- 1Department of Ophthalmology, Ninth People’s Hospital, Shanghai Jiao Tong University School of Medicine, Shanghai, China
- 2Shanghai Key Laboratory of Orbital Diseases and Ocular Oncology, Shanghai, China
The integration of photothermal therapy and chemotherapy has been recognized to be an efficient strategy through the instant thermally ablation and long-term chemical inhibition, thus achieving high therapeutical effect. In the present work, we designed and prepared Cu7S4@SiO2/DOX nanocomposites and used them as efficient nanoplatforms for synergistic photothermal-chemo therapy on melanoma tumors. The Cu7S4@SiO2/DOX was constructed by firstly synthesizing Cu7S4 nanocrystals, then in situ growing SiO2 shell on the surface of Cu7S4 nanocrystals, and finally loading DOX within SiO2 shell. The Cu7S4@SiO2/DOX was composed of Cu7S4 core as the photothermal transducer, SiO2 shell as DOX carrier and DOX as the model of anticancer drug. Once exposed to a 1064 nm laser, the Cu7S4@SiO2/DOX could simultaneous generate heat for photothermal therapy and accelerate the DOX release. When the Cu7S4@SiO2/DOX was injected into the center of tumor, the tumor exhibit rapid temperature elevation once exposed to the NIR laser and the tumor growth is significantly inhibited through the synergistic photothermal-chemo therapy, in comparison to the limited therapeutical effect of photothermal therapy or chemotherapy alone. Therefore, the Cu7S4@SiO2/DOX with photothermal-chemo function can be used as excellent nanoplatforms for treating solid tumor with high theoretical effect.
Introduction
The near infrared (NIR) light-driven cancer treatments have caught a numerous attention for years due to the NIR light with higher tissue-penetration depth than visible light and the better safety than ultraviolet light (Cheng et al., 2014; Vankayala and Hwang, 2018). Among these NIR-induced therapy modalities, the photothermal therapy is an emerging one that utilizes NIR absorbents as energy transducers to convert NIR laser energy into heat (>42°C), so that thermally ablate cancer cells (Zou et al., 2016; Yu et al., 2018b). For the development of photothermal therapy, the photothermal nanoagents are the key point and they should be capable of strong and broad NIR photoabsorption and high photothermal conversion efficiency. Apart from the noble metal nanostructures (Dreaden et al., 2011; Huang et al., 2011) and the organic-based nanoparticles (Liu et al., 2013; Zha et al., 2013), the recent progress of photothermal nanoagents focus on semiconductor nanomaterials because of their abundant types, tunable composites, photostability as well as efficient photothermal effect (Huang et al., 2017). The semiconductor-based photothermal nanomaterials mainly include the metal oxides such as the doped TiO2–x nanocrystals (Ren et al., 2015; Ou et al., 2016; Yu et al., 2017) and oxygen-deficient WO3–x nanocrystals (Xu et al., 2015; Wen et al., 2016), and the metal sulfides including Cu2–xS nanostructures (Tian et al., 2011a,b) and Bi2S3 nanodots (Li et al., 2016). For example, Cu7.2S4 nanoparticles were prepared by the thermolysis of Cu(DEDTC)2 precursors and they exhibited strong NIR absorption, photostability and photothermal conversion efficiency up to 56.7%, which were used as a photothermal nanoagent for the photothermal ablation of cancer cells (Li et al., 2014). However, the therapeutical effect of photothermal therapy is executed only under the illumination of laser and it would disappear instantly when laser is switched off. Thus, to achieve the long-term therapeutical effect for photothermal nanoagents, it is quite necessary to combine with other therapeutical modalities.
The integration of photothermal therapy and chemotherapy has been recognized to be an efficient strategy through the instant thermally ablation and long-term chemical inhibition (You et al., 2012; Yu et al., 2018a; Zhang et al., 2020). Importantly, the photothermal effect can accelerate the drug releasing rate and induce the chemo-sensitization effect, thus achieving the synergistic effect with higher therapeutical results than photothermal therapy or chemotherapy alone. Up to data, there are a number of nanoagents with the photothermal effect and drug loading capacity, which can be allocated into two types. The first type consists the photothermal nanomaterials with a large volume of inner cavity or high specific surface area, such as CuS hollow nanospheres (Wang et al., 2018), two-dimensional MoS2 nanosheets (Liu et al., 2014) and metal-organic frameworks (Zhang et al., 2018). For instance, CuS hollow nanospheres with 87.7% of high doxorubicin (DOX) content were prepared while the DOX release rate at pH 7.4 was only 4.6% and at pH 5.0 was 10.3% within 10 h (Wang et al., 2018). The second type is the combination of photothermal nanoagent and drug carrier, which includes Cu9S5@mSiO2-PEG core-shell structures (Song et al., 2013), Au@copolymer-liposome nanostructures (Zheng et al., 2016) and CuS@gel nanocomposites (Meng et al., 2016). For example, a thermosensitive MEO2MA@MEO2MA-co-OEGMA nanogels were firstly prepared and then CuS nanoagents were in situ deposited within nanogels, whereas the DOX loading content was less than 10%, (Meng et al., 2016). Therefore, it is of great importance to design and synthesis of photothermal-chemo nanoagents with the high photothermal conversion efficiency and high drug loading capacity.
In order to integrate synergistic photothermal-chemo functions, we prepared a Cu7S4@SiO2/DOX. The Cu7S4@SiO2/DOX was constructed by firstly synthesizing Cu7S4 nanocrystals, then in situ growing SiO2 shell on the surface of Cu7S4 nanocrystals, and finally loading DOX within SiO2 shell. The Cu7S4@SiO2 nanoplatforms exhibited the strong and broad NIR absorption and could rapidly convert 1064 nm laser energy into heat with the efficiency of 48.2%, and they also demonstrated large specific surface area and pores with high DOX loading content of 59.8%. Importantly, After the irradiation cycles, 90.1% of DOX was released from Cu7S4@SiO2/DOX with the help of 1064 nm NIR laser at pH 5.4 in comparison to 61.5% of the released DOX without irradiation, indicating photothermal effect accelerated the DOX release. More importantly, when Cu7S4@SiO2/DOX was intratumorally injected into tumor-bearing mice, the tumor growth was heavily inhibited through the synergistic photothermal-chemo therapy compared with the limited therapeutical effect of photothermal therapy or chemotherapy alone. Therefore, the Cu7S4@SiO2/DOX with high photothermal conversion efficiency and drug loading capacity can be used for treating solid tumor with high therapeutical effect.
Materials and Methods
Materials
Sodium diethyldithiocarbamate (SDEDTC), CuCl2⋅2H2O (AR), oleic acid (AR), oleylamine (80–90%), cetyltrimethylammonium bromide (CTAB), sodium hydroxide (NaOH, AR), doxorubicin hydrochloride (DOX) and tetraethylorthosilicate (TEOS, GR) were brought from Sigma Aldrich.
Preparation of Cu7S4 Nanocrystals
The Cu7S4 nanocrystals were prepared by a typical thermolysis method (Li et al., 2014). Firstly, CuCl2⋅2H2O (20 mmol) was dissolved into 10 mL deionized water, which was then dropwise added into aqueous solution of SDEDTC (90 mL, 50 mmol) under magnetically stirring. After stirring for 2 h, the above solution was centrifuged (5000 rpm, 5 min) and washed with deionized water. The precipitate [Cu(DEDTC)2 precursor] was dried in vacuum at 50°C for further use. Secondly, oleic acid (15 mL) and oleylamine (10 mL) were added into three-neck bottle and heated to 280°C within 30 min under the continuous nitrogen flow to remove any moisture and oxygen. The Cu(DEDTC)2 precursor (1 mmol) dissolved in 2 mL oleic acid was injected to the three-neck bottle and heated at 280°C for 10 min. The dark green mixture was quickly cooled to 60°C by air flow and then 20 mL ethanol was introduced to precipitate Cu7S4 nanocrystals. Finally, the Cu7S4 nanocrystals were dispersed in ethanol followed by centrifuging and washed with ethanol for three times.
Preparation of Cu7S4@SiO2 Nanoplatforms
The Cu7S4@SiO2 nanoplatforms were prepared by in situ growing SiO2 shell on the surface of Cu7S4 nanocrystals. Firstly, the hydrophobic Cu7S4 nanocrystals were converted into hydrophilic Cu7S4 through the surface-modification with CTAB. The Cu7S4 nanocrystals in chloroform solution (5 mL, 10 mg/mL) were added into aqueous solution of CTAB (20 mL, 100 mg/mL), which was stirred vigorously at 40°C for 24 h and then centrifuged to collect hydrophilic Cu7S4 nanocrystals. Secondly, the hydrophilic Cu7S4 nanocrystals were dispersed into 50 mL deionized water, followed by the introduction of NaOH solution (0.1 mL, 10 mg/mL) and TEOS (0.1 mL) under sonication. After 1 h of sonication, then 100 μL of PEG-silane was added and the mixture was maintained at 40°C for another 8 h. The products were centrifuged and washed with deionized water for three times for collecting Cu7S4@SiO2 nanoplatforms.
