- 1Department of Biomedical Engineering, The Hong Kong Polytechnic University, Hong Kong, China
- 2State Key Laboratory of Molecular Engineering of Polymers, Department of Orthopedic Surgery, Fudan University, Shanghai, China
- 3General Hospital, Shanghai Jiaotong University School of Medicine, Shanghai, China
Cardiovascular stenting is an effective method for treating cardiovascular diseases (CVDs), yet thrombosis and restenosis are the two major clinical complications that often lead to device failure. Nitric oxide (NO) has been proposed as a promising small molecule in improving the clinical performance of cardiovascular stents thanks to its anti-thrombosis and anti-restenosis ability, but its short half-life limits the full use of NO. To produce NO at lesion site with sufficient amount, NO-producing coatings (including NO-releasing and NO-generating coatings) are fashioned. Its releasing strategy is achieved by introducing exogenous NO storage materials like NO donors, while the generating strategy utilizes the in vivo substances such as S-nitrosothiols (RSNOs) to generate NO flux. NO-producing stents are particularly promising in future clinical use due to their ability to store NO resources or to generate large NO flux in a controlled and efficient manner. In this review, we first introduce NO-releasing and -generating coatings for prevention of thrombosis and restenosis. We then discuss the advantages and drawbacks on releasing and generating aspects, where possible further developments are suggested.
Introduction
Cardiovascular diseases (CVDs) are a common cause of morbidity worldwide, accounting for 18 million deaths per year, and serve as a third of all global deaths (Frieden and Jaffe, 2018; Yusuf et al., 2020). To treat CVDs, surgical interventions including heart valve replacements (Saito et al., 2003), angioplasty (Stone et al., 2002), and intravascular stents (Zhu et al., 2014) are employed. Among these surgical procedures, cardiovascular stent is most commonly used in coronary heart disease, myocardial infarction, and stenocardia due to its effectiveness at dilating blood vessels and maintaining the circulation of blood (Kubo et al., 2008; Geng et al., 2013; Wei et al., 2013). In the United States alone, ~2 million patients undergo stent implantation each year (Cicha et al., 2016). However, stenting has a high tendency to cause thrombosis and restenosis. Intravascular injuries during surgical implantation stimulate coagulation pathways to facilitate platelet adhesion (Furie and Furie, 2008), and the production of thrombin further contributes to platelet activation and converses fibrinogen into fibrin-formed thrombus (Zhao et al., 2014). The accumulation of platelets and leucocytes causes acute inflammatory response and results in endothelial malfunction, which can lead to excessive proliferation of smooth muscle cells (SMCs) (Naghavi et al., 2013). The extracellular matrix (ECM) produced by SMCs then induces thickening of the vessel wall, contributing to neointimal hyperplasia and restenosis (Scott and Panitch, 2014). As a result, thrombosis and restenosis hamper the long-term effectiveness of cardiovascular devices and induce other related symptoms.
To ease the risk of thrombosis and restenosis, cardiovascular stents are modified with different polymers, biomolecules, or coatings. Compared to the traditional bare metal stents (BMSs) with unacceptable levels of thrombosis and restenosis (Nakamura et al., 2016), drug-eluting stents (DESs) can reduce neointimal hyperplasia and preserve vessel patency by releasing drugs from surface polymers (Joner et al., 2006). However, DESs may induce late thrombosis, which can be attributed to the depletion of drug reservoir, off-target effect of drugs, and the inflammatory response (Mcfadden et al., 2004; Ma et al., 2010). The most recent DES technology fabricates dual-therapy stents (DTSs) that combine two therapeutic regents like aspirin and adenosine diphosphate-receptor blockade for anti-platelet therapy (Ohkubo et al., 2011). Meanwhile, bioresorbable vascular scaffolds (BVSs) can decrease the propensity for thrombosis, since BVSs allow implants to degrade over time and leave an intact vessel (Stone, 2016). Furthermore, bio-engineered stents (BESs) adopt biocompatible materials, cell capture technology, or autologous venous tissue for better therapeutic effects (Stone et al., 2002; Hara et al., 2006; Colombo et al., 2019). In addition to material innovation, novel stent designs also employ coatings to improve the surface properties and clinical behaviors (Yang et al., 2020a). The coating materials possess the feasibility to directly attach or deposit onto the stents' surface and the ability to maintain a high local therapeutic concentration at specific site (Yang et al., 2017). Drugs and biomolecules such as paclitaxel (Palmerini et al., 2015), adhesive peptides (Wei et al., 2013), anti-CD 34 antibodies (Yoon et al., 2002), and nitric oxide (NO)-producing moieties (Gunasekera et al., 2018) are employed to improve the clinical behaviors of stents (see Table 1 for details). Although these molecules achieved good therapeutic effects, they still present some limitations. For example, high toxicity and non-selective function of paclitaxel raises safety concerns about its side effects while high cost and potential complications of antibodies and adhesive peptides may hinder treatment efficacy (Pacelli et al., 2017). Among all therapeutic molecules, while NO still possesses some drawbacks such as low diffusion distance in blood, it remains highly effective because of its anti-thrombosis and anti-restenosis ability. As an endogenous substance, its pro-proliferative activity to endothelium and anti-adhesion/aggregation effect on platelets additionally allow NO to serve as a guardian of cardiovascular implants (Winther et al., 2018). As reported by the ClinicalTrials.gov website, there are 678 NO-related clinical studies in CVD treatment by April 2020, which reveals the highly promising research and clinical application prospects of NO.
In a healthy vasculature, endothelial cells (ECs) produce NO to achieve thrombotic homeostasis by preventing platelet activation (Seabra et al., 2015). The mechanism of NO inhibition of platelet activity is multifaceted. The main way is to activate the soluble guanylate cyclase (sGC), thereby increasing concentrations of cyclic guanosine monophosphate (cGMP) and stimulating the downstream platelet inhibition molecule—Protein Kinase G (PKG) (Francis et al., 2010; Kraehling and Sessa, 2017). The other anti-platelet activity of NO is to inhibit the thromboxane receptor on platelet membranes (Fuentes and Palomo, 2016). Meanwhile, NO is also closely related to cardiovascular homeostasis. In the cardiovascular system, NO can relax the surrounding smooth muscle, lead to vasodilation, and increase blood flow (Jon O Lundberg et al., 2015). Moreover, NO stimulates EC migration and suppresses SMC proliferation (Mel et al., 2011). It also influences angiogenesis and vascular remodeling as well as kills various pathogens (Devine et al., 2019).
