- 1Center of Nanotechnology, King Abdulaziz University, Jeddah, Saudi Arabia
- 2Department of Biochemistry, Faculty of Science, King Abdulaziz University, Jeddah, Saudi Arabia
- 3Department of Physics, Faculty of Science, King Abdulaziz University, Jeddah, Saudi Arabia
- 4Department of Medical Laboratory Technology, Faculty of Applied Medical Sciences, King Abdulaziz University, Jeddah, Saudi Arabia
- 5Department of Electrical and Computer Engineering, Faculty of Engineering, King Abdulaziz University, Jeddah, Saudi Arabia
Three-dimensional (3D) printing has experienced a steady increase in popularity for direct manufacturing, where complex geometric items can be produced without the aid of templating tools, and manufacturing waste can be remarkably reduced. While customized medical devices and daily life items can be made by 3D printing of thermoplastics, microbial contamination has been a serious obstacle during their usage. A very clever approaches to overcome this challenge is to incorporate antimicrobial metal or metal oxide (M/MO) nanoparticles within the thermoplastics during or prior to 3D printing. Many M/MO nanoparticles can prevent contamination from a wide range of microorganism, including antibiotic-resistant bacteria via various antimicrobial mechanisms. Additionally, they can be easily printed with thermoplastic without losing their integrity and functionality. In this mini review, we summarize recent advancements and discuss future trends related to the development of 3D printed antimicrobial thermoplastic nanocomposites by addition of M/MO nanoparticles.
Introduction
Three-dimensional (3D) printing has garnered great interest among not only manufacturers for quick prototyping but also amongst the general public (MacDonald and Wicker, 2016). According to Wohlers Report 2020, the size of the global 3D printing market is $13.7 billion, exhibiting 25% annual growth since 2014 (Wohlers Talk, 2020). The technology allows for end-use products with complex shapes to be precisely fabricated by a layered additive approach without templating tools such as molds (MacDonald and Wicker, 2016; Valino et al., 2019). Accordingly, customized production using this technique can remarkably reduce the cost for low-volume manufacturing and even provide opportunities for customers to make their own “mini factory” (Gibson et al., 2014; Memic et al., 2017; Poelma and Rolland, 2017). Moreover, traditional subtractive manufacturing causes massive materials waste, which can be reduced by as much as 90% when 3D printing is utilized (Valino et al., 2019). Due to these advantages, 3D printing has become a promising alternative for producing aerospace, automotive, healthcare and consumer devices (MacDonald and Wicker, 2016; Martin et al., 2017; Gerdes et al., 2020). However, current 3D printing potential is hampered by limited material choices that meet required performance specifications for a particular application (MacDonald and Wicker, 2016). Therefore, developing multifunctional advanced materials that can easily satisfy end-use needs has become one of the central foci for researchers in this field.
Thermoplastics can be inexpensive, easy to process, chemically stable, lightweight, and flexible materials making them very attractive for 3D printing applications (Poelma and Rolland, 2017; Blok et al., 2018). Many personal and medical devices are mainly made of thermoplastics (Blok et al., 2018; Valino et al., 2019), making them the main material source for customer-level desktop printing systems. Furthermore, they are easily used in 3D printing applications, and are printable via different approaches such as selective laser sintering (SLS), stereolithography (SLA) and fused deposition modeling (FDM). Among them, FDM is one of the most popular and cost-effective printing techniques and is almost unique for printing of thermoplastics (Valino et al., 2019). However, microbial contamination still represent a challenge for biomedical applications of 3D printing, especially for handheld and medical devices (González-Henríquez et al., 2019a; Memic et al., 2019). In general, contamination associated with medical devices has been a serious issue in clinical treatment. Bacterial contamination can pose a critical threat to patients and considerably increase the healthcare cost due to need for reoperation and/or replacement of infected devices (Vasilev et al., 2009; Tamayo et al., 2016). For example, urinary catheters, indwelling vascular catheters and mechanical ventilation are responsible for 95% of urinary tract infections, 87% of bloodstream infections and 86% of pneumonia cases, respectively (Richards et al., 1999; Weinstein and Darouiche, 2001). Accumulation of bacteria on polymeric substrates could cause the formation of biofilms in which bacteria are 1,000 to 10,000 times more resistant to antibiotics than those not in a biofilm (Vasilev et al., 2009; Gnanadhas et al., 2015). Thus, it is vital to develop 3D printable antimicrobial thermoplastic materials to minimize risk of infection during their usage.
The current standard to fight infections, namely the usage of antibiotics, is facing a challenge attributed to ongoing mutation of bacterial microorganisms. As mutations are constantly occurring, so is the development of resistance to antibiotics (Gold et al., 2018). Alternatively, integration of metals and metal oxides (M/MO) within thermoplastics is one of the most promising approaches to design flexible plastic devices with antimicrobial properties (Emamifar, 2011; Tamayo et al., 2016). Most of the essential metals such as copper, zinc, magnesium and their oxides have strong biocidal effects, while other non-essential M/MO including silver, gold and cerium oxide are popular antibacterial agents (Al-Shawafi et al., 2017; Gold et al., 2018). Recent advancements in nanotechnology have enabled the synthesis of M/MO in the nano-size range, which greatly enhance their antimicrobial performance (Martinez-Gutierrez et al., 2010; Gold et al., 2018). Low concentrations, ranging from 0.1 to 4% of M/MO nanoparticles added in the polymer matrix can be functionally sufficient for prevention of most infections; a concentration often compatible with mammalian cells with negligible toxicity (Martinez-Gutierrez et al., 2010; Diez-Pascual and Diez-Vicente, 2017). Additionally M/MO nanoparticles inhibit or stunt bacterial growth via various mechanisms dissimilar to antibiotics, which would require a specific type of bacteria to undergo multiple gene mutations to generate any kind of resistance, if any at all (Gold et al., 2018). Furthermore, M/MO do not chemically deteriorate or thermally degrade in the temperature range of 3D printing of thermoplastics (Rhim et al., 2013; Palza, 2015). To best of our knowledge, this is one of the first reviews to summarize the progress made in this sub-field.
In this mini review, we will summarize the latest progress, advances and trends related to M/MO induced thermoplastic composites fabricated using 3D printing. First, we will discuss general aspects of the antimicrobial mechanism of thermoplastic nanocomposites. Next, we will describe the incorporation of nanoparticles in 3D printing and discuss key factors affecting antimicrobial activity of thermoplastic composites. Finally, we will highlight the impact of 3D printed antimicrobial thermoplastics in different applications and give insights for future developments. The overall purpose of this mini review is to highlight the impact of antimicrobial thermoplastic composites on progressive application of 3D printing.