Characterizations
The size, morphology, phase of Cu7S4@SiO2 nanoplatforms were characterized by using JEOL 2100F transmission electron microscopy (TEM). The photoabsorption of Cu7S4@SiO2 nanoplatforms was studied on a Shimadzu UV-1900 spectrophotometer. The concentration of copper irons released from the Cu7S4@SiO2 nanoplatforms was determined by an inductively coupled plasma atomic emission spectroscopy (ICP-AES). The specific surface area and pore diameter of Cu7S4@SiO2 powder were investigated on the Autosorb-iQ/Autosorb-iQ Brunauer-Emmett-Teller (BET).
Photothermal Conversion Efficiency
The photothermal performance of Cu7S4@SiO2 nanoplatforms was investigated by illuminating their aqueous dispersion at a series of concentrations under a 1064 nm laser with the output power density of 0.6 Wcm–2. The temperature change was recorded by using a thermal imaging camera. The photothermal conversion efficiency of Cu7S4@SiO2 was calculated according to the previous report (Li et al., 2014) by applying the below equations:
Where I and A1064, respectively, stand for the NIR laser intensity and the absorbance at 1064 nm. ΔTmax,dis and ΔTmax,H_2O are the temperature change of deionized water and the solution containing Cu7S4@SiO2 nanoplatforms. The h and A are the heat transfer coefficient and the surface area, and the value of hA is determined from Eq. 2 by using the system time constant τs with the help of the mass (mD) and the heat capacity (CD) of deionized water.
Dox Loading and Releasing
For loading DOX, Cu7S4@SiO2 (2.5 mL, 10 mg/mL) and DOX (10 mg) were dispersed into PBS solution, which was magnetically stirred in the dark. After 24 h of stir, the mixture was centrifuged (12,000 rpm, 30 min) and the supernatant was collected. The DOX loading content was calculated based on (load weight of DOX/Cu7S4@SiO2/DOX) × 100%, in which the load weight of DOX was determined by (10 mg – DOX in the supernatant). For DOX releasing, Cu7S4@SiO2/DOX was dispersed into PBS at pH 7.4 or pH 5.4 and divided into two groups. One group was used as control and 0.5 mL solution were taken out which was centrifuged (12,000 rpm, 15 min) at each time point. Another group was irradiated by a 1064 nm NIR laser (0.6 Wcm–2) at a specific time. The DOX releasing rate was calculated by using (the release weight of DOX/the load weight of DOX) × 100%.
Photothermal-Chemo Therapy in vitro
Melanoma cells were seeded into a 96-well plate (1.2 × 104 cells per well) at 37°C in the presence of 5% CO2 for 24 h. After incubation, the cell medium was removed, the cells were divided into four groups: (1) control, (2) DOX, (3) Cu7S4@SiO2/DOX+NIR, (4) Cu7S4@SiO2+NIR. Hundred microliter of the dispersion at varied concentrations was then added into the wells. After incubation for another 24 h, the cells were washed with PBS buffer solution for three times. Then the cells were irradiated with/without a 1064 nm laser. Cell viability was measured using the CCK-8 assay.
To visually compare the viability difference in cellular level among four groups, cells were seeded into a 24-well plate at a density of 1.2 × 105 cells per well. After the cells in the four groups were treated, the cells were stained with calcein AM (live cells) and propidium iodide (dead cells) to distinguish live cells with green fluorescence and dead cells with red fluorescence.
Photothermal-Chemo Therapy in vivo and Histological Examination
The BALB/c mice (∼16 g, male) with 4T1 tumors (the surface diameter of 0.3∼0.5 cm) on the back were divided into four groups (n = 3): (1) The control group; (2) DOX group; (3) Cu7S4@SiO2+NIR group; (4) Cu7S4@SiO2/DOX+NIR group. The mice in (2) group were intratumorally injected with DOX PBS solution (50 μL, 80 μg), and mice in (3 and 4) group were, respectively, injected with Cu7S4@SiO2 (50 μL, 0.1 mg mL–1) or Cu7S4@SiO2/DOX PBS solution (50 μL, 0.1 mg mL–1). The tumors on mice in (3, 4) group were exposed to a 1064 nm NIR laser (0.6 Wcm–2) at the 0.5 h post-injection and mice body. After treatments, mice in all groups were observed regarding their body weight and tumor sizes. When a tumor size was beyond 1.0 cm, mice in all groups were sacrificed and tumors were extracted for histological examination.
Results and Discussion
The Cu7S4@SiO2 nanoplatforms were prepared by in situ growing SiO2 shell on the surface of Cu7S4 nanocrystals. Firstly, Cu7S4 nanocrystals were synthesized through a typical thermolysis method, where the Cu(DEDTC)2 precursor with Cu source and S source was heated to 280°C for 10 min to produce uniform Cu7S4 nanocrystals. Secondly, the Cu7S4 nanocrystals were served as the core to allow the coating of SiO2 shell through the hydrolysis of TEOS. During the SiO2 coating, CTAB were used as a soft template. SiO2 grew around the template due to electrostatic interactions. Supplementary Figure S1 shows the size and zeta potential of nanoparticles during the process of synthesis. FTIR spectra in Supplementary Figure S2 demonstrated that PEG was coated on the surface of Cu7S4@SiO2 nanoplatforms, thus the nanoplatforms could be realized the bioapplication directly. The as-obtained Cu7S4@SiO2 nanoplatforms have a uniform morphology and the average size of 100 nm, as shown in the TEM image (Figure 1a). Obviously, the Cu7S4@SiO2 nanoplatforms consist of Cu7S4 as the core and SiO2 as the shell, and the Cu7S4 nanocrystals have the average diameter of 50 nm and the SiO2 shells have the average thickness of 25 nm. The nanoplatforms showed good dispersion as the size in water showed little change over time demonstrated by dynamic light scattering (DLS, Supplementary Figure S3). Furthermore, the high-resolution (HR-TEM) image in Figure 1b demonstrates the apparent lattice with an interplane d spacing of ∼0.277 nm, which can be indexed to the (110) plane of the orthorhombic Cu7S4 (JCPDS card no. 22-0250), which verifies the core is Cu7S4 nanocrystals. Subsequently, the phase of Cu7S4@SiO2 powders was characterized by using XRD. The XRD pattern (Figure 1c) reveals that there are four prominent peaks centered at 27.87°, 32.30°, 46.40°, and 54.70°, which can be respectively, corresponded to the (202), (220), (224), and (422) planes for the orthorhombic Cu7S4 (JCPDS card no. 22-0250). It should be noted that there is a broad peak between 10° and 40°, which should be attributed to the characteristic peak originating from the amorphous SiO2 shell. Supplementary Figure S4 shows the XPS spectra of Cu 2p in the Cu7S4@SiO2 nanoparticles. The binding energy peaks at 932.8 and 954.6 eV can be assigned to Cu+ coordinated to Cu in Cu7S4@SiO2 nanoparticles, whereas the binding energy peak at 943.2 eV is formally described as Cu2+. The coexistence of Cu+ and Cu2+ indicated the Cu vacancies on the surface of Cu7S4 nanocrystals (Li et al., 2015). Thus, the above results confirmed the successful preparation of Cu7S4@SiO2 nanoplatforms with Cu7S4 core and SiO2 shell.
