- State Key Laboratory of Materials-Oriented Chemical Engineering, College of Biotechnology and Pharmaceutical Engineering, Nanjing Tech University, Nanjing, China
Bioelectrochemical systems are revolutionary new bioengineering technologies which integrate microorganisms or enzymes with the electrochemical method to improve the reducing or oxidizing metabolism. Generally, the bioelectrochemical systems show the processes referring to electrical power generation or achieving the reducing reaction with a certain potential poised by means of electron transfer between the electron acceptor and electron donor. Researchers have focused on the selection and optimization of the electrode materials, design of electrochemical device, and screening of electrochemically active or inactive model microorganisms. Notably, all these means and studies are related to electron transfer: efflux and consumption. Thus, here we introduce the basic concepts of bioelectrochemical systems, and elaborate on the extracellular and intracellular electron transfer, and the hypothetical electron transfer mechanism. Also, intracellular energy generation and coenzyme metabolism along with electron transfer are analyzed. Finally, the applications of bioelectrochemical systems and the prospect of microbial electrochemical technologies are discussed.
Introduction
Bioelectrochemical systems, revolutionary new bioengineering technologies, integrate microorganisms or other bio-based catalysts with an electrochemical method to improve the reducing or oxidizing metabolism. Generally, bioelectrochemical systems show the process of electrical power generation or achieve the reduction reaction with a certain potential poised by means of electron transfer between the electron acceptor and electron donor (Fernandez et al., 2015).
Previously, bioelectrochemical systems have been widely applied in the form of microbial fuel cells (MFCs), since Michael Potter (1911) first studied the generation of an electrical current by several microorganisms, which convert chemical energy into electrical energy by degradation of various substrates, especially organic compounds from waste water (Gul and Ahmad, 2019). As a reversal process compared to MFCs, microbial electrolytic cells (MECs) were used to convert electrical energy to chemical energy with the help of microorganisms or enzymes to produce useful products such as formate, methanol, ethanol, or hydrocarbons. These molecules were then converted or used directly as a sustainable alternative to fossil fuels (Yuan et al., 2019). With the growing desire for environment-friendly and energy-saving processes (Zhang and Angelidaki, 2015), bioelectrochemical systems attracted much attention for their green and sustainable characteristics (Ghosh and Ghangrekar, 2015).
To expand the application of bioelectrochemical systems, researchers have focused on the selection and optimization of the electrode materials, design of the electrochemical devices, and screening of electrochemically active or inactive model microorganisms. Recently, bioelectrochemical systems have been widely applied in nitrate removal, solid waste processing, desalination and materials science (Zhen et al., 2016). Notably, all of these uses and studies are related to electron transfer and energy transformation, i.e., the interchange of chemical energy and electrical energy.
Here, we first introduce the basic concepts of bioelectrochemical systems, and elaborate on the mechanisms of extracellular and intracellular electron transfer. We also analyze the hypothetical electron transfer mechanism, intracellular energy generation and coenzyme metabolism. Finally, the applications of bioelectrochemical systems and prospective microbial electrochemical technologies are discussed.
Classification of Bioelectrochemical Systems
An electrochemical system is a series of electrochemical models, or devices, in which electronic behaviors occur with different types of catalysts. In terms of bioelectrochemical systems, it mainly describes a series of technologies that are used for biotechnology applications including electricity generation (Butler et al., 2010) and the production of valuable products (Villano et al., 2011). Generally, they can be divided into two categories depending on the catalyst adopted. The first one are those that use microorganisms as catalysts, and the second are those that use enzymes as the catalyst.
Microbial Electrochemical Systems
According to the direction of electron transfer and the type of reaction, microbial electrochemical systems can be divided into MFCs and microbial electrolysis or electro-synthesis cells (MECs). MFCs, are electrochemical systems with microorganisms acting as biocatalysts in the anode chamber, have been widely used for electricity generation with various substrates (Table 1). In MFCs, electrons released through intracellular metabolism (substrate oxidation) transfer to the anode, and are finally captured by the cathode electrode via an external circuit to be used for the reduction of oxygen, or another electron accepter, with the generation of current (Logan et al., 2006). Recently, many MFCs have been reported that act as an innovative wastewater treatment technique for pollution removal and energy generation, due to their high degradation rate (He et al., 2015; Yazdi et al., 2015; Zhao et al., 2015a).
Bioelectrochemical systems have been successfully applied in MFCs using diverse microorganisms such as Shewanella putrefaciens (Yang et al., 2017), Shewanella oneidensis MR-1 (Bretschger et al., 2007) and Escherichia coli DH5α (Li et al., 2018), which can produce a maximum power density range from 3800 to 4400 mW/m2. However, these fuel cells have a low cost-effectiveness due to high material costs and mediocre power generation, which are still the major limitations to extending the range of applications (Zhou et al., 2013). Also, some electrodes are manufactured with gold, platinum and other expensive materials which raises the cost (Sadeghifar and Rashid-Nadimi, 2017).
Microbial fuel cells systems adopt bacteria as catalysts, and the biofilm is formed on the surface of the anode, which can improve the electron transfer. Thus, the practical application of MFCs may be further confined by microorganisms - since the efficiency and stability of microbial reactions mainly relies on the external environment which is far different from their indigenous environments (Pareek et al., 2019), due to issues such as low temperatures, high salinity and high toxicity (Zhang et al., 2017). Another limitation is the internal resistance of the fuel cell caused mainly by the proton exchange membrane, which results in low current density and power density (Shreeram et al., 2018). Table 1 lists the recent research on MFCs.
Microbial electrolytic cells also use microorganisms as catalysts, and the cells are inoculated into the cathode chamber which acts as an electron acceptor and gains electrons, thus accelerating the intracellular reduction metabolism (Villano et al., 2011; Ding et al., 2012; Jafary et al., 2015). Essentially, MECs are a reverse process compared to MFCs. In MFCs, the oxidation reaction occurs in the anode chamber, after which the released electrons are transferred to the cathode chamber in a process that involves substrate reduction. For MECs, the potential poised on the cathode chamber is the most important factor and is determined by the bacteria and electron shuttles added, as they can have different potential differences (Table 2). However, the potential poised on the cathode is not equal to the theoretical potential difference of electron shuttles as the electron or energy losses in bioelectrochemical system (Zhen et al., 2016). There are also many issues restraining the scale up of MECs, especially as most of the microbial electrosynthesis systems suffer from low energy efficiencies and low production rates (Su and Ajo-Franklin, 2019). Another limitation on MECs is chemical incompatibility between the abiotic and biotic catalysis, for example, fouling of the electrode by microbes, or toxicity to the microbes caused by electrode leaching (Gildemyn et al., 2017). A vital index in the application of MECs is the long-term stability, which suffers from low turnover frequencies and oxygen sensitivity of certain enzymes relevant to CO2 reduction (Yuan et al., 2019).
By using cyclic voltammogram detection, it was found that the potential poised cathode can drive the electron shuttle reduction at high rates and then the intracellular reducing metabolism can be accomplished by current stimulation. During the whole process, the cathode provides electrons to pump intracellular reducing power and energy generation (She et al., 2006). However, it remains to be investigated whether the electrons released from the cathode can be efficiently transferred between the cathode and bacteria (Schroder, 2007). In a word, in these two microbial electrochemical systems, the possibility of electron transfer and the rate of electron transfer between cells and electrodes are the key factors used to determine the efficiency of the entire system (Li et al., 2015; Santoro et al., 2015).
