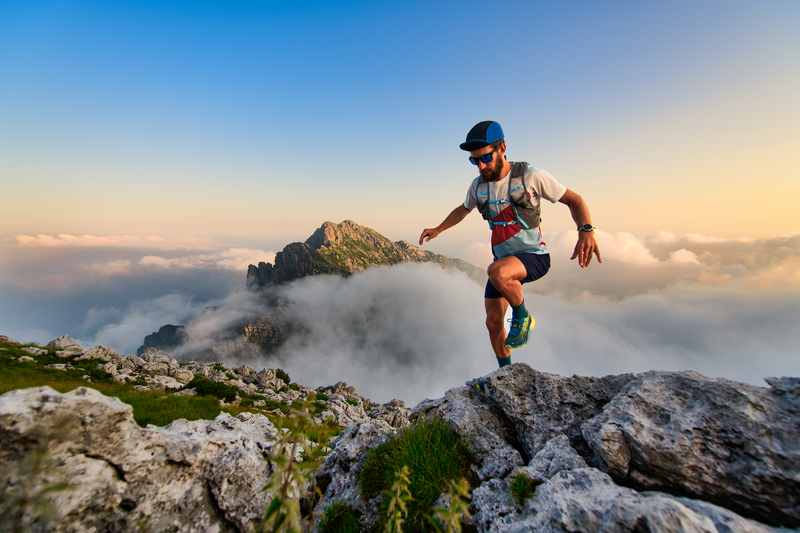
95% of researchers rate our articles as excellent or good
Learn more about the work of our research integrity team to safeguard the quality of each article we publish.
Find out more
REVIEW article
Front. Bioeng. Biotechnol. , 24 June 2020
Sec. Bioprocess Engineering
Volume 8 - 2020 | https://doi.org/10.3389/fbioe.2020.00640
This article is part of the Research Topic Stem Cell Systems Bioengineering View all 15 articles
Human mesenchymal stem cells (hMSCs) are a promising candidate in cell therapy as they exhibit multilineage differentiation, homing to the site of injury, and secretion of trophic factors that facilitate tissue healing and/or modulate immune response. As a result, hMSC-derived products have attracted growing interests in preclinical and clinical studies. The development of hMSC culture platforms for large-scale biomanufacturing is necessary to meet the requirements for late-phase clinical trials and future commercialization. Microcarriers in stirred-tank bioreactors have been widely utilized in large-scale expansion of hMSCs for translational applications because of a high surface-to-volume ratio compared to conventional 2D planar culture. However, recent studies have demonstrated that microcarrier-expanded hMSCs differ from dish- or flask-expanded cells in size, morphology, proliferation, viability, surface markers, gene expression, differentiation potential, and secretome profile which may lead to altered therapeutic potency. Therefore, understanding the bioprocessing parameters that influence hMSC therapeutic efficacy is essential for the optimization of microcarrier-based bioreactor system to maximize hMSC quantity without sacrificing quality. In this review, biomanufacturing parameters encountered in planar culture and microcarrier-based bioreactor culture of hMSCs are compared and discussed with specific focus on cell-adhesion surface (e.g., discontinuous surface, underlying curvature, microcarrier stiffness, porosity, surface roughness, coating, and charge) and the dynamic microenvironment in bioreactor culture (e.g., oxygen and nutrients, shear stress, particle collision, and aggregation). The influence of dynamic culture in bioreactors on hMSC properties is also reviewed in order to establish connection between bioprocessing and stem cell function. This review addresses fundamental principles and concepts for future design of biomanufacturing systems for hMSC-based therapy.
Human mesenchymal stem cells (hMSCs) are a rising candidate for cell therapy and have attracted growing interests due to their ability of immunomodulation and trophic effects beyond tri-lineage differentiation. With thousands of in vitro and in vivo studies and more than 1000 hMSC-based clinical trials completed or in progress on ClinicalTrials.gov, the potential of hMSCs in therapeutic applications is very promising (Atkinson et al., 2017; Tsuchiya et al., 2019). However, to confirm the effectiveness of hMSCs in cell therapy, late phases of clinical trials require a large amount of cells for transplantation and administration into patients (Yin et al., 2019). In addition, as an immunomodulator, hMSCs exhibit immunoprivileged/immunoevasive properties and can be used in allogeneic therapies, which also demand large-scale biomanufacturing because of the cost of goods (Rowley et al., 2012; Simaria et al., 2014; Zhang et al., 2015; Schnitzler et al., 2016). Due to the limited number of hMSCs acquired from a single donor, in vitro expansion under current Good Manufacturing Practices (cGMP) has to be performed to reach pratical cell numbers for dosage requirements in clinical applications (Rojewski et al., 2013; Barckhausen et al., 2016; McGrath et al., 2019). In addition, as an anchorage-dependent cell type, the number of harvested hMSCs should be proportional to the culture surface area in biomanufacturing. Thus, increasing culture surface without sacrificing spacial and labor costs is critical in designing culture vessels in hMSC biomanufacturing. One current technique uses multi-layer vessels designed for cell expansion by stacking layers into one chamber to increase the culture surface. However, these labor-extensive and semi-closed processes require clean room facilities and class-A laminar biosafety cabinets for each step of operation (dos Santos et al., 2013; Martin et al., 2017). Alternatively, automated well-controlled bioreactors provide efficient mixing in a closed system for large-scale expansion in lot size at reduced labor and time, but these automated bioreactors are not readily available (Grayson and Stephenson, 2018; Olsen et al., 2018; Moutsatsou et al., 2019). Among various types of bioreactors that are commercially available, stirred-tank bioreactors with microcarriers are the most commonly used system for scaling-up manufacturing of hMSCs as the microcarriers provide a high surface-to-volume ratio for high density cell culture with a cost of goods reduction ($0.044 per cm2) compared to plate stacks ($0.061 per cm2) (Simon, 2015). Moreover, microcarrier suspension culture allows real-time cell sampling and off-line analysis for monitoring culture parameters and evaluating critical stem cell properties during expansion. Different feeding strategies, such as batch, fed-batch, and perfusion (dos Santos et al., 2014; Fernandes-Platzgummer et al., 2016), with bead-to-bead transfer can support hMSC stable proliferation under short- and long-term expansion (Panchalingam et al., 2015).
The advantages of microcarrier culture in stirred-tank bioreactors include the scalable design, even cell distribution, homogeneous nutrition and oxygen access, and the timely assessment of medium composition and evaluation of cell properties. Nevertheless, recent studies have shown that microcarrier-expanded hMSCs differ from dish- or flask-expanded cells in size, morphology, proliferation, viability, surface marker, gene expression, differentiation capacity, and secretion of cytokines, which may lead to the alteration of their therapeutic potency (Goh et al., 2013; Hupfeld et al., 2014; Lin et al., 2016; Teixeira et al., 2016). Thus, hMSC properties exhibited in planar culture may not be consistent with microcarrier culture. As seen in our previous studies, these deviations likely result from the altered microenvironment between planar and microcarrier culture in seeding, attaching, expanding, and harvesting, as well as the change of adhesion surface geography and flow-induced dynamic environment (Ma et al., 2016). Therefore, it is important to understand how to optimize bioreactor conditions to maximize hMSC quantity without sacrificing quality and therapeutic potency (Castilla-Casadiego et al., 2020). In this review, influences on hMSC properties from manufacturing parameters in microcarrier bioreactor culture (e.g., discontinuous surface, curvature, microcarrier stiffness, porosity, roughness, coating, and charges) and flow dynamics (e.g., oxygen and nutrient diffusion, shear stress, particle collision, and aggregation) are discussed. Advanced techniques and processes to improve hMSC expansion in microcarrier-based bioreactors are also reviewed.
Originally, hMSCs are isolated from bone marrows, and only occupy 0.001–0.01% of mononuclear cells for healthy adults (Pittenger et al., 1999). hMSCs isolated from other tissues, such as adipose tissues, dermal tissue, dental pump, placenta, and umbilical cords, contain a higher percentage of hMSCs (Fernandes-Platzgummer et al., 2016). For instance, human adipose tissue on average contains 1.2% hMSCs (Fraser et al., 2006), and umbilical cords have 0.3% hMSCs (Wegmeyer et al., 2013). Even though these numbers are 10 to 100 times higher than bone marrow, it is still far below the cell number requirement of one single therapeutic dose (35–350 million hMSCs per dose) in clinical applications (Jossen et al., 2016). Therefore, in vitro expansion is necessary to achieve sufficient cells for cell-based therapies. As the demand for hMSC-based therapeutics is increasing exponentially, development of noval biomanufacturing techniques for culture expansion is in urgent need (Olsen et al., 2018).
While most cell therapy products rely on autologous cells for immunologic compatibility (Duijvestein et al., 2010; Honmou et al., 2012), hMSCs provide a possibility in allogeneic therapies as an “off-the-shelf” product (Pittenger and Martin, 2004; Newman et al., 2009; Zhang et al., 2012). According to Robb et al.'s study, 44.05% of clinical trials were targeting allogenic therapies (Robb et al., 2019). Among the 13 commercialized hMSC products, 9 of them use allogeneic approaches (Jossen et al., 2018). In autologous therapies, a rapid expansion of patient-specific cells in a fully closed and automated bioreactor system is important to ensure the product free of cross-contamination with a reasonable yield in minimal production time. The scale-out system can satisfy the need of autologous therapies to parallelly produce personalized products for specific patients (Hourd et al., 2014). In allogeneic therapies, hMSCs manufactured from one or several selective donors are used as a universal drug for multiple patients. The scale-up manufacturing system can reduce the cost of goods to meet the dosage requirement and “off-the-shelf” standard (Pigeau et al., 2018). The importance of the scale-up process is to obtain homogeneous cells from a single batch and eliminate the lot-to-lot variations to manufacture hMSCs as a standardized product. Therefore, the current hurdle for hMSC large-scale expansion in allogeneic therapies is to minimize donor-to-donor variations, reduce bioprocessing fluctuations, and eliminate the cell heterogeneity.
Due to the diversity of characteristics displayed by hMSCs from different sources with various isolation methods, the International Society for Cellular Therapy (ISCT) recommended minimal criteria to define hMSCs: (1) cell adhesion on the plastic surface, (2) specific positive and negative surface markers, and (3) in vitro tri-lineage differentiation (Dominici et al., 2006). Although these criteria are helpful to establish the baseline of hMSC characterizations, they cannot be used to evaluate hMSC therapeutic potentials as most of the hMSCs from different research groups satisfy ISCT's criteria while still exhibiting functional variance (Samsonraj et al., 2015; Yuan et al., 2019). For effective cell therapies, hMSC-based products have to be characterized for efficacy, function, and potency. For instance, ISCT reported the test for the levels of vascular endothelial growth factor (VEGF), chemokine (C-X-C motif) ligand 5 (CXCL5), and interleukin (IL)-8 of manufactured MultiStem® (a cellular therapy product of hMSCs) to assess the potency in angiogenesis (Bravery et al., 2013). Similarly, ISCT also required immunological characterization for hMSCs to treat immunological diseases with standardized approaches (Krampera et al., 2013; Galipeau et al., 2016). Although many research groups have reported donor-to-donor variations and tissue source comparisons (Alge et al., 2010; Al-Nbaheen et al., 2013; Menard et al., 2013; Marquez-Curtis et al., 2015; Billing et al., 2016; Isobe et al., 2016), only few studies explored the impact of bioprocessing parameters on hMSC therapeutic potency (Hupfeld et al., 2014; Teixeira et al., 2016; Cunha et al., 2017; Martin et al., 2017).
Distinct from typical bioprocesses for protein production using organisms, such as E. coli, yeast, and Chinese hamster ovary cells, hMSCs themselves are the final products for cell-based therapy. Thus, the focus is on the fold increase in cell number and population doublings rather than the extracellular factor production, although extracellular vesicles have attracted tremendous attentions recently (Phinney et al., 2015; Koniusz et al., 2016). Isolation from non-adherent hematopoietic cells and in vitro expansion of hMSCs in planar culture is the initial process, although the resulting cell number can not meet the clinical requirements (Chen et al., 2013; Siddiquee and Sha, 2014; Simaria et al., 2014). Thus, it is necessary to develop new platforms to efficiently expand functional hMSCs on a larger scale to reach sufficient cell number and dosages, which theoretically determines the success of clinical trials (Rowley et al., 2012; Olsen et al., 2018).
The manufacturing processes under cGMP regulations normally require complete defined serum-free or xeno-free media, effective feeding strategies, automated closed systems, efficient harvest process, cell purification, and cryopreservation methods (Sotiropoulou et al., 2006; Sensebé et al., 2013; Li et al., 2015). The expansion time and population doublings are commonly used as critical quality attributes of the products to evaluate these operational parameters. Knowledge of process control and the parameters acquired from static planar cultures may not be appropriate to directly translate into dynamic bioreactor culture systems without modifications due to the dramatic change in the microenvironment (Sart et al., 2014a; Ma et al., 2016). Therefore, the impacts of bioprocess parameters on hMSC phenotype, cell fate, and therapeutic potency should be investigated systematically. Figure 1 illuminates the types of mass transfer in static culture and dynamic culture in biomanufacturing.
Figure 1. Mass transfer for microcarrier-based culture. For static culture, hMSCs are expanded as monolayers in planar culture or multilayers in the fixed/packed or hollow fiber bioreactor. Mass transfer only relies on diffusion in planar culture, whereas additional convection enhances the mass transfer efficiency in the fixed/packed bed and hollow fiber bioreactor. For dynamic culture in the microcarrier-based bioreactor, hMSCs first attach and grow as monolayers on isolated microcarriers in which convection flows play a more important role in transport. Later, multiple microcarriers are aggregated into a cluster, the internal area of which requires further diffusion for mass transfer like multilayers.
To manufacture adherent hMSCs, the producion depends on the accessible surface area. Large-scale production of hMSCs requires large surface area, to support efficient cell proliferation while preserving their innate biological properties. Ameliorating from T-flasks, multi-layer vessels, including Nunc™ Cell Factory™ (Thermo Fisher Scientific) and Corning® CellSTACK® (Corning), allow for cell expansion in one chamber by stacking the layers to save the space for incubation, and thus can produce >100 times of cells from a single T-flask. For example, a 40-layer vessel provides a surface of 25,280 cm2 which is 144 times of a conventional T-175 flask. The challenge in multi-layer vessel production is the labor-intensive and time-consuming operation. Advanced equipment like automatic robot systems, such as Nunc™ Automatic Cell Factory™ Manipulator System (Thermo Fisher Scientific), are designed for more convenient operations and lowering the risk of contamination.
hMSCs are highly sensitive to biological, chemical, and physical cues in the culture microenvironment, and changes in gene expression and phenotypical markers were observed in planar culture (Bara et al., 2014). Thus, hMSCs cultured in multi-layer vessels exploiting the similar microenvironment to original T-flasks make the bioprocess transfer easier, such as coating surface, seeding density, feeding regimes, and cell harvesting (Ma et al., 2016). So far, multi-layer vessels are commonly used in commercial manufacturing but still with the limited lot size, ranging from 100 to 400 billion cells (Rowley et al., 2012). Furthermore, limitation in characterizations of cell growth and behaviors still exists. For example, real-time observation of cell morphological changes may not be applicable under a regular microscope.