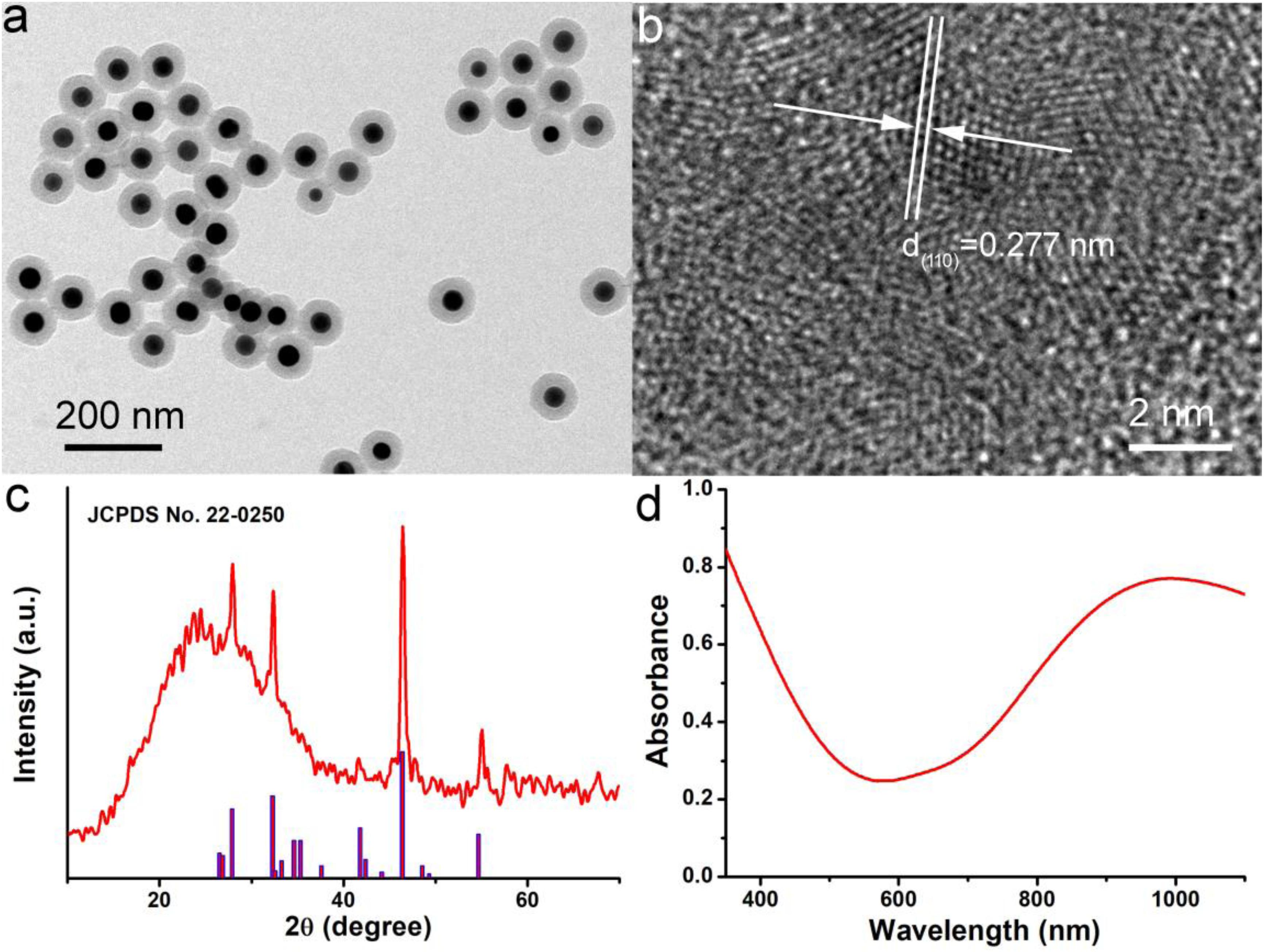
Figure 1. (a) TEM image of Cu7S4@SiO2 nanoplatforms showing the Cu7S4 core and mesoporous SiO2 shell. (b) HR-TEM image of Cu7S4 core with the clear lattice. (c) XRD pattern of Cu7S4@SiO2 nanoparticles. (d) The typical UV-vis-NIR photoabsorption spectrum of Cu7S4@SiO2 dispersed in deionized water.
Subsequently, the photoabsorption of Cu7S4@SiO2 nanoplatforms was studied by using UV-vis-NIR spectrometer. The Cu7S4@SiO2 nanoplatforms can be well dispersed into deionized water and their aqueous solution shows the dark green. As demonstrated in UV-vis-NIR photoabsorption spectra (Figure 1d), the aqueous solution containing Cu7S4@SiO2 nanoplatforms exhibits the strong absorption in the UV-vis region with the edge at ∼590 nm, which can be ascribed to the bandgap absorption of Cu7S4 as a typical semiconductor. Importantly, the aqueous solution of Cu7S4@SiO2 demonstrates a strong and broad NIR absorption (>650 nm) and the absorption intensity goes up with the increase of wavelength up to 1100 nm. Compared to the bandgap-induced UV-vis absorption, this kind of NIR absorption should be attributed to the localized surface plasmon resonances (LSPR) effect because of Cu vacancies on the surface of Cu7S4 nanocrystals, which has been reported for other Cu2–xS nanocrystals (Zhao et al., 2009; Luther et al., 2011). By determining the Cu7S4@SiO2 nanoparticle concentration via ICP-AES, the extinction coefficient of the nanoparticles at 1064 nm was measured to be 13.9 L g–1 cm–1, which was higher than the that of cobalt/manganese oxide (CMO) nanocrystals (Liu et al., 2019). Thus, Cu7S4@SiO2 nanoplatforms are capable of strong and broad NIR photoabsorption because of Cu7S4 core.
Owing to the strong NIR absorption, we further explored the photothermal performance of Cu7S4@SiO2 nanoparticles. The wavelength of NIR laser is quite important for photothermal therapy, and two biological transparency windows are reported as NIR-I (650–950 nm) and NIR-II (1000–1350 nm). Compared to the commonly used 808 nm, 915 m and 980 nm NIR laser, 1064 nm NIR laser can offer more efficient tissue penetration depth by considering absorption and scattering effects (Tsai et al., 2013). Therefore, we selected 1064 nm NIR laser to study the photothermal effect of Cu7S4@SiO2 nanoparticles. When exposed to a 1064 nm NIR laser at the intensity of 0.6 W cm–2, the temperature of deionized water increases slightly (∼1.3°C) within 5 min of irradiation, which confirmed the negligible photothermal effect from deionized water (Figure 2A). In contrast, once exposed to laser, the aqueous solutions containing Cu7S4@SiO2 nanoparticles exhibit rapid temperature elevation within 120 s and then show a slow temperature elevation due to the balance between heat production and loss. Figure 2B summarizes the temperature elevation versus to the concentration, and they are determined to be 14.2, 21.8, 31.5, and 39.4°C for the concentration of 10, 20, 30, and 40 ppm, respectively. To further clarify the photothermal performance of Cu7S4@SiO2 nanoparticles, we carried out an experiment to calculate its photothermal conversion efficiency. The aqueous solution of Cu7S4@SiO2 nanoparticles were subjected to a 1064 nm NIR laser on/off, and the whole temperature change was recorded in Figure 2C. The system time constant τs can be obtained by linearly plotting the time data with ln(θ), as shown in Figure 2D. By referencing to the previous reports, the photothermal conversion efficiency of Cu7S4@SiO2 nanoparticles is 48.2% which is comparable to the reported CuS nanomaterials (Li et al., 2014). Therefore, the Cu7S4@SiO2 nanoparticles can rapidly and efficiently convert 1064 nm NIR laser energy into heat with the concentration-relied photothermal performance.
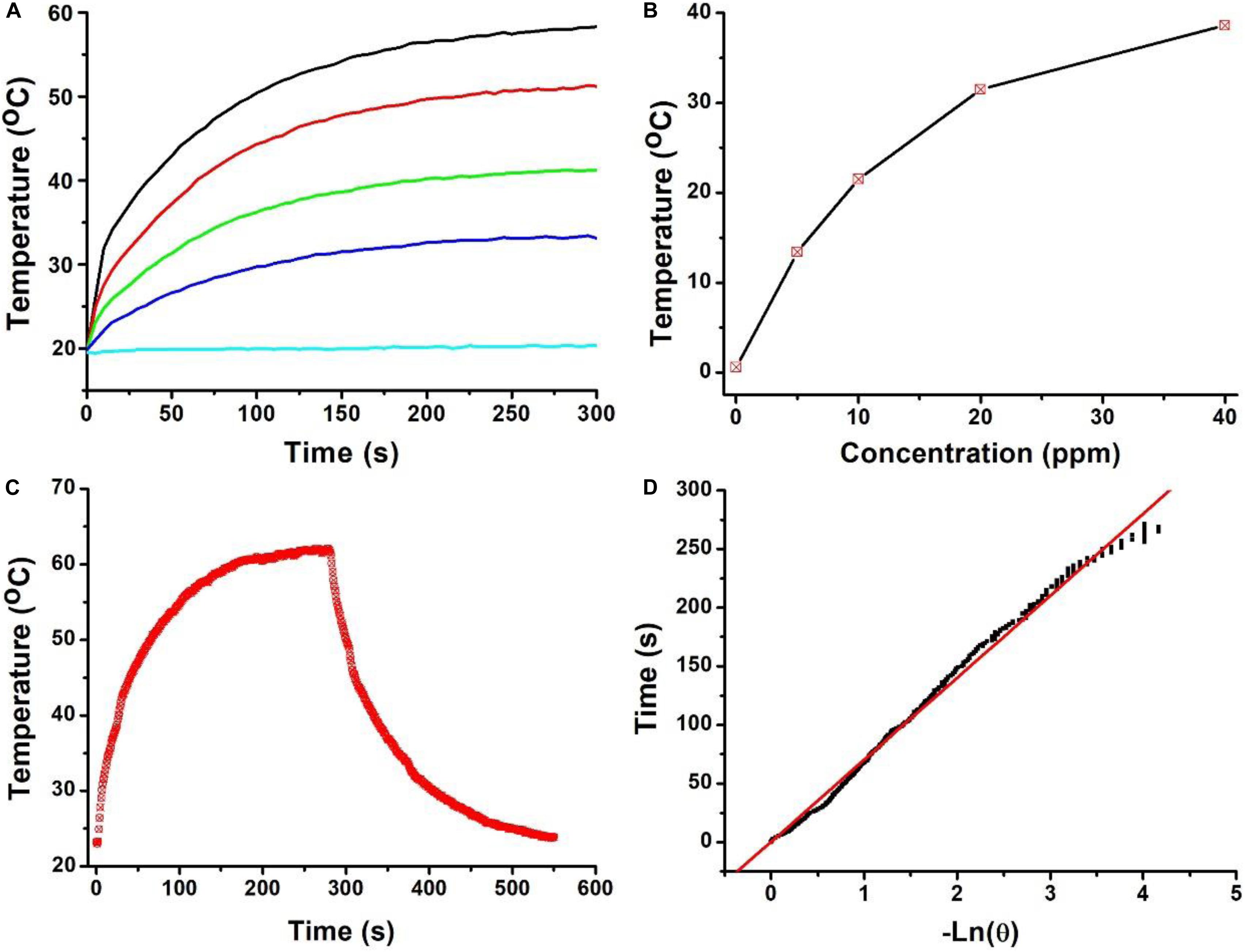
Figure 2. (A) Temperature curves of solution containing the Cu7S4@SiO2 nanoparticles with the Cu concentration of 0–40 ppm. (B) The Temperature elevation versus the concentrations of Cu7S4@SiO2 nanoparticles. (C) Temperature curve of a solution containing the Cu7S4@SiO2 nanoparticles with 1064 nm NIR laser on/off. (D) The linear fit of time data with ln(θ) to obtain system time constant τs.