Antimicrobial Mechanism of the Nanocomposites
Antimicrobial activity of metals such as silver and copper has been observed since ancient times, as they were used for water sterilization and wound healing (Palza, 2015; Tamayo et al., 2016). However, the broad potential of M/MO antimicrobial agents has more recently been recognized due to the advancements in nanotechnology (Hasan et al., 2018). Metals and their oxides can form nanoparticles through numerous top-down and bottom-top synthesis approaches including sol-gel, co-precipitation, electrochemical, green synthesis, microwave, and sonochemical amongst other approaches (Salah et al., 2019). M/MO nanoparticles exhibit significantly superior antibacterial performance compared to the micro/macro particles or the bulk surface, often correlated with their smaller size and their high surface area that aid in their bioactivity and mechanism of action discussed below (Gold et al., 2018). Additionally, M/MO nanoparticles have advantages including the prevention of biofilm formation by inhibiting planktonic growth, altering lipids and proteins, damaging DNA, and interfering with enzyme activities of bacteria (Gold et al., 2018). Hence, antibiotic-resistant bacteria could be effectively inhibited by M/MO nanoparticles, as they exert antimicrobial activities via other mechanisms different from ones exerted by antibiotics (Raghunath and Perumal, 2017). However, over-dosage and/or an immediate release of the nanoparticles could cause injuries to certain eukaryotic cells (Gold et al., 2018).
Incorporation of M/MO nanoparticles into polymers could improve overall antibacterial efficacy of the nanocomposite, due to the synergetic polymer and nanoparticle effects (Tamayo et al., 2016). In most M/MO thermoplastic nanocomposites, the antimicrobial mechanism is mainly associated with toxicity of M/MO nanoparticles (Sánchez-López et al., 2020). On the other hand, polymer matrix as a support regulates the release behavior of the nanoparticles and ions as a response to bacterial adhesion on the composite surface (Supplementary Figure S1). Namely, water molecules could diffuse from the bacterial medium into the polymer matrix, and cause release of metal ions. Although antimicrobial mechanisms of M/MO are still a subject of meticulous research, owing to the continuous mutation of bacteria, there are three major mechanisms (Gold et al., 2018).
Metal Ion Limitation
Metal and metal oxide nanoparticles can inhibit bacterial growth by attacking the electronegative phospholipid bilayers of the bacterial cell wall with electropositive metal ions (Chandrangsu et al., 2017; Raghunath and Perumal, 2017). They release cations to the anionic sites of the cell membrane and neutralize charges within the cell via electrostatic interaction. This charge difference favors accumulation of metallic ions, which in turn permeates the cell membrane and recruit intrinsic metals to be adsorbed into it (Raghunath and Perumal, 2017). In response, the homeostatic system in the cell releases more ions from its storage to make up for the loss. This cycle continues until the cell is starved of its essential metals and metal-dependent metabolic processes come to a halt (Gold et al., 2018). This antimicrobial mechanism has been demonstrated in iron oxide and zinc-based nanoparticles (Raghunath and Perumal, 2017).
Biomolecular Interaction
Another bactericidal mechanism of M/MO nanoparticles is through interaction with DNA and cytosolic enzymes of various microorganism (Raghunath and Perumal, 2017). Metals and their ions could crosslink DNA and disrupt its helical structure halting bacterial proliferation. Furthermore, metals and their ions could directly bind to enzymes and disrupt their tertiary structures, which in turn disrupt digestion and respiration processes (Imlay, 2014). As a result, bacterial cells start to lose metabolic activity and die. Metal ions like copper (I), zinc (II), manganese (II), and iron (II) have been reported to exhibit this antibacterial mechanism (Skaar and Raffatellu, 2015).
Reactive Oxygen Species (ROS) Formation
Many transitional metals and their oxides have strong redox properties allowing them to gain electrons from reactive donor sites (Raghunath and Perumal, 2017). Hence the intermolecular interaction between M/MO nanoparticles and the bacterial cells initiates the formation of many ROS such as hydroxyl radicals (OH•), superoxide anion (O–2) and hydrogen peroxide (H2O2). Further propogation of ROS via different reactions increase their concentrations, causing oxidative stress (Nel et al., 2006). This stress mediates the damage of cell macromolecules, distortion of proteins and lipids, alteration of DNA/RNA, inhibition of enzymes activities, and eventually death of the microbe (Gold et al., 2018). For example, silver, gold (Zheng et al., 2017), zinc oxide (He et al., 2011) and magnesium oxide have been reported to prompt ROS formation (Gold et al., 2018).
3D Printing of Thermoplastic Nanocomposites
In general, 3D printing is a process to build a structure by adding materials layer-by-layer according to a computer aided design (CAD) model (Jiménez et al., 2019). Initially a CAD model can be created by various 3D design software and stored in a format adapted for 3D printing (Valino et al., 2019). Afterwards, the designed model is transferred to slicer programs to set the printing options such as the thickness of the model, the height of the layers, filling ratio and printing speed, which can be saved as a G-code file (Jiménez et al., 2019). Finally, various types of 3D printers use the G-code file to build the models to be ready for post-manufacturing removal of supports, sanding and filing processes (Guo et al., 2019).
Fused deposition modeling (FDM), one of the most common 3D printing techniques, applies thermoplastic based polymers and nanocomposites (Figure 1). In this technique, filaments are heat-melted and extruded from a nozzle and allowed to solidify in a printing bed. The hot nozzle commonly moves in X and Y direction to build the first layer, while succeeding layers are continuously being built by moving the nozzle up in the Z direction (Ngo et al., 2018; Jiménez et al., 2019). Addition of M/MO nanoparticles is usually achieved during formation of filaments prior to 3D printing. Melt-blending, solvent-casting and surface coating (Supplementary Figure S2) are few of the approaches to mix the nanoparticles with thermoplastics (González-Henríquez et al., 2019b).