Instead of providing a planar surface, fixed-bed/packed-bed bioreactors and hollow fiber bioreactors create a spatial microenvironment for cell growth. In fixed/packed-bed bioreactors, hMSCs are captured within the bed that can be filled with various scaffolds composed of biocompatible polystyrene pellets, glass beads, or fibrous materials to provide large surface area for cell expansion (Zhao et al., 2007; Weber et al., 2010; Osiecki et al., 2015; Tsai et al., 2016). Similarly, in hollow fiber bioreactors (Nold et al., 2013; Mizukami et al., 2018), hMSCs grow in the interstitial space between hollow fibers that were used to mimic blood capillaries (Hanley et al., 2014; Martin-Manso and Hanley, 2015). In these two types of bioreactors, the network of frame structures in hMSC microenvironment not only prevents cells from direct fluidic force, but also supports three-dimensional (3D) cell growth with retention of extracellular matrix (ECM). In addition, 3D architecture better mimics the in vivo physiological environment. In the absence of direct exposure to the flow, hMSCs still can benefit from perfused flow, mainly relying on diffusion for mass transfer of nutrients, waste, and oxygen. As a result, cells do not suffer damages from shear stress and physical collisions (Figure 1). Therefore, hMSCs can be cultured in a highly compact 3D system while still reaching a high cell number.
However, fixed/packed-bed bioreactors and hollow fiber bioreactors still have some challenges in the large scale of biomanufacturing: (1) the non-homogeneity of the culture systems; (2) the depletion of nutrition and accumulation of waste when the cell density is too high and the interstitial flow is insufficient to satisfy mass transfer needs; and (3) cell dissociation from a highly condensed 3D clusters (Meuwly et al., 2007; Barckhausen et al., 2016). Other crucial issues include limited potential in scaling-up and inability for continuous long-term culture without enzymatic treatment and passaging. To avoid the depletion of nutrients and accumulation of metabolic waste, perfusion mode can be applied to increase the flow rate according to the real time in culture. A real time analysis of cell state is also possible in fixed bed bioreactor, for example, via oxygen measurements at inlet and outlet and the calculation of cell numbers over oxygen consumption.
Microcarriers, designed to replace T-flasks and petri dishes as the adhesion surface for cell growth, have been used for human cell culture since 1967 (Van Wezel, 1967). Most studies on hMSC expansion with microcarriers were conducted in spinner flasks or stirred-tank bioreactors (António et al., 2016), though some other dynamic systems, such as rotating wall vessels or wave motion bioreactors, have also been reported. The apparent advantages for microcarrier suspension culture include the scalable design, homogeneous culture environment, real-time monitoring of cells and medium, and the feasibility to maintain long-term culture via bead-to-bead transfer without enzymatic treatment/passaging (Leber et al., 2017; Rafiq et al., 2018). Moreover, due to the high surface to volume ratio, less culture medium (a main cost driver) is used in hMSC-microcarrier bioprocessing. Therefore,the production cost is reduced. Although this platform has been widely exploited in academia and industry (Badenes et al., 2016a,b), recent studies have shown that cells cultured in spinner flasks or stirred-tank bioreactors are exposed to high and non-homogeneous fluid shear stresses due to the mixing agitation (Ismadi et al., 2014; van Eikenhorst et al., 2014), possibly resulting in reduced hMSC qualities and therapeutic potentials. For example, increasing fluid shear stress during hMSC culture can induce osteogenic and chondrogenic differentiation (Knippenberg et al., 2005; Zhao et al., 2007; Li et al., 2009; Yourek et al., 2010; Schatti et al., 2011). Therefore, application of the slowest agitation rate is always recommended to support both the required mixing of culture and the undifferentiated state of stem cells. Additionally, the fluid flow leads to frequent cell-cell collisions and may result in spontaneous aggregation and clustering, which should be cautiously considered at the late stage of stem cell culture (Caruso et al., 2014; Yuan et al., 2018).
To date, a wide variety of microcarriers are commercially available. These microcarriers are made of diverse materials, such as polystyrene, dextran, cellulose, gelatin, glass, or decellularized tissue, with different surface properties (Chen et al., 2013; Yu et al., 2017). The size of microcarriers ranges from 100 to 300 μm in diameter, which is large enough for cell adherence. The density of microcarriers is designed between 1.02 and 1.1 g/cm3, not only for settling down the microcarriers when changing the medium, but also for reducing input energy of agitation for the submerged suspension. Therefore, screening and comparing these commercial microcarriers to assess hMSC expansion is the initial step.
In addition to the size and density, microcarriers should be evaluated for cell adhesion, expansion, and dissociation. Recently, some commercial microcarriers have been modified from traditional non-porous, uncoated, uncharged surfaces to porous, collagen- or fibronectin-coated, and/or surfaces with positive charge for enhancing cell attachment efficiency (Rafiq et al., 2013a). Based on literature, hMSC attachment on microcarriers can achieve 70% to 90% under the static or dynamic condition in serum-containing medium, whereas the efficiency significantly decreases to 22% to 23% in serum- and xeno-free medium (dos Santos et al., 2011; Timmins et al., 2012). But there is also evidence that hMSCs can grow fast and achieve the same cell density in a xeno-free serum compared to serum-containing culture despite the low adhesion efficiency (Moreira et al., 2020). Currently, most studies on screening microcarriers for bioprocessing only emphasize the seeding efficiency and expansion fold; however, the recovery efficiency or yield is equally critical for the large-scale production (Schnitzler et al., 2012; Timmins et al., 2012; Goh et al., 2013; Rafiq et al., 2016; Moloudi et al., 2019). The cell detachment from microcarriers is not considered as a key factor and has not been optimized in traditional bioprocessing of protein production. Nevertheless, as the final products in cell-based therapies, hMSCs have to be isolated and harvested from these bioreactors. Thus, the detachment efficiency and recovery rate can not be ignored. For example, hMSCs have high expansion fold using Cytodex I microcarriers, but the enzymatic detachment efficiency is low, and it eventually leads to the low cell yield after cell harvest (Weber et al., 2007; Sun et al., 2010; Schnitzler et al., 2012; Timmins et al., 2012; Loubière et al., 2019). Similarly, although macropores provide more surface area for cell expansion and protect cells from direct flows, i.e., shear stress resulted from the eddies at the Kolmogorov scale, the detachment efficiency is generally not applicable for hMSC expansion (Nienow et al., 2016).
In addition to commercial microcarriers, customized microcarriers can be developed to improve the bioprocessing for hMSC attachment, proliferation, and harvest as well as maintaining their phenotype and therapeutic potency. For example, hydrogel-based microcarriers can improve cell attachment and harvesting efficiency (Chui et al., 2019). Thermo-responsive surfaces on microcarriers have been developed to improve harvest efficiency without enzymatic treatment (Yang et al., 2010; Song et al., 2016; Yuan et al., 2018). Biodegradable, implantable, or enzyme-dissolvable microcarriers can avoid the dissociation or separation step during cell harvesting (Sart et al., 2009; Sun et al., 2010; Park et al., 2013; Shekaran et al., 2016; Confalonieri et al., 2017; Wang et al., 2017; Rodrigues et al., 2019). Microcarriers coated with chemically-defined polymers can better control culture systems and increase the reproducibility (Krutty et al., 2019). Hollow microcarriers provide inner surface for cells to grow and are able to protect hMSCs from direct flow damage (YekrangSafakar et al., 2018). Magnetic microcarriers can speedily settle down when changing medium, and are easily separated from cells after enzymatic treatment to improve cell yield (Lin et al., 2014). Modification of surface charge may be another approach to enhance hMSC attachment or detachment (Rafiq et al., 2016). However, each method should be carefully reviewed and modified for large-scale bioprocessing as most studies were conducted at the laboratory scale. For example, when using the microcarriers with a thermo-sensitive surface, the temperature should be well-controlled throughout bioprocessing. To simplify the harvesting process, novel dispersible and dissolvable porous microcarriers have also been reported recently (Yan et al., 2020).
Agitation in stirred-tank bioreactors provides the driving force to generate convective flow, and proper agitation is needed for homogenization of culture microenvironment, dispersion of gas and nutrients, optimal mixing, reduction of the laminar boundary layer, and to increase the convective mass transfer coefficients, all of which are important in MSC bioprocessing. For bioreactors at different scales and with different microcarriers, the operation of agitation should be evaluated for hMSC adhesion, expansion, and dissociation.
To maximize seeding efficiency with an even cell distribution, it is recommended to utilize intermittent agitation and reduced initial working volume. For example, the use of intermittent agitation (3 min agitation at 60 rpm followed by no agitation for 27 min) showed 1.5 to 2-fold higher attachment efficiency than the continuous agitation (60 rpm) in the first 24 h for hMSC expansion on CultiSpher-S microcarriers (Yuan et al., 2014).
To determine the agitation speed for hMSC expansion, NS1 and NS1u were introduced to represent the criteria of agitation for microcarrier suspension culture. NS1 stands for the minimum impeller speed that fully suspends the microcarriers, and NS1u represents the agitation speed that merely allows microcarriers moving along the bottom of bioreactors (Schirmaier et al., 2014; Jossen et al., 2016). It is noted that NS1 and NS1u are highly dependent on working volume, microcarrier type and concentration. For instance, Kaiser et al. found no significant difference in expansion when culturing adipose tissue-derived hMSCs at 49, 60, and 82 rpm on Hillex® II and ProNectin®-F-coated microcarriers, and it is possible that the agitation speed is still within the optimal NS1 range (Kaiser et al., 2013). A follow-up study tested a broader range of agitation speeds (25–120 rpm) in spinner flasks with 100 mL working volume, and the results showed that hMSCs reached the highest expansion (117-fold) at 49 rpm, and only 71-fold and 19-fold increase were observed at 25 and 120 rpm, respectively (Jossen et al., 2016). Another study showed that hMSCs cultured on CultiSpher-S microcarriers at 60 and 90 rpm had slightly higher expansion (5.5-fold) compared to the culture at 115 rpm (4.3-fold) after an 8-days culture (Yuan et al., 2014). Undoubtedly, optimized agitation speed is critical for hMSC expansion, because higher agitation speed may inhibit hMSC growth due to cell exposure to high shear stress, while low agitation speed cannot fully suspend microcarriers, leading to microcarrier clustering (Jossen et al., 2014; Takahashi et al., 2017). Furthermore, the agitation speed has a linear correlation to the average fluid shear stress in spinner flasks (Ismadi et al., 2014). Based on this observation, Nienow reported a method to detach hMSCs from Plastic P102-L microcarriers with a short time of exposure to the high agitation (220 rpm), which generates high shear stress and reduces Kolmogorov scale (Nienow et al., 2014). Our study also showed the hMSC expansion when cultured on Cytodex-1 microcarriers (Tsai and Ma, 2016). The 30 rpm agitation was observed to support active metabolic activity and glucose consumption, which is usually associated with high cell growth rate (Figure 2).
Figure 2. Example of hMSC growth in microcarrier-based spinner flasks. (A) Spinner flask bioreactor and internal components (top). Cytodex I microcarriers (bottom). (B) hMSC proliferation measured by MTT intensities at day 1, 3, 5, and 7. (C) Concentration of glucose and lactate in the media over a 7-days culture period. (D) The glucose consumption and lactate production with the lactate/glucose ratio over a 7-days culture period. (E) MTT staining of hMSCs on Cytodex I at day 1, 3, 5, and 7. The spinner flask was operated at 30 rpm. Reference: Tsai and Ma (2016). Copyright was permitted.
It needs to be noted that the agitation speed using rpm cannot be compared among different culture systems. It is better to use Reynolds number or tip speed [m/s], with the knowledge of the exact reactor set up and geometry. In particular, to transfer the knowledge from spinner flasks to large stirred tank bioreactors, a dimensionless number, such as Reynolds number should be used.
Considering the high and non-homogeneous shear stress in the spinner flasks or stirred-tanks, several bioreactor geometries have been designed to reduce the effects of shear stress. For example, an indentation on the bottom of the spinner flasks can prevent microcarriers from accumulation and clustering below the impeller where the lowest flow velocity occurs (Kaiser et al., 2013). Side baffles can convert the rotational flow to radial flow and axial flow to enhance the microcarrier suspension (Nienow, 2006; Rafiq et al., 2013a). The dimensions, shapes, locations, and orientations of impellers exhibit specific different hydrodynamics, in terms of microcarrier suspension and shear stress exposure (Nienow, 2006; Ismadi et al., 2014; Collignon et al., 2016). A pitched-blade impeller design has been commonly used in stirred-tank bioreactors for hMSC expansion (Goh et al., 2013; Rafiq et al., 2013a; dos Santos et al., 2014; Siddiquee and Sha, 2014; Fernandes-Platzgummer et al., 2016).
Compared to stirred-tank bioreactors, spinner flasks (Figure 2) also create dynamic environment due to stirring and can be easily set up in a regular incubator for process optimization and characterization. The available volume for commercial spinner flasks are from 25 mL to 36 L, with 50–250 mL commonly selected for lab use (Eibes et al., 2010; dos Santos et al., 2011; Hewitt et al., 2011; Kaiser et al., 2013; Caruso et al., 2014; Hervy et al., 2014; Schirmaier et al., 2014; Heathman et al., 2015; Petry et al., 2016; Tsai and Ma, 2016). Although spinner flasks have comparable design to stirred-tank bioreactors, there still exist some differences (Table 1). Volumetric productivity and fold increase can be used to evaluate hMSC expansion. Rafiq et al. (2013a) demonstrated that 5 L stirred-tank bioreactors can support slightly higher expansion fold (6.02 and 7.02) of hMSCs than 100 mL spinner flasks (3.66 and 5). Similar trends were also reported by Goh et al. using 1 L stirred-tank bioreactors in comparison with 100 mL spinner flasks (Goh et al., 2013). Though, scaling-up normally deals with non-homogeneous quality and a lower yield because of extensive handling and mass transfer limitations (Hernandez, 2016), there are considerations in bioprocessing for choosing stirred-tank bioreactors rather than spinner flasks. As an open system, spinner flask is suitable for laboratory expansion and cannot be scale-up with processing control and automation. Moreover, hMSC products of clinical grade have requirements for product standardization and robustness, which can only be achieved through closed bioreactor systems. Due to a better control on culture environment (e.g., pH, oxygen), improved hMSC expansion can be observed in stirred-tank bioreactors compared to spinner flasks.
