- 1Department of Mathematics and Physics “Ennio De Giorgi,” University of Salento, Lecce, Italy
- 2National Research Council Nanotec Institute of Nanotechnology, Lecce, Italy
In the last decade, the onset of neurodegenerative (ND) diseases is strongly widespread due to the age increase of the world population. Despite the intensive investigations boosted by the scientific community, an efficacious therapy has not been outlined yet. The drugs commonly used are only able to relieve symptom severity; following their oral or intravenous administration routes, their effectiveness is strictly limited due to their low ability to reach the Central Nervous System (CNS) overcoming the Blood Brain Barrier (BBB). Starting from these assumptions, the engineered-nanocarriers, such as lipid-nanocarriers, are suitable agents to enhance the delivery of drugs into the CNS due to their high solubility, bioavailability, and stability. Liposomal delivery systems are considered to be the ideal carriers, not only for conventional drugs but also for neuroprotective small molecules and green-extracted compounds. In the current work, the LP-based drug delivery improvements in in vivo applications against ND disorders were carefully assessed.
Introduction
Pharmacological research is oriented to develop new effective therapeutic strategies in order to treat diseases and, at same time, to improve the life quality of patients. Many studies aim to discover new drugs, taking in consideration that their effectiveness is strictly dependent on the administration route and also on their intrinsic ability to access the organs and tissues in suitable amounts and times. The uptake of drugs is particularly difficult in specific districts of body, such as the Central Nervous System (CNS), in which the presence of a Blood Brain Barrier (BBB) constitutes the major obstacle. The BBB often inhibits neuroreparative and neuroprotective therapies that should carry out their pharmacological action directly on target site. According to the latest available data, it is estimated that 10 % of the world population will be affected by neurological disorders, without geographical and socioeconomic distinctions (Organization, 2006). For this reason, the World Health Organization recommends great efforts to overcome the difficulties in the brain drugs administration. Despite the neuropharmaceutical field constituting the largest sector of drug industry, its development is limited by the complication regarding the BBB crossing. It has been estimated that ca. 98% of the drugs available today for the treatment of neurological diseases, including recombinant proteins, monoclonal antibodies, and genes are not able to effectively overcome the BBB. This is true due to their deficiency to exploit specific transport mechanisms and their high molecular weight together with polarity (Pardridge and Boado, 2012). In light of this, the regulatory mechanisms of the BBB are fundamental for the development of new therapies dedicated to large groups of brain injuries such as neurodegenerative diseases.
Neurodegenerative Diseases
The age-dependent disorders are becoming increasingly prevalent, in part because the elderly population has increased in recent years (Heemels, 2016).
Examples of neurodegenerative diseases are amyotrophic lateral sclerosis, Parkinson's disease, Alzheimer's disease, Huntington's disease, frontotemporal dementia, and the spinocerebellar ataxias. All these pathologies are different in pathophysiologic properties but, at the same time, are associated with memory loss, cognitive impairments, and other adverse effects including a person's inability to move, breathe, and speak (Abeliovich and Gitler, 2016; Wyss-Coray, 2016; Gitler et al., 2017). These pathologies show selective neuronal vulnerability combined with the degeneration of specific brain regions; in addition, an abnormal protein deposit (extracellular or intracellular) occurs in neurons or other types of brain cells (Ross and Poirier, 2004).
Alzheimer's disease (AD) is characterized by neuron degeneration; in particular, in the basal forebrain and hippocampus. In addition, the alteration of synaptic and neuronal connections is involved in the pathogenesis (Selkoe, 2002). The accumulation of amyloid-β (Aβ) peptides and tau protein boosts the development of the pathology progression (Combs et al., 2016). In detail, the Amyloid beta, a 39–43-amino acid residue peptide, is a physiological presence in human brain and is derived from the proteolysis process of the amyloid precursor protein (APP). The extracellular accumulation of amyloid-β (Aβ) peptides induces the formation of amyloid plaques, also called senile plaques, which form the synaptic dysfunction and neuronal loss (Citron, 2002). The tau proteins (T proteins) play a key role in microtubule stabilization and they are abundant in hippocampal areas, cortical areas, and the entorhinal cortex. In AD disease, this protein undergoes a hyperphosphorylation phenomenon that induces tau protein clumping with the consequence of developing intracellular neurofibrillary tangles (NFT): these formations lead to axon degeneration and microtubule disaggregation (Goedert, 2004). The onset of AD may be due to rare genetic mutations, which were found in the APP and in some transmembrane proteins (presenilins) that cleave the APP. Mutations of tau28 protein have been found in non-Parkinson frontotemporal dementia.
Parkinson's disease (PD) is provoked by degeneration of dopaminergic neurons in the substantia nigra of the midbrain and other monoaminergic neurons in the brain stem (Vernier et al., 2004). Despite several progresses, the main cause of PD remains unknown. It is probably born by complicate connections between environmental and genetic factors that are strong effects on cells that trigger mitochondrial dysfunction, oxidative stress, impairment of the ubiquitin proteosome process, as well as defective autophagy process (Dawson et al., 2010; Angeles et al., 2014). However, the exact mechanisms leading to neuronal death are unknown. As a consequence, the loss of several neurotransmitters and neuromodulators in the extranigral neurons, such as noradrenaline (NA), acetylcholine (ACh), glutamate, and serotonin (5-hydroxytryptaminc [5-HT]) occur. These phenomena induce the slowing of movement, muscular rigidity, and tremor (Alexander, 2004).
Numerous gene mutations could induce the early onset of PD, in particular, point mutations or increased gene dosage of the α-synuclein gene. These boost an autosomal dominant PD. Instead, the mutations of genes encoding for DJ-1 or PINK132 and parkin could trigger a recessive early-onset PD. In general, the adult PD onset regards an inclusion body near the nucleus of neurons, immersed in cytoplasm, called Lewy body, in the form of fibrillar aggregates. Several investigations demonstrated the presence of Lewy bodies in the substantial nigra in monoaminergic, cerebral, cortical, and other neurons principally constituted of α-synuclein protein (Stefanis, 2012).
The Blood Brain Barrier (BBB)
The human brain contains about 100 billion of capillaries with a total length of 600 km. This dense vascular network covers an area of about 20 m2 and represents an effective interface between the blood and the brain, supplying brain cells with oxygen and essential metabolites that support brain functions (Risau and Wolburg, 1990). In addition, the brain consumes 20% of the body's total glucose, increasing the blood flow and oxygen in order to quickly reach the different body's districts (Begley and Brightman, 2003). Capillaries are the major site of blood barrier brain (BBB) that constitutes a real barrier between the bloodstream and the central nervous system acting as selective filter, allowing or preventing substances (ions, glucose, proteins etc.) to move from the blood to the brain parenchyma and to cerebrospinal fluid (CSF). Thanks to this selectivity, the BBB protects the sickly chemical–physical homeostasis of the cerebral fluid environment carrying out a protective role toward CSF and the nervous tissue (Abbott and Romero, 1996).
This can be satisfied by keeping the ionic environment stable and preserving the low amino-acid gradient of the excitatory neurotransmitters (glutamic acids, aspartic acid, and glycine) that is characteristic of the brain extracellular fluid. This phenomenon is critical for reliable synaptic transmission and efficient neuroregulatory activity. BBB prevents the toxic compound uptake in cells, such as metabolites and neurotoxins both endogenous and xenobiotic, which are potentially fatal. In addition, the BBB promotes the longevity of the CNS and prevents premature cell death and neurodegeneration.
The BBB is formed by the endothelium of the brain capillaries, the processes perivascular of the astrocytes surrounding the endothelial cells and from the pericytes which are contractile connective cells that partially surround them. The anatomical structure of the BBB is responsible for its functional peculiarities, such as limited permeability to most substances and limited paracellular and transcellular transport (Zlokovic, 2008; Kisler et al., 2017). The brain capillaries are anatomically different from peripheral systemic ones since the cells that compose them form a continuous non-fenestrated endothelium, with a reduced number of pinocytic cells (Engelhardt and Liebner, 2014). Brain endothelial cells are connected by tight and adherent junctions. The first prevents the free diffusion of solutes from the blood sector (peripheral or systemic) to the CSF and brain (intrathecal), both at the level of the cerebral capillaries and the chorioid epithelium. The tight junctions involve occluding, claudin I, claudin 3, claudin 5, claudin 12, and the membrane-associated guanylate kinases, proteins ZO1, ZO2, and ZO3. The adherent junctions include cadherins, platelet endothelial cell adhesion molecules (PECAM1), and the junctional adhesions molecules (JAMs) JAMA, JAMB, and JAMC. The gas exchange (oxygen and carbon dioxide) occurs across the endothelium, whereas the pinocytosis deficit is responsible for the limitation of solutes passage (Abbott et al., 2010; Zhao et al., 2015).
Then, in order to target a specific drug to the brain, it is necessary to consider the structural and functional characteristics of the BBB and, at the same time, evaluate the chemical-physical properties of the drug (pKa, molecular weight, lipophilicity, etc.). In addition, it is important to consider the intrinsic ability of BBB to form bonds with the plasma proteins that prevent the CNS crossing, the degree of ionization (pH), and the lipid/water partition coefficient of the drug (Warren, 2018). In particular, it has been demonstrated that small lipid-soluble molecules (high partition coefficient) with a molecular weight <400 Da or containing <8 hydrogen bonds can cross BBB by simple transmembrane diffusion. Contrary, the drugs with low passive diffusion coefficient can be internalized in the brain only by active transports systems (Pardridge, 2015).
Transport Through the BBB
Oxygen, carbon dioxide, glucose, nucleosides, vitamins, and some of fat-soluble drugs can cross the BBB by passive diffusion mechanisms or by specific transport mechanisms. The transport systems (Figure 1) can be located on the luminal or abluminal side of the BBB and can be classified into three categories (Abbott and Romero, 1996; Begley, 2004): (i) CMT (Carrier Mediated Transport) consists of a transport mediated by specific transport carrier proteins; they are able to move carbohydrates, fatty acids, amino acids, nucleotides, hormones, vitamins, organic cations and anions through BBB; (ii) AET (Active Efflux Transport) consists of an active transport mechanism capable of expelling a large variety of molecules from the brain compartment to the bloodstream; and (iii) RMT (Receptor Mediated Transport) which consists of receptors mediated transport system, able to drive large compounds (peptides and proteins) through an intracellular process in both directions: insulin and transferrin from blood to brain, as well as apolipoproteins (Demeule et al., 2008; Sagare et al., 2012). The transport of native plasma proteins or peptides is limited, but the cationization phenomenon can increase their uptake by adsorptive-mediated transport (AMT).
