- 1Neuro-Computing and Neuro-Robotics Research Group, Complutense University of Madrid, Madrid, Spain
- 2Innovation Group, Institute for Health Research San Carlos Clinical Hospital (IdISSC), Madrid, Spain
- 3Department of Biomedical Engineering, Tufts University, Medford, MA, United States
- 4Silk Biomed SL, Madrid, Spain
- 5Ophthalmology Service, La Paz University Hospital, Madrid, Spain
- 6Center for Biomedical Technology, Universidad Politécnica de Madrid, Madrid, Spain
- 7Department of Material Science, Civil Engineering Superior School, Universidad Politécnica de Madrid, Madrid, Spain
- 8Biomedical Research Networking Center in Bioengineering, Biomaterials and Nanomedicine (CIBER-BBN), Madrid, Spain
Age-related Macular Degeneration (AMD) is an up-to-date untreatable chronic neurodegenerative eye disease of multifactorial origin, and the main causes of blindness in over 65 years old people. It is characterized by a slow progression and the presence of a multitude of factors, highlighting those related to diet, genetic heritage and environmental conditions, present throughout each of the stages of the illness. Current therapeutic approaches, mainly consisting of intraocular drug delivery, are only used for symptoms relief and/or to decelerate the progression of the disease. Furthermore, they are overly simplistic and ignore the complexity of the disease and the enormous differences in the symptomatology between patients. Due to the wide impact of the AMD and the up-to-date absence of clinical solutions, the development of biomaterials-based approaches for a personalized and controlled delivery of therapeutic drugs and biomolecules represents the main challenge for the defeat of this neurodegenerative disease. Here we present a critical review of the available and under development AMD therapeutic approaches, from a biomaterials and biotechnological point of view. We highlight benefits and limitations and we forecast forthcoming alternatives based on novel biomaterials and biotechnology methods. In the first part we expose the physiological and clinical aspects of the disease, focusing on the multiple factors that give origin to the disorder and highlighting the contribution of these factors to the triggering of each step of the disease. Then we analyze available and under development biomaterials-based drug-delivery devices (DDD), taking into account the anatomical and functional characteristics of the healthy and ill retinal tissue.
Introduction
Age-related macular degeneration is a chronic neurodegenerative eye disease of multifactorial origin, characterized by the appearance of alterations in the central part of the retina, the macula. According to the WHO, there are 196 million cases worldwide and are expected to reach 288 million by 2040, making AMD one of the leading causes of irreversible loss of vision in people over 65 years in developed countries (Evans et al., 2004; Ferris et al., 2005; Lim et al., 2012; Garciìa and Martinez, 2013; IAPB Vision Atlas, 2017; World Health Organization, 2018). In the United States there are more than 2 million people affected by AMD and it is estimated that they will exceed 4 million by 2030 (Friedman et al., 2004; Klein et al., 2011; Friedman et al., 2012; Degeneration et al., 2017). In Western Europe, the IAPB estimates 4.8 million cases of AMD for 2020, making it the second most common cause of eye disease, preceded only by cataracts (Damián et al., 2006; Casado, 2009; Klein et al., 2011; Degeneration et al., 2017; Jeffries et al., 2017).
Despite the great research efforts dedicated in the development of AMD therapies, nowadays available treatments only are able to treat symptomatology and/or slowing down the progression of the disease.
In the present paper we briefly analyze the biomolecular and cellular processes responsible for the development of the AMD, and we review available and under development biomaterials-based drug-delivery devices (DDD) for possible treatments of disease.
Natural History
Risk Factors
The pathogenesis mechanisms of AMD are heterogeneous and not yet understood (Lacour et al., 2002; Ambati and Fowler, 2012; Kabiesz and Nowak, 2015; Michalska-Małecka et al., 2015), however, it is known that one of the main risk factor for AMD is aging (Lacour et al., 2002; Ardeljan and Chan, 2013; Daneault et al., 2016). Other risk factors are sex (higher rates in women), race (higher rates in the Caucasian population) and environmental factors that increase oxidative stress in the RPE, such as: blue light exposure, smoking, alcohol consumption, obesity, low antioxidant diet (e.g., lack of vitamins A and E, zinc, lutein and omega-3 fatty acids) as well as systemic factors associated with cardiovascular risks (Thornton et al., 2005; Michalska-Małecka et al., 2015). The risk of AMD is also increased by ocular factors such as macular pigment optical density, iris pigmentation (less risk in dark eyes), cataract surgery and high refractive errors (Michalska-Małecka et al., 2015). In addition, several genetic factors have been reported (Table 1).
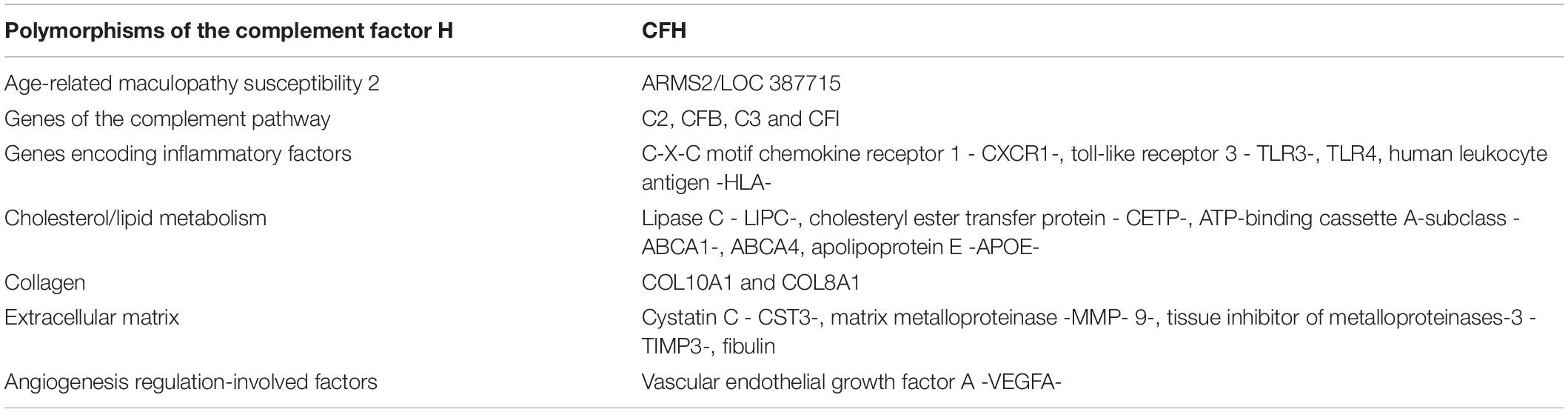
Table 1. List of possible genes that have a determining influence on AMD (Klein et al., 2005; Rivera et al., 2005; Zareparsi et al., 2005; Khan et al., 2006; Nozaki et al., 2006; Ratnapriya and Chew, 2013; Michalska-Małecka et al., 2015; Sergejeva et al., 2016).
Clinical Aspects
An outline of the stages involved in the disease, and the fundus images of affected patients are shown in Figures 1, 2, respectively. The early stages of AMD are detected by the presence of drusen between the basal membrane of the RPE and the Bruch’s membrane (BrM). Drusen are lipids, cellular and metabolic debris that originate in the RPE and surrounding tissues. In more advanced stages of the disease, these deposits may be also found between the RPE and the neuroretina. In both cases, metabolic abnormalities in the RPE cells is one of the causes of the formation of drusen (Winkler et al., 1999; Anderson et al., 2002; McConnell and Silvestri, 2005; Damián et al., 2006; Nowak, 2006; Jager et al., 2008). Depending on the number and extension of the drusen and the degree of the macular atrophy, AMD is customarily classified into three stages (Table 2): Early AMD; characterized by the presence of a few, small or medium-sized (<124 μm) drusen and minimal RPE cell alterations leading to some pigmentary anomalies (hypo- and hyper-pigmentation). Patients are normally asymptomatic. Intermediate AMD; characterized by the presence of one or more large (>124 μm) drusen, together with geographic atrophy that does not reach the central area of the macula. Patients are either asymptomatic or complain of decreased contrast sensitivity, abnormal scotopic vision or blurred vision while reading. Advanced AMD; either manifested in dry (D-AMD) or wet (W-AMD) form. D-AMD is characterized by the presence of large white or yellow drusen, the RPE atrophy and the photoreceptors’ deterioration. Patients suffer from a decreased visual acuity and metamorphopsias. W-AMD is characterized by choroidal neovascularization, macular edema, aneurysms, hemorrhages, RPE and/or retinal detachment and photoreceptors death. Patients present important loss of visual acuity or total blindness, metamorphopsias and photopsia.
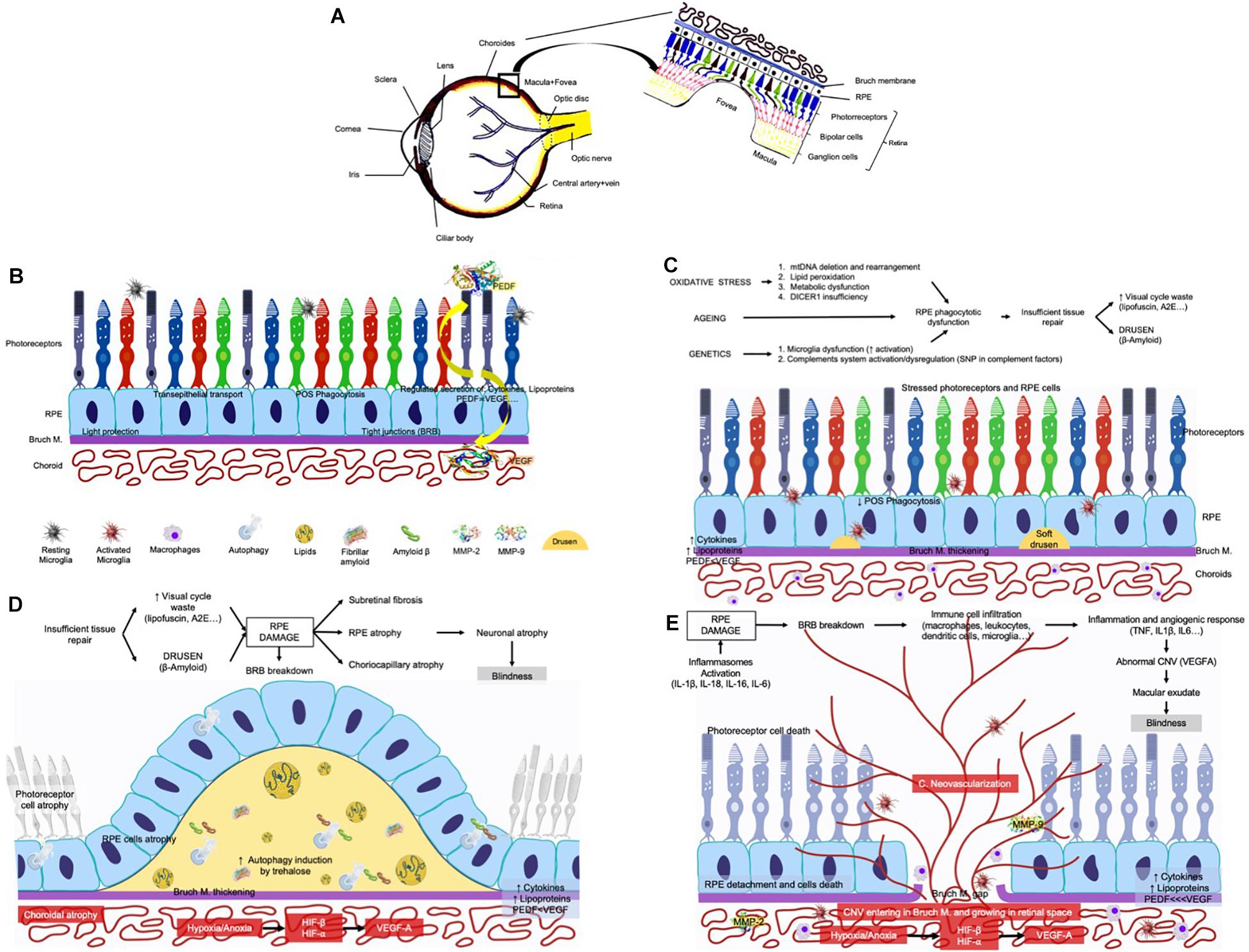
Figure 1. Schematic representation of the eye and the stages of the AMD. (A) The eye and the retina. (B) Normal retina in a cross section of the eye where appear: the layer of the photoreceptors, the choroid, the RPE and the Bruch’s membrane (BrM). The main functions of the normal RPE are: (1) transport of nutrients, ions, and water (2) absorption of light and protection against photooxidation, (3) re-isomerization of all-trans-retinal into 11-cis-retinal, which is a key element of the visual cycle, (4) phagocytosis of shed photoreceptor membranes, and (5) secretion of various essential factors for the structural integrity of the retina. POS, photoreceptors outer segment; BRB, blood–retinal barrier; PEDF, pigment epithelium-derived factor, the most potent inhibitor of angiogenesis in the mammalian eye; VEGF, vascular endothelial growth factor. VEGF is essential for development and maintenance of functionally efficient retinal vasculature as well as for integrity of the RPE, BrM, and choroidal endothelial cells. (C) Early and intermediate AMD phases in a cross section of the eye. Without apparent cause, debris from the RPE cells layer as well as from the surrounding tissues begin to accumulate between RPE and BrM forming clusters called drusen. (D) Progress of the dry AMD in a cross section of the eye where the clusters called drusen start growing in size and accumulate as the disease progresses. The accumulation of drusen and the degeneration of RPE provokes the beginning of photoreceptor’s atrophy. (E) The progress of wet AMD. As drusen accumulate, they can cause inflammation. Inflammatory cells are then recruited by the retina, and these cells, together with the RPE begin to release vascular growth factors (mainly VEGF) that cause growth of the blood vessels. In this case, the degeneration is characterized by choroidal neovascularization, neovessels penetrating the BrM, presence of inflammatory cells, exudates of lipid content, hemorrhage, and the destruction of the RPE and photoreceptors (wet AMD).
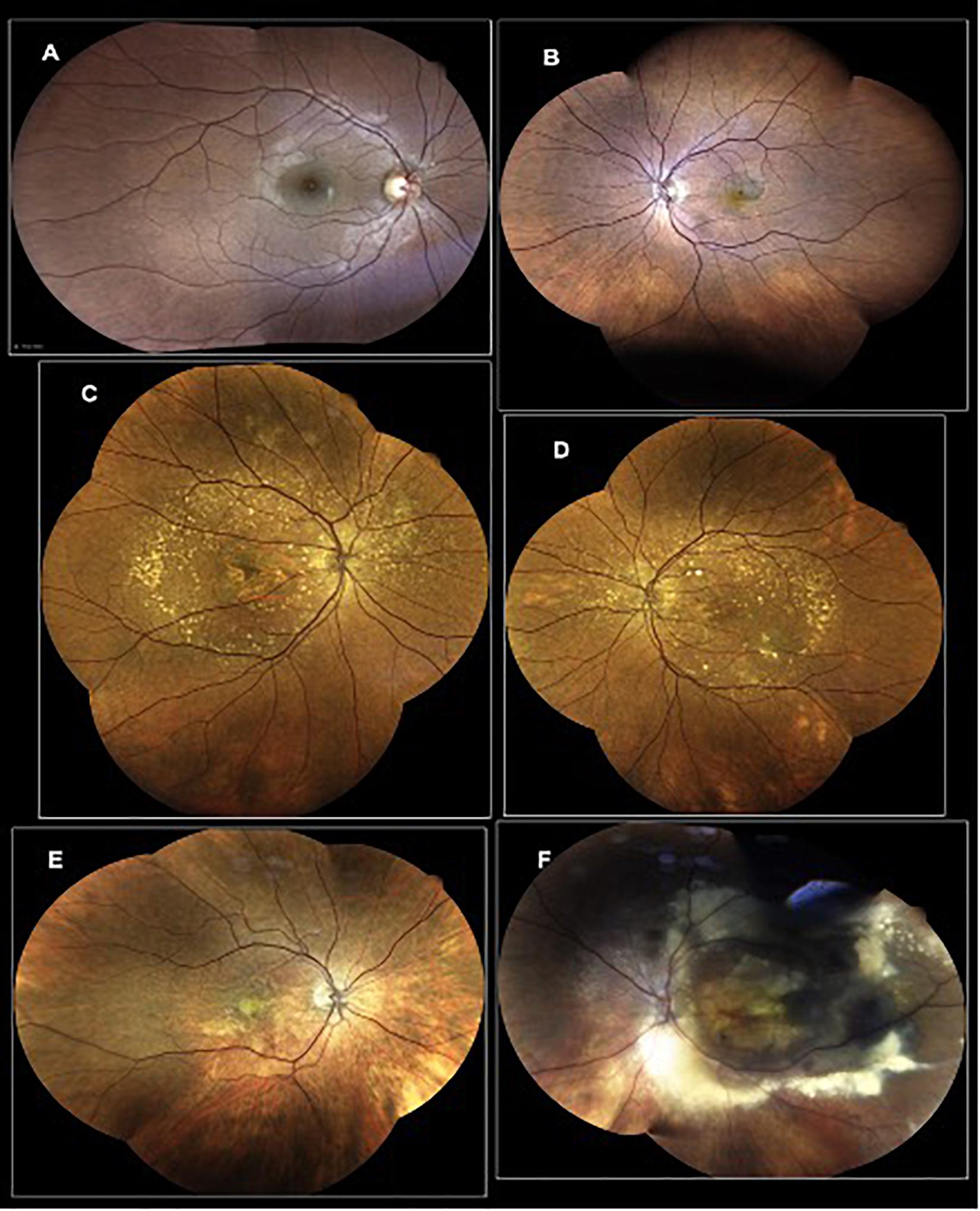
Figure 2. Representative photographs of AMD-affected eyes. (A) Eye of a healthy subject; (B) AMD patient with macular hemorrhage and active exudation; (C) AMD patient with wide macular geographic atrophy and several druses in the back of the eye; (D) AMD patient with macular pigment changes, several druses as well as a macular hemorrhage secondary to an active neovascular complex; (E) patient with an active macular neovascular complex, secondary to an AMD; (F) AMD patient with an active AMD with a pseudo-tumoral lesion.
Pathogenesis
Most pathways and causes of the AMD are very well-established. However, this fact does not imply a straightforward identification of the AMD-triggering mechanisms. Such identification is challenging because of the multifactorial and extremely complex etiology of this disease: (I) It is not still clear if all of them have been identified, (II) None of them has been proved as the sole cause of the disease, and (III) Synergistic relations between causes that determine each phase triggering of the disease has not yet been identified. As we have already mentioned, AMD is the result of several molecular and cellular processes of different origins that interact with each other. Oxidative stress and inflammation are at the origin of neovascularization processes. Neovascularization, in turn, affects the function of the retina and causes additional inflammation, establishing a vicious circle that encourages the progress of AMD.
Oxidative Stress
Oxidative stress refers to an imbalance between the number of oxidants (reactive oxygen species) produced in a cell and the capacity of different scavenging enzymes and other molecules with anti-oxidant properties to reduce such an imbalance (Winkler et al., 1999; Beatty et al., 2000; Lin and Beal, 2006; Ambati and Fowler, 2012; Jarrett and Boulton, 2012; Cuenca et al., 2014; Hanus et al., 2015; Tang and Le, 2016). The antioxidant effect is achieved by a cell defense reducing process, carried out by superoxide dismutase, peroxidase, and catalase enzymes.
At early and intermediate stages (I, II) of the disease, key factors for the development of AMD are increased levels of oxidative stress and toxin accumulation in the retina in connection with dysfunction and atrophy of RPE cells responsible for the elimination of toxic accumulations (Winkler et al., 1999; Ambati and Fowler, 2012; Jarrett and Boulton, 2012). Toxic accumulations in the retina can form intracellular remains (lipofuscin and oxidized mitochondria), extracellular waste (drusen) or reactive oxygen species, all of them being some of the multifactorial causes of AMD (Ambati and Fowler, 2012). Among the different waste products being accumulating as the retina ages and as the disease progresses, we would like to underlie the accumulations of lipofuscin -a mixture of oxidized proteins, lipids, carbohydrates, and other types of bioorganic molecules- in the RPE, similar to the accumulation of β-amyloid and tau-protein in other neurodegenerative diseases. The accumulation of these molecules seems to be related to a possible vulnerability of RPE cells to oxidative stress (Crouch et al., 2015; Handa et al., 2019).
Lipids accumulation is considered one of the causes of the inflammation process as well as of the accumulation of complement factors which occurs later. Particularly important is the cholesterol because of the lack of recycling and disposal processes of this type of molecule (Handa et al., 2019). Another component present in lipofuscin is A2E, a natural product of the visual cycle, which accumulates as the disease progresses (Crouch et al., 2015). Closely related to smoking habits, cadmium is another AMD-triggering accumulated molecule. It is one of the toxic products present in tobacco, and it is accumulated in the retinas during the evolution of the disease (Wills et al., 2008).
Altered Cell Functions
Atrophy and disfunction of RPE cells appear in the intermediate phase. These cells are responsible for the elimination of toxic accumulations in the retina, so their atrophy provokes uncontrolled growth of such cumuli which, in turn, generates positive feedback to the previous mechanism. In the dry stage, toxin accumulation also appears within the cells of the RPE (Ambati and Fowler, 2012; Kinnunen et al., 2012; Tarallo et al., 2012).
Also, local inflammation and functional deficits of retinal glial cells impede the development of the molecular processes that are responsible for retinal tissue repair (Cuenca et al., 2014; Tang and Le, 2016). Glia has a strong contribution to AMD development. Under physiological conditions microglia and macroglia (astrocytes and Müller cells) are in continuous communication, reporting on neural activity and maintaining immunomodulatory activity that is responsible for neuroprotection and regulation of cellular activity. In this way, the activation of microglial cells together with their immune response to the lesions contribute to the development and progression of the disease, provoking tissue degeneration, early changes in the pigmentation of the RPE and the formation of drusen (Polazzi and Monti, 2010; Marín-Teva et al., 2011; Cherry et al., 2014; Tang and Le, 2016; Vilhardt et al., 2017; Rathnasamy et al., 2019).
Finally, molecules damaged by oxidants and cellular deposits due to non-immune damage, induce macrophage infiltration and activation and deposition of complement fragments.
Neovascularization
In 10–20% of D-AMD cases, the pathology progresses and the subretinal space is invaded by blood vessels of choroidal origin (choroidal neovascularization) that break into the retinal tissue, provoke inflammation and macular edema, induce serum and/or hemorrhagic detachment of both, the RPE or the neural retina, and, eventually result in fibrovascular disciform scar-associated death of the photoreceptors (Jager et al., 2008; Ambati and Fowler, 2012). Neovascularization alters the geometry, the morphology and the structure of the retina at the macular level that leads to rapid and permanent loss of central vision. Such progression is called wet or exudative AMD (W-AMD). In the transition from D-AMD to W-AMD, the key factor is neovascularization of the retina (Ambati and Fowler, 2012; Cuenca et al., 2014; Tang and Le, 2016). Oxidative stress causes an increase of inflammatory and pro-angiogenic molecules in the subretinal space, which provokes rapid and uncontrolled angiogenesis in the choroid and an invasion of the subretinal space by neovascular vessels.
Chronic inflammation has very important consequences in disease progression. This process triggers the secretion of pro-angiogenic factors by the RPE and other immune cells and also gives rise to further increases in oxidative stress. This increases due to the imbalance between generation and elimination of reactive-oxygen species that creates a toxic retinal microenvironment which eventually leads to the death of the pigment epithelium retinal cells through cell death mechanisms. Furthermore, due to their atrophy, retinal epithelium cells are no longer capable of activating/regulating certain biomolecules derived from the inflammatory process, such as complement factors C3a and C5a (chemotactic agents that cause an increase of leukocytes in the choroid) or some cytokines such as IL1 (Nozaki et al., 2006).
Neovascularization is triggered by increased expression of the vascular endothelial growth factor A (VEGF-A), in charge of increased vascular permeability and vascular endothelial cells recruitment, proliferation and migration. The increased number of macrophages attracted by lipofuscin, cause the secretion of proteolytic enzymes, such as collagenase and elastase (MMP2 and MMP9), which erode BrM, facilitating the penetration of the choroidal neovessels (Risau, 1997; Grossniklaus et al., 2002; Spaide, 2017). In addition, both, astrocytes and Müller cells participate in the development of neo-vessels, releasing angiogenic factors in response to pathogenic stimuli. Müller cells alter the blood-retina barrier, causing infiltration of several blood components including growth factors, cytokines, inflammatory factors and blood-derived immune cells; allowing the growing neo-vessels from the choroid to access the retina through the damaged Bruch’s membrane and the RPE (Bringmann et al., 2009; Nash et al., 2011; Rathnasamy et al., 2019).
Age-related macular degeneration progression is often very slow in the early stages and accelerates in the wet phase. D-AMD usually takes about 5 years to evolve to the wet form (Nussenblatt and Ferris, 2007; Ardeljan and Chan, 2013; Fleckenstein et al., 2018). Although only 10–20% of patients develop W-AMD, this stage is the most important one because it is the origin of more than 80% of severe and very severe vision loss: approximately 40% of patients not correctly treated develop almost complete blindness.
Anti-VEGF Drugs
Even though there is no cure for neither form of AMD, there are several EMA/Food and Drug Administration (FDA)-approved therapeutic approaches focusing on symptomatology relief and on slowing down the progression of the disease, all of them exclusively dedicated to the “wet” form of the disease.
Among them, there are several drugs target directly or indirectly the vascular endothelial growth factor (VEGF). These drugs include pegaptanib, ranibizumab, aflibercept, and brolucizumab. Bevacizumab is another drug, non-FDA-approved for W-AMD treating, but it has been used off-label since 2005, with very good results (Martin et al., 2012). These drugs are IVT administered with monthly or bimonthly frequency (Freund et al., 2013) (anti-VEGF agents are summarized in Table 3). Frequent injections are necessary due to the limited volume of drugs that can be injected in the vitreous each time (Tabandeh et al., 2014) and their limited time of efficacy. The risk of bleeding, retinal detachment, endophthalmitis, cataracts, and infection increases with the number of IVT injections (Jager et al., 2004; Falavarjani and Nguyen, 2013).
An intent to overcome this problem was the development of new molecular drugs with different administration routes, for example oral or topic, which are currently under test (McLaughlin et al., 2013; Jackson et al., 2017; Adams et al., 2018). Unfortunately, even though these routes are non-invasive (e.g., ocular drops), drug arrival to the targeted area, the posterior segment of the eye, is hindered by the several barriers the drugs have to cross (Patel et al., 2013), which severely limits drug bioavailability.
For this reason, work is currently underway to extend the IVT administration beyond 2 months by developing DDD (known also as drug delivery systems, or DDS) that allow a controlled release of the drug, widening its action window and thus lowering the number of interventions needed for AMD treatment per year. The ideal DDD should maintain effective levels of the drug for extended periods, reducing the necessary interventions.
Innovative DDD based on biomaterials in the form of hydrogels, liposomes, colloidal particles, nanoparticles, micelles, dendrimers, or combinations of either of them is currently being tested for the delivery of anti-VEGFs drugs to the posterior segment of the eye. Researchers are working with both, synthetic and natural materials, include hyaluronic acid (Awwad et al., 2019), dextran (Yu et al., 2019), Silk fibroin (Lovett et al., 2015), poly (lactic-co-glycolic acid) (PLGA) (Varshochian et al., 2015), poly(ethylene glycol) (PEG) (Yu et al., 2014), N-isopropyl acrylamide (NIPAAm) (Liu et al., 2019b), etc. Different materials have been modified or combined to create DDD sustainably releasing the drug through time, either right after injection, or on-demand, responding to precise external stimuli, such as light (Basuki et al., 2017; Jiang et al., 2019).
Age-related macular degeneration represents both a challenge and an opportunity for biologists, physicians and biomedical engineers, among others, since many of the processes that give rise to the disease could be controlled by new therapies employing advanced biomaterials-based carriers loaded with biomolecules or drugs that can be introduced into the eye. In the present paper we review the different biomaterial-based anti-angiogenic delivery systems developed and/or tested as AMD therapeutic strategies. We examine hydrogels, colloidal particles, nanoparticles, and implantable drug-delivery devices, with special attention to their capacity of preservation of drugs bioactivity within the biomaterial.
Technologies for Controlled Intraocular Drug-Delivery
As any other ocular disease treatment, AMD pharmacological therapy depends on the pharmacodynamics of the involved agents (molecular characteristics of the drug) which are conditioned by the specific tissue barriers (tear film barrier, corneal and conjunctival barrier, blood-aqueous barrier and finally the blood–retinal barrier-BRB), which in term determine the optimal administration route. Blood–retinal barrier (BRB) increase eye’s resistance to exposure to foreign substances but also to pharmacological agents (Cunha-Vaz et al., 2011; Campbell and Humphries, 2013). There is little convection of molecules, since BRB has no cellular components and is selectively permeable to the most lipophilic molecules (Yasukawa et al., 2004; Kang-Mieler et al., 2020). To avoid BRB, medication to the anterior segment of the eye can be delivered topically and by sub-conjunctival or intracameral injections. In the case of the posterior segment, medication can be delivered topically, systemically, periocularly through the suprachoroidal space, and by IVT or subretinal injections (Kang-Mieler et al., 2014, 2020). As mentioned above, AMD treatments often require repeated IVT injections, whose frequency and duration depend on the course of the pathology in the individual patients, and the same occurs in all other eye degenerative diseases. Although certain benefits are obtained, the excessive repetition of the treatment leads to a worsening of the problem. To reduce that repeat burden we need minimally invasive pharma delivery systems, capable to maintain the necessary drug levels throughout several months or even years and this is precisely one of the actual research lines (Kang-Mieler et al., 2020).
Anti-VEGF molecules are proteins with fragile tertiary and quaternary structure, and they are very sensitive to environmental factors, like heat, pH changes, and proteolytic enzymes, so they require strong preservation measures along the administration route, to maintain intact their physical structure and, consequently, their pharmacological activity (Oo and Kalbag, 2016). Furthermore, since they cannot penetrate the structural barriers of the eye, they have to be IVT injected every 1–3 months (Edington et al., 2017).
Advances in biomaterial engineering and nanotechnology have fueled a growth of the research in prolonged DDD, made by biodegradable microparticles and nanoparticles, hydrogels or eye implanted devices, making them an attractive alternative to the frequent IVT injections (Kang-Mieler et al., 2020). Optimal biomaterials for sustained retinal drugs supply must meet the following properties [Figures 3, 4 (Seah et al., 2020)]:
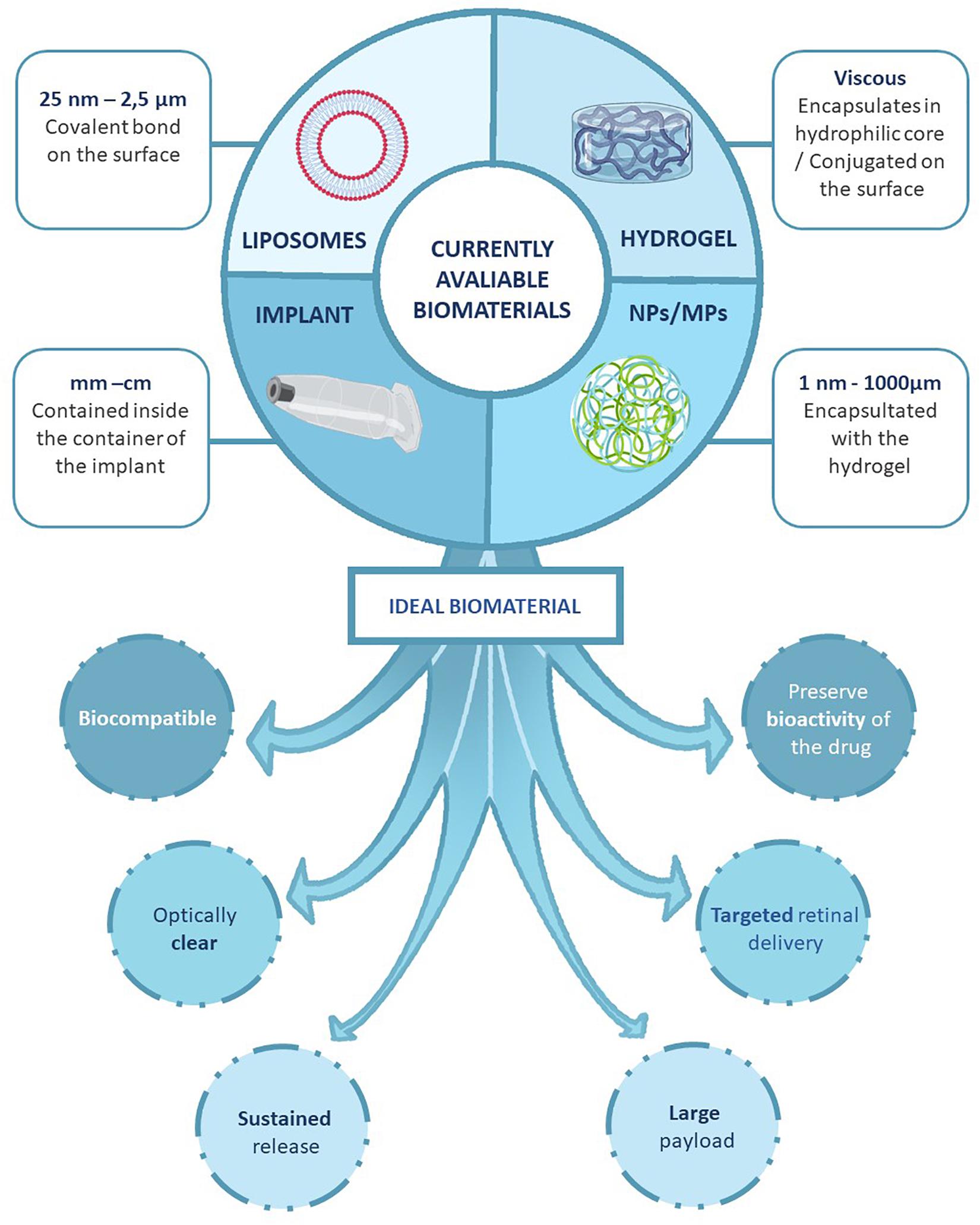
Figure 3. Developing strategies for the sustained drug delivery to the retina. We describe the type of systems that can be used to encapsulate the different molecules can be classified based on the size. The nano-formulations allows the encapsulation of the drugs directly to a molecule that favors the administration and the survival of the substance. The bulk systems protect the drug and allows a progressive administration of the substance during longer periods of time. And, we also represent the ideal characteristics of a sustained drug delivery platform for AMD.
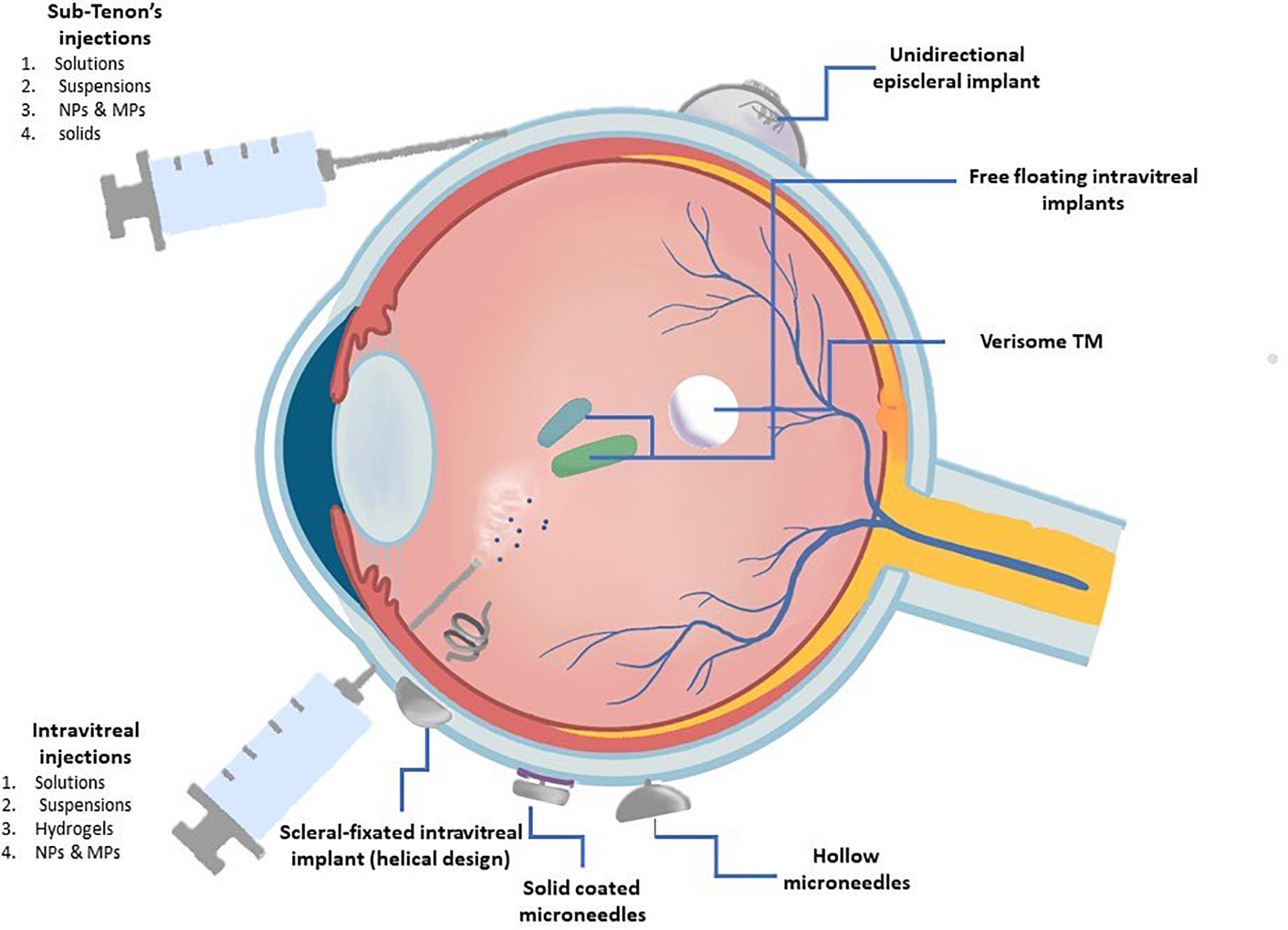
Figure 4. Representation of the ocular drug delivery methods discussed on this review. The treatment of retinal diseases is challenging due to the anatomic barriers and physiological clearance mechanism of the eye. This figure represents the methods currently used in the clinical setting to treat posterior segments diseases classified by their administration route.
• Be able to preserve the bioactivity of the molecule, protecting its tertiary and quaternary structure from denaturation, changes in pH or enzymatic degradation.
• Being able to deliver the molecules to the retina.
• Avoid raising intraocular pressure during administration. The biomaterial should be able to encapsulate a large amount of the drug in a minimal volume; in the case of an anti-VEGF, that means 0.5–2.0 mg of drug within a maximum volume of 0.05–0.1 ml.
• After inoculation, it should be able to maintain molecules’ release for >1 month, thus avoiding frequent administrations.
• Be biocompatible and remain optically transparent within the vitreous humor to avoid any interference with vision.
Drug Delivery Devices (DDD)
Ganciclovir, for retinitis treatment by cytomegalovirus, was the first FDA-approved implantable DDD. With this implant, controlled release rates were obtained, which maintained active drug concentrations below toxic levels, while achieving high drug concentrations with limited systemic side effects (Yasukawa et al., 2004; Ebrahim et al., 2005). With the success of this implant, numerous biodegradable and non-biodegradable devices have been developed and many of them are in clinical use (Kang-Mieler et al., 2020). Implantable devices aim at providing structural/mechanical support for the localization of the drugs in the eyeball and to function as vehicles for controlled releases. Such DDD potentially locate therapeutic agents in the vitreous, lowering systemic exposure and, thus, reducing possible toxicity and/or side effects (Lim et al., 1999; Bourges et al., 2006; Kang-Mieler et al., 2020). They have many advantages over the more traditional administration methods since they improve the convenience, safety and efficacy of pharmacological therapies, bypass BRB and allow the sustained release of the drug directly at the target site, while decreasing the risk of infection or retinal detachment.
Drug delivery devices can be either biodegradable [e.g., PCL, PLA, PGA, PLGA, among others; or non-biodegradable (e.g., silicone), the latter needing to be surgically removed post use]. Ordinarily, implants are placed in the vitreous cavity by surgical intervention, although newer models are placed periocularly (e.g., transsclerally or suprachoroidally) or by IVT injection (del Amo and Urtti, 2008; Gaudana et al., 2009; Patel et al., 2013; Journal et al., 2018). Table 4 show currently available devices, the majority of them still in trial stages.
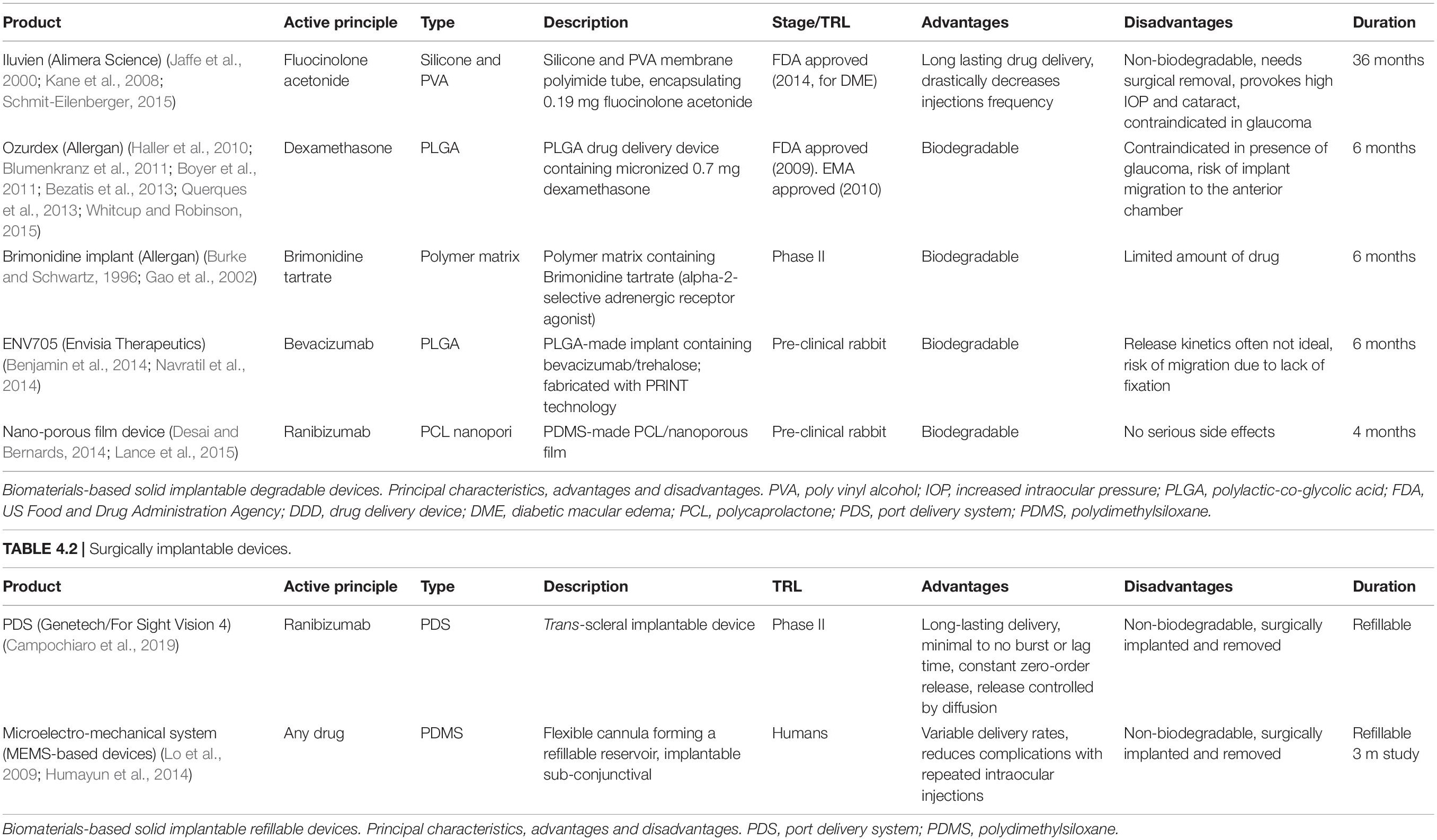
Table 4. Anti-VEGF intravitreally injectable and surgically implantable devices. TABLE 4.1 | Anti-VEGF intravitreally injectable devices (AVEGF-IID).
Intravitreally Injectable Devices
DurasertTM, an EyePoint Pharmaceuticals (Watertown, MA, United States) is a 3.5 mm-long and Ø 0.37 mm solid polymer implant, IVT injectable with a small gauge needle and capable of up-to 3 years release of small molecules. It allows customization of release duration, linear release kinetics, and high drug loading (Callanan et al., 2008; Haghjou et al., 2011; Kang-Mieler et al., 2020). DurasertTM gave origin to two different versions for the administration of Fluocinolone acetonide (Iluvien®) and Dexamethasone (Ozurdex®), as a plausible treatment for macular pathologies. Iluvien (Alimera Sciences, 2019) is a small non-biodegradable silicone implant to diffuse a low dose of fluocinolone acetonide (0.23–0.45 μg/day) for 18–36 months through a PVA capsule. The implant has to be removed surgically, it increases intraocular pressure, provokes cataract and is contraindicated for glaucoma patients (Jaffe et al., 2000; Kane et al., 2008; Ghasemi Falavarjani, 2009; Kuno and Fujii, 2010; Schmit-Eilenberger, 2015; Mandal et al., 2017; Alimera Sciences, 2019; ClinicalTrials.gov, 2020d, f). Iluvien was approved by the FDA in 2014 for the treatment of diabetic macular edema and by the United Kingdom Agency in 2012 for chronic diabetic macular edema-associated visual impairments (ILUVIEN)1. Ozurdex (Allergan) is a biodegradable poly lactic-co-glycolic acid (PLGA) solid implant which delivers dexamethasone. It permits long-lasting drug delivery, but it has serious collateral effects, like increased intraocular pressure, cataract formation and uveitis, due to the side effects of the corticosteroids and to the traction exerted to the vitreous humor. This system is contraindicated in patients with glaucoma. The device was approved by the FDA in 2009 and by the EMA in 2010 (Kuppermann et al., 2007; Haller et al., 2010; Kuno and Fujii, 2010; Blumenkranz et al., 2011; Boyer et al., 2011; Bezatis et al., 2013; Querques et al., 2013; Whitcup and Robinson, 2015; Mandal et al., 2017).
Brimonidine (Allergan) is a biodegradable polymer matrix containing Brimonidine tartrate (alpha-2-selective adrenergic receptor agonist). This molecule is normally used in glaucoma but recent studies suggest it also provides protection of retinal cells from degeneration in AMD geographic atrophy (Burke and Schwartz, 1996; Gao et al., 2002; Ghasemi Falavarjani, 2009; Campochiaro et al., 2012; Lai, 2013). Brimonidine is efficient and lacks toxicity, however, the amount of drug that can be loaded is limited because of the size of the molecule. Additionally, the hydrophilic nature of the molecule limits the sustained-release possibilities. The drug has achieved Phase II clinical trials (ClinicalTrials.gov, 2020c).
ENV705 (Envisia Therapeutics) is an IVT injectable biodegradable implant made with PGA and fabricated with PRINT technology, which provides a sustained release of Bevacizumab for up to 6 months. This implant is capable of releasing effective therapeutic drug concentration, but the release kinetics are often not ideal. The drug is currently in preclinical trials (Benjamin et al., 2014; Navratil et al., 2014).
Zordera is a Ø 10 mm biodegradable nano-porous cylindric device for long-lasting sustained release of Ranibizumab. Made by sandwiched PCL films with millions of porous of the molecule size of the drugs (Bernards et al., 2012; Schlesinger et al., 2015). The device is IVT implanted by a syringe (Desai and Bernards, 2014; Lance et al., 2016). The device is still in preclinical phase (Radhakrishnan et al., 2017; Mandal et al., 2018).
Surgically Implantable Devices
Subconjunctivally implantable pumps are versatile and affordable alternatives, permitting long-lasting, sustained and well controlled drug releases that avoid the need for repeated surgical interventions. Pumps consist of a small reservoir connected to a cannula which is inserted through the sclera into the eye, and they are empowered with a valve to control both, number of the doses and doses volume, thus optimizing and personalizing drug release profiles. They are refillable through a thin needle.
Port Delivery Systems, PDS (Genentech/For sight Vision4, Inc.) is a semipermeable, non-biodegradable refillable transscleral port delivery system, surgically implanted through a 2–3 mm incision and fixed to the sclera to avoid migration. The device has been used for long-lasting sustained release of Ranibizumab. Because of the sclera surface this device permits easy and low invasive transscleral drug delivery with increased absorption rate. However, serious adverse effects were reported, including endophthalmitis and persistent vitreous hemorrhage (Mainardes et al., 2005; Shah et al., 2010; Kim et al., 2017; Mandal et al., 2017; Campochiaro et al., 2019). Phase II clinical trials showed that 15 months recharge frequency with drug concentration of 100 mg/ml have equivalent visual and anatomical effects to patients treated with monthly IVT ranibizumab injections (Campochiaro et al., 2019). Also, implant insertion and reloading procedures were well tolerated by patients. The system is now under Phase III trials, expecting to reach 3 years implants with recharges every 6 months: PORTAL (NCT03683251) and ARCHWAY (NCT03677934) trials, expected to end in January 2022 and April 2022, respectively (ClinicalTrials.gov, 2020b, e).
Replenish® is a sub-conjunctival refillable, non-biodegradable MEMS for long-lasting sustained drug release. It is a sub-conjunctively placed PDMS 0.6 ml refillable reservoir with a flexible cannula and a valve. The cannula is inserted through the sclera and fixed with sutures (Lo et al., 2009). It is refillable up to 10 times with a thin needle. Replenish, Inc., was planning to start Clinical trials for AMD treatment (Lo et al., 2009; Gutiérrez-Hernández et al., 2014; Humayun et al., 2014).
Injectable Hydrogels
To overcome some of the limitations of implantable DDD and to avoid surgeries, injectable hydrogels have emerged as an alternative for IVT drug administration using different biomaterial formats.
A hydrogel is a three-dimensional network of hydrophilic polymers that can swell in water and retain a large amount of H2O molecules while maintaining its solid state (Li and Mooney, 2016). Despite the high solubility of hydrophilic polymers (in water), hydrogels resist dissolution and even interact favorably with H2O molecules, because their hydrophilic polymer chains form a strongly crosslinked network (Li and Mooney, 2016). Their high H2O percentage (>10% of the total weight) make them easy-to-manipulate, flexible materials. Furthermore, their porous structure makes them suitable for encapsulation of drugs, biomolecules, or even stem cells. Hydrogels are highly biocompatible and can undergo a volume phase, or gel-sol phase transition, either spontaneously after injection (in situ-forming hydrogels) or triggered by specific external stimuli (changes of pH, temperature, pressure, light intensity, etc.) (Bahram et al., 2016; Chang et al., 2019). Injected into the eye, hydrogels provide both, structural support and long-term sustained release of the incorporated drugs, molecules or stem cells’ secretome (Alexander et al., 2014; Kirchhof et al., 2015a; Su et al., 2015; Yu et al., 2015; Seah et al., 2020).
The employed polymers can be natural, synthetic or hybrid. Natural polymers (cellulose, chitosan, alginate, hyaluronic acid, silk fibroin, etc.) are degraded by the enzymes of the body, so their biodegradation rate cannot be adjusted (Lovett et al., 2015; Yu et al., 2015). Synthetic polymers (PCL, PEG, PLGA, PLLA, NIPAAm, etc.) properties such as porosity, swelling capacity, stability, mechanical resistance and biocompatibility can be adjustable by varying their chemical composition and preparation methods. Within this group, we find both biodegradable polymers such as PEG (Anwary et al., 2018), PLGA (Arranz-Romera et al., 2019), etc. which can be degraded by the action of biological factors such as changes in pH, increase in temperature, enzymatic activity or by the immune system itself; and non-biodegradable polymers such as PDMS (Pirmoradi et al., 2011; Humayun et al., 2014). Hybrid polymers display the characteristics of their individual components (Yu et al., 2015, 2019; Osswald and Kang-Mieler, 2016; Awwad et al., 2019). Incorporation of therapeutic agents (e.g., anti-VEGF drugs) to a hydrogel strongly depends on the crosslinking of gel’s polymers. Molecules incorporation to a covalently crosslinked gel requires chemical actions, which often result in modification and/or inactivation of the bioactive molecule as well as in increased toxicity (Kang Derwent and Mieler, 2008; Matanović et al., 2014; Awwad et al., 2018, 2019; Seah et al., 2020). On the other side, molecules incorporation to a non-covalently crosslinked gel needs an ad hoc tuning of the manufacturing parameters, so important characteristics of the gel (e.g., gelation time and diffusion rate) become difficult to predict (Seah et al., 2020).
Injectable hydrogels allow reducing drug administration frequency and, even more important, avoid eye procedures-associated complications, including blurred vision or irritation However, hydrogels require IVT administration, show poor penetrability and specificity, and present difficulties for correct sterilization (Alexander et al., 2014; Kirchhof et al., 2015a; Su et al., 2015; Yu et al., 2015; Galante et al., 2018; Seah et al., 2020).
Several hydrogels have been used for drug delivery in different non-ocular pathologies, with favorable results (Sharpe et al., 2014). Despite their theoretical advantages, hydrogels for the ocular route face serious difficulties to meet safety, tolerability, manufacturability, degradability, and facility of administration requirements, while maintaining the bioactivity and clinical effectiveness of the desired drug (Chang et al., 2019). To our knowledge, no hydrogel has been approved by FDA or EMA and only one of them has reached clinical trials stage (Ocular Therapeutix), all other being still in a preclinical stage, Table 5).
Using a bioabsorbable hydrogel (OTX-TKI), Ocular Therapeutix (Bedford, MA, United States) developed a TKI implant, capable to supply TKI for up to 12 months (Jarrett et al., 2017). OTX-TKI is under Phase I clinical study in Australia, to test its safety, durability and tolerability (Eyewire News, 2020). The same company in collaboration with Regeneron (Tarrytown, NY, United States), is developing an injectable version of OTX-IVT to provide sustained aflibercept release for 4–6 months (OTX-IVT, 2020).
Poly (2-ethyl-2-oxazoline)-b poly (ε-caprolactone)-b-poly (2-ethyl-2-oxazoline) (PEOzPCL-PEOz) copolymer-based thermosensitive biodegradable hydrogels for prolonged releases of bevacizumab were shown to be biocompatible in vitro and in vivo with a human retinal pigment cell line and in a lagomorph model, respectively, for 2 months (Wang et al., 2012).
Hydrogels of PEG synthesized via thiol-maleimide reaction from PEG-Mal and PEG-SH, loaded with bevacizumab did not generate cytotoxicity after 7 days of incubation and maintained a sustained drug release for 14 days in vitro, reaching 70% release in this time interval (Yu et al., 2014). Maintenance of drug bioactivity was not tested.
Long-term bevacizumab release from a ESHU hydrogel in vivo was well tolerated, without inflammation and without intraocular pressure alterations in rabbit retinas for 9 weeks. ESHU maintained continuous release with no initial burst and drug concentrations 4.7 times higher than free bevacizumab injections (Rauck et al., 2013).
A thermosensitive poly (N-isopropylacrylamide) hydrogel (PNIPAAm) made by crosslinking PNIPAAm with poly (ethylene glycol) diacrylate (PEG-DA) or poly (ethylene glycol diacrylate)-co-(L-lactic acid) (PEG-PLLA-DA) was capable of locally releasing bevacizumab or ranibizumab for 1 month, without inducing long-term effects on retinal function (Turturro et al., 2011; Drapala et al., 2014).
Silk fibroin hydrogels were studied for bevacizumab sustained delivery in a rabbit model for 3 months showing higher concentrations in day 90 than those in day 30 after direct injections and the gels started to biodegrade after 3 months (Lovett et al., 2015).
Yu et al., 2015 assessed the biocompatibility and release of bevacizumab of an in situ gelling hydrogel made by thiolated functionalized hyaluronic acid and thiolated dextran (HA-VS/Dex-SH) maintained relevant therapeutic concentrations, over 100 times higher than the freely injected drug, 6 months after injection and without inducing pathologies, in a lagomorph model (Yu et al., 2015). In primates, no cytotoxic effects were observed 21 weeks post-injection (Yu et al., 2019).
A thermosensitive hydrogel of methoxy-poly (ethylene glycol)-block-poly (lactic-co-glycolic acid) (mPEG-PLGA-BOX) released bevacizumab, with no burst release nor cytotoxicity and maintaining drug activity during 35 days in a rabbit model (Hu et al., 2019).
NIPAAM and Ac-HA hydrogel combined with bevacizumab was performed in the PK-EyeTM model, an in vitro ocular flow model developed by Awwad et al. (2015) that simulates the times protein clearance at the posterior chamber of the human eye. This hydrogel formulation bevacizumab release for at least 50 days, maintaining a therapeutic dose in the posterior cavity of the eye model and with zero order kinetics post 5 days (Awwad et al., 2019).
Bevacizumab and aflibercept have also been encapsulated in a multiblock EPC thermoresponsive hydrogel consisting of PEG, poly (propylene glycol) (PPG) and poly(ε-caprolactone) (PCL) (Xue et al., 2019). Both anti-VEGF were released similarly and almost linearly for up to 40 days, remaining bioactive in a HUVECs cellular line model in vitro, as well as inhibiting vessel outgrowth in rat ex vivo choroidal explants. In an in vivo retinal neovascularization rabbit model, aflibercept anti-angiogenic bioactivity was maintained for 28 days and animals showed a reduction of vascular leakage.
An injectable polysaccharide cross-linked hydrogel (Alginate-Chitosan) was developed for Bevacizumab delivery and tested in vitro. It achieved a 3-days sustained with 4-h initial bursting (Xu et al., 2013).
Diels-Alder hydrogels synthesized and functionalized with [furyl (-Fur) and maleimide groups (-Mal)] for bevacizumab delivery showed >6 weeks in vitro release (Kirchhof et al., 2015b).
Colloidal Particles
Colloidal systems (liquid suspensions with nanometric carriers) including nanosuspensions, micro/nanoparticles, liposomes, dendrimers and hydrogels have been postulated as promising alternatives for AMD therapy. They are micro/nanosized particles, based on either natural or synthetic materials and composed of highly stable lipophilic, hydrophilic or amphiphilic molecules, loaded with drug molecules (Kandatsu et al., 2005). These particles improve drugs solubility and act as reservoirs for long-term sustained release or for enhanced transport of proteins through the eye barriers (Hora et al., 1990; Herrero-Vanrell and Refojo, 2001; Medina et al., 2009; Li, 2012; Varshochian et al., 2013, 2015; Yandrapu et al., 2013; Ye et al., 2015; Imperiale et al., 2018). Such particles are usually composed of PLA, PLLA, PLGA, PEG, PEG-DA, alginate-chitosan and PLV and are injected IVT; being completely degradable, they do not require subsequent extraction surgery (Mi et al., 2002; Kang-Mieler et al., 2020).
Micro/nanoparticles capacity for tissue penetration, protection capabilities against proteolysis and prolonged drug-release periods depend on the characteristics of their external layer, which in turn depend on the polymer these micro/nanoparticles are made of Sakurai et al. (2001), Sahoo et al. (2008), Honda et al. (2013) and Seah et al. (2020).
Fluorescein-labeled polystyrene micro/nanoparticles studies in a lagomorph model showed that IVT microparticles are transported to the vitreous cavity and to the trabecular meshwork, while nanoparticles are transported to the retinal tissue (Sakurai et al., 2001).
Micro/nanoparticles provide several benefits as drug carriers for posterior ocular drug delivery, but the main one is that they are capable of increasing drug penetration across the conjunctival and corneal epithelia by temporarily altering the tight junctions when administered topically (Hans and Lowman, 2002). In addition to their ability to increase trans-corneal penetration, the ability to improve solubility of lipophilic drugs may play an important role in increasing IVT half-life and thus bioavailability of lipophilic drugs. This approach also provides sustained release of the encapsulated molecules. In cases with low encapsulation efficiency where it is difficult to establish or control the conditions of drug release, the systems can be modified for tissue-specific uptake and to protect the therapeutic molecules from degradation. Despite its advantages, there are limitations associated with formulation stability, high initial burst release, protein denaturation, clearance by the immune system, control of particle size, control of drug release rate and large-scale manufacturing of sterile preparations (Sakurai et al., 2001; Hans and Lowman, 2002; Kang-Mieler et al., 2020; Seah et al., 2020). Still, many of these particles are being evaluated preclinically for the administration of drugs into the posterior segment of the eye as carriers of anti-VEGF factors for AMD.
Intravitreal injections of PLGA microspheres-encapsulated bevacizumab in a lagomorph model showed higher concentrations in the vitreous and aqueous humor than of freely-injected drug, and a maintenance of their pharmacological activity for over 42 days (Ye et al., 2015). However, authors did not determine drug’s ability to reach the target tissue, neither its long-lasting effects.
PLGA-albumin nanoparticles for sustained supply of bevacizumab, made from a double water-in-oil-in-water emulsion showed bioactive IVT concentrations (>500 ng/ml) for 8 weeks after IVT injection in a rabbit model (Varshochian et al., 2015). More recently, PLGA nanospheres-encapsulated fenofibrate showed full biocompatibility and attenuation of both, VEGF expression and vascular tissue disruption for up to 60 weeks after IVT injection in a rat W-AMD model, a diabetic retinopathy rat model and a very low-density lipoprotein receptor knockout (Vldlr–/–) mouse model (Qiu et al., 2019). Despite PLGA’s good biodegradability and biocompatibility, one of the main problems is that PLGA degeneration causes an accumulation of lactic and glycolic acid in the sphere, which provokes a decrease of the pH and a consequent denaturation of the drug (Liu et al., 2019a, b; Qiu et al., 2019). A possible solution to this problem is the manufacture of monomer conjugated PLGA microspheres which prevent rapid degradation of the biomaterial and preserve the biocompatibility of the drug for a longer time.
Silk fibroin abilities as nanocarrier for anti-VEGF particles has been tested by encapsulating bovine serum albumin (BSA, macromolecular model with similar weight to the antibodies that are usually used in AMD) showing a prolonged retention compared to free BSA solution and better distribution in in vitro studies with ARPE-19 and in vivo studies in a rabbit model (Yang et al., 2019).
The biggest limitation of the use of micro/nanoparticles is the small amount of drug that they are able to encapsulate inside, therefore, prolonged treatments require frequent IVT injections that can cause discomfort to the patient and even eye damage during the procedure (Seah et al., 2020).
Another colloidal system used today are liposomes (Abrishami et al., 2009; Shah et al., 2010; Patel et al., 2013; Davis et al., 2014; Ameeduzzafar et al., 2016), vesicles consisting of a phospholipid bilayer membrane incorporating water-soluble and lipid-soluble drugs in aqueous and lipid phases, respectively. Compared to nanoparticles, liposomes usually have low immunological reactivity or toxicity since phospholipids are easily metabolized once the liposome is degraded (Seah et al., 2020).
These vesicles provide sustained release of drugs with decreased frequency of dosing. The systems also allow for modifications of the rate of drug release and the ability to provide stimulus-sensitive drug release. However, these vesicles present some limitations including blurred vision after injection of the liposomal suspension into the vitreous body, low reproducibility, instability of the macromolecules during production, variable size distribution and storage conditions specific to the composition of the drug and liposome.
Numerous animal studies have shown that IVT injected liposomal drugs release their aqueous contents slowly, protecting the intercalated substances from degradation and clearance. This process avoids the toxicity from the high peak concentrations normally seen after injection of free drug. Therefore, the liposomal drug release formulations may lead to greater clinical efficacy by maintaining therapeutic concentrations for longer time intervals. Liposomal applications in the posterior segment provide longer clearance times, less toxicity, and specific delivery.
Furthermore, the surface of the liposomes can be functionalized to improve penetration through the different tissues and to reach the target tissue. In a study by Davis et al. (2014), the liposome surface was modified using annexin-A5 with the aim of improving the administration of Bevacizumab through the epithelial barriers of the cornea (Davis et al., 2014). This study verified that annexin functionalization allowed liposomes to reach the retina more easily (however, the results still require improvement since only 1% of the dose reached the target).
In other studies, such as Honda et al. (2013) in which the effects of Semaxanib-SU5416 (VEGF receptor proteinTKI, an angiogenesis inhibitor) were evaluated, it was demonstrated that the use of liposomes allowed to decrease choroidal neovascularization (CNV) activity in animal model compared to the control group. That is, liposomes allowed the drug to reach the target tissue more easily than the drug alone (Honda et al., 2013).
Studies have shown that the release of anti-VEGF drugs in liposomes can be maintained for more than a month without causing cytotoxicity (Mu et al., 2018). In the latter study, they demonstrated that changing the aqueous/lipid ratio improved the encapsulation efficiency of the drug (Bevacizumab) in Brown-Norway rats with laser-induced choroidal neovascularization. IVT liposome-treated eyes with bevacizumab in rabbits maintained high drug levels for >56 days.
The limitations that exist in the use of liposomes as a drug transport system must be taken into account. One of the main limitations of this colloidal system is the lack of studies that exist today. Drug release from liposomes to target tissue is highly dependent on environmental conditions such as pH or enzyme activity (Villegas et al., 2017). Because there are so many factors that can affect liposome stability, it is difficult to predict drug release. Therefore, although liposomes have structural characteristics that allow effective administration of the drug and degradation of the transport system that does not cause toxicity, a more exhaustive study of the environmental factors that cause the degradation of liposomes is necessary.
A slightly different device is Verisome IBI-20089 (Icon bioscience), actually in Phase I/II clinical trials (ClinicalTrials.gov, 2020a), consisting of a biodegradable non-polymer injectable liquid (carbonates, tocopherols, and citrate ester) that forms small spherules once injected into the vitreous. It has been formulated to deliver intravitreal injections of triamcinolone acetonide (TA) in combination with ranibizumab in W-AMD for >1 year, showing good safety and efficacy (Lim et al., 2015).
Table 6 summarizes some of the colloidal systems (micro/nanoparticles and liposomes) for AMD therapies.
Compound Particles-Hydrogels Drug Delivery Technologies
Despite the already discussed advantages of injectable polymeric micro/nanoparticles, one of their main limitations is locating them at the injection site of the eye. A normal eye can eliminate microparticles in 50 days and vitrectomized ones in 14 days (Morirero et al., 1991). As a solution to limit the movement of these particles, the use of injectable particles-hydrogels has been proposed, to provide a prolonged and localized release of the drug after injection (Kang-Mieler et al., 2017). These compound systems offer advantages over each of the two platforms separately, by reducing the initial explosion and extending the release time (Osswald and Kang-Mieler, 2015, 2016).
A thermosensitive biodegradable hydrogel-microspheres fabricated by suspending, poly (lactic-co-glycolic acid) (PLGA) microspheres with ranibizumab within a poly (ethylene glycol)-co-(L-lactic-acid) diacrylate/N-isopropylacrylamide (PEG-PLLA-DA/NIPAAm) hydrogel, achieved a controlled release of ranibizumab and aflibercept for 6 months (Liu et al., 2019b, c), while efficacy was tested in vivo in a rodent choroidal neovascularization model (Osswald et al., 2017; Liu et al., 2019c).
The anti-angiogenic and anti-inflammatory properties of cerium oxide nanoparticles coated with oligo-chitosan in alginate hydrogel were studied in an in vitro model of human retinal pigment epithlium-19 (ARPE-19) and umbilical endothelial cell lines. Sustained nanoparticles release and controlled hydrogel degradation with strong antioxidant properties and reduction of apoptosis were observed for 2 months. Furthermore, in the same model, they suppress bacterial lipopolysaccharides-induced inflammatory responses, inhibit VEGF expression and reduced cell apoptosis (Wang et al., 2018).
Externally Light-Triggered Drug Release Hydrogels or Nanoparticles
Extending the half-life of the therapeutic agent can reduce dosage, costs and injections frequency. Alternative strategies to increase the half-life of anti-angiogenic drugs after IVT injection without losing effectiveness include nanotechnology-based DDD, for example hydrogels, liposome, microsphere, micro/nanoparticles, etc. (Fuchs and Igney, 2017; Machinaga et al., 2018; Nayak and Misra, 2018). Nevertheless, none of them allows an external control of the timing of the release.
To overcome this drawback several photoactive biomaterials have been developed which release the drug after being exposed to an external ultraviolet (UV) or visible light stimulus. Light-responsive systems can be classified into three categories according to the light-triggering mechanism for drug release: (1) photochemical, where light breaks a covalent bond (2) photoisomerization, where light induces conformational changes, and (3) photothermal, where light produces heat after photoexcitation to selectively affect thermally modulated components of the system (Linsley and Wu, 2017). This is a well-known technology, mainly tested in cancer therapy, translated to posterior segment eye diseases due to the transparency of the cornea, which allow easy light stimulation of the back of the eye. Nevertheless, very few studies have been dedicated to AMD treatment (Table 7).
We have identified two systems with photochemical and two with photothermal activation of drug release for AMD treatment.
A nanoparticle storage platform for on-demand drug delivery, based on a UV ultra-degradable polymer, which releases drug after a brief exposure to 365 nm UV light (Fomina et al., 2010; Huu et al., 2015). This polymer contains a fraction of o-nitrobenzyl in each monomer, responding to the absorption of UV by degrading into fragments and small molecules through quinone-methide rearrangements. The system releases drug for >30 weeks post-injection and authors reported light-induced release of Nintedanib, an angiogenesis inhibitor, as well as suppression of choroidal neovascularization in rats for >10 weeks (Huu et al., 2015).
A nanoparticles-based DDD which, injected intravenously, can release the drug into the eye by 400 nm light activation was developed and tested by Wang et al. (2019). Nanoparticles (NP-CPP) were composed of poly (ethylene oxide)-poly (D, L-lactic acid) (PEG-PLA) chains modified with Tat-C peptide (CPP), that allows their entry into cells. CPP is reversely binded by a covalent bond to a DEACM group that prevents entry into cells. After the exposure to light, DEACM releases CPP, which migrates from the center of the nanoparticle to the surface, activating it. Toxicity and biocompatibility of the system were tested both, in vitro and in vivo. Irradiation of the eyes after intravenous injection provoked NP-CPP accumulation in mouse neovascular lesions. Doxorubicin-loaded NP-CPP significantly reduced the size of the neovascular lesion, the majority of the drug being released 48 h post-irradiation.
Chromophores coupled with a thermally responsive drug-releasing materials gave origin to two different photothermal DDD (Basuki et al., 2017; Jiang et al., 2019).
The former is based on a photo-thermal interaction of polymer-coated AuNPs within an agarose hydrogel. Stimulation by 400–500 nm-light radiation increases local temperature which in turn causes a reversible softening of the hydrogel matrix and a consequent release of the drug. Release profile is adjusted by modifying AuNPs and agarose concentrations, light intensity and exposure time. Bevacizumab was showed to maintain affinity for binding to recombinant human VEGF-165 up to 15 days in vitro (Basuki et al., 2017).
A dual drug release system, acting by near-infrared light (808 nm) stimulation was developed and tested by Jiang et al. (2019). Nanogel-crosslinked thermosensitive hydrogels, embedded in gold nanorods (AuNR) were prepared by the precipitation polymerization of poly (N-isopropylacrylamide) (NIPAAm) with MBA as crosslinker and the hydrogels were prepared by radical polymerization of NIPAM with nanogels as crosslinker. Two types of drugs are encapsulated, one in the nanogel (doxorubicin) and the other in the AuNR-enriched hydrogel (curcumin). NIR light provokes a heating effect in the AuNRs, which induces a volume phase transition of the hydrogel. As a result, the drugs are released. In the first cycle, 19% of curcumin and 10% of doxorubicin were released. Curcumin is released first, and doxorubicin is released second (Jiang et al., 2019).
Drug Administration
Non-invasive drug administration methods represent a very worthy objective but in clinical ophthalmology this is very long-term challenge. For eye treatment, these methods need to strongly improve tissue penetrability and targeting while reducing drug dispersion levels. As stated above, the structural complexity of the eye is a serious obstacle, especially when drugs have to reach the back segment. On the other side, eye barriers exclude several types of treatments, like the topically administered ones.
Anti-VEGF IVT injections, although less aggressive against drugs’ structural conformation, necessary for the effectiveness of their therapeutic principles, are not efficient. This is due to the small percentage of the drug capable of reaching the target tissue, mainly due to its dispersion and a rapid deterioration in the aqueous medium. Furthermore, IVT injections are associated with multiple complications, like infections, which can cause permanent loss of vision (Mason et al., 2008; Bhavsar et al., 2009). Consequently, multiple injections (monthly or bimonthly) are necessary to treat chronic eye diseases, which ultimately increases infection risks, patients’ discomfort and caregiving complexity.
Biomaterial-based drugs administration to extend the time between injections is the most promising approach for short-term and mid-term clinical solutions. In recent years, the use of biomaterials has been widely studied and characterized as a transport vehicle for drug administration in the eye, especially for non-biodegradable implants, colloidal systems and hydrogels. Solid carriers improve visual recovery speed, with lower vision loss risk, and avoiding multiple injections-associated side effects. However, they can increase intraocular pressure as well as to induce local adverse effects and cataract progression due to traction exerted on the vitreous humor. Solid carriers have also an inherent risk of tissue damage. As mentioned above, an ideal biomaterial for drug delivery must be able (i) to preserve physical and chemical integrity of the transporting substance (ii) to prevent its dispersion in the eye tissues/media (iii) to make it reach the target tissue and (iv) to encapsulate a large amount of drug in the smallest possible volume.
Retention of Bioactivity Within Drug Delivery Depots
Most of the molecules employed in AMD treatment formulations are sensible to external factors such as temperature, pH, and enzymatic activity (Oo and Kalbag, 2016), and, like conventional drugs formulations, they exhibit chemical stability problems. If we focus on macromolecules, such as proteins, chemical stability challenges pairs with physical stability problems related to their tertiary structure, the maintenance of which is determines the bioactivity of the molecule (Rakesh, 2019). Chemical stability is disrupted when covalent bonds are broken and/or created in the molecule; in contrast, physical degradation occurs when non-covalent forces required to maintain the secondary, tertiary, or quaternary structure of the molecule are broken (Deb et al., 2018). Encapsulating drugs on biomaterials avoid some of these stability problems, which prevents an initial exposure of the drug to the external environment. Drug encapsulation has other benefits like the controlled drug release and decreased need for new doses administration. Moreover, the porosity of the material provides a high surface area-to-volume ratio, enhancing drug loading capacity and improving drug release profile. Nevertheless, drug encapsulation compromises bioactivity stability, with many drugs losing it when formulated in a drug delivery depot (Koerselman et al., 2020).
Large molecules are more prone to lose bioactivity via cleavage or conformational changes when encapsulated. This loss of bioactivity can be influenced by the distribution of the drug within a fibrous material (Tang, 2015). An adequate distribution of the drug far from the surface, within the material, also avoids burst release profiles (Awwad et al., 2018). Moreover, the methods used in the encapsulation process play a great role in the preservation of the bioactivity. For instance, hydrosoluble molecules are more like to maintain their bioactivity if they are exposed to less organic solvents during the process (Wilkinson et al., 2017). Another strategy that has proven to preserve drug bioactivity is to embed drugs in electrospun fibers, with hydrophilic solution core (Saraf et al., 2009). Besides, coaxial fibers fabricated by coaxial electrospinning (Qin, 2017), have shown greater potential in maintaining bioactivity and extended-release of drugs than conventional electrospun fibers (Saraf et al., 2009). Activity preservation faces other challenges in drug encapsulation in nanofibrous scaffolds. Here, drug suspension has to have good solubility, be as homogeneous as possible, be regularly immobilized in the material, and be capable to maintain its bioactivity. Needless to say, these requirements are difficult to meet, and both, pre- and post-fabrication methods need a radical improvement to reach the goals (Tiwari and Tiwari, 2013).
The principal challenges of refillable non-biodegradable implants capable for prolonged drug delivery periods [among the most advanced DDD, currently under clinical trials (Seah et al., 2020)] are to increase the volume capacity of the devices and, at the same time, to achieve a slow and uniform release speed. The idea is to dispose of large amounts of drug, to prevent the dispersion of drug molecules, as well as to slow down drug spreading and degradation rate and, consequently, to reduce interventions frequency to <1/year (Bansal et al., 2016; Rupenthal, 2017; Shen et al., 2018). Functionalize biomaterials by embedding specific drug molecules can provide a sustained, diffusion-independent drug release format (Bansal et al., 2016; Rupenthal, 2017; Shen et al., 2018), adaptable to the desired release kinetics by adjusting the degradation dynamics of the employed scaffold.
Port delivery system and micropumps have been efficient in both, preclinical and clinical trials for chronic retinopathies’ treatments (Campochiaro et al., 2011; Haghjou et al., 2011; Pirmoradi et al., 2011; Kang-Mieler et al., 2020), respectively. PDS systems are one of the very few transport systems that have been able to successfully complete clinical trials, with controlled long-term diffusion of the drug: a 220 patients Phase II clinical trial with Ranibizumab reported good PDS tolerance and maintenance of the therapeutic levels during the 9 months of the study (Campochiaro et al., 2019). However, benefits are counterbalanced by collateral risks, either related to the surgical processes or to the non-biodegradability of the implant which may lead to inflammatory processes. Indeed, in the above trial, about 5% of the patients developed surgery-originated vitreous hemorrhage.
Technological Solutions
Hydrogels are very promising materials for sustained ophthalmic drug delivery. Their high-water content makes them very biocompatible and transparent and their porous structure acts as a protective barrier against denaturant environmental agents while allowing a large amount of drug to be loaded (Kang Derwent and Mieler, 2008; Matanović et al., 2014; Anwary et al., 2018). “Intelligent” hydrogels are able to specifically react in presence of external environmental factors like pH or temperature (Kang Derwent and Mieler, 2008; Matanović et al., 2014; Seah et al., 2020).
Several preclinical and clinical trials provided evidence for sustained drug supply, improvement of lesions and retinal functionality, with little or no toxicity of the biomaterials (Turturro et al., 2011; Agrawal et al., 2012; Drapala et al., 2014; Yu et al., 2015; OTX-IVT, 2020). However, hydrogels present several disadvantages like sterilization difficulties, risk of harm to biopharmaceuticals due to chemical crosslinking reactions, risk of toxic effects caused by polymerization initiator, presence of toxic residuals after polymerization, and difficulties in the control of drug release rate and degradation kinetics due to water absorption during swelling (Kanjickal et al., 2008; Karajanagi et al., 2011; Hammer et al., 2015; Kirchhof et al., 2015a; Baino and Kargozar, 2020).
Colloidal biofunctionalized materials have been designed to provide a format independent sustained drug release (Bansal et al., 2016; Rupenthal, 2017; Shen et al., 2018). Administration route, release rate and diffusion of the drug into the tissue directly depend on the size, structure and stability of the employed molecule (Seah et al., 2020). Drug release can also be tuned to the desired kinetics by adjusting the degradation dynamics of the scaffolding biomaterial.
Liposomes can incorporate either hydrophobic or hydrophilic compounds (e.g., anti-VEGF). They have adjustable drug release kinetics, phospholipids are metabolized as the liposome is being degraded and, compared to polymeric microparticles, they have longer half-time and lower immunogenicity and toxicity (Seah et al., 2020). Furthermore, their surface can be modified with proteins or polymers, providing additional functionalities, such as light or chemical activation, allowing specific and precise pharmacological release (Baino and Kargozar, 2020). Verteporfin was the first liposome-based drug used for photodynamic therapy of neovascular AMD, improving the topical release of bevacizumab with the use of surface-modified liposomes (Bressler et al., 2002; Fenton and Perry, 2006; Lajavardi et al., 2007). In animal models, several IVT- administered liposomes-formulated DDD have reached >1 month sustained anti-VEGF release rates (Abrishami et al., 2009; Mu et al., 2018). However, it is still unknown how liposomes interact with physiological processes, being difficult to predict the duration of sustained supply (Honda et al., 2013). For example, their stability can be compromised by the enzymatic activity or the pH of the eye, while liposomes themselves can be destabilized and ingested by macrophages (Yatvin et al., 1980; Moghimi et al., 1989). Furthermore, they have a limited drug encapsulation capability; complications with sterilization procedures and they present a risk of blurred vision after IVT injections is high (Baino and Kargozar, 2020).
Nanoparticles can encapsulate molecules, proteins, peptides and vaccines, as well as hydrophilic and hydrophobic biological macromolecules and their low cytotoxicity makes them good candidates for safe eye DDD. Their physical and chemical characteristics can be modified to fit specific pharmacological objectives (Hans and Lowman, 2002).
They have achieved several weeks of slow and sustained drug release, with some therapeutic effects, like a reduction of neovascularization damages and some improvement of the visual function (Varshochian et al., 2013, 2015; Tanetsugu et al., 2017; Narvekar et al., 2019; Qiu et al., 2019).
Despite their advantages, these systems have a low protein encapsulation efficiency (<60% for microparticles and <30% for nanoparticles), high initial release rates (20–50% of the drug in 24 h), incomplete release and loss of bioactivity of the drug during release (Yeo and Park, 2004; Manoharan and Singh, 2009; Herrero-Vanrell et al., 2014). Movement of microparticles has also been observed from the target site (Morirero et al., 1991). To limit such movement it has been proposed the simultaneous use of injectable hydrogels, to limit particles’ mobility and allow a prolonged drug release (Kang-Mieler et al., 2017). These particulate-hydrogel systems offer advantages over each of the platforms separately, as the release time is extended and the initial burst of the drug is reduced (Osswald and Kang-Mieler, 2015, 2016). Besides, since the number of microspheres suspended in the hydrogel can be controlled, the total amount of drug delivered can be controlled without changing the volume and injectability of the system (Osswald and Kang-Mieler, 2015, 2016; Bhatt et al., 2019; Liu et al., 2019c). Also, these hydrogels combined with microparticles are biocompatible and inhibit the expression of VEGF (Wang et al., 2018). However, studies to date only consist of in vitro and in vivo preclinical trials, so studies in patients have not yet been achieved. Finally, unlike other PLGA-based applications which have been proven to be safe, the small size of the micro/nanoparticles systems has, to date, limited a through test of biocompatibility and safety of the materials (Sakurai et al., 2001).
Conclusion
To date, AMD is an untreatable neurodegenerative disease that represents a major and growing worldwide public health burden. The use of biomaterials for the sustained administration of drugs in the treatment of AMD is a promising methodology but it needs a long personal-, material- and time-effort to approximate clinical application. The immense majority of the biomaterials are still far from performing clinical trials. One of the main problems of the proposed therapies is their limited capacity for drug storage. IVT injectable and surgically implantable devices are the less elegant and oldest technology DDD, not lacking serious side effect consequences. However, they are the only available therapeutic offer for AMD patients, and they will continue to be in the near future. Improvement of these technologies is and will be extremely important for both, AMD suffering persona and for the pharma industry. Hydrogels are promising candidates due to the number of biodegradable and biocompatible components, in addition to their structural characteristics (resistance, transparency, water-holding capacity, etc.). Colloid particles are postulated as better candidates, but the development of real alternatives is still in the beginning. Probably the best therapies will combine several of the above materials and strategies to increase therapeutic effects, to reduce interventions frequency and to decrease the risks associated with such interventions.
Author Contributions
NJ-D and AG-D: bibliographic research and information synthesis, AMD course, writing the manuscript, equal contribution. MF-A, NS-B, and NA-L: bibliographic research and information synthesis. FA-M: clinical aspects. JP-R, FR, GG, and DK: biomaterials aspects. DG-N: biomaterials, cell and tissue therapy. GG, JP-R, DK, and DG-N: manuscript revision. FP: manuscript design, information synthesis, supervision, writing the manuscript. All authors contributed to the article and approved the submitted version.
Funding
The authors gratefully acknowledge financial support received from the Spanish Ministerio de Economía y Competitividad through grants MAT2016-76847-R, MAT2016-79832-R and MAT2015-66666-C3-3-R; from the Comunidad de Madrid, Spain through grants Neurocentro-B2017/BrMD-3760 and IND2018/BrMD-9804, from the National Institutes of Health (P41EB002520); and predoctoral FPI grant from the Spanish Ministerio de Economía y Competitividad (AG-D) and research contract from the Comunidad de Madrid, Spain (MF-A).
Conflict of Interest
The authors declare that the research was conducted in the absence of any commercial or financial relationships that could be construed as a potential conflict of interest.
Abbreviations
A2E, N-retinyl -N-retinylidene ethanolamine; AC-H, alginate-chitosan hydrogels; Ac-HA, acylated hyaluronic acid; AMD, age-related macular degeneration; ARPE-19, human retinal pigment epithelial cell line-19; AuNPs, gold nanoparticles; BRB, blood–retinal barrier; BrM, Bruch’s membrane; CPP, cell penetrating peptide; D-AMD, Dry Age-Related Macular Degeneration; DDD, drug delivery device; DEACM, 7-(diethylamino) coumarin-4-yl] methyl carboxyl; Dex-SH, thiol-functionalized polysaccharide dextran; EMA, European Medicines Agency; EPC, poly (ether ester urethane); ESHU, poly (ethylene glycol)-poly (serinol hexamethylene urethane); FDA, US Food and Drug Administration Agency; IAPB, International Agency for the Prevention of Blindness; IL, interleukins; IVT, intravitreally; MBA, N, N ′-methylenebisacrylamide; MEMS, microelectromechanical system; mPEG-PLGA, methoxy-poly (ethylene glycol) block-poly lactic-co-glycolic acid; mPEG-PLGA-BOX, methoxy-poly (ethylene glycol)-block-poly (lactic-co-glycolic acid); PCL, polycaprolactone; PDMS, polydimethylsiloxane; PDS, port delivery system; PEG, poly (ethylene glycol); PEG-DA, polyethylene glycol diacrylate; PEG-PLA, poly (ethylene oxide)-poly (D, L-lactic acid); PEG-PLLA-DA, poly (ethylene glycol diacrylate)-co-(L-lactic acid); PEG-PLLA-DA/NIPAAm, poly (ethylene glycol) -co- (L-lactic-acid) diacrylate/N-isopropylacrylamide; PEOzPCL-PEOz, Poly (2-ethyl-2-oxazoline)-b poly (ε -caprolactone)-b-poly (2-ethyl-2-oxazoline); PGA, polyglycolic acid; PLA, polylactic acid; PLGA, poly(lactic-co-glycolic) acid; PLLA, poly (L-lactic acid); PLLA/PLGA, poly (L-lactic acid)/poly (lactic-co-glycolic acid); PLV, phospholipid vesicles or liposomes; PNIPAAm, Poly N-isopropylacrylamide; PPG, poly (propylene glycol); PVA, poly vinyl alcohol; RPE, retinal pigment epithelium; TKI, tyrosine kinase inhibitor; TRL, clinical trials; UV, ultraviolet; VEGF, vascular endothelium growth factor; VS-HA, vinylsulfone functionalized glycosaminoglycan hyaluronic acid; HA-VS/Dex-SH, thiolated functionalized hyaluronic acid and thiolated dextran; W-AMD, Wet Age-Related Macular Degeneration; WHO, World Health Organization.
Footnotes
- ^ ILUVIEN. 190 Micrograms Intravitreal Implant in Applicator - Summary of Product Characteristics (SmPC) - (emc). Available online at: https://www.medicines.org.uk/emc/medicine/27636#AUTHDATE (accessed August 15, 2020).
References
Abrishami, M., Zarei-Ghanavati, S., Soroush, D., Rouhbakhsh, M., Jaafari, M. R., and Malaekeh-Nikouei, B. (2009). Preparation, characterization, and in vivo evaluation of nanoliposomes-encapsulated bevacizumab (avastin) for intravitreal administration. Retina 29, 699–703. doi: 10.1097/IAE.0b013e3181a2f42a
Adams, C. M., Anderson, K., Artman, G., Bizec, J. C., Cepeda, R., Elliott, J., et al. (2018). The Discovery of N-(1-Methyl-5-(trifluoromethyl)-1H-pyrazol-3-yl)-5-((6- ((methylamino)methyl)pyrimidin-4-yl)oxy)-1H-indole-1-carboxamide (Acrizanib), a VEGFR-2 inhibitor specifically designed for topical ocular delivery, as a therapy for neovascular age-. J. Med. Chem. 61, 1622–1635. doi: 10.1021/acs.jmedchem.7b01731
Agrawal, A. K., Das, M., and Jain, S. (2012). In situ gel systems as “smart” carriers for sustained ocular drug delivery. Expert Opin. Drug Deliv. 9, 383–402. doi: 10.1517/17425247.2012.665367
Alexander, A., Ajazuddin, Khan, J., Saraf, S., and Saraf, S. (2014). Polyethylene glycol (PEG)–Poly(N-isopropylacrylamide) (PNIPAAm) based thermosensitive injectable hydrogels for biomedical applications. Eur. J. Pharm. Biopharm. 88, 575–585. doi: 10.1016/J.EJPB.2014.07.005
Alimera Sciences (2019). Alimera Sciences. Available online at: https://alimerasciences. com/ (accessed March 19, 2019).
Ambati, J., and Fowler, B. J. (2012). Mechanisms of age-related macular degeneration. Neuron 75, 26–39. doi: 10.1016/j.neuron.2012.06.018
Ameeduzzafar, A., Ali, J., Fazil, M., Qumbar, M., Khan, N., and Ali, A. (2016). Colloidal drug delivery system: amplify the ocular delivery. Drug Deliv. 23, 710–726. doi: 10.3109/10717544.2014.923065
Anderson, D. H., Mullins, R. F., Hageman, G. S., and Johnson, L. V. (2002). A role for local inflammation in the formation of drusen in the aging eye. Am. J.Ophthalmol. 134, 411–431.
Anwary, M., Kumar, P., du Toit, L. C., Choonara, Y. E., and Pillay, V. (2018). Polymeric, injectable, intravitreal hydrogel devices for posterior segment applications and interventions. Artif. Cells Nanomed. Biotechnol. 46, 1074–1081.
Ardeljan, D., and Chan, C.-C. (2013). Aging is not a disease: distinguishing age-related macular degeneration from aging. Prog. Retin. Eye Res. 37, 68–89. doi: 10.1016/j.preteyeres.2013.07.003
Arranz-Romera, A., Davis, B. M., Bravo-Osuna, I., Esteban-Pérez, S., Molina-Martínez, I. T., Shamsher, E., et al. (2019). Simultaneous co-delivery of neuroprotective drugs from multi-loaded PLGA microspheres for the treatment of glaucoma. J. Control. Release 297, 26–38. doi: 10.1016/j.jconrel.2019.01.012
Awwad, S., Abubakre, A., Angkawinitwong, U., Khaw, P. T., and Brocchini, S. (2019). In situ antibody-loaded hydrogel for intravitreal delivery. Eur. J. Pharm. Sci. 137:104993. doi: 10.1016/j.ejps.2019.104993
Awwad, S., Al-Shohani, A., Khaw, P. T., and Brocchini, S. (2018). Comparative study of in situ loaded antibody and PEG-Fab NIPAAM Gels. Macromol. Biosci. 18:1700255. doi: 10.1002/mabi.201700255
Awwad, S., Lockwood, A., Brocchini, S., and Khaw, P. T. (2015). The PK-Eye?: a novel in vitro ocular flow model for use in preclinical drug development. J. Pharm. Sci. 104, 3330–3342. doi: 10.1002/jps.24480
Bahram, M., Mohseni, N., and Moghtader, M. (2016). “An introduction to hydrogels and some recent applications,” in Emerging Concepts in Analysis and Applications of Hydrogels, ed. S. B. Majee (London: InTech), doi: 10.5772/64301
Baino, F., and Kargozar, S. (2020). Regulation of the ocular cell/tissue response by implantable biomaterials and drug delivery systems. Bioengineering 7, 1–30. doi: 10.3390/bioengineering7030065
Bansal, P., Garg, S., Sharma, Y., and Venkatesh, P. (2016). Posterior segment drug delivery devices: current and novel therapies in development. J. Ocular Pharmacol. Ther. 32, 135–144. doi: 10.1089/jop.2015.0133
Basuki, J. S., Qie, F., Mulet, X., Suryadinata, R., Vashi, A. V., Peng, Y. Y., et al. (2017). Photo-modulated therapeutic protein release from a hydrogel depot using visible light. Angew. Chem, Int. Ed. 56, 966–971. doi: 10.1002/anie.201610618
Beatty, S., Koh, H., Phil, M., Henson, D., and Boulton, M. (2000). The role of oxidative stress in the pathogenesis of age-related macular degeneration. Survey Ophthalmol. 45, 115–134. doi: 10.1016/s0039-6257(00)00140-5
Benjamin, M., Garcia, A., Tully, J., Owens, G., Robeson, R., Navratil, T., et al. (2014). Development of precisely-engineered biodegradable drug delivery systems for posterior ocular drug delivery: PRINT® PLGA extended release implants for anti-VEGF biologics. Invest. Ophthalmol. Vis. Sci. 55:5269.
Bernards, D. A., Lance, K. D., Ciaccio, N. A., and Desai, T. A. (2012). Nanostructured thin film polymer devices for constant-rate protein delivery NIH public access. Nano Lett. 12, 5355–5361. doi: 10.1021/nl302747y
Bezatis, A., Spital, G., Höhn, F., Maier, M., Clemens, C. R., Wachtlin, J., et al. (2013). Functional and anatomical results after a single intravitreal Ozurdex injection in retinal vein occlusion: a 6-month follow-up – The SOLO study. Acta Ophthalmol. 91, e340–e347. doi: 10.1111/AOS.12020
Bhatt, P., Narvekar, P., Lalani, R., Chougule, M. B., Pathak, Y., and Sutariya, V. (2019). An in vitro assessment of thermo-reversible gel formulation containing sunitinib nanoparticles for neovascular age-related macular degeneration. AAPS PharmSciTech 20:281. doi: 10.1208/s12249-019-1474-0
Bhavsar, A. R., Googe, J. M., Stockdale, C. R., Bressler, N. M., Brucker, A. J., Elman, M. J., et al. (2009). Risk of endophthalmitis after intravitreal drug injection when topical antibiotics are not required: the diabetic retinopathy clinical research network laser-ranibizumab-triamcinolone clinical trials. Arch. Ophthalmol. 127, 1581–1583. doi: 10.1001/archophthalmol.2009.304
Blumenkranz, M. S., Chan, L.-S., and Leung, M. S. (2011). Critical appraisal of the clinical utility of the dexamethasone intravitreal implant (Ozurdex®) for the treatment of macular edema related to branch retinal vein occlusion or central retinal vein occlusion. Clin. Ophthalmol. 5:1043. doi: 10.2147/OPTH.S13775
Bourges, J. L., Bloquel, C., Thomas, A., Froussart, F., Bochot, A., Azan, F., et al. (2006). Intraocular implants for extended drug delivery: therapeutic applications. Adv. Drug Deliv. Rev. 58, 1182–1202. doi: 10.1016/j.addr.2006.07.026
Boyer, D. S., Faber, D., Gupta, S., Patel, S. S., Tabandeh, H., Li, X.-Y., et al. (2011). Dexamethasone intravitreal implant for treatment of diabetic macular edema in vitrectomized patients. Retina 31, 915–923. doi: 10.1097/IAE.0b013e318206d18c
Bressler, N. M., Arnold, J., Benchaboune, M., Blumenkranz, M. S., Fish, G. E., Gragoudas, E. S., et al. (2002). Verteporfin therapy of subfoveal choroidal neovascularization in patients with age-related macular degeneration additional information regarding baseline lesion composition’s impact on vision outcomes-TAP Report No. 3 treatment of age-related macular degeneration with photodynamic therapy (TAP) study group∗. Arch. Oftalmol. 120, 1443–1454.
Bringmann, A., Iandiev, I., Pannicke, T., Wurm, A., Hollborn, M., Wiedemann, P., et al. (2009). Cellular signaling and factors involved in Müller cell gliosis: neuroprotective and detrimental effects. Prog. Retin. Eye Res. 28, 423–451. doi: 10.1016/j.preteyeres.2009.07.001
Burke, J., and Schwartz, M. (1996). Preclinical evaluation of brimonidine. Survey Ophthalmol. 41(Suppl. 1), S9–S18.
Callanan, D. G., Jaffe, G. J., Martin, D. F., Pearson, P. A., and Comstock, T. L. (2008). Treatment of posterior uveitis with a fluocinolone acetonide implant three-year clinical trial results. Arch Ophthalmol. 126, 1191–1201.
Campbell, M., and Humphries, P. (2013). The blood-retina barrier tight junctions and barrier modulation. Adv. Exp. Med. Biol. 763, 70–84. doi: 10.1007/978-1-4614-4711-5_3
Campochiaro, P. A., Brown, D. M., Pearson, A., Chen, S., Boyer, D., Ruiz-Moreno, J., et al. (2012). Sustained delivery fluocinolone acetonide vitreous inserts provide benefit for at least 3 years in patients with diabetic macular Edema. Ophthalmology 119, 2125–2132. doi: 10.1016/j.ophtha.2012.04.030
Campochiaro, P. A., Brown, D. M., Pearson, A., Ciulla, T., Boyer, D., Holz, F. G., et al. (2011). Long-term benefit of sustained-delivery fluocinolone acetonide vitreous inserts for diabetic macular edema. Ophthalmology 118, 626.e2–635.e2. doi: 10.1016/j.ophtha.2010.12.028
Campochiaro, P. A., Marcus, D. M., Awh, C. C., Regillo, C., Adamis, A. P., Bantseev, V., et al. (2019). The port delivery system with ranibizumab for neovascular age-related macular degeneration: results from the randomized phase 2 ladder clinical trial. Ophthalmology 126, 1141–1154. doi: 10.1016/j.ophtha.2019.03.036
Casado, J. M. (2009). Origin of the diseases suffered by the aged, today: behavior, life style, diet, greater life expectancy. Servir 57, 46–56.
Chang, D., Park, K., and Famili, A. (2019). Hydrogels for sustained delivery of biologics to the back of the eye. Drug Discov. Today 24, 1470–1482. doi: 10.1016/j.drudis.2019.05.037
Cherry, J. D., Olschowka, J. A., and O’Banion, M. (2014). Neuroinflammation and M2 microglia: the good, the bad, and the inflamed. J. Neuroinflamm. 11:98. doi: 10.1186/1742-2094-11-98
ClinicalTrials.gov (2020a). 20089 TA+Lucentis Combo Intravitreal Injections for Treatment of Neovascular Age-related Macular Degeneration (AMD) - Full Text View. Available online at: https://clinicaltrials.gov/ct2/show/NCT01175395?term=IBI-20089&draw=2&rank=1 (accessed August 6, 2020).
ClinicalTrials.gov (2020b). A Phase III Study to Evaluate the Port Delivery System With Ranibizumab Compared With Monthly Ranibizumab Injections in Participants With Wet Age-Related Macular Degeneration - Full Text View. Available online at: https://clinicaltrials.gov/ct2/show/NCT03677934 (accessed August 6, 2020).
ClinicalTrials.gov (2020c). A Safety, and Efficacy Study of Brimonidine Intravitreal Implant in Geographic Atrophy Secondary to Age-related Macular Degeneration - Full Text View. Available online at: https://clinicaltrials.gov/ct2/show/NCT02087085?term=Brimonidine+Implant&draw=2&rank=1 (accessed August 6, 2020).
ClinicalTrials.gov (2020d). Combined Ranibizumab, and Iluvien for Diabetic Macular Oedema - Full Text View. Available online at: https://clinicaltrials.gov/ct2/show/NCT03784443 (accessed August 6, 2020).
ClinicalTrials.gov (2020e). Extension Study for the Port Delivery System With Ranibizumab(Portal) - Full Text View. Available online at: https://clinicaltrials.gov/ct2/show/NCT03683251 (Accessed August 6, 2020).
ClinicalTrials.gov (2020f). Fluocinolone Acetonide in Diabetic Macular Edema (FAME) Extension Study - Full Text View. Available online at: https://clinicaltrials.gov/ct2/show/NCT01304706 (accessed August 6, 2020).
Crouch, R. K., Koutalos, Y., Kono, M., Schey, K., and Ablonczy, Z. (2015). Chapter twenty-six - A2E and lipofuscin. Prog. Mol. Biol. Transl. Sci. 134, 449–463. doi: 10.1016/bs.pmbts.2015.06.005
Cuenca, N., Fernández-Sánchez, L., Campello, L., Maneu, V., De la Villa, P., Lax, P., et al. (2014). Cellular responses following retinal injuries and therapeutic approaches for neurodegenerative diseases. Prog. Retin. Eye Res. 43, 17–75. doi: 10.1016/j.preteyeres.2014.07.001
Cunha-Vaz, J., Bernardes, R., and Lobo, C. (2011). Blood-retinal barrier. Eur. J. Ophthalmol. 21, 3–9. doi: 10.5301/EJO.2010.6049
Damián, J., Pastor, R., Armadá, F., and Arias, L. (2006). Epidemiología de la degeneración macular asociada con la edad. Situación en España. Aten. Primaria 38, 51–57. doi: 10.1157/13090016
Daneault, V., Dumont, M., Massé, É, Vandewalle, G., and Carrier, J. (2016). Light-sensitive brain pathways and aging. J. Physiol. Anthropol. 35:9. doi: 10.1186/s40101-016-0091-9
Davis, B. M., Normando, E. M., Guo, L., Turner, L. A., Nizari, S., O’Shea, P., et al. (2014). Topical delivery of avastin to the posterior segment of the eye in vivo using annexin A5-associated liposomes. Small 10, 1575–1584. doi: 10.1002/smll.201303433
Deb, P. K., Al-Attraqchi, O., Jaber, A. Y., Amarji, B., and Tekade, R. K. (2018). “Physicochemical aspects to be considered in pharmaceutical product development,” in Dosage Form Design Considerations, Vol. I, ed. R. K. Tekade (Amsterdam: Elsevier), 57–83. doi: 10.1016/B978-0-12-814423-7.00002-2
Degeneration, A. M., Jonas, J. B., Ming, C., and Cheung, G. (2017). Updates on the epidemiology of age-related macular degeneration. Asia Pacific J. Ophthalmol. 6, 493–497. doi: 10.22608/apo.2017251
del Amo, E. M., and Urtti, A. (2008). Current and future ophthalmic drug delivery systems: a shift to the posterior segment. Drug Discov. Today 13, 135–143. doi: 10.1016/J.DRUDIS.2007.11.002
Desai, T., and Bernards, D. A. (2014). Zordera. Available online at: www.ondrugdelivery.com (accessed March 19, 2019).
Drapala, P. W., Jiang, B., Chiu, Y. C., Mieler, W. F., Brey, E. M., Kang-Mieler, J. J., et al. (2014). The effect of glutathione as chain transfer agent in PNIPAAm-based thermo-responsive hydrogels for controlled release of proteins. Pharm. Res. 31, 742–753. doi: 10.1007/s11095-013-1195-0
Ebrahim, S., Peyman, G. A., and Lee, P. J. (2005). Applications of liposomes in ophthalmology. Surv. Ophthalmol. 50, 167–182. doi: 10.1016/j.survophthal.2004.12.006
Edington, M., Connolly, J., and Chong, N. V. (2017). Pharmacokinetics of intravitreal anti-VEGF drugs in vitrectomized versus non-vitrectomized eyes. Expert Opin. Drug Metab. Toxicol. 13, 1217–1224. doi: 10.1080/17425255.2017.1404987
Evans, J. R., Fletcher, A. E., and Wormald, R. P. (2004). Causes of visual impairment in people aged 75 years and older in Britain: an add-on study to the MRC Trial of Assessment and Management of Older People in the Community. Br. J. Ophthalmol. 88, 365–370.
Eyewire News (2020). Ocular Therapeutix Announces Dosing of First Patient in Phase 1 Clinical Trial for the Treatment of Wet AMD. Available online at: https://eyewire.news/articles/ocular-therapeutix-announces-dosing-of-first-patient-in-phase-1-clinical-trial-for-the-treatment-of-wet-amd/ (accessed June 28, 2020).
Falavarjani, K. G., and Nguyen, Q. D. (2013). Adverse events and complications associated with intravitreal injection of anti-VEGF agents: a review of literature. Eye 27, 787–794. doi: 10.1038/eye.2013.107
Fenton, C., and Perry, C. M. (2006). Verteporfin: a review of its use in the management of subfoveal choroidal neovascularisation. Drugs Aging 23, 421–445. doi: 10.2165/00002512-200623050-00006
Ferris, F. L., Davis, M. D., Clemons, T. E., Lee, L. Y., Chew, E. Y., Lindblad, A. S., et al. (2005). A simplified severity scare for age-related macular degeneration: AREDS Report no.18. Arch Ophthalmol. 123, 1570–1574.
Fleckenstein, M., Mitchell, P., Freund, K. B., Sadda, S., Holz, F. G., Brittain, C., et al. (2018). The progression of geographic atrophy secondary to age-related macular degeneration. Ophthalmology 125, 369–390. doi: 10.1016/j.ophtha.2017.08.038
Fomina, N., McFearin, C., Sermsakdi, M., Edigin, O., and Almutairi, A. (2010). UV and near-IR triggered release from polymeric nanoparticles. J. the Am. Chem. Soc. 132, 9540–9542. doi: 10.1021/ja102595j
Freund, K. B., Mrejen, S., and Gallego-Pinazo, R. (2013). An update on the pharmacotherapy of neovascular age-related macular degeneration. Expert Opin. Pharm. 14, 1017–1028. doi: 10.1517/14656566.2013.787410
Friedman, D., O’Colmain, B., Tomany, S., McCarty, C., de Jong, P., Nemesure, B., et al. (2004). Prevalence of age-related macular degeneration in the US population. Arch. Ophthalmol. 122, 564–572.
Friedman, D. S., O’Colmain, B., and Mestril, I. (2012). Vision Problems in the U.S, 5th Edn. Washington DC: Prevent Blindness America.
Fuchs, H., and Igney, F. (2017). Binding to ocular albumin as a half-life extension principle for intravitreally injected drugs: evidence from mechanistic rat and rabbit studies. J. Ocul. Pharmacol. Ther. 33, 115–122. doi: 10.1089/jop.2016.0083
Galante, R., Pinto, T. J. A., Colaço, R., and Serro, A. P. (2018). Sterilization of hydrogels for biomedical applications: a review. J. Biomed. Mater. Res. Part B Appl. Biomater. 106, 2472–2492. doi: 10.1002/jbm.b.34048
Gao, H., Qiao, X., Cantor, L. B., and WuDunn, D. (2002). Up-regulation of brain-derived neurotrophic factor expression by brimonidine in rat retinal ganglion cells. Arch. Ophthalmol. 120, 797–803.
Garciìa, M. A., and Martinez, S. E. (2013). Degeneracioìn macular asociada a la edad (DMAE). Guiìa cliìnica optomeìtrica, a partir de estudios basados en la evidencia. Gaceta de Optometriìa y Oìptica Oftaìlmica 487, 24–30.
Gaudana, R., Jwala, J., Boddu, S. H. S., and Mitra, A. K. (2009). Recent perspectives in ocular drug delivery. Pharm. Res. 26, 1197–1216. doi: 10.1007/s11095-008-9694-0
Ghasemi Falavarjani, K. (2009). Implantable posterior segment drug delivery devices; novel alternatives to currently available treatments. J. Ophthalmic Vis. Res. 4, 191–193.
Grossniklaus, H. E., Ling, J. X., Wallace, T. M., Dithmar, S., Lawson, D. H., Cohen, C., et al. (2002). Macrophage and retinal pigment epithelium expression of angiogenic cytokines in choroidal neovascularization. Mol. Vis. 8, 119–126.
Gutiérrez-Hernández, J.-C., Caffey, S., Abdallah, W., Calvillo, P., González, R., Shih, J., et al. (2014). One-year feasibility study of replenish micropump for intravitreal drug delivery: a pilot Study. Transl. Vis. Sci. Technol. 3:8. doi: 10.1167/tvst.3.4.1
Haghjou, N., Soheilian, M., and Abdekhodaie, M. J. (2011). Sustained release intraocular drug delivery devices for treatment of uveitis. J. Ophthalmic Vis. Res. 6, 317–319.
Haller, J. A., Bandello, F., Belfort, R., Blumenkranz, M. S., Gillies, M., Heier, J., et al. (2010). Randomized, sham-controlled trial of dexamethasone intravitreal implant in patients with macular edema due to retinal vein occlusion. Ophthalmology 117, 1134.e3–1146.e3. doi: 10.1016/j.ophtha.2010.03.032
Hammer, N., Brandl, F. P., Kirchhof, S., Messmann, V., and Goepferich, A. M. (2015). Protein compatibility of selected cross-linking reactions for hydrogels. Macromol. Biosci. 15, 405–413. doi: 10.1002/mabi.201400379
Handa, J. T., Bowes Rickman, C., Dick, A. D., Gorin, M. B., Miller, J. W., Toth, C. A., et al. (2019). A systems biology approach towards understanding and treating non-neovascular age-related macular degeneration. Nat. Commun. 10:3347. doi: 10.1038/s41467-019-11262-1
Hans, M. L., and Lowman, A. M. (2002). Biodegradable nanoparticles for drug delivery and targeting. Curr. Opin. Solid State Mater. Sci. 6, 319–327. doi: 10.1016/S1359-0286(02)00117-1
Hanus, J., Anderson, C., and Wang, S. (2015). RPE necroptosis in response to oxidative stress and in AMD. Ageing Res. Rev. 24, 286–298. doi: 10.1016/j.arr.2015.09.002
Herrero-Vanrell, R., Bravo-Osuna, I., Andrés-Guerrero, V., Vicario-de-la-Torre, M., and Molina-Martínez, I. T. (2014). The potential of using biodegradable microspheres in retinal diseases and other intraocular pathologies. Prog. Retin. Eye Res. 42, 27–43. doi: 10.1016/j.preteyeres.2014.04.002
Herrero-Vanrell, R., and Refojo, M. F. (2001). Biodegradable microspheres for vitreoretinal drug delivery. Adv. Drug Deliv. Rev. 52, 5–16. doi: 10.1016/S0169-409X(01)00200-9
Honda, M., Asai, T., Oku, N., Araki, Y., Tanaka, M., and Ebihara, N. (2013). Liposomes and nanotechnology in drug development: focus on ocular targets. Int. J. Nanomed. 8, 495–504. doi: 10.2147/IJN.S30725
Hora, M. S., Rana, R. K., Nunberg, J. H., Tice, T. R., Gilley, R. M., and Hudson, M. E. (1990). Release of human serum albumin from poly(lactide-co-glycolide) microspheres. Pharm. Res. 7, 1190–1194.
Hu, C. C., Chiu, Y. C., Chaw, J. R., Chen, C. F., and Liu, H. W. (2019). Thermo-responsive hydrogel as an anti-VEGF drug delivery system to inhibit retinal angiogenesis in Rex rabbits. Technol Health Care 27, 153–163. doi: 10.3233/THC-199015
Humayun, M., Santos, A., Altamirano, J. C., Ribeiro, R., Gonzalez, R., de la Rosa, A., et al. (2014). Implantable micropump for drug delivery in patients with diabetic macular edema. Transl. Vis. Sci. Technol. 3:5. doi: 10.1167/tvst.3.6.5
Huu, V. A. N., Luo, J., Zhu, J., Zhu, J., Patel, S., Boone, A., et al. (2015). Light-responsive nanoparticle depot to control release of a small molecule angiogenesis inhibitor in the posterior segment of the eye. J. Control. Release? 200, 71–77. doi: 10.1016/j.jconrel.2015.01.001
IAPB Vision Atlas (2017∗). Global Vision Database Maps. Global Vision Database Maps. London: IAPB Vision Atlas.
Imperiale, J. C., Acosta, G. B., and Sosnik, A. (2018). Polymer-based carriers for ophthalmic drug delivery. J. Control. Release 285, 106–141. doi: 10.1016/j.jconrel.2018.06.031
Jackson, T. L., Boyer, D., Brown, D. M., Chaudhry, N., Elman, M., Liang, C., et al. (2017). Oral tyrosine kinase inhibitor for neovascular age-related macular degeneration: a phase 1 dose-escalation study. JAMA Ophthalmol. 135, 761–767. doi: 10.1001/jamaophthalmol.2017.1571
Jaffe, G. J., Yang, C. H., Guo, H., Denny, J. P., Lima, C., and Ashton, P. (2000). Safety and pharmacokinetics of an intraocular fluocinolone acetonide sustained delivery device. Inves. Ophthalmol. Vis. Sci. 41, 3569–3575.
Jager, R. D., Aiello, L. P., Patel, S. C., and Cunningham, E. T. (2004). Risks of intravitreous injection: a comprehensive review. Retina 24, 676–698. doi: 10.1097/00006982-200410000-00002
Jager, R. D., Mieler, W. F., and Miller, J. W. (2008). Age-related macular degeneration. N. Engl. J. Med. 358, 2606–2617. doi: 10.1056/NEJMra0801537
Jarrett, S. G., and Boulton, M. E. (2012). Consequences of oxidative stress in age-related macular degeneration. Mol. Aspects Med. 33, 399–417. doi: 10.1016/j.mam.2012.03.009
Jarrett, T., Elhayek, R. F., Lattrell, Z., McGrath, M., Takach, S., Jarrett, P. K., et al. (2017). Pharmacokinetics of a 6 month sustained hydrogel delivery system for tyrosine kinase inhibitors in dutch belted rabbits. Invest. Ophthalmol. Vis. Sci. 58:1984.
Jeffries, A. M., Killian, N. J., and Pezaris, J. S. (2014). Mapping the primate lateral geniculate nucleus: a review of experiments and methods. J. Physiol. Paris 108, 3–10. doi: 10.1016/j.jphysparis.2013.10.001
Jiang, S., Wang, K., Dai, Y., Zhang, X., and Xia, F. (2019). Near-infrared light-triggered dual drug release using gold nanorod-embedded thermosensitive nanogel-crosslinked hydrogels. Macromol. Mater. Eng. 304:1900087. doi: 10.1002/mame.201900087
Journal, A. I., Anwary, M., Kumar, P., Toit, L. C., and Choonara, Y. E. (2018). Polymeric, injectable, intravitreal hydrogel devices for posterior segment applications and interventions. Artif. Cells Nanomed. Biotechnol. 46, 1074–1081. doi: 10.1080/21691401.2018.1478845
Kabiesz, A., and Nowak, M. (2015). Age related macular degeneration – challenge for future?: pathogenesis and new perspectives for the treatment. Eur. Geriatr. Med. 6, 69–75. doi: 10.1016/j.eurger.2014.09.007
Kandatsu, N., Nan, Y.-S., Feng, G.-G., Nishiwaki, K., Hirokawa, M., Ishikawa, K., et al. (2005). Opposing effects of isoflurane and sevoflurane on neurogenic pulmonary edema development in an animal model. Anesthesiology 102, 1182–1189. doi: 10.1097/00000542-200506000-00018
Kane, F. E., Burdan, J., Cutino, A., and Green, K. E. (2008). Iluvien TM?: a new sustained delivery technology for posterior eye disease. Expert Opin. Drug Deliv. 5, 1039–1046. doi: 10.1517/17425247.5.9.1039
Kang Derwent, J. J., and Mieler, W. F. (2008). Thermoresponsive hydrogels as a new ocular drug delivery platform to the posterior segment of the eye. Trans. Am. Ophthalmol. Soc. 106, 206–213. discussion 213-4
Kang-Mieler, J. J., Dosmar, E., Liu, W., and Mieler, W. F. (2017). Extended ocular drug delivery systems for the anterior and posterior segments: biomaterial options and applications. Expert Opin. Drug Deliv. 14, 611–620. doi: 10.1080/17425247.2016.1227785
Kang-Mieler, J. J., Osswald, C. R., and Mieler, W. F. (2014). Advances in ocular drug delivery: emphasis on the posterior segment. Expert Opin. Drug Deliv. 11, 1647–1660. doi: 10.1517/17425247.2014.935338
Kang-Mieler, J. J., Rudeen, K. M., Liu, W., and Mieler, W. F. (2020). Advances in ocular drug delivery systems. Eye 34, 1371–1379. doi: 10.1038/s41433-020-0809-0
Kanjickal, D., Lopina, S., Evancho-Chapman, M. M., Schmidt, S., and Donovan, D. (2008). Effects of sterilization on poly(ethylene glycol) hydrogels. J. Biomed. Mater. Res. Part A 87, 608–617. doi: 10.1002/jbm.a.31811
Karajanagi, S. S., Yoganathan, R., Mammucari, R., Park, H., Cox, J., Zeitels, S. M., et al. (2011). Application of a dense gas technique for sterilizing soft biomaterials. Biotechnol. Bioeng. 108, 1716–1725. doi: 10.1002/bit.23105
Khan, J. C., Thurlby, D. A., Shahid, H., Clayton, D. G., Yates, J. R. W., Bradley, M., et al. (2006). Smoking and age related macular degeneration: the number of pack years of cigarette smoking is a major determinant of risk for both geographic atrophy and choroidal neovascularisation. Br. J. Ophthalmol. 90, 75–80. doi: 10.1136/bjo.2005.073643
Kim, C. E., Lee, J. H., Yeon, Y. K., Park, C. H., and Yang, J. (2017). Effects of silk fibroin in murine dry eye. Nat. Publ. Group 7:44364. doi: 10.1038/srep44364
Kinnunen, K., Petrovski, G., Moe, M. C., and Kaarniranta, K. (2012). Molecular mechanisms of retinal pigment epithelium damage and development of age-related macular degeneration. Acta Ophthalmol. 90, 299–309. doi: 10.1111/j.1755-3768.2011.02179.x
Kirchhof, S., Goepferich, A. M., and Brandl, F. P. (2015a). Hydrogels in ophthalmic applications. Eur. J. Pharm. Biopharm. 95, 227–238. doi: 10.1016/j.ejpb.2015.05.016
Kirchhof, S., Gregoritza, M., Messmann, V., Hammer, N., Goepferich, A. M., and Brandl, F. P. (2015b). Diels–Alder hydrogels with enhanced stability: first step toward controlled release of bevacizumab. Eur. J. Pharm. Biopharm. 96, 217–225. doi: 10.1016/J.EJPB.2015.07.024
Klein, R., Chou, C.-F., Klein, B. E. K., Zhang, X., Meuer, S. M., and Saaddine, J. B. (2011). Prevalence of age-related macular degeneration in the us population. Arch. Ophthalmol. 129, 75–80. doi: 10.1001/archophthalmol.2010.318
Klein, R. J., Zeiss, C., Chew, E. Y., Tsai, J.-Y., Sackler, R. S., Haynes, C., et al. (2005). Complement factor H polymorphism in age-related macular degeneration. Science 308, 385–389. doi: 10.1126/science.1109557
Koerselman, M., Warmink, K., Korthagen, N. M., van Midwoud, P., Verrips, T., Weinans, H. H., et al. (2020). Intra-articular drug depots for controlled release of heavy chain only antibodies blocking joint inflammation. Osteoarthr. Cartil. 28:S37. doi: 10.1016/j.joca.2020.02.061
Kuno, N., and Fujii, S. (2010). Biodegradable intraocular therapies for retinal disorders. Drugs Aging 27, 117–134. doi: 10.2165/11530970-000000000-00000
Kuppermann, B. D., Blumenkranz, M. S., Haller, J. A., Williams, G. A., Weinberg, D. V., Chou, C., et al. (2007). Randomized controlled study of an intravitreous dexamethasone drug delivery system in patients with persistent macular edema. Arch. Ophthalmol. 125, 309–317. doi: 10.1001/archopht.125.3.309
Lacour, M., Kiilgaard, J. F., and Nissen, M. H. (2002). Age-related macular degeneration. Drugs Aging 19, 101–133. doi: 10.2165/00002512-200219020-00003
Lai, J.-Y. (2013). Influence of solvent composition on the performance of carbodiimide cross-linked gelatin carriers for retinal sheet delivery. J. Mater. Sci. 24, 2201–2210. doi: 10.1007/s10856-013-4961-y
Lajavardi, L., Bochot, A., Camelo, S., Goldenberg, B., Naud, M. C., Behar-Cohen, F., et al. (2007). Downregulation of endotoxin-induced uveitis by intravitreal injection of vasoactive intestinal peptide encapsulated in liposomes. Invest. Ophthalmol. Vis. Sci. 48, 3230–3238. doi: 10.1167/iovs.06-1305
Lance, K. D., Bernards, D. A., Ciaccio, N. A., Good, S. D., Mendes, T. S., Kudisch, M., et al. (2016). In vivo and in vitro sustained release of ranibizumab from a nanoporous thin-film device. Drug Deliv. Transl. Res. 6, 771–780. doi: 10.1007/s13346-016-0298-7
Lance, K. D., Good, S. D., Mendes, T. S., Ishikiriyama, M., Chew, P., Estes, L. S., et al. (2015). In vitro and in vivo sustained zero-order delivery of rapamycin (Sirolimus) from a biodegradable intraocular device. Invest. Opthalmol. Vis. Sci. 56:7331. doi: 10.1167/iovs.15-17757
Li, F. (2012). Controlled release of bevacizumab through nanospheres for extended treatment of age-related macular degeneration. Open Ophthalmol. J. 6, 54–58. doi: 10.2174/1874364101206010054
Li, J., and Mooney, D. J. (2016). Designing hydrogels for controlled drug delivery. Nat. Rev. Mater. 1:16071. doi: 10.1038/natrevmats.2016.71
Lim, J. H., Wickremasinghe, S. S., Xie, J., Chauhan, D. S., Baird, P. N., Robman, L. D., et al. (2012). Delay to treatment and visual outcomes in patients treated with anti-vascular endothelial growth factor for age-related macular degeneration. Am. J. Ophthalmol. 153, 678–686.
Lim, J. I., Niec, M., and Wong, V. (2015). One year results of a phase 1 study of the safety and tolerability of combination therapy using sustained release intravitreal triamcinolone acetonide and ranibizumab for subfoveal neovascular AMD. Br. J. Ophthalmol. 99, 618–623. doi: 10.1136/bjophthalmol-2014-306002
Lim, J. I., Wolitz, R. A., Dowling, A. H., Bloom, H. R., Irvine, A. R., and Schwartz, D. M. (1999). Visual and anatomic outcomes associated with posterior segment complications after ganciclovir implant procedures in patients with AIDS and cytomegalovirus retinitis. Am. J. Ophthalmol. 127, 288–293.
Lin, M. T., and Beal, M. F. (2006). Mitochondrial dysfunction and oxidative stress in neurodegenerative diseases. Nature 443, 787–795. doi: 10.1038/nature05292
Linsley, C. S., and Wu, B. M. (2017). Recent advances in light-responsive on-demand drug-delivery systems. Ther. Deliv. 8, 89–107. doi: 10.4155/tde-2016-0060
Liu, J., Li, S., Li, G., Li, X., Yu, C., Fu, Z., et al. (2019a). Highly bioactive, bevacizumab-loaded, sustained-release PLGA/PCADK microspheres for intravitreal therapy in ocular diseases. Int. J. Pharm. 563, 228–236. doi: 10.1016/j.ijpharm.2019.04.012
Liu, W., Borrell, M. A., Venerus, D. C., Mieler, W. F., and Kang-Mieler, J. J. (2019b). Characterization of biodegradable microsphere-hydrogel ocular drug delivery system for controlled and extended release of ranibizumab. Transl. Vis. Sci. Technol. 8:12. doi: 10.1167/tvst.8.1.12
Liu, W., Lee, B. S., Mieler, W. F., and Kang-Mieler, J. J. (2019c). Biodegradable microsphere-hydrogel ocular drug delivery system for controlled and extended release of bioactive aflibercept in vitro. Curr. Eye Res. 44, 264–274. doi: 10.1080/02713683.2018.1533983
Lo, R., Li, P.-Y., Saati, S., Agrawal, R. N., Humayun, M. S., and Meng, E. (2009). A passive MEMS drug delivery pump for treatment of ocular diseases. Biomed. Microdev. 11, 959–970. doi: 10.1007/s10544-009-9313-9
Lovett, M. L., Wang, X., Yucel, T., York, L., Keirstead, M., Haggerty, L., et al. (2015). Silk hydrogels for sustained ocular delivery of anti-vascular endothelial growth factor (anti-VEGF) therapeutics. Eur. J. Pharm. Biopharm. 95, 271–278. doi: 10.1016/J.EJPB.2014.12.029
Machinaga, N., Ashley, G. W., Reid, R., Yamasaki, A., Tanaka, K., Nakamura, K., et al. (2018). A controlled release system for long-acting intravitreal delivery of small molecules. Transl. Vis. Sci. Technol. 7:21. doi: 10.1167/tvst.7.4.21
Mainardes, R. M., Urban, M. C. C., Cinto, P. O., Khalil, N. M., Chaud, M. V., Evangelista, R. C., et al. (2005). Colloidal carriers for ophthalmic drug delivery. Curr. Drug Targ. 6, 363–371.
Mandal, A., Bisht, R., Rupenthal, I. D., and Mitra, A. K. (2017). Polymeric micelles for ocular drug delivery?: from structural frameworks to recent preclinical studies. J. Control. Release 248, 96–116. doi: 10.1016/j.jconrel.2017.01.012
Mandal, A., Pal, D., Agrahari, V., Trinh, H. M., Joseph, M., and Mitra, A. K. (2018). Ocular delivery of proteins and peptides: challenges and novel formulation approaches. Adv. Drug Deliv. Rev. 126, 67–95. doi: 10.1016/j.addr.2018.01.008
Manoharan, C., and Singh, J. (2009). Insulin loaded PLGA microspheres: effect of zinc salts on encapsulation, release, and stability. J. Pharm. Sci. 98, 529–542. doi: 10.1002/jps.21445
Marín-Teva, J. L., Cuadros, M. A., Martín-Oliva, D., and Navascués, J. (2011). Microglia and neuronal cell death. Neuron Glia Biol. 7, 25–40. doi: 10.1017/S1740925X12000014
Martin, D. F., Maguire, M. G., Fine, S. L., Ying, G., Jaffe, G. J., Grunwald, J. E., et al. (2012). Ranibizumab and bevacizumab for treatment of neovascular age-related macular degeneration. Ophthalmology 119, 1388–1398. doi: 10.1016/j.ophtha.2012.03.053
Mason, J. O., White, M. F., Feist, R. M., Thomley, M. L., Albert, M. A., Persaud, T. O., et al. (2008). Incidence of acute onset endophthalmitis following intravitreal bevacizumab (avastin) injection. Retina 28, 564–567. doi: 10.1097/IAE.0b013e3181633fee
Matanović, M. R., Kristl, J., and Grabnar, P. A. (2014). Thermoresponsive polymers: insights into decisive hydrogel characteristics, mechanisms of gelation, and promising biomedical applications. Int. J. Pharm. 472, 262–275. doi: 10.1016/j.ijpharm.2014.06.029
McConnell, V., and Silvestri, G. (2005). Age-related macular degeneration. Ulster Med. J. 74, 82–92.
McLaughlin, M. M., Paglione, M. G., Slakter, J., Tolentino, M., Ye, L., Xu, C. F., et al. (2013). Initial exploration of oral pazopanib in healthy participants and patients with age-related macular degeneration. JAMA Ophthalmol. 131, 1595–1601. doi: 10.1001/jamaophthalmol.2013.5002
Medina, C., Santos-Martinez, M. J., Radomski, A., Corrigan, O. I., and Radomski, M. W. (2009). Nanoparticles: pharmacological and toxicological significance. Br. J. Pharmacol. 150, 552–558. doi: 10.1038/sj.bjp.0707130
Mi, F.-L., Lin, Y.-M., Wu, Y.-B., Shyu, S.-S., and Tsai, Y.-H. (2002). Chitin/PLGA blend microspheres as a biodegradable drug-delivery system: phase-separation, degradation and release behavior. Biomaterials 23, 3257–3267.
Michalska-Małecka, K., Kabiesz, A., Nowak, M., and Śpiewak, D. (2015). Age related macular degeneration – challenge for future: pathogenesis and new perspectives for the treatment. Eur. Geriatr. Med. 6, 69–75. doi: 10.1016/J.EURGER.2014.09.007
Moghimi, S. M., Patel, H. M., and Patel, H. M. (1989). Serum opsonins and phagocytosis of saturated and unsaturated phospholipid liposomes. Biochim. Biophys. Acta 984, 384–387.
Morirero, T., Ogura, Y., Hondo, Y., Suong-Hyu Hyoaf, R. W., and Ikadaf, Y. (1991). Microspheres of Biodegradable Polymers as a Drug-Delivery System in the Vitreous. Invest. Ophthalmol. Vis. Sci. 32, 1785–1790.
Mu, H., Wang, Y., Chu, Y., Jiang, Y., Hua, H., Chu, L., et al. (2018). Multivesicular liposomes for sustained release of bevacizumab in treating laser-induced choroidal neovascularization. Drug Deliv. 25, 1372–1383. doi: 10.1080/10717544.2018.1474967
Narvekar, P., Bhatt, P., Fnu, G., and Sutariya, V. (2019). Axitinib-loaded Poly(Lactic-Co-Glycolic Acid) nanoparticles for age-related macular degeneration: formulation development and in vitro characterization. Assay Drug Dev. Technol. 17, 167–177. doi: 10.1089/adt.2019.920
Nash, B., Ioannidou, K., and Barnett, S. C. (2011). Astrocyte phenotypes and their relationship to myelination. J. Anat. 219, 44–52. doi: 10.1111/j.1469-7580.2010.01330.x
Navratil, T., Maynor, B., and Yerxa, B. (2014). Improving outcomes: inophthalmology via sustained drug delivery. ONdrugDelivery 48, 10–13.
Nayak, K., and Misra, M. (2018). Biomedicine & Pharmacotherapy A review on recent drug delivery systems for posterior segment of eye. Biomed. Pharmacother. 107, 1564–1582. doi: 10.1016/j.biopha.2018.08.138
Nowak, J. Z. (2006). Age-related macular degeneration (AMD): pathogenesis and therapy. Pharmacol. Rep. 58, 353–363.
Nozaki, M., Raisler, B. J., Sakurai, E., Sarma, J. V., Barnum, S. R., Lambris, J. D., et al. (2006). Drusen complement components C3a and C5a promote choroidal neovascularization. Proc. Natil. Acad. Sci. U.S.A. 103, 2328–2333. doi: 10.1073/pnas.0408835103
Nussenblatt, R. B., and Ferris, F. (2007). Age-related macular degeneration and the immune response: implications for therapy. Am. J. Ophthalmol. 144, 618–626. doi: 10.1016/j.ajo.2007.06.025
Oo, C., and Kalbag, S. S. (2016). Leveraging the attributes of biologics and small molecules, and releasing the bottlenecks: a new wave of revolution in drug development. Expert Rev. Clin. Pharmacol. 9, 747–749. doi: 10.1586/17512433.2016.1160778
Osswald, C. R., Guthrie, M. J., Avila, A., Valio, J. A., Mieler, W. F., and Kang-Mieler, J. J. (2017). In vivo efficacy of an injectable microsphere-hydrogel ocular drug delivery system. Curr. Eye Res. 42, 1293–1301. doi: 10.1080/02713683.2017.1302590
Osswald, C. R., and Kang-Mieler, J. J. (2015). Controlled and extended release of a model protein from a microsphere-hydrogel drug delivery system. Ann. Biomed. Eng. 43, 2609–2617. doi: 10.1007/s10439-015-1314-7
Osswald, C. R., and Kang-Mieler, J. J. (2016). Controlled and extended in vitro release of bioactive anti-vascular endothelial growth factors from a microsphere-hydrogel drug delivery system. Curr. Eye Res. 41, 1216–1222. doi: 10.3109/02713683.2015.1101140
OTX-IVT (2020). (anti-VEGF antibody implant) – Ocular Therapeutix. Available online at: https://www.ocutx.com/research/otx-ivt/ (accessed June 28, 2020).
Pan, C. K., Durairaj, C., Kompella, U. B., Agwu, O., Oliver, S. C. N., Quiroz-Mercado, H., et al. (2011). Comparison of long-acting bevacizumab formulations in the treatment of choroidal neovascularization in a rat model. J. Ocul. Pharmacol. Ther. 27, 219–224. doi: 10.1089/jop.2010.0158
Pandit, J., Sultana, Y., and Aqil, M. (2017). Chitosan-coated PLGA nanoparticles of bevacizumab as novel drug delivery to target retina: optimization, characterization, and in vitro toxicity evaluation. Artif. Cells Nanomed. Biotechnol. 45, 1397–1407. doi: 10.1080/21691401.2016.1243545
Patel, A., Cholkar, K., Agrahari, V., and Mitra, A. K. (2013). Ocular drug delivery systems: an overview. World J. Pharmacol. 2, 47–64. doi: 10.5497/wjp.v2.i2.47
Pirmoradi, F. N., Jackson, J. K., Burt, H. M., and Chiao, M. (2011). On-demand controlled release of docetaxel from a battery-less MEMS drug delivery device. Lab Chip 11, 2744–2752. doi: 10.1039/c1lc20134d
Polazzi, E., and Monti, B. (2010). Microglia and neuroprotection: from in vitro studies to therapeutic applications. Prog. Neurobiol. 92, 293–315. doi: 10.1016/j.pneurobio.2010.06.009
Qin, X. (2017). “Coaxial electrospinning of nanofibers,” in Electrospun Nanofibers, ed. M. Afshari (Amsterdam: Elsevier Inc), 41–71. doi: 10.1016/B978-0-08-100907-9.00003-9
Qiu, F., Meng, T., Chen, Q., Zhou, K., Shao, Y., Matlock, G., et al. (2019). Fenofibrate-loaded biodegradable nanoparticles for the treatment of experimental diabetic retinopathy and neovascular age-related macular degeneration. Mol. Pharm. 16, 1958–1970. doi: 10.1021/acs.molpharmaceut.8b01319
Querques, L., Querques, G., Lattanzio, R., Gigante, S. R., Del Turco, C., Corradetti, G., et al. (2013). Repeated intravitreal dexamethasone implant (ozurdex®) for retinal vein occlusion. Ophthalmologica 229, 21–25. doi: 10.1159/000342160
Radhakrishnan, K., Sonali, N., Moreno, M., Nirmal, J., Fernandez, A. A., Venkatraman, S., et al. (2017). Protein delivery to the back of the eye: barriers, carriers and stability of anti-VEGF proteins. Drug Discov. Today 22, 416–423. doi: 10.1016/j.drudis.2016.10.015
Rakesh, T. (2019). “Advances in pharmaceutical product development and research,” in Biomaterials and Bionanotechnology, 1st Edn, ed. T. Rakesh (Amsterdam: Elsevier).
Rathnasamy, G., Foulds, W. S., Ling, E.-A., and Kaur, C. (2019). Retinal microglia – A key player in healthy and diseased retina. Prog. Neurobiol. 173, 18–40. doi: 10.1016/j.pneurobio.2018.05.006
Ratnapriya, R., and Chew, E. Y. (2013). Age-related macular degeneration-clinical review and genetics update. Clin. Genet. 84, 160–166. doi: 10.1111/cge.12206
Rauck, B. M., Friberg, T. R., Medina Mendez, C. A., Park, D., Shah, V., Bilonick, R. A., et al. (2013). Biocompatible reverse thermal gel sustains the release of intravitreal bevacizumab in vivo. Invest. Ophthalmol. Vis. Sci. 55, 469–470. doi: 10.1167/iovs.13-13120
Rivera, A., Fisher, S. A., Fritsche, L. G., Keilhauer, C. N., Lichtner, P., Meitinger, T., et al. (2005). Hypothetical LOC387715 is a second major susceptibility gene for age-related macular degeneration, contributing independently of complement factor H to disease risk. Hum. Mol. Genet. 14, 3227–3236. doi: 10.1093/hmg/ddi353
Rupenthal, I. D. (2017). Drug-device combination approaches for delivery to the eye. Curr. Opin. Pharmacol. 36, 44–51. doi: 10.1016/j.coph.2017.08.003
Sahoo, S. K., Dilnawaz, F., and Krishnakumar, S. (2008). Nanotechnology in ocular drug delivery. Drug Discov. Today 13, 144–151. doi: 10.1016/j.drudis.2007.10.021
Sakurai, E., Ozeki, H., Kunou, N., and Ogura, Y. (2001). Effect of particle size of polymeric nanospheres on intravitreal kinetics. Ophthalmic Res. 33, 31–36. doi: 10.1159/000055638
Santhanam, S., Liang, J., Struckhoff, J., Hamilton, P. D., and Ravi, N. (2016). Biomimetic hydrogel with tunable mechanical properties for vitreous substitutes. Acta Biomater. 43, 327–337. doi: 10.1016/j.actbio.2016.07.051
Saraf, A., Lozier, G., Haesslein, A., Kasper, F. K., Raphael, R. M., Baggett, L. S., et al. (2009). Fabrication of nonwoven coaxial fiber meshes by electrospinning. Tissue Eng. Part C15, 333–344. doi: 10.1089/ten.tec.2008.0422
Schlesinger, E., Ciaccio, N., and Desai, T. A. (2015). Polycaprolactone thin-film drug delivery systems: empirical and predictive models for device design. Mater. Sci. Eng. C 57, 232–239. doi: 10.1016/j.msec.2015.07.027
Schmit-Eilenberger, V. (2015). A novel intravitreal fluocinolone acetonide implant (Iluvien®) in the treatment of patients with chronic diabetic macular edema that is insufficiently responsive to other medical treatment options: a case series. Clin. Ophthalmol. 9, 801–811. doi: 10.2147/OPTH.S79785
Seah, I., Zhao, X., Lin, Q., Liu, Z., Su, S. Z. Z., Yuen, Y., et al. (2020). Use of biomaterials for sustained delivery of anti-VEGF to treat retinal diseases. Eye 34, 1341–1356. doi: 10.1038/s41433-020-0770-y
Sergejeva, O., Botov, R., Liutkevièienë, R., and Kriauèiûnienë, L. (2016). Genetic factors associated with the development of age-related macular degeneration. Medicina 52, 79–88. doi: 10.1016/j.medici.2016.02.004
Shah, S. S., Denham, L. V., Elison, J. R., Bhattacharjee, P. S., Clement, C., Huq, T., et al. (2010). Drug delivery to the posterior segment of the eye for pharmacologic therapy. Expert Rev. Ophthalmol. 5, 75–93. doi: 10.1586/eop.09.70
Sharpe, L. A., Daily, A. M., Horava, S. D., and Peppas, N. A. (2014). Therapeutic applications of hydrogels in oral drug delivery. Expert Opin. Drug Deliv. 11, 901–915. doi: 10.1517/17425247.2014.902047
Shen, J., Lu, G. W., and Hughes, P. (2018). Targeted ocular drug delivery with pharmacokinetic/pharmacodynamic considerations. Pharm. Res. 35:217. doi: 10.1007/s11095-018-2498-y
Spaide, R. F. (2017). Choroidal neovascularization. Retina 37, 609–610. doi: 10.1097/IAE.0000000000001575
Su, X., Tan, M. J., Li, Z., Wong, M., Rajamani, L., Lingam, G., et al. (2015). Recent progress in using biomaterials as vitreous substitutes. Biomacromolecules 16, 3093–3102. doi: 10.1021/acs.biomac.5b01091
Tabandeh, H., Boscia, F., Sborgia, A., Ciracì, L., Dayani, P., Mariotti, C., et al. (2014). Endophthalmitis associated with intravitreal injections. Retina 34, 18–23. doi: 10.1097/IAE.0000000000000008
Tanetsugu, Y., Tagami, T., Terukina, T., Ogawa, T., Ohta, M., and Ozeki, T. (2017). Development of a sustainable release system for a ranibizumab biosimilar using Poly(lactic-co-glycolic acid) biodegradable polymerbased microparticles as a platform. Biol. Pharm. Bull. 40, 145–150.
Tang, B. (2015). Electrospinning: Principles, Practice and Possibilities. Available online at: https://books.google.es/books?hl=es&lr=&id=znMoDwAAQBAJ&oi= fnd&pg=PP1&dq=Tang,+B.+Z.+(2015).+Electrospinning:+principles,+practice +and+possibilities.+Royal+Society+of+Chemistry.&ots=UdRDF2txrB&sig=y-iwskx-KpMKRAIvoKDMrtd8sAE (accessed July 9, 2020).
Tang, Y., and Le, W. (2016). Differential roles of M1 and M2 microglia in neurodegenerative diseases. Mol. Neurobiol. 53, 1181–1194. doi: 10.1007/s12035-014-9070-5
Tarallo, V., Hirano, Y., Gelfand, B. D., Dridi, S., Kerur, N., Kim, Y., et al. (2012). DICER1 Loss and Alu RNA induce age-related macular degeneration via the NLRP3 Inflammasome and MyD88. Cell 149, 847–859. doi: 10.1016/j.cell.2012.03.036
Thornton, J., Edwards, R., Mitchell, P., Harrison, R. A., Buchan, I., and Kelly, S. P. (2005). Smoking and age-related macular degeneration: a review of association. Eye 19, 935–944. doi: 10.1038/sj.eye.6701978
Tiwari, A., and Tiwari, A. (2013). Nanomaterials in Drug Delivery, Imaging, and Tissue Engineering. Hoboken, NJ: John Wiley & Sons.
Turturro, S. B., Guthrie, M. J., Appel, A. A., Drapala, P. W., Brey, E. M., Pérez-Luna, V. H., et al. (2011). The effects of cross-linked thermo-responsive PNIPAAm-based hydrogel injection on retinal function. Biomaterials 32, 3620–3626. doi: 10.1016/j.biomaterials.2011.01.058
Varshochian, R., Jeddi-Tehrani, M., Mahmoudi, A. R., Khoshayand, M. R., Atyabi, F., Sabzevari, A., et al. (2013). The protective effect of albumin on bevacizumab activity and stability in PLGA nanoparticles intended for retinal and choroidal neovascularization treatments. Eur. J. of Pharm. Sci. 50, 341–352. doi: 10.1016/j.ejps.2013.07.014
Varshochian, R., Riazi-Esfahani, M., Jeddi-Tehrani, M., Mahmoudi, A. R., Aghazadeh, S., Mahbod, M., et al. (2015). Albuminated PLGA nanoparticles containing bevacizumab intended for ocular neovascularization treatment. J. Biomed. Mater. Res. Part A 103, 3148–3156. doi: 10.1002/jbm.a.35446
Vilhardt, F., Haslund-Vinding, J., Jaquet, V., and McBean, G. (2017). Microglia antioxidant systems and redox signalling. Br. J. Pharmacol. 174, 1719–1732. doi: 10.1111/bph.13426
Villegas, V. M., Aranguren, L. A., Kovach, J. L., Schwartz, S. G., and Flynn, H. W. (2017). Current advances in the treatment of neovascular age-related macular degeneration. Expert Opin. Drug Deliv. 14, 273–282. doi: 10.1080/17425247.2016.1213240
Wang, C. H., Hwang, Y. S., Chiang, P. R., Shen, C. R., Hong, W. H., and Hsiue, G. H. (2012). Extended release of bevacizumab by thermosensitive biodegradable and biocompatible hydrogel. Biomacromolecules 13, 40–48. doi: 10.1021/bm2009558
Wang, K., Mitra, R. N., Zheng, M., and Han, Z. (2018). Nanoceria-loaded injectable hydrogels for potential age-related macular degeneration treatment. J. Biomed. Mater. Res. Part A 106, 2795–2804. doi: 10.1002/jbm.a.36450
Wang, Y., Liu, C. H., Ji, T., Mehta, M., Wang, W., Marino, E., et al. (2019). Intravenous treatment of choroidal neovascularization by photo-targeted nanoparticles. Nat. Commun. 10:804. doi: 10.1038/s41467-019-08690-4
Whitcup, S. M., and Robinson, M. R. (2015). Development of a dexamethasone intravitreal implant for the treatment of noninfectious posterior segment uveitis. Ann. N. Y. Acad. Sci. 1358, 1–12. doi: 10.1111/nyas.12824
Wilkinson, C., Schachat, A., Hinton, D., Freund, K. B., Sarraf, D., and Wiedemann, P. (2017). Ryan’s Retina -, 6th Edn, ed. S. V. Sadda (Amsterdam: Elsevier).
William, F. R., Sailor, M., Chen, M., and Cheng, L. (2012). Nanostructured porous silicon dioxide microparticles as an intravitreal injectable drug delivery system for avastin (Bevacizumab) lasting six months. Invest. Ophthalmol. Vis. Sci. 53:456.
Wills, N. K., Ramanujam, V. M. S., Chang, J., Kalariya, N., Lewis, J. R., Weng, T. X., et al. (2008). Cadmium accumulation in the human retina: effects of age, gender, and cellular toxicity. Exp. Eye Res. 86, 41–51. doi: 10.1016/j.exer.2007.09.005
Winkler, B. S., Boulton, M. E., Gottsch, J. D., and Sternberg, P. (1999). Oxidative damage and age-related macular degeneration. Mol. Vis. 5:32.
Xu, X., Weng, Y., Xu, L., and Chen, H. (2013). Sustained release of avastin§from polysaccharides cross-linked hydrogels for ocular drug delivery. Int. J. Biol.Macromol. 60, 272–276. doi: 10.1016/j.ijbiomac.2013.05.034
Xue, K., Zhao, X., Zhang, Z., Qiu, B., Tan, Q. S. W., Ong, K. H., et al. (2019). Sustained delivery of anti-VEGFs from thermogel depots inhibits angiogenesis without the need for multiple injections. Biomater. Sci. 7, 4603–4614. doi: 10.1039/c9bm01049a
Yandrapu, S. K., Upadhyay, A. K., Petrash, J. M., and Kompella, U. B. (2013). Nanoparticles in porous microparticles prepared by supercritical infusion and pressure quench technology for sustained delivery of bevacizumab. Mol. Pharm. 10, 4676–4686. doi: 10.1021/mp400487f
Yang, P., Dong, Y., Huang, D., Zhu, C., Liu, H., Pan, X., et al. (2019). Silk fibroin nanoparticles for enhanced bio-macromolecule delivery to the retina. Pharm. Dev. Technol. 24, 575–583. doi: 10.1080/10837450.2018.1545236
Yasukawa, T., Ogura, Y., Tabata, Y., Kimura, H., Wiedemann, P., and Honda, Y. (2004). Drug delivery systems for vitreoretinal diseases. Prog. Retin. Eye Res. 23, 253–281. doi: 10.1016/j.preteyeres.2004.02.003
Yatvin, M. B., Kreutz, W., Horwitz, B. A., and Shinitzky, M. (1980). pH-sensitive liposomes: possible clinical implications. Science 210, 1253–1255. doi: 10.1126/science.7434025
Ye, Z., Ji, Y.-L., Ma, X., Wen, J.-G., Wei, W., and Huang, S.-M. (2015). Pharmacokinetics and distributions of bevacizumab by intravitreal injection of bevacizumab-PLGA microspheres in rabbits. Int. J. Ophthalmol. 8, 653–658. doi: 10.3980/j.issn.2222-3959.2015.04.02
Yeo, Y., and Park, K. (2004). Control of encapsulation efficiency and initial burst in polymeric microparticle systems. Arch. Pharm. Res. 27, 1–12.
Yu, J., Xu, X., Yao, F., Luo, Z., Jin, L., Xie, B., et al. (2014). In situ covalently cross-linked PEG hydrogel for ocular drug delivery applications. Int. J. Pharm. 470, 151–157. doi: 10.1016/j.ijpharm.2014.04.053
Yu, Y., Lau, L. C. M., Lo, A. C., and Chau, Y. (2015). Injectable chemically crosslinked hydrogel for the controlled release of bevacizumab in vitreous: a 6-month in vivo study. Transl. Vis. Sci. Technol. 4:5. doi: 10.1167/tvst.4.2.5
Yu, Y., Lin, X., Wang, Q., He, M., and Chau, Y. (2019). Long-term therapeutic effect in nonhuman primate eye from a single injection of anti-VEGF controlled release hydrogel. Bioeng. Transl. Med. 4:e10128. doi: 10.1002/btm2.10128
Keywords: retinal pigment epithelium, Bruch’s membrane, retina, biomaterials, neuroprotection, ocular drug delivery, nanocarriers, VEGF
Citation: Jemni-Damer N, Guedan-Duran A, Fuentes-Andion M, Serrano-Bengoechea N, Alfageme-Lopez N, Armada-Maresca F, Guinea GV, Pérez-Rigueiro J, Rojo F, Gonzalez-Nieto D, Kaplan DL and Panetsos F (2020) Biotechnology and Biomaterial-Based Therapeutic Strategies for Age-Related Macular Degeneration. Part I: Biomaterials-Based Drug Delivery Devices. Front. Bioeng. Biotechnol. 8:549089. doi: 10.3389/fbioe.2020.549089
Received: 04 April 2020; Accepted: 06 October 2020;
Published: 03 November 2020.
Edited by:
Zhengwei Mao, Zhejiang University, ChinaReviewed by:
Timothy Charles Hughes, Commonwealth Scientific and Industrial Research Organisation (CSIRO), AustraliaPaYaM ZarrinTaj, Oklahoma State University, United States
Copyright © 2020 Jemni-Damer, Guedan-Duran, Fuentes-Andion, Serrano-Bengoechea, Alfageme-Lopez, Armada-Maresca, Guinea, Pérez-Rigueiro, Rojo, Gonzalez-Nieto, Kaplan and Panetsos. This is an open-access article distributed under the terms of the Creative Commons Attribution License (CC BY). The use, distribution or reproduction in other forums is permitted, provided the original author(s) and the copyright owner(s) are credited and that the original publication in this journal is cited, in accordance with accepted academic practice. No use, distribution or reproduction is permitted which does not comply with these terms.
*Correspondence: Fivos Panetsos, fivos@ucm.es
†These authors have contributed equally to this work