After demonstrating the photothermal performance of Cu7S4@SiO2 nanoparticles, we evaluated their DOX loading capacity due to the SiO2 shell. Prior to loading DOX, the Brunauer-Emmett-Teller (BET) surface area and pore size were investigated by using nitrogen adsorption-desorption curves. The nitrogen adsorption/desorption isotherms (Figure 3A) illustrate that the Cu7S4@SiO2 nanoparticles have a specific surface area of 125.9 m2/g, showing the high surface area. In addition, the pore diameter of Cu7S4@SiO2 nanoparticles was also recorded and the average pore diameter was determined to be ∼4.5 nm (Figure 3B). Therefore, the mesoporous SiO2 shell confers high specific surface area and pores, which will facilitate the following DOX loading.
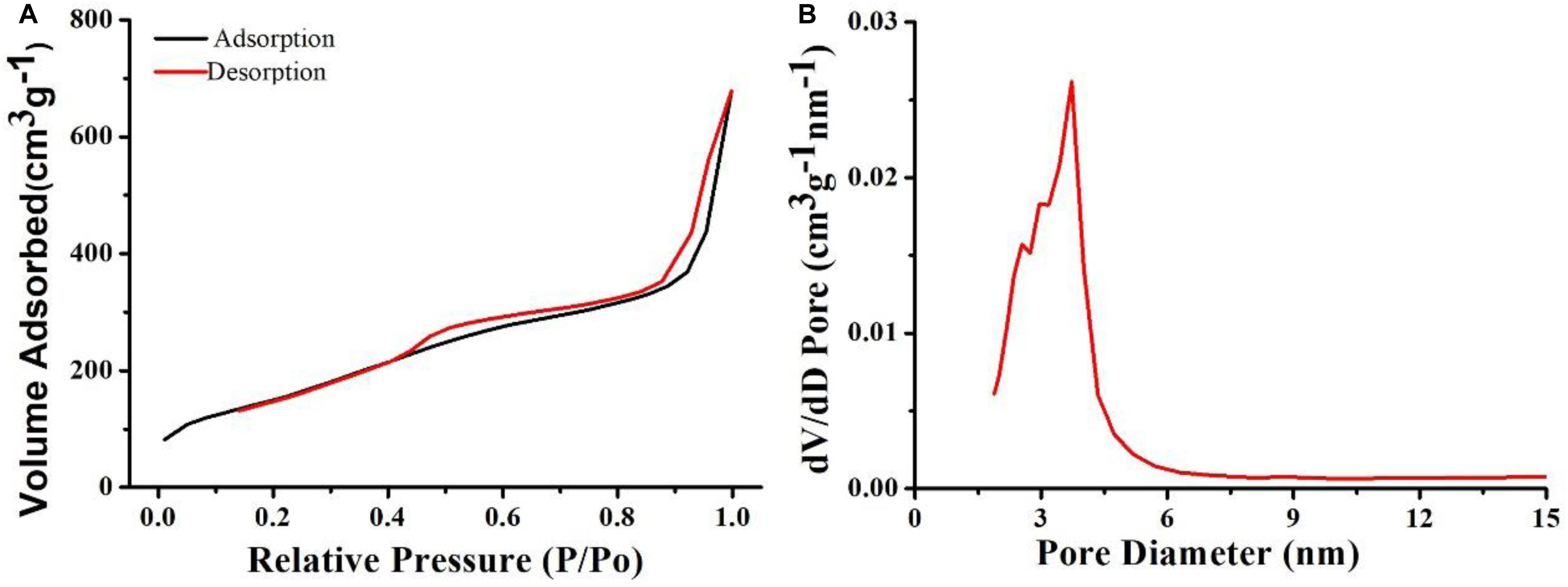
Figure 3. (A) The nitrogen adsorption-desorption curve of Cu7S4@SiO2 nanoparticles. (B) The pore diameter of Cu7S4@SiO2 nanoparticles.
The release ability of DOX from Cu7S4@SiO2/DOX (the DOX loading content is 29.8%) was studied by dispersing Cu7S4@SiO2/DOX into PBS solution. At the time point of 1, 2, 3, 4, 6, 8, and 10 h, part of solution was taken out from the original 10 mL dispersion and centrifuged for collecting the released DOX in the supernatant, and the amount of DOX in the supernatant was calculated by applying absorption-concentration curve. Figure 4A shows the DOX releasing profile at pH 7.4, and it is clear that, with the prolong of time, the DOX releasing rate unceasingly goes up which can be determined to be 19.5% at 1 h, 28.4% at 2 h, and 39.4% at 4 h. After 4 h, the DOX releasing rate becomes very slow. In order to study the effect of NIR laser-induced photothermal effect on the DOX releasing rate, we irradiated the Cu7S4@SiO2/DOX dispersion at the time point of 1, 2, 3, 4, 6, 8, and 10 h by using a 1064 nm NIR laser (5 min, 0.6 W cm–2). For instance, after the firstly irradiation, the DOX releasing rate is calculated to be 45.8% which is much higher than that (28.4%) at the 2 h, indicating NIR laser-induced photothermal effect can significantly enhance the DOX releasing rate. After the irradiation cycles, 75.6% of DOX is released from Cu7S4@SiO2/DOX with the help of 1064 nm NIR laser in comparison to 47.4% of the released DOX without irradiation, within 10 h. Thereby, it is concluded that the Cu7S4 nanocrystals within Cu7S4@SiO2/DOX can produce heat to accelerate the DOX release. To study the DOX release behavior from Cu7S4@SiO2/DOX at the simulating tumor microenvironment, the release of the DOX against buffer solution at pH 5.4 was measured. It was found that the drug release rate became much faster at pH 5.4 (Figure 4B) due to the increase in the solubility of the DOX under acidic conditions, which is beneficial for cancer therapy since the microenvironments of extracellular tissues of tumors and intracellular lysosomes and endosomes are acidic.
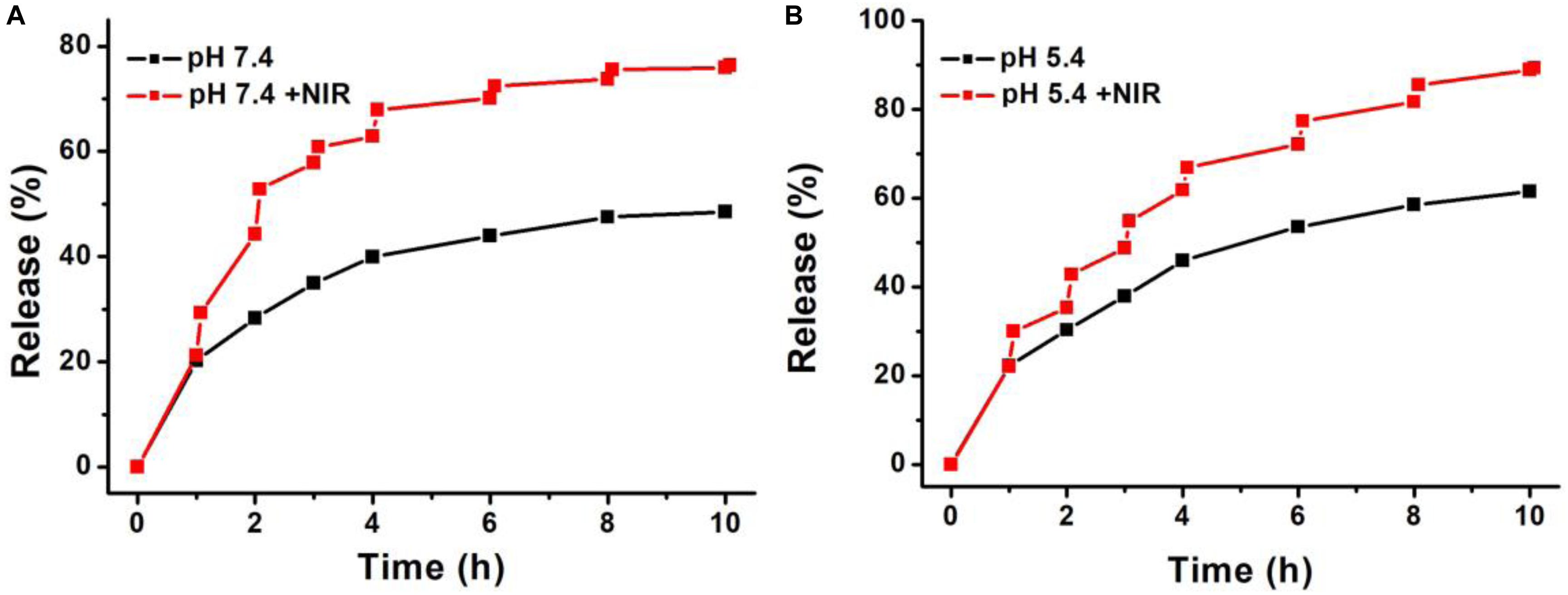
Figure 4. The DOX-releasing profile of Cu7S4@SiO2/DOX in (A) PBS solution (pH = 7.4) and (B) acetate buffer (pH 5.4) in the absence or presence of 1064 nm NIR laser.
Giving the efficient photothermal conversion effect, the high DOX loading capacity and the NIR-enhanced DOX releasing, Cu7S4@SiO2/DOX can be used as excellent nanoagents for photothermal-chemo therapy. To demonstrate the synergy effect of photothermal-chemo therapy, the combination index of Cu7S4@SiO2/DOX nanoparticles was measured according to previous work (Wang et al., 2013). The half-maximal inhibitory concentration (IC50) of cancer cells incubated with Cu7S4@SiO2/DOX nanoparticles for combination photothermal-chemo therapy is 0.52 mg mL–1, while the IC50 for the sole photothermal therapy and chemotherapy is 0.67 and 1.35 mg mL–1, respectively. The combination index was calculated to evaluate the combination effect of different therapies and found to be 0.823, which demonstrated the synergistic effect of Cu7S4@SiO2/DOX nanoparticles for photothermal-chemo therapy. Additionally, to visualize the efficiency of photothermal-chemo therapy, cells after the indicated treatments were co-stained with calcein-AM and propidium iodide (PI, Figure 5). The results further demonstrated the synergetic effect.
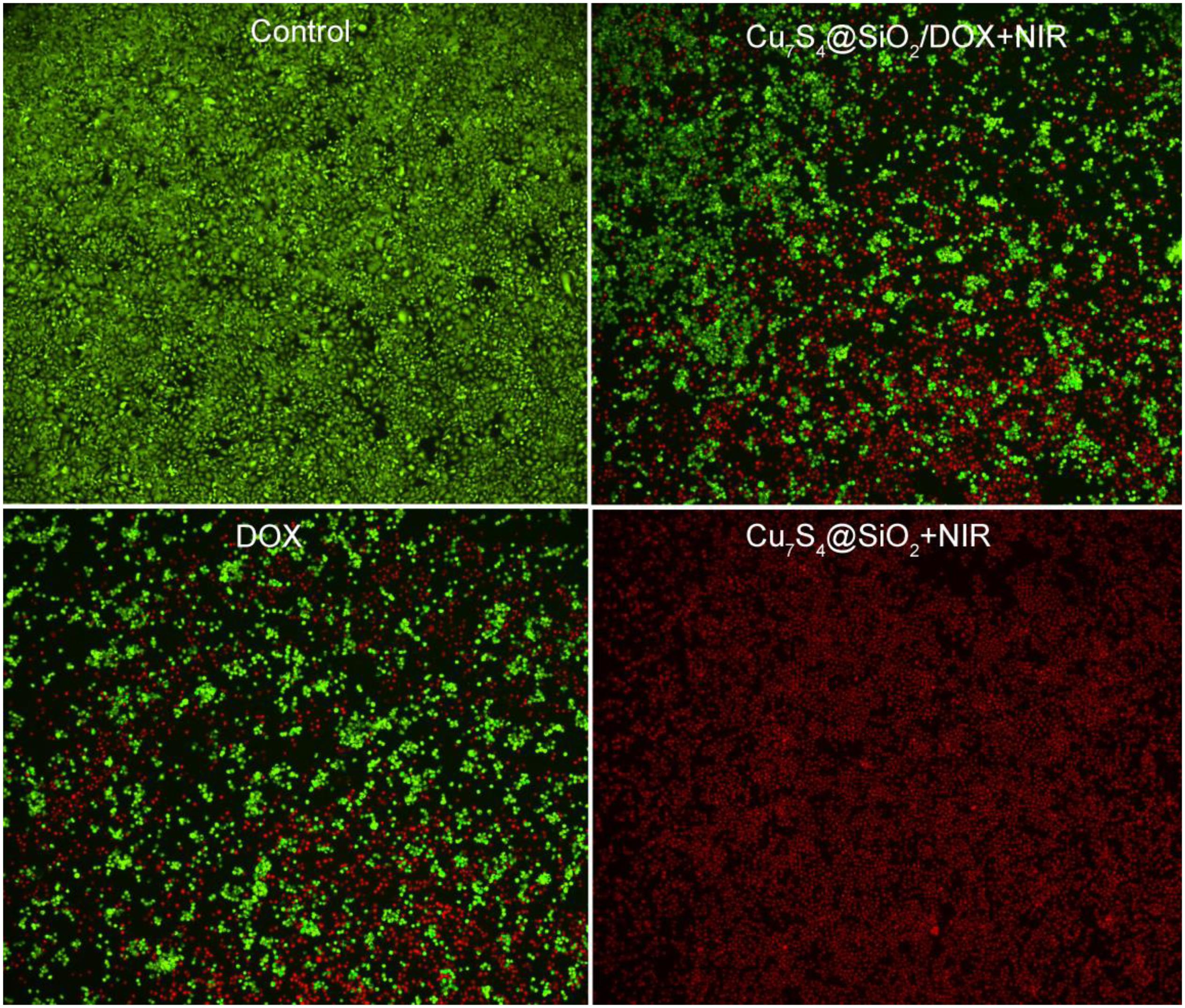
Figure 5. Confocal images of calcein AM (green, live cells) and propidium iodide (red, dead cells) co-stained cells after incubation with Cu7S4@SiO2/DOX for different treatments. Magnification: 100 times.
Before realizing bioapplication, the Cu2+ release of Cu7S4@SiO2 nanoparticles in PBS was evaluated because of Cu2+ being toxic. It was revealed that the concentration of the released Cu2+ from Cu7S4@SiO2 nanoparticles was very low (Supplementary Figure S5), which caused almost no toxicity. We then evaluated the toxicity in vivo of Cu7S4@SiO2. In the experimental group, the material was injected intravenously into mice, while mice in the control group were injected with PBS. After a month, HE analysis of the main organs of mice in the two groups showed no significant difference (Supplementary Figure S6). To perform photothermal-chemo therapy of tumors, mice bearing with melanoma tumor were randomly allocated into four groups as follow: (1) The control group; (2) DOX group; (3) Cu7S4@SiO2+NIR group; (4) Cu7S4@SiO2/DOX+NIR group. The mice in (2) group were intratumorally injected with DOX PBS solution (50 μL, 80 μg), and mice in (3 and 4) group were intratumorally injected with Cu7S4@SiO2 (50 μL, 0.1 mg mL–1) and Cu7S4@SiO2/DOX PBS solution (50 μL, 0.1 mg mL–1). Figure 6a shows the typical thermal image of mice with the tumor area exposed to a 1064 nm NIR laser (0.6 Wcm–2), in which the tumor treated with Cu7S4@SiO2/DOX shows the bright red while the tumor received PBS exhibits the normal color. The surface temperature of tumor treated with Cu7S4@SiO2/DOX increases from ∼34.2°C to the balanced ∼57.9 °C at 300 s, resulting in temperature elevation of 23.7°C which was much higher than that (3.5°C) for the tumor received PBS (Figure 6b). Thus, the Cu7S4@SiO2/DOX within tumor remain high photothermal conversion effect, which can convert 1064 nm NIR laser energy into enough heat to thermally ablate cancer cells.
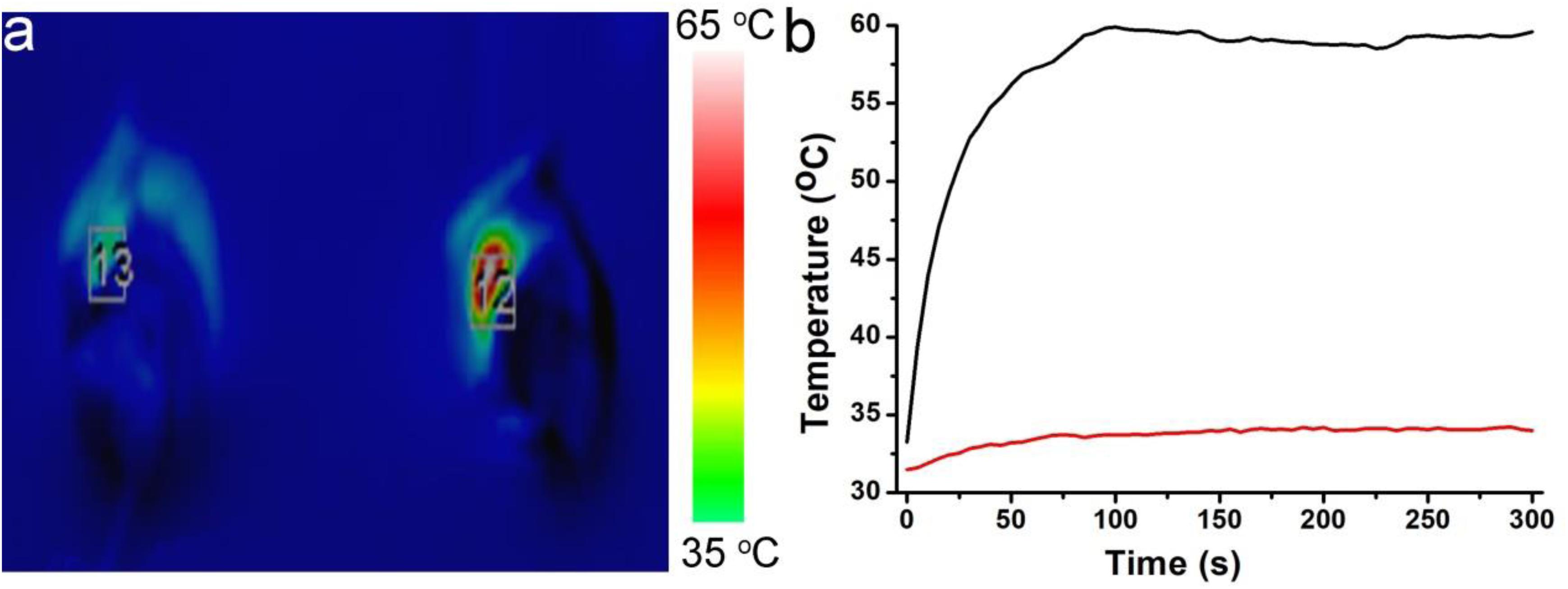
Figure 6. (a) The thermal image of mice after intratumorally injecting with Cu7S4@SiO2/DOX PBS solution (right) or PBS solution (left) under the irradiation of 1064 nm NIR laser at the power density of 0.6 Wcm– 2. (b) The temperature curves showing the temperature change in tumor area.
After different treatments, mice in all groups were raised under the standard condition for the long-term observation of cancer treatment efficacy. The tumor sizes and body weights were recorded. The change of relative tumor volumes is demonstrated in Figure 7A. Obviously, the tumor volume in the control group increases greatly which was five times the initial volume. For the tumors in the DOX group and Cu7S4@SiO2+NIR group, their growth has been inhibited, due to the cytotoxicity of DOX for DOX group and the photothermal therapy for Cu7S4@SiO2+NIR group. Interestingly, in the case of the tumors in the Cu7S4@SiO2/DOX+NIR group, their volume goes down continuously with the significant inhibition efficiency compared to the other three groups. The high inhibition efficiency should be due to the synergistic photothermal-chemo effect from the combination of Cu7S4@SiO2/DOX and NIR laser irradiation. On the one side, the photothermal therapy can instantly kill part of cancer cells through high temperature, and on the other side, the load DOX within Cu7S4@SiO2/DOX can continuous release to kill the remaining cancer cells, thus achieving the synergistic effect on melanoma tumors. In addition, there was no any sign of loss in body weight for all groups (Figure 7B). Therefore, the satisfactory inhibition efficiency can be realized from the synergistic photothermal-chemo therapy by the combination of Cu7S4@SiO2/DOX and 1064 nm NIR laser, while no obvious side effect for mice.
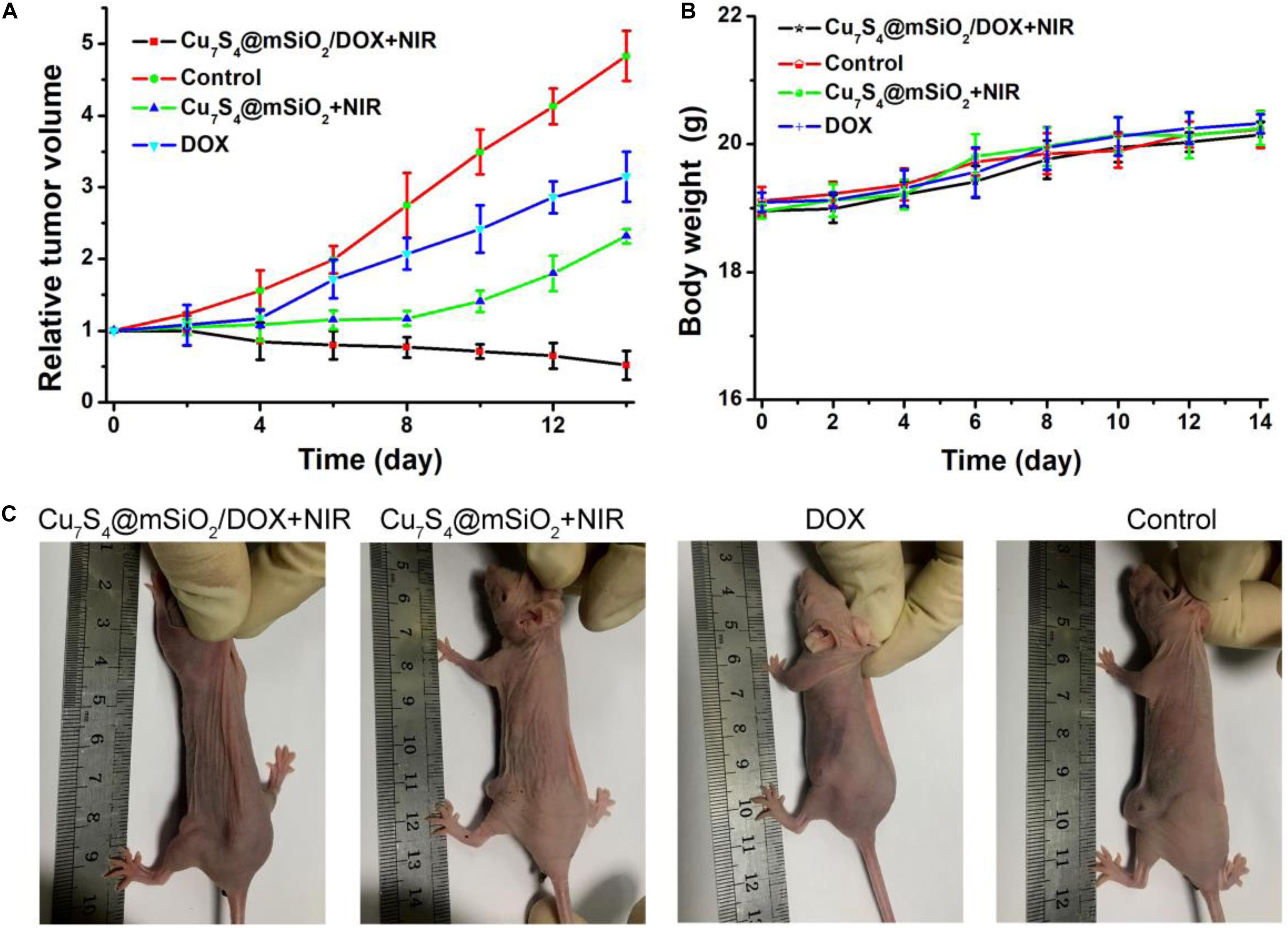
Figure 7. (A) The relative tumor volumes of mice after different treatments over a period of 14 days. (B) The body weight of mice in these groups. (C) The typical photographs of mice at the 14th day.
Furthermore, mice in all groups were sacrificed when a tumor size was beyond 1.0 cm (Figure 7C). The tumors were extracted for the sacrificed mice, which were embedded in paraffin and crysectioned into slices. After stained with H&E assay, the tumor slices were imaged for histological examination. Figure 8 manifests the typical morphology of tumor cells, and the cancer cells shows the normal and complete morphology in regard to the cell size, shape and nuclear. The significant cell damage is noticed for Cu7S4@SiO2/DOX+NIR group (Figure 8a), including the destroyed cell membranes and the condensed nucleus. For the tumor cells in the Cu7S4@SiO2+NIR group (Figure 8b) and DOX group (Figure 8c), there are some cells showing the destroyed morphology, indicating the limited therapeutical effect through photothermal therapy or chemotherapy. On the contrary, the cell size and shape showed no difference in the control group (Figure 8d). The above histological examination solidly verifies the higher therapeutical effect of photothermal-chemo therapy than photothermal therapy or chemotherapy.