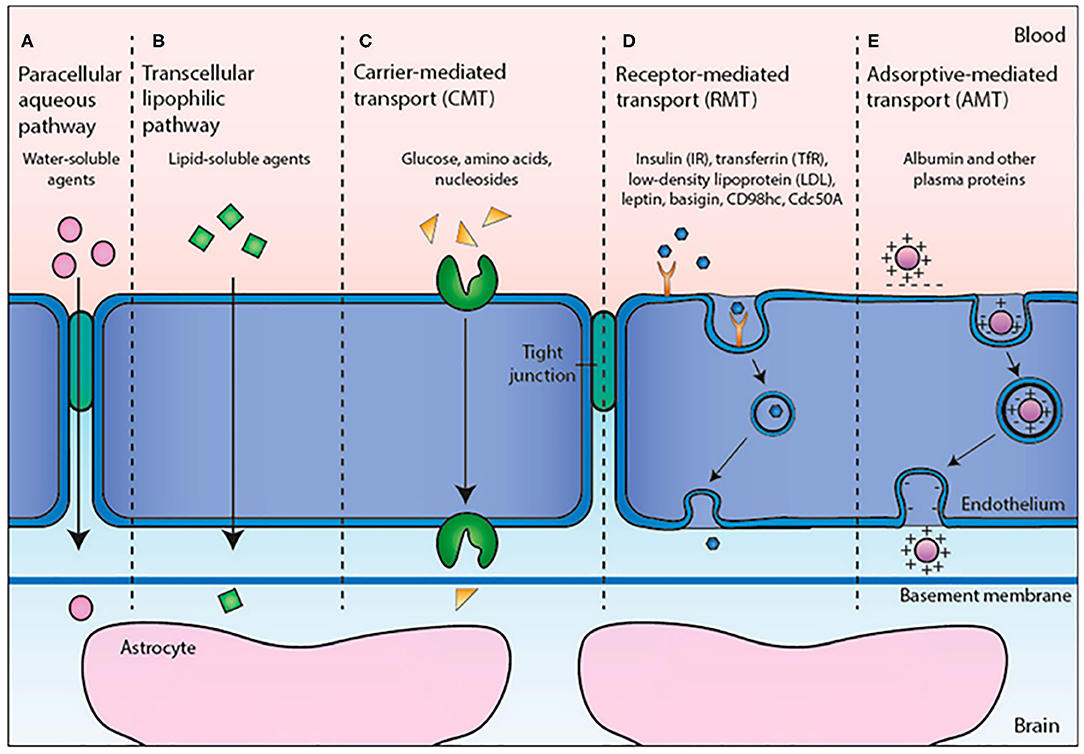
Figure 1. Transport mechanisms through BBB. Reproduced with permission from Cavaco et al. (2020).
The CMT and AET systems are responsible for small molecule transport between the blood and the brain, while RMT allows large molecule delivery through the BBB. Among these, the transmembrane transporters called ABC transporters, expressed at the luminal side of BBB, play an important role due to the presence of two cytoplasmic domains useful to bind the ATP boosting transport against the unidirectional gradient (from cytoplasm to extracellular space) (Abbott et al., 2010; Mokgokong et al., 2014).
Therefore, ABC prevents the brain accumulation of xenobiotic agents, and drugs exert an important role in the body detoxification. The first ABC transporter identified is the P-glycoprotein (P-gp or ABCB1) coded by the MDR1 gene. This gene is known for its several polymorphisms (ca. 50) at the level of a single nucleotide, and for this reason, it is responsible for a strong individual variability in absorption and tolerance to drugs (Yan-Hong et al., 2006; Bartels, 2011). In addition to the ABC, OATP (Organic Anion Transporting-Polypeptide) and the OAT (Organic Anion Transporter) members are expressed in the endothelial cells of the BBB. The main function of OATP 1A2 and 2B1 carriers is to uptake several endogenous and xenobiotic compounds (10.1021/acs.molpharmaceut.0c00159), but, unlike the members of the ABC family, the OATP does not hydrolyze ATP, and consequently, they fail to uptake drugs against the concentration gradient (de Boer et al., 2003). Their presence allows the ions exchange according to the ionic gradient. In addition to the possibilities of exploiting or inhibiting these and other physiologically present transport mechanisms on the BBB (Pardridge and Boado, 2012), other approaches that can guarantee are being studied for a more effective delivery of drugs to the CNS. A spread class of carriers, abundant in mammalian neurocytes and brain capillary epithelium membrane, is also represented by glucose transporter proteins (GLUTs), GLUT1, and GLUT3 (McEwen and Reagan, 2004).
The approaches to achieve effective CNS drug concentrations can be invasive (temporary break of tight junctions, intracerebral injection, or use of intracerebral implants such as catheters, microchips, or erodible polymeric systems). It still comes cost-effective and potentially dangerous for patients, since the direct delivery of the drug exposes patients to the risk of developing serious brain infections with a consequent decrease in their compliance. Starting from these assumptions, the non-invasive (chemical, biological, or technological) approach is the hope to treat the brain pathologies (Scherrmann, 2002; Tosi et al., 2011).
In the last years, the use of nanoparticles (NPs) represents a powerful approach to improve the BBB penetration of bioactive molecules; NPs have small size (3 ÷ 200 nm) and good stability, and they can be easy functionalized by surface modifications (attaching active ligands or changing the superficial charge), offering good advantages over the free drug. The NPs can reduce the side effects on healthy cells, improving the pharmacokinetic and pharmacodynamics drug profile and increase drug concentration at the specific sites allowing their application in the neurodegenerative disease treatment.
Lipid Nanocarriers for Drug Delivery Across the BBB
The effectiveness of drugs acting on the CNS has strong limits, due to their inability to overcome the BBB in adequate amounts (de Boer and Gaillard, 2007). The issue of the limited drugs crossing through the BBB is of great interest to the scientific and medical community, which is focused on the execution and optimization of innovative therapeutic strategies against neurodegenerative diseases. In this perspective, the carriers with dimensions at nanoscale level represent a promising drug delivery platform solution for enhancing the drug crossing through the BBB (Chen and Liu, 2012; Pardridge, 2012; Upadhyay, 2014). Theoretically, the tuneable physicochemical properties of nanocarriers permit optimizing the delivery of different kinds of drugs at the specific therapeutic target; nevertheless, a careful design of drug nanocarriers needs to consider many factors, such as biocompatibility, reduction of drug toxic effects, enhancement in drug stability, and optimization of the specific target functionalization. Furthermore, the engineering of such nanocarriers become harder when the therapeutic target is the CNS, as listed by Lockman and colleagues (Lockman et al., 2002).
The growing interest in the suitable drug delivery systems research has encouraged the production of a wide range of nano-scaled carriers (Mishra et al., 2010). Among these, lipid-based carriers are recognized as promising strategies for drug delivery, due to their ability to mimic natural lipid environment constituting the biomembranes, including BBB (Pujara, 2012). In addition, lipid vectors protect the drug from degradation during transport to the target sites, reduce the toxicity, and increase the drug biocompatibility with respect to free administration (Chen et al., 2010; Nunes et al., 2017).
Among different lipid carriers, liposomes (LPs) have been extensively studied for nanomedicine applications (Fenske and Cullis, 2008; Fenske et al., 2008). The mechanism transport across the BBB is still unclear (Agrawal et al., 2017). Initially, it was thought that the liposomes spontaneously crossed several biological membranes including BBB by electrostatic interactions. However, this theory was replaced by the possible mechanism through active transport carriers by transcytosis or a specific receptor (Spuch and Navarro, 2011). The latter hypothesis is more consolidated due to the binding of specific molecules, such as glucose and glutathione, on liposome surfaces that are able to overcome the BBB promoting the liposome translocation (Noble et al., 2014).
Their biochemical architecture (Figure 2) is similar to the human cellular membrane; in fact, it is formed by phospholipid double layers that enclose an aqueous core. In these artificial vesicles, with spherical shape, lipophilic and hydrophilic drugs can be encapsulated: instead of lipophilic molecules only confined into phospholipidic bilayers, hydrophilic molecules can be loaded both inside the aqueous cores of LPs and in the external water phase. The encapsulation efficiency is almost equal to the loading rate in the case of lipophilic molecules, whereas it depends on composition and the LP synthetic approach method in the case of hydrophilic drug (Johnsson and Edwards, 2003). However, in any case it was found that drug toxicity is strongly reduced following internalization in the LP.
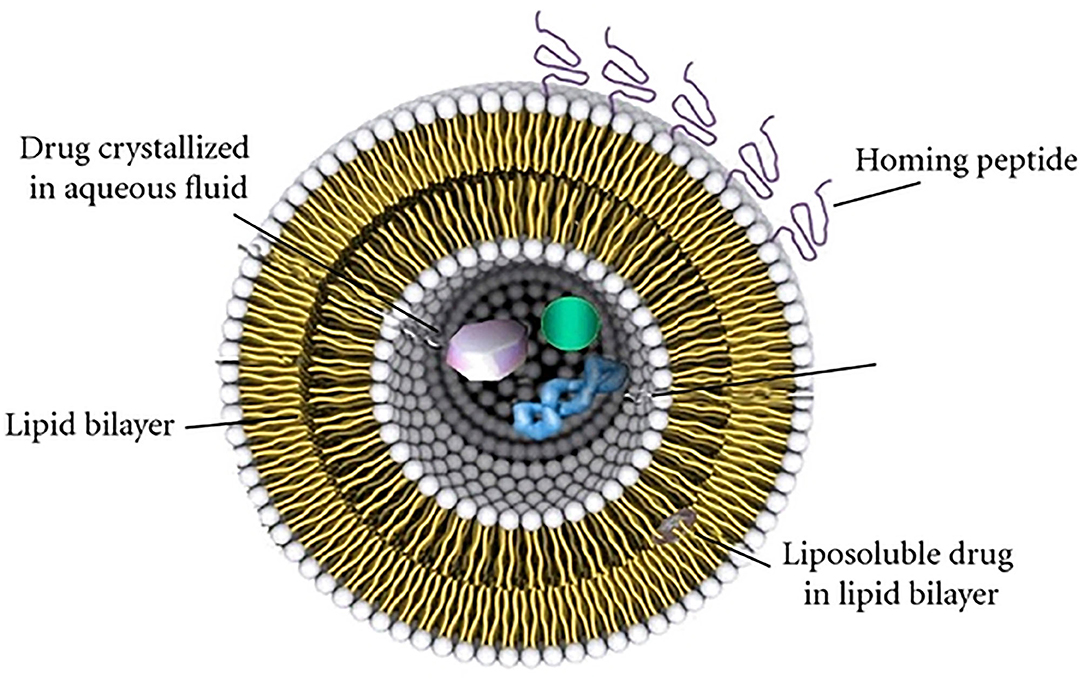
Figure 2. Representation of fundamental architecture of LPs. Reproduced with permission from Bitounis et al. (2012).
Conventionally, a first classification of LPs is based on the number of bilayers (lamellarity) and on the size of the structure (Woodle and Papahadjopoulos, 1989) because these two parameters deeply influence both the circulation half-life and the drug encapsulation efficiency. Small unilamellar vesicles (SUVs) and large unilamellar vesicles (LUVs) indicate LPs formed from a single layer with a dimension of (25 ÷ 50) nm or (200 ÷ 800) nm, respectively, while multilamellar vesicles (MLV) are referred to LPs formed by concentric multiple lipid layers, separated from each other by an aqueous solution. Most specific classifications regard the chemical composition, lipid organization, surface charge, or preparation methods (Euliss et al., 2006; Bozzuto and Molinari, 2015).
For the drug delivery aim, the LPs appear a promising system, not only for their ability to protect their cargo to enzymes degradation phenomenon, but also for their unique properties, including low toxicity, flexibility, biocompatibility, biodegradability, and non-immunogenicity. Unfortunately, LPs suffer of some limitations for specific therapeutic applications, namely shelf life and the drug-loading efficiency. In the last decades, several efforts have been made toward the ingegnerization of optimal LPs, and new categories of liposomal carriers were obtained.
Solid lipid nanoparticles (SLN) have established themselves as an alternative system to conventional LPs, due to significant advantages: controlled and targeted drug release, high stability, and effective encapsulation efficiency of drugs. The SLNs structures consist of a solid lipid matrix (tri-, di-, and mono-glycerides, fatty acids, steroids, or waxes) that outlines an aqueous volume in which lipophilic and hydrophilic molecules can be internalized (Uner and Yener, 2007). However, some of the drugs could flow out during the transport following the natural recrystallization process of the SLN shell, formed by a single lipid. For that reason, the delivery efficacy results are jeopardized by the SLN architecture (Blasi et al., 2007).
