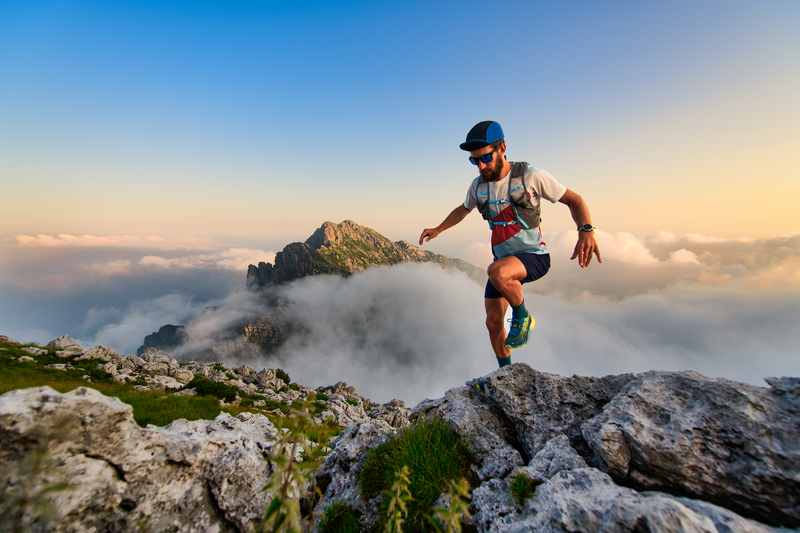
95% of researchers rate our articles as excellent or good
Learn more about the work of our research integrity team to safeguard the quality of each article we publish.
Find out more
ORIGINAL RESEARCH article
Front. Bioeng. Biotechnol. , 07 April 2020
Sec. Bioprocess Engineering
Volume 8 - 2020 | https://doi.org/10.3389/fbioe.2020.00305
This article is part of the Research Topic 2nd Synthetic Biology Forum: System and Synthetic Biology for Biofuels and Bioproducts View all 20 articles
Bacitracin is a broad-spectrum veterinary antibiotic that widely used in the fields of veterinary drug and feed additive. S-Adenosylmethionine (SAM) is a critical factor involved in many biochemical reactions, especially antibiotic production. However, whether SAM affects bacitracin synthesis is still unknown. Here, we want to analyze the relationship between SAM supply and bacitracin synthesis, and then metabolic engineering of SAM synthetic pathway for bacitracin production in Bacillus licheniformis. Firstly, our results implied that SAM exogenous addition benefited bacitracin production, which yield was increased by 12.13% under the condition of 40 mg/L SAM addition. Then, SAM synthetases and Methionine (Met) synthetases from B. licheniformis, Corynebacterium glutamicum, and Saccharomyces cerevisiae were screened and overexpressed to improve SAM accumulation, and the combination of SAM synthetase from S. cerevisiae and Met synthetase from B. licheniformis showed the best performance, and 70.12% increase of intracellular SAM concentration (31.54 mg/L) and 13.08% increase of bacitraicn yield (839.54 U/mL) were achieved in resultant strain DW2-KE. Furthermore, Met transporters MetN and MetP were, respectively, identified as Met exporter and importer, and bacitracin yield was further increased by 5.94% to 889.42 U/mL via deleting metN and overexpressing metP in DW2-KE, attaining strain DW2-KENP. Finally, SAM nucleosidase gene mtnN and SAM decarboxylase gene speD were deleted to block SAM degradation pathways, and bacitracin yield of resultant strain DW2-KENPND reached 957.53 U/mL, increased by 28.97% compared to DW2. Collectively, this study demonstrated that SAM supply served as the critical role in bacitracin synthesis, and a promising strain B. licheniformis DW2-KENPND was attained for industrial production of bacitracin.
Bacitracin, an important peptide antibiotic that consists of 11 kinds of amino acids, is mainly produced by B. licheniformis and Bacillus subtilis. Bacitracin biosynthetase gene cluster in B. licheniformis spans over 45 kd of DNA, which containing four genes, bacT, bacA, bacB and back. Gene bacT encodes thioesterase, and the relationship between bacitracin synthesis with bacT is unclear. BacA is responsible for activating and polymerizing five kinds of amino acids (Ile, Cys, Leu, D-Glu, and Ile) at bacitracin tail, BacB and BacC are responsible for activating and polymerizing seven kinds of amino acids in heptapeptide loop (Wang D. et al., 2017). Bacitracin owns the advantages of wide antimicrobial spectrum, rapid excretion rate, low absorption of livestock and poultry, not easy to produce resistance etc., and it inhibits the synthesis of cell wall of most gram-positive and a few of gram-negative bacteria, thus, bacitracin is widely used as the feed additive in feed industry, however, the low-yield has hindered its application (Fang et al., 2017).
With the development of synthetic biology and metabolic engineering, many elements and strategies have been developed for antibiotic metabolic engineering breeding (Zhou et al., 2017). The Leu-responsive regulatory protein Lrp has been engineered to improve erythromycin and actinorhodin production in Streptomyces (Liu et al., 2017), and deletion of phosphorus metabolism regulator gene phop was proven to be beneficial for avermection, pimaricin, etc., production (Sola-Landa et al., 2003; Martin et al., 2017; Mendes et al., 2007). In addition, precursor supply served as the critical role in secondary metabolite synthesis (Wohlleben et al., 2012). Branched chain amino acid (BCAA) served as the critical role in erythromycin production, and strengthening BCAA supplies led to a 41% increase of erythromycin yield, via deleting lrp and overexpressing BCAA transporter SACE_5387 (Liu et al., 2017). Moreover, acety-CoA carboxylase was overexpressed to improve malonyl-CoA supply, which further led to a 43% increase of surfactin production, reached 13.37 g/L (Wang et al., 2019). In addition, efficient use of substrate is also critical for metabolite production. Previously, maltose ABC transporter MalEFG was strengthened to improve utilization rate of corn starch, resulting in a 3.3-fold increase of ivermectin production in Streptomyces (Li et al., 2010). Generally, S-adenosylmethionine (SAM) served as the critical role in antibiotic biosynthesis, and strengthening SAM supply has been proven as an efficient strategy for lincomycin, avermectin, novobiovin, cephalosporin, etc., production (Zhao et al., 2010). However, whether SAM supply affects bacitracin synthesis has not been investigated in Bacillus.