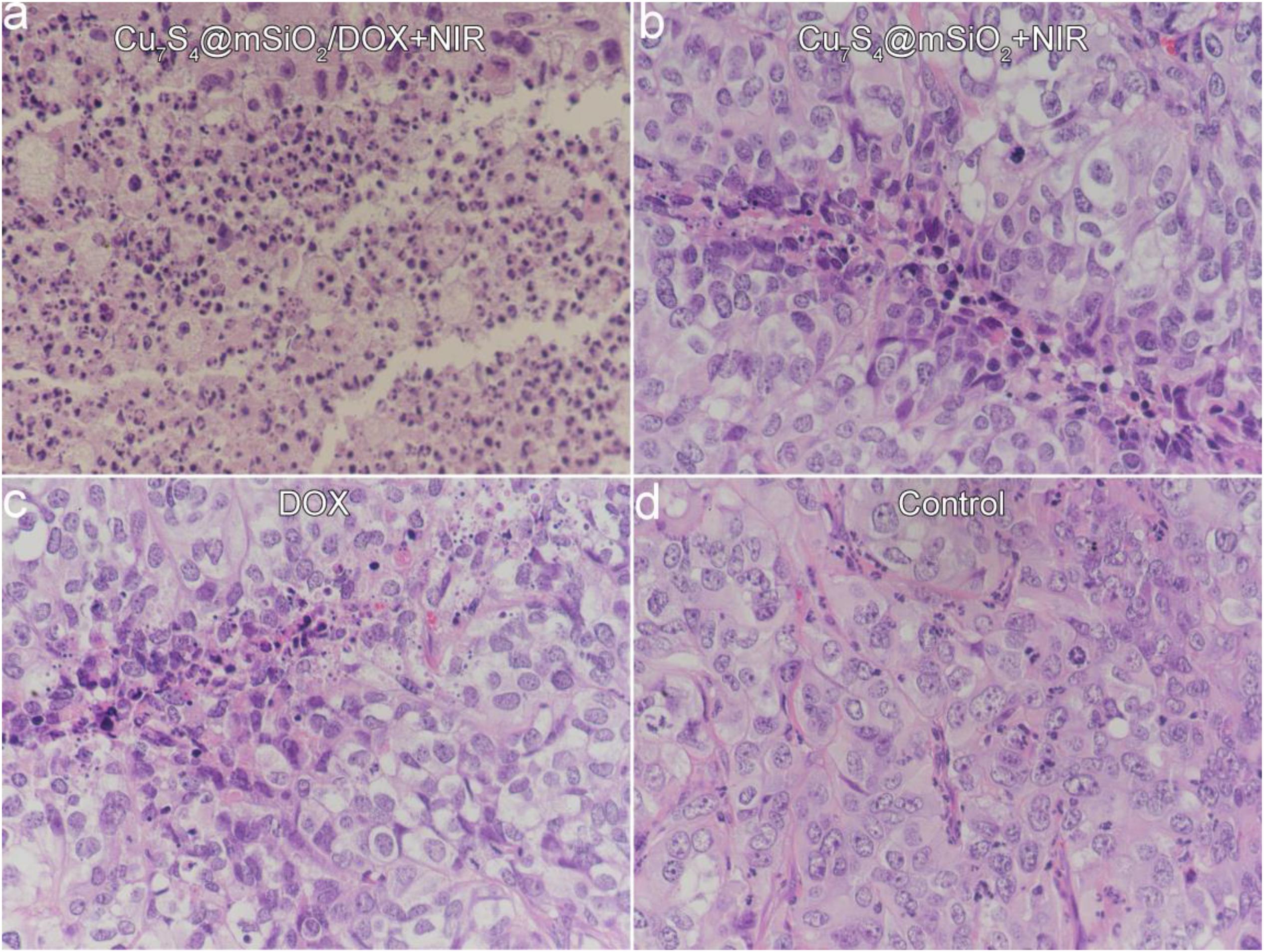
Figure 8. The typical images of tumor slices stained with H&E assay for (a) Cu7S4@SiO2/DOX+NIR group, (b) Cu7S4@SiO2+NIR group, (c) DOX group, and (d) Control group.
Conclusion
In summary, we prepared the Cu7S4@SiO2/DOX and used them as efficient nanoplatforms for synergistic photothermal-chemo therapy on melanoma tumors. The Cu7S4@SiO2/DOX was prepared by firstly synthesizing Cu7S4 nanocrystals, then in situ growing SiO2 shell on the surface of Cu7S4 nanocrystals, and finally loading DOX. The Cu7S4@SiO2 consisted of Cu7S4 core with the average diameter of 50 nm and SiO2 shell with the average thickness of 25 nm. The Cu7S4@SiO2 nanoplatforms exhibited the strong and broad NIR absorption and rapidly converted 1064 nm laser energy into heat, and they also demonstrated high specific surface area and a large amount of pores with high DOX-loading content of 59.8%. Importantly, under the irradiation of 1064 nm laser, Cu7S4@SiO2/DOX simultaneous generated heat and accelerated the DOX release. Give these advantages, mice were intratumorally injected with Cu7S4@SiO2/DOX and irradiated with1064 nm laser, which achieved the highest therapeutical effect through synergistic photothermal-chemo therapy compared to photothermal therapy or chemotherapy alone. Therefore, the Cu7S4@SiO2/DOX can be served as novel and efficient photothermal-chemo nanoagents for efficient tumor therapy.
Data Availability Statement
All datasets presented in this study are included in the article/Supplementary Material.
Ethics Statement
The animal study was reviewed and approved by the Ninth People’s Hospital, Shanghai Jiao Tong University School of Medicine.
Author Contributions
LZ and HP contributed equally to this work. LZ, HP, and XH designed the project and wrote the manuscript. LZ, HP, and YL carried out the experiment. LZ and FL performed the experimental data analysis. All the authors contributed to discussion of the results.
Funding
This work was supported by grants from the National Natural Science Foundation of China (81402258, 81702781, 81972524, and 81602366) and the Science and Technology Commission of Shanghai (17DZ2260100).
Conflict of Interest
The authors declare that the research was conducted in the absence of any commercial or financial relationships that could be construed as a potential conflict of interest.
The reviewer XH declared a shared affiliation, with no collaboration, with the authors to the handling editor.
Supplementary Material
The Supplementary Material for this article can be found online at: https://www.frontiersin.org/articles/10.3389/fbioe.2020.579439/full#supplementary-material
References
Cheng, L., Wang, C., Feng, L., Yang, K., and Liu, Z. (2014). Functional nanomaterials for phototherapies of cancer. Chem. Rev. 114, 10869–10939. doi: 10.1021/cr400532z
Dreaden, E. C., Mackey, M. A., Huang, X. H., Kang, B., and El-Sayed, M. A. (2011). Beating cancer in multiple ways using nanogold. Chem. Soc. Rev. 40, 3391–3404. doi: 10.1039/c0cs00180e
Huang, X., Zhang, W., Guan, G., Song, G., Zou, R., and Hu, J. (2017). Design and functionalization of the nir-responsive photothermal semiconductor nanomaterials for cancer theranostics. Acc. Chem. Res. 50, 2529–2538. doi: 10.1021/acs.accounts.7b00294
Huang, X. Q., Tang, S. H., Mu, X. L., Dai, Y., Chen, G. X., Zhou, Z. Y., et al. (2011). Freestanding palladium nanosheets with plasmonic and catalytic properties. Nat. Nanotechnol. 6, 28–32. doi: 10.1038/nnano.2010.235
Li, B., Wang, Q., Zou, R., Liu, X., Xu, K., Li, W., et al. (2014). Cu7.2S4 nanocrystals: a novel photothermal agent with a 56.7% photothermal conversion efficiency for photothermal therapy of cancer cells. Nanoscale 6, 3274–3282. doi: 10.1039/c3nr06242b
Li, B., Ye, K., Zhang, Y., Qin, J., Zou, R., Xu, K., et al. (2015). Photothermal theragnosis synergistic therapy based on bimetal sulphide nanocrystals rather than nanocomposites. Adv. Mater. 27, 1339–1345. doi: 10.1002/adma.201404257
Li, Z. L., Hu, Y., Chan, M. L., Howard, K. A., Fan, X. L., Sun, Y., et al. (2016). Highly porous PEGylated Bi2S3 nano-urchins as a versatile platform for in vivo triple-modal imaging, photothermal therapy and drug delivery. Nanoscale 8, 16005–16016. doi: 10.1039/c6nr03398a
Liu, J. C., Wu, G. F., Tang, Z. Y., Sun, Q., Wu, J. X., and Lv, R. F. (2019). Cobalt/manganese oxides as theragnosis nanoplatforms with magnetic resonance/Near-infrared imaging for efficient photothermal therapy of tumors. Front. Mater. 6:286. doi: 10.3389/Fmats.2019.00286
Liu, T., Wang, C., Gu, X., Gong, H., Cheng, L., Shi, X. Z., et al. (2014). Drug delivery with PEGylated MoS2 nano-sheets for combined photothermal and chemotherapy of cancer. Adv. Mater. 26, 3433–3440. doi: 10.1002/adma.201305256
Liu, Y. L., Ai, K. L., Liu, J. H., Deng, M., He, Y. Y., and Lu, L. H. (2013). Dopamine-melanin colloidal nanospheres: an efficient near-infrared photothermal therapeutic agent for in vivo cancer therapy. Adv. Mater. 25, 1353–1359. doi: 10.1002/adma.201204683
Luther, J. M., Jain, P. K., Ewers, T., and Alivisatos, A. P. (2011). Localized surface plasmon resonances arising from free carriers in doped quantum dots. Nat. Mater. 10, 361–366. doi: 10.1038/nmat3004
Meng, Z., Wei, F., Wang, R., Xia, M., Chen, Z., Wang, H., et al. (2016). NIR-laser-switched in vivo smart nanocapsules for synergic photothermal and chemotherapy of tumors. Adv. Mater. 28, 245–253. doi: 10.1002/adma.201502669
Ou, G., Li, Z., Li, D., Cheng, L., Liu, Z., and Wu, H. (2016). Photothermal therapy by using titanium oxide nanoparticles. Nano. Res. 9, 1236–1243. doi: 10.1007/s12274-016-1019-8
Ren, W. Z., Yan, Y., Zeng, L. Y., Shi, Z. Z., Gong, A., Schaaf, P., et al. (2015). A near infrared light triggered hydrogenated black TiO2 for cancer photothermal therapy. Adv. Healthc. Mater. 4, 1526–1536. doi: 10.1002/adhm.201500273