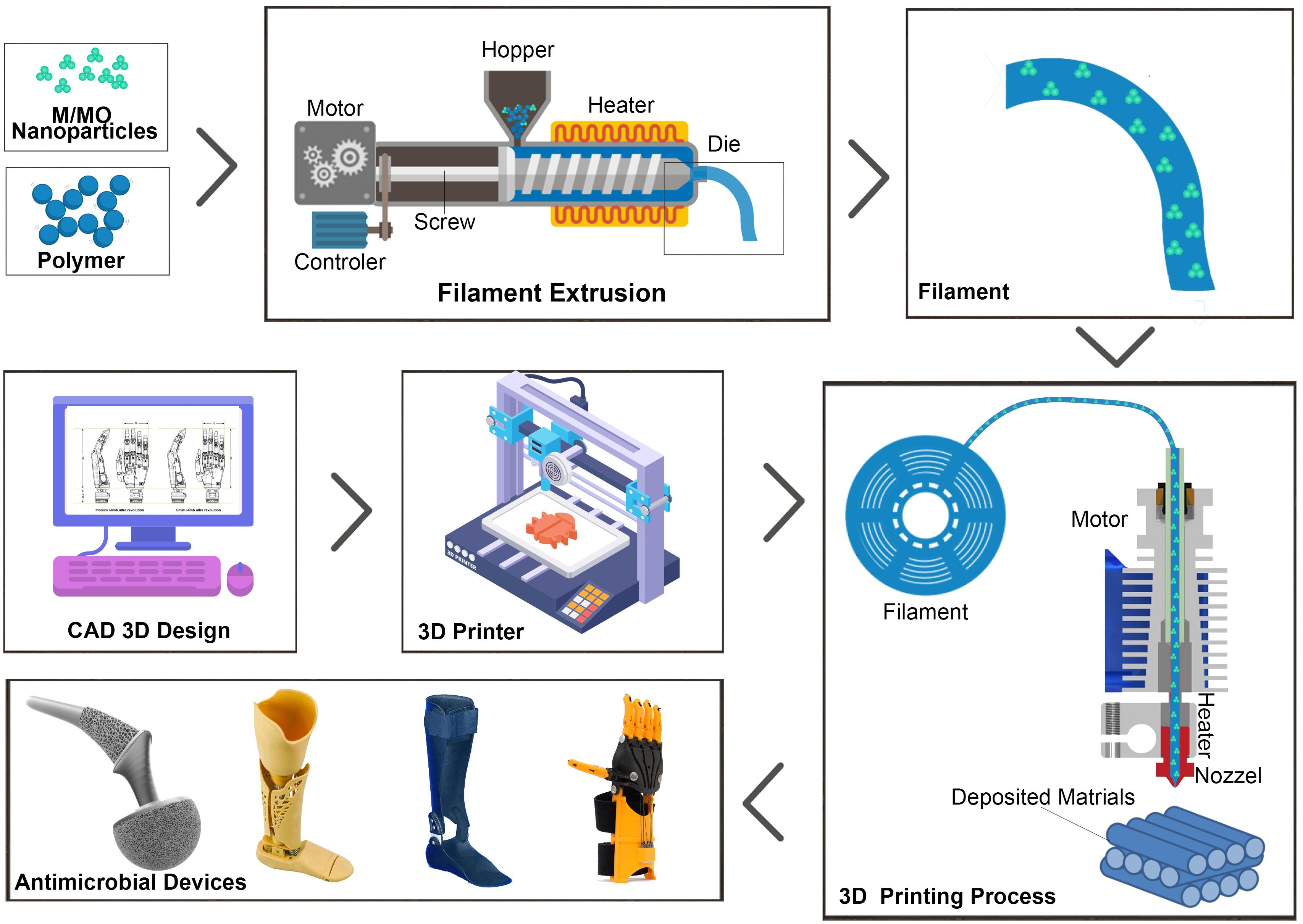
Figure 1. Schematic illustration of 3D printing of M/MO incorporated thermoplastic nanocomposites using FDM process. Composite filaments can be made by extruding mixture of the M/MO nanoparticles with the thermoplastics. Then filaments are heat-melted and extruded from a nozzle and allowed to solidify in a printing bed. Pre-programmed computer aided design (CAD) model is used to control the printing process.
Another approach to print thermoplastic nanocomposites is SLS, in which a laser selectively sinters the particles of a polymer powder and fuses them together, building the intended part layer-by-layer (Mazzoli, 2013). The process starts with the powder and the build area being heated to just near the melting temperature of the polymer. Then, a recoating blade spreads a thin layer of powder over the build platform (Paul and Anand, 2012). Next, a laser fuses powder polymers together. After scanning each cross-section of the component, the power bed is lowered down to the next layer, and another layer is built on top. This process is repeated until the intended model is completed (Paul and Anand, 2012; Valino et al., 2019). For this approach, addition of powder M/MO nanoparticles with the polymer powder is done by mechanical mixers such as rotary tumblers (Turner et al., 2020). The mixing process is easier and can result in a more uniform distribution of nanoparticles when compared to FDM. Yet another method utilized to create antibacterial thermoplastic nanocomposite 3D printed models is SLA. In this method, M/MO nanoparticles are first dispersed into the monomer solution prior to its polymerization (Totu et al., 2017). The resin is then deposited layer-by-layer based on the designed model. In final step the polymerization is effectuated by UV or other light sources (Weng et al., 2016). In addition, liquid deposition molding (LDM), solvent-cast 3D printing (SCP) and melt electrowriting (MEW) can also be used to print the thermoplastic nanocomposites (Hewitt et al., 2019; Nonato et al., 2019). However, regardless of the printing approach and method of nanocomposite preparation much research remains to be done.
Factors Influencing Antimicrobial Properties of Nanocomposites
As discussed above, M/MO nanoparticles can play a key role in the antibacterial properties of the thermoplastic nanocomposites, in which, their size, ionic status and concentration, strongly influence the antimicrobial performance of the nanocomposite (Salah et al., 2020). Moreover, ionic release of M/MO nanoparticles is critical to the balance between immediate antimicrobial activity and long-term efficacy of the nanocomposites. Accordingly, polymer properties affecting the ionic release of M/MO nanoparticles such as hydrophilicity, density and crystallinity should be carefully considered in the design of antimicrobial nanocomposites for different applications (Tamayo et al., 2016).
Antimicrobial performance of nanocomposites can significantly differ depending on the M/MO incorporated into the same polymer matrix. For example, Muwaffak et al. (2017) suggests that silver in polycaprolactone (PCL) exhibits a superior antibacterial effect against Staphylococcus aureus when compared to copper or zinc. Studies also showed that antibacterial activity of the nanocomposite can be greatly enhanced by size reduction of these nanoparticles. For example, polylactic acid (PLA) filled with 10% silver wires can inhibit only 50% of Escherichia coli growth when the wire diameter is 330 nm (Walker et al., 2020), while 100% inhibition of the same bacterial strain can be achieved by adding only 1% silver nanowires with the average diameter of 60 nm (Bayraktar et al., 2019). Furthermore, oxidized or ionized metal particles are recommended for higher antibacterial efficacy over pure metal particles. For instance, Delgado et al. (2011) investigated the effect of adding copper or copper oxide on antimicrobial activity of polypropylene nanocomposite. Their study revealed that copper oxide fillers are much more efficient at eliminating bacteria when compared to copper fillers, when tested against Escherichia coli (Delgado et al., 2011). Finally, increasing concentration of the antibacterial nanoparticles is a common strategy to enhance overall antibacterial efficiency of the nanocomposites (Kalakonda et al., 2017). Nonetheless, it is important to consider other effects of the printed nanocomposites, such as biocompatibility and environmental safety according to their potential applications.