Enzymatic Electrochemical Systems
Another type of bioelectrochemical system is that using enzymes as catalysts. The electrodes with enzymes serve as external electron donors or electron acceptors (Amano et al., 2016; Lee et al., 2016). Since the enzymatic reaction is the sole reaction occurring in this electrochemical system, and the electron transfer kinetic potential is predetermined, and the oxidoreductase can be regenerated by capturing or releasing electrons at the surface of electrode, the enzymatic electrochemical system is widely used in the study of electron transfer mechanisms in vitro (Kracher et al., 2016).
Since previous studies have applied electrochemical methods to study the biological electron and ion transfer and proved its high sensitivity and reliability, it would be an efficient and convenient strategy to analyze the mechanism of enzyme reaction. When NADH:quinone complex I was fixed on the surface of a gold modified electrode, the process of electron and proton transfer recurred effectively in vivo with the electrode as the sole electron acceptor (Oscar et al., 2014). Moreover, in order to increase the load of the enzyme for a higher catalytic rate, high surface area materials were introduced into this system with a high density current output (Bari et al., 2016).
Although all these electrochemical systems have been studied for decades, upgraded electrochemical devices and novel biocompatible electrode materials are absolutely imperative. Moreover, the mechanisms of energy output and electron transfer are still in their infancy.
Electron Transfer in the Bioelectrochemical System
Extracellular Electron Transfer
Electron Transfer in Enzyme Electrochemical Systems
In enzyme electrochemical systems, the oxidoreductases are selected, purified and fixed on the surface of modified electrodes, which act as electron donors or electron acceptors and participate in enzymatic reactions along with the interaction of an electrode (Compagnone and Guilbault, 1997; Bari et al., 2016). So, the key problem to be solved is the bidirectional electron transfer from the electrode to the active site of the enzymes. However, the transition and transfer of electrons between the electron carriers have certain restrictions, as the relative distance between the two given electron carriers within the enzyme increases, the electron transfer rate will decline rapidly and affect the efficiency of the enzymatic reaction. When the distance is longer than 10 angstroms, electron transfer will only be achieved through the presence of electron mediators (Mayo et al., 1986). Therefore, the electrodes are modified to facilitate the immobilization of the enzyme and thus effectively accelerate electron transfer between oxidases and the electrodes (Freguia et al., 2012; Oscar et al., 2014). Furthermore, conductive nano-particles can also be used to assist the long distance transfer of electrons. In this way, a long distance electron transfer from FAD/FADH2 (glucose oxidase) to an electrode was achieved (Degani and Heller, 1987).
Although efficient electron transfer can be achieved by various means, the applications of enzyme electrochemical are restricted to a small field by taking into consideration the decreasing activity of enzymes during recycling. Among them, enzyme electrochemical sensor systems is a relatively mature field and widely used for substance detection, such as heavy metals (Ruecha et al., 2015), glucose (Gutierrez et al., 2016) and other organic substances (Wang et al., 2016). Additionally, enzyme electrochemical systems have also been successfully applied to studying the mechanism of electron transfer during enzyme reactions. By cyclic voltammetry detection, Kracher et al. (2016) verified the mechanism of electron transfer in lytic polysaccharide monooxygenases (LPMOs) during the oxidative degradation of cellulose with multi-enzyme modified electrodes.
Extracellular Electron Transfer in Microbial Electrochemical Systems
Unlike enzyme electrochemical systems, microorganisms act as catalysts in MFCs and MECs. In MFCs, reducing equivalents stored in the organic substrate are released in the form of electrons, which are captured by the anode and then transferred to the cathode through the external circuit with the generation of electricity (Santoro et al., 2016). In MECs, a given voltage is poised at the cathode. The electrons involved in intracellular reduction and energy metabolism are released from the cathode electrode and captured by strains (Carmona-Martínez et al., 2015; Yin et al., 2016). Since the cell membrane is insulated, a necessary prerequisite for the electrochemical reactions to occur is that the electrons can smoothly transfer across the membrane (Kracher et al., 2016). Due to reactions occurring on the electrodes of different substances exhibiting different electrochemical behaviors and the different mechanisms of electron transfer between microorganisms and electrodes, three major mechanisms exist in electron transfer between the electrodes and the microorganisms (Figure 1). They are reling on nanowires (conductive pili), relying on outer membrane proteins and/or mediated by endogenous or exogenous electron shuttles (Choi and Sang, 2016).
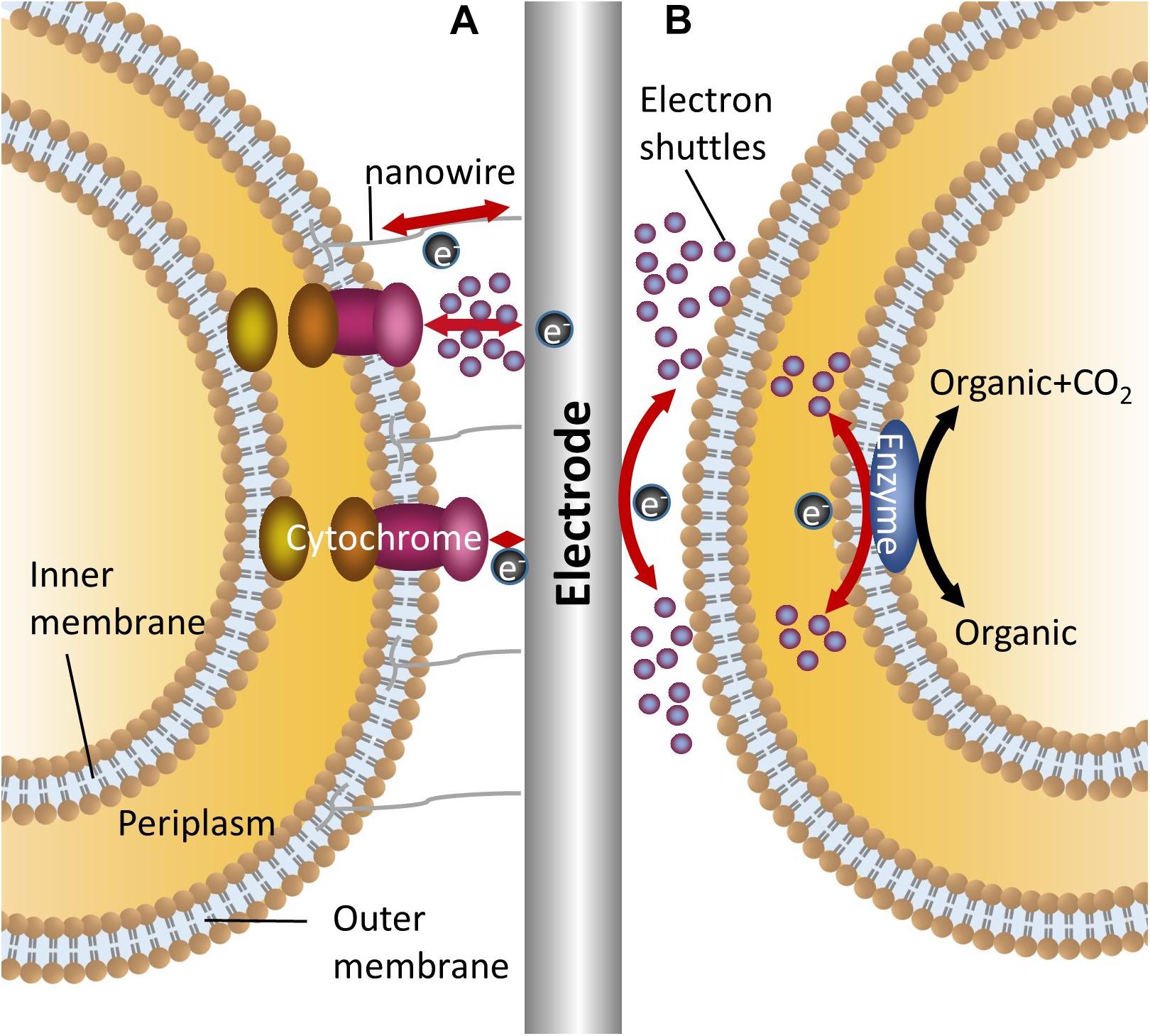
Figure 1. Mechanisms for bidirectional electron transfer between bacteria and electrodes. (A) Represents two mechanisms of direct electron transfer, one is mediated by nanowire, the other is mediated by outer membrane cytochromes with or without electron shuttles; (B) Shows the indirect electron transfer mediated by electron shuttles.