S-Adenosylmethionine is one of the most widely used cofactor for group transfer reactions that involved in various metabolic processes, and it serves as the main methyl donor for DNA methylation, protein and secondary metabolite syntheses (Ruan et al., 2019), and SAM-dependent methylation is also the critical step for metabolite production. In addition, SAM addition improves antibiotic synthesis might via activating the transcription of pathway-specific regulatory genes or increasing the auto-phosphorylation of regulatory kinase (Kim et al., 2003). Generally, SAM is synthesized from aspartate, which generated from glucose via glycolytic and tricarboxylic acid (TCA) cycle, and methionine (Met) serves as the direct precursor for SAM biosynthesis (Ruan et al., 2019). Recently, several strategies have been conducted to improve SAM production, including: (i) enhancing activity of SAM synthetase, (ii) deleting cystathionin–β-synthase, (iii) releasing the feedback inhibitions of SAM to SAM synthetase and methylenetetrahydrofolate reductase (Chu et al., 2013). In addition, SAM titer was significantly enhanced via coupling SAM synthetic pathway with TCA cycle in Bacillus amyloliquefaciens (Ruan et al., 2019).
Bacillus licheniformis DW2 is an industrial strain for bacitracin production (Cai et al., 2019b), and several metabolic engineering approaches have been developed to improve bacitracin production. The synthetic pathways of Lysine (Lys) and Ornithine (Orn) were strengthened, which led to 28.95 and 16.5% increases of bacitracin yields, respectively (Wu et al., 2019; Yu et al., 2019). BCAA transporters BrnQ and YdhG were engineered to improve intracellular BCAA accumulations for bacitraicn synthesis (Li et al., 2018; Zhu et al., 2018). Additionally, the main regulators in carbon, nitrogen and phosphorus metabolisms were engineered, which led to a 35.72% increase of bacitraicn yield (Cai et al., 2019b). Due to the importance of SAM supply on antibiotic synthesis, in this study, we want to improve bacitracin production via rewiring SAM synthetic pathways, including in SAM synthetic, degradation and Met transportation pathways. Our results demonstrated that SAM supply served as a critical role on bacitracin synthesis, and this study provided a promising strain for industrial production of bacitracin.
The strains and plasmids used in this research were listed in Table 1. B. licheniformis DW2 acted as the original strain for constructing recombinants (Zhu et al., 2019), and Escherichia coli DH5α served as the host for vector construction. The plasmid T2(2)-Ori was applied for gene deletion, promoter replacement and gene integration in B. licheniformis, pHY300PLK was applied for constructing gene expression vector. All primers used in this research were provided in Supplementary Table S1.
Luria-Bertani (LB) medium was served as the basic medium for strain cultivation, and corresponding antibiotics (20 mg/L kanamycin, 20 mg/L tetracycline or 50 mg/L ampicillin) were added when necessary. The seed culture was inoculated in a 250 mL flask containing 20 mL LB medium for 6 h, and transferred (1 mL) into bacitracin production medium (10% soybean meal, 4.5% corn starch, 0.1% (NH4)2SO4, 0.6% CaCO3, natural pH), and then cultivated at 37°C, 230 r/min for 48 h. In order to analyze the function roles of Met transporters MetN and MetP, ME medium (20 g/L glucose, 20 g/L sodium glutamate, 10 g/L sodium citrate, 7 g/L NH4Cl, 0.5 g/L K2HPO4 3H2O, 0.5 g/L MgSO4 7H2O, 0.04 g/L FeCl3cdot6H2O, 0.104 g/L MnSO4cdotH2O, 0.15 g/L CaCl2 2H2O, pH 7.2) was applied (Birrer et al., 1994). All the fermentation experiments were repeated at least three times.
The gene expression vector was constructed according to our previous research (Zhu et al., 2019), based on pHY300PLK, and SAM synthetase MetK from B. licheniformis expression vector pHY-metKBl was served as an example. Briefly, P43 promoter from B. subtilis 168, gene metK and amyL terminator from B. licheniformis DW2 were amplified by corresponding primers (Supplementary Table S1), and fused by Splicing Overlap Extension (SOE)-PCR. The fused fragment was inserted into pHY300PLK at restriction sites EcoRI/XbaI, diagnostic PCR and DNA sequence confirmed that gene expression vector was constructed successfully, named as pHY-metKBl. Similarly, other gene expression vectors were attained by the same method.
The method for gene deletion in B. licheniformis was referred to our previously reported research (Cai et al., 2019b), and Met transporter gene metN deletion strain was served as an example. In brief, the upstream and downstream homology arms of metN were amplified from B. licheniformis DW2, and fused by SOE-PCR. The fused fragment was inserted into T2(2)-Ori at restriction sites SacI/XbaI, diagnostic PCR and DNA sequence confirmed that gene deletion vector was constructed successfully, named T2-metN. Then, T2-metN was transferred into B. licheniformis DW2 via electroporation, and the positive transformants were cultivated in LB medium with 20 mg/L kanamycin at 45°C, and sub-cultured for three generations. Then, transferred into LB medium and sub-cultured for six generations at 37°C. The gene metN deletion strain was attained via homologous double crossover, diagnostic PCR and DNA sequence confirmed that metN deletion strain was constructed successfully, named as DW2ΔmetN.
To construct the strain which overexpressing SAM synthetase SAM2 from Saccharomyces cerevisiae, gene SAM2 mediated by P43 promoter was integrated into the chromosome of B. licheniformis DW2, following to previously reported protocol (Cai et al., 2018), diagnostic PCR and DNA sequence confirmed that the gene integrated overexpression strain was constructed successfully.
The method for gene promoter replacement in B. licheniformis was referred to our previously reported research (Wu et al., 2019), and the procedure for promoter of Met synthetase MetH replaced by PbacA, a strong promoter that has been proven in our previous research (Shi et al., 2019), was served as an example. Briefly, the upstream and downstream homology arms of PmetH, promoter PbacA, were amplified to form T2-PbacA-PmetH, and promoter PmetH was replaced by PbacA via homologous double crossover, which procedure was the same as that of gene deletion.
Bacitracin yield was measured by Agilent 1260 high performance liquid chromatography (HPLC), equipped with C18 column (ZORBAX SB-C18). Cell biomass was determined by dilution coating method (Cai et al., 2019a). SAM concentration was determined by HPLC (Ruan et al., 2019), and concentrations of intracellular and extracellular amino acids were measured by gas chromatography (GC), according to our previously reported research (Cai et al., 2019b). The transcriptional levels of bacirtacin synthetase gene cluster were determined by RT-qPCR, and gene 16S rDNA was served as the reference for data normalization (Cai et al., 2017).
All data were conducted to analyze the variance at P < 0.05 and P < 0.01, and a t-test was applied to compare the mean values using the software package Statistica 6.0, and bars represented the standard deviations, ∗P < 0.05; and ∗∗P < 0.01 indicated the significance levels between recombinant strains and control (Cai et al., 2018).
Previously, SAM was proven to own the critical role in the syntheses numerous kinds of antibiotic (Zhao et al., 2010), however, whether SAM supply affect bacitracin synthesis has not been clarified in Bacillus. Here, different concentrations of SAM was, respectively, added into bacitracin production medium at 24 h (The beginning period of bacitracin synthesis), and our results in Figure 1A implied that exogenous SAM addition benefited bacitracin production, and the maximum bacitracin yield (832.45 U/mL) was attained under the condition of 40 mg/L SAM addition, increased by 12.13% compared to control (742.43 U/mL). Transcriptional levels of bacitracin synthetase genes bacT, bacA, bacB and bacC were measured under optimal condition, and our results implied that SAM addition improved transcription of bacitracin synthetase, increased by 1. 43-, 1. 34-, 1. 31-, and 1.29-fold, respectively (Figure 1B). In addition, our results demonstrated that SAM addition have no effects on cell biomass (Supplementary Figure S1). Moreover, the concentrations of intracellular SAM and Met were determined before (24 h) and after (36 h) SAM addition. Our results implied that the concentrations of intracellular SAM was 7.54 mg/L at 24 h, and which was increased to 17.29 mg/L at 30 h when SAM addition, increased by 53.69% compared to the control group (11.25 mg/L). While, Met concentrations showed no significant differences between two groups (Supplementary Figure S2). Taken together, these above results demonstrated that SAM served as the key role in bacitracin synthesis, and strengthening SAM supply was conducive to bacitracin production.
Figure 1. Effects of exogenous SAM addition on bacitracin production. (A) Effects of different concentrations of SAM (20, 30, 40, 50, and 60 mg/L) additions on bacitracin yield, (B) Effects of SAM addition on the transcriptional levels of bacitracin synthetase genes bacT, bacA, bacB, and bacC. ∗P < 0.05 and ∗∗P < 0.01 indicate the significance levels between recombinant strains and control strain.
S-Adenosylmethionine synthetase served as the key role in SAM formation from Met and ATP (Kanai et al., 2017), and overexpression of SAM synthetase benefited SAM production (Ruan et al., 2019). The SAM synthetic pathway of B. licheniformis was showed in Figure 2, here, SAM synthetases from B. licheniformis, Corynebacterium glutamicum, S. cerevisiae were strengthened in B. licheniformis DW2, attaining recombinant strains DW2/pHY-MetKBl, DW2/pHY-MetKCg and DW2/pHY-SAM2, respectively.
Figure 2. Metabolic engineering of SAM synthetic and degradation, Met transportation pathways for SAM accumulation and bacitracin synthesis in B. licheniformis.
Then, all these recombinant strains were cultivated in bacitracin production medium, as well as controls DW2 and DW2/pHY300. Based on our results of Figure 3A, bacitracin yields produced by DW2/pHY-MetKBl, DW2/pHY-MetKCg and DW2/pHY-SAM2 were 724.32 U/mL, 743.42 U/mL and 765.31 U/mL, increased by 8.40, 11.24, and 14.51% compared with that of DW2/pHY300 (668.27 U/mL). In addition, cell biomasses of these strains showed no significant differences, and bacitracin produced by per cell were 1.95∗10–8 U/CFU, 2.00∗10–8 U/CFU and 2.05 U∗10–8/CFU, 7.97%, 10.46 and 13.43% higher than that of DW2/pHY300 (1.81∗10–8 U/CFU), respectively. Furthermore, SAM2 expression cassette was inserted into chromosome of DW2, attaining SAM2 integrated overexpression strain DW2-K. Based on the results of Figure 3B, bacitracin yield of DW2-K reached 795.42 U/mL, increased by 7.14% compared to DW2 (742.43 U/mL). The bacitracin produced by per cell was 1.98∗10–8 U/CFU, increased by 6.30% (1.84∗10–8 U/CFU). The concentration of intracellular SAM (36 h) in DW2-K was 26.32 mg/L, 41.96% higher than that of DW2 (18.54 mg/L). Additionally, transcriptional levels of genes bacT, bacA, bacB and bacC were also increased significantly (Figure 3C), as the increase of SAM accumulation in DW2-K.
Figure 3. Effects of strengthening SAM synthetase expression on SAM accumulation and bacitracin production. (A) Effects of SAM synthetase overexpression on bacitracin yield and cell biomass, (B) Effects of integrated overexpression of SAM2 from S. cerevisiae on bacitraicn yield, cell biomass and SAM accumulation, (C) Effects of SAM2 overexpression on the transcriptional levels of bacitracin synthetase genes bacT, bacA, bacB, and bacC. ∗P < 0.05 and ∗∗P < 0.01 indicate the significance levels between recombinant strains and control strain.
Acting as the precursor for SAM synthesis, Met supply might affect the synthetic efficiency of SAM, which further affect bacitracin production. Here, Met synthetases from B. licheniformis (MetH), C. glutamicum (MetH), S. cerevisiae (Met6) were strengthened in DW2-K, attaining strains DW2-K/pHY-MetHBl, DW2-K/pHY-MetHCg and DW2-K/pHY-Met6, respectively. Our results implied that overexpression of MetH from B. licheniformis benefited bacitracin synthesis, however, strengthening Met synthases from C. glutamicum and S. cerevisiae has no positive effect on bacitracin production. After that, the promoter of metH of DW2-K was replaced by bacitracin synthetase cluster promoter PbacA, a proven strong promoter that has been confirmed in our previous research (Shi et al., 2019), attaining MetH overexpression strain DW2-KE. Based on our results, bacitracin produced by DW2-KE reached 839.54 U/mL, increased by 5.55 and 13.08% compared to DW2-K and DW2, respectively. Bacitracin produced by per cell was 2.03∗10–8 U/mL, increased by 11.89 and 3.51% (Figure 4A). In addition, the intracellular Met and SAM concentrations (36 h) were 39.54 mg/L and 31.54 mg/L, increased by 34.40 and 19.83% compared with those of DW2-K (29.42 mg/L and 26.32 mg/L), respectively (Figure 4B). Taken together, all these above results demonstrated that strengthening Met synthetic pathway benefited SAM accumulation and bacitracin production.
Figure 4. Effects of methionine synthetase overexpression on bacitracin production and cell biomass. (A) Bacitracin yield and cell biomass, (B) The concentrations of intracellular SAM and Met. ∗P < 0.05 and ∗∗P < 0.01 indicate the significance levels between recombinant strains and control strain.
Apart from metabolic pathway, engineering transportation pathway is also regarded as an efficient approach to improve target metabolite production. Previously, amino acid transporters LysE, BrnFE, and TcyP have been identified and engineered for Lys, Val and Cys production (Chen et al., 2015; Ohtsu et al., 2015; Dong et al., 2016), and deleting Lys transporter LysE and BCAA permease YhdG were also proven to be beneficial for bacitracin syntheses (Li et al., 2018; Wu et al., 2019). Here, MetN and MetP were annotated as Met transporters in B. licheniformis DW2, however, transportation modules of them have not been clarified, nor their roles on bacitracin production.
To elucidate the transportation modes of these two transporters, genes metN and metP were deleted and overexpressed in DW2, attaining DW2△metN, DW2△metP, DW2/pHY-MetN and DW2/pHY-MetP, respectively. Then, these strains were cultivated in ME medium, and the concentrations of intracellular and extracellular Met were measured after 24 h cultivation. Based on the results of Figure 5A, strengthening MetP expression benefited Met import, while the concentration of intracellular Met was dropped significantly in MetN overexpression strain, and vice versa. Collectively, these above results suggested that MetN and MetP might act as the Met exporter and importer in B. licheniformis DW2, respectively.
Figure 5. Identifying and engineering Met transporters for enhancement production of bacitracin. (A) The extracellular and intracellular of Met in ME medium, (B) Effects of deletion and overexpression of metN and metP on bacitracin production and cell biomass, (C) The concentrations of intracellular Met and SAM, (D) The concentrations of extracellular Met and SAM, (E) Effects of metN deletion and metP overexpression on bacitracin yields and intracellular SAM concentrations. ∗P < 0.05 and ∗∗P < 0.01 indicate the significance levels between recombinant strains and control strain.
Then, all these strains were cultivated in bacitracin production medium, and further results implied that metN deletion and metP overexpression benefited bacitracin production, which yields were increased by 9.55 and 8.05%, meanwhile, bacitracin yields were decreased by 9.58 and 27.73% in metN overexpression and metP deletion strains, compared to DW2 and DW2/pHY300, respectively (Figure 5B). Moreover, the intracellular Met concentrations were increased by 24.71 and 13.71% in DW2△metN and DW2/pHY-MetP, which concentrations were dropped by 18.17 and 15.59% in DW2△metP and DW2/pHY-MetN, indicated that metN deletion and MetP overexpression benefited intracellular Met accumulation (Figures 5C,D). Since Met served as the critical role in SAM synthesis and accumulation, the transporters MetN and MetP were confirmed to act as Met exporter and importer, respectively.
To further improve bacitracin synthesis capability of DW2-KE, gene metN was deleted to attain DW2-KEN, and metP was further overexpressed via promoter replacement, attaining strain DW2-KENP. Bacitracin fermentation results implied that 889.42 U/mL bacitracin was produced by DW2-KENP, increased by 19.80 and 5.94%, compared to DW2 and DW2-KE, and the specific bacitracin yield of DW2-KENP were increased by 20.31 and 5.71%, respectively. Meanwhile, the intracellular SAM concentration was increased to 37.98 mg/L, increased by 104.85 and 20.42%, respectively (Figure 5E).
In the above work, although concentration of intracellular SAM was enhanced significantly, the accumulated SAM might also be transformed into byproducts S-D-Ribosyl-L-homocysteine and S-Adenosyl-methioninamine, under the catalyses of SAM nucleosidase MtnN and SAM decarboxylase SpeD, respectively, which was not conducive to SAM accumulation. Here, genes mtnN and speD were deleted in DW2-KENP, resulting in DW2-KENP△mtnN and DW2-KENP△speD, respectively. As shown in Figure 6, deletion of mtnN and speD were beneficial for intracellular SAM accumulation, and bacitracin yields were also enhanced in gene deletion strains. Furthermore, the strain DW2-KENPND was attained via deleting mtnN and speD simultaneously, and bacitracin yield of DW2-KENPND reached 957.25 U/mL, increased by 28.93 and 7.63% compared to DW2 and DW2-KENP, respectively (Figure 6A). The intracellular SAM concentration of DW2-KENPND was 47.53 mg/L, increased by 1.56-fold compared to DW2 (Figure 6B). In addition, the harmful by-products during bacitraicn production, cadaverine and putrescine, produced by DW2-KENPND were 53.24and 37.52 mg/L, decreased by 27.50 and 37.04% compared with those of DW2 (73.43 mg/L and 52.25 mg/L), as the weakened expression of amino acid decarboxylase (Figure 6C). Moreover, the transcriptional levels of bacT, bacA, bacB and bacC were increased by 3. 63-, 3. 35-, 3. 37-, and 3.23-fold, respectively (Figure 6D).
Figure 6. Effects of mtnN and speD deletion on bacitraicn production. (A) Bacitracin yield and cell biomass, (B) The concentration of intracellular SAM, (C) The concentrations of cadaverine and putrescine, (D) Transcriptional level analysis. ∗P < 0.05 and ∗∗P < 0.01 indicate the significance levels between recombinant strains and control strain.
Furthermore, the fermentation curves of B. licheniformis DW2 and DW2-KENPND were measured, and cell biomass and bacitracin yields were determined during fermentation process. Based on the results of Figure 7, bacitracin was synthesized from 12 h, and bacitracin yields of DW2-KENPND were higher than those of DW2 throughout the whole fermentation process, and the maximum yield produced by DW2-KENPND reached 957.53 U/mL, increased by 28.97%. In addition, the maximum cell biomass of DW2-KENPND was 428.54∗108 CFU/mL, increased by 6.22% compared to DW2 (403.43∗108 CFU/mL). The bacitracin produced by per cell was 2.23∗10–8 U/CFU, 21.42% higher than that of DW2 (1.84∗10–8U/CFU).
Bacitracin is a widely used veterinary antibiotic that mainly produced by Bacillus, and low production efficiency limits its promotion and application. SAM is an important cofactor in cell metabolism, which serves as the critical roles in syntheses of multiple antibiotics (Zhao et al., 2010). However, whether SAM affects antibiotic synthesis in Bacillus, especially for bacitracin, is still unknown. In this study, our results confirmed that strengthening intracellular SAM accumulation was beneficial for bacitracin production, and a metabolic engineered strain B. licheniformis DW2-KENPND with bacitracin yield of 957.53 U/mL was attained, via rewiring SAM synthetic and Met transportation pathways.
Acting as a critical methyl donor, SAM serves as the important role in the biosynthesis of multiple antibiotics (Zhang et al., 2008), in addition, SAM could increase the transcription of pathway-specific regulatory genes or auto-phosphorylation of regulatory kinase (Berney et al., 2015), therefore, strengthening SAM supply has been proven to be beneficial for production of lincomycin (Pang et al., 2015; Xu et al., 2018), avermectin (Tian et al., 2017), erythromycin (Wang et al., 2007), actinorhodin (Kim et al., 2003), etc. Moreover, SAM is not only involved in the transfer of sulfenyl and ribosyl groups, but also regulates intracellular metabolites that involved in primary and second metabolisms (Ruan et al., 2019). In this research, bacitracin is produced by Non-Ribosomal Polypeptide Synthetases (NRPS) in B. licheniformis (Rietkotter et al., 2008), different from those of above antibiotics (Polyketide Synthase) that which were mainly produced by Streptomyces, moreover, our results implied that strengthening SAM supply increased the transcription of bacitracin synthetase gene cluster, and transcriptional levels of genes bacT, bacA, bacB, and bacC were enhanced all under the condition of SAM addition. In addition, the transcriptional level of regulator gene abrB was decreased by 32.54% (Supplementary Figure S3). Since regulator AbrB has been proven as the negative regulator of bacitracin synthetase cluster bacTABC (Wang D. et al., 2017), our results suggested that additional SAM attenuated the inhibitory effect of AbrB on bacitracin synthase, and then improved bacitracin synthesis. Nevertheless, more work needs to be done to resolve the relationship between SAM and bacitracin synthetase.
Based on the previous researches, Met yield produced by microbial is generally at a high level (Huang et al., 2018), while SAM yield is quite low (Chu et al., 2013; Wendisch, 2019), indicating the low efficiency of SAM synthetic and transportation capabilities. Here, several SAM synthetases from B. licheniformis, C. glutamicum and S. cerevisiae were tested, and SAM2 from S. cerevisiae showed the best performances on SAM accumulation and bacitracin synthesis, consistent with the previous results of our group (Ruan et al., 2019). Along with developments of metabolic engineering and synthetic biology, various strategies have been conducted to improve SAM accumulation, including in strengthening SAM synthetic pathways (Zhang et al., 2008), coupling with TCA cycle (Ruan et al., 2019), increasing ATP level (Kanai et al., 2017), etc. In this research, the SAM synthetic and degradation pathways, Met transportation pathways were engineered to improve intracellular SAM accumulation for bacitracin production, and SAM concentration was increased to 47.53 mg/L by 1.56-fold, which led to a 28.97% increase of bacitracin yield. Based on the previous researches, the corresponding SAM transporters have been identified in E. coli, Streptomyces coelicolor etc., (Lee et al., 2012; Yang et al., 2014; Husna et al., 2018), also, the intracellular SAM concentration at 30 h was increased by 53.69% after SAM addition, suggested that additional SAM could be imported by amino acid transporter, thus, we suggested that SAM transporter is also present in B. licheniformis DW2, although it has not been identified or annotated. In addition, SAM decarboxylase gene speD was deleted to block synthetic pathway of byproduct, meantime, based on our results, two other harmful by-products, cadaverine and putrescine (Li et al., 2019), were also decreased by 27.50 and 37.04%, due to the weakening of amino acid decarboxylase (Figure 6C), and this result was positively correlated with our previous research (Wu et al., 2019). Furthermore, since SAM synthetase catalyzed the formation of SAM from Met and ATP, as well as the critical role of ATP supply in physiological metabolism (Cai et al., 2018), the ATP pool of DW2-KENPND should be enhanced in our future work.
Engineering transporter has been proven as an efficiency tactic for enhancement production of metabolite. Overexpression of ABC transporter AvtAB benefited avermectin secretion, and further led to the 50% increase of avermectin production (Qiu et al., 2011). Lys transporter LysE has been overexpressed to improve Lys production in C. glutamicum (Dong et al., 2016). However, bacitracin transporter in Bacillus has not been identified until now, although much work has been done on bacitracin microbial breeding and regulation (Fang et al., 2017; Wang D. et al., 2017; Shu et al., 2018). Here, two Met transporters MetN and MetP were screened, identified and engineered for bacitracin production at the first time. Based on our results, transporter MetN acted as Met exporter and MetP functioned as Met importer, and both of them served as the critical roles in Met distribution and bacitracin synthesis. Since soybean meal contains Met (Wang Q. et al., 2017), overexpression of MetP improved the accumulation of intracellular Met, which further benefit bacitracin production. Meantime, several other amino acid transporters (YdhG, BrnQ, and LysE) have also been engineered for bacitracin production (Li et al., 2018; Zhu et al., 2018; Wu et al., 2019), indicated that engineering amino acid transporter was an efficient strategy for bacitraicn production. Similar to Met transportation, several other amino acids also have multiple corresponding transporters. Such as, transporters YdhG, BrnQ, YvbW, and BraB were responsible for BCAAs transportation (Cai et al., 2019b), LysE, LysP and YvsH for lysine transportation (Wu et al., 2019), TcyP and YdeD for cysteine transportation (Ohtsu et al., 2015). The synergy of multiple transporters benefited the relative stability of intracellular and extracellular amino acids.
S-Adenosylmethionine served as the critical role in antibiotic production, however, whether it affects bacitracin synthesis is still unknown. Here, our results confirmed that exogenous SAM addition benefited bacitracin production, and a metabolic engineered strain B. licheniformis DW2-KENPND was attained via rewiring SAM synthetic and degradation, Met transportation pathways. Based on our results, SAM synthetase SAM2 from S. cerevisiae and Met synthetase MetH from B. licheniformis showed the best performance on SAM accumulation and bacitracin syntheses, and Met transporters MetN and MetP were identified as Met exporter and importer in B. licheniformis DW2, respectively. Finally, The concentration of intracellular SAM of DW2-KENPND was 47.53 U/mL, increased by 1.56-fold compared to DW2, and bacitracin yield reached 957.53 U/mL, increased by 28.97%. Taken together, this research demonstrated that SAM served as the critical role in bacitraicn synthesis, and a promising strain B. licheniformis DW2-KENPND was attained for industrial production of bacitracin.
The datasets generated for this study are available on request to the corresponding author.
DC and SC designed the study. DC, BZ, JZ, and HX carried out the molecular biology studies and construction of engineering strains. DC, BZ, JZ, and PL carried out the fermentation studies. DC, ZW, JL, ZY, XM, and SC analyzed the data and wrote the manuscript. All authors read and approved the final manuscript.
This work was supported by the National Key Research and Development Program of China (2018YFA0900300), the Technical Innovation Special Fund of Hubei Province (2018ACA149), the Natural Science Foundation of Hubei Province of China (2019CFB319), the China Postdoctoral Science Foundation (2018M642802), and the Open Funding Project of State Key Laboratory of Biocatalysis and Enzyme Engineering (SKLBEE2018005).
JL was employed by Lifecome Biochemistry Co., Ltd., in China.
The remaining authors declare that the research was conducted in the absence of any commercial or financial relationships that could be constructed as a potential conflict of interest.
The Supplementary Material for this article can be found online at: https://www.frontiersin.org/articles/10.3389/fbioe.2020.00305/full#supplementary-material
BCAAs, branched chain amino acid; GC, gas chromatography; HPLC, high performance liquid chromatography; Lys, lysine; Met, methionine; NRPS, non-ribosomal polypeptide synthetases; Orn, ornithine; SAM, S-Adenosylmethionine.
Berney, M., Berney-Meyer, L., Wong, K. W., Chen, B., Chen, M., Kim, J., et al. (2015). Essential roles of methionine and S-adenosylmethionine in the autarkic lifestyle of Mycobacterium tuberculosis. Proc. Natl. Acad. Sci. U.S.A. 112, 10008–10013. doi: 10.1073/pnas.1513033112
Birrer, G. A., Cromwick, A. M., and Gross, R. A. (1994). Gamma-poly(glutamic acid) formation by Bacillus licheniformis 9945a: physiological and biochemical studies. Int. J. Biol. Macromol. 16, 265–275. doi: 10.1016/0141-8130(94)90032-9
Cai, D., Chen, Y., He, P., Wang, S., Mo, F., Li, X., et al. (2018). Enhanced production of Poly-gamma-glutamic acid by improving ATP supply in metabolically engineered Bacillus licheniformis. Biotechnol. Bioeng. 115, 2541–2553. doi: 10.1002/bit.26774
Cai, D., Wang, H., He, P., Zhu, C., Wang, Q., Wei, X., et al. (2017). A novel strategy to improve protein secretion via overexpression of the SppA signal peptide peptidase in Bacillus licheniformis. Microb. Cell Fact. 16:70. doi: 10.1186/s12934-017-0688-7
Cai, D., Zhang, B., Rao, Y., Li, L., Zhu, J., Li, J., et al. (2019a). Improving the utilization rate of soybean meal for efficient production of bacitracin and heterologous proteins in the aprA-deficient strain of Bacillus licheniformis. Appl. Microbiol. Biotechnol. 103, 4789–4799. doi: 10.1007/s00253-019-09804-0
Cai, D., Zhu, J., Zhu, S., Lu, Y., Zhang, B., Lu, K., et al. (2019b). Metabolic engineering of main transcription factors in carbon, nitrogen, and phosphorus metabolisms for enhanced production of bacitracin in Bacillus licheniformis. ACS Synth. Biol. 8, 866–875. doi: 10.1021/acssynbio.9b00005
Chen, C., Li, Y., Hu, J., Dong, X., and Wang, X. (2015). Metabolic engineering of Corynebacterium glutamicum ATCC13869 for L-valine production. Metab. Eng. 29, 66–75. doi: 10.1016/j.ymben.2015.03.004
Chu, J., Qian, J., Zhuang, Y., Zhang, S., and Li, Y. (2013). Progress in the research of S-adenosyl-L-methionine production. Appl. Microbiol. Biotechnol. 97, 41–49. doi: 10.1007/s00253-012-4536-8
Dong, X., Zhao, Y., Hu, J., Li, Y., and Wang, X. (2016). Attenuating l-lysine production by deletion of ddh and lysE and their effect on l-threonine and l-isoleucine production in Corynebacterium glutamicum. Enzyme Microb. Technol. 93-94, 70–78. doi: 10.1016/j.enzmictec.2016.07.013
Fang, C., Nagy-Staron, A., Grafe, M., Heermann, R., Jung, K., Gebhard, S., et al. (2017). Insulation and wiring specificity of BceR-like response regulators and their target promoters in Bacillus subtilis. Mol. Microbiol. 104, 16–31. doi: 10.1111/mmi.13597
Huang, J. F., Shen, Z. Y., Mao, Q. L., Zhang, X. M., Zhang, B., Wu, J. S., et al. (2018). Systematic analysis of bottlenecks in a multibranched and multilevel regulated pathway: the molecular fundamentals of l-methionine biosynthesis in Escherichia coli. ACS Synth. Biol. 7, 2577–2589. doi: 10.1021/acssynbio.8b00249
Husna, A. U., Wang, N., Cobbold, S. A., Newton, H. J., Hocking, D. M., Wilksch, J. J., et al. (2018). Methionine biosynthesis and transport are functionally redundant for the growth and virulence of Salmonella Typhimurium. J. Biol. Chem. 293, 9506–9519. doi: 10.1074/jbc.RA118.002592
Kanai, M., Mizunuma, M., Fujii, T., and Iefuji, H. (2017). A genetic method to enhance the accumulation of S-adenosylmethionine in yeast. Appl. Microbiol. Biotechnol. 101, 1351–1357. doi: 10.1007/s00253-017-8098-7
Kim, D. J., Huh, J. H., Yang, Y. Y., Kang, C. M., Lee, I. H., Hyun, C. G., et al. (2003). Accumulation of S-adenosyl-L-methionine enhances production of actinorhodin but inhibits sporulation in Streptomyces lividans TK23. J. Bacteriol. 185, 592–600. doi: 10.1128/jb.185.2.592-600.2003
Lee, S. K., Mo, S., and Suh, J. W. (2012). An ABC transporter complex containing S-adenosylmethionine (SAM)-induced ATP-binding protein is involved in antibiotics production and SAM signaling in Streptomyces coelicolor M145. Biotechnol. Lett. 34, 1907–1914. doi: 10.1007/s10529-012-0987-3
Li, L., Zou, D., Ruan, L., Wen, Z., Chen, S., Xu, L., et al. (2019). Evaluation of the biogenic amines and microbial contribution in traditional Chinese sausages. Front. Microbiol. 10:872. doi: 10.3389/fmicb.2019.00872
Li, M., Chen, Z., Zhang, X., Song, Y., Wen, Y., and Li, J. (2010). Enhancement of avermectin and ivermectin production by overexpression of the maltose ATP-binding cassette transporter in Streptomyces avermitilis. Bioresour. Technol. 101, 9228–9235. doi: 10.1016/j.biortech.2010.06.132
Li, Y., Wu, F., Cai, D., Zhan, Y., Li, J., Chen, X., et al. (2018). [Enhanced production of bacitracin by knocking out of amino acid permease gene yhdG in Bacillus licheniformis DW2]. Sheng Wu Gong Cheng Xue Bao 34, 916–927. doi: 10.13345/j.cjb.170500
Liu, J., Chen, Y., Wang, W., Ren, M., Wu, P., Wang, Y., et al. (2017). Engineering of an Lrp family regulator SACE_Lrp improves erythromycin production in Saccharopolyspora erythraea. Metab. Eng. 39, 29–37. doi: 10.1016/j.ymben.2016.10.012
Martin, J. F., Rodriguez-Garcia, A., and Liras, P. (2017). The master regulator PhoP coordinates phosphate and nitrogen metabolism, respiration, cell differentiation and antibiotic biosynthesis: comparison in Streptomyces coelicolor and Streptomyces avermitilis. J. Antibiot. 70, 534–541. doi: 10.1038/ja.2017.19
Mendes, M. V., Tunca, S., Anton, N., Recio, E., Sola-Landa, A., Aparicio, J. F., et al. (2007). The two-component phoR-phoP system of Streptomyces natalensis: inactivation or deletion of phoP reduces the negative phosphate regulation of pimaricin biosynthesis. Metab. Eng. 9, 217–227. doi: 10.1016/j.ymben.2006.10.003
Ohtsu, I., Kawano, Y., Suzuki, M., Morigasaki, S., Saiki, K., Yamazaki, S., et al. (2015). Uptake of L-cystine via an ABC transporter contributes defense of oxidative stress in the L-cystine export-dependent manner in Escherichia coli. PLoS One 10:e0120619. doi: 10.1371/journal.pone.0120619
Pang, A. P., Du, L., Lin, C. Y., Qiao, J., and Zhao, G. R. (2015). Co-overexpression of lmbW and metK led to increased lincomycin A production and decreased byproduct lincomycin B content in an industrial strain of Streptomyces lincolnensis. J. Appl. Microbiol. 119, 1064–1074. doi: 10.1111/jam.12919
Qiu, J., Zhuo, Y., Zhu, D., Zhou, X., Zhang, L., Bai, L., et al. (2011). Overexpression of the ABC transporter AvtAB increases avermectin production in Streptomyces avermitilis. Appl. Microbiol. Biotechnol. 92, 337–345. doi: 10.1007/s00253-011-3439-4
Rietkotter, E., Hoyer, D., and Mascher, T. (2008). Bacitracin sensing in Bacillus subtilis. Mol. Microbiol. 68, 768–785. doi: 10.1111/j.1365-2958.2008.06194.x
Ruan, L., Li, L., Zou, D., Jiang, C., Wen, Z., Chen, S., et al. (2019). Metabolic engineering of Bacillus amyloliquefaciens for enhanced production of S-adenosylmethionine by coupling of an engineered S-adenosylmethionine pathway and the tricarboxylic acid cycle. Biotechnol. Biofuels 12:211. doi: 10.1186/s13068-019-1554-0
Shi, J., Zhan, Y., Zhou, M., He, M., Wang, Q., Li, X., et al. (2019). High-level production of short branched-chain fatty acids from waste materials by genetically modified Bacillus licheniformis. Bioresour. Technol. 271, 325–331. doi: 10.1016/j.biortech.2018.08.134
Shu, C. C., Wang, D., Guo, J., Song, J., Chen, S., Chen, L., et al. (2018). Analyzing AbrB-knockout effects through genome and transcriptome sequencing of Bacillus licheniformis DW2. Front. Microbiol. 9:307. doi: 10.3389/fmicb.2018.00307
Sola-Landa, A., Moura, R. S., and Martin, J. F. (2003). The two-component PhoR-PhoP system controls both primary metabolism and secondary metabolite biosynthesis in Streptomyces lividans. Proc. Natl Acad. Sci. U.S.A. 100, 6133–6138. doi: 10.1073/pnas.0931429100
Tian, P., Cao, P., Hu, D., Wang, D., Zhang, J., Wang, L., et al. (2017). Comparative metabolomics reveals the mechanism of avermectin production enhancement by S-adenosylmethionine. J. Ind. Microbiol. Biotechnol. 44, 595–604. doi: 10.1007/s10295-016-1883-y
Wang, D., Wang, Q., Qiu, Y., Nomura, C. T., Li, J., and Chen, S. (2017). Untangling the transcription regulatory network of the bacitracin synthase operon in Bacillus licheniformis DW2. Res. Microbiol. 168, 515–523. doi: 10.1016/j.resmic.2017.02.010
Wang, Q., Zheng, H., Wan, X., Huang, H., Li, J., Nomura, C. T., et al. (2017). Optimization of inexpensive agricultural by-products as raw materials for bacitracin production in Bacillus licheniformis DW2. Appl. Biochem. Biotechnol. 183, 1146–1157. doi: 10.1007/s12010-017-2489-1
Wang, M., Yu, H., and Shen, Z. (2019). Antisense RNA-based strategy for enhancing surfactin production in Bacillus subtilis TS1726 via overexpression of the unconventional biotin carboxylase II to enhance ACCase activity. ACS Synth. Biol. 8, 251–256. doi: 10.1021/acssynbio.8b00459
Wang, Y., Wang, Y., Chu, J., Zhuang, Y., Zhang, L., and Zhang, S. (2007). Improved production of erythromycin A by expression of a heterologous gene encoding S-adenosylmethionine synthetase. Appl. Microbiol. Biotechnol. 75, 837–842. doi: 10.1007/s00253-007-0894-z
Wendisch, V. F. (2019). Metabolic engineering advances and prospects for amino acid production. Metab. Eng. 58, 17–34. doi: 10.1016/j.ymben.2019.03.008
Wohlleben, W., Mast, Y., Muth, G., Rottgen, M., Stegmann, E., and Weber, T. (2012). Synthetic biology of secondary metabolite biosynthesis in actinomycetes: engineering precursor supply as a way to optimize antibiotic production. FEBS Lett. 586, 2171–2176. doi: 10.1016/j.febslet.2012.04.025
Wu, F., Cai, D., Li, L., Li, Y., Yang, H., Li, J., et al. (2019). Modular metabolic engineering of lysine supply for enhanced production of bacitracin in Bacillus licheniformis. Appl. Microbiol. Biotechnol. 103, 8799–8812. doi: 10.1007/s00253-019-10110-y
Xu, Y., Tan, G., Ke, M., Li, J., Tang, Y., Meng, S., et al. (2018). Enhanced lincomycin production by co-overexpression of metK1 and metK2 in Streptomyces lincolnensis. J. Ind. Microbiol. Biotechnol. 45, 345–355. doi: 10.1007/s10295-018-2029-1
Yang, J., Sun, B., Huang, H., Jiang, Y., Diao, L., Chen, B., et al. (2014). High-efficiency scarless genetic modification in Escherichia coli by using lambda red recombination and I-SceI cleavage. Appl. Environ. Microbiol. 80, 3826–3834. doi: 10.1128/AEM.00313-14
Yu, W., Li, D., Jia, S., Liu, Z., Nomura, C. T., Li, J., et al. (2019). Systematic metabolic pathway modification to boost L-ornithine supply for bacitracin production in Bacillus licheniformis DW2. Appl. Microbiol. Biotechnol. 103, 8383–8392. doi: 10.1007/s00253-019-10107-7
Zhang, X., Fen, M., Shi, X., Bai, L., and Zhou, P. (2008). Overexpression of yeast S-adenosylmethionine synthetase metK in Streptomyces actuosus leads to increased production of nosiheptide. Appl. Microbiol. Biotechnol. 78, 991–995. doi: 10.1007/s00253-008-1394-5
Zhao, X. Q., Gust, B., and Heide, L. (2010). S-Adenosylmethionine (SAM) and antibiotic biosynthesis: effect of external addition of SAM and of overexpression of SAM biosynthesis genes on novobiocin production in Streptomyces. Arch. Microbiol. 192, 289–297. doi: 10.1007/s00203-010-0548-x
Zhou, S., Ding, R., Chen, J., Du, G., Li, H., and Zhou, J. (2017). Obtaining a panel of cascade promoter-5’-UTR complexes in Escherichia coli. ACS Synth. Biol. 6, 1065–1075. doi: 10.1021/acssynbio.7b00006
Zhu, J., Cai, D., Xu, H., Liu, Z., Zhang, B., Wu, F., et al. (2018). Enhancement of precursor amino acid supplies for improving bacitracin production by activation of branched chain amino acid transporter BrnQ and deletion of its regulator gene lrp in Bacillus licheniformis. Synth. Syst. Biotechnol. 3, 236–243. doi: 10.1016/j.synbio.2018.10.009
Keywords: bacitracin, S-Adenosylmethionine, Bacillus licheniformis, methionine, metabolic engineering
Citation: Cai D, Zhang B, Zhu J, Xu H, Liu P, Wang Z, Li J, Yang Z, Ma X and Chen S (2020) Enhanced Bacitracin Production by Systematically Engineering S-Adenosylmethionine Supply Modules in Bacillus licheniformis. Front. Bioeng. Biotechnol. 8:305. doi: 10.3389/fbioe.2020.00305
Received: 04 February 2020; Accepted: 20 March 2020;
Published: 07 April 2020.
Edited by:
Mingjie Jin, Nanjing University of Science and Technology, ChinaReviewed by:
Xixian Xie, Tianjin University of Science and Technology, ChinaCopyright © 2020 Cai, Zhang, Zhu, Xu, Liu, Wang, Li, Yang, Ma and Chen. This is an open-access article distributed under the terms of the Creative Commons Attribution License (CC BY). The use, distribution or reproduction in other forums is permitted, provided the original author(s) and the copyright owner(s) are credited and that the original publication in this journal is cited, in accordance with accepted academic practice. No use, distribution or reproduction is permitted which does not comply with these terms.
*Correspondence: Zhifan Yang, c2FpbHlhbmd6aGZAaHVidS5lZHUuY24=; Shouwen Chen, bWVsMjEyQDEyNi5jb20=
Disclaimer: All claims expressed in this article are solely those of the authors and do not necessarily represent those of their affiliated organizations, or those of the publisher, the editors and the reviewers. Any product that may be evaluated in this article or claim that may be made by its manufacturer is not guaranteed or endorsed by the publisher.
Research integrity at Frontiers
Learn more about the work of our research integrity team to safeguard the quality of each article we publish.