Under physical condition, NO can only diffuse around 100 μm and degrades within seconds in blood, so NO must be administered or produced at the diseased site (Thomas, 2015). Hence, incorporating NO production moieties with stent coatings will work as a functional platform to achieve the anti-thrombosis and anti-restenosis purpose. In this review, we will discuss NO-producing coatings, with the emphasis on the NO-releasing and -generating strategies (Figure 1 and Table 2). NO-releasing materials such as NO donors or peptides work as a finite reservoir, while NO-generating strategies utilize catalytic substances or genes to achieve endogenous NO supply. These coatings overcome the challenges of NO administration, and serve as great delivery platforms since they can maintain the physiologically relevant concentrations of NO at a specific site.
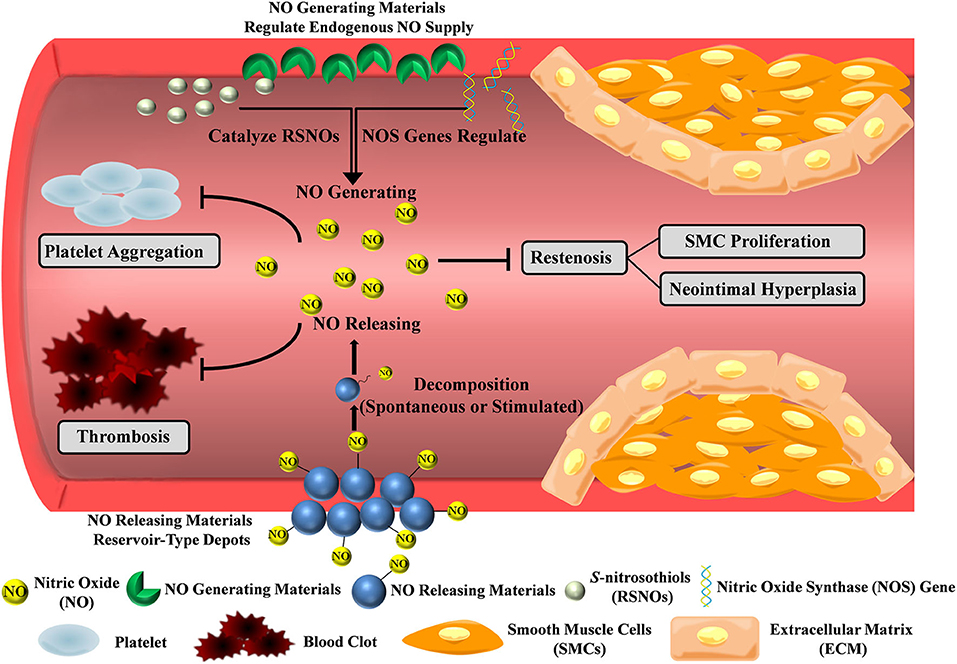
Figure 1. NO-producing strategies for prevention of thrombosis and restenosis. NO-releasing materials such as NO donors and peptides work as a finite reservoir, while NO-generating materials utilize catalytic substances or genes to achieve endogenous NO supply. The NO flux inhibits platelet activation and SMC proliferation, thereby preventing thrombosis and restenosis.
No-Releasing Coatings
In NO-releasing strategies, the potential NO can be stored in small molecules or biomolecules, and through connecting, dispersing, or encapsulating those functional molecules as a part of delivery system, the platform will act as a reservoir for conditional and spontaneous release of NO.
NO Donors
NO donors [e.g., N-diazeniumdiolates (NONOates), S-nitroso-N-acetylpenicillamine (SNAP)] are pharmacologically active substances that carry NO for localized release (Major et al., 2010; Midgley et al., 2019). NONOates can be synthesized by reacting gaseous NO with secondary amines under high pressure (5 atm) and spontaneously decomposed to release two moles of NO per mole of donor at physiological condition (Midgley et al., 2019), while SNAP, a kind of synthetic S-nitrosothiols (RSNOs), requires reactions between nitrosating agents and thiols, and NO can be exhausted from SNAP by heat, light (340 and 590 nm), or copper ions (Naghavi et al., 2013).
For example, Joung et al. fabricated NONOates containing liposome stent coatings via layer-by-layer (LBL) method to control the release of NO (Elnaggar et al., 2016). The NO release profiles showed that the release rate sustained up to 5 days with good thrombo-resistant effect. As thrombus involves the formation of fibrin and activation of platelets, Yang et al. proposed a novel concept to integrate anti-coagulant agent bivalirudin (BVLD) and NO as a bulk synergetic modification on surface coating (Yang et al., 2020b). BVLD was covalently connected with the primary amine groups on plasma polymerized allylamine (PPAm)-coated surface, and then the coating was immersed in basic solution under high-pressure NO gas to form a NONOate-like bulk depot. For circumventing NO burst release, hydrophobic hexafluoroethane was introduced into this system, which prolonged the release time of NO to more than 8 h. Compared to 316L SS, the occlusion rate and thrombus weight in BVLD/NO-PPAmF were reduced from 58.7 ± 3.9% to 3.3 ± 1.2% and 64.1 ± 3.9 mg to 1.7 ± 1.2 mg after 2 h of extracorporeal circulation (ECC), respectively. Since the complexity of thrombogenic process requires multi-therapy procedures, the combination of anti-platelet and anti-coagulant dual functions is a promising strategy to integrate into cardiovascular coatings.
However, the NO release period in these studies was limited. To extend the NO release time, Hopkins et al. designed a highly stable NO-releasing coating by covalently attaching SNAP with poly(dimethylsiloxane) (PDMS) (Hopkins et al., 2018). Previous studies demonstrated that SNAP-based polymers have exhibited significant leaching of SNAP, which will result in non-localized NO release (Brisbois et al., 2016). Through tethering to PDMS, SNAP leaching into surrounding environment was prevented and allowed for lengthened potency. The NO flux formation of SNAP-PDMS reached up to 125 days in vitro and maintained at 0.1 × 10−10 mol × cm−2 × min−1 till the end of the testing period. The SNAP-PDMS showed long-duration anti-bacterial efficacy, and it exhibited 78% thrombus reduction (compared with blank control) after testing by ECC over 4 h. Although this strategy achieved long-term NO release, the low NO levels in the later release stages limited the long-lasting anti-thrombotic effect.
Despite these efforts on NO donor applications, the long-term therapeutic effect after implantation was unsatisfying. Integrating NO-donor loaded liposomes with stents faces the risk of low loading capacity, carrier detachment from the stents and delayed NO release. Chemical covalent bond offers bulk NO donor storage, but it also brings extra reaction regents and shows relative low NO flux. Besides, the instability of the NO donors is a big obstacle that cannot be solved by simple connection or encapsulation. Hence, many other coatings have been developed for better application.
NO Prodrugs
Since NO donors are unstable under thermal, acidic, or physiological conditions, researchers use enzyme prodrug therapy (EPT) to maintain the stability of donors and control the release (Chandrawati et al., 2017). By fabricating NO donors (e.g., NONOates) with enzyme-sensitive linkers, the formed prodrugs remain stable and inactive in non-enzymatic environment and only release NO upon enzyme-triggering.
In a study of NO EPT, Wang et al. designed a galactosidase (Gal) immobilized surface coating and prepared glycosylated NONOate (Gal-NONOate) as NO prodrug (Wang et al., 2015). After the in vivo implantation of enzyme-functional platform, Gal-NONOate was administrated by tail vein injection, it then circulated until contact with the coating and the enzyme would catalyze the decomposition of the prodrug to release NO locally. The in situ release of NO was verified using a fluorescent probe to trace the NO flux. The immobilized enzyme retained the catalytic property up to 1 month in vivo and the results revealed effective inhibition of thrombosis and enhancement of vascular tissue regeneration and remodeling in EPT group. To engineer EPT for diverse medicinal implants, Zelikin et al. optimized EPT coating by adopting LBL method to fabricate multilayered polyelectrolyte coating with immobilized β-Gal enzyme (Winther et al., 2018). This method was all-aqueous and solution-based, which could accommodate modification of any substrate with no restriction on surface topography and geometry.
In addition to maintaining NO donors' stability, through using different concentrations of prodrugs or regulating administration time to adjust physiological effect, EPT can achieve personalized, fine-tuned therapeutic delivery of NO (Pan et al., 2015). Nevertheless, EPT requires systemic delivery of NO prodrugs, which only has effect at the enzyme-modified lesion site. This means that even with a bulky administration, only a small portion of drugs would work, which causes drug waste and side effects due to the high cytotoxicity of the drug. Scientists thus have developed other biomolecules to achieve NO release.
NO-Releasing Peptide Amphiphiles
Peptide amphiphiles (PAs) consist of hydrophobic tails coupled to hydrophilic functional peptide sequences that are attractive in biomimetic scaffolds, since enzyme-mediated degradable sites and cell adhesion ligands can be incorporated into PAs to mimic some biochemical properties (Jun et al., 2005; Cheng et al., 2015).
In a report by Matson et al., a NO-releasing PA coating was designed (Kushwaha et al., 2010). The functional peptide sequences involved a matrix metalloprotease-2 (MMP2) mediated cleavage site Gly-Thr-Ala-Gly-Leu-Ile-Gly-Gln (GTAGLIGQ), coupled to an EC-adhesive ligand Tyr-Ile-Gly-Ser-Arg (YIGSR) or a polylysine (KKKKK) group to form NO donating residue peptide. Burst release of NO occurred within 48 h, followed by sustained release for 30 days. This functional peptide increased initial adhesion of ECs from 51 ± 3% to 67 ± 2%, while the proliferation of SMCs were inhibited from 35 ± 2% to 16 ± 3% after 48 h of incubation. Additionally, compared with the positive control collagen group, the peptide group showed 150-fold decrease in platelet attachment, suggesting the potential of such coating for modification of various cardiovascular implants. Similarly, Alexander et al. used the same PAs to form a nanomatrix coating (Alexander et al., 2016), and they proved the vasodilatory effects ex vivo and the anti-inflammatory ability in vitro. This coating could address the shortcomings of the implanted stents and had the potential to be developed in animal models with cardiovascular stents. However, it is difficult to popularize peptide clinical application due to the high cost of peptides and the lack of control over their activity.
NO-releasing strategies have showed appropriate therapeutic effects on thrombosis and restenosis, but they still possess some shortcomings. In addition to the problems of different NO-releasing materials discussed above, all these strategies have to face the biggest obstacle—the finite reservoir of NO. With the NO release reaction proceeding, the elements will be exhausted within a short time frame. Therefore, reliable long-term and sufficient NO supplies need to be achieved.
No-Generating Coatings
Radical NO species are short-lived. To overcome this drawback, NO-generating materials were developed, which utilize genes and catalytic substances such as selenium (Se) or copper ions to stimulate NO production. These strategies can achieve sufficient and long-term NO release depending on the endogenous regulation and continuous supply of NO resources.
NOS Gene Therapy
In the cardiovascular system, nitric oxide synthase (NOS) especially endothelial NOS (eNOS) and inducible NOS (iNOS) play a very important role. eNOS is highly related to the function of the endothelium, which can catalyze the production of NO via enzymatic effect (Zhao et al., 2013). iNOS is normally absent in the vasculature under physiological conditions, but it expresses in blood vessels under pathological situations (Sessa, 2004), and it can generate large amounts of NO over long periods of time (Kleinert et al., 2005). Since NOS has emerged as an active player in NO generation, a lot of therapeutic strategies have been developed around it. Gene therapy in CVDs can improve the selectivity of exerted effects toward certain cells and has the potential for combination therapy. Many researchers have thus investigated the therapeutic effect of NOS genes (Forstermann et al., 2017). In a study, DiMuzio et al. developed a natural vascular tissue mimetic stent by seeding it with autologous adipose-derived stem cells (ASCs) differentiated endothelial-like cells, and they used the eNOS gene to transfect these ASCs (McIlhenny et al., 2013). The transfection initiated eNOS production, yielding eNOS to generate functional NO gas. They found that the transfected ASCs produced NO (247 ± 10 nM) at a similar level to EC controls (288 ± 29 nM) in vitro, and exhibited a non-thrombogenic surface compared to unseeded controls in vivo.
In addition to in vitro gene transfection therapy, gene delivery is another approach to achieve targeted protein expression. To deliver NOS gene, Levy et al. described a gene delivery platform that provided local arterial gene transfer via iNOS-encoding adeno-associated virus serotype 2 (AAV2) vectors (Fishbein et al., 2017). Through affinity effect, the iNOS-cDNA sequence loaded AAV2 vectors could be immobilized onto stent surfaces. Compared to the non-gene group, AAV2iNOS showed a 16-fold higher NO production in vitro. AAV2iNOS demonstrated escalating expression of encoded transgene for 12 weeks and showed the anti-restenosis efficacy with 95% inhibition rate in vivo.
Although there are many attempts in using NOS gene strategies, the instability, deliverability, release kinetics, off-target transduction, and high cost of gene limit the clinical application. Researchers are hence working hard on more effective NO-generating pathways.
Endogenous NO Catalytic Approaches
RSNOs, a kind of endogenous NO donors in the blood, has highly promising opportunities to achieve localized synthesis of NO for continuous supply (Li et al., 2018). It was found that glutathione peroxidase (GPx) and Se or copper ions with GPx-like catalytic activity can catalyze the decomposition of RSNOs into NO in vivo (Weng et al., 2011; McCarthy et al., 2016).
Recently, our group developed one-pot approach to incorporate selenocystamine (SeCA) in the framework of dopamine (DA) via co-immobilization (Yang et al., 2018). The NO release rates could be controlled (from 0.5 to 2.2 × 10−10 mol × cm−2 × min−1) by regulating the SeCA–DA molar ratio. The study showed that the SeCA/DA coating could release NO for more than 60 days, which achieved long-lasting prevention of thrombosis and restenosis. Additionally, the simple operation avoided the tedious process and toxic chemicals. This coating is believed to be universally formed on diverse types of materials with great clinical potential. We further improved the NO production ability by employing CuII instead of SeCA, since CuII possesses superior NO catalytic efficacy (Zhang et al., 2019). The NO release rates could reach to natural endothelium rates (0.5 to 4 × 10−10 mol × cm−2 × min−1) by adjusting the dose of CuII with NO flux ranging from 0.4 to 6.5 × 10−10 mol × cm−2 × min−1. After implantation for 3 months, the DA-CuII-coated stents not only inhibited thrombosis, but also promoted re-endothelialization and reduced neointimal formation. We then additionally endowed our stents with anti-bacterial properties (Tu et al., 2019). In this system, cystamine (CySA) was chosen to fabricate the metal-phenolic-amine-based coatings with gallic acid (GA) and CuII. GA was added as chelating agent with anti-bacterial properties, which worked synergistically with CuII in bacterial inhabitation. The integrated coating showed 99% anti-bacterial rate in vitro and 3.4 ± 0.2% occlusion rate in vivo after implantation for 30 days. In our most recent study, we further immobilized vascular endothelial growth factor (VEGF) with DA-CuII coating (Figure 2A) (Tu et al., 2020). The introduction of VEGF was found to accelerate the early-stage EC adhesion, migration, and growth, forming a new and complete endothelium on the stents. The NO flux generated by the DA-CuII coatings successfully suppressed thrombosis (Figure 2B). Scanning electron microscope images showed that after 1 and 3 months of implantation, VEGF/CuII-DA coating demonstrated greater re-endothelialization compared to 316L SS (Figure 2C). Altogether, the multiple programmed therapeutic strategies for spatiotemporal dual delivery of NO and VEGF significantly prevented thrombosis and restenosis.
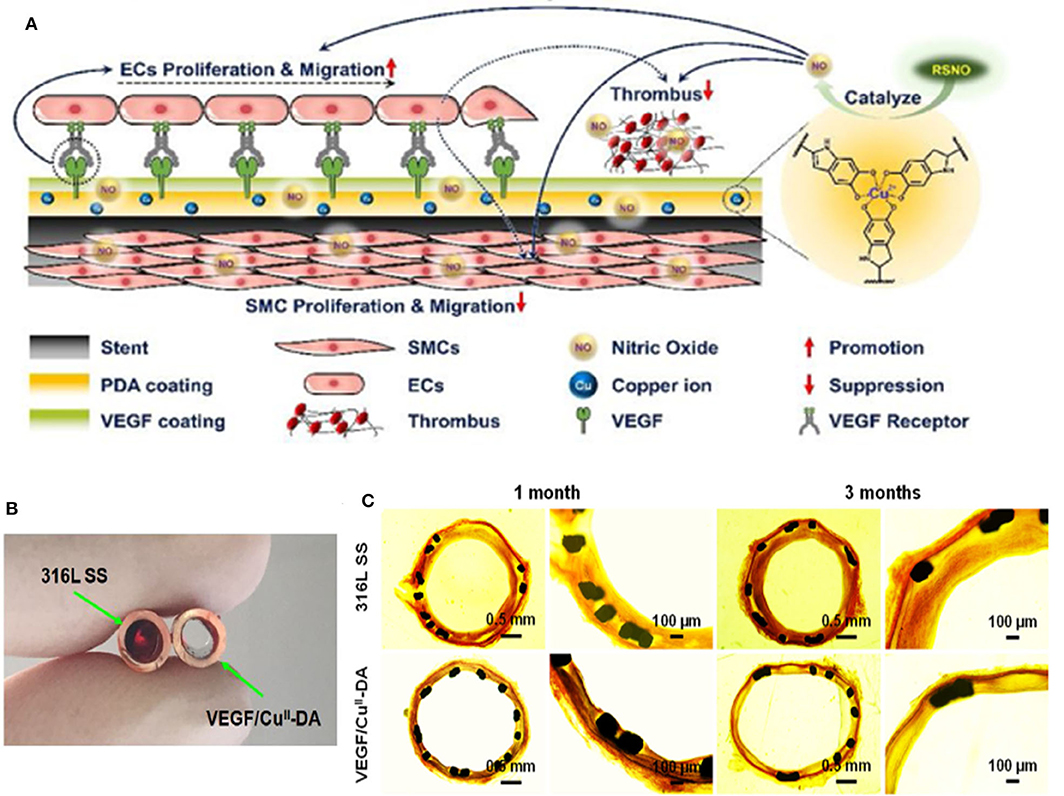
Figure 2. Cardiovascular stents with VEGF/CuII-DA coatings for enhanced re-endothelialization and reduced thrombosis. (A) Mechanism of thrombosis and restenosis prevention of VEGF/CuII-DA-coated stents. The spatiotemporal dual delivery of VEGF and NO enabled the fast re-endothelialization of stents and prevented thrombosis and restenosis. (B) Images of cross-sectioned tubing containing uncoated and VEGF/CuII-DA-coated 316L SS foils exposed for 1.5 h to blood flow in a rabbit arteriovenous shunt model. (C) Histomorphometric analysis on restenosis at bare 316L SS and VEGF/CuII-DA-coated stents using Van Gieson's staining. Image source: Tu et al. (2020).
Nevertheless, these RSNO catalytic stents face difficulty in smart control of NO production. Additionally, different stages of disease and therapeutic purposes require different amounts of NO, a flexible, extended, and controllable NO supply is therefore highly sought after.
No-Releasing and -Generating Integrating Coatings
There are substantial achievements of the anti-thrombosis and anti-restenosis therapeutic effects of NO-releasing and -generating strategies, but limitations still exist. Researchers thus make effort to combine those two strategies to supplement NO delivery. Maintaining NO release within the physiological region (0.5 to 4 × 10−10 mol × cm−2 × min−1, and preferably at the up end) can be more efficient for biomedical applications, but the NO donors such as SNAP have been shown to release NO near the low end of the physiological levels. Based on this, Handa et al. incorporated SNAP in a medical grade polymer coated with copper nanoparticles to achieve better regulation of NO release (Pant et al., 2017). This strategy not only ensured the supply of exogenous NO by SNAP, but also provided the enzymatic NO release via the reaction of copper ions on endogenous RSNOs in the blood. The Cu-SNAP had increased NO flux values to around 4.48 or 4.84 × 10−10 mol × cm−2 × min−1 when using different dosage of Cu. Additionally, significant reduction in bacterial growth and effective prevention of platelet adhesion has been achieved. Similarly, Brisbois et al. developed a multi-layered SNAP-doped polymer with a blended Se interface, and they focused on the different needs during the therapy process (Mondal et al., 2019). The first few hours after device implantation are crucial in preventing infection, since biofilm formation can occur rapidly after insertion (<24 h). The initial NO flux of generating materials may, however, be inadequate in preventing platelet activation or infection at early onslaught. Hence, in this strategy, SNAP offered initial NO release and Se interface could continuously generate NO in the presence of RSNOs. The results showed that there was a burst NO release on day 1, then the coating maintained a high and continuous NO release in the subsequent days. As a result, the enhanced initial NO flux would provide a potent anti-microbial activity acutely at the time of surgical placement, while the continuous NO generation contributed to inhibiting blood clot formation and protecting chronic or late device infections.
In addition to the above strategies to regulate the NO flux by using different coating compositions, Chandrawati et al. incorporated zinc oxide (ZnO) particles with EPT (Yang et al., 2020c). ZnO possess innate glutathione peroxidase and glycosidase activities, which allowed ZnO to catalytically decompose both endogenous (RSNOs) and exogenous (β-Gal-NONOate) donors to produce NO at physiological conditions. This strategy could solve problems such as finite pool of NO donors, short shelf life and low stability of natural enzymes in EPT. ZnO preserved its catalytic property for at least 6 months and the activity in producing NO was demonstrated. By tuning ZnO and NO prodrugs, physiologically NO levels were achieved. This method will be beneficial in long-term NO production and extra NO supplement can be achieved by on-demand NO prodrug administration, which has promising development in diverse blood-contacting devices.
In a word, the combination of NO-releasing and NO-generating chemistries within a single platform allows for a release profile that achieves the best of both worlds. However, further studies should effectively control the amount of NO flux to avoid excessive NO production and related potential toxicity.
Conclusion and Future Work
The progress of the NO-producing stent coatings are highly inspiring in the prevention of thrombosis and restenosis, and researchers have also attempted to improve the fabrication process and committed to developing simple materials for future clinical application. However, the immune reaction, inflammation, anaphylaxis, and biocompatibility of the coatings need to be further investigated. Although these problems cannot be solved temporarily, in the long term, what can be done is to promote the development of NO production strategies from those achievable directions: (1) Combination strategies of NO and other pathway regulation need to be carefully considered about the dosage ratio between the drugs to avoid the antagonism effect and ensure synergistic effect. (2) The toxicity and metabolism of the NO reaction, by-products [like N-Acetyl-D-penicillamine (NAP) and disulfide] should be taken into account. (3) Scientists should develop precise methods of NO quantitative detection in vivo to meet different needs. (4) Smart NO production system can be further developed to respond to the microenvironment changes and to produce NO flux on-demand during different pathologic process. (5) Most researchers use healthy animals to evaluate the effect of NO; however, differences between the healthy and the CVD individuals should be assessed. Researchers should also combine the effects of NO-producing coatings and the implanted materials as a whole to investigate the therapeutic effect in their corresponding disease models, which will have much more clinical significance.
In summary, NO-releasing and NO-generating strategies still have significant room for improvement, and a thorough understanding of NO production and solutions for the corresponding pathological reaction will ensure the safe clinical practices for the next generation of technological devices. We hope that this review can be helpful for the further development in this research field.
Author Contributions
XZ and HL supervised the whole review. JR and HP wrote the manuscript. YY and YL performed literature search and revised the manuscript. All the authors approved the review for publication.
Conflict of Interest
The authors declare that the research was conducted in the absence of any commercial or financial relationships that could be construed as a potential conflict of interest.
Acknowledgments
The authors acknowledge the financial support from the seed projects of the Hong Kong Innovation and Technology Support Programme (ITS/065/19), the Program of Outstanding Medical Talent of Shanghai Municipal Health Bureau (grant number 2017BR034), and the State Key Laboratory of Molecular Engineering of Polymers of Fudan University (K2019-20).
References
Alexander, G. C., Vines, J. B., Hwang, P. T. J., Kim, T., Kim, J., Brott, B. C., et al. (2016). Novel multifunctional nanomatrix reduces inflammation in dynamic conditions in vitro and dilates arteries ex vivo. ACS Appl. Mater. Inter. 8, 5178–5187. doi: 10.1021/acsami.6b00565
Brisbois, E. J., Kim, M., Wang, X., Mohammed, A., Major, T. C., Wu, J., et al. (2016). Improved hemocompatibility of multilumen catheters via nitric oxide (NO) release from S-Nitroso-N-acetylpenicillamine (SNAP) composite filled lumen. ACS Appl. Mater. Interfaces 8, 29270–29279. doi: 10.1021/acsami.6b08707
Carpenter, A. W., and Schoenfisch, M. H. (2012). Nitric oxide release: part II. Therapeutic applications. Chem. Soc. Rev. 41, 3742–3752. doi: 10.1039/c2cs15273h
Chandrawati, R., Chang, J. Y., Reinatorres, E., Jumeaux, C., Sherwood, J. M., Stamer, W. D., et al. (2017). Localized and controlled delivery of nitric oxide to the conventional outflow pathway via enzyme biocatalysis: toward therapy for glaucoma. Adv. Mater. 29:1604932. doi: 10.1002/adma.201604932
Cheng, Y., Cheng, H., Zhao, X., Xu, X., Zhuo, R., and He, F. (2015). Self-assembled micelles of a multi-functional amphiphilic fusion (MFAF) peptide for targeted cancer therapy. Polym. Chem. 6, 3512–3520. doi: 10.1039/C5PY00125K
Chuang, T., and Masters, K. S. (2009). Regulation of polyurethane hemocompatibility and endothelialization by tethered hyaluronic acid oligosaccharides. Biomaterials 30, 5341–5351. doi: 10.1016/j.biomaterials.2009.06.029
Cicha, I., Singh, R., Garlichs, C. D., and Alexiou, C. (2016). Nano-biomaterials for cardiovascular applications: clinical perspective. J. Control. Release 229, 23–36. doi: 10.1016/j.jconrel.2016.03.015
Colombo, A., Chandrasekhar, J., Aquino, M., Ong, T. K., Sartori, S., Baber, U., et al. (2019). Safety and efficacy of the COMBO bio-engineered stent in an all-comer PCI cohort: 1-year final clinical outcomes from the MASCOT post-marketing registry. Int. J. Cardiol. 283, 67–72. doi: 10.1016/j.ijcard.2019.01.053
De Visscher, G., Mesure, L., Meuris, B., Ivanova, A., and Flameng, W. (2012). Improved endothelialization and reduced thrombosis by coating a synthetic vascular graft with fibronectin and stem cell homing factor SDF-1α. Acta Biomater. 8, 1330–1338. doi: 10.1016/j.actbio.2011.09.016
Devine, R., Singha, P., and Handa, H. (2019). Versatile biomimetic medical device surface: hydrophobin coated, nitric oxide-releasing polymer for antimicrobial and hemocompatible applications. Biomater. Sci. 7, 3438–3449. doi: 10.1039/C9BM00469F
Elnaggar, M. A., Seo, S., Gobaa, S., Lim, K. S., Bae, I., Jeong, M. H., et al. (2016). Nitric oxide releasing coronary stent: a new approach using layer-by-layer coating and liposomal encapsulation. Small 12, 6012–6023. doi: 10.1002/smll.201600337
Fishbein, I., Guerrero, D. T., Alferiev, I. S., Foster, J. B., Minutolo, N. G., Chorny, M., et al. (2017). Stent-based delivery of adeno-associated viral vectors with sustained vascular transduction and iNOS-mediated inhibition of in-stent restenosis. Gene Ther. 24, 717–726. doi: 10.1038/gt.2017.82
Forstermann, U., Xia, N., and Li, H. (2017). Roles of vascular oxidative stress and nitric oxide in the pathogenesis of atherosclerosis. Circ. Res. 120, 713–735. doi: 10.1161/CIRCRESAHA.116.309326
Francis, S. H., Busch, J. L., and Corbin, J. D. (2010). cGMP-dependent protein kinases and cGMP phosphodiesterases in nitric oxide and cGMP action. Pharmacol. Rev. 62, 525–563. doi: 10.1124/pr.110.002907
Frieden, T. R., and Jaffe, M. (2018). Saving 100 million lives by improving global treatment of hypertension and reducing cardiovascular disease risk factors. J. Clin. Hypertens. 20, 208–211. doi: 10.1111/jch.13195
Fuentes, E., and Palomo, I. (2016). Role of oxidative stress on platelet hyperreactivity during aging. Life Sci. 148, 17–23. doi: 10.1016/j.lfs.2016.02.026
Furie, B., and Furie, B. C. (2008). Mechanisms of thrombus formation. N. Engl. J. Med. 359, 938–949. doi: 10.1056/NEJMra0801082
Geng, W., Zhang, T., Yang, Y., Song, D., Meng, H., Liu, Q., et al. (2013). GW24-e0802 The study of polymer-free paclitaxel-eluting stent in treatment of coronary heart disease. Heart 99:A169. doi: 10.1136/heartjnl-2013-304613.468
Gunasekera, B., Diwan, C. A., Altawallbeh, G., Kalil, H. F., Maher, S., Xu, S., et al. (2018). Functional layer-by-layer thin films of inducible nitric oxide (NO) synthase oxygenase and polyethylenimine: modulation of enzyme loading and NO-release activity. ACS Appl. Mater. Inter. 10, 7745–7755. doi: 10.1021/acsami.7b17575
Gurbel, P. A., and Bliden, K. P. (2003). Platelet activation after stenting with heparin-coated versus noncoated stents. Am. Heart J. 146:E10. doi: 10.1016/S0002-8703(03)00317-X
Hara, H., Nakamura, M., Palmaz, J. C., and Schwartz, R. S. (2006). Role of stent design and coatings on restenosis and thrombosis. Adv. Drug Deliver. Rev. 58, 377–386. doi: 10.1016/j.addr.2006.01.022
Hopkins, S. P., Pant, J., Goudie, M. J., Schmiedt, C. W., and Handa, H. (2018). Achieving long-term biocompatible silicone via covalently immobilized S-Nitroso- N-acetylpenicillamine (SNAP) that exhibits 4 months of sustained nitric oxide release. ACS Appl. Mater. Interfaces 10, 27316–27325. doi: 10.1021/acsami.8b08647
Hristov, M., Erl, W., and Weber, P. (2003). Endothelial progenitor cells mobilization, differentiation, and homing. Arterioscl. Throm. Vas. 23, 1185–1189. doi: 10.1161/01.ATV.0000073832.49290.B5
Joner, M., Finn, A. V., Farb, A., Mont, E., Kolodgie, F. D., Ladich, E., et al. (2006). Pathology of drug-eluting stents in humans: delayed healing and late thrombotic risk. J. Am. Coll. Cardiol. 48, 193–202. doi: 10.1016/j.jacc.2006.03.042
Jun, H., Yuwono, V. M., Paramonov, S. E., and Hartgerink, J. D. (2005). Enzyme-mediated degradation of peptide-amphiphile nanofiber networks. Adv. Mater. 17, 2612–2617. doi: 10.1002/adma.200500855
Kleinert, H., Pautz, A., Linker, K., and Schwarz, P. M. (2005). Regulation of the expression of inducible nitric oxide synthase. Nitric Oxide 384, 75–93. doi: 10.1016/j.niox.2010.04.007
Kraehling, J. R., and Sessa, W. C. (2017). Contemporary approaches to modulating the nitric oxide-cGMP pathway in cardiovascular disease. Circ. Res. 120, 1174–1182. doi: 10.1161/CIRCRESAHA.117.303776
Kubo, T., Imanishi, T., Kitabata, H., Kuroi, A., Ueno, S., Yamano, T., et al. (2008). Comparison of vascular response after sirolimus-eluting stent implantation between patients with unstable and stable angina pectoris: a serial optical coherence tomography study. Cardiovasc. Imaging 1, 475–484. doi: 10.1016/j.jcmg.2008.03.012
Kushwaha, M., Anderson, J. M., Bosworth, C. A., Andukuri, A., Minor, W. P., Lancaster, J. R., et al. (2010). A nitric oxide releasing, self assembled peptide amphiphile matrix that mimics native endothelium for coating implantable cardiovascular devices. Biomaterials 31, 1502–1508. doi: 10.1016/j.biomaterials.2009.10.051
Li, X., Qiu, H., Gao, P., Yang, Y., Yang, Z., and Huang, N. (2018). Synergetic coordination and catecholamine chemistry for catalytic generation of nitric oxide on vascular stents. NPG Asia Mater. 10, 482–496. doi: 10.1038/s41427-018-0052-3
Lundberg, J. O., Gladwin, M. T., and Weitzberg, E. (2015). Strategies to increase nitric oxide signalling in cardiovascular disease. Nat. Rev. Drug Discov. 14, 623–641. doi: 10.1038/nrd4623
Ma, X., Hibbert, B., Dhaliwal, B., Seibert, T., Chen, Y., Zhao, X., et al. (2010). Delayed re-endothelialization with rapamycin-coated stents is rescued by the addition of a glycogen synthase kinase-3β inhibitor. Cardiovasc. Res. 86, 338–345. doi: 10.1093/cvr/cvq047