For the electrochemical active strains, different groups of strains evolved different conductive mechanisms. Shewanella has a complete set of extracellular electron transport chains. The electron transport channels which were formed by outer membrane cytochromes (Mtr and CymA system) mediate the direct electron transfer between cells and electrodes (Ross et al., 2012; Carmona-Martínez et al., 2013). Meanwhile, the synthesis and release of riboflavin assists electron transmembrane transfer, but this process requires a specific transport system (Brutinel and Gralnick, 2012). Another model electrochemical active strain, Geobacter, can synthesize conductive pili (nanowires) through cell growth and is directed involved in the extracellular electron transfer between cell and electrodes (Schroder, 2007; Lovley, 2008). Also, outer membrane cytochromes are necessary for electron capturing (Butler et al., 2010).
Nanowires, outer membrane cytochromes and other appendages are key components for effective electron capture in electrochemical active strains. In order to improve the extracellular electron transfer efficiency, the development of biofilms can benefit the whole process (Verea et al., 2014; Laura et al., 2015). The presence of biofilm ensures direct long distance electron transfer, high catalytic rates and high power outputs. In recent years, newly developed and modified porous conductive materials have been used for facilitating biofilm formation on electrode surfaces (Karthikeyan et al., 2015; Yuan et al., 2016). Although the electrodes that have porous or three-dimensional surfaces in MFCs will improve the performance of the whole system with enhanced efficiency of electricity output, it also depends on the conductive characteristic of the selected electrode material (Ledezma et al., 2015). When using stainless steel and carbon felt as electrodes for current generation, Dumas et al. (2008) found that carbon felt has high porosity characteristics for microbial attachment, but weaker conductivity compared to stainless steel electrodes which caused lower level current generation. However, in terms of other electrochemical active or inactive strains, the electrons cannot transfer directly between the cells and electrodes, and biofilm on electrode surfaces limited the electron and mass transfer (Rabaey et al., 2007; Freguia et al., 2008).
The electrochemically inactive bacteria do not have a fully functional extracellular electron transfer system and almost all the strains of this type are not able to secret electron carriers (Masuda et al., 2010). However, electrochemically inactive bacteria can react with electrodes through the addition of an electron shuttle. In the majority of model strains, such as Escherichia coli, Actinobacillus succinogenes (Park et al., 1999) and Clostridium (He et al., 2016), bidirectional electron transfer can be achieved in the presence of electron shuttles. With neutral red, A. succinogenes can gain electrons from a cathode and use these for intracellular metabolism (Park et al., 1999). Although bacteria can be divided into electrochemically active and inactive types, the boundary is not so clear. For example, one study has demonstrated that the screened E. coli gained the ability of direct electron transfer from intracellular to extracellular and achieved electricity generation without electron shuttles (Park et al., 1999). However, that does not mean all the electrochemically inactive bacteria can gain the same ability of direct electron transfer.
Interspecies Electron Transfer
After the microorganisms were incubated into electrochemical systems, a start-up period was needed to activate the process of electricity generation. During this period, electrochemically active strains, in which electrons could be transferred directly were absorbed onto the surface of the electrode to form biofilm. And thus, a shorter start-up period of MFC could be obtained as the distance of electron transfer decreased (Zhao et al., 2015b).
A co-culture of Geobacter and Methanosaeta or Methanosarcina species has been confirmed to promote the electro-synthesis of methane as strains shared electrons via direct interspecies electron transfer (Zheng et al., 2015). In this way, interspecies electron transfer can promote and strengthen the symbiotic electrochemical behavior with microbial co-culture fermentation, which was beneficial to achieve synergy between different microbial species and the production of bio-gas or other high value biofuels from cheap raw materials (Zheng et al., 2015).
The more general concept is that conductive materials can promote interspecies electron transfer and strengthen co-culture fermentation. In order to introduce more species to symbiotic co-culture systems and expand the application of interspecies electron transfer, electron shuttles can also be used for indirect electron transfer among strains. It is demonstrated that electron transfer mediated by active carbon particles within cells does not need to rely on cellular conductive structures (such as conductive pili or nanowires) or the assistance of cytochromes (Liu et al., 2012; Amelia-Elena et al., 2014). Moreover, the interspecific electron transfer can be achieved in the presence of activated carbon particles, and the catalytic properties of the mixed bacteria can be strengthened even when co-cultured with electrochemically inactive bacteria (Qu et al., 2012).
It is worthy of notice that co-cultures of bacteria with different electron shuttles may have different functions. In the presence of AQDS, co-cultures of Geobacter metallireducens and Geobacter sulfurreducens can achieve higher rates of ethanol consumption and better cell growth as more energy is generated (Smith et al., 2015). When the co-cultured bacteria were Geobacter metallireducens and Monascus barkeri, no obvious effects on the synthesis of methane from ethanol was obtained because of the higher potential of AQDS (Table 2; Liu et al., 2012). This can be explained due to the differing levels of intracellular energy that can be gained along with electron transfer from electron donor to electron acceptor via various electron shuttles and electron transfer chains.
Intracellular Electron Transfer Chain
The only goal of nanowires, electron shuttles and modified electrodes is to strengthen the interaction between cells and electrodes, and to increase the rate of extracellular electron transfer. The intracellular electron transfer chain (ETC, also called the respiratory chain) consists of a series of electron or proton carriers, including cytochromes, coenzyme Q and lots of oxidoreductases. Within the ETC, electrons are transferred from high potential electron donor to a low potential electron acceptor along with ATP synthesis (Hara and Kondo, 2015).
For electrochemically active bacteria, cytochromes that are anchored at the membrane can facilitate electron transfer and intracellular metabolism (Richter et al., 2009). As electrochemically active bacteria, Shewanella and Geobacter have an excellent ability for intracellular electron transfer, and thus are widely used in MFCs (Lovley, 2012). Previous studies have demonstrated that some strains can change electron transfer routes depending on the potential difference of available electron donors or acceptors (Kracke et al., 2015), and Shewanella can achieve bidirectional electron transfer with only one intracellular electron transfer system in MFCs and MECs (Figure 2; Shi et al., 2010; Ross et al., 2012). The only difference was the electron mediator that was used. The MFC adopted ubiquinone, while the MEC adopted menaquinone, as it has a lower potential difference to ubiquinone. Compared with Shewanella, three electron leaking mechanisms exist in Geobacter: electron transfer OmcZ between cells, OmcE/OmcS used for Fe (III) reduction, and nanowire used for interactions with electrodes (Richter et al., 2009; Shi et al., 2010; Ross et al., 2012; Sturm et al., 2015).