With the aim to overcome these drawbacks, the nanostructured lipid carriers (NLC) were conceived. The matrix of the NLC structure is composed by a combination of solid and liquid lipids. This latter induces the nanostructuring process into the inner lipid shell. These results allow the increase of the volume available for drug loading and the boost of a more stable lipid configuration (Iqbal et al., 2012; Beloqui et al., 2016).
Several synthetic routes have been proposed to obtain SLN and NLCs; among these, high-pressure homogenization (HPH) and microemulsion are the most efficient. The microemulsion technique requires the use of hazardous toxic organic solvents; for this reason, the lipidic systems obtained through this procedure are unsuitable for medical purposes. In this perspective, the use of HPH procedure is preferred; however, this technique permits the internalization only of thermostable drugs (Das and Chaudhury, 2011).
The SLN/NLCs systems have been largely employed in vivo for the delivery of several kinds of drugs, by intranasal, intragastric, oral, and intravenous administrations. In order to optimize the carriers for dermal delivery, a new class of LPs has been proposed by Touitou and co-workers (Touitou et al., 2000). They modified LPs, adding ethanol, in order to obtain high efficiency in the delivery of different drugs through the skin.
These modified LPs (Figure 3), called ethosomes, are lipid vesicular systems composed primarily of phospholipids, water, and ethanol. This latter, added in high concentration (20 ÷ 45 %), interacts with the polar heads of the phospholipids; finally, it is inserted in the lipid stratum corneum, lowering the melting-point of lipidic bilayer. This last phenomenon makes them to be more fluid and permeable for the cell membrane. In addition, in comparison with LPs, ethosomes have small dimensions due to the negative superficial charge induced by ethanol inclusion.
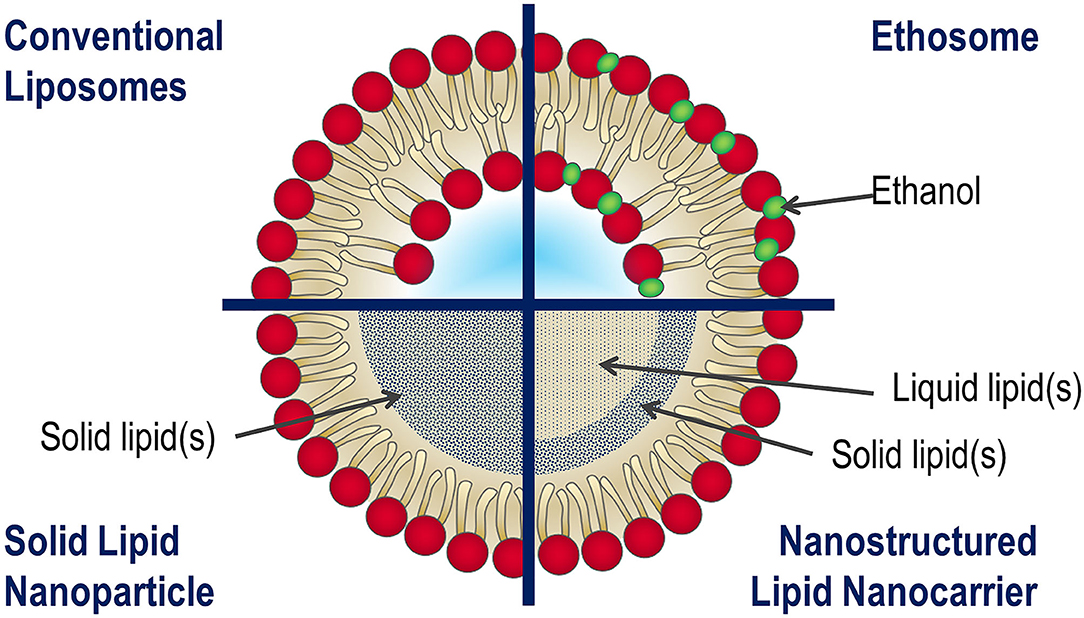
Figure 3. Schematic representation of main structure of LPs, ethosome, solid lipid nanoparticles (SLNs), and nanostructured lipid carriers (NLCs).
The improved transport mechanism of bioactive molecules through the skin can be applied to the drug delivery to the CNS; in fact, the characteristics of the ethosomes could be useful to promote the BBB penetration.
Therefore, the improvement of the liposomal delivery systems has required the optimization of the physiochemical properties of the liposomal surface in relation to the chemical nature of entrapped drug molecules.
Conventional Therapeutic Approach in Liposomal Formulation Against ND Progression
Currently, the treatment of ND pathologies is still not available; however, many molecules and compounds can mitigate the symptoms and slow down the ND progression (Duraes et al., 2018). The first therapeutic approaches were based on the administration of small hydrophilic molecules (levodopa, rivastigmine, tacrine, donepezil), which can penetrate the BBB through the lipid-mediated diffusion process, exerting neuroprotective effects in several ND models. In recent years, the attention of medical and scientific communities has been focused on natural compounds, to overcome the side effects induced by chemical drug treatments against ND disorders. Due to anticholinesterase and the antioxidative and anti-inflammatory outcomes of different phytochemicals, these could be promising therapeutic agents against ND progression. In detail, the neuroprotective and anti-protein aggregation effects induced by curcumin, quercetin, and resveratrol were extensively investigated.
Despite numerous therapeutic agents showing promising results for ND treatments, the experimental evidences obtained in vivo fell short of expectations; in fact, the efficacy of all these molecules is strongly limited due to the low bioavailability and low penetration capability in BBB. In addition, the phytochemicals compounds are poorly water and blood soluble and highlighted a short biological half-life as well as low uptake in the brain (Darvesh et al., 2012; Yang et al., 2014; Kulkarni and Canto, 2015; Tellone et al., 2015; Ay et al., 2017).
The advances in the nanotechnological field have enabled the development of engineered nanocarriers to deliver drugs and to release them in a controlled manner in specific body compartments. In particular, the LPs have become the best theranostic system for anti-ND molecules, since they allow them to overcome their inherent limitations (Table 1).
In this section, we describe how LP-based formulations improve the delivery to the brain of conventional therapeutic drugs.
Solubility, Bioavailability, and Stability
The bioavailability of given administered drug depends by several factors, such as the aqueous or blood solubility, BBB permeability and metabolic stability (Helen Chan and Stewart, 1996). Rivastigmine and Donepezil are small hydrophobic molecules largely used in Alzheimer's treatment, because they act as acetylcholinesterase inhibitor, preventing the acetylcholine degradation and increasing its availability at the synapses (Di Stefano et al., 2011).
In order to improve the bioavailability of these drugs: Nageeb El-Helaly et al. (2017) fabricated electrosteric stealth (ESS) LPs in which rivastigmine was encapsulated; meanwhile, Al Asmari et al. (2016) prepared a new liposomal formulation using 1,2-distearyl-sn-glycero-3-phosphocholine, cholesterol, and polyethylene glycol to entrap Donepezil. In details, Nageeb El-Helaly and co-workers (Nageeb El-Helaly et al., 2017) obtained an augmented stability by steric hindrance due to addition of didecyldimethyl ammonium bromide and 1,2-distearoyl-sn-glycero-3-phosphoethanolamine-N-(methoxy (polyethyleneglycol)-2000) to l-α-lecithin from soybean; consequently, the in vivo studies performed on 36 rabbits showed that the bioavailability of rivastigmine strongly increased in plasma and brain compartments after intranasal injection. Analogously, the Donepezil bioavailability resulted two times higher in the brain, after intranasal administration, compared to free drug in plasma (Al Asmari et al., 2016).
Among phytochemical compounds, curcumin induces neuroprotective and anti-protein aggregation effects. The low water solubility and bioavailability can be enhanced by internalization into liposomal carriers: Cheng et al. (2017) optimized a pH-driven method to prepare curcumin-loaded LPs (Figure 4), testing their bioavailability by the reproduction of gastrointestinal tissue. They found an increased bioavailability (more than twofold) with respect to curcumin solution.
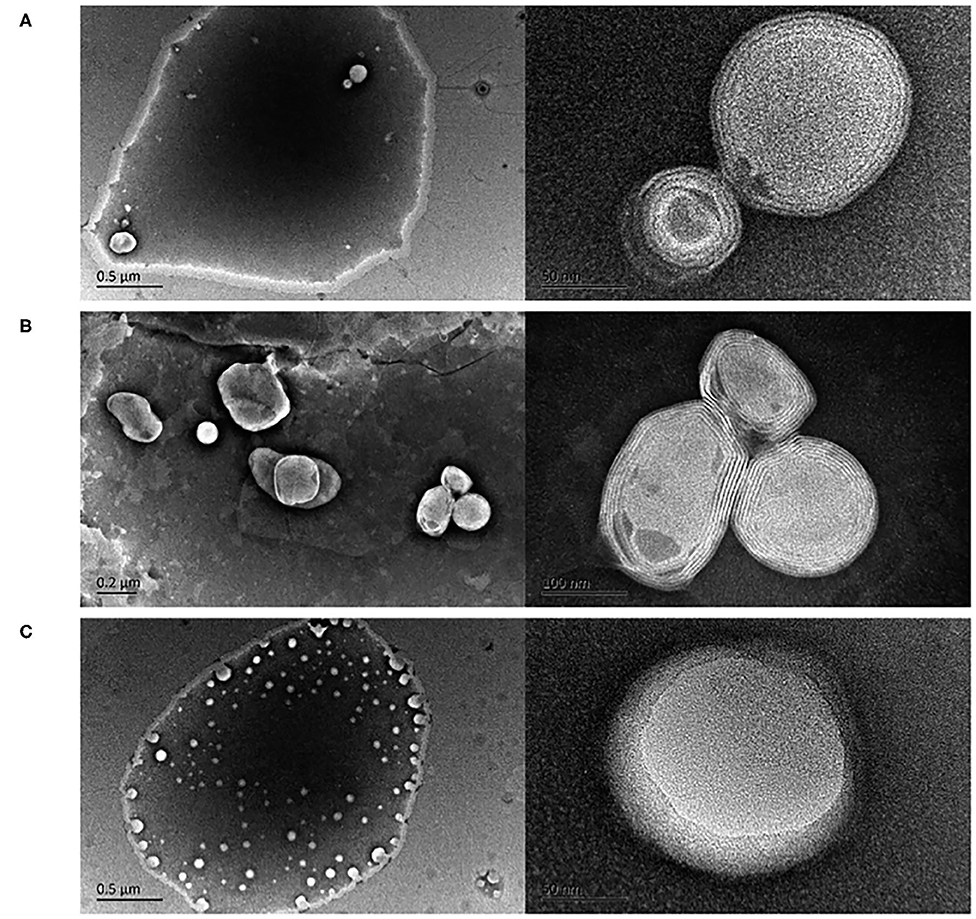
Figure 4. Transmission electron microscopy images of curcumin LPs produced by the (A) pH-driven method, (B) thin film method, and (C) ethanol injection method. Reproduced from Cheng et al. (2017) with permission of The Royal Society of Chemistry.
Bulboaca et al. (2018) investigated the therapeutic potential of curcumin in addition to the traditional sumatriptan treatment for migraine disease using an in vivo rat model, demonstrating that, after intravenous administration, the shelf life of curcumin largely increased when it was encapsulated in liposomal vectors.
The polyphenolic compound resveratrol (3,4,5-trihydroxystilbene) gained increased attention as a therapeutic agent against ND diseases, due to their ability to promote the non-amyloidogenic cleavage of the amyloid precursor proteins, to improve β amyloid-peptide clearance and to limit neuronal injury (Li et al., 2012). As well as other natural compounds, the encapsulation in liposomal systems augments the drug effectiveness.
The enhancement in resveratrol bioavailability was demonstrated by Neves and collaborators by in vitro (Neves et al., 2013) and in vivo experiments (Jose et al., 2014), loading it in SLNs and NLCs. The in vivo biodistribution study using Wistar rats demonstrated that SLN could significantly increase the brain concentration of resveratrol (17.28 ± 0.6344 μg/g) as compared to non-liposomal formulation (3.45 ± 0.3961 μg/g). In pharmacokinetic studies conducted in male Wistar rats, Pandita et al. (2014) showed that the oral bioavailability of resveratrol was increased 8.035-fold when loaded in stearic acid-based SLNs stabilized by mixture of surfactants PHOSPHOLIPON® 90G/poloxamer 188.
In light of these experimental evidences, it is undeniable that encapsulation in liposomal carriers may offer interesting opportunities to enhance the delivery of anti-ND drugs.
Therapeutic Efficacy and Drug Toxicity Reduction
The encapsulation of anti-NDs drugs in liposomal-based carriers permits to minimize their adverse effects and simultaneously to enhance their therapeutic action.
Among small hydrophobic molecules, Levodopa (L-dopa, L-3, 4-dihydroxyphenylalanine) is the most used molecules in ND treatment; in particular, in the last 4 decades Levodopa (L-DOPA)-based therapy become the golden standard therapy against Parkinson disease (Poewe et al., 2010). After oral administration, <10% of L-DOPA dose reaches the CNS, while the remaining 90% tends to accumulate into the liver and muscles, inducing dyskinesia (Dodel et al., 2001). These adverse effects can be reduced loading L-DOPA in chitosan-coated nano-LPs. Chitosan is a natural hydrogel cationic polysaccharide of co-polymers glucosamine and N-acetyl glucosamine obtained by alkaline deacetylation of chitin that is constitutive of crustacean shells. In addition, it shows a positive charge that can be exploited to form polyelectrolyte vesicular complexes able to act as delivery systems (Elieh-Ali-Komi and Hamblin, 2016). Cao and colleagues performed in vivo studies on rats, demonstrating that the abnormal involuntary movement rate significantly decreased in rats treated with chitosan-coated L-DOPA NLs than those who received free L-DOPA (Cao et al., 2016).
In the case of phytochemical compounds, the ND synthoms became more attenuated after the administration of curcumin and quercetin encapsulated in liposomal carriers with respect to free formulations. In details, in vivo experiments conducted on a rat model by Sadegh Malvajerd et al. (2019) showed a reduction in inflammatory state due to increased anti-oxidant activity after intravenous administrations of encapsulated curcumin into SLN and NLC carriers with respect to free drug; in addition, the pharmacokinetic studies demonstrated that the NLC option ensures higher accumulation into rat brain. Another interesting approach was suggested by Kuo et al. (2017) proposing the combined use of curcumin (CRM) and nerve growth factor (NGF) conjugated with cardiolipin (CL) to treat Alzheimer disorders. In details, the human neuroblastoma (SK-N-MC) cell line insulted with β-amyloid peptide (Aβ) shows how treatment with curcumin CRM-CL LPs prevented the neurodegeneration process through the inhibition of the p38, JNK, and tau protein phosphorylation. Meanwhile, the exposure to NGF-CL/LIP up-regulated the expression of the p-TrkA and p-ERK5, responsible to ensure neuronal viability. The effects of the combined use of these LPs were assessed in Wistar rat models, after intraperitoneal injections. To improve the permeation rate across the BBB, CRM-CL and CRM-CL LPs were implemented with germ agglutinin (WGA). The combined action of WGA-grafted CRM-CL and CRM-CL strongly affected the Aβ plaque formation, increasing the percentage of healthy neurons and promoting the cholinergic activity in the rat hippocampus.
Sokolik et al. compared the efficacy of intranasal administrated curcumin against Alzheimer's disorder in soluble form with liposomal formulations. After neuroinflammatory quantification analysis, they performed cognitive tests on rats to evaluate the impact of curcumin exposure on mnestic functions. They evaluated the impact on the angiotensin converting enzyme activity and cytokine productions in the damaged rat brain. Treatment with CLs reduced cytokines (interleukin-1β, interleukin-6, interleukin-10, tumor necrosis factor α) and angiotensin expression in cerebral cortex and hippocampus area. Finally, the cognitive tests show a reduction in the latent period of conditioned reflex reactions (Sokolik and Shulga, 2016; Sokolik et al., 2016, 2017).
Sarkar and Das have reported that, conversely to free formulation, quercetin loaded in mannosylated LPs and administrated via carotid injection in male Sprague-Dawley rats, in which the ischemia event was induced, meaningfully sustained antioxidant enzyme activity, preventing edema formation (Sarkar and Das, 2006). In addition, Das et al. demonstrated the oral administration of LP-encapsulated quercetin enhanced the antioxidative enzyme activity in adult female Swiss albino rat brains neurodamaged after arsenic exposure (Ghosh et al., 2009). Phachonpai and colleagues (Phachonpai et al., 2010) treated male Wistar rats, used as Alzheimer's disease model, with quercetin in LPs via intranasal administration. After treatment (0.5 mg/daily for 2 weeks), the neuroprotective quercetin action was recorded: the activities of superoxide dismutase, catalase, and glutathione peroxidase increased while the malondialdehyde levels were decreased. The reduction of oxidative stress confirmed the effectiveness of quercetin against Alzheimer's disease. The extension of the time treatment until 4 weeks increased the cholinergic neuron viability in hippocampus and ameliorated cognitive performances (Terdthai et al., 2010).
In the last years, the combination of resveratrol in LPs was explored as potential strategy for ND treatment. Ethemoglu et al. (2017) demonstrated how the resveratrol administration influenced the incidence of epileptic events in rats, in which brain ND disorder was induced by penicillin administration. The treatment with resveratrol reduced the epileptic activity, probably due to the reduction of oxidative stress at the cellular level. This rate further decreased when resveratrol was encapsulated in liposomal carriers.
Wang et al. (2013) assessed the impact of resveratrol derived from Polygonum cuspidatum on rats in which Parkinson's outcome was induced. After 14 days of oral treatment, the apoptosis rate of nigral tissue cells and ROS level was decreased while the antioxidant capability increased. These effects were strongly augmented when resveratrol was administered in liposomal vectors, probably due to the enhanced bioavailability.
Permeability
The main limitation of anti-ND drug efficacy is their poor BBB penetration capability, in fact <98% of the small molecules and nearly 100% of large molecules are able to reach the brain. The encapsulation in liposomal-based carriers can enhance the bioavailability in brain of therapeutic agents; for example Aramugam et al. (2008) treated Wistar rat with rivastigmine encapsulated in conventional multilamellar LPs: after intranasal injection, the rivastigmine level reveled in brain tissue resulted in higher respect to free drug. However, in order to improve the uptake of anti-ND drugs, active targeting strategies have been developed. The chemical modification of LP surfaces essentially consists in attaching ligands which interact with proteins expressed by BBB.
Yang et al. (2013) produced CCP LPs using cell-penetrating peptide (CPP), with the aim to enhance the brain distribution of rivastigmine. In detail, they proceeded by an intranasal administration in order to minimize the collateral effects (including hepatic first pass metabolism and gastrointestinal adverse outcomes), enhancing the permeability of the CPP-LP complex across both the nasal olfactory pathway and the murine BBB. Relative to the extended release and absorption, an intense inhibition of acetylcholinesterase and butyrylcholinesterase activities was detected.
Among receptor-mediated transcytosis, the transferrin (Tf) receptors are the one of the most explored as targets of the drug delivery system (Sharma et al., 2016). In particular, several in vitro and in vivo studies were performed in order to improve the targeting efficacy of therapeutic molecules across BBB (Pulgar, 2018).
Mourtas et al. (2014) produced a curcumin derivative in nanosized LPs functionalized with the anti-Tf antibody to direction them against Aβ protein in amyloid plaques. The potential efficacy of curcumin-derivative LPs and curcumin-derivative anti-Tf LPs was assessed in ex vivo experiments performed on post-mortem extracted brain samples from patients affected by Alzheimer disease. Both LP types exhibited high binding affinity with Aβ proteins; indeed, the thioflavin assay confirmed their ability to slow down the Aβ1-42 peptide aggregation. Although the efficacy of examined carriers was comparable, the anti-Tf receptor-decorated LPs strongly enhanced the brain permeation.
With the aim to improve the efficacy of the neuroprotectant ZL006 against ischemic stroke, Wang and colleagues (Wang et al., 2015) encapsulated it in modified LPs with HAIYPRH (T7) peptide, which directly interacts with TfR. The in vivo experiments performed on rat models (Sprague-Dawley and ICR mice) revealed that the T7 functionalization considerably improved the penetration across the BBB. This implied a reduced infarct volume and enhanced neurological deficit with respect to the untargeted LPs or SKfree ZL006.
The Tf functionalization is currently used to improve BBB targeting and transcytosis. In order to enhance the neuronal transfection in gene therapy, Dos Santos Rodrigues et al. (Dos Santos Rodrigues et al., 2018) conceived LPs with double functionalization: Tf and penetratin (Pen); the latter enabled overcoming receptor saturation and increasing the number of internalized LPs. Hydrophilic plasmid for β-galactosidase (pβgal), used as a gene model, were loaded in Pen-Tf LPs in order to explore the efficacy in gene therapy applications. After injection in C57BL/6J mice, the plasmid biodistribution was quantified in different tissues: the liposomal formulation involved a significant increase in βgal amount in brain, liver, and kidneys with respect to the endogenous levels. In addition, the Pen-Tf functionalization improved the transfection capability only in the brain.
α-Mangostin is a polyphenolic xanthone, largely used in medicine; recently, it was demonstrated its capability to slow down AD progression (Wang et al., 2012); nevertheless, its efficacy is limited by the low penetration capability across the BBB. Moving from these evidences, Chen and colleagues (Chen et al., 2016) modified LPs with Tf in order to enhance the penetration rate of α-Mangostin in brain. α-M was intravenously administrated (5 mg/kg) into the Sprague Dawley rats in different formulations: α-M solution, α-M LP, and Tf(α-M) LP. The pharmacokinetic experiments revealed that encapsulation in Tf LPs augmented the α-M bioavailability in plasma and drug accumulation rate in brain tissue. The authors proposed Tf-LPs as a promising drug delivery system, demonstrating their ability to improve the brain permeation of α-M; however, α-M distribution quantification in different body compartments showed that Tf(α-M) LPs tended to accumulate also in liver tissue; this is due to the expression of TfR on hepatocytes.
The fact that Tf receptors were expressed on several cellular types, including hepatocytes, monocytes, erythrocytes, intestinal cells, epithelial cell of choroid plexus, and neuron besides BBB, represents an important issue in targeting of delivery systems. In addition, the efficiency of the Tf targeting could be dramatically compromised by the physiological presence of high levels of endogenous Tf in the plasma, which saturated the TfR endothelial cells (Sharma et al., 2016).
Like TfR, low-density lipoprotein (LDL) receptors, involved in the specific BBB transport mechanisms, could be exploited in the increase of the drug rate permeation in brain. In particular, human apolipoprotein E (apoE) plays a key role in lipid transport in the CNS (Fan et al., 2001; Hatters et al., 2006). In addition, biological membranes contain a great variety of phospholipids; one among them is phosphatidic acid (PA), which has a special attraction for Aβ and may help rescue neurons from Aβ-induced toxicity. Drug carriers associated with PA and ApoE may improve the affinity to binding to Aβ in vitro (Re et al., 2011; Salvati et al., 2013).