Applications
3D printed antimicrobial thermoplastic nanocomposites have ample application potential in the medical and healthcare fields, as they can provide an on-demand support for the customization and personalization of medical devices (Zuniga and Cortes, 2020). Specifically, they are very attractive for surgical prosthesis since some of the thermoplastics (e.g., PLA, PCL) have been extensively studied for tissue engineering and regenerative medicine application (Table 1). With the integration of M/MO nanoparticles, bacterial infection can be avoided during surgical implantation, which otherwise could affect tissue growth, postpone surgical recovery, upsurge risks of complications, and even cause death (Abudula et al., 2020). For example, Zou et al. (2020) prepared nanocomposite scaffolds containing poly(lactic-co-glycolic acid) (PLGA), copper/zinc based zeolitic-imidazolate-frameworks (Cu@ZIP-8) by FDM-based 3D printing for infected bone repair application. They suggested that a sustainable release of Cu@ZIP-8 in aqueous media ensures long-term antibacterial efficacy of the developed scaffolds (Zou et al., 2020). Afghah et al. (2020) developed a PCL-poly propylene succinate (PCL-PPSu) composite with silver-doped biocidal properties, which can be printable by FDM. They suggested that the printed scaffolds can be potentially applied for skin tissue engineering due to their inhibition effects against various microorganism, a good cyto-compatibility and biodegradability (Afghah et al., 2020). Similarly, Totu et al. (2017) developed an antibacterial poly methylmethacrylate/TiO2 nanocomposite that was employed for the fabrication of complete denture sets prototyped by SLA 3D printing. Although the composite only contained 0.4% of TiO2 nanoparticles, it exhibited sufficient antimicrobial activity against Candida species. They also tested these nanocomposites with real patients and demonstrated that this manufacturing approach is a promising treatment option for patients diagnosed with complete edentulism (Totu et al., 2017; Cristache et al., 2020). Turner et al. (2020) introduced a SLS printing of antibacterial nanocomposite by mixing polyamide 12 with 1% of commercially available B65003 silver additives to make delicate, complex, and personalized devices for medical and healthcare applications (Turner et al., 2020).
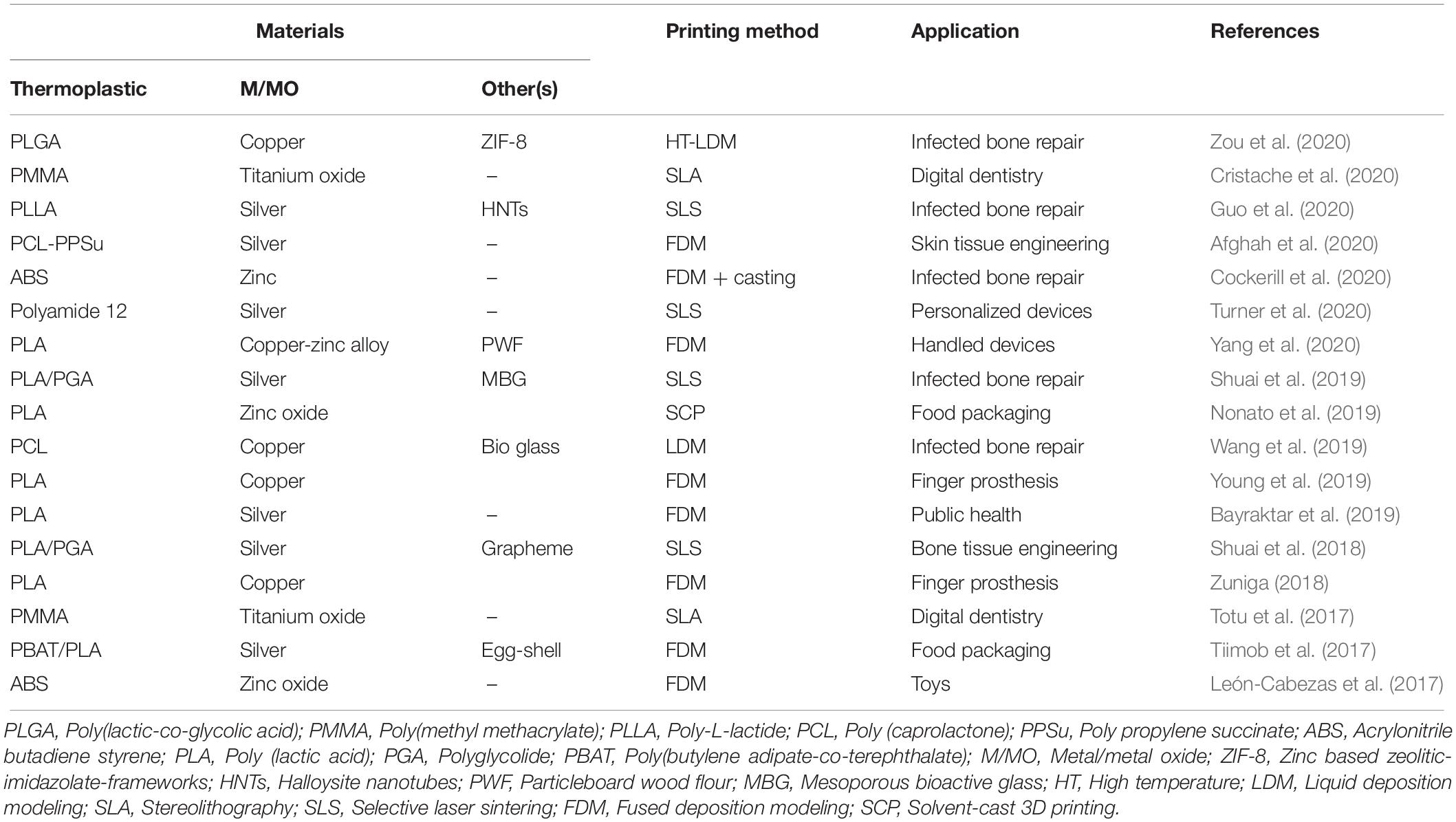
Table 1. Examples of 3D printing of metal/metal oxide incorporated thermoplastic nanocomposites with antibacterial properties for various applications.
Likewise, tuning the mechanical properties by incorporation of M/MO nanoparticles could be of great benefit especially to the food-packaging industry. More specifically, nanocomposites can be a versatile alternative to traditionally manufactured plastics for reducing food contamination and provide safer degradable options for packaging. For example, Tiimob et al. (2017) incorporated silver nanoparticles into a polymer blend composed of 70% poly butylene-co-adipate-terephthalate and 30% PLA to design 3D printed nanocomposites by FDM. Their research suggested that the developed composites can be used for food packaging due to their robust mechanical properties and ability to control growth of microorganisms in food (Tiimob et al., 2017).
Finally, 3D printed antibacterial nanocomposites can also be widely integrated in daily-life products (Huang et al., 2016). Many of the devices and tools that are used routinely in day-to-day tasks can be made with antibacterial materials to reduce the potential negative effects of pathogenic bacteria and fungi. Notably, objects associated with public use can be made safer by 3D printing with antimicrobial nanocomposites. Yang et al. (2020) fabricated a particleboard wood flour/PLA composite filament reinforced by copper-zinc alloy nanoparticles for FDM-based 3D printing. They suggested that the developed filament can be used to print personalized furniture, public benches and toys, owing to its good antimicrobial properties and environmental safety (Yang et al., 2020). In short, these examples provide a clear vision of how 3D printing of antimicrobial nanocomposites can be applied to make imaginative and customized products.
Challenges and Future Scope
In conclusion, M/MO nanoparticles as antimicrobial fillers can greatly increase the value of 3D printed thermoplastic products. Nonetheless, challenges remain in commercializing these thermoplastic nanocomposites for a wider range of applications. For example, one of the critical issues related to their clinical application is that many antibacterial tests have been performed in vitro, in which some factors that influence antibacterial performance in vivo, such as inflammation, are neglected (Wang et al., 2016; Jiao et al., 2019). A similar challenge is having comprehensive compatibility testing of these nanocomposites that are essential for their use with the human body. Long-term exposure and biosafety remain a major challenge when consider their application in the medical field, particularly toxic effect of M/MO on a wide range of cells and tissue need to be assessed. For example, Ickrath et al. (2017) suggested cytotoxic and genotoxic effects of zinc oxide nanoparticles after long-term and repetitive exposure to human mesenchymal stem cells. Therefore, currently reported short-term, single cell-line reference data is often not enough to provide a generalized biocompatibility profile of the developed materials in many if not most cases (Tamayo et al., 2016; Rtimi et al., 2019). In addition, burst-release of antimicrobial agents (i.e., metal ions or particles) can represent a significant limitation of the 3D printed composites. One way to overcome this challenge would be by entrapping antibacterial particles with an additional polymer layer using coaxial nozzle configurations (Yao et al., 2019). Meanwhile, release properties of antibacterial components could be also controlled by stimuli-responsive materials, especially if the nanocomposites can be release in response to bacterial adhesion to the material (González-Henríquez et al., 2019b). In addition, controlled release of nanoparticles as ions or metallic particles from the polymer matrix is often key to maintaining the balance between antimicrobial performance and mammalian cytocompatibility (Tamayol et al., 2017; Mas-Moruno et al., 2019).
Other challenges include addition of M/MO nanoparticles into 3D printed thermoplastics often through a multistage process. For example, the nanoparticles first need to be synthesized, then homogeneously dispersed into the polymer matrix and finally 3D printed. This not only increases the total manufacturing cost, but can also deteriorate 3D print quality by inducing filler defects, poor adhesion and particle agglomeration or uneven distribution. Alternatively, in situ synthesis of nanoparticles could possibly be a way to overcome such challenges (Palza, 2015). The concept of in situ printing has recently emerged, in which personalized healthcare devices and prosthesis can be directly printed on the defected tissue or organ without 3D scanning and computational design. Particularly, robotic assisted in situ printing could be one of the most exciting techniques introduced in the future, which would elevate novelty of 3D printing to a new level (Ma et al., 2020). Finally, there are many possible application areas yet to be studied using 3D printed nanocomposites, particularly considering their capacity to tailor the product design on a personal level (Ishack and Lipner, 2020). Specifically, there is a lot of potential for developing personal protective equipment, including masks, ventilators tubes, gloves, which could be especially important during the current global pandemic (Zuniga and Cortes, 2020).
Author Contributions
The manuscript was written and edited with contribution from all authors. All authors have given approval to the final version of the manuscript.
Funding
This project was funded by the Science and Technology Unit – King Abdulaziz University – Kingdom of Saudi Arabia award number UE-41-106.
Conflict of Interest
The authors declare that the research was conducted in the absence of any commercial or financial relationships that could be construed as a potential conflict of interest.
Acknowledgments
This project was funded by the Science and Technology Unit – King Abdulaziz University – Kingdom of Saudi Arabia award number UE-41-106.
Supplementary Material
The Supplementary Material for this article can be found online at: https://www.frontiersin.org/articles/10.3389/fbioe.2020.568186/full#supplementary-material
References
Abudula, T., Gauthaman, K., Hammad, A. H., Joshi Navare, K., Alshahrie, A. A., Bencherif, S. A., et al. (2020). Oxygen-releasing antibacterial nanofibrous scaffolds for tissue engineering applications. Polymers 12:1233. doi: 10.3390/polym12061233
Afghah, F., Ullah, M., Zanjani, J. S. M., Sut, P. A., Sen, O., Emanet, M., et al. (2020). 3D printing of silver-doped polycaprolactone-poly (propylene succinate) composite scaffolds for skin tissue engineering. Biomed. Mater. 15:035015.
Al-Shawafi, W. M., Salah, N., Alshahrie, A., Ahmed, Y. M., Moselhy, S. S., Hammad, A. H., et al. (2017). Size controlled ultrafine CeO2 nanoparticles produced by the microwave assisted route and their antimicrobial activity. J. Mater. Sci. 28:177. doi: 10.1007/s10856-017-5990-5998
Bayraktar, I., Doganay, D., Coskun, S., Kaynak, C., Akca, G., and Unalan, H. E. (2019). 3D printed antibacterial silver nanowire/polylactide nanocomposites. Composit. Part B Eng. 172, 671–678. doi: 10.1016/j.compositesb.2019.05.059
Blok, L. G., Longana, M. L., Yu, H., and Woods, B. K. (2018). An investigation into 3D printing of fibre reinforced thermoplastic composites. Add. Manufact. 22, 176–186. doi: 10.1016/j.addma.2018.04.039
Chandrangsu, P., Rensing, C., and Helmann, J. D. (2017). Metal homeostasis and resistance in bacteria. Nat. Rev. Microbiol. 15:338. doi: 10.1038/nrmicro.2017.15
Cockerill, I., Su, Y., Sinha, S., Qin, Y.-X., Zheng, Y., Young, M. L., et al. (2020). Porous zinc scaffolds for bone tissue engineering applications: a novel additive manufacturing and casting approach. Mater. Sci. Eng. C 110:110738. doi: 10.1016/j.msec.2020.110738
Cristache, C. M., Totu, E. E., Iorgulescu, G., Pantazi, A., Dorobantu, D., Nechifor, A. C., et al. (2020). Eighteen months follow-up with patient-centered outcomes assessment of complete dentures manufactured using a hybrid nanocomposite and additive CAD/CAM protocol. J. Clin. Med. 9:324. doi: 10.3390/jcm9020324
Delgado, K., Quijada, R., Palma, R., and Palza, H. (2011). Polypropylene with embedded copper metal or copper oxide nanoparticles as a novel plastic antimicrobial agent. Lett. Appl. Microbiol. 53, 50–54. doi: 10.1111/j.1472-765x.2011.03069.x
Diez-Pascual, A. M., and Diez-Vicente, A. L. (2017). Antibacterial SnO2 nanorods as efficient fillers of poly (propylene fumarate-co-ethylene glycol) biomaterials. Mater. Sci. Eng. C 78, 806–816. doi: 10.1016/j.msec.2017.04.114
Emamifar, A. (2011). “Applications of antimicrobial polymer nanocomposites in food packaging,” Advances in Nanocomposite Technology, 299–318.
Gerdes, S., Mostafavi, A., Ramesh, S., Memic, A., Rivero, I. V., Rao, P., et al. (2020). Process-structure-quality relationships of three-dimensional printed poly (Caprolactone)-hydroxyapatite scaffolds. Tissue Eng. Part A 26, 279–291. doi: 10.1089/ten.tea.2019.0237
Gibson, I., Rosen, D. W., and Stucker, B. (2014). Additive Manufacturing Technologies. Berlin: Springer.
Gnanadhas, D. P., Elango, M., Janardhanraj, S., Srinandan, C., Datey, A., Strugnell, R. A., et al. (2015). Successful treatment of biofilm infections using shock waves combined with antibiotic therapy. Sci. Rep. 5:17440.
Gold, K., Slay, B., Knackstedt, M., and Gaharwar, A. K. (2018). Antimicrobial activity of metal and metal-oxide based nanoparticles. Adv. Therap. 1:1700033. doi: 10.1002/adtp.201700033
González-Henríquez, C. M., Sarabia-Vallejos, M. A., and Rodríguez Hernandez, J. (2019a). Antimicrobial polymers for additive manufacturing. Intern. J. Mol. Sci. 20:1210. doi: 10.3390/ijms20051210
González-Henríquez, C. M., Sarabia-Vallejos, M. A., and Rodriguez-Hernandez, J. (2019b). Polymers for additive manufacturing and 4D-printing: materials, methodologies, and biomedical applications. Prog. Poly. Sci. 94, 57–116. doi: 10.1016/j.progpolymsci.2019.03.001
Guo, H., Lv, R., and Bai, S. (2019). Recent advances on 3D printing graphene-based composites. Nano Mater. Sci. 1, 101–115. doi: 10.1016/j.nanoms.2019.03.003
Guo, W., Liu, W., Xu, L., Feng, P., Zhang, Y., Yang, W., et al. (2020). Halloysite nanotubes loaded with nano silver for the sustained-release of antibacterial polymer nanocomposite scaffolds. J. Mater. Sci. Technol. 46, 237–247. doi: 10.1016/j.jmst.2019.11.019
Hasan, A., Morshed, M., Memic, A., Hassan, S., Webster, T. J., and Marei, H. E.-S. (2018). Nanoparticles in tissue engineering: applications, challenges and prospects. Intern. J. Nanomed. 13:5637. doi: 10.2147/ijn.s153758
He, L., Liu, Y., Mustapha, A., and Lin, M. (2011). Antifungal activity of zinc oxide nanoparticles against Botrytis cinerea and Penicillium expansum. Microbiol. Res. 166, 207–215. doi: 10.1016/j.micres.2010.03.003
Hewitt, E., Mros, S., McConnell, M., Cabral, J. D., and Ali, A. (2019). Melt-electrowriting with novel milk protein/PCL biomaterials for skin regeneration. Biomed. Mater. 14:055013.
Huang, K.-S., Yang, C.-H., Huang, S.-L., Chen, C.-Y., Lu, Y.-Y., and Lin, Y.-S. (2016). Recent advances in antimicrobial polymers: a mini-review. Intern. J. Mol. Sci. 17:1578. doi: 10.3390/ijms17091578
Ickrath, P., Wagner, M., Scherzad, A., Gehrke, T., Burghartz, M., Hagen, R., et al. (2017). Time-dependent toxic and genotoxic effects of zinc oxide nanoparticles after long-term and repetitive exposure to human mesenchymal stem cells. Intern. J. Environ. Res. Public Health 14:1590. doi: 10.3390/ijerph14121590
Imlay, J. A. (2014). The mismetallation of enzymes during oxidative stress. J. Biol. Chem. 289, 28121–28128. doi: 10.1074/jbc.r114.588814
Ishack, S., and Lipner, S. R. (2020). Applications of 3D printing technology to address COVID-19 related supply shortages. Am. J. Med. 133, 771–773. doi: 10.1016/j.amjmed.2020.04.002
Jiao, Y., Tay, F. R., Niu, L.-N., and Chen, J.-H. (2019). Advancing antimicrobial strategies for managing oral biofilm infections. Intern. J. Oral Sci. 11, 1–11. doi: 10.1038/ijos.2014.65
Jiménez, M., Romero, L., Domínguez, I. A., Espinosa, M. D. M., and Domínguez, M. (2019). Additive manufacturing technologies: an overview about 3D printing methods and future prospects. Complexity 2019, 1–30. doi: 10.1155/2019/9656938
Kalakonda, P., Aldhahri, M. A., Abdel-Wahab, M. S., Tamayol, A., Moghaddam, K. M., Rached, F. B., et al. (2017). Microfibrous silver-coated polymeric scaffolds with tunable mechanical properties. RSC Adv. 7, 34331–34338. doi: 10.1039/c6ra25151j
León-Cabezas, M. A., Martínez-García, A., and Varela-Gandía, F. J. (2017). Innovative functionalized monofilaments for 3D printing using fused deposition modeling for the toy industry. Proc. Manufact. 13, 738–745. doi: 10.1016/j.promfg.2017.09.130
Ma, K., Zhao, T., Yang, L., Wang, P., Jin, J., Teng, H., et al. (2020). Application of robotic-assisted in situ 3D printing in cartilage regeneration with HAMA hydrogel: an in vivo study. J. Adv. Res. 23, 123–132. doi: 10.1016/j.jare.2020.01.010
MacDonald, E., and Wicker, R. (2016). Multiprocess 3D printing for increasing component functionality. Science 353:aaf2093. doi: 10.1126/science.aaf2093
Martin, J. H., Yahata, B. D., Hundley, J. M., Mayer, J. A., Schaedler, T. A., and Pollock, T. M. (2017). 3D printing of high-strength aluminium alloys. Nature 549:365. doi: 10.1038/nature23894
Martinez-Gutierrez, F., Olive, P. L., Banuelos, A., Orrantia, E., Nino, N., Sanchez, E. M., et al. (2010). Synthesis, characterization, and evaluation of antimicrobial and cytotoxic effect of silver and titanium nanoparticles. Nanomed. Nanotechnol. Biol. Med. 6, 681–688. doi: 10.1016/j.nano.2010.02.001
Mas-Moruno, C., Su, B., and Dalby, M. J. (2019). Multifunctional coatings and nanotopographies: toward cell instructive and antibacterial implants. Adv. Healthc. Mater. 8:1801103. doi: 10.1002/adhm.201801103
Mazzoli, A. (2013). Selective laser sintering in biomedical engineering. Med. Biol. Eng. Comput. 51, 245–256. doi: 10.1007/s11517-012-1001-x
Memic, A., Abudula, T., Mohammed, H. S., Joshi Navare, K., Colombani, T., and Bencherif, S. A. (2019). Latest progress in electrospun nanofibers for wound healing applications. ACS Appl. Bio. Mater. 2, 952–969. doi: 10.1021/acsabm.8b00637
Memic, A., Navaei, A., Mirani, B., Cordova, J. A. V., Aldhahri, M., Dolatshahi-Pirouz, A., et al. (2017). Bioprinting technologies for disease modeling. Biotechnol. Lett. 39, 1279–1290. doi: 10.1007/s10529-017-2360-z
Muwaffak, Z., Goyanes, A., Clark, V., Basit, A. W., Hilton, S. T., and Gaisford, S. (2017). Patient-specific 3D scanned and 3D printed antimicrobial polycaprolactone wound dressings. Int. J. Pharm. 527, 161–170. doi: 10.1016/j.ijpharm.2017.04.077
Nel, A., Xia, T., Mädler, L., and Li, N. (2006). Toxic potential of materials at the nanolevel. Science 311, 622–627. doi: 10.1126/science.1114397
Ngo, T. D., Kashani, A., Imbalzano, G., Nguyen, K. T. Q., and Hui, D. (2018). Additive manufacturing (3D printing): a review of materials, methods, applications and challenges. Composit. Part B Eng. 143, 172–196. doi: 10.1016/j.compositesb.2018.02.012
Nonato, R. C., Mei, L. H. I., Bonse, B. C., Chinaglia, E. F., and Morales, A. R. (2019). Nanocomposites of PLA containing ZnO nanofibers made by solvent cast 3D printing: production and characterization. Eur. Poly. J. 114, 271–278. doi: 10.1016/j.eurpolymj.2019.02.026
Palza, H. (2015). Antimicrobial polymers with metal nanoparticles. Intern. J. Mol. Sci. 16, 2099–2116. doi: 10.3390/ijms16012099
Paul, R., and Anand, S. (2012). Process energy analysis and optimization in selective laser sintering. J. Manufact. Syst. 31, 429–437. doi: 10.1016/j.jmsy.2012.07.004
Poelma, J., and Rolland, J. (2017). Rethinking digital manufacturing with polymers. Science 358, 1384–1385. doi: 10.1126/science.aaq1351
Raghunath, A., and Perumal, E. (2017). Metal oxide nanoparticles as antimicrobial agents: a promise for the future. Int. J. Antimicrob. Agents 49, 137–152. doi: 10.1016/j.ijantimicag.2016.11.011
Rhim, J.-W., Park, H.-M., and Ha, C.-S. (2013). Bio-nanocomposites for food packaging applications. Prog. Poly. Sci. 38, 1629–1652.
Richards, M. J., Edwards, J. R., Culver, D. H., and Gaynes, R. P. (1999). Nosocomial infections in medical intensive care units in the United States. Crit. Care Med. 27, 887–892. doi: 10.1097/00003246-199905000-00020
Rtimi, S., Dionysiou, D. D., Pillai, S. C., and Kiwi, J. (2019). Advances in catalytic/photocatalytic bacterial inactivation by nano Ag and Cu coated surfaces and medical devices. Appl. Catal. B Environ. 240, 291–318. doi: 10.1016/j.apcatb.2018.07.025
Salah, N., Alfawzan, A. M., Allafi, W., Baghdadi, N., Saeed, A., Alshahrie, A., et al. (2020). Size-controlled, single-crystal CuO nanosheets and the resulting polyethylene-carbon nanotube nanocomposite as antimicrobial materials. Polym. Bull. 1–21.
Salah, N., Al-Shawafi, W. M., Alshahrie, A., Baghdadi, N., Soliman, Y. M., and Memic, A. (2019). Size controlled, antimicrobial ZnO nanostructures produced by the microwave assisted route. Mater. Sci. Eng. C 99, 1164–1173. doi: 10.1016/j.msec.2019.02.077
Sánchez-López, E., Gomes, D., Esteruelas, G., Bonilla, L., Lopez-Machado, A. L., Galindo, R., et al. (2020). Metal-based nanoparticles as antimicrobial agents: an overview. Nanomaterials 10:292.
Shuai, C., Guo, W., Wu, P., Yang, W., Hu, S., Xia, Y., et al. (2018). A graphene oxide-Ag co-dispersing nanosystem: dual synergistic effects on antibacterial activities and mechanical properties of polymer scaffolds. Chem. Eng. J. 347, 322–333. doi: 10.1016/j.cej.2018.04.092
Shuai, C., Xu, Y., Feng, P., Wang, G., Xiong, S., and Peng, S. (2019). Antibacterial polymer scaffold based on mesoporous bioactive glass loaded with in situ grown silver. Chem. Eng. J. 374, 304–315. doi: 10.1016/j.cej.2019.03.273
Skaar, E. P., and Raffatellu, M. (2015). Metals in infectious diseases and nutritional immunity. Metallomics 7, 926–928. doi: 10.1039/c5mt90021b
Tamayo, L., Azócar, M., Kogan, M., Riveros, A., and Páez, M. (2016). Copper-polymer nanocomposites: an excellent and cost-effective biocide for use on antibacterial surfaces. Mater. Sci. Eng. C 69, 1391–1409. doi: 10.1016/j.msec.2016.08.041
Tamayol, A., Najafabadi, A. H., Mostafalu, P., Yetisen, A. K., Commotto, M., Aldhahri, M., et al. (2017). Biodegradable elastic nanofibrous platforms with integrated flexible heaters for on-demand drug delivery. Sci. Rep. 7, 1–10.