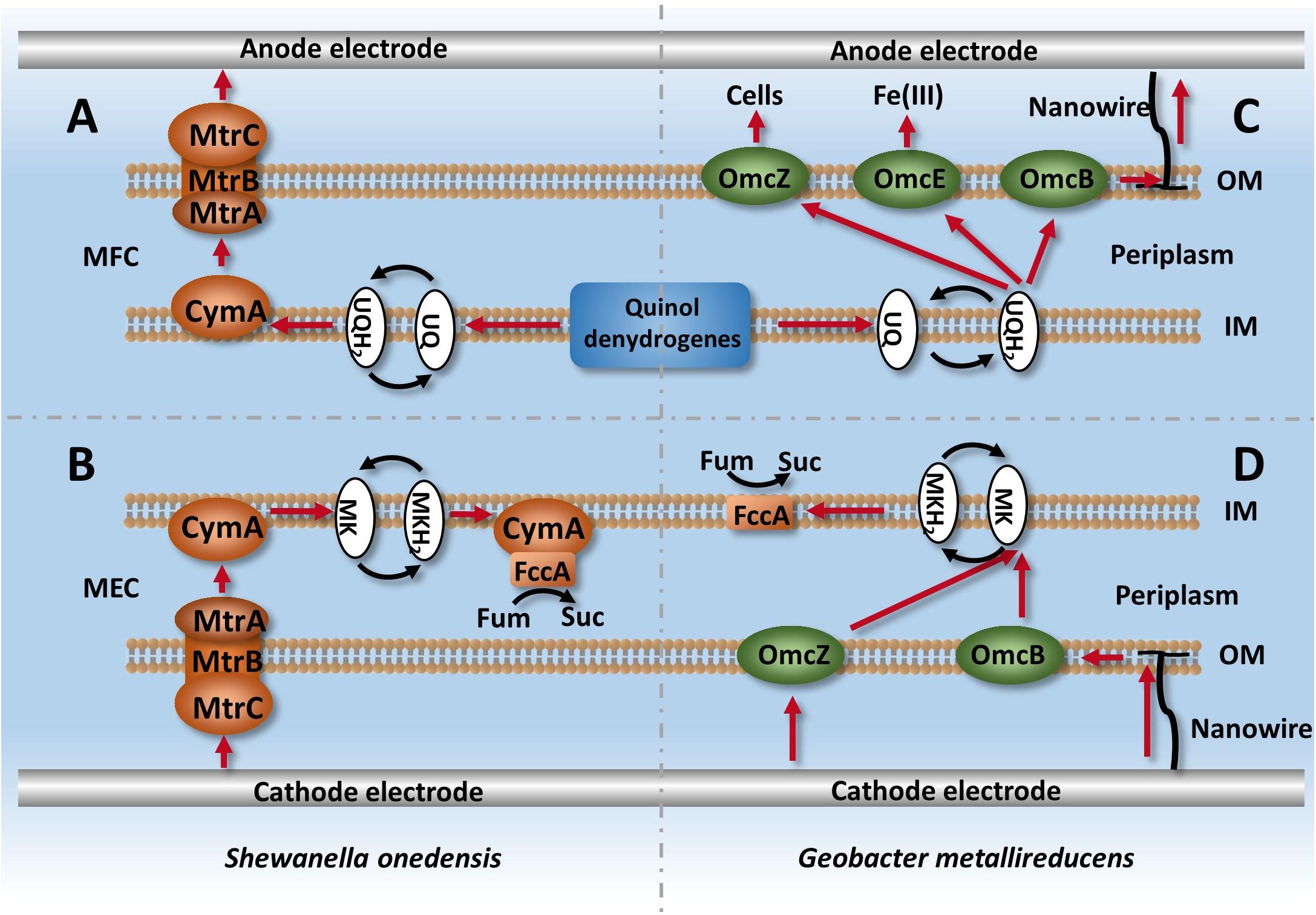
Figure 2. Intracellular electron transfer chains in Shewanella and Geobacter. (A) Describes the process of electron leaking from intracellular metabolism via Mtr and CymA systems in Shewanella, (B) Describes the process of electron capturing by strains from cathodes and used for fumarate reduction. (C,D) describe the ETCs of Geobacter in MFC and MEC systems, respectively.
Electrochemically inactive bacteria can interact with electrodes in the presence of electron shuttles (Table 2). Bio-based electron shuttles, such as riboflavin, coenzyme Q or its analogs, can integrate into inherent electron transfer chains directly via membrane transporters or diffusion, and participate in intracellular reducing and energy metabolism (Schroder, 2007). Compared with bio-based electron shuttles, chemical based electron shuttles have a faster diffusion rate, and their high polarization characteristics ensure effective bidirectional transportation across membranes (Federico et al., 2007; Pandit and Mahadevan, 2011). However, the relationship between chemical-based electron shuttles and intracellular ETC is not clear. Some studies showed that neutral red can integrate into membranes and execute the function of native electron transfer mediators (Park et al., 1999). Recently, Harrington et al. (2015) verified that neutral red can interact with menaquinone and then transfer the electrons into intracellular ETC. Another type of electron shuttles are reduced chemical substances, such as hydrogen and formate (Zhao et al., 2006).
Until now, it has been confirmed that electron shuttles can capture or release electrons between electrodes and intracellular ETC even though the mechanism is not clear. Compared with electrochemically active strains, the electron transfer efficiency of intracellular ETC in electrochemically inactive strains in not effective. Thus, metabolic engineering strategies have been applied to reconstruct the intracellular ETC to promote electron transfer in electrochemically inactive bacteria. Sturm-Richter et al. (Sturm et al., 2015) found that heterologous expression of intracellular ETC from Shewanella (CymA and Mtr system) in E. coli can reprogram the intracellular metabolism and accelerate the intracellular electron transfer rate by 183% (Sturm et al., 2015). In addition, higher electricity power output was achieved with the assistance of methylene blue. Similar results showed that the rate of extracellular electron transfer can also be increased by heterologous introduction of a synthetic flavin pathway in Shewanella (Yang et al., 2015).
The Effects and Applications of Bioelectrochemical Systems in Microbial Metabolism
Electrons are not simply transferred along with the potential gradient between the electrode and cell, or by intracellular electron transfer chains in bioelectrochemical systems, and the reason for the presence of electron carriers is not just to transfer electrons by a simple pattern from an electrode to intracellular ETC. The capturing of electrons is often accompanied by the cotransport of protons (Pandit and Mahadevan, 2011), which can be released and involved in intracellular reduction and energy metabolism. In addition, the reducing power (NADH or FADH2) and ATP play a vital role in intracellular redox metabolism, metabolite synthesis and transportation, stress responses and transcriptional regulation (Balzer et al., 2013; Man et al., 2016). The perturbation of intracellular ATP and NADH levels has effects on the whole cellular metabolism and redirects the metabolic flux (Holm et al., 2010).
Energy Metabolism
In bioelectrochemical systems, bacteria can gain energy in two ways. First, the native respiration chain is the main route of energy generation. For electrochemically active strains, insoluble metals act as electron acceptors and participate in extracellular ETC in nature. When Geobacter and Shewanella were inoculated in MFC system, they could gain energy continuously by degrading organic acids for cell growth and metabolism. And the redox balance was maintained by releasing electrons to the anode, which replaces insoluble metals as the final electron accepter (Lin et al., 2018; Li et al., 2019; Wang et al., 2019).