Starting from these evidences, Bana et al. (2014) used a Balb/c mice model to test the uptake in brain of modified LPs mApoE-PA-LPs, obtained by functionalizing PA and a synthetic peptide (mApoE; CWGLRKLRKRLLR) that includes the apolipoprotein-E receptor-binding domain. The biodistribution experiments, performed after intravenous administration, demonstrated that mApoE-PA-LPs were more capable of penetrating the BBB respect to PA-LPs.
The apolipoprotein E (ApoE) conjugated with PA was also used by Kuo and co-worker (Kuo et al., 2018) to functionalize LPs (ApoE-PA-LP) able to deliver quercetin and rosmarinic acid to brain, in order to inhibit β-amyloid (1–42) (Aβ1-42) aggregation. The permeation capacity strongly increased in functionalization case respect to free drugs and drugs encapsulated in undecorated LPs. The administration of quercetin and rosmarinic acid in ApoE-PA-LP formulation induced in Aβ-insulted SD rats the diminishment of the acetylcholinesterase activity and the reduction in Aβ plaque formation.
Recently, other potential targeting molecules have been explored to improve the drug internalization exploiting the receptor-mediated transcytosis process. In detail, the nutrient transporters can be used for brain delivery. Among these, glutathione (GSH) is an endogenous tripeptide able to penetrate the BBB as sodium-dependent transporter; this latter is preferentially expressed in CNS and BBB of all mammalian species (Smeyne and Smeyne, 2013; Kuo et al., 2018).
Rip and co-workers fabricated PEGylated LPs with GSH, and they investigated the potential improvement in brain uptake of drug encapsulated in this carrier; for this purpose the authors loaded a fluorescent tracer (carboxifluorescein) in the PEG-GSH modified LPs (Rip et al., 2014). Quantification of carboxifluorescein in brain was obtained by microdialysis experiment on Wistar rats, in which this fluorescent molecule was intravenously administrated in three different forms: free, non-targeted PEG LPs, and GSH-PEG LPs. The results showed that the carboxifluorescein level in brain increased in liposomal formulation; in particular, the GSH-PEG LP option made this level fourfold higher with respect to undecorated PEG LPs. The efficiency of GSH-PEG LP delivery in the brain was also tested for ribavirin drug (Rip et al., 2010; Maussang et al., 2016). The concentration of drug in brain tissue increased in modified LP formulation in Wistar rats after administration by intravenous route; the permeation rate beyond the BBB became proportional to the amount of GSH coating (ranging from 0 to 2%).
In addition, in literature several works reported how the GSH-PEG LP formulation improves in brain delivery of the opioid peptide DAMGO (H-Tyr-d-Ala-Gly-MePhe-Gly-ol) (Lindqvist et al., 2013), the anti-amyloid single-domain antibody fragment (Rotman et al., 2015), flucytosine (Salem et al., 2015), methylprednisolone hemisuccinate (Kanhai et al., 2018), etc.
Another promising strategy to improve penetration capability of anti-ND drug is based on the use of cationic polyelectrolyte in LP preparation. Cationic LPs (CLs) can penetrate the BBB through adsorptive-mediated endocytosis process (Herve et al., 2008). This occurs because the surface of CLs exhibits a positive charge at physiological pH; thus, it electrostatically interacts with the polyanions present in the BBB (Miller et al., 1998).
This event was examined in vivo on Sprague–Dawley rats, in which cationic, anionic, and charge-neutral LPs were directly injected into carotid arteries. Joshi et al. reported that superficial charged LPs more actively interact with BBB endothelial cell respect to neutral LPs; in particular, the authors recorded the highest cationic concentration with respect to the anionic LPs into the brain tissue (Joshi et al., 2014a,b; Joshi et al., 2015).
Migliore et al. (2010) stated that the use of CLs as a delivery system enhanced the brain uptake of drug administered by intranasal route in Sprague–Dawley rats.
Recently, another work (Dhaliwal et al., 2020) reported how it is possible to take advantage of cationic LPs to improve the mRNA delivery. After optimization of the liposomal formulation, mRNA was administered by intranasal route to CD-1 mice. Through the GFP and luciferase reporter systems, the authors have quantified the mRNA biodistribution in vivo. The findings demonstrated the cationic LPs improved the penetration rate of mRNA and its accumulation into specific cerebral areas (cortex, striatum, and midbrain).
Although it was demonstrated that the CL formulation enhanced the anti-ND drug penetration rate in the brain, it must be admitted that this rate is limited by non-specific uptake phenomena in peripheral tissues. In addition, the superficial charge of LPs could be reduced by interaction with serum protein before reaching the BBB (Tagalakis et al., 2014).
These issues could be overcome by increasing the amounts of use of CLs, but keeping in consideration their cytotoxic potential.
Administration Routes
The main challenge in the treatment of CNS diseases is represented by the presence of the BBB, which makes complicated the delivery of therapeutic agents into the brain. At first, invasive approaches have performed in order to overcome the BBB, including the neurosurgery-based cerebral infusions or implants and the physical or chemical disruption of the BBB to permit the penetration of drug via osmotic shift (Chen et al., 2004). However, these techniques are highly dangerous carry risks of infection and brain tissue damage, and are able to induce chronic neuropathological effects in treated patients (Chen et al., 2004). In recent decades, several non-invasive strategies, based on physiological brain transport mechanisms, have been developed.
As already described in this review, the drug loading in LPs increases its own uptake in the brain; later on, once the importance of LP usage was proven, various studies have tried several routes of administration for finding out if they are more or less adequate.
The intravenous routes are certainly the most common, preferred to the oral route, because in this latter case there is a loss in terms of drug percentage in the gastrointestinal ambient: drug is degraded by the stomach fluids and poorly absorbed by the intestine, while increasing the hepatic and renal load; although in different reported works, it has been proven that the drug loading in LPs prevent the drug degradation. Although the intravenous injection is the most applied, the interest in alternative parenteral routes increased, for finding new direct administrations to the BBB. Among these, the intranasal way gained a noticeable increase by the scientific community, due to its ability to let the drug reach the brain via different pathways: direct ones, through olfactive epithelium, thanks to the presence of olfactory nerves; or indirect ones, through the respiratory epithelium first and the circulatory system after (Hong et al., 2019).
In the last decade, there is a growing interest of administering them via intradermal routes. In this perspective, ethosomes represent the better choice among liposomal systems, due to their ability to increase the drug permeation across the derma. For instance, Ropinirole hydrochloride is commonly used in Parkinson disease. With the aim to evaluate the possibility to administrate Ropinirole hydrochloride via transdermal route, Mishra et al. (2013) synthetized different ethosomal carriers, varying ethanol and lecithin concentrations. The experimental in vivo study showed that RHC encapsulated in ethosomes (30 and 4% w/v of ethanol and lecithin, respectively) increased their blood circulation time, becoming comparable to the oral administration case.
Flurbiprofen is largely used in ND treatments for its anti-inflammatory effect. The permeation of ethosomal–flurbiprofen (EF) formulations was assessed in the albino rat model (Paliwal et al., 2019). The reported results showed a high encapsulation drug efficiency (95 %); in addition, the permeation efficacy was equal to (82.56 ± 2.11) g/cm2 in 24 h, and transdermal flux was found as 226.1 μg/cm2/h.
Ligustrazine phosphate (LP) is an antioxidant used for the Alzheimer disorder. Shi and co-workers (Shi et al., 2012) optimized the ethosome-based carrier for LP transdermal delivery; in addition, they evaluated the therapeutic impact on rats, in which amnesia disease was induced by scopolamine. Experimental evidence indicated that the penetration ability of the LP ethosomal system was strongly higher than the LP in aqueous solution. Moreover, the LP ethosomal treatment normalized the levels both of antioxidant enzymes and the lipid peroxidation in a healthy rat model.
These in vivo evidences suggested the goodness of ethosome-based strategy; nevertheless, for ND disease, only a few studies explored the potential of dermal administration ethosome-mediated.
Conclusions and Perspective
In the last decades, many efforts have been made to develop an optimal therapeutic strategy against neurodegenerative disorders due to their dramatic incidence worldwide. In this perspective, several new molecules including phytochemical extracts were investigated along with the conventional drugs. Unfortunately, their therapeutic potential was strongly compromised owing to the presence of high selective barrier (BBB) that protects the brain from treatment (CNS).
The effectiveness of drugs against ND diseases increases when they are encapsulated into liposomal-based vectors.
Generally, LPs represent a promising platform for theranostic medicine purposes, thanks to their size, biocompatibility, high biological affinity, immunogenicity, biodegradability, and low toxicity. In addition, LPs have a good ability to deliver both hydrophobic and hydrophilic molecules through BBB.
In this review article, the recent state of the art about the use of liposomal-based carriers for anti-neurodegenerative drug delivery and the in vivo applications are extensively examined. In the reported researches, the enhanced efficacy of drug following liposomal encapsulation has been demonstrated, in terms of improved bioavailability, stability, and permeation capability across BBB.
Albeit a great deal of progress has been made in liposomal formulations and their efficiency was demonstrated in several in vivo studies, some issues are still present.
The scientific community is called to perform further clinical trials in order to evaluate the LP accumulation effects induced by long-term exposure and, simultaneously, to optimize the ingegnerization of these carriers in the human body.
Author Contributions
MC and VDM: conceptualization and writing (original draft preparation). MC, VDM, SL, and RR: review and editing manuscript. SL and RR: funding acquisition.
Conflict of Interest
The authors declare that the research was conducted in the absence of any commercial or financial relationships that could be construed as a potential conflict of interest.
Acknowledgments
MC and VDM kindly acknowledge Programma Operativo Nazionale (PON) Ricerca e Innovazione 2014-2020 Asse I Capitale Umano, Azione I.2, Avviso ‘A.I.M: Attraction and International Mobility [F88D18000070001] [CUP F88D1800060001]. SL has been partially supported by Progetto Fondo Integrativo Speciale per la Ricerca (FISR-CNR) Tecnopolo di Nanotecnologia e Fotonica per la Medicina di Precisione [CUP B83B17000010001].
References
Abbott, N. J., Patabendige, A. A., Dolman, D. E., Yusof, S. R., and Begley, D. J. (2010). Structure and function of the blood-brain barrier. Neurobiol. Dis. 37, 13–25. doi: 10.1016/j.nbd.2009.07.030
Abbott, N. J., and Romero, I. A. (1996). Transporting therapeutics across the blood-brain barrier. Mol. Med. Today 2, 106–113. doi: 10.1016/1357-4310(96)88720-X
Abeliovich, A., and Gitler, A. D. (2016). Defects in trafficking bridge parkinson's disease pathology and genetics. Nature 539, 207–216. doi: 10.1038/nature20414
Agrawal, M., Ajazuddin, T. D. K, Saraf, S., Saraf, S., Antimisiaris, S. G., Mourtas, S., et al. (2017). Recent advancements in liposomes targeting strategies to cross blood-brain barrier (bbb) for the treatment of alzheimer's disease. J. Control. Release 260, 61–77. doi: 10.1016/j.jconrel.2017.05.019
Al Asmari, A. K., Ullah, Z., Tariq, M., and Fatani, A. (2016). Preparation, characterization, and in vivo evaluation of intranasally administered liposomal formulation of donepezil. Drug Des. Devel. Ther. 10, 205–215. doi: 10.2147/DDDT.S93937
Alexander, G. E. (2004). Biology of parkinson's disease: Pathogenesis and pathophysiology of a multisystem neurodegenerative disorder. Dialogues Clin. Neurosci. 6, 259–280.