Tiimob, B. J., Mwinyelle, G., Abdela, W., Samuel, T., Jeelani, S., and Rangari, V. K. (2017). Nanoengineered eggshell-silver tailored copolyester polymer blend film with antimicrobial properties. J. Agric. Food Chem. 65, 1967–1976. doi: 10.1021/acs.jafc.7b00133
Totu, E. E., Nechifor, A. C., Nechifor, G., Aboul-Enein, H. Y., and Cristache, C. M. (2017). Poly (methyl methacrylate) with TiO2 nanoparticles inclusion for stereolitographic complete denture manufacturing- the fututre in dental care for elderly edentulous patients? J. Dent. 59, 68–77. doi: 10.1016/j.jdent.2017.02.012
Turner, R. D., Wingham, J. R., Paterson, T. E., Shepherd, J., and Majewski, C. (2020). Use of silver-based additives for the development of antibacterial functionality in Laser Sintered polyamide 12 parts. Sci. Rep. 10, 1–11.
Valino, A. D., Dizon, J. R. C., Espera, A. H., Chen, Q., Messman, J., and Advincula, R. C. (2019). Advances in 3D printing of thermoplastic polymer composites and nanocomposites. Prog. Polym. Sci. 98:101162. doi: 10.1016/j.progpolymsci.2019.101162
Vasilev, K., Cook, J., and Griesser, H. J. (2009). Antibacterial surfaces for biomedical devices. Expert Rev. Med. Dev. 6, 553–567. doi: 10.1586/erd.09.36
Walker, J. S., Arnold, J., Shrestha, C., and Smith, D. (2020). Antibacterial silver submicron wire-polylactic acid composites for fused filament fabrication. Rapid Prototyp. J. 26, 32–38. doi: 10.1108/rpj-04-2019-0100
Wang, B., Han, Y., Lin, Q., Liu, H., Shen, C., Nan, K., et al. (2016). In vitro and in vivo evaluation of xanthan gum-succinic anhydride hydrogels for the ionic strength-sensitive release of antibacterial agents. J. Mater. Chem. B 4, 1853–1861. doi: 10.1039/c5tb02046h
Wang, X., Molino, B. Z., Pitkänen, S., Ojansivu, M., Xu, C., Hannula, M., et al. (2019). 3D scaffolds of polycaprolactone/copper-doped bioactive glass: architecture engineering with additive manufacturing and cellular assessments in a coculture of bone marrow stem cells and endothelial cells. ACS Biomater. Sci. Eng. 5, 4496–4510. doi: 10.1021/acsbiomaterials.9b00105
Weinstein, R. A., and Darouiche, R. O. (2001). Device-associated infections: a macroproblem that starts with microadherence. Clin. Infect. Dis. 33, 1567–1572. doi: 10.1086/323130
Weng, Z., Zhou, Y., Lin, W., Senthil, T., and Wu, L. (2016). Structure-property relationship of nano enhanced stereolithography resin for desktop SLA 3D printer. Composit. Part A Appl. Sci. Manufact. 88, 234–242. doi: 10.1016/j.compositesa.2016.05.035
Wohlers Talk (2020). 3D Printing And Additive Manufacturing State Of The Industry. Annual Worldwide Progress Report. Fort Collins, CO: Wohlers Associates.
Yang, F., Zeng, J., Long, H., Xiao, J., Luo, Y., Gu, J., et al. (2020). Micrometer copper-zinc alloy particles-reinforced wood plastic composites with high gloss and antibacterial properties for 3D printing. Polymers 12:621. doi: 10.3390/polym12030621
Yao, Z.-C., Wang, J.-C., Ahmad, Z., Li, J.-S., and Chang, M.-W. (2019). Fabrication of patterned three-dimensional micron scaled core-sheath architectures for drug patches. Mater. Sci. Eng. C 97, 776–783. doi: 10.1016/j.msec.2018.12.110
Young, K. J., Pierce, J. E., and Zuniga, J. M. (2019). Assessment of body-powered 3D printed partial finger prostheses: a case study. 3D Print. Med. 5, 1–8.
Zheng, K., Setyawati, M. I., Leong, D. T., and Xie, J. (2017). Antimicrobial gold nanoclusters. ACS Nano 11, 6904–6910. doi: 10.1021/acsnano.7b02035
Zou, F., Jiang, J., Lv, F., Xia, X., and Ma, X. (2020). Preparation of antibacterial and osteoconductive 3D-printed PLGA/Cu (I)@ ZIF-8 nanocomposite scaffolds for infected bone repair. J. Nanobiotechnol. 18, 1–14.
Zuniga, J. M. (2018). 3D printed antibacterial prostheses. Appl. Sci. 8:1651. doi: 10.3390/app8091651
Keywords: 3D printing, antimicrobial, metals, metal oxides, thermoplastics, nanocomposites
Citation: Abdullah T, Qurban RO, Bolarinwa SO, Mirza AA, Pasovic M and Memic A (2020) 3D Printing of Metal/Metal Oxide Incorporated Thermoplastic Nanocomposites With Antimicrobial Properties. Front. Bioeng. Biotechnol. 8:568186. doi: 10.3389/fbioe.2020.568186
Received: 31 May 2020; Accepted: 13 August 2020;
Published: 15 September 2020.
Edited by:
Eun-Bum Cho, Seoul National University of Science and Technology, South KoreaReviewed by:
Fatemeh Kabirian, Materials and Energy Research Center, IranShun Duan, Beijing University of Chemical Technology, China
Copyright © 2020 Abdullah, Qurban, Bolarinwa, Mirza, Pasovic and Memic. This is an open-access article distributed under the terms of the Creative Commons Attribution License (CC BY). The use, distribution or reproduction in other forums is permitted, provided the original author(s) and the copyright owner(s) are credited and that the original publication in this journal is cited, in accordance with accepted academic practice. No use, distribution or reproduction is permitted which does not comply with these terms.
*Correspondence: Adnan Memic, YW1lbWljQGthdS5lZHUuc2E=