The second way is also derived from electron transfer. As electrons transferred from cathodes into an intracellular environment, along with the cotransport of protons, the hypothetical mechanism of ATP generation is that the released proton will promote the formation of proton motive force (PMF) and drive ATP synthesis (Rose and Regan, 2015).
Compared with aerobic respiration, the energy generated by oxidative phosphorylation is not enough for cell growth at high rates under anaerobic conditions. The main reason is that due to the low supply level of intracellular ATP, as the intermediate metabolites acted as electron acceptors they were not matched by oxygen levels (Unden and Bongaerts, 1997). Generally, as synthesis of the cell appendages is an energy consuming process, an electron shuttle can be used for supplying more energy through highly effective electron transfer under the same conditions (Liu et al., 2012). In some cases, bacteria can gain enough energy to maintain cell growth and metabolism in MEC systems, even though they do not have a complete native electron transfer chain (Smith et al., 2015).
Co-enzyme Metabolism
The concentration of co-enzymes, NADH or NADPH, represents the level of intracellular reducing power and are involved in many oxidation-reduction reactions. As redox reactions occur inevitably along with electron generation and consumption, the release or capture of electrons in bioelectrochemical systems will disturb the intracellular steady state environment and cause a shift of metabolic flux (Chen et al., 2012).
In electrochemical systems, oxidation and reduction reactions are performed in the anode and cathode chamber, respectively. In MECs, Geobacter can catalyze fumarate reduction with the cathode electrode as the sole electron donor, and this reduction was catalyzed by fumarate dehydrogenase (NADH-dependent) (Mahadevan et al., 2006). For electrochemically inactive bacteria, previous studies have also found that the electrons can be transferred from the cathode to ferric iron via NADH generation when using ferric citrate as the electron acceptor by E. coli, and the whole process can be achieved without any membrane electron transport carriers (Emde et al., 1989). Meanwhile, an analysis of the theory revealed that the intracellular reducing power (NADH) could be enhanced through biological electrolytic synthesis, and the increased concentration of NADH could affect the intracellular reducing and energy reaction (Pandit and Mahadevan, 2011).
Reversely, the efflux of electrons from the anode chamber may create a relative oxidizing intracellular environment. In an MFC system with Lactococcus lactis, homolactic fermentation switched to mixed acid fermentation to keep the balance of intracellular reducing power along with the electricity power output (Freguia et al., 2009).
The Applications of Bioelectrochemical Systems
Based on the effects of bioelectrochemical systems in microbial metabolism, diverse microbial electrochemical technologies were mainly applied to the production of valuable compounds and the generation of power (Fan et al., 2018).
Microbial electrosynthesis is a novel hybrid of biobased and electrochemical approaches to utilize microbial cells to convert dissolved CO2 into value-added organic compounds, such as CH4 production with Methanococcus maripaludis through a self-secreted compounds to promote CO2 reduction (Deutzmann et al., 2015), and acetate production with Sporomusa ovata using a novel cathode to facilitate direct delivery of CO2 to microbes (Bian et al., 2018).
Electro-fermentation (EF) also uses electrochemistry to affect microbial metabolism. The electron transfer in either anodic EF or cathodic EF can regulate the ORP and the NAD+/NADH ratio and then affect the intracellular metabolism (Moscoviz et al., 2016). Recently, an anodic electro-fermentation was carried out using Corynebacterium glutamicum to produce L-lysine, and the results showed that adoption of anodic electro-fermentation can balance the redox and energy states of C. glutamicum and thus improve the anaerobic production of L-lysine (Vassilev et al., 2018). Cathodic electro-fermentation was also performed for simultaneous biogas upgrading and biochemical production, and the highest biogas content [96% (v/v)] and acetate production (358 mg/L) were achieved (Omar et al., 2018).
Photosynthetic MFCs that combined photosynthesis and generation of electric energy, have also gained much attention recently due to their more sustainable energy production than that of non-photosynthetic MFCs (Pillot et al., 2019). The microbes in photosynthetic MFCs usually contain certain specialized light harvesting complexes that function as the units of photosynthesis. These light harvesting units can sustainably convert solar energy into chemical energy, which are then utilized by traditional exoelectrogens to produce electric energy (Rashid et al., 2019). The integration of photosynthesis with MFC technology has opened several neoteric possibilities for sustainable bioenergy generation.
Future Prospects and Conclusion
All types of microbial electrochemical technologies are based on the energy interchange: chemical energy into electrical energy (MFCs), electric energy into chemical energy (MECs) and solar energy into electrical energy (Photosynthetic MFCs). For all types of bioelectrochemical systems, energy conversion efficiency is the key factor that determines bioelectrochemical system performance, especially the energy conversion step in which electrical energy is involved. Electron transfer plays an important role in this step, which it is implied is a future direction for METs research. The electron’s behavior, intracellular reducing power and energy metabolism in bioelectrochemical systems is of increasing concern in the context of precise regulation of fermentation and degradation. Understanding the mechanism of electron transfer via extracellular and intracellular electron transfer chains would extend the future application of bioelectrochemical systems.
Author Contributions
TZ, JL, and YJ wrote the manuscript. WZ, YF, FX, WD, PW, and MJ provided literature and data. JM contributed to the writing of the manuscript and the overall manuscript design.
Funding
This work was supported by the National Key Research and Development Program of China (2018YFA0901500), the National Natural Science Foundation of China (21727818 and 21706124), the Key Science and Technology Project of Jiangsu Province (BE2016389), and the Jiangsu Synergetic Innovation Center for Advanced Bio-Manufacture of China.
Conflict of Interest
The authors declare that the research was conducted in the absence of any commercial or financial relationships that could be construed as a potential conflict of interest.
References
Amano, Y., Koto, A., Matsuzaki, S., Sakamoto, H., Satomura, T., and Suye, S. I. (2016). Construction of a biointerface on a carbon nanotube surface for efficient electron transfer. Mater. Lett. 174, 184–187. doi: 10.1016/j.matlet.2016.03.113
Amelia-Elena, R., Pravin Malla, S., Fanghua, L., Beatrice, M., Shanshan, C., Nevin, K. P., et al. (2014). Direct interspecies electron transfer between Geobacter metallireducens and Methanosarcina barkeri. Appl. Environ. Microbiol. 80:4599. doi: 10.3389/fmicb.2016.00236
Balzer, G. J., Thakker, C., Bennett, G. N., and San, K. Y. (2013). Metabolic engineering of Escherichia coli to minimize byproduct formate and improving succinate productivity through increasing NADH availability by heterologous expression of NAD+-dependent formate dehydrogenase. Metab. Eng. 20, 1–8. doi: 10.1016/j.ymben.2013.07.005
Bari, C. D., Goñi-Urtiaga, A., Pita, M., Shleev, S., Toscano, M. D., Sainz, R., et al. (2016). Fabrication of high surface area graphene electrodes with high performance towards enzymatic oxygen reduction. Electrochim. Acta 191, 500–509. doi: 10.1016/j.electacta.2016.01.101
Bian, B., Manal, A., Krishna, K., Defei, L., Suman, B., Zhiping, L., et al. (2018). Porous nickel hollow fiber cathodes coated with CNTs for efficient microbial electrosynthesis of acetate from CO2 using Sporomusa ovata. J. Mater. Chem. A 6.35, 17201–17211. doi: 10.1039/c8ta05322g
Bretschger, O., Obraztsova, A., Sturm, C. A., Chang, I. S., Gorby, Y. A., Reed, S. B., et al. (2007). Current production and metal oxide reduction by Shewanella oneidensis MR-1 wild type and mutants. Appl. Environ. Microbiol. 73, 7003–7012. doi: 10.1128/aem.01087-07
Brutinel, E. D., and Gralnick, J. A. (2012). Shuttling happens: soluble flavin mediators of extracellular electron transfer in Shewanella. Appl. Microbiol. Biotechnol. 93, 41–48. doi: 10.1007/s00253-011-3653-0
Butler, J. E., Young, N. D., and Lovley, D. R. (2010). Evolution of electron transfer out of the cell: comparative genomics of six Geobacter genomes. BMC Genom. 11:40. doi: 10.1186/1471-2164-11-40
Carmona-Martínez, A. A., Harnisch, F., Kuhlicke, U., Neu, T. R., and Schröder, U. (2013). Electron transfer and biofilm formation of Shewanella putrefaciens as function of anode potential. Bioelectrochemistry 93, 23–29. doi: 10.1016/j.bioelechem.2012.05.002
Carmona-Martínez, A. A., Trably, E., Milferstedt, K., Lacroix, R., Etcheverry, L., and Bernet, N. (2015). Long-term continuous production of H2 in a microbial electrolysis cell (MEC) treating saline wastewater. Water Res. 81, 149–156. doi: 10.1016/j.watres.2015.05.041
Chen, F., Feng, X. H., Liang, J. F., and Xu, H. (2012). An oxidoreduction potential shift control strategy for high purity propionic acid production by Propionibacterium freudenreichii CCTCC M207015 with glycerol as sole carbon source. Bioprocess Biosyst. Eng. 36, 1165–1176. doi: 10.1007/s00449-012-0843-9
Choi, O., and Sang, B. I. (2016). Extracellular electron transfer from cathode to microbes: application for biofuel production. Biotechnol. Biofuels 9, 1–14. doi: 10.1186/s13068-016-0426-0
Commault, A. S., Gavin, L., and Weld, R. J. (2015). Maintenance of Geobacter-dominated biofilms in microbial fuel cells treating synthetic wastewater. Bioelectrochemistry 106, 150–158. doi: 10.1016/j.bioelechem.2015.04.011
Compagnone, D., and Guilbault, G. G. (1997). Glucose oxidase/hexokinase electrode for the determination of ATP. Anal. Chim. Acta 340, 109–113. doi: 10.1016/s0003-2670(96)00451-5
Degani, Y., and Heller, A. (1987). Direct electrical communication between chemically modified enzymes and metal electrodes. I. Electron transfer from glucose oxidase to metal electrodes via electron relays, bound covalently to the enzyme. J. Am. Chem. Soc. 110, 708–715.
Deutzmann, J. S., Sahin, M., and Spormann, A. M. (2015). Extracellular enzymes facilitate electron uptake in biocorrosion and bioelectrosynthesis. mBio 6:e00496-15. doi: 10.1128/mBio.00496-15
Ding, A., Yu, Y., Sun, G., and Wu, D. (2012). Impact of applied voltage on methane generation and microbial activities in an anaerobic microbial electrolysis cell (MEC). Chem. Eng. J. 283, 260–265. doi: 10.1016/j.cej.2015.07.054
Dumas, C., Basseguy, R., and Bergel, A. (2008). Microbial electrocatalysis with Geobacter sulfurreducens biofilm on stainless steel cathodes. Electrochim. Acta 53, 2494–2500. doi: 10.1016/j.electacta.2007.10.018
Emde, R., Swain, A., and Schink, B. (1989). Anaerobic oxidation of glycerol by Escherichia coli in an amperometric poised-potential culture system. Appl. Microbiol. Biotechnol. 32, 170–175. doi: 10.1007/bf00165883
Fan, L., Guo, K. J., Duan, H. W., Shao, L. M., and He, P. J. (2018). Exploit carbon materials to accelerate initiation and enhance process stability of CO anaerobic open-culture Fermentation. ACS Sustain. Chem. Eng. 6, 2787–2796. doi: 10.1021/acssuschemeng.7b04589
Farzaneh, R., Defeng, X., Rachel, W., Regan, J. M., Richard, T. L., and Logan, B. E. (2009). Simultaneous cellulose degradation and electricity production by Enterobacter cloacae in a microbial fuel cell. Appl. Environ. Microbiol. 75, 3673–3678. doi: 10.1128/AEM.02600-08
Federico, A., Alessandro, C., Mauro, M., Stefania, P., Priscilla, R., and Simona, R. (2007). Electron transfer from a solid-state electrode assisted by methyl viologen sustains efficient microbial reductive dechlorination of TCE. Environ. Sci. Technol. 41, 2554–2559. doi: 10.1021/es0624321
Fernandez, M. D. L., Sanromán, M. D. L., Marks, S., Makinia, J., Campo, A. G. D., Rodrigo, M., et al. (2015). A grey box model of glucose fermentation and syntrophic oxidation in microbial fuel cells. Bioresour. Technol. 200:396. doi: 10.1016/j.biortech.2015.10.010
Freguia, S., Masuda, M., Tsujimura, S., and Kano, K. (2009). Lactococcus lactis catalyses electricity generation at microbial fuel cell anodes via excretion of a soluble quinone. Bioelectrochemistry 76, 14–18. doi: 10.1016/j.bioelechem.2009.04.001
Freguia, S., Rabaey, K., Yuan, Z., and Keller, J. (2008). Sequential anode–cathode configuration improves cathodic oxygen reduction and effluent quality of microbial fuel cells. Water Res. 42, 1387–1396. doi: 10.1016/j.watres.2007.10.007
Freguia, S., Virdis, B., Harnisch, F., and Keller, J. (2012). Bioelectrochemical systems: microbial versus enzymatic catalysis. Electrochim. Acta 82, 165–174. doi: 10.1002/cssc.201100835
Ghosh, R. S., and Ghangrekar, M. M. (2015). Enhancing organic matter removal, biopolymer recovery and electricity generation from distillery wastewater by combining fungal fermentation and microbial fuel cell. Bioresour. Technol. 176, 8–14. doi: 10.1016/j.biortech.2014.10.158
Gildemyn, S., Verbeeck, K., Jansen, R., and Rabaey, K. (2017). The type of ion selective membrane determines stability and production levels of microbial electrosynthesis. Bioresour. Technol. 224, 358–364. doi: 10.1016/j.biortech.2016.11.088
Gul, M. M., and Ahmad, K. S. (2019). Bioelectrochemical systems: sustainable bio-energy powerhouses. Biosens. Bioelectron. 142:111576. doi: 10.1016/j.bios.2019.111576
Gutierrez, F. A., Rubianes, M. D., and Rivas, G. A. (2016). Electrochemical sensor for amino acids and glucose based on glassy carbon electrodes modified with multi-walled carbon nanotubes and copper microparticles dispersed in polyethylenimine. J. Electroanalyt. Chem. 765, 16–21. doi: 10.1016/j.jelechem.2015.10.029
Hara, K. Y., and Kondo, A. (2015). ATP regulation in bioproduction. Microb. Cell Factor. 14:198. doi: 10.1186/s12934-015-0390-6
Harrington, T. D., Tran, V. N., Mohamed, A., Renslow, R., Biria, S., Orfe, L., et al. (2015). The mechanism of neutral red-mediated microbial electrosynthesis in Escherichia coli: menaquinone reduction. Bioresour. Technol. 192, 689–695. doi: 10.1016/j.biortech.2015.06.037
He, A. Y., Yin, C. Y., Xu, H., Kong, X. P., Xue, J. W., Zhu, J., et al. (2016). Enhanced butanol production in a microbial electrolysis cell by Clostridium beijerinckii IB4. Bioprocess Biosyst. Eng. 39, 245–254. doi: 10.1007/s00449-015-1508-2
He, C. S., Mu, Z. X., Yang, H. Y., Wang, Y. Z., Mu, Y., and Yu, H. Q. (2015). Electron acceptors for energy generation in microbial fuel cells fed with wastewaters: a mini-review. Chemosphere 140, 12–17. doi: 10.1016/j.chemosphere.2015.03.059
Holm, A. K., Blank, L. M., Marco, O., Andreas, S., Christian, S., Jensen, P. R., et al. (2010). Metabolic and transcriptional response to cofactor perturbations in Escherichia coli. J. Biol. Chem. 285, 17498–17506. doi: 10.1074/jbc.M109.095570
Jafary, T., Wan, R. W. D., Ghasemi, M., Kim, B. H., Jahim, J. M., Ismail, M., et al. (2015). Biocathode in microbial electrolysis cell; present status and future prospects. Renew. Sustain. Energy Rev. 47, 23–33.
Karthikeyan, R., Wang, B., Xuan, J., Wong, J. W. C., Lee, P. K. H., and Leung, M. K. H. (2015). Interfacial electron transfer and bioelectrocatalysis of carbonized plant material as effective anode of microbial fuel cell. Electrochim. Acta 157, 314–323. doi: 10.1016/j.electacta.2015.01.029
Kracher, D., Scheiblbrandner, S., Felice, A. K. G., Breslmayr, E., Preims, M., Ludwicka, K., et al. (2016). Extracellular electron transfer systems fuel cellulose oxidative degradation. Science 352:aaf3165. doi: 10.1126/science.aaf3165
Kracke, F., Vassilev, I., and Krömer, J. O. (2015). Microbial electron transport and energy conservation – the foundation for optimizing bioelectrochemical systems. Front. Microbiol. 6:575. doi: 10.3389/fmicb.2015.00575
Laura, R., Yolanda, R., Baeza, J. A., Albert, G., and Pilar, C. (2015). Microbial community analysis in a long-term membrane-less microbial electrolysis cell with hydrogen and methane production. Bioelectrochemistry 106, 359–368. doi: 10.1016/j.bioelechem.2015.06.003
Ledezma, P., Donose, B. C., Freguia, S., and Keller, J. (2015). Oxidised stainless steel: a very effective electrode material for microbial fuel cell bioanodes but at high risk of corrosion. Electrochim. Acta 158, 356–360. doi: 10.1016/j.electacta.2015.01.175
Lee, C. Y., Reuillard, B., Sokol, K. P., Laftsoglou, T., Lockwood, C. W., Rowe, S. F., et al. (2016). A decahaem cytochrome as an electron conduit in protein-enzyme redox processes. Chem. Commun. 52, 7390–7393. doi: 10.1039/c6cc02721k
Li, F., An, X., Wu, D., Xu, J., Chen, Y., Li, W., et al. (2019). Engineering mcrobial consortia for high-performance cellulosic hydrolyzates-fed microbial fuel cells. Front. Microbiol. 10:6937. doi: 10.3389/fmicb.2019.00409
Li, H., Liao, B., Xiong, J., Zhou, X., Zhi, H., Liu, X., et al. (2018). Power output of microbial fuel cell emphasizing interaction of anodic binder with bacteria. J. Power Sources 379, 115–122. doi: 10.1016/j.jpowsour.2018.01.040
Li, J. J., Gao, M. M., Zhang, G., Wang, X. H., Wang, S. G., Song, C., et al. (2015). Perchlorate reduction in microbial electrolysis cell with polyaniline modified cathode. Bioresour. Technol. 177, 74–79. doi: 10.1016/j.biortech.2014.11.065
Lin, T., Ding, W., Sun, L., Wang, L., Liu, C.-G., and Song, H. (2018). Engineered Shewanella oneidensis-reduced graphene oxide biohybrid with enhanced biosynthesis and transport of flavins enabled a highest bioelectricity output in microbial fuel cells. Nano Energy 50, 639–648. doi: 10.1016/j.nanoen.2018.05.072
Liu, F., Rotaru, A. E., Shrestha, P. M., Malvankar, N. S., and Lovley, D. R. (2012). Promoting direct interspecies electron transfer with activated carbon. Energy Environ. Sci. 5, 8982–8989. doi: 10.1016/j.biortech.2018.03.050
Logan, B. E., Bert, H., René, R., Uwe, S. D., Jürg, K., Stefano, F., et al. (2006). Microbial fuel cells: methodology and technology. Environ. Sci. Technol. 40, 5181–5192.
Lovley, D. R. (2008). The microbe electric: conversion of organic matter to electricity. Curr. Opin. Biotechnol. 19, 564–571. doi: 10.1016/j.copbio.2008.10.005
Lovley, D. R. (2012). Electromicrobiology. Ann. Rev. Microbiol. 66:391. doi: 10.1146/annurev-micro-092611-150104
Mahadevan, R., Bond, D., Butler, J. N., Coppi, M., Palsson, B., Schilling, C., et al. (2006). Characterization of metabolism in the Fe(III)-reducing organism Geobacter sulfurreducens by constraint-based modeling. Appl. Environ. Microbiol. 72, 1558–1568. doi: 10.1128/aem.72.2.1558-1568.2006
Man, Z., Rao, Z., Xu, M., Guo, J., Yang, T., Zhang, X., et al. (2016). Improvement of the intracellular environment for enhancing L-arginine production of Corynebacterium glutamicum by inactivation of H2O2-forming flavin reductases and optimization of ATP supply. Metab. Eng. 38, 310–321. doi: 10.1016/j.ymben.2016.07.009
Masuda, M., Freguia, S., Wang, Y. F., Tsujimura, S., and Kano, K. (2010). Flavins contained in yeast extract are exploited for anodic electron transfer by Lactococcus lactis. Bioelectrochemistry 78, 173–175. doi: 10.1016/j.bioelechem.2009.08.004
Mayo, S. L., Ellis, W. R., Crutchley, R. J., and Gray, H. B. (1986). Long-range electron transfer in heme proteins. Science 233, 948–952. doi: 10.1126/science.3016897
Moscoviz, R., Toledo-Alarcón, J., Trably, E., and Bernet, N. (2016). Electro-fermentation: how to drive fermentation using electrochemical systems. Trends Biotechnol. 34, 856–865. doi: 10.1016/j.tibtech.2016.04.009
Omar, B., Abou-Shanab, R., El-Gammal, M., Fotidis, I. A., Kougias, P. G., Zhang, Y., et al. (2018). Simultaneous biogas upgrading and biochemicals production using anaerobic bacterial mixed cultures. Water Res. 142, 86–95. doi: 10.1016/j.watres.2018.05.049
Oscar, G. S., David, O., Marcos, P., Batista, A. P., Alvaro, A., Pereira, M. M., et al. (2014). Reconstitution of respiratory complex I on a biomimetic membrane supported on gold electrodes. Langmuir 30, 9007–9015. doi: 10.1021/la501825r
Pandit, A. V., and Mahadevan, R. (2011). In silico characterization of microbial electrosynthesis for metabolic engineering of biochemicals. Microb. Cell Factor. 10, 1–14. doi: 10.1186/1475-2859-10-76
Pareek, A., Shanthi Sravan, J., and Venkata Mohan, S. (2019). Exploring chemically reduced graphene oxide electrode for power generation in microbial fuel cell. Mater. Sci. Energy Technol. 2, 600–606. doi: 10.1016/j.mset.2019.06.006
Park, D. L. M., Guettler, M. V., Jain, M. K., and Zeikus, J. G. (1999). Microbial utilization of electrically reduced neutral red as the sole electron donor for growth and metabolite production. Appl. Environ. Microbiol. 65, 2912–2917. doi: 10.1128/aem.65.7.2912-2917.1999
Pillot, G., Davidson, S., Auria, R., Combet-Blanc, Y., Godfroy, A., and Liebgott, P.-P. (2019). Production of current by syntrophy between exoelectrogenic and fermentative hyperthermophilic microorganisms in heterotrophic biofilm from a deep-sea hydrothermal chimney. Microb. Ecol. 79, 38–49. doi: 10.1007/s00248-019-01381-z
Potter, M. C. (1911). Electrical effects accompanying the decomposition of organic compounds. Proc. R. Soc. B Biol. Sci. 84, 260–276. doi: 10.1098/rspb.1911.0073
Qu, Y., Feng, Y., Wang, X., and Logan, B. E. (2012). Use of a coculture to enable current production by geobacter sulfurreducens. Appl. Environ. Microbiol. 78, 3484–3487. doi: 10.1128/AEM.00073-12
Rabaey, K., Rodríguez, J., Blackall, L. L., Keller, J., Gross, P., Batstone, D., et al. (2007). Microbial ecology meets electrochemistry: electricity-driven and driving communities. ISME J. 1, 9–18. doi: 10.1038/ismej.2007.4
Rashid, N., Lee, B., and Chang, Y.-K. (2019). “Recent trends in microalgae research for sustainable energy production and biorefinery applications,” in Microalgae Biotechnology for Development of Biofuel and Wastewater Treatment, eds M. Alam and Z. Wang (Singapore: Springer), 3–20. doi: 10.1007/978-981-13-2264-8_1
Richter, H., Nevin, K. P., Jia, H., Lowy, D. A., Lovley, D. R., and Tender, L. M. (2009). Cyclic voltammetry of biofilms of wild type and mutant Geobacter sulfurreducens on fuel cell anodes indicates possible roles of OmcB, OmcZ, type IV pili, and protons in extracellular electron transfer. Energy Environ. Sci. 2:506. doi: 10.1039/b816647a
Rose, N. D., and Regan, J. M. (2015). Changes in phosphorylation of adenosine phosphate and redox state of nicotinamide-adenine dinucleotide (phosphate) in Geobacter sulfurreducens in response to electron acceptor and anode potential variation. Bioelectrochemistry 106, 213–220. doi: 10.1016/j.bioelechem.2015.03.003
Ross, D. E., Flynn, J. M., Baron, D. B., Gralnick, J. A., and Bond, D. R. (2012). Towards electrosynthesis in shewanella: energetics of reversing the mtr pathway for reductive metabolism. PLoS One 6:e16649. doi: 10.1371/journal.pone.0016649
Ruecha, N., Rodthongkum, N., Cate, D. M., Volckens, J., Chailapakul, O., and Henry, C. S. (2015). Sensitive electrochemical sensor using a graphene–polyaniline nanocomposite for simultaneous detection of Zn(II) Cd(II), and Pb(II). Anal. Chim. Acta 874, 40–48. doi: 10.1016/j.aca.2015.02.064
Sadeghifar, M., and Rashid-Nadimi, S. (2017). Nanoporous gold electrode prepared from gold compact disk as the anode for the microbial fuel cell. J. Iran. Chem. Soc. 15, 607–612. doi: 10.1007/s13738-017-1260-4
Santoro, C., Serov, A., Villarrubia, C. W. N., Stariha, S., Babanova, S., Artyushkova, K., et al. (2015). High catalytic activity and pollutants resistivity using Fe-AAPyr cathode catalyst for microbial fuel cell application. Sci. Rep. 5:16596. doi: 10.1038/srep16596
Santoro, C., Soavi, F., Serov, A., Arbizzani, C., and Atanassov, P. (2016). Self-powered supercapacitive microbial fuel cell: the ultimate way of boosting and harvesting power. Biosens. Bioelectron. 78, 229–235. doi: 10.1016/j.bios.2015.11.026
Schroder, U. (2007). Anodic electron transfer mechanisms in microbial fuel cells and their energy efficiency. Phys. Chem. Chem. Phys. 9, 2619–2629. doi: 10.1039/b703627m
She, P., Song, B., Xing, X. H., Loosdrecht, M. V., and Liu, Z. (2006). Electrolytic stimulation of bacteria Enterobacter dissolvens by a direct current. Biochem. Eng. J. 28, 23–29. doi: 10.1016/j.bej.2005.08.033
Shi, L., Squier, T., Zachara, J., and Fredrickson, J. (2010). Respiration of metal (hydr)oxides by Shewanella and Geobacter: a key role for multihaem c-type cytochromes. Mol. Microbiol. 65, 12–20. doi: 10.1111/j.1365-2958.2007.05783.x
Shreeram, D. D., Panmanee, W., Mcdaniel, C. T., Daniel, S., Schaefer, D. W., and Hassett, D. J. (2018). Effect of impaired twitching motility and biofilm dispersion on performance of Pseudomonas aeruginosa-powered microbial fuel cells. J. Indust. Microbiol. Biotechnol. 45, 103–109. doi: 10.1007/s10295-017-1995-z
Smith, J. A., Nevin, K. P., and Lovley, D. R. (2015). Syntrophic growth via quinone-mediated interspecies electron transfer. Front. Microbiol. 6:121. doi: 10.3389/fmicb.2015.00121
Song, T. S., Jin, Y., Bao, J., Kang, D., and Xie, J. (2016). Graphene/biofilm composites for enhancement of hexavalent chromium reduction and electricity production in a biocathode microbial fuel cell. J. Hazard. Mater. 317, 73–80. doi: 10.1016/j.jhazmat.2016.05.055
Sturm, G., Richter, K., Doetsch, A., Heide, H., Louro, R. O., and Gescher, J. (2015). A dynamic periplasmic electron transfer network enables respiratory flexibility beyond a thermodynamic regulatory regime. ISME J. 9, 1802–1811. doi: 10.1038/ismej.2014.264
Su, L., and Ajo-Franklin, C. M. (2019). Reaching full potential: bioelectrochemical systems for storing renewable energy in chemical bonds. Curr. Opin. Biotechnol. 57, 66–72. doi: 10.1016/j.copbio.2019.01.018
Unden, G., and Bongaerts, J. (1997). Alternative respiratory pathways of Escherichia coli: energetics and transcriptional regulation in response to electron acceptors. Biochim. Biophys. Acta Bioenerget. 1320, 217–234. doi: 10.1016/s0005-2728(97)00034-0
Vassilev, I., Gießelmann, G., Schwechheimer, S. K., Wittmann, C., Virdