies for central nervous system diseases: a biomaterials approach. Neuropsychopharmacology 39, 169–188. doi: 10.1038/npp.2013.237
Tao, S., Young, C., Redenti, S., Zhang, Y., Klassen, H., Desai, T., et al. (2007). Survival, migration and differentiation of retinal progenitor cells transplanted on micro-machined poly(methyl methacrylate) scaffolds to the subretinal space. Lab Chip 7, 695–701. doi: 10.1039/b618583e
Tao, W., Wen, R., Goddard, M. B., Sherman, S. D., O’Rourke, P. J., Stabila, P. F., et al. (2002). Encapsulated cell-based delivery of CNTF reduces photoreceptor degeneration in animal models of retinitis pigmentosa. Invest. Ophthalmol. Vis. Sci. 43, 3292–3298.
Taylor, A. W. (2016). Ocular immune privilege and transplantation. Front. Immunol. 7:37. doi: 10.3389/fimmu.2016.00037
Taylor, A. W., and Ng, T. F. (2018). Negative regulators that mediate ocular immune privilege. J. Leukoc. Biol. 103, 1179–1187. doi: 10.1002/JLB.3MIR0817-337R
Taylor, L., Arner, K., Engelsberg, K., and Ghosh, F. (2013). Effects of glial cell line-derived neurotrophic factor on the cultured adult full-thickness porcine retina. Curr. Eye Res. 38, 503–515. doi: 10.3109/02713683.2013.763989
Taylor, L., Arnér, K., Holmgren Taylor, I., and Ghosh, F. (2014). Feet on the ground: physical support of the inner retina is a strong determinant for cell survival and structural preservation in vitro. Invest. Ophthalmol. Vis. Sci. 55, 2200–2213. doi: 10.1167/iovs.13-13535
Tezel, T. H., Del Priore, L. V., Berger, A. S., and Kaplan, H. J. (2007). Adult retinal pigment epithelial transplantation in exudative age-related macular degeneration. Am. J. Ophthalmol. 143, 584–595. doi: 10.1016/j.ajo.2006.12.007
Thakur, A., Mishra, S., Pena, J., Zhou, J., Redenti, S., Majeska, R., et al. (2018). Collective adhesion and displacement of retinal progenitor cells upon extracellular matrix substrates of transplantable biomaterials. J. Tissue Eng. 9:2041731417751286. doi: 10.1177/2041731417751286
Thangaraj, G., Greif, A., and Layer, P. G. (2011). Simple explant culture of the embryonic chicken retina with long-term preservation of photoreceptors. Exp. Eye Res. 93, 556–564. doi: 10.1016/j.exer.2011.07.011
Tomita, M., Lavik, E., Klassen, H., Zahir, T., Langer, R., and Young, M. J. (2005). Biodegradable Polymer Composite Grafts Promote the Survival and Differentiation of Retinal Progenitor Cells. Stem Cells 23, 1579–1588. doi: 10.1634/stemcells.2005-0111
Tu, H. Y., Watanabe, T., Shirai, H., Yamasaki, S., Kinoshita, M., Matsushita, K., et al. (2019). Medium- to long-term survival and functional examination of human iPSC-derived retinas in rat and primate models of retinal degeneration. EBioMedicine 39, 562–574. doi: 10.1016/j.ebiom.2018.11.028
Tucker, B. A., Park, I. H., Qi, S. D., Klassen, H. J., Jiang, C., Yao, J., et al. (2011). Transplantation of adult mouse iPS cell-derived photoreceptor precursors restores retinal structure and function in degenerative mice. PLoS One 6:e18992. doi: 10.1371/journal.pone.0018992
Tucker, B. A., Redenti, S. M., Jiang, C., Swift, J. S., Klassen, H. J., Smith, M. E., et al. (2010). The use of progenitor cell/biodegradable MMP2-PLGA polymer constructs to enhance cellular integration and retinal repopulation. Biomaterials 31, 9–19. doi: 10.1016/j.biomaterials.2009.09.015
U.S. National Library of Medicine (2020a). A Safety Study of CNTO 2476 in Patients With Age-Related Macular Degeneration – Tabular View – ClinicalTrials.gov. Available online at: https://clinicaltrials.gov/ct2/show/record/NCT01226628 (accessed July 21, 2020).
U.S. National Library of Medicine (2020b). A Study of an Encapsulated Cell Technology (ECT) Implant for Patients With Atrophic Macular Degeneration – Full Text View – ClinicalTrials.gov. Available online at: https://clinicaltrials.gov/ct2/show/NCT00447954 (accessed July 22, 2020).
U.S. National Library of Medicine (2020c). A Study Of Implantation Of Retinal Pigment Epithelium In Subjects With Acute Wet Age Related Macular Degeneration – Full Text View – ClinicalTrials.gov. Available online at: https://clinicaltrials.gov/ct2/show/NCT01691261 (accessed July 22, 2020).
U.S. National Library of Medicine (2020d). Autologous Transplantation of Induced Pluripotent Stem Cell-Derived Retinal Pigment Epithelium for Geographic Atrophy Associated With Age-Related Macular Degeneration – Full Text View – ClinicalTrials.gov. Available online at: https://clinicaltrials.gov/ct2/show/NCT04339764 (accessed July 22, 2020).
U.S. National Library of Medicine (2020f). Clinical Trial of Autologous Intravitreal Bone-marrow CD34+ Stem Cells for Retinopathy – Full Text View – ClinicalTrials.gov. Available online at: https://clinicaltrials.gov/ct2/show/NCT01736059 (accessed July 22, 2020).
U.S. National Library of Medicine (2020g). Evaluation of Safety of Ciliary Neurotrophic Factor Implants in the Eye – Full Text View – ClinicalTrials.gov. Available online at: https://clinicaltrials.gov/ct2/show/NCT00063765 (accessed July 22, 2020).
U.S. National Library of Medicine (2020h). Intravitreal Bone Marrow-Derived Stem Cells in Patients With Macular Degeneration – Full Text View – ClinicalTrials.gov. Available online at: https://clinicaltrials.gov/ct2/show/NCT01518127 (accessed July 22, 2020).
U.S. National Library of Medicine (2020i). Long-Term Follow-up Safety Study of Human Central Nervous System Stem Cells in Subjects With Geographic Atrophy of Age-Related Macular Degeneration – Full Text View – ClinicalTrials.gov. Available online at: https://clinicaltrials.gov/ct2/show/NCT02137915 (accessed July 22, 2020).
U.S. National Library of Medicine (2020j). Safety and Efficacy of Intravitreal Injection of Human Retinal Progenitor Cells in Adults With Retinitis Pigmentosa – Full Text View – ClinicalTrials.gov. Available online at: https://clinicaltrials.gov/ct2/show/NCT03073733 (accessed July 22, 2020).
U.S. National Library of Medicine (2020k). Safety and Efficacy Study of OpRegen for Treatment of Advanced Dry-Form Age-Related Macular Degeneration – Full Text View – ClinicalTrials.gov. Available online at: https://clinicaltrials.gov/ct2/show/NCT02286089 (accessed July 8, 2020).
U.S. National Library of Medicine (2020l). Safety and Tolerability of hRPC in Retinitis Pigmentosa – Full Text View – ClinicalTrials.gov. Available online at: https://clinicaltrials.gov/ct2/show/NCT02464436 (accessed July 22, 2020).
U.S. National Library of Medicine (2020m). Safety and Tolerability of Sub-retinal Transplantation of hESC Derived RPE (MA09-hRPE) Cells in Patients With Advanced Dry Age Related Macular Degeneration – Full Text View – ClinicalTrials.gov. Available online at: https://clinicaltrials.gov/ct2/show/NCT01344993 (accessed July 8, 2020).
U.S. National Library of Medicine (2020n). Safety of a Single, Intravitreal Injection of Human Retinal Progenitor Cells (jCell) in Retinitis Pigmentosa – Study Results – ClinicalTrials.gov. Available online at: https://clinicaltrials.gov/ct2/show/results/NCT02320812 (accessed July 22, 2020).
U.S. National Library of Medicine (2020o). Safety Study in Retinal Transplantation for Dry Age Related Macular Degeneration. – Full Text View – ClinicalTrials.gov. Available online at: https://clinicaltrials.gov/ct2/show/NCT00346060 (accessed July 22, 2020).
U.S. National Library of Medicine (2020p). Stem Cell Ophthalmology Treatment Study – Full Text View – ClinicalTrials.gov. Available online at: https://www.clinicaltrials.gov/ct2/show/NCT01920867 (accessed July 22, 2020]
U.S. National Library of Medicine (2020q). Study of HUCNS-SC Subretinal Transplantation in Subjects With GA of AMD – Full Text View – ClinicalTrials.gov. Available online at: https://clinicaltrials.gov/ct2/show/NCT02467634 (accessed July 22, 2020).
U.S. National Library of Medicine (2020r). Study of Human Central Nervous System Stem Cells (HuCNS-SC) in Age-Related Macular Degeneration (AMD) – Full Text View – ClinicalTrials.gov. Available online at: https://clinicaltrials.gov/ct2/show/NCT01632527 (accessed July 22, 2020).
U.S. National Library of Medicine (2020s). Study of Subretinal Implantation of Human Embryonic Stem Cell-Derived RPE Cells in Advanced Dry AMD – Full Text View – ClinicalTrials.gov. Available online at: https://clinicaltrials.gov/ct2/show/NCT02590692 (accessed July 8, 2020).
U.S. National Library of Medicine (2020u). Study of the Intravitreal Implantation of NT-503-3 Encapsulated Cell Technology (ECT) for the Treatment of Recurrent Choroidal Neovascularization (CNV) Secondary to Age-Related Macular Degeneration (AMD) – Full Text View – ClinicalTrials.gov. Available online at: https://clinicaltrials.gov/ct2/show/NCT02228304 (accessed July 22, 2020).
van Zeeburg, E. J. T., Maaijwee, K. J. M., Missotten, T. O. A. R., Heimann, H., and van Meurs, J. C. (2012). A free retinal pigment epithelium–choroid graft in patients with exudative age-related macular degeneration: results up to 7 years. Am. J. Ophthalmol. 153, 120.e2–127.e2. doi: 10.1016/j.ajo.2011.06.007
Völkner, M., Zschätzsch, M., Rostovskaya, M., Overall, R. W., Busskamp, V., Anastassiadis, K., et al. (2016). Retinal organoids from pluripotent stem cells efficiently recapitulate retinogenesis. Stem Cell Rep. 6, 525–538. doi: 10.1016/j.stemcr.2016.03.001
Waldron, P. V., Di Marco, F., Kruczek, K., Ribeiro, J., Graca, A. B., Hippert, C., et al. (2018). Transplanted donor- or stem cell-derived cone photoreceptors can both integrate and undergo material transfer in an environment-dependent manner. Stem Cell Rep. 10, 406–421. doi: 10.1016/j.stemcr.2017.12.008
Wang, S. W., Mu, X., Bowers, W. J., and Klein, W. H. (2002). Retinal ganglion cell differentiation in cultured mouse retinal explants. Methods 28, 448–456. doi: 10.1016/S1046-2023(02)00264-5
Warnke, P. H., Alamein, M., Skabo, S., Stephens, S., Bourke, R., Heiner, P., et al. (2013). Primordium of an artificial Bruch’s membrane made of nanofibers for engineering of retinal pigment epithelium cell monolayers. Acta Biomater. 9, 9414–9422. doi: 10.1016/j.actbio.2013.07.029
Weiner, L. P. (2008). Definitions and criteria for stem cells. Methods Mol. Biol. 438, 3–8. doi: 10.1007/978-1-59745-133-8_1
Weiss, J. N., Levy, S., and Malkin, A. (2015). Stem cell ophthalmology treatment study (SCOTS) for retinal and optic nerve diseases: a preliminary report. Neural Regen. Res. 10, 982–988. doi: 10.4103/1673-5374.158365
Wenk, E., Merkle, H. P., and Meinel, L. (2011). Silk fibroin as a vehicle for drug delivery applications. J. Control. Release 150, 128–141. doi: 10.1016/j.jconrel.2010.11.007
West, E. L., Pearson, R. A., MacLaren, R. E., Sowden, J. C., and Ali, R. R. (2009). Cell Transplantation Strategies for Retinal Repair. Amsterdam: Elsevier, doi: 10.1016/S0079-6123(09)17501-5
Wong, W. L., Su, X., Li, X., Cheung, C. M. G., Klein, R., Cheng, C.-Y., et al. (2014). Global prevalence of age-related macular degeneration and disease burden projection for 2020 and 2040: a systematic review and meta-analysis. Lancet Glob. Health 2, e106–e116. doi: 10.1016/S2214-109X(13)70145-1
Wu, H. J., Li, X. X., Dong, J. Q., Pei, W. H., and Chen, H. D. (2007). Effects of subretinal implant materials on the viability, apoptosis and barrier function of cultured RPE cells. Graefes Arch. Clin. Exp. Ophthalmol. 245, 135–142. doi: 10.1007/s00417-006-0296-4
Xian, B., Luo, Z., Li, K., Li, K., Tang, M., Yang, R., et al. (2019). Dexamethasone Provides Effective Immunosuppression for Improved Survival of Retinal Organoids after Epiretinal Transplantation. Stem Cells Int. 2019:7148032. doi: 10.1155/2019/7148032
Xiang, P., Wu, K.-C., Zhu, Y., Xiang, L., Li, C., Chen, D.-L., et al. (2014). A novel Bruch’s membrane-mimetic electrospun substrate scaffold for human retinal pigment epithelium cells. Biomaterials 35, 9777–9788. doi: 10.1016/j.biomaterials.2014.08.040
Yang, T. C., Chuang, J. H., Buddhakosai, W., Wu, W. J., Lee, C. J., Chen, W. S., et al. (2017). Elongation of axon extension for human iPSC-derived retinal ganglion cells by a nano-imprinted scaffold. Int. J. Mol. Sci. 18:2013. doi: 10.3390/ijms18092013
Yang, Y., Mohand-Said, S., Léveillard, T., Fontaine, V., Simonutti, M., and Sahel, J. A. (2010). Transplantation of photoreceptor and total neural retina preserves cone function in p23h rhodopsin transgenic rat. PLoS One 5:e13469. doi: 10.1371/journal.pone.0013469
Yao, J., Ko, C. W., Baranov, P. Y., Regatieri, C. V., Redenti, S., Tucker, B. A., et al. (2015). Enhanced differentiation and delivery of mouse retinal progenitor cells using a micropatterned biodegradable thin-film polycaprolactone scaffold. Tissue Eng. Part A 21, 1247–1260. doi: 10.1089/ten.tea.2013.0720
Yao, J., Tao, S. L., Young, M. J., Yao, J., Tao, S. L., and Young, M. J. (2011). Synthetic polymer scaffolds for stem cell transplantation in retinal tissue engineering. Polymers 3, 899–914. doi: 10.3390/polym3020899
Zarbin, M. (2016). Cell-based therapy for degenerative retinal disease. Trends Mol. Med. 22, 115–134. doi: 10.1016/j.molmed.2015.12.007
Zarbin, M. (2019). Cell-based therapy for retinal disease: the new frontier. Methods Mol. Biol. 1834, 367–381. doi: 10.1007/978-1-4939-8669-9_23
Zhang, D., Ni, N., Chen, J., Yao, Q., Shen, B., Zhang, Y., et al. (2015). Electrospun SF/PLCL nanofibrous membrane: a potential scaffold for retinal progenitor cell proliferation and differentiation. Sci. Rep. 5:14326. doi: 10.1038/srep14326
Appendix I
Abbreviations List
AMD, Age-Related Macular Degeneration
ARPE-19, human retinal pigment epithelial cell line-19
BrM, Bruch’s membrane
CNTF, ciliary neurotrophic factor
CNV, choroidal neovascularization
D-AMD, Dry Age-Related Macular Degeneration
dRPE, Pluripotent differentiated RPE
ECT, encapsulated cell technology
ELRs, elastin-like recombinamers
ESCs, embryonic- or fetal- derived stem cells
ePTFE, expanded polytetrafluoroethylene
fhRPE, fetal human RPE
FDA, US Food and Drug Administration Agency
GFP, green fluorescent protein
Gnat12/2 mice, model of congenital stationary night blindness
hESCs, human embryonic stem cells
hESCs-RPE cells, human embryonic stem cell-derived RPE cells
hiPSC, human induced pluripotent stem cells
hPSCs-PRs, human pluripotent stem cells-derived photoreceptors
hRPCs, human retinal progenitor cells
hRPE, human RPE
IPE, iris pigment epithelium
IPM, interphotoreceptor matrix
iPSC, induced pluripotent stem cell
mESC, mouse embryonic stem cell
miPSC, mouse induced pluripotent stem cell
MMP2, matrix metallopeptidase 2
mPEG-PLGA, methoxy-poly (ethylene glycol) block-poly lactic-co-glycolic acid
mPEG-PCL, methoxy poly (ethylene glycol) -b-copolymers of polycaprolactone
mSC, mesenchymal stem cell
NP, nanoparticles
NpHR, Natronomonas pharaonis halorhodopsin
PCL, poly(caprolactone)
PDMS, plasma-modified polydimethylsiloxane
PDMS-PmL, plasma-modified polydimethylsiloxane (PDMS) with laminin
PEGDMA, polyethylene glycol dimethacrylate
PEG, poly (ethylene glycol)
PET, polyethylene terephthalate
PGA, polyglycolic acid
PGS, poly(glycerol-sebacate)
PI, polyimide
PLA, poly (DL-lactic acid)
P(LA-co-CL), poly(l-lactide-co-ε-caprolactone)
PLDLA, copolymer 96/4 L-lactide/D-lactide
PLGA, poly(lactic-co-glycolic) acid
PLLA, poly (L-lactic acid)
PLLA/PLGA, poly (L-lactic acid)/poly (lactic-co-glycolic acid)
PMMA, poly (methyl methacrylate)
PPCs, photoreceptor precursor cells
PR, photoreceptors
Prph2+/Δ307, model of retinitis pigmentosa
PSCs, pluripotent stem cells
PTFE, Polytetrafluoroethylene
RGD, arginine–glycine–aspartic acid
RPCs, retinal progenitor cell
RPE, retinal pigment epithelium
RPESC, retinal pigment epithelium stem cells
RSCs, retinal stem cells
SC, stem cell
TDSC, tissue-derived stem cells
TRL, clinical trials
VEGF, vascular endothelium growth factor
VEGF-Fc, VEGF receptor fragment crystallizable region
W-AMD, Wet Age-Related Macular Degeneration
Keywords: retinal pigment epithelium, Bruch’s membrane, photoreceptors, biomaterials, cell therapy, tissue engineering, biotechnology, cell replacement
Citation: Jemni-Damer N, Guedan-Duran A, Fuentes-Andion M, Serrano-Bengoechea N, Alfageme-Lopez N, Armada-Maresca F, Guinea GV, Perez-Rigueiro J, Rojo F, Gonzalez-Nieto D, Kaplan DL and Panetsos F (2020) Biotechnology and Biomaterial-Based Therapeutic Strategies for Age-Related Macular Degeneration. Part II: Cell and Tissue Engineering Therapies. Front. Bioeng. Biotechnol. 8:588014. doi: 10.3389/fbioe.2020.588014
Received: 28 July 2020; Accepted: 19 November 2020;
Published: 10 December 2020.
Edited by:
Zhengwei Mao, Zhejiang University, ChinaReviewed by:
PaYaM ZarrinTaj, Oklahoma State University, United StatesJindan Wu, Zhejiang Sci-Tech University, China
Copyright © 2020 Jemni-Damer, Guedan-Duran, Fuentes-Andion, Serrano-Bengoechea, Alfageme-Lopez, Armada-Maresca, Guinea, Perez-Rigueiro, Rojo, Gonzalez-Nieto, Kaplan and Panetsos. This is an open-access article distributed under the terms of the Creative Commons Attribution License (CC BY). The use, distribution or reproduction in other forums is permitted, provided the original author(s) and the copyright owner(s) are credited and that the original publication in this journal is cited, in accordance with accepted academic practice. No use, distribution or reproduction is permitted which does not comply with these terms.
*Correspondence: Fivos Panetsos. Zml2b3NAdWNtLmVz
†These authors have contributed equally to this work