Angeles, D. C., Ho, P., Chua, L. L., Wang, C., Yap, Y. W., Ng, C., et al. (2014). Thiol peroxidases ameliorate lrrk2 mutant-induced mitochondrial and dopaminergic neuronal degeneration in drosophila. Hum. Mol. Genet. 23, 3157–3165. doi: 10.1093/hmg/ddu026
Arumugam, K., Subramanian, G. S., Mallayasamy, S. R., Averineni, R. K., Reddy, M. S., and Udupa, N. A. (2008). Study of rivastigmine liposomes for delivery into the brain through intranasal route. Acta Pharm. 58, 287–297. doi: 10.2478/v10007-008-0014-3
Ay, M., Luo, J., Langley, M., Jin, H., Anantharam, V., Kanthasamy, A., et al. (2017). Molecular mechanisms underlying protective effects of quercetin against mitochondrial dysfunction and progressive dopaminergic neurodegeneration in cell culture and mitopark transgenic mouse models of parkinson's disease. J. Neurochem. 141, 766–782. doi: 10.1111/jnc.14033
Bana, L., Minniti, S., Salvati, E., Sesana, S., Zambelli, V., Cagnotto, A., et al. (2014). Liposomes bi-functionalized with phosphatidic acid and an apoe-derived peptide affect abeta aggregation features and cross the blood-brain-barrier: implications for therapy of alzheimer disease. Nanomedicine 10, 1583–1590. doi: 10.1016/j.nano.2013.12.001
Bartels, A. L. (2011). Blood-brain barrier p-glycoprotein function in neurodegenerative disease. Curr. Pharm. Des. 17, 2771–2777. doi: 10.2174/138161211797440122
Begley, D. J. (2004). Delivery of therapeutic agents to the central nervous system: the problems and the possibilities. Pharmacol. Ther. 104, 29–45. doi: 10.1016/j.pharmthera.2004.08.001
Begley, D. J., and Brightman, M. W. (2003). Structural and functional aspects of the blood-brain barrier. Progress in drug research. Fortschritte der Arzneimittelforschung. Progr. Rec. Pharm. 61, 39–78. doi: 10.1007/978-3-0348-8049-7_2
Beloqui, A., Solinis, M. A., Rodriguez-Gascon, A., Almeida, A. J., and Preat, V. (2016). Nanostructured lipid carriers: promising drug delivery systems for future clinics. Nanomedicine 12, 143–161. doi: 10.1016/j.nano.2015.09.004
Bitounis, D., Fanciullino, R., Iliadis, A., and Ciccolini, J. (2012). Optimizing druggability through liposomal formulations: new approaches to an old concept. ISRN Pharm. 2012:738432. doi: 10.5402/2012/738432
Blasi, P., Giovagnoli, S., Schoubben, A., Ricci, M., and Rossi, C. (2007). Solid lipid nanoparticles for targeted brain drug delivery. Adv. Drug Deliv. Rev. 59, 454–477. doi: 10.1016/j.addr.2007.04.011
Bozzuto, G., and Molinari, A. (2015). Liposomes as nanomedical devices. Int. J. Nanomed. 10, 975–999. doi: 10.2147/IJN.S68861
Bulboaca, A. E., Bolboaca, S. D., Stanescu, I. C., Sfrangeu, C. A., Porfire, A., Tefas, L., et al. (2018). The effect of intravenous administration of liposomal curcumin in addition to sumatriptan treatment in an experimental migraine model in rats. Int. J. Nanomed. 13, 3093–3103. doi: 10.2147/IJN.S162087
Cao, X., Hou, D., Wang, L., Li, S., Sun, S., Ping, Q., et al. (2016). Effects and molecular mechanism of chitosan-coated levodopa nanoliposomes on behavior of dyskinesia rats. Biol. Res. 49:32. doi: 10.1186/s40659-016-0093-4
Cavaco, M., Gaspar, D., Arb Castanho, M., and Neves, V. (2020). Antibodies for the treatment of brain metastases, a dream or a reality? Pharmaceutics 12:62. doi: 10.3390/pharmaceutics12010062
Chen, C., Han, D., Cai, C., and Tang, X. (2010). An overview of liposome lyophilization and its future potential. J. Control. Release 142, 299–311. doi: 10.1016/j.jconrel.2009.10.024
Chen, Y., Dalwadi, G., and Benson, H. A. (2004). Drug delivery across the blood-brain barrier. Curr. Drug Deliv. 1, 361–376. doi: 10.2174/1567201043334542
Chen, Y., and Liu, L. (2012). Modern methods for delivery of drugs across the blood-brain barrier. Adv. Drug Deliv. Rev. 64, 640–665. doi: 10.1016/j.addr.2011.11.010
Chen, Z. L., Huang, M., Wang, X. R., Fu, J., Han, M., Shen, Y. Q., et al. (2016). Transferrin-modified liposome promotes alpha-mangostin to penetrate the blood-brain barrier. Nanomedicine 12, 421–430. doi: 10.1016/j.nano.2015.10.021
Cheng, C., Peng, S., Li, Z., Zou, L., Liu, W., and Liu, C. (2017). Improved bioavailability of curcumin in liposomes prepared using a ph-driven, organic solvent-free, easily scalable process. RSC Adv. 7, 25978–25986. doi: 10.1039/C7RA02861J
Citron, M. (2002). Alzheimer's disease: treatments in discovery and development. Nat. Neurosci. 5, 1055–1057. doi: 10.1038/nn940
Combs, B., Hamel, C., and Kanaan, N. M. (2016). Pathological conformations involving the amino terminus of tau occur early in alzheimer's disease and are differentially detected by monoclonal antibodies. Neurobiol. Dis. 94, 18–31. doi: 10.1016/j.nbd.2016.05.016
Darvesh, A. S., Carroll, R. T., Bishayee, A., Novotny, N. A., Geldenhuys, W. J., and Van der Schyf, C. J. (2012). Curcumin and neurodegenerative diseases: a perspective. Expert Opin. Investig. Drugs 21, 1123–1140. doi: 10.1517/13543784.2012.693479
Das, S., and Chaudhury, A. (2011). Recent advances in lipid nanoparticle formulations with solid matrix for oral drug delivery. AAPS PharmSciTech 12, 62–76. doi: 10.1208/s12249-010-9563-0
Dawson, T. M., Ko, H. S., and Dawson, V. L. (2010). Genetic animal models of parkinson's disease. Neuron 66, 646–661. doi: 10.1016/j.neuron.2010.04.034
de Boer, A. G., and Gaillard, P. J. (2007). Drug targeting to the brain. Annu. Rev. Pharmacol. Toxicol. 47, 323–355. doi: 10.1146/annurev.pharmtox.47.120505.105237
de Boer, A. G., van der Sandt, I. C., and Gaillard, P. J. (2003). The role of drug transporters at the blood-brain barrier. Annu. Rev. Pharmacol. Toxicol. 43, 629–656. doi: 10.1146/annurev.pharmtox.43.100901.140204
Demeule, M., Currie, J. C., Bertrand, Y., Che, C., Nguyen, T., Regina, A., et al. (2008). Involvement of the low-density lipoprotein receptor-related protein in the transcytosis of the brain delivery vector angiopep-2. J. Neurochem. 106, 1534–1544. doi: 10.1111/j.1471-4159.2008.05492.x
Dhaliwal, H. K., Fan, Y., Kim, J., and Amiji, M. M. (2020). Intranasal delivery and transfection of mrna therapeutics in the brain using cationic liposomes. Mol. Pharm. 17, 1996–2005. doi: 10.1021/acs.molpharmaceut.0c00170
Di Stefano, A., Iannitelli, A., Laserra, S., and Sozio, P. (2011). Drug delivery strategies for alzheimer's disease treatment. Expert Opin. Drug Deliv. 8, 581–603. doi: 10.1517/17425247.2011.561311
Dodel, R. C., Berger, K., and Oertel, W. H. (2001). Health-related quality of life and healthcare utilisation in patients with parkinson's disease: impact of motor fluctuations and dyskinesias. Pharmacoeconomics 19, 1013–1038. doi: 10.2165/00019053-200119100-00004
Dos Santos Rodrigues, B., Oue, H., Banerjee, A., Kanekiyo, T., and Singh, J. (2018). Dual functionalized liposome-mediated gene delivery across triple co-culture blood brain barrier model and specific in vivo neuronal transfection. J. Control. Release 286, 264–278. doi: 10.1016/j.jconrel.2018.07.043
Duraes, F., Pinto, M., and Sousa, E. (2018). Old drugs as new treatments for neurodegenerative diseases. Pharmaceuticals 11:44. doi: 10.3390/ph11020044
Elieh-Ali-Komi, D., and Hamblin, M. R. (2016). Chitin and chitosan: production and application of versatile biomedical nanomaterials. Int. J. Adv. Res. 4, 411–427.
Engelhardt, B., and Liebner, S. (2014). Novel insights into the development and maintenance of the blood-brain barrier. Cell Tissue Res, 355, 687–699. doi: 10.1007/s00441-014-1811-2
Ethemoglu, M. S., Seker, F. B., Akkaya, H., Kilic, E., Aslan, I., Erdogan, C. S., et al. (2017). Anticonvulsant activity of resveratrol-loaded liposomes in vivo. Neuroscience 357, 12–19. doi: 10.1016/j.neuroscience.2017.05.026
Euliss, L. E., DuPont, J. A., Gratton, S., and DeSimone, J. (2006). Imparting size, shape, and composition control of materials for nanomedicine. Chem. Soc. Rev. 35, 1095–1104. doi: 10.1039/b600913c
Fan, Q. W., Iosbe, I., Asou, H., Yanagisawa, K., and Michikawa, M. (2001). Expression and regulation of apolipoprotein e receptors in the cells of the central nervous system in culture: a review. J. Am. Aging Assoc. 24, 1–10. doi: 10.1007/s11357-001-0001-9
Fenske, D. B., Chonn, A., and Cullis, P. R. (2008). Liposomal nanomedicines: an emerging field. Toxicol. Pathol. 36, 21–29. doi: 10.1177/0192623307310960
Fenske, D. B., and Cullis, P. R. (2008). Liposomal nanomedicines. Expert Opin. Drug Deliv. 5, 25–44. doi: 10.1517/17425247.5.1.25
Ghosh, A., Mandal, A. K., Sarkar, S., Panda, S., and Das, N. (2009). Nanoencapsulation of quercetin enhances its dietary efficacy in combating arsenic-induced oxidative damage in liver and brain of rats. Life Sci. 84, 75–80. doi: 10.1016/j.lfs.2008.11.001
Gitler, A. D., Dhillon, P., and Shorter, J. (2017). Neurodegenerative disease: models, mechanisms, and a new hope. Dis. Model. Mech. 10, 499–502. doi: 10.1242/dmm.030205
Goedert, M. (2004). Tau protein and neurodegeneration. Semin. Cell Dev. Biol. 15, 45–49. doi: 10.1016/j.semcdb.2003.12.015
Hatters, D. M., Peters-Libeu, C. A., and Weisgraber, K. H. (2006). Apolipoprotein e structure: insights into function. Trends Biochem. Sci. 31, 445–454. doi: 10.1016/j.tibs.2006.06.008
Helen Chan, O., and Stewart, B. H. (1996). Physicochemical and drug-delivery considerations for oral drug bioavailability. Drug Discov. Today 1, 461–473. doi: 10.1016/1359-6446(96)10039-8
Herve, F., Ghinea, N., and Scherrmann, J. M. (2008). Cns delivery via adsorptive transcytosis. AAPS J. 10, 455–472. doi: 10.1208/s12248-008-9055-2
Hong, S. S., Oh, K. T., Choi, H. G., and Lim, S. J. (2019). Liposomal formulations for nose-to-brain delivery: recent advances and future perspectives. Pharmaceutics 11:540. doi: 10.3390/pharmaceutics11100540
Iqbal, M. A., Md, S., Sahni, J. K., Baboota, S., Dang, S., and Ali, J. (2012). Nanostructured lipid carriers system: recent advances in drug delivery. J. Drug Target 20, 813–830. doi: 10.3109/1061186X.2012.716845
Johnsson, M., and Edwards, K. (2003). Liposomes, disks, and spherical micelles: aggregate structure in mixtures of gel phase phosphatidylcholines and poly(ethylene glycol)-phospholipids. Biophys. J. 85, 3839–3847. doi: 10.1016/S0006-3495(03)74798-5
Jose, S., Anju, S. S., Cinu, T. A., Aleykutty, N. A., Thomas, S., and Souto, E. B. (2014). In vivo pharmacokinetics and biodistribution of resveratrol-loaded solid lipid nanoparticles for brain delivery. Int. J. Pharm. 474, 6–13. doi: 10.1016/j.ijpharm.2014.08.003
Joshi, S., Singh-Moon, R., Wang, M., Chaudhuri, D. B., Ellis, J. A., Bruce, J. N., et al. (2014b). Cationic surface charge enhances early regional deposition of liposomes after intracarotid injection. J. Neurooncol. 120, 489–497. doi: 10.1007/s11060-014-1584-1
Joshi, S., Singh-Moon, R. P., Ellis, J. A., Chaudhuri, D. B., Wang, M., Reif, R., et al. (2015). Cerebral hypoperfusion-assisted intra-arterial deposition of liposomes in normal and glioma-bearing rats. Neurosurgery 76, 92–100. doi: 10.1227/NEU.0000000000000552
Joshi, S., Singh-Moon, R. P., Wang, M., Chaudhuri, D. B., Holcomb, M., Straubinger, N. L., et al. (2014a). Transient cerebral hypoperfusion assisted intraarterial cationic liposome delivery to brain tissue. J. Neurooncol. 118, 73–82. doi: 10.1007/s11060-014-1421-6
Kanhai, K. M. S., Zuiker, R., Stavrakaki, I., Gladdines, W., Gaillard, P. J., Klaassen, E. S., et al. (2018). Glutathione-pegylated liposomal methylprednisolone in comparison to free methylprednisolone: slow release characteristics and prolonged lymphocyte depression in a first-in-human study. Br. J. Clin. Pharmacol 84, 1020–1028. doi: 10.1111/bcp.13525
Kisler, K., Nelson, A. R., Montagne, A., and Zlokovic, B. V. (2017). Cerebral blood flow regulation and neurovascular dysfunction in alzheimer disease. Nat. Rev. Neurosci. 18, 419–434. doi: 10.1038/nrn.2017.48
Kulkarni, S. S., and Canto, C. (2015). The molecular targets of resveratrol. Biochim. Biophys. Acta 1852, 1114–1123. doi: 10.1016/j.bbadis.2014.10.005
Kuo, Y.-C., Chen, I.-Y., and Rajesh, R. (2018). Use of functionalized liposomes loaded with antioxidants to permeate the blood–brain barrier and inhibit β-amyloid-induced neurodegeneration in the brain. J. Taiwan Inst. Chem. Eng. 87, 1–14. doi: 10.1016/j.jtice.2018.03.001
Kuo, Y. C., Lin, C. Y., Li, J. S., and Lou, Y. I. (2017). Wheat germ agglutinin-conjugated liposomes incorporated with cardiolipin to improve neuronal survival in alzheimer's disease treatment. Int. J. Nanomed. 12, 1757–1774. doi: 10.2147/IJN.S128396
Li, F., Gong, Q., Dong, H., and Shi, J. (2012). Resveratrol, a neuroprotective supplement for alzheimer's disease. Curr. Pharm. Des. 18, 27–33. doi: 10.2174/138161212798919075
Lindqvist, A., Rip, J., Gaillard, P. J., Bjorkman, S., and Hammarlund-Udenaes, M. (2013). Enhanced brain delivery of the opioid peptide damgo in glutathione pegylated liposomes: a microdialysis study. Mol. Pharm. 10, 1533–1541. doi: 10.1021/mp300272a
Lockman, P. R., Mumper, R. J., Khan, M. A., and Allen, D. D. (2002). Nanoparticle technology for drug delivery across the blood-brain barrier. Drug Dev. Ind. Pharm. 28, 1–13. doi: 10.1081/DDC-120001481
Maussang, D., Rip, J., van Kregten, J., van den Heuvel, A., van der Pol, S., van der Boom, B., et al. (2016). Glutathione conjugation dose-dependently increases brain-specific liposomal drug delivery in vitro and in vivo. Drug Discov. Today Technol. 20, 59–69. doi: 10.1016/j.ddtec.2016.09.003
McEwen, B. S., and Reagan, L. P. (2004). Glucose transporter expression in the central nervous system: relationship to synaptic function. Eur. J. Pharmacol. 490, 13–24. doi: 10.1016/j.ejphar.2004.02.041
Migliore, M. M., Vyas, T. K., Campbell, R. B., Amiji, M. M., and Waszczak, B. L. (2010). Brain delivery of proteins by the intranasal route of administration: a comparison of cationic liposomes versus aqueous solution formulations. J. Pharm. Sci. 99, 1745–1761. doi: 10.1002/jps.21939
Miller, C. R., Bondurant, B., McLean, S. D., McGovern, K. A., and O'Brien, D. F. (1998). Liposome-cell interactions in vitro: effect of liposome surface charge on the binding and endocytosis of conventional and sterically stabilized liposomes. Biochemistry 37, 12875–12883. doi: 10.1021/bi980096y
Mishra, A. D., Patel, C. N., and Shah, D. R. (2013). Formulation and optimization of ethosomes for transdermal delivery of ropinirole hydrochloride. Curr. Drug Deliv. 10, 500–516. doi: 10.2174/1567201811310050002
Mishra, B., Patel, B. B., and Tiwari, S. (2010). Colloidal nanocarriers: a review on formulation technology, types and applications toward targeted drug delivery. Nanomedicine 6, 9–24. doi: 10.1016/j.nano.2009.04.008
Mokgokong, R., Wang, S., Taylor, C. J., Barrand, M. A., and Hladky, S. B. (2014). Ion transporters in brain endothelial cells that contribute to formation of brain interstitial fluid. Pflugers Arch. 466, 887–901. doi: 10.1007/s00424-013-1342-9
Mourtas, S., Lazar, A. N., Markoutsa, E., Duyckaerts, C., and Antimisiaris, S. G. (2014). Multifunctional nanoliposomes with curcumin-lipid derivative and brain targeting functionality with potential applications for alzheimer disease. Eur. J. Med. Chem. 80, 175–183. doi: 10.1016/j.ejmech.2014.04.050
Nageeb El-Helaly, S., Abd Elbary, A., Kassem, M. A., and El-Nabarawi, M. A. (2017). Electrosteric stealth rivastigmine loaded liposomes for brain targeting: preparation, characterization, ex vivo, bio-distribution and in vivo pharmacokinetic studies. Drug Deliv. 24, 692–700. doi: 10.1080/10717544.2017.1309476
Neves, A. R., Lucio, M., Martins, S., Lima, J. L., and Reis, S. (2013). Novel resveratrol nanodelivery systems based on lipid nanoparticles to enhance its oral bioavailability. Int. J. Nanomed. 8, 177–187. doi: 10.2147/IJN.S37840
Noble, G. T., Stefanick, J. F., Ashley, J. D., Kiziltepe, T., and Bilgicer, B. (2014). Ligand-targeted liposome design: challenges and fundamental considerations. Trends Biotechnol. 32, 32–45. doi: 10.1016/j.tibtech.2013.09.007
Nunes, S., Madureira, A. R., Campos, D., Sarmento, B., Gomes, A. M., Pintado, M., et al. (2017). Solid lipid nanoparticles as oral delivery systems of phenolic compounds: overcoming pharmacokinetic limitations for nutraceutical applications. Crit. Rev. Food Sci. Nutr. 57, 1863–1873. doi: 10.1080/10408398.2015.1031337
Organization W. H. (2006). Neurological Disorders: Public Health Challenges. Genève: World Health Organization (WHO/OMS).
Paliwal, S., Tilak, A., Sharma, J., Dave, V., Sharma, S., Yadav, R., et al. (2019). Flurbiprofen loaded ethosomes - transdermal delivery of anti-inflammatory effect in rat model. Lipids Health Dis. 18:133. doi: 10.1186/s12944-019-1064-x
Pandita, D., Kumar, S., Poonia, N., and Lather, V. (2014). Solid lipid nanoparticles enhance oral bioavailability of resveratrol, a natural polyphenol. Food Res. Int. 62, 1165–1174. doi: 10.1016/j.foodres.2014.05.059
Pardridge, W. M. (2012). Drug transport across the blood-brain barrier. J. Cereb. Blood Flow Metab. 32, 1959–1972. doi: 10.1038/jcbfm.2012.126
Pardridge, W. M. (2015). Targeted delivery of protein and gene medicines through the blood-brain barrier. Clin. Pharmacol. Ther. 97, 347–361. doi: 10.1002/cpt.18
Pardridge, W. M., and Boado, R. J. (2012). Reengineering biopharmaceuticals for targeted delivery across the blood-brain barrier. Meth. Enzymol. 503, 269–292. doi: 10.1016/B978-0-12-396962-0.00011-2
Phachonpai, W., Wattanathorn, J., Muchimapura, S., Tong-Un, T., and Preechagoon, D. (2010). Neuroprotective effect of quercetin encapsulated liposomes: a novel therapeutic strategy against alzheimer's disease. Am. J. Appl. Sci. 7, 480–485. doi: 10.3844/ajassp.2010.480.485
Poewe, W., Antonini, A., Zijlmans, J. C., Burkhard, P. R., and Vingerhoets, F. (2010). Levodopa in the treatment of parkinson's disease: an old drug still going strong. Clin. Interv. Aging 5, 229–238. doi: 10.2147/CIA.S6456
Pujara, N. D. (2012). Self emulsifying drug delivery system: a novel approach. Int. J. Curr. Pharm. Res. 4, 18–23.
Pulgar, V. M. (2018). Transcytosis to cross the blood brain barrier, new advancements and challenges. Front. Neurosci. 12:1019. doi: 10.3389/fnins.2018.01019
Re, F., Cambianica, I., Sesana, S., Salvati, E., Cagnotto, A., Salmona, M., et al. (2011). Functionalization with apoe-derived peptides enhances the interaction with brain capillary endothelial cells of nanoliposomes binding amyloid-beta peptide. J. Biotechnol. 156, 341–346. doi: 10.1016/j.jbiotec.2011.06.037
Rip, J., Appeldoorn, C., Manca, F., Dorland, R., Van Kregten, J., and Gaillard, P. (2010). Receptor-Mediated Delivery of Drugs Across the Blood-Brain Barrier. Brussels: Pharmacology and Toxicology of the Blood-Brain Barrier: State of the Art, Needs for Future Research and Expected Benefits for the EU.
Rip, J., Chen, L., Hartman, R., van den Heuvel, A., Reijerkerk, A., van Kregten, J., et al. (2014). Glutathione pegylated liposomes: pharmacokinetics and delivery of cargo across the blood-brain barrier in rats. J. Drug Target 22, 460–467. doi: 10.3109/1061186X.2014.888070
Risau, W., and Wolburg, H. (1990). Development of the blood-brain barrier. Trends Neurosci. 13, 174–178. doi: 10.1016/0166-2236(90)90043-A
Ross, C. A., and Poirier, M. A. (2004). Protein aggregation and neurodegenerative disease. Nat. Med. 10, S10–17. doi: 10.1038/nm1066
Rotman, M., Welling, M. M., Bunschoten, A., de Backer, M. E., Rip, J., Nabuurs, R. J., et al. (2015). Enhanced glutathione pegylated liposomal brain delivery of an anti-amyloid single domain antibody fragment in a mouse model for alzheimer's disease. J. Control. Release 203, 40–50. doi: 10.1016/j.jconrel.2015.02.012
Sadegh Malvajerd, S., Azadi, A., Izadi, Z., Kurd, M., Dara, T., Dibaei, M., et al. (2019). Brain delivery of curcumin using solid lipid nanoparticles and nanostructured lipid carriers: Preparation, optimization, and pharmacokinetic evaluation. ACS Chem. Neurosci. 10, 728–739. doi: 10.1021/acschemneuro.8b00510
Sagare, A. P., Deane, R., and Zlokovic, B. V. (2012). Low-density lipoprotein receptor-related protein 1: a physiological abeta homeostatic mechanism with multiple therapeutic opportunities. Pharmacol. Ther. 136, 94–105. doi: 10.1016/j.pharmthera.2012.07.008
Salem, H. F., Ahmed, S. M., Hassaballah, A. E., and Omar, M. M. (2015). Targeting brain cells with glutathione-modulated nanoliposomes: in vitro and in vivo study. Drug Des. Devel. Ther. 9, 3705–3727. doi: 10.2147/DDDT.S85302
Salvati, E., Re, F., Sesana, S., Cambianica, I., Sancini, G., Masserini, M., et al. (2013). Liposomes functionalized to overcome the blood-brain barrier and to target amyloid-beta peptide: the chemical design affects the permeability across an in vitro model. Int. J. Nanomed. 8, 1749–1758. doi: 10.2147/IJN.S42783
Sarkar, S., and Das, N. (2006). Mannosylated liposomal flavonoid in combating age-related ischemia-reperfusion induced oxidative damage in rat brain. Mech. Ageing Dev. 127, 391–397. doi: 10.1016/j.mad.2005.12.010
Scherrmann, J.-M. (2002). Drug delivery to brain via the blood–brain barrier. Vascul. Pharmacol. 38, 349–354. doi: 10.1016/S1537-1891(02)00202-1
Selkoe, D. J. (2002). Alzheimer's disease is a synaptic failure. Science 298, 789–791. doi: 10.1126/science.1074069
Sharma, G., Lakkadwala, S., Modgil, A., and Singh, J. (2016). The role of cell-penetrating peptide and transferrin on enhanced delivery of drug to brain. Int. J. Mol. Sci. 17:806. doi: 10.3390/ijms17060806
Shi, J., Wang, Y., and Luo, G. (2012). Ligustrazine phosphate ethosomes for treatment of alzheimer's disease, in vitro and in animal model studies. AAPS PharmSciTech 13, 485–492. doi: 10.1208/s12249-012-9767-6
Smeyne, M., and Smeyne, R. J. (2013). Glutathione metabolism and parkinson's disease. Free Radic. Biol. Med. 62, 13–25. doi: 10.1016/j.freeradbiomed.2013.05.001
Sokolik, V., Berchenko, O., and Shulga, S. (2017). Comparative analysis of nasal therapy with soluble and liposomal forms of curcumin on rats with alzheimer's disease model. J. Alzheimers. Dis. Parkinsonism 7:357. doi: 10.4172/2161-0460.1000357
Sokolik, V. V., Koliada, O. K., and Shulga, S. M. (2016). Effect of beta-amyloid peptide 42 on the dynamics of expression and formation of capital a, cyrillicbeta40, il−1beta, tnf alpha, il−6, il−10 by peripheral blood mononuclear cells in vitro and its correction by curcumin. Ukrain. Biochem. J. 88, 109–118. doi: 10.15407/ubj88.01.109
Sokolik, V. V., and Shulga, S. M. (2016). Effect of curcumin on accumulation in mononuclear cells and secretion in incubation medium of capital a, cyrillicbeta(40) and cytokines under local excess of capital a, cyrillicbeta(42)-homoaggregates. Ukrain. Biochem. J. 88, 83–91. doi: 10.15407/ubj88.03.083
Spuch, C., and Navarro, C. (2011). Liposomes for targeted delivery of active agents against neurodegenerative diseases (alzheimer's disease and parkinson's disease). J. Drug Deliv. 2011:469679. doi: 10.1155/2011/469679
Stefanis, L. (2012). Alpha-synuclein in parkinson's disease. Cold Spring Harb. Perspect. Med. 2:a009399. doi: 10.1101/cshperspect.a009399
Tagalakis, A. D., Kenny, G. D., Bienemann, A. S., McCarthy, D., Munye, M. M., Taylor, H., et al. (2014). Pegylation improves the receptor-mediated transfection efficiency of peptide-targeted, self-assembling, anionic nanocomplexes. J. Control. Release 174, 177–187. doi: 10.1016/j.jconrel.2013.11.014
Tellone, E., Galtieri, A., Russo, A., Giardina, B., and Ficarra, S. (2015). Resveratrol: a focus on several neurodegenerative diseases. Oxid. Med Cell Longev. 2015:392169. doi: 10.1155/2015/392169
Terdthai, T.-U., Panakaporn, W., Jintanaporn, W., and Wathita, P. (2010). Cognitive-enhancing and antioxidant activities of quercetin liposomes in animal model of alzheimer's disease. Online J. Biol. Sci. 10, 84–91. doi: 10.3844/ojbsci.2010.84.91
Tosi, G., Fano, R. A., Bondioli, L., Badiali, L., Benassi, R., Rivasi, F., et al. (2011). Investigation on mechanisms of glycopeptide nanoparticles for drug delivery across the blood-brain barrier. Nanomedicine 6, 423–436. doi: 10.2217/nnm.11.11
Touitou, E., Dayan, N., Bergelson, L., Godin, B., and Eliaz, M. (2000). Ethosomes - novel vesicular carriers for enhanced delivery: characterization and skin penetration properties. J. Control. Release 65, 403–418. doi: 10.1016/S0168-3659(99)00222-9
Uner, M., and Yener, G. (2007). Importance of solid lipid nanoparticles (sln) in various administration routes and future perspectives. Int. J. Nanomed. 2, 289–300.
Upadhyay, R. K. (2014). Drug delivery systems, cns protection, and the blood brain barrier. BioMed Res. Int. 2014:869269. doi: 10.1155/2014/869269
Vernier, P., Moret, F., Callier, S., Snapyan, M., Wersinger, C., and Sidhu, A. (2004). The degeneration of dopamine neurons in parkinson's disease: Insights from embryology and evolution of the mesostriatocortical system. Ann. N Y Acad. Sci. 1035, 231–249. doi: 10.1196/annals.1332.015
Wang, D. G., Liu, W. Y., and Chen, G. T. (2013). A simple method for the isolation and purification of resveratrol from polygonum cuspidatum. J. Pharm. Anal. 3, 241–247. doi: 10.1016/j.jpha.2012.12.001
Wang, Y., Xia, Z., Xu, J. R., Wang, Y. X., Hou, L. N., Qiu, Y., et al. (2012). Alpha-mangostin, a polyphenolic xanthone derivative from mangosteen, attenuates beta-amyloid oligomers-induced neurotoxicity by inhibiting amyloid aggregation. Neuropharmacology 62, 871–881. doi: 10.1016/j.neuropharm.2011.09.016
Wang, Y., Xu, H., Fu, Q., Ma, R., and Xiang, J. (2011). Protective effect of resveratrol derived from polygonum cuspidatum and its liposomal form on nigral cells in parkinsonian rats. J. Neurol. Sci. 304, 29–34. doi: 10.1016/j.jns.2011.02.025
Wang, Z., Zhao, Y., Jiang, Y., Lv, W., Wu, L., Wang, B., et al. (2015). Enhanced anti-ischemic stroke of zl006 by t7-conjugated pegylated liposomes drug delivery system. Sci. Rep 5:12651. doi: 10.1038/srep12651
Warren, K. E. (2018). Beyond the blood: brain barrier: the importance of central nervous system (cns) pharmacokinetics for the treatment of cns tumors, including diffuse intrinsic pontine glioma. Front. Oncol. 8:239. doi: 10.3389/fonc.2018.00239
Woodle, M. C., and Papahadjopoulos, D. (1989). Liposome preparation and size characterization. Meth. Enzymol. 171, 193–217. doi: 10.1016/S0076-6879(89)71012-0
Wyss-Coray, T. (2016). Ageing, neurodegeneration and brain rejuvenation. Nature 539, 180–186. doi: 10.1038/nature20411
Yang, J., Song, S., Li, J., and Liang, T. (2014). Neuroprotective effect of curcumin on hippocampal injury in 6-ohda-induced parkinson's disease rat. Pathol. Res. Pract. 210, 357–362. doi: 10.1016/j.prp.2014.02.005
Yang, Z. Z., Zhang, Y. Q., Wang, Z. Z., Wu, K., Lou, J. N., and Qi, X. R. (2013). Enhanced brain distribution and pharmacodynamics of rivastigmine by liposomes following intranasal administration. Int. J. Pharm. 452, 344–354. doi: 10.1016/j.ijpharm.2013.05.009
Yan-Hong, L., Yong-Hua, W., Yan, L., and Ling, Y. (2006). Mdr1 gene polymorphisms and clinical relevance. Acta Genet. Sinica 33, 93–104. doi: 10.1016/S0379-4172(06)60027-9
Zhao, Z., Nelson, A. R., Betsholtz, C., and Zlokovic, B. V. (2015). Establishment and dysfunction of the blood-brain barrier. Cell 163, 1064–1078. doi: 10.1016/j.cell.2015.10.067
Keywords: neurodegenarative diseases, Blood Brain Barrier (BBB), liposome, solid lipid nanoparticles, nanostrured lipid particles, ethosomes, drug delivery
Citation: Cascione M, De Matteis V, Leporatti S and Rinaldi R (2020) The New Frontiers in Neurodegenerative Diseases Treatment: Liposomal-Based Strategies. Front. Bioeng. Biotechnol. 8:566767. doi: 10.3389/fbioe.2020.566767
Received: 28 May 2020; Accepted: 14 September 2020;
Published: 26 October 2020.
Edited by:
Christian Celia, University of Studies G. d'Annunzio Chieti and Pescara, ItalyReviewed by:
Giovanni Tosi, University of Modena and Reggio Emilia, ItalyMaria Chiara Cristiano, Magna Græcia University of Catanzaro, Italy
Gert Fricker, Heidelberg University, Germany
Copyright © 2020 Cascione, De Matteis, Leporatti and Rinaldi. This is an open-access article distributed under the terms of the Creative Commons Attribution License (CC BY). The use, distribution or reproduction in other forums is permitted, provided the original author(s) and the copyright owner(s) are credited and that the original publication in this journal is cited, in accordance with accepted academic practice. No use, distribution or reproduction is permitted which does not comply with these terms.
*Correspondence: Mariafrancesca Cascione, bWFyaWFmcmFuY2VzY2EuY2FzY2lvbmVAdW5pc2FsZW50by5pdA==; Stefano Leporatti, c3RlZmFuby5sZXBvcmF0dGlAbmFub3RlYy5jbnIuaXQ=