Song, G., Wang, Q., Wang, Y., Lv, G., Li, C., Zou, R., et al. (2013). A low-toxic multifunctional nanoplatform based on Cu9S5@mSiO2 core-shell nanocomposites: combining photothermal- and chemotherapies with infrared thermal imaging for cancer treatment. Adv. Funct. Mater. 23, 4281–4292. doi: 10.1002/adfm.201370178
Tian, Q. W., Jiang, F. R., Zou, R. J., Liu, Q., Chen, Z. G., Zhu, M. F., et al. (2011a). Hydrophilic Cu9S5 nanocrystals: a photothermal agent with a 25.7% heat conversion efficiency for photothermal ablation of cancer cells in vivo. ACS Nano 5, 9761–9771. doi: 10.1021/nn203293t
Tian, Q. W., Tang, M. H., Sun, Y. G., Zou, R. J., Chen, Z. G., Zhu, M. F., et al. (2011b). Hydrophilic flower-like CuS superstructures as an efficient 980 nm laser-driven photothermal agent for ablation of cancer cells. Adv. Mater. 23, 3542–3547. doi: 10.1002/adma.201101295
Tsai, M. F., Chang, S. H. G., Cheng, F. Y., Shanmugam, V., Cheng, Y. S., Su, C. H., et al. (2013). Au nanorod design as light-absorber in the first and second biological near-infrared windows for in vivo photothermal therapy. ACS Nano 7, 5330–5342. doi: 10.1021/nn401187c
Vankayala, R., and Hwang, K. C. (2018). Near-Infrared-light-activatable nanomaterial-mediated phototheranostic nanomedicines: an emerging paradigm for cancer treatment. Adv. Mater. 30:1706320. doi: 10.1002/adma.201706320
Wang, D., Dong, H., Li, M., Cao, Y., Yang, F., Zhang, K., et al. (2018). Erythrocyte-cancer hybrid membrane camouflaged hollow copper sulfide nanoparticles for prolonged circulation life and homotypic-targeting photothermal/chemotherapy of melanoma. ACS Nano 12, 5241–5252. doi: 10.1021/acsnano.7b08355
Wang, Y., Wang, K. Y., Zhao, J. F., Liu, X. G., Bu, J., Yan, X. Y., et al. (2013). Multifunctional mesoporous silica-coated graphene nanosheet used for chemo-photothermal synergistic targeted therapy of glioma. J. Am. Chem. Soc. 135, 4799–4804. doi: 10.1021/ja312221g
Wen, L., Chen, L., Zheng, S., Zeng, J., Duan, G., Wang, Y., et al. (2016). Ultrasmall biocompatible WO3-x nanodots for multi-modality imaging and combined therapy of cancers. Adv. Mater. 28, 5072–5079. doi: 10.1002/adma.201506428
Xu, W., Meng, Z., Yu, N., Chen, Z., Sun, B., Jiang, X., et al. (2015). PEGylated CsxWO3 nanorods as an efficient and stable 915 nm-laser-driven photothermal agent against cancer cells. RSC Adv. 5, 7074–7082. doi: 10.1039/C4RA15524F
You, J., Zhang, R., Zhang, G. D., Zhong, M., Liu, Y., Van Pelt, C. S., et al. (2012). Photothermal-chemotherapy with doxorubicin-loaded hollow gold nanospheres: a platform for near-infrared light-trigged drug release. J. Controll. Release 158, 319–328. doi: 10.1016/j.jconrel.2011.10.028
Yu, N., Hu, Y., Wang, X., Liu, G., Wang, Z., Liu, Z., et al. (2017). Dynamically tuning near-infrared-induced photothermal performances of TiO2 nanocrystals by Nb doping for imaging-guided photothermal therapy of tumors. Nanoscale 9, 9148–9159. doi: 10.1039/c7nr02180a
Yu, N., Li, J., Wang, Z., Yang, S., Liu, Z., Wang, Y., et al. (2018a). Blue Te nanoneedles with strong nir photothermal and laser-enhanced anticancer effects as “all-in-one” nanoagents for synergistic thermo-chemotherapy of tumors. Adv. Healthc. Mater. 7:1800643. doi: 10.1002/adhm.201800643
Yu, N., Wang, Z., Zhang, J., Liu, Z., Zhu, B., Yu, J., et al. (2018b). Thiol-capped Bi nanoparticles as stable and all-in-one type theranostic nanoagents for tumor imaging and thermoradiotherapy. Biomaterials 161, 279–291. doi: 10.1016/j.biomaterials.2018.01.047
Zha, Z., Yue, X., Ren, Q., and Dai, Z. (2013). Uniform polypyrrole nanoparticles with high photothermal conversion efficiency for photothermal ablation of cancer cells. Adv. Mater. 25, 777–782. doi: 10.1002/adma.201202211
Zhang, K., Meng, X., Cao, Y., Yang, Z., Dong, H., Zhang, Y., et al. (2018). Metal-organic framework nanoshuttle for synergistic photodynamic and low-temperature photothermal therapy. Adv. Funct. Mater. 28:1804634. doi: 10.1002/adfm.201804634
Zhang, M. Y., Liu, X. J., Luo, Q., Wang, Q., Zhao, L. J., Deng, G. Y., et al. (2020). Tumor environment responsive degradable CuS@mSiO2@MnO2/DOX for MRI guided synergistic chemo-photothermal therapy and chemodynamic therapy. Chem. Eng. J. 389:124450. doi: 10.1016/J.Cej.2020.124450
Zhao, Y., Pan, H., Lou, Y., Qiu, X., Zhu, J., and Burda, C. (2009). Plasmonic Cu2-xS nanocrystals: optical and structural properties of copper-deficient copper(I) sulfides. J. Am. Chem. Soc. 131, 4253–4261. doi: 10.1021/ja805655b
Zheng, T. T., Li, G. G., Zhou, F., Wu, R., Zhu, J. J., and Wang, H. (2016). Gold-nanosponge-based multistimuli-responsive drug vehicles for targeted chemo-photothermal therapy. Adv. Mater. 28, 8218–8226. doi: 10.1002/adma.201602486
Keywords: Cu2–xS nanocrystals, mesoporous SiO2, photothermal therapy, chemotherapy, melanoma
Citation: Zhang L, Pan H, Li Y, Li F and Huang X (2020) Constructing Cu7S4@SiO2/DOX Multifunctional Nanoplatforms for Synergistic Photothermal–Chemotherapy on Melanoma Tumors. Front. Bioeng. Biotechnol. 8:579439. doi: 10.3389/fbioe.2020.579439
Received: 02 July 2020; Accepted: 24 August 2020;
Published: 15 September 2020.
Edited by:
Guanjie He, University of Lincoln, United KingdomReviewed by:
Xiaojuan Huang, Shanghai Jiao Tong University, ChinaKaibing Xu, Donghua University, China
Jichun Liu, Jianghuai Military Region Rear Hospital, China
Copyright © 2020 Zhang, Pan, Li, Li and Huang. This is an open-access article distributed under the terms of the Creative Commons Attribution License (CC BY). The use, distribution or reproduction in other forums is permitted, provided the original author(s) and the copyright owner(s) are credited and that the original publication in this journal is cited, in accordance with accepted academic practice. No use, distribution or reproduction is permitted which does not comply with these terms.
*Correspondence: Xiaolin Huang, ZHJtYXVyZWVuaHVhbmdAMTYzLmNvbQ==