In spinner flasks, pH value is regulated by the ambient CO2 concentration in the incubator and there is no control on dissolved oxygen (DO). Conversely, most large-scale stirred-tank bioreactors can balance the pH value by adding diluted acids or bases, and compensate the DO level by adjusting partial oxygen tension input or turning on the gas sparging. Another discrepancy is that large-scale stirred-tank bioreactors are usually equipped with a condenser to avoid medium evaporation, which is alleviated by the humidified ambiance in the incubator (95% relative humidity at 37°C) for spinner flasks. Positive pressure in the head space also prevents evaporation in bioreactors. Other differences, including the extra perfusion system to attenuate media variation, the baffles on the side wall to enhance vertical mixing, and the homogenization at different scales of working volume with different impeller geometry, may further promote hMSC production in stirred-tank bioreactors.
The challenges in large scale manufacturing of hMSCs lie in the mixing of circulation system and non-homogeneous cell quality. The process parameters from several studies for scale-up production of hMSCs are summarized in Table 2. Only a few cases achieved successful expansion of hMSCs in bioreactors at large scale. For example, Schirmaier et al. reported that production of 1 × 1010 hMSCs can be achieved at a harvest density of 3 × 105 cells/mL in a 50-L single-use stirred-tank bioreactor (CultiBag® STR 50 L, Sartorius Stedim Biotech) with 35 L working volume (Schirmaier et al., 2014). Similarly, Lawson et al. reported that 1.28 × 1010 hMSCs were harvested at the density of 2.56 × 105 cells/mL in a 50-L single-use stirred-tank bioreactor (Mobius® 50 L, MilliporeSigma) with 50 L working volume (Lawson et al., 2017). In spite of the lower harvest cell density in large scale bioreactors (Table 2), comparable volumetric productivity in UniVessel® SU 2 L bioreactors (Sartorius Stedim Biotech) and CultiBag® STR 50 L bioreactors was also reported (Schirmaier et al., 2014). The commonly used scale up criteria include similar power input per volume, gas flow rate per reactor volume (vvm), and oxygen transfer coefficients (Xu et al., 2017). The correspondingly adjusted operational parameters, such as the geometry of vessels and impellers, agitation speed, microcarrier concentration, working volume, aeration (type and vvm), feeding strategies, and seeding/harvesting procedures, all impact culture microenvironment and thus cell production (Castilla-Casadiego et al., 2020).
Table 2. Case studies of microcarrier-based bioreactor systems for human mesenchymal stem cell expansion.
hMSCs cultured on microcarriers are exposed to a significantly different microenvironment from planar culture, therefore it is still unclear how much knowledge obtained from planar culture can be translated to microcarrier culture. Most studies mainly reported the increased folds in cell number, the expression of surface markers, colony formation ability, and differentiation capability of microcarrier-expanded hMSCs. However, these criteria only represent the minimal properties of hMSCs and do not indicate their therapeutic potency. In addition, bioprocessing parameters, such as type of microcarriers, controlled agitation, and culture scale, all may influence hMSC properties. Thus, understanding how the microcarrier culture acts on cellular behaviors and how these process parameters change therapeutic potency are beneficial for hMSC-based therapies and the associated biomanufacturing (Table 3).
Table 3. Alteration of hMSC properties from planar culture to microcarrier-based bioreactor culture.
In microcarrier culture of hMSCs, a long lag phase in cell proliferation is commonly observed (Eibes et al., 2010; Sun et al., 2010; dos Santos et al., 2011; Hewitt et al., 2011; Goh et al., 2013), suggesting that the cells need longer time to adjust themselves to the culture environment in bioreactors compared to planar culture. The microcarrier culture systems have not been maturely developed for hMSC expansion yet, and may not have reached the maximum expansion potential. hMSCs grow faster in planar culture than microcarrier culture, showing higher proliferation rates and lower doubling times (Sun et al., 2010; Goh et al., 2013). However, when only considering the exponential phase, microcarrier culture can have comparable or increased growth rate after optimization (Sun et al., 2010). Moreover, long-term culture can be achieved by bead-to-bead transfer in microcarrier bioreactors. Hence, as long as the lag phase can be reduced, microcarrier bioreactors are suitable for the production of hMSCs within a comparable time to planar culture.
In planar culture, cell density is typically expressed as cells/cm2. In microcarrier culture, cell density can also be described by cells/mL. Using vendor's information on the surface area per unit mass of microcarriers (cm2/g), growth area at a certain microcarrier concentration in the vessel can be calculated. Loss of microcarriers may occur in each step of washing, transferring, medium changing, and harvesting. Extra microcarriers, about 5–10%, can be added for compensation if needed. With online systems, e.g., impedance spectroscopy, cell biomass on the microcarriers can be determined at real time with no loss due to sampling.
Current studies on microcarrier expansion of hMSCs usually provide results for the expression of positive surface markers (CD73, CD90, and CD105) and negative surface markers (CD45, CD34, CD14 or CD11b, CD79α or CD19, and HLA-DR) along with in vitro tri-lineage differentiation capacity (dos Santos et al., 2011; Goh et al., 2013; Kehoe et al., 2013; Rafiq et al., 2013a; Caruso et al., 2014; Siddiquee and Sha, 2014; Jossen et al., 2016; Petry et al., 2016). It is well-known that surface marker expression can be different for hMSCs due to various donors and tissue sources. Hupfeld et al. (2014) manifested that the bioprocessing (planar vs. microcarrier) may affect the expression of hMSC phenotypic marker CD349 (frizzled 9) in three different donors. In particular, flask-expanded hMSCs derived from amniotic membrane and umbilical cord positively expressed CD349, whereas microcarrier-expanded hMSCs did not. And CD349− hMSCs derived from placenta can effectively recover blood flow after vascular occlusion in a mouse model rather than CD349+ hMSCs (Tran et al., 2011), suggesting that microcarrier-expanded hMSCs may have higher capacity of arteriogenesis and angiogenesis (Hupfeld et al., 2014). Other surface markers, including CD136, CD143, CD146, and CD200, were expressed inconsistently in Hupfeld's study. Interestingly, Shekaran et al. reported that a significant decrease of CD146 expression, known as a pericyte- and endothelial-specific marker (Shi and Gronthos, 2003) or a marker of hMSC multi-potency (Russell et al., 2010), in microcarrier-expanded fetal hMSCs compared to planar culture (Shekaran et al., 2015). Moreover, the expression of CD105 was reported to decrease from more than 90% to 85.9 ± 7.9 and 86.7 ± 2.4 for Cultispher-S and plastic microcarriers, respectively (dos Santos et al., 2011; Mizukami et al., 2018).
In addition, microcarrier-expanded hMSCs were reported to have higher expression of early osteogenic gene markers, such as RUNX2, ALPL, and Osterix/SP7, and late osteogenic marker IBSP during osteogenic differentiation, indicating that hMSCs from microcarrier culture may favor osteogenic lineage commitment (Shekaran et al., 2016). Similarly, microcarrier culture was reported to up-regulate RUNX2, ALP, COL1, and SOX9 gene expression of placenta-derived hMSCs compared to planar culture (Tseng et al., 2012). Microcarrier-expanded hMSCs were also reported to have higher gene expression of crucial chondrogenic transcriptional regulators, such as SOX9, SOX5, and SOX6, as well as the chondrogenic ECM marker COL2A1, suggesting that microcarrier culture may augment chondrogenic commitment (Lin et al., 2016). In addition, hMSCs harvested from microcarriers were found to have slightly lower CFU-F number in comparison with hMSCs from monolayers (Goh et al., 2013). Altogether, hMSCs manufactured from microcarrier culture systems still meet the release criteria defined by ISCT, although the cells exhibit differences in surface markers, CFU-F, and lineage-specific gene expression.
To date, more than 1000 completed or ongoing hMSC-based clinical trials have been reported (Tsuchiya et al., 2019), including bone and cartilage regeneration, graft-vs.-host disease, kidney injury, liver disease, myocardial infarction, and type I and II diabetes (Chen et al., 2013; Simaria et al., 2014). These clinical applications of hMSCs are attributed to unique stem cell properties: renewability of regeneration, capacity for multi-lineage differentiation, migration ability to inflammatory tissue, and secretion of anti-inflammatory and pro-angiogenic trophic factors (Aggarwal and Pittenger, 2005; Prockop et al., 2010; Wang et al., 2012; Bianco et al., 2013; Stroncek et al., 2014). However, most therapeutic characterizations are based on hMSCs expanded from planar culture system. Thus, it is necessary to characterize hMSCs manufactured from microcarrier culture, particularly in therapeutic efficacy which is generally indicated by differentiation potential, migratory ability, and secretory function.
It is well-acknowledged that hMSCs are able to differentiate into osteoblasts, adipocytes, and chondrocytes in vitro using inducing factors in the medium (Pittenger et al., 1999). The tri-lineage differentiation capability is included in the release criteria of hMSCs by ISCT (Dominici et al., 2006). Beyond biochemical signaling, physical cues including gravity, adhesion geometry, surface elasticity, adhesion force, and fluid shear stress all contribute to lineage commitment and differentiation (Zayzafoon et al., 2004; Engler et al., 2006; Kilian et al., 2010; Yourek et al., 2010; Mathieu and Loboa, 2012). For bone and cartilage regeneration, high yield of differentiated cells from hMSCs within a short time frame is preferred. Many groups have reported that microcarrier culture improves hMSC osteogenic and chondrogenic differentiation potential in vitro or in vivo (Tseng et al., 2012; Goh et al., 2013; Shekaran et al., 2015, 2016; Lin et al., 2016; Gupta et al., 2018, 2019). For example, microcarrier-expanded hMSCs were reported to have considerably increased pellet size and DNA content, as well as higher production of glycosaminoglycan and collagen II per pellet, after 28-days chondrogenic differentiation, compared to those from planar culture (Lin et al., 2016). The microcarrier-expanded hMSCs were also found to have increased osteogenic gene expression, alkaline phosphatase activity, calcium deposition, and collagen I secretion compared to the planar control (Tseng et al., 2012). The enhancement of osteogenesis may arise from increased cytoskeletal tension and actomyosin contraction of hMSCs on microcarriers, which can be inhibited by latrunculin B and blebbistatin (Tseng et al., 2012). By contrast, lower gene expression of adipocyte markers, such as PPARγ2 was observed in microcarrier culture, which demonstrates that microcarrier culture may down-regulate adipogenic differentiation potential (Tseng et al., 2012). Indeed, dynamic microcarrier culture system could alter hMSC commitment and differentiation compared to planar culture.
hMSCs demonstrate homing and migration ability to the injured or disordered tissues in vivo after administration. Thus, migratory capacity is an important indicator for hMSC-based therapies. While not many studies examined hMSC migratory ability after microcarrier expansion, some evidence of migratory ability changes can be found. It has been reported that microcarrier-expanded hMSCs have smaller size, and display higher CXCR4 expression in comparison with planer culture (Sun et al., 2010; Levato et al., 2015), suggesting that microcarrier-culture possibly enhanced hMSCs' migration ability. Further in vitro and in vivo examinations should be performed to elucidate this possibility.
hMSCs also play an important role in secreting growth factors, chemokines, and cytokines to maintain the physiological environment and attenuate immunogenicity in their original niche (Aggarwal and Pittenger, 2005; Ren et al., 2008; Liang et al., 2014). It has been reported that microcarrier-expanded fetal hMSCs secreted higher levels of IL-6 (an immunomodulatory cytokine), IL-8 (a pro-angiogenic chemokine), and CXCL5 (a chemokine) than planar culture-expanded cells (Shekaran et al., 2015). Also, changes in secretome of hMSCs cultured on microcarriers facilitated neuroregulatory function and differentiation of neural progenitor cells in vitro and in vivo as compared to those from planar culture (Teixeira et al., 2016). Specific proteins involved in the central nervous system, such as cystatin C, glia-derived nexin, galectin-1, and pigment epithelium-derived factor, were upregulated in microcarrier systems as well. Similarly, after tumor necrosis factor-α and interferon γ stimulation, microcarrier-expanded umbilical cord-derived hMSCs exhibited higher secretion of VEGF, interleukin 1 receptor antagonist (IL-1ra), and stromal cell-derived factor 1-alpha (SDF-1α) compared with flask-expanded hMSCs. Likewise, amniotic membrane-derived hMSCs cultured in microcarrier bioreactors secreted more VEGF, basic fibroblast growth factor, macrophage colony-stimulating factor (M-CSF), nerve growth factor, monocyte chemotactic protein-1 (MCP-1), and hepatocyte growth factor than flask-cultured hMSCs (Hupfeld et al., 2014). Upregulated secretion of brain-derived neurotrophic factor and insulin-like growth factor 1 was also reported in microcarrier systems (Teixeira et al., 2016). Furthermore, functional studies with immune cells under inflammation indicated that MSCs cultured on microcarrier exhibited improved IDO activity and thus maintained their immunomodulatory potentials characterized by inhibition of T cell proliferation (Lawson et al., 2017; Das et al., 2019). Taken together, microcarrier culture likely promotes hMSC's therapeutic potency in immunomodulation and angiogenesis by altering their secretome profiles.
The alteration of hMSC properties is most likely associated with the discernible differences in culture microenvironment between planar and microcarrier culture (Sart et al., 2013; Ma et al., 2016). This part is to elucidate the possible relationship between hMSC properties and bioprocessing parameters/microenvironment based on two factors: the adhesion surface and the hydrodynamics.
The primary adhesion surface change is the substrate topography which means cells colonize on individual disconnected microcarriers with a convex curvature. Other properties of adhesion substrate including rigidity, roughness, porousness, coating, charge, hydrophilicity, and wettability may also influence cellular microenvironment.
Upon inoculation, the microenvironment in microcarrier culture immediately changes compared to planar culture. Microcarriers provide abundant accessible surface per unit volume while lack of a bridge between individual microcarriers impedes cell migration from bead to bead. In planar culture, however, migration can compensate non-homogeneous cell distribution at a certain level. Migration on microcarriers is completely different as cells can only migrate within the single bead under dynamic environment. Thus, simply counting cell attachment efficiency is not informative without considering the percentage of microcarriers with attached cells. Generally, inoculation cell density is determined by the cell-to-bead ratio which needs to reach the threshold of critical cell number per microcarrier (Hu et al., 1985). In literature, a seeding density of 5 cells per microcarrier is commonly used for hMSCs (Rafiq et al., 2013a). Initial colonization of microcarriers by cells theoretically can be estimated by Poisson distribution (Frauenschuh et al., 2007), and the expected percentage of occupied microcarriers at a cell-to-bead ratio of 5 is 99.3%. Due to discontinuous surfaces, the homogeneous cell attachment on microcarriers and initial percentage of colonized microcarriers are critical for maintaining culture microenvironment and preventing uneven distribution of cells and mass transfer limitations.