Mahara, A., Somekawa, S., Kobayashi, N., Hirano, Y., Kimura, Y., Fujisato, T., et al. (2015). Tissue-engineered acellular small diameter long-bypass grafts with neointima-inducing activity. Biomaterials 58, 54–62. doi: 10.1016/j.biomaterials.2015.04.031
Major, T. C., Brant, D. O., Reynolds, M. M., Bartlett, R. H., Meyerhoff, M. E., Handa, H., et al. (2010). The attenuation of platelet and monocyte activation in a rabbit model of extracorporeal circulation by a nitric oxide releasing polymer. Biomaterials 31, 2736–2745. doi: 10.1016/j.biomaterials.2009.12.028
McCarthy, C. W., Guillory, R. J., Goldman, J., and Frost, M. C. (2016). Transition metal mediated release of nitric oxide (NO) from S-Nitroso-N-acetylpenicillamine (SNAP): potential applications for endogenous release of NO on the surface of stents via corrosion products. Acs Appl. Mater. Interfaces 16, 10128–10135. doi: 10.1021/acsami.6b00145
Mcfadden, E. P., Stabile, E., Regar, E., Cheneau, E., Ong, A. T. L., Kinnaird, T., et al. (2004). Late thrombosis in drug-eluting coronary stents after discontinuation of antiplatelet therapy. Lancet 364, 1519–1521. doi: 10.1016/S0140-6736(04)17275-9
McIlhenny, S., Zhang, P., Tulenko, T., Comeau, J., Fernandez, S., Policha, A., et al. (2013). eNOS transfection of adipose-derived stem cells yields bioactive nitric oxide production and improved results in vascular tissue engineering. J. Tissue Eng. Regenerat. Med. 9, 1277–1285. doi: 10.1002/term.1645
Mel, A. D., Murad, F., and Seifalian, A. M. (2011). Nitric oxide: a guardian for vascular grafts? Chem. Rev. 111, 5742–5767. doi: 10.1021/cr200008n
Midgley, A. C., Wei, Y., Li, Z., Kong, D., and Zhao, Q. (2019). Nitric-oxide-releasing biomaterial regulation of the stem cell microenvironment in regenerative medicine. Adv. Mater. 32:1805818. doi: 10.1002/adma.201805818
Mondal, A., Douglass, M., Hopkins, S. P., Singha, P., Tran, M., Handa, H., et al. (2019). Multifunctional S-Nitroso-N-acetylpenicillamine-incorporated medical-grade polymer with selenium interface for biomedical applications. ACS Appl. Mater. Interfaces 11, 34652–34662. doi: 10.1021/acsami.9b10610
Naghavi, N., De Mel, A., Alavijeh, O. S., Cousins, B. G., and Seifalian, A. M. (2013). Nitric oxide donors for cardiovascular implant applications. Small 9, 22–35. doi: 10.1002/smll.201200458
Nakamura, K., Keating, J., and Edelman, E. R. (2016). Pathology of endovascular stents. Interv. Cardiol. Clin. 5, 391–403. doi: 10.1016/j.iccl.2016.02.006
Ohkubo, K., Kobayashi, Y., Nakamura, Y., and Miyazaki, A. (2011). Incidence of side-effects of dual antiplatelet therapy with clopidogrel and aspirin after coronary stent implantation. Cardiovasc. Interv. Ther. 26, 33–37. doi: 10.1007/s12928-010-0031-2
Pacelli, S., Basu, S., Whitlow, J., Chakravarti, A. R., Acosta, F. M., Varshney, A., et al. (2017). Strategies to develop endogenous stem cell recruiting bioactive materials for tissue repair and regeneration. Adv. Drug Deliv. Rev. 120, 50–70. doi: 10.1016/j.addr.2017.07.011
Palmerini, T., Benedetto, U., Biondizoccai, G., Riva, D. D., Bacchireggiani, L., Smits, P. C., et al. (2015). Long-term safety of drug-eluting and bare-metal stents: evidence from a comprehensive network meta-analysis. J. Am. Coll. Cardiol. 65, 2496–2507. doi: 10.1016/j.jacc.2015.04.017
Pan, G., Liu, S., Zhao, X., Zhao, J., Fan, C., and Cui, W. (2015). Full-course inhibition of biodegradation-induced inflammation in fibrous scaffold by loading enzyme-sensitive prodrug. Biomaterials 53, 202–210. doi: 10.1016/j.biomaterials.2015.02.078
Pant, J., Goudie, M. J., Hopkins, S. P., Brisbois, E. J., and Handa, H. (2017). Tunable nitric oxide release from S-Nitroso-N-acetylpenicillamine via catalytic copper nanoparticles for biomedical applications. ACS Appl. Mater. Inter. 11, 15254–15264. doi: 10.1021/acsami.7b01408
Park, D. W., Kim, Y. H., Yun, S. C., Kang, S. J., Lee, S. W., Lee, C. W., et al. (2010). Comparison of zotarolimus-eluting stents with sirolimus- and paclitaxel-eluting stents for coronary revascularization: the ZEST (comparison of the efficacy and safety of zotarolimus-eluting stent with sirolimus-eluting and paclitaxel-eluting stent for coronary lesions) randomized trial. J. Am. Coll. Cardiol. 56, 1187–1195. doi: 10.1016/j.jacc.2010.03.086
Poh, C. K., Shi, Z., Lim, T. Y., Neoh, K. G., and Wang, W. (2010). The effect of VEGF functionalization of titanium on endothelial cells in vitro. Biomaterials 31, 1578–1585. doi: 10.1016/j.biomaterials.2009.11.042
Saito, G., Swanson, J. A., and Lee, K. D. (2003). Drug delivery strategy utilizing conjugation via reversible disulfide linkages: role and site of cellular reducing activities. Adv. Drug Deliver. Rev. 55, 199–215. doi: 10.1016/S0169-409X(02)00179-5
Scott, R. A., and Panitch, A. (2014). Macromolecular approaches to prevent thrombosis and intimal hyperplasia following percutaneous coronary intervention. Biomacromolecules 15, 2825–2832. doi: 10.1021/bm5007757
Seabra, A. B., Justo, G. Z., and Haddad, P. S. (2015). State of the art, challenges and perspectives in the design of nitric oxide-releasing polymeric nanomaterials for biomedical applications. Biotechnol. Adv. 33, 1370–1379. doi: 10.1016/j.biotechadv.2015.01.005
Shin, Y. M., Lee, Y. B., Kim, S. J., Kang, J. K., Park, J., Jang, W., et al. (2012). Mussel-inspired immobilization of vascular endothelial growth factor (VEGF) for enhanced endothelialization of vascular grafts. Biomacromolecules 13, 2020–2028. doi: 10.1021/bm300194b
Stone, G. W. (2016). Bioresorbable vascular scaffolds. JACC Cardiovasc. Inte. 9:575. doi: 10.1016/j.jcin.2016.01.012
Stone, G. W., Grines, C. L., Cox, D. A., Garcia, E., Tcheng, J. E., Griffin, J. J., et al. (2002). Comparison of angioplasty with stenting, with or without abciximab, in acute myocardial infarction. N. Engl. J. Med. 346, 957–966. doi: 10.1056/NEJMoa013404
Thomas, D. D. (2015). Breathing new life into nitric oxide signaling: a brief overview of the interplay between oxygen and nitric oxide. Redox Biol. 5, 225–233. doi: 10.1016/j.redox.2015.05.002
Tu, Q., Shen, X., Liu, Y., Zhang, Q., Zhao, X., Maitz, M. F., et al. (2019). A facile metal–phenolic–amine strategy for dual-functionalization of blood-contacting devices with antibacterial and anticoagulant properties. Mater. Chem. Front. 3, 265–275. doi: 10.1039/C8QM00458G
Tu, Q., Zhao, X., Liu, S., Li, X., Zhang, Q., Yu, H., et al. (2020). Spatiotemporal dual-delivery of therapeutic gas and growth factor for prevention of vascular stent thrombosis and restenosis. Appl. Mater. Today. 19:100546. doi: 10.1016/j.apmt.2019.100546
Wang, Z., Lu, Y., Qin, K., Wu, Y., Tian, Y., Wang, J., et al. (2015). Enzyme-functionalized vascular grafts catalyze in-situ release of nitric oxide from exogenous NO prodrug. J. Control. Release 210, 179–188. doi: 10.1016/j.jconrel.2015.05.283
Wei, Y., Ji, Y., Xiao, L., Lin, Q., Xu, J., Ren, K., et al. (2013). Surface engineering of cardiovascular stent with endothelial cell selectivity for in vivo re-endothelialisation. Biomaterials 34, 2588–2599. doi: 10.1016/j.biomaterials.2012.12.036
Weng, Y., Song, Q., Zhou, Y., Zhang, L., Wang, J., Chen, J., et al. (2011). Immobilization of selenocystamine on TiO2 surfaces for in situ catalytic generation of nitric oxide and potential application in intravascular stents. Biomaterials 32, 1253–1263. doi: 10.1016/j.biomaterials.2010.10.039
Winther, A. K., Fejerskov, B., Ter Meer, M., Jensen, N. B. S., Dillion, R., Schaffer, J. E., et al. (2018). Enzyme prodrug therapy achieves site-specific, personalized physiological responses to the locally produced nitric oxide. ACS Appl. Mater. Inter. 10, 10741–10751. doi: 10.1021/acsami.8b01658
Yang, T., Du, Z., Qiu, H., Gao, P., Zhao, X., Wang, H., et al. (2020a). From surface to bulk modification: plasma polymerization of amine-bearing coating by synergic strategy of biomolecule grafting and nitric oxide loading. Bioac. Mater. 5, 17–25. doi: 10.1016/j.bioactmat.2019.12.006
Yang, T., Fruergaard, A. S., Winther, A. K., Zelikin, A. N., and Chandrawati, R. (2020b). Zinc oxide particles catalytically generate nitric oxide from endogenous and exogenous prodrugs. Small 5:1906744. doi: 10.1002/smll.201906744
Yang, T., Zelikin, A. N., and Chandrawati, R. (2017). Progress and promise of nitric oxide-releasing platforms. Adv. Sci. 5:1701043. doi: 10.1002/advs.201701043
Yang, Y., Gao, P., Wang, J., Tu, Q., Bai, L., Xiong, K., et al. (2020c). Endothelium-mimicking multifunctional coating on cardiovascular stents via a stepwise metal-catechol-(amine) surface engineering strategy. Research 2020:9203906. doi: 10.34133/2020/9203906
Yang, Z., Yang, Y., Zhang, L., Xiong, K., Li, X., Zhang, F., et al. (2018). Mussel-inspired catalytic selenocystamine-dopamine coatings for long-term generation of therapeutic gas on cardiovascular stents. Biomaterials 178, 1–10. doi: 10.1016/j.biomaterials.2018.06.008
Yoon, J. H., Wu, C. J., Homme, J., Tuch, R. J., Wolff, R. G., Topol, E. J., et al. (2002). Local delivery of nitric oxide from an eluting stent to inhibit neointimal thickening in a porcine coronary injury model. Yonsei Med. J. 43, 242–251. doi: 10.3349/ymj.2002.43.2.242
Yusuf, S., Joseph, P., Rangarajan, S., Islam, S., Mente, A., Hystad, P., et al. (2020). Modifiable risk factors, cardiovascular disease, and mortality in 155 722 individuals from 21 high-income, middle-income, and low-income countries (PURE): a prospective cohort study. Lancet 395, 795–808. doi: 10.1016/S0140-6736(19)32008-2
Zhang, F., Zhang, Q., Li, X., Huang, N., Zhao, X., and Yang, Z. (2019). Mussel-inspired dopamine-CuII coatings for sustained in situ generation of nitric oxide for prevention of stent thrombosis and restenosis. Biomaterials 194, 117–129. doi: 10.1016/j.biomaterials.2018.12.020
Zhao, P., Metcalf, M., and Bunnett, N. W. (2014). Biased signaling of protease-activated receptors. Front. Endocrinol. 5:67. doi: 10.3389/fendo.2014.00067
Zhao, Q., Zhang, J., Song, L., Ji, Q., Yao, Y., Cui, Y., et al. (2013). Polysaccharide-based biomaterials with on-demand nitric oxide releasing property regulated by enzyme catalysis. 34, 8450–8458. doi: 10.1016/j.biomaterials.2013.07.045
Keywords: cardiovascular stents, surface coating, nitric oxide, restenosis, thrombosis
Citation: Rao J, Pan Bei H, Yang Y, Liu Y, Lin H and Zhao X (2020) Nitric Oxide-Producing Cardiovascular Stent Coatings for Prevention of Thrombosis and Restenosis. Front. Bioeng. Biotechnol. 8:578. doi: 10.3389/fbioe.2020.00578
Received: 26 March 2020; Accepted: 12 May 2020;
Published: 24 June 2020.
Edited by:
Chao Zhao, University of Alabama, United StatesReviewed by:
Francesca Taraballi, Houston Methodist Research Institute, United StatesFederico Vozzi, Institute of Clinical Physiology (CNR), Italy
Copyright © 2020 Rao, Pan Bei, Yang, Liu, Lin and Zhao. This is an open-access article distributed under the terms of the Creative Commons Attribution License (CC BY). The use, distribution or reproduction in other forums is permitted, provided the original author(s) and the copyright owner(s) are credited and that the original publication in this journal is cited, in accordance with accepted academic practice. No use, distribution or reproduction is permitted which does not comply with these terms.
*Correspondence: Haodong Lin, aGFvZG9uZ2xpbiYjeDAwMDQwO2hvdG1haWwuY29t; Xin Zhao, eGluLnpoYW8mI3gwMDA0MDtwb2x5dS5lZHUuaGs=