Even if perfect homogeneous cell attachment on microcarriers can be achieved, the natural heterogeneity of hMSC populations still exists (Phinney, 2007). These heterogeneous characteristics including proliferation rate, metabolic activity, and contact limit would result in different confluence on each microcarrier at harvesting. Some cells on microcarriers may reach the stationary phase, while other cells are still in the lag phase or exponential phase. In addition, the heterogeneity of microcarriers also contributes to the heterogeneity of hMSCs. For example, SoloHill® plastic microcarriers formed by cross-linked polystyrene have the size range of 125–212 μm in diameter, and 1.7 times of difference in diameter would result in 2.9 times of difference in surface area. Also, therapeutic potency may vary for cells harvested at different confluency (Lam et al., 2019). Thus, the right timing for harvest should ensure that a majority of microcarriers are ready.
Geometric cues can regulate cell fate and differentiation commitment in planar culture via the changes of mechanical microenvironment, leading to a reorganization of cytoskeletons and formation of myosin-generated contractility (Chen et al., 2003; Discher et al., 2005; Kilian et al., 2010). These geometric cues include the dimension, shape, and curvature of the culture surface. In microcarrier culture, the accessible surface area provided by a single microcarrier with a diameter of 100–300 μm is in the range of 0.03–0.28 mm2, which is much larger than the area for a spreading single cell (Figure 3). Therefore, the curvature would be the dominant factor in topographic cues. The curvature of a surface is defined as the reciprocal of the radius of the sphere fitting to the camber (Xu et al., 2011; Ueki and Kidoaki, 2015). The curvature of a microcarrier is ranged from 1/50 to 1/150 μm−1, and the level of the curvature is inverse to the microcarrier size.
Figure 3. Effects of substrate curvature. Compared to cells cultured on the planar surface (Left), cells cultured on the convex surface (Middle and Right), like microcarriers, have a higher contact angle and thus under a higher mechanical force, against which cells develop more actin stress fibers, as a result of increased cytoskeletal tension. This trend shows more significant when the microcarriers are smaller.
To investigate the role of curvature, both microcarriers and planar surfaces should be made of the same materials with the same biophysical characteristics, and with cells cultured under the same flow condition. Indeed, it is difficult to isolate the effect of curvature during microcarrier suspension culture. Researchers have tried to use microprinting techniques to generate different geometric cues to address the influence of dimension and geometric shape on cytoskeleton distribution and mechanical contractility (Théry et al., 2006; James et al., 2008; Kilian et al., 2010; Théry, 2010), though it is still not the optimized model to mimic the underlying curvature on microcarriers. Recently, Werner et al. (2017) cultured hMSCs on the stereolithographic convex spherical structures with the curvature of 1/125, 1/175, 1/250, and 1/375 μm−1, which have the similar topography to semi-spheres, partially mimicking the curvature on microcarriers. The results reveal that cells on convex spherical structures have higher F-actin intensity and higher osteocalcin levels compared to cells on flat surfaces after 10-days expansion or osteogenic induction, and the enhancement becomes more significant when decreasing the convex diameters (Werner et al., 2017). The enhanced mechanical stress may be attributed to the push-force by the perinuclear actin cap to deform the nucleus (Werner et al., 2017). Based on the tensegrity theory, a numerical model was used by Vassaux to investigate the influence of biophysical environment on adherent cells on concave hemispheres (Ingber, 2003a). The results indicate that cells can experience higher mechanical microenvironment and become stiffer if the underlying substrate becomes more convex (Vassaux and Milan, 2017). Thus, higher underlying curvature from the smaller microcarrier size may contribute to higher mechanical stress and improved osteogenic differentiation. Another study was performed by culturing hMSCs on 4, 3, 2, 1.1 mm-, 900, 750, and 500 μm-diameter glass balls, which were half-embedded in polyacrylamide gels. hMSCs exhibited the increased gene expression of adipogenesis when cultured on the beads with the size in diameter equal or below 1.1 mm (Lee and Yang, 2017).
To measure cell contractility, cells were seeded on a microprinted surface covered by crowded micropillars. Through the direction and the level of localized deformation of the micropillar top, the contractile force can be calculated (Du Roure et al., 2005; Ghassemi et al., 2012; Trichet et al., 2012). To further understand the effect of the biophysical microenvironment in microcarrier culture, similar techniques should be developed and applied to the microcarrier-like curvature.
The biophysical cues, such as substrate elasticity, have been reported to influence hMSC lineage specification (Discher et al., 2005; Engler et al., 2006). hMSCs can be induced into osteogenic differentiation on a surface with the elasticity of 25–40 kPa, but into myogenic differentiation on a softer surface (8–17 kPa) and neurogenic differentiation on the softest surface (0.1–1 kPa). Consequent studies demonstrated that cells sense the stiffness of ECM via heterodimeric integrin receptors of α and β subunits through focal adhesions with involved proteins, including talin, paxillin, and vinculin (Liu et al., 2000). Followed by actin polymerization and elongation, the stress fibers are formed, which are induced by the RhoA-Rho-associated coiled-coil containing protein kinase (ROCK)- myosin light chain phosphatase (MLCP) signaling pathway responsible for cell skeletal tension along the edges (Galbraith et al., 2007). The integrin-mediated mechanotransducers, e.g., ROCK, activated by RhoA activity and influenced by cell adhesion and actin-myosin tension regulate hMSC lineage commitment through the mitogen activated protein kinase (MAPK)/the extracellular-signal-regulated kinase (ERK) and Yes-associated protein/transcriptional coactivator with PDZ-binding motif (TAZ) signaling pathways (McBeath et al., 2004; Bhadriraju et al., 2007; Dupont et al., 2011; Shih et al., 2011; Kim et al., 2014). The surface stiffness with the capacity of regulating hMSC differentiation is identified in planar culture, but whether the surface stiffness still has the same effects in microcarrier system, and whether it is possible to use the particular microcarrier stiffness to produce a specific lineage of hMSCs have not been well-investigated.
To date, the characteristics of microcarriers provided by the vendors usually only contain the information of size, composition, density, porosity, surface area, coating, and surface charge, but not the elasticity, rigidity, or stiffness. Most microcarriers are composed of a mixture of peptides or polymers, and the elasticity of these materials differs with the combination and concentration of molecules (i.e., degree of crosslinking) and the average length of polymers (i.e., degree of polymerization). For example, Lück et al. (2016) proposed a fabrication method of synthetic hydrogel microcarriers by telechelic poly(2-oxazoline) crosslinkers and methacrylate monomers, and the microcarrier rigidity can be regulated from 2 to 20 kPa in Young's modulus. Microcarrier rigidity can be measured by the high-resolution elasticity microscope (Cohn et al., 2000) with colloidal probe spectroscopy, an atomic force microscopy modified by gluing a micron-sized spherical force sensor to the end of cantilever (Kappl and Butt, 2002; Butt et al., 2005; Lück et al., 2016). To date, the influence of microcarrier rigidity on hMSC properties has not been well-investigated, possibly due to the confounded curvature effect on mechanical stress from the substrate. Hence, cells on microcarriers may not be as sensitive to the stiffness as they are on the flat surface.
The flow in microcarrier culture provides dynamic environment capable of resuspending microcarriers for homogeneous mixing and induces non-homogeneous shear stress and microcarrier-microcarrier collisions, all of which have critical impacts on the extracellular microenvironment of hMSCs.
In microcarrier culture, fluid flow plays a critical role in maintaining microcarriers in suspension and induces shear stress in culture microenvironment. Flush-mounted film probes (Dantec Dynamics, Germany) can be used to measure flow-induced shear stress, but have to be stuck on the wall (Kalmbach et al., 2011). Particle image velocimetry is able to generate comprehensive profiles of fluid shear stress and velocity in the spinner flasks (Ismadi et al., 2014). Computational fluid dynamics also offers an alternative approach to predict fluid shear stress (Jossen et al., 2016; Tsai et al., 2017). From these results, the maximum shear stress is 0.07–0.1 Pa at 25 rpm and 0.22–0.31 Pa at 60 rpm. Although only a small population of cells are exposed to the localized high shear stress and the volume-weighted mean of shear stress is very low (e.g., 0.0032 Pa at 25 rpm and 0.0067 Pa at 60 rpm), accumulated influence induced by shear stress is still significant on cell growth (Yuan et al., 2014; Jossen et al., 2016). Accordingly, alternative bioreactor designs have been developed to provide a low-shear stress environment, such as WAVE Bioreactor™ (GE Healthcare), BIOSTAT® CultiBag RM bioreactor (Sartorius), and Vertical-Wheel™ bioreactor (PBS Biotech) (Timmins et al., 2012; Sousa et al., 2015; Jossen et al., 2016; da Silva et al., 2019).
When flow-induced physical force directly acts on the cells, the cells may respond in several possible ways. For example, Ingber proposed a theory of tensegrity that a cell is structured by a hierarchical framework of cytoskeletons (Ingber, 2003a,b). Thus, the external forces acting at any point of the cell would be translated into the whole internal cell structure to revoke the force effect by cytoskeletal reorganization. Another possibility is that shear stress mechanically influences the integrins that connect ECM and cytoskeletons through the focal adhesion and the anchorage proteins to mechanotransduce the biomechanical stress into biochemical signals (Ingber, 2003b). Moreover, the cell membrane become more fluidized to change membrane composition and ion channels for the mechanotransduction pathways under elevated fluid shear stress (Barakat et al., 2006). These responses expedite hMSCs' commitment to osteogenic lineage by regulating opening and closing of membrane ion channels to increase intracellular Ca2+ concentration, and reorganizing cytoskeleton which activates focal adhesion kinase (FAK)/ERK1/2 pathways to trigger Runx2 and AP-1 and initiate the transcripted osteogenic differentiation (Liu et al., 2010). In addition to osteogenic differentiation, microcarrier culture has also been reported to improve chondrogenic differentiation (Lin et al., 2016). hMSCs undergo chondrogenic differentiation normally requiring cells clustering into spheroids that provide the unique mechanical microenvironment (Sart et al., 2014b; Tsai et al., 2015). Thus, shear stress in microcarrier culture reshapes the microenvironment for hMSCs to exhibit lineage specificity.
In bioreactors, microcarrier-impeller and microcarrier-microcarrier collisions occur frequently when mixing microcarriers in suspension. Microcarrier concentration and agitation speed determine the collision frequency and microcarrier kinetic energy, respectively.
Increased microcarrier concentration can increase the volumetric production at a lower cost, and higher cell culture density can induce more concentrated autocrine and paracrine factors which accelerate cell growth and facilitate cell function. However, high microcarrier concentration may promote collision frequency and damage cell growth. For example, addition of Sephadex G-50 beads (Pharmacia) was used to change the collision frequency. Severely decreased cell growth was observed at high collision frequency, which may detach cells from microcarriers (Croughan et al., 1988). Furthermore, increased microcarrier concentration normally requires frequent medium change and results in nutrient limitations or toxic by-product accumulation due to high cell density (Chen et al., 2015), which can be overcome by a perfusion culture system.
Agitation speed associated with microcarrier kinetic energy has been reported to influence hMSC expansion, and the optimal cell growth was observed at 49 rpm in a 100 mL spinner flask (Yuan et al., 2014; Jossen et al., 2016). Jossen et al. demonstrated that hMSC expansion significantly decreased when the agitation speed reached above 90 rpm (Jossen et al., 2016). Besides the shear stress effect, another possible explanation is the increased physical collisions due to the elevated kinetic energy and collision frequency (Yuan et al., 2014). The agitation speed of above 90 rpm may reach the threshold of microcarrier kinetic energy for initiating cell death (Jossen et al., 2016). The highest velocity at the tips of impeller can be calculated by agitation speed and impeller diameter (Ismadi et al., 2014; Odeleye et al., 2014). For example, in a 100 mL spinner flask with a standard impeller of 4 cm in diameter, the tip speed is 0.19 m/s at 90 rpm.
The accumulated collision impact likely leads to cell death and phenotypic alteration. Although the damage from collisions depends on the frequency and the intensity, it is difficult to isolate the shear stress effect. Moreover, cell concentration or cell coverage on microcarriers can also impact collision damage at the late stage of cultivation. Clusters bridged by cells may form due to the microcarrier-microcarrier collisions and non-proper mixing, especially when cells are confluent (Cherry and Papoutsakis, 1988; Caruso et al., 2014). Once multiple-microcarrier clusters are formed, cells tend to migrate to interstitial space between microcarriers, and multi-cellular aggregation has been well-known to promote cell differentiation, migration, and secretion (Sart et al., 2014b; Cesarz and Tamama, 2016; Zhang et al., 2016). Nevertheless, cells in the clusters may endure the limitation of mass transfer since diffusion is dominant over convection. Besides, shear stress randomly tears apart bridged microcarriers and may further provoke cell death (Cherry and Papoutsakis, 1988; Takahashi et al., 2017). Therefore, the microcarrier concentration and the agitation speed along with culture time need to be optimized to avoid severe collision damage and oversized clusters (Chen et al., 2015).
To expand hMSCs under cGMP compliant regulations, advanced biotechnologies have been developed, such as multi-layer vessels, fixed/packed bed bioreactors, hollow fiber bioreactors, and microcarrier suspension bioreactors. However, there is no guarantee that hMSC therapeutic potency is well-preserved, owing to the fundamental change in culture microenvironment. As a result, standardized characterization of manufactured hMSCs from different bioprocesses is necessary to certify their therapeutic potency for specific disease models. This article focuses on the microcarrier bioreactor systems and discusses the influences of bioprocessing parameters (e.g., agitation speed, heterogeneous shear stress exposure, microcarrier size, rigidity, adhesive force, coating charge, and cell-cell collision and aggregation) on the differentiation, migratory ability, and secretory function of manufactured hMSCs. Based on literature, microcarrier culture has been reported to enhance hMSC osteogenic and chondrogenic differentiation but impair their adipogenic differentiation compared to planar culture. Moreover, the improved migration and secretion abilities suggest microcarrier culture may augment hMSC therapeutic potency in immunomodulation, angiogenesis, and neural differentiation. Overall, microcarrier culture in bioreactors provides the possibility to scale up hMSC production and regulates hMSC therapeutic properties for clinical applications. Future studies should focus on improving the robustness of hMSC biomanufacturing system as well as engineering hMSCs with desired stem cell properties.
A-CT wrote the majority of the manuscript. RJ and XC revised the manuscript. XY and YL conceived the whole study, revised, and finalized the manuscript.
This work was supported by National Science Foundation of USA (CBET-1743426 and 1917618). This work was also partially supported by the National Institutes of Health (NIH; R01 NS102395). The content is solely the responsibility of the authors and does not necessarily represent the official views of the NIH.
The authors declare that the research was conducted in the absence of any commercial or financial relationships that could be construed as a potential conflict of interest.
Aggarwal, S., and Pittenger, M. F. (2005). Human mesenchymal stem cells modulate allogeneic immune cell responses. Blood 105, 1815–1822. doi: 10.1182/blood-2004-04-1559
Alge, D. L., Zhou, D., Adams, L. L., Wyss, B. K., Shadday, M. D., Woods, E. J., et al. (2010). Donor-matched comparison of dental pulp stem cells and bone marrow-derived mesenchymal stem cells in a rat model. J. Tissue Eng. Regen. Med. 4, 73–81. doi: 10.1002/term.220
Al-Nbaheen, M., Ali, D., Bouslimi, A., Al-Jassir, F., Megges, M., Prigione, A., et al. (2013). Human stromal (mesenchymal) stem cells from bone marrow, adipose tissue and skin exhibit differences in molecular phenotype and differentiation potential. Stem Cell Rev. Rep. 9, 32–43. doi: 10.1007/s12015-012-9365-8
António, M., Fernandes-Platzgummer, A., da Silva, C. L., and Cabral, J. M. (2016). Scalable microcarrier-based manufacturing of mesenchymal stem/stromal cells. J. Biotechnol. 236, 88–109. doi: 10.1016/j.jbiotec.2016.08.007
Atkinson, K., Timmins, N., Kiel, G., Heazlewood, C., Doran, M., and Brooke, G. (2017). Closed automated large-scale bioreactors for manufacturing mesenchymal stromal cells for clinical use. Biol. Ther. Appl. Mesenchymal Cells 2017, 616–618. doi: 10.1002/9781118907474.ch42
Badenes, S. M., Fernandes, T. G., Cordeiro, C. S., Boucher, S., Kuninger, D., Vemuri, M. C., et al. (2016b). Defined essential 8™ medium and vitronectin efficiently support scalable xeno-free expansion of human induced pluripotent stem cells in stirred microcarrier culture systems. PLoS ONE 11:e0151264. doi: 10.1371/journal.pone.0151264
Badenes, S. M., Fernandes, T. G., Rodrigues, C. A., Diogo, M. M., and Cabral, J. M. (2016a). Microcarrier-based platforms for in vitro expansion and differentiation of human pluripotent stem cells in bioreactor culture systems. J. Biotechnol. 234, 71–82. doi: 10.1016/j.jbiotec.2016.07.023
Bara, J. J., Richards, R. G., Alini, M., and Stoddart, M. J. (2014). Concise Review: Bone marrow-derived mesenchymal stem cells change phenotype following in vitro culture: implications for basic research and the clinic. Stem Cells 32, 1713–1723. doi: 10.1002/stem.1649
Barakat, A. I., Lieu, D. K., and Gojova, A. (2006). Secrets of the code: do vascular endothelial cells use ion channels to decipher complex flow signals? Biomaterials 27, 671–678. doi: 10.1016/j.biomaterials.2005.07.036
Barckhausen, C., Rice, B., Baila, S., Sensebé, L., Schrezenmeier, H., Nold, P., et al. (2016). GMP-compliant expansion of clinical-grade human mesenchymal stromal/stem cells using a closed hollow fiber bioreactor. Methods Mol. Biol. 416, 389–412. doi: 10.1007/978-1-4939-3584-0_23
Bhadriraju, K., Yang, M., Ruiz, S. A., Pirone, D., Tan, J., and Chen, C. S. (2007). Activation of ROCK by RhoA is regulated by cell adhesion, shape, and cytoskeletal tension. Exp. Cell Res. 313, 3616–3623. doi: 10.1016/j.yexcr.2007.07.002
Bianco, P., Cao, X., Frenette, P. S., Mao, J. J., Robey, P. G., Simmons, P. J., et al. (2013). The meaning, the sense and the significance: translating the science of mesenchymal stem cells into medicine. Nat. Med. 19, 35–42. doi: 10.1038/nm.3028
Billing, A. M., Hamidane, H. B., Dib, S. S., Cotton, R. J., Bhagwat, A. M., Kumar, P., et al. (2016). Comprehensive transcriptomic and proteomic characterization of human mesenchymal stem cells reveals source specific cellular markers. Sci. Rep. 6:21507. doi: 10.1038/srep21507
Bravery, C. A., Carmen, J., Fong, T., Oprea, W., Hoogendoorn, K. H., Woda, J., et al. (2013). Potency assay development for cellular therapy products: an ISCT review of the requirements and experiences in the industry. Cytotherapy 15, 9–19. doi: 10.1016/j.jcyt.2012.10.008
Butt, H. J., Cappella, B., and Kappl, M. (2005). Force measurements with the atomic force microscope: technique, interpretation and applications. Surf. Sci. Rep. 59, 1–152. doi: 10.1016/j.surfrep.2005.08.003
Caruso, S. R., Orellana, M. D., Mizukami, A., Fernandes, T. R., Fontes, A. M., Suazo, C. A., et al. (2014). Growth and functional harvesting of human mesenchymal stromal cells cultured on a microcarrier-based system. Biotechnol. Prog. 30, 889–895. doi: 10.1002/btpr.1886
Castilla-Casadiego, D. A., Reyes-Ramos, A. M., Domenech, M., and Almodovar, J. (2020). Effects of physical, chemical, and biological stimulus on h-MSC expansion and their functional characteristics. Ann. Biomed. Eng. 48, 519–535. doi: 10.1007/s10439-019-02400-3
Cesarz, Z., and Tamama, K. (2016). Spheroid culture of mesenchymal stem cells. Stem Cells Int. 2016:9176357. doi: 10.1155/2016/9176357
Chen, A. K. L., Chew, Y. K., Tan, H. Y., Reuveny, S., and Oh, S. K. W. (2015). Increasing efficiency of human mesenchymal stromal cell culture by optimization of microcarrier concentration and design of medium feed. Cytotherapy 17, 163–173. doi: 10.1016/j.jcyt.2014.08.011
Chen, A. K. L., Reuveny, S., and Oh, S. K. W. (2013). Application of human mesenchymal and pluripotent stem cell microcarrier cultures in cellular therapy: achievements and future direction. Biotechnol. Adv. 31, 1032–1046. doi: 10.1016/j.biotechadv.2013.03.006
Chen, C. S., Alonso, J. L., Ostuni, E., Whitesides, G. M., and Ingber, D. E. (2003). Cell shape provides global control of focal adhesion assembly. Biochem. Biophys. Res. Commun. 307, 355–361. doi: 10.1016/S0006-291X(03)01165-3
Cherry, R. S., and Papoutsakis, E. T. (1988). Physical mechanisms of cell damage in microcarrier cell culture bioreactors. Biotechnol. Bioeng. 32, 1001–1014. doi: 10.1002/bit.260320808
Chui, C. Y., Odeleye, A., Nguyen, L., Kasoju, N., Soliman, E., and Ye, H. (2019). Electrosprayed genipin cross-linked alginate–chitosan microcarriers for ex vivo expansion of mesenchymal stem cells. J. Biomed. Mater. Res. Part A 107, 122–133. doi: 10.1002/jbm.a.36539
Cohn, N. A., Kim, B. S., Erkamp, R. Q., Mooney, D. J., Emelianov, S. Y., Skovoroda, A. R., et al. (2000). High-resolution elasticity imaging for tissue engineering. IEEE Trans. Ultrason. Ferroelectr. Freq. Control 47, 956–966. doi: 10.1109/58.852079
Collignon, M. L., Delafosse, A., Calvo, S., Martin, C., Marc, A., Toye, D., et al. (2016). Large-Eddy simulations of microcarrier exposure to potentially damaging eddies inside mini-bioreactors. Biochem. Eng. J. 108, 30–43. doi: 10.1016/j.bej.2015.10.020
Confalonieri, D., La Marca, M., van Dongen, E. M. W. M., Walles, H., and Ehlicke, F. (2017). An injectable recombinant collagen I peptide–based macroporous microcarrier allows superior expansion of C2C12 and human bone marrow-derived mesenchymal stromal cells and supports deposition of mineralized matrix. Tissue Eng. Part A 23, 946–957. doi: 10.1089/ten.tea.2016.0436
Croughan, M. S., Hamel, J. F. P., and Wang, D. I. (1988). Effects of microcarrier concentration in animal cell culture. Biotechnol. Bioeng. 32, 975–982. doi: 10.1002/bit.260320805
Cunha, B., Aguiar, T., Carvalho, S. B., Silva, M. M., Gomes, R. A., Carrondo, M. J., et al. (2017). Bioprocess integration for human mesenchymal stem cells: from up to downstream processing scale-up to cell proteome characterization. J. Biotechnol. 248, 87–98. doi: 10.1016/j.jbiotec.2017.01.014
da Silva, J. D. S., Mizukami, A., Gil, L. V. G., de Campos, J. V., Assis, O. B., Covas, D. T., et al. (2019). Improving wave-induced motion bioreactor performance for human mesenchymal stromal cell expansion. Process Biochem. 84, 143–152. doi: 10.1016/j.procbio.2019.06.004
Das, R., Roosloot, R., van Pel, M., Schepers, K., Driessen, M., Fibbe, W. E., et al. (2019). Preparing for cell culture scale-out: establishing parity of bioreactor-and flask-expanded mesenchymal stromal cell cultures. J. Transl. Med. 17:241. doi: 10.1186/s12967-019-1989-x
Demuth, C., Varonier, J., Jossen, V., Eibl, R., and Eibl, D. (2016). Novel probes for pH and dissolved oxygen measurements in cultivations from millilitre to benchtop scale. Appl. Microbiol. Biotechnol. 100, 3853–3863. doi: 10.1007/s00253-016-7412-0
Discher, D. E., Janmey, P., and Wang, Y. (2005). Tissue cells feel and respond to the stiffness of their substrate. Science 310, 1139–1143. doi: 10.1126/science.1116995
Dominici, M., Le Blanc, K., Mueller, I., Slaper-Cortenbach, I., Marini, F., Krause, D., et al. (2006). Minimal criteria for defining multipotent mesenchymal stromal cells. The International Society for Cellular Therapy position statement. Cytotherapy 8, 315–317. doi: 10.1080/14653240600855905
dos Santos, F., Andrade, P. Z., Abecasis, M. M., Gimble, J. M., Chase, L. G., Campbell, A. M., et al. (2011). Toward a clinical-grade expansion of mesenchymal stem cells from human sources: a microcarrier-based culture system under xeno-free conditions. Tissue Eng. Part C Methods 17, 1201–1210. doi: 10.1089/ten.tec.2011.0255
dos Santos, F., Andrade, P. Z., da Silva, C. L., and Cabral, J. M. (2013). Bioreactor design for clinical-grade expansion of stem cells. Biotechnol. J. 8, 644–654. doi: 10.1002/biot.201200373
dos Santos, F., Campbell, A., Fernandes-Platzgummer, A., Andrade, P. Z., Gimble, J. M., Wen, Y., et al. (2014). A xenogeneic-free bioreactor system for the clinical-scale expansion of human mesenchymal stem/stromal cells. Biotechnol. Bioeng. 111, 1116–1127. doi: 10.1002/bit.25187
Du Roure, O., Saez, A., Buguin, A., Austin, R. H., Chavrier, P., Siberzan, P., et al. (2005). Force mapping in epithelial cell migration. Proc. Natl. Acad. Sci. U.S.A. 102, 2390–2395. doi: 10.1073/pnas.0408482102
Duijvestein, M., Vos, A. C. W., Roelofs, H., Wildenberg, M. E., Wendrich, B. B., Verspaget, H. W., et al. (2010). Autologous bone marrow-derived mesenchymal stromal cell treatment for refractory luminal Crohn's disease: results of a phase I study. Gut 59, 1662–1669. doi: 10.1136/gut.2010.215152
Dupont, S., Morsut, L., Aragona, M., Enzo, E., Giulitti, S., Cordenonsi, M., et al. (2011). Role of YAP/TAZ in mechanotransduction. Nature 474, 179–183. doi: 10.1038/nature10137
Eibes, G., dos Santos, F., Andrade, P. Z., Boura, J. S., Abecasis, M. M., da Silva, C. L., et al. (2010). Maximizing the ex vivo expansion of human mesenchymal stem cells using a microcarrier-based stirred culture system. J. Biotechnol. 146, 194–197. doi: 10.1016/j.jbiotec.2010.02.015
Engler, A. J., Sen, S., Sweeney, H. L., and Discher, D. E. (2006). Matrix elasticity directs stem cell lineage specification. Cell 126, 677–689. doi: 10.1016/j.cell.2006.06.044
Fernandes-Platzgummer, A., Carmelo, J. G., da Silva, C. L., and Cabral, J. M. (2016). Clinical-grade manufacturing of therapeutic human mesenchymal stem/stromal cells in microcarrier-based culture systems. Methods Mol. Biol. 1416, 375–388. doi: 10.1007/978-1-4939-3584-0_22
Fraser, J. K., Schreiber, R., Strem, B., Zhu, M., Alfonso, Z., Wulur, I., et al. (2006). Plasticity of human adipose stem cells toward endothelial cells and cardiomyocytes. Nat. Clin. Pract. Cardiovasc. Med. 3, S33–S37. doi: 10.1038/ncpcardio0444
Frauenschuh, S., Reichmann, E., Ibold, Y., Goetz, P. M., Sittinger, M., and Ringe, J. (2007). A microcarrier-based cultivation system for expansion of primary mesenchymal stem cells. Biotechnol. Prog. 23, 187–193. doi: 10.1021/bp060155w
Galbraith, C. G., Yamada, K. M., and Galbraith, J. A. (2007). Polymerizing actin fibers position integrins primed to probe for adhesion sites. Science 315, 992–995. doi: 10.1126/science.1137904
Galipeau, J., Krampera, M., Barrett, J., Dazzi, F., Deans, R. J., DeBruijn, J., et al. (2016). International Society for Cellular Therapy perspective on immune functional assays for mesenchymal stromal cells as potency release criterion for advanced phase clinical trials. Cytotherapy 18, 151–159. doi: 10.1016/j.jcyt.2015.11.008
Ghassemi, S., Meacci, G., Liu, S., Gondarenko, A. A., Mathur, A., Roca-Cusachs, P., et al. (2012). Cells test substrate rigidity by local contractions on submicrometer pillars. Proc. Natl. Acad. Sci. U.S.A. 109, 5328–5333. doi: 10.1073/pnas.1119886109
Goh, T. K. P., Zhang, Z. Y., Chen, A. K. L., Reuveny, S., Choolani, M., Chan, J. K. Y., et al. (2013). Microcarrier culture for efficient expansion and osteogenic differentiation of human fetal mesenchymal stem cells. BioRes. Open Access 2, 84–97. doi: 10.1089/biores.2013.0001
Grayson, W. L., and Stephenson, M. (2018). Recent advances in bioreactors for cell-based therapies. F1000Res. 7:517. doi: 10.12688/f1000research.12533.1
Gupta, P., Geris, L., Luyten, F. P., and Papantoniou, I. (2018). An integrated bioprocess for the expansion and chondrogenic priming of human periosteum-derived progenitor cells in suspension bioreactors. Biotechnol. J. 13:e1700087. doi: 10.1002/biot.201700087
Gupta, P., Hall, G. N., Geris, L., Luyten, F. P., and Papantoniou, I. (2019). Human platelet lysate improves bone forming potential of human progenitor cells expanded in microcarrier-based dynamic culture. Stem Cells Transl. Med. 8, 810–821. doi: 10.1002/sctm.18-0216
Hanley, P. J., Mei, Z., Durett, A. G. M, da Graca Cabreira-Harrison Klis, M., Li, W., et al. (2014). Efficient manufacturing of therapeutic mesenchymal stromal cells with the use of the Quantum Cell Expansion System. Cytotherapy 16, 1048–1058. doi: 10.1016/j.jcyt.2014.01.417
Heathman, T. R., Glyn, V. A., Picken, A., Rafiq, Q. A., Coopman, K., Nienow, A. W., et al. (2015). Expansion, harvest and cryopreservation of human mesenchymal stem cells in a serum-free microcarrier process. Biotechnol. Bioeng. 112, 1696–1707. doi: 10.1002/bit.25582
Hernandez, R. (2016). Scale-up of complex biologics. BioPharm. Int. 29, 26–30. doi: 10.1111/tri.12886
Hervy, M., Weber, J. L., Pecheul, M., Dolley-Sonneville, P., Henry, D., Zhou, Y., et al. (2014). Long term expansion of bone marrow-derived hMSCs on novel synthetic microcarriers in xeno-free, defined conditions. PLoS ONE 9:e92120. doi: 10.1371/journal.pone.0092120
Hewitt, C. J., Lee, K., Nienow, A. W., Thomas, R. J., Smith, M., and Thomas, C. R. (2011). Expansion of human mesenchymal stem cells on microcarriers. Biotechnol. Lett. 33, 2325–2335. doi: 10.1007/s10529-011-0695-4
Honmou, O., Onodera, R., Sasaki, M., Waxman, S. G., and Kocsis, J. D. (2012). Mesenchymal stem cells: therapeutic outlook for stroke. Trends Mol. Med. 18, 292–297. doi: 10.1016/j.molmed.2012.02.003
Hoshan, L., Jiang, R., Moroney, J., Bui, A., Zhang, X., Hang, T. C., et al. (2019). Effective bioreactor pH control using only sparging gases. Biotechnol. Prog. 35:e2743. doi: 10.1002/btpr.2743
Hourd, P., Chandra, A., Medcalf, N., and Williams, D. J. (2014). Regulatory Challenges for the Manufacture and Scale-Out of Autologous Cell Therapies, StemBook. Cambridge, MA: Harvard Stem Cell Institute.
Hu, W., Meier, J., and Wang, D. (1985). A mechanistic analysis of the inoculum requirement for the cultivation of mammalian cells on microcarriers. Biotechnol. Bioeng. 27, 585–595. doi: 10.1002/bit.260270507
Hupfeld, J., Gorr, I. H., Schwald, C., Beaucamp, N., Wiechmann, K., Kuentzer, K., et al. (2014). Modulation of mesenchymal stromal cell characteristics by microcarrier culture in bioreactors. Biotechnol. Bioeng. 111, 2290–2302. doi: 10.1002/bit.25281
Ingber, D. E. (2003a). Tensegrity I. Cell structure and hierarchical systems biology. J. Cell Sci. 116, 1157–1173. doi: 10.1242/jcs.00359
Ingber, D. E. (2003b). Tensegrity II. How structural networks influence cellular information processing networks. J. Cell Sci. 116, 1397–1408. doi: 10.1242/jcs.00360
Ismadi, M. Z., Hourigan, K., and Fouras, A. (2014). Experimental characterisation of fluid mechanics in a spinner flask bioreactor. Processes 2, 753–772. doi: 10.3390/pr2040753
Isobe, Y., Koyama, N., Nakao, K., Osawa, K., Ikeno, M., Yamanaka, S., et al. (2016). Comparison of human mesenchymal stem cells derived from bone marrow, synovial fluid, adult dental pulp, and exfoliated deciduous tooth pulp. Int. J. Oral Maxillofac. Surg. 45, 124–131. doi: 10.1016/j.ijom.2015.06.022
James, J., Goluch, E. D., Hu, H., Liu, C., and Mrksich, M. (2008). Subcellular curvature at the perimeter of micropatterned cells influences lamellipodial distribution and cell polarity. Cell Motil. Cytoskeleton 65, 841–852. doi: 10.1002/cm.20305
Jossen, V.C, van den Bos Eibl, R., and Eibl, D. (2018). Manufacturing human mesenchymal stem cells at clinical scale: process and regulatory challenges. Appl. Microbiol. Biotechnol. 102, 3981–3994. doi: 10.1007/s00253-018-8912-x
Jossen, V., Eibl, R., Kaiser, S. C., Kraume, M., Pörtner, R., and Eibl, D. (2014). Mass production of mesenchymal stem cells: impact of bioreactor design and flow conditions on proliferation and differentiation. Cells Biomater. Regen. Med. 2014, 119–172. doi: 10.5772/59385
Jossen, V., Schirmer, C., Mostafa Sindi, D., Eibl, R., Kraume, M., Pörtner, R., et al. (2016). Theoretical and practical issues that are relevant when scaling up hMSC microcarrier production processes. Stem Cells Intl. 2016:4760414. doi: 10.1155/2016/4760414
Kaiser, S., Jossen, V., Schirmaier, C., Eibl, D., Brill, S. C., et al. (2013). Fluid Flow and Cell Proliferation of mesenchymal adipose-derived stem cells in small-scale, stirred, single-use bioreactors. Chem. Ingen. Tech. 85, 95–102. doi: 10.1002/cite.201200180
Kalmbach, A., Bordás, R., Öncül, A. A., Thévenin, D., Genzel, Y., and Reichl, U. (2011). Experimental characterization of flow conditions in 2-and 20-l bioreactors with wave-induced motion. Biotechnol. Prog. 27, 402–409. doi: 10.1002/btpr.516
Kang, H., Lu, S., Peng, J., Yang, Q., Liu, S., Zhang, L., et al. (2015). Chondrogenic differentiation of human adipose-derived stem cells using microcarrier and bioreactor combination technique. Mol. Med. Rep. 11, 1195–1199. doi: 10.3892/mmr.2014.2820
Kappl, M., and Butt, H. J. (2002). The colloidal probe technique and its application to adhesion force measurements. Part Part Syst. Charact. 19, 129–143. doi: 10.1002/1521-4117(200207)19:3<129::AID-PPSC129>3.0.CO;2-G
Kehoe, D., Niss, K., Rook, M., Punreddy, S., Murrel, J., Sunil, N., et al. (2013). Growth kinetics of human mesenchymal stem cells in a 3-L single-use, stirred-tank bioreactor. BioPharm. Int. 26, 28–38.
Kilian, K. A., Bugarija, B., Lahn, B. T., and Mrksich, M. (2010). Geometric cues for directing the differentiation of mesenchymal stem cells. Proc. Natl. Acad. Sci. U.S.A. 107, 4872–4877. doi: 10.1073/pnas.0903269107
Kim, K. M., Choi, Y. J., Hwang, J. H., Kim, A. R., Cho, H. J., Hwang, E. S., et al. (2014). Shear stress induced by an interstitial level of slow flow increases the osteogenic differentiation of mesenchymal stem cells through TAZ activation. PLoS ONE 9:e92427. doi: 10.1371/journal.pone.0092427
Knippenberg, M., Helder, M. N., Zandieh Doulabi, B., Semeins, C. M., Wuisman, P. I., and Klein-Nulend, J. (2005). Adipose tissue-derived mesenchymal stem cells acquire bone cell-like responsiveness to fluid shear stress on osteogenic stimulation. Tissue Eng. 11, 1780–1788. doi: 10.1089/ten.2005.11.1780
Koniusz, S., Andrzejewska, A., Muraca, M., Srivastava, A. K., Janowski, M., and Lukomska, B. (2016). Extracellular vesicles in physiology, pathology, and therapy of the immune and central nervous system, with focus on extracellular vesicles derived from mesenchymal stem cells as therapeutic tools. Front. Cell Neurosci. 10:109. doi: 10.3389/fncel.2016.00109
Krampera, M., Galipeau, J., Shi, Y., Tarte, K., and Sensebe, L. (2013). Immunological characterization of multipotent mesenchymal stromal cells—The International Society for Cellular Therapy (ISCT) working proposal. Cytotherapy 15, 1054–1061. doi: 10.1016/j.jcyt.2013.02.010
Krutty, J. D., Dias, A. D., Yun, J., Murphy, W. L., and Gopalan, P. (2019). Synthetic, chemically defined polymer-coated microcarriers for the expansion of human mesenchymal stem cells. Macromol. Biosci. 19:1800299. doi: 10.1002/mabi.201800299
Lam, A. T. L., Sim, E. J. H., Shekaran, A., Li, J., Teo, K. L., Goggi, J. L., et al. (2019). Sub-confluent culture of human mesenchymal stromal cells on biodegradable polycaprolactone microcarriers enhances bone healing of rat calvarial defect. Cytotherapy 21, 631–642. doi: 10.1016/j.jcyt.2019.03.004
Lawson, T., Kehoe, D. E., Schnitzler, A. C., Rapiejko, P. J., Der, K. A., Philbrick, K., et al. (2017). Process development for expansion of human mesenchymal stromal cells in a 50L single-use stirred tank bioreactor. Biochem. Eng. J. 120, 49–62. doi: 10.1016/j.bej.2016.11.020
Leber, J., Barekzai, J., Blumenstock, M., Pospisil, B., Salzig, D., and Czermak, P. (2017). Microcarrier choice and bead-to-bead transfer for human mesenchymal stem cells in serum-containing and chemically defined media. Process Biochem. 59, 255–265. doi: 10.1016/j.procbio.2017.03.017
Lee, S. J., and Yang, S. (2017). Substrate curvature restricts spreading and induces differentiation of human mesenchymal stem cells. Biotechnol. J. 12:1700360. doi: 10.1002/biot.201700360
Levato, R., Planell, J. A., Mateos-Timoneda, M. A., and Engel, E. (2015). Role of ECM/peptide coatings on SDF-1α triggered mesenchymal stromal cell migration from microcarriers for cell therapy. Acta Biomater. 18, 59–67. doi: 10.1016/j.actbio.2015.02.008
Levinson, Y., Eylon, Y., Heymann, A., and Zaretsky-Rits, A. (2015). Foundation elements for cell therapy smart scaling. Bioprocess. Int. 13, 10–19.
Li, C., Wu, X., Tong, J., Yang, X., Zhao, J., Zheng, Q., et al. (2015). Comparative analysis of human mesenchymal stem cells from bone marrow and adipose tissue under xeno-free conditions for cell therapy. Stem Cell Res. Ther. 6:55. doi: 10.1186/s13287-015-0066-5
Li, Z., Yao, S. J., Alini, M., and Stoddart, M. J. (2009). Chondrogenesis of human bone marrow mesenchymal stem cells in fibrin–polyurethane composites is modulated by frequency and amplitude of dynamic compression and shear stress. Tissue Eng. Part A 16, 575–584. doi: 10.1089/ten.tea.2009.0262
Liang, X., Ding, Y., Zhang, Y., Tse, H. F., and Lian, Q. (2014). Paracrine mechanisms of mesenchymal stem cell-based therapy: current status and perspectives. Cell Transplant. 23, 1045–1059. doi: 10.3727/096368913X667709
Lin, C. Y., Huang, C. H., Wu, Y. K., Cheng, N. C., and Yu, J. (2014). Maintenance of human adipose derived stem cell (hASC) differentiation capabilities using a 3D culture. Biotechnol. Lett. 36, 1529–1537. doi: 10.1007/s10529-014-1500-y
Lin, Y. M., Lim, J. F. Y., Lee, J., Choolani, M., Chan, J. K. Y., Reuveny, S., et al. (2016). Expansion in microcarrier-spinner cultures improves the chondrogenic potential of human early mesenchymal stromal cells. Cytotherapy 18, 740–753. doi: 10.1016/j.jcyt.2016.03.293
Liu, L., Yuan, W., and Wang, J. (2010). Mechanisms for osteogenic differentiation of human mesenchymal stem cells induced by fluid shear stress. Biomech. Model. Mechanobiol. 9, 659–670. doi: 10.1007/s10237-010-0206-x
Liu, S., Calderwood, D. A., and Ginsberg, M. H. (2000). Integrin cytoplasmic domain-binding proteins. J. Cell Sci. 113, 3563–3571.
Loubière, C., Sion, C., De Isla, N., Reppel, L., Guedon, E., Chevalot, I., et al. (2019). Impact of the type of microcarrier and agitation modes on the expansion performances of mesenchymal stem cells derived from umbilical cord. Biotechnol. Prog. 35:e2887. doi: 10.1002/btpr.2887
Lück, S., Schubel, R., Rüb, J., Hahn, D., Mathieu, E., Zimmermann, H., et al. (2016). Tailored and biodegradable poly (2-oxazoline) microbeads as 3D matrices for stem cell culture in regenerative therapies. Biomaterials 79, 1–14. doi: 10.1016/j.biomaterials.2015.11.045
Ma, T., Tsai, A. C., and Liu, Y. (2016). Biomanufacturing of human mesenchymal stem cells in cell therapy: influence of microenvironment on scalable expansion in bioreactors. Biochem. Eng. J. 108, 44–50. doi: 10.1016/j.bej.2015.07.014
Marquez-Curtis, L. A., Janowska-Wieczorek, A., McGann, L. E., and Elliott, J. A. (2015). Mesenchymal stromal cells derived from various tissues: biological, clinical and cryopreservation aspects. Cryobiology 71, 181–197. doi: 10.1016/j.cryobiol.2015.07.003
Martin, C., Olmos, É., Collignon, M. L., De Isla, N., Blanchard, F., Chevalot, I., et al. (2017). Revisiting MSC expansion from critical quality attributes to critical culture process parameters. Process Biochem. 59, 231–243. doi: 10.1016/j.procbio.2016.04.017
Martin-Manso, G., and Hanley, P. J. (2015). Using the quantum cell expansion system for the automated expansion of clinical-grade bone marrow-derived human mesenchymal stromal cells, Methods Mol. Biol. 1283, 53–63. doi: 10.1007/7651_2014_164
Mathieu, P. S., and Loboa, E. G. (2012). Cytoskeletal and focal adhesion influences on mesenchymal stem cell shape, mechanical properties, and differentiation down osteogenic, adipogenic, and chondrogenic pathways. Tissue Eng. Part B Rev. 18, 436–444. doi: 10.1089/ten.teb.2012.0014
McBeath, R., Pirone, D. M., Nelson, C. M., Bhadriraju, K., and Chen, C. S. (2004). Cell shape, cytoskeletal tension, and RhoA regulate stem cell lineage commitment. Dev. Cell 6, 483–495. doi: 10.1016/S1534-5807(04)00075-9
McGrath, M., Tam, E., Sladkova, M., AlManaie, A., Zimmer, M., and de Peppo, G. M. (2019). GMP-compatible and xeno-free cultivation of mesenchymal progenitors derived from human-induced pluripotent stem cells. Stem Cell Res. Ther. 10, 1–13. doi: 10.1186/s13287-018-1119-3
Menard, C., Pacelli, L., Bassi, G., Dulong, J., Bifari, F., Bezier, I., et al. (2013). Clinical-grade mesenchymal stromal cells produced under various good manufacturing practice processes differ in their immunomodulatory properties: standardization of immune quality controls. Stem Cells Dev. 22, 1789–1801. doi: 10.1089/scd.2012.0594
Meuwly, F., Ruffieux, P. A., Kadouri, A., and Von Stockar, U. (2007). Packed-bed bioreactors for mammalian cell culture: bioprocess and biomedical applications. Biotechnol. Adv. 25, 45–56. doi: 10.1016/j.biotechadv.2006.08.004
Mirro, R., and Voll, K. (2009). Which impeller is right for your cell line. BioProcess. Int. 7, 52–57.
Mizukami, A., Chilima, T. D. P., Orellana, M. D., Neto, M. A., Covas, D. T., Farid, S. S., et al. (2018). Technologies for large-scale umbilical cord-derived MSC expansion: experimental performance and cost of goods analysis. Biochem. Eng. J. 135, 36–48. doi: 10.1016/j.bej.2018.02.018
Moloudi, R., Oh, S., Yang, C., Teo, K. L., Lam, A. T. L., Ebrahimi Warkiani, M., et al. (2019). Scaled-up inertial microfluidics: retention system for microcarrier-based suspension cultures. Biotechnol. J. 14:e1800674. doi: 10.1002/biot.201800674
Moreira, F., Mizukami, A., de Souza, L. E. B., Cabral, J. M., da Silva, C. L., Covas, D. T., et al. (2020). Successful use of human AB serum to support the expansion of adipose tissue-derived mesenchymal sem/stromal cell in a microcarrier-based platform. Front. Bioeng. Biotechnol. 8:307. doi: 10.3389/fbioe.2020.00307
Moutsatsou, P., Ochs, J., Schmitt, R., Hewitt, C., and Hanga, M. (2019). Automation in cell and gene therapy manufacturing: from past to future. Biotechnol. Lett. 41, 1245–1253. doi: 10.1007/s10529-019-02732-z
Newman, R. E., Yoo, D., LeRoux, M. A., and Danilkovitch-Miagkova, A. (2009). Treatment of inflammatory diseases with mesenchymal stem cells. Inflamm. Allergy Drug Targets 8, 110–123. doi: 10.2174/187152809788462635
Nienow, A. W. (2006). Reactor engineering in large scale animal cell culture. Cytotechnology 50, 9–33. doi: 10.1007/s10616-006-9005-8
Nienow, A. W., Hewitt, C. J., Heathman, T. R., Glyn, V. A., Fonte, G. N., Hanga, M. P., et al. (2016). Agitation conditions for the culture and detachment of hMSCs from microcarriers in multiple bioreactor platforms. Biochem. Eng. J. 108, 24–29. doi: 10.1016/j.bej.2015.08.003
Nienow, A. W., Rafiq, Q. A., Coopman, K., and Hewitt, C. J. (2014). A potentially scalable method for the harvesting of hMSCs from microcarriers. Biochem. Eng. J. 85, 79–88. doi: 10.1016/j.bej.2014.02.005
Nold, P., Brendel, C., Neubauer, A., Bein, G., and Hackstein, H. (2013). Good manufacturing practice-compliant animal-free expansion of human bone marrow derived mesenchymal stroma cells in a closed hollow-fiber-based bioreactor. Biochem. Biophys. Res. Commun. 430, 325–330. doi: 10.1016/j.bbrc.2012.11.001
Odeleye, A., Marsh, D., Osborne, M., Lye, G., and Micheletti, M. (2014). On the fluid dynamics of a laboratory scale single-use stirred bioreactor. Chem. Eng. Sci. 111, 299–312. doi: 10.1016/j.ces.2014.02.032
Olsen, T. R., Ng, K. S., Lock, L. T., Ahsan, T., and Rowley, J. A. (2018). Peak MSC—are we there yet? Front. Med. 5:178. doi: 10.3389/fmed.2018.00178
Osiecki, M. J., Michl, T. D., Babur, B. K., Kabiri, M., Atkinson, K., Lott, W. B., et al. (2015). Packed bed bioreactor for the isolation and expansion of placental-derived mesenchymal sromal cells. PLoS ONE 10:e0144941. doi: 10.1371/journal.pone.0144941
Panchalingam, K. M., Jung, S., Rosenberg, L., and Behie, L. A. (2015). Bioprocessing strategies for the large-scale production of human mesenchymal stem cells: a review. Stem Cell Res. Ther. 6:225. doi: 10.1186/s13287-015-0228-5
Park, J. H., Pérez, R. A., Jin, G. Z., Choi, S. J., Kim, H. W., and Wall, I. B. (2013). Microcarriers designed for cell culture and tissue engineering of bone. Tissue Eng. Part B Rev. 19, 172–190. doi: 10.1089/ten.teb.2012.0432
Petry, F., Smith, J. R., Leber, J., Salzig, D., Czermak, P., and Weiss, M. L. (2016). Manufacturing of human umbilical cord mesenchymal stromal cells on microcarriers in a dynamic system for clinical use. Stem Cells Int. 2016:4834616. doi: 10.1155/2016/4834616
Phinney, D. G. (2007). Biochemical heterogeneity of mesenchymal stem cell populations: clues to their therapeutic efficacy. Cell Cycle 6, 2884–2889. doi: 10.4161/cc.6.23.5095
Phinney, D. G., Di Giuseppe, M., Njah, J., Sala, E., Shiva, S., St. Croix, C. M., et al. (2015). Mesenchymal stem cells use extracellular vesicles to outsource mitophagy and shuttle microRNAs. Nat Commun. 6:8472. doi: 10.1038/ncomms9472
Pigeau, G. M., Csaszar, E., and Dulgar-Tulloch, A. (2018). Commercial scale manufacturing of allogeneic cell therapy. Front. Med. 5:233. doi: 10.3389/fmed.2018.00233
Pittenger, M. F., Mackay, A. M., Beck, S. C., Jaiswal, R. K., Douglas, R., Mosca, J. D., et al. (1999). Multilineage potential of adult human mesenchymal stem cells. Science 284, 143–147. doi: 10.1126/science.284.5411.143
Pittenger, M. F., and Martin, B. J. (2004). Mesenchymal stem cells and their potential as cardiac therapeutics. Circ. Res. 95, 9–20. doi: 10.1161/01.RES.0000135902.99383.6f
Prockop, D. J., Kota, D. J., Bazhanov, N., and Reger, R. L. (2010). Evolving paradigms for repair of tissues by adult stem/progenitor cells (MSCs). J. Cell. Mol. Med. 14, 2190–2199. doi: 10.1111/j.1582-4934.2010.01151.x
Rafiq, Q. A., Brosnan, K. M., Coopman, K., Nienow, A. W., and Hewitt, C. J. (2013a). Culture of human mesenchymal stem cells on microcarriers in a 5 l stirred-tank bioreactor. Biotechnol. Lett. 35, 1233–1245. doi: 10.1007/s10529-013-1211-9
Rafiq, Q. A., Coopman, K., and Hewitt, C. J. (2013b). Scale-up of human mesenchymal stem cell culture: current technologies and future challenges. Curr. Opin. Chem. Eng. 2, 8–16. doi: 10.1016/j.coche.2013.01.005
Rafiq, Q. A., Coopman, K., Nienow, A. W., and Hewitt, C. J. (2016). Systematic microcarrier screening and agitated culture conditions improves human mesenchymal stem cell yield in bioreactors. Biotechnol. J. 11, 473–486. doi: 10.1002/biot.201400862
Rafiq, Q. A., Ruck, S., Hanga, M. P., Heathman, T. R., Coopman, K., Nienow, A. W., et al. (2018). Qualitative and quantitative demonstration of bead-to-bead transfer with bone marrow-derived human mesenchymal stem cells on microcarriers: utilising the phenomenon to improve culture performance. Biochem. Eng. J. 135, 11–21. doi: 10.1016/j.bej.2017.11.005
Ren, G., Zhang, L., Zhao, X., Xu, G., Zhang, Y., Roberts, A. I., et al. (2008). Mesenchymal stem cell-mediated immunosuppression occurs via concerted action of chemokines and nitric oxide. Cell Stem Cell 2, 141–150. doi: 10.1016/j.stem.2007.11.014
Robb, K. P., Fitzgerald, J. C., Barry, F., and Viswanathan, S. (2019). Mesenchymal stromal cell therapy: progress in manufacturing and assessments of potency. Cytotherapy 21, 289–306. doi: 10.1016/j.jcyt.2018.10.014
Rodrigues, A. L., Rodrigues, C. A., Gomes, A. R., Vieira, S. F., Badenes, S. M., Diogo, M. M., et al. (2019). Dissolvable microcarriers allow scalable expansion and harvesting of human induced pluripotent stem cells under xeno-free conditions. Biotechnol. J. 14:e1800461. doi: 10.1002/biot.201800461
Rojewski, M. T., Fekete, N., Baila, S., Nguyen, K., Fürst, D., Antwiler, D., et al. (2013). GMP-compliant isolation and expansion of bone marrow-derived MSCs in the closed, automated device quantum cell expansion system. Cell Transplant. 22, 1981–2000. doi: 10.3727/096368912X657990
Rowley, J., Abraham, E., Campbell, A., Brandwein, H., and Oh, S. (2012). Meeting lot-size challenges of manufacturing adherent cells for therapy. BioProcess. Int. 10:7.
Russell, K. C., Phinney, D. G., Lacey, M. R., Barrilleaux, B. L., Meyertholen, K. E., and O'Connor, K. C. (2010). In vitro high-capacity assay to quantify the clonal heterogeneity in trilineage potential of mesenchymal stem cells reveals a complex hierarchy of lineage commitment. Stem Cells 28, 788–798. doi: 10.1002/stem.312
Samsonraj, R. M., Rai, B., Sathiyanathan, P., Puan, K. J., Rötzschke, O., Hui, J. H., et al. (2015). Establishing criteria for human mesenchymal stem cell potency. Stem Cells 33, 1878–1891. doi: 10.1002/stem.1982
Sart, S., Agathos, S. N., and Li, Y. (2013). Engineering stem cell fate with biochemical and biomechanical properties of microcarriers. Biotechnol. Prog. 29, 1354–1366. doi: 10.1002/btpr.1825
Sart, S., Schneider, Y. J., and Agathos, S. N. (2009). Ear mesenchymal stem cells: an efficient adult multipotent cell population fit for rapid and scalable expansion. J. Biotechnol. 139, 291–299. doi: 10.1016/j.jbiotec.2008.12.011
Sart, S., Schneider, Y. J., Li, Y., and Agathos, S. N. (2014a). Stem cell bioprocess engineering towards cGMP production and clinical applications. Cytotechnology 66, 709–722. doi: 10.1007/s10616-013-9687-7
Sart, S., Tsai, A. C., Li, Y., and Ma, T. (2014b). Three-dimensional aggregates of mesenchymal stem cells: cellular mechanisms, biological properties, and applications. Tissue Eng. Part B Rev. 20, 365–380. doi: 10.1089/ten.teb.2013.0537
Schatti, O., Grad, S., Goldhahn, J., Salzmann, G., Li, Z., Alini, M., et al. (2011). A combination of shear and dynamic compression leads to mechanically induced chondrogenesis of human mesenchymal stem cells. Eur. Cell Mater. 22:b97. doi: 10.22203/eCM.v022a17
Schirmaier, C., Jossen, V., Kaiser, S. C., Jüngerkes, F., Brill, S., Safavi-Nab, A., et al. (2014). Scale-up of adipose tissue-derived mesenchymal stem cell production in stirred single-use bioreactors under low-serum conditions. Eng. Life Sci. 14, 292–303. doi: 10.1002/elsc.201300134
Schnitzler, A., Kehoe, D., Simler, J., DiLeo, A., and Ball, A. (2012). Scale-up of human mesenchymal stem cells on microcarriers in suspension in a single-use bioreactor. BioPharm. Int. 25, 28–38.
Schnitzler, A. C., Verma, A., Kehoe, D. E., Jing, D., Murrell, J. R., Der, K. A., et al. (2016). Bioprocessing of human mesenchymal stem/stromal cells for therapeutic use: current technologies and challenges. Biochem. Eng. J. 108, 3–13. doi: 10.1016/j.bej.2015.08.014
Sensebé, L., Gadelorge, M., and Fleury-Cappellesso, S. (2013). Production of mesenchymal stromal/stem cells according to good manufacturing practices: a review. Stem Cell Res. Ther. 4:1. doi: 10.1155/2013/678063
Shekaran, A., Lam, A., Sim, E., Jialing, L., Jian, L., Wen, J. T. P., et al. (2016). Biodegradable ECM-coated PCL microcarriers support scalable human early MSC expansion and in vivo bone formation. Cytotherapy 18, 1332–1344. doi: 10.1016/j.jcyt.2016.06.016
Shekaran, A., Sim, E., Tan, K. Y., Chan, J. K., Choolani, M., Reuveny, S., et al. (2015). Enhanced in vitro osteogenic differentiation of human fetal MSCs attached to 3D microcarriers versus harvested from 2D monolayers. BMC Biotechnol. 15:102. doi: 10.1186/s12896-015-0219-8
Shi, S., and Gronthos, S. (2003). Perivascular niche of postnatal mesenchymal stem cells in human bone marrow and dental pulp. J. Bone Miner. Res. 18, 696–704. doi: 10.1359/jbmr.2003.18.4.696
Shih, Y. R. V., Tseng, K. F., Lai, H. Y., Lin, C. H., and Lee, O. K. (2011). Matrix stiffness regulation of integrin-mediated mechanotransduction during osteogenic differentiation of human mesenchymal stem cells. J. Bone Miner. Res. 26, 730–738. doi: 10.1002/jbmr.278
Siddiquee, K., and Sha, M. (2014). Large-scale production of human mesenchymal stem cells in BioBLU® 5c single-use vessels. Appl. Note 334, 1–10.
Simaria, A. S., Hassan, S., Varadaraju, H., Rowley, J., Warren, K., Vanek, P., et al. (2014). Allogeneic cell therapy bioprocess economics and optimization: single-use cell expansion technologies. Biotechnol. Bioeng. 111, 69–83. doi: 10.1002/bit.25008
Simon, M. (2015). Bioreactor design for adherent cell culture: the bolt-on bioreactor project, part 1—volumetric productivity. BioProcess Int. 13.
Song, K., Yang, Y., Wu, S., Zhang, Y., Feng, S., Wang, H., et al. (2016). In vitro culture and harvest of BMMSCs on the surface of a novel thermosensitive glass microcarrier. Mater. Sci. Eng. C 58, 324–330. doi: 10.1016/j.msec.2015.08.033
Sotiropoulou, P. A., Perez, S. A., Salagianni, M., Baxevanis, C. N., and Papamichail, M. (2006). Characterization of the optimal culture conditions for clinical scale production of human mesenchymal stem cells. Stem Cells 24, 462–471. doi: 10.1634/stemcells.2004-0331
Sousa, M. F., Silva, M. M., Giroux, D., Hashimura, Y., Wesselschmidt, R., Lee, B., et al. (2015). Production of oncolytic adenovirus and human mesenchymal stem cells in a single-use, vertical-wheel bioreactor system: impact of bioreactor design on performance of microcarrier-based cell culture processes. Biotechnol. Prog. 31, 1600–1612. doi: 10.1002/btpr.2158
Stroncek, D. F., Sabatino, M., Ren, J., England, L., Kuznetsov, S. A., Klein, H. G., et al. (2014). Establishing a bone marrow stromal cell transplant program at the National Institutes of Health Clinical Center. Tissue Eng. Part B Rev. 20, 200–205. doi: 10.1089/ten.teb.2013.0529
Sun, L. Y., Hsieh, D. K., Syu, W. S., Li, Y. S., Chiu, H. T., and Chiou, T. W. (2010). Cell proliferation of human bone marrow mesenchymal stem cells on degradable microcarriers enhances in vitro differentiation potential. Cell Prolif. 43, 445–456. doi: 10.1111/j.1365-2184.2010.00694.x
Takahashi, I., Sato, K., Mera, H., Wakitani, S., and Takagi, M. (2017). Effects of agitation rate on aggregation during beads-to-beads subcultivation of microcarrier culture of human mesenchymal stem cells. Cytotechnology 69, 503–509. doi: 10.1007/s10616-016-9999-5
Teixeira, F. G., Carvalho, M. M., Panchalingam, K. M., Rodrigues, A. J., Mendes-Pinheiro, B., Anjo, S., et al. (2017). Impact of the secretome of human mesenchymal stem cells on brain structure and animal behavior in a rat model of Parkinson's disease. Stem Cells Transl. Med. 6, 634–646. doi: 10.5966/sctm.2016-0071
Teixeira, F. G., Panchalingam, K. M., Assunção-Silva, R., Serra, S. C., Mendes-Pinheiro, B., Patrício, P., et al. (2016). Modulation of the mesenchymal stem cell secretome using computer-controlled bioreactors: impact on neuronal cell proliferation, survival and differentiation. Sci. Rep. 6:27791. doi: 10.1038/srep27791
Théry, M. (2010). Micropatterning as a tool to decipher cell morphogenesis and functions. J. Cell Sci. 123, 4201–4213. doi: 10.1242/jcs.075150
Théry, M., Pépin, A., Dressaire, E., Chen, Y., and Bornens, M. (2006). Cell distribution of stress fibres in response to the geometry of the adhesive environment. Cell Motil. Cytoskeleton 63, 341–355. doi: 10.1002/cm.20126
Timmins, N., Kiel, M., Günther, M., Heazlewood, C., Doran, M., Brooke, G., et al. (2012). Closed system isolation and scalable expansion of human placental mesenchymal stem cells. Biotechnol. Bioeng. 109, 1817–1826. doi: 10.1002/bit.24425
Tran, T. C., Kimura, K., Nagano, M., Yamashita, T., Ohneda, K., Sugimori, H., et al. (2011). Identification of human placenta-derived mesenchymal stem cells involved in re-endothelialization. J. Cell Physiol. 226, 224–235. doi: 10.1002/jcp.22329
Trichet, L., Le Digabel, J., Hawkins, R. J., Vedula, S. R. K., Gupta, M., Ribrault, C., et al. (2012). Evidence of a large-scale mechanosensing mechanism for cellular adaptation to substrate stiffness. Proc. Natl. Acad. Sci. U.S.A. 109, 6933–6938. doi: 10.1073/pnas.1117810109
Tsai, A. C., Liu, Y., and Ma, T. (2016). Expansion of human mesenchymal stem cells in fibrous bed bioreactor. Biochem. Eng. J. 108, 51–57. doi: 10.1016/j.bej.2015.09.002
Tsai, A. C., Liu, Y., Yuan, X., Chella, R., and Ma, T. (2017). Aggregation kinetics of human mesenchymal stem cells under wave motion. Biotechnol. J. 12:1600448. doi: 10.1002/biot.201600448
Tsai, A. C., Liu, Y., Yuan, X., and Ma, T. (2015). Compaction, fusion, and functional activation of three-dimensional human mesenchymal stem cell aggregate. Tissue Eng. Part A 21, 1705–1719. doi: 10.1089/ten.tea.2014.0314
Tsai, A. C., and Ma, T. (2016). Expansion of human mesenchymal stem cells in a microcarrier bioreactor. Methods Mol. Biol. 1502, 77–86. doi: 10.1007/7651_2016_338
Tseng, P. C., Young, T. H., Wang, T. M., Peng, H. W., Hou, S. M., and Yen, M. L. (2012). Spontaneous osteogenesis of MSCs cultured on 3D microcarriers through alteration of cytoskeletal tension. Biomaterials 33, 556–564. doi: 10.1016/j.biomaterials.2011.09.090
Tsuchiya, A., Takeuchi, S., Watanabe, T., Yoshida, T., Nojiri, S., Ogawa, M., et al. (2019). Mesenchymal stem cell therapies for liver cirrhosis: MSCs as “conducting cells” for improvement of liver fibrosis and regeneration. Inflamm. Regen. 39, 1–6. doi: 10.1186/s41232-019-0107-z
Ueki, A., and Kidoaki, S. (2015). Manipulation of cell mechanotaxis by designing curvature of the elasticity boundary on hydrogel matrix. Biomaterials 41, 45–52. doi: 10.1016/j.biomaterials.2014.11.030
van Eikenhorst, G., Thomassen, Y. E. L. A, and van der Pol Bakker, W. A. (2014). Assessment of mass transfer and mixing in rigid lab-scale disposable bioreactors at low power input levels. Biotechnol. Prog. 301269–1276. doi: 10.1002/btpr.1981
Van Wezel, A. (1967). Growth of cell-strains and primary cells on micro-carriers in homogeneous culture. Nature 216, 64–65. doi: 10.1038/216064a0
Vassaux, M., and Milan, J. (2017). Stem cell mechanical behaviour modelling: substrate's curvature influence during adhesion. Biomech. Model. Mechanobiol. 16, 1295–1308. doi: 10.1007/s10237-017-0888-4
Wang, S., Qu, X., and Zhao, R. C. (2012). Clinical applications of mesenchymal stem cells. J. Hematol. Oncol. 5:19. doi: 10.1186/1756-8722-5-19
Wang, Z., Wu, D., Zou, J., Zhou, Q., Liu, W., Zhang, W., et al. (2017). Development of demineralized bone matrix-based implantable and biomimetic microcarrier for stem cell expansion and single-step tissue-engineered bone graft construction. J. Mater. Chem. B 5, 62–73. doi: 10.1039/C6TB02414A
Weber, C., Freimark, D., Pörtner, R., Pino-Grace, P., Pohl, S., Wallrapp, C., et al. (2010). Expansion of human mesenchymal stem cells in a fixed-bed bioreactor system based on non-porous glass carrier—part A: inoculation, cultivation, and cell harvest procedures. Int. J. Artif. Organs 33:512. doi: 10.1177/039139881003300802
Weber, C., Pohl, S., Pörtner, R., Wallrapp, C., Kassem, M., Geigle, P., et al. (2007). Expansion and harvesting of hMSC-TERT. Open Biomed. Eng. J. 1, 38–46. doi: 10.2174/1874120700701010038
Wegmeyer, H., Bröske, A. M., Leddin, M., Kuentzer, K., Nisslbeck, A. K., Hupfeld, J., et al. (2013). Mesenchymal stromal cell characteristics vary depending on their origin. Stem Cells Dev. 22, 2606–2618. doi: 10.1089/scd.2013.0016
Werner, M., Blanquer, S. B., Haimi, S. P., Korus, G., Dunlop, J. W., Duda, G. N., et al. (2017). Surface curvature differentially regulates stem cell migration and differentiation via altered attachment morphology and nuclear deformation. Adv. Sci. 4:1600347. doi: 10.1002/advs.201600347
Wilson, J. R., and Kowol, E. (1994). Pressure Control System for a Bioreactor. U.S. Patent No 5,330,915. Alexandria, VA: United States Patent and Trademark Office.
Xu, J., Chen, C., Jiang, X., Xu, R., Tambe, D., Zhang, X., et al. (2011). Effects of micropatterned curvature on the motility and mechanical properties of airway smooth muscle cells. Biochem. Biophys. Res. Commun. 415, 591–596. doi: 10.1016/j.bbrc.2011.10.111
Xu, S., Hoshan, L., Jiang, R., Gupta, B., Brodean, E., O'Neill, K., et al. (2017). A practical approach in bioreactor scale-up and process transfer using a combination of constant P/V and vvm as the criterion. Biotechnol. Prog. 33, 1146–1159. doi: 10.1002/btpr.2489
Yan, X., Zhang, K., Yang, Y., Deng, D., Lyu, C., Xu, H., et al. (2020). Dispersible and dissolvable porous microcarrier tablets enable efficient large scale hMSC expansion. Tissue Eng. Part C Methods. 26, 263–275. doi: 10.1089/ten.tec.2020.0039
Yang, H. S., Jeon, O., Bhang, S. H., Lee, S. H., and Kim, B. S. (2010). Suspension culture of mammalian cells using thermosensitive microcarrier that allows cell detachment without proteolytic enzyme treatment. Cell Transplant. 19, 1123–1132. doi: 10.3727/096368910X516664
YekrangSafakar, A., Acun, A., Choi, J. W., Song, E., Zorlutuna, P., and Park, K. (2018). Hollow microcarriers for large-scale expansion of anchorage-dependent cells in a stirred bioreactor. Biotechnol. Bioeng. 115, 1717–1728. doi: 10.1002/bit.26601
Yin, J. Q., Zhu, J., and Ankrum, J. A. (2019). Manufacturing of primed mesenchymal stromal cells for therapy. Nat. Biomed. Eng. 3, 90–104. doi: 10.1038/s41551-018-0325-8
Yourek, G., McCormick, S. M., Mao, J. J., and Reilly, G. C. (2010). Shear stress induces osteogenic differentiation of human mesenchymal stem cells. Regen. Med. 5, 713–724. doi: 10.2217/rme.10.60
Yu, C., Kornmuller, A., Brown, C., Hoare, T., and Flynn, L. E. (2017). Decellularized adipose tissue microcarriers as a dynamic culture platform for human adipose-derived stem/stromal cell expansion. Biomaterials 120, 66–80. doi: 10.1016/j.biomaterials.2016.12.017
Yuan, X., Logan, T. M., and Ma, T. (2019). Metabolism in human mesenchymal stromal cells: a missing link between hMSC biomanufacturing and therapy? Front. Immunol. 10:977. doi: 10.3389/fimmu.2019.00977
Yuan, X., Tsai, A. C., Farrance, I., Rowley, J. A., and Ma, T. (2018). Aggregation of culture expanded human mesenchymal stem cells in microcarrier-based bioreactor. Biochem. Eng. J. 131, 39–46. doi: 10.1016/j.bej.2017.12.011
Yuan, Y., Kallos, M. S., Hunter, C., and Sen, A. (2014). Improved expansion of human bone marrow-derived mesenchymal stem cells in microcarrier-based suspension culture. J. Tissue Eng. Regen. Med. 8, 210–225. doi: 10.1002/term.1515
Zayzafoon, M., Gathings, W. E., and McDonald, J. M. (2004). Modeled microgravity inhibits osteogenic differentiation of human mesenchymal stem cells and increases adipogenesis. Endocrinology 145, 2421–2432. doi: 10.1210/en.2003-1156
Zhang, J., Huang, X., Wang, H., Liu, X., Zhang, T., Wang, Y., et al. (2015). The challenges and promises of allogeneic mesenchymal stem cells for use as a cell-based therapy. Stem Cell Res. Ther. 6:234. doi: 10.1186/s13287-015-0240-9
Zhang, Y., Mao, H., Gao, C., Li, S., Shuai, Q., Xu, J., et al. (2016). Enhanced biological functions of human mesenchymal stem-cell aggregates incorporating E-cadherin-modified PLGA microparticles. Adv. Healthc. Mater. 5, 1949–1959. doi: 10.1002/adhm.201600114
Zhang, Z. Y., Teoh, S. H., Hui, J. H., Fisk, N. M., Choolani, M., and Chan, J. K. (2012). The potential of human fetal mesenchymal stem cells for off-the-shelf bone tissue engineering application. Biomaterials 33, 2656–2672. doi: 10.1016/j.biomaterials.2011.12.025
Keywords: human mesenchymal stem cells, microcarriers, bioreactors, shear stress, microenvironment, expansion
Citation: Tsai A-C, Jeske R, Chen X, Yuan X and Li Y (2020) Influence of Microenvironment on Mesenchymal Stem Cell Therapeutic Potency: From Planar Culture to Microcarriers. Front. Bioeng. Biotechnol. 8:640. doi: 10.3389/fbioe.2020.00640
Received: 18 March 2020; Accepted: 26 May 2020;
Published: 24 June 2020.
Edited by:
Tiago G. Fernandes, University of Lisbon, PortugalReviewed by:
Denise Salzig, University of Applied Sciences Mittelhessen, GermanyCopyright © 2020 Tsai, Jeske, Chen, Yuan and Li. This is an open-access article distributed under the terms of the Creative Commons Attribution License (CC BY). The use, distribution or reproduction in other forums is permitted, provided the original author(s) and the copyright owner(s) are credited and that the original publication in this journal is cited, in accordance with accepted academic practice. No use, distribution or reproduction is permitted which does not comply with these terms.
*Correspondence: Xuegang Yuan, eHkxM2JAbXkuZnN1LmVkdQ==; Yan Li, eWxpNEBmc3UuZWR1
†Present Address: Ang-Chen Tsai, University of Florida, Gainesville, FL, United States
Disclaimer: All claims expressed in this article are solely those of the authors and do not necessarily represent those of their affiliated organizations, or those of the publisher, the editors and the reviewers. Any product that may be evaluated in this article or claim that may be made by its manufacturer is not guaranteed or endorsed by the publisher.
Research integrity at Frontiers
Learn more about the work of our research integrity team to safeguard the quality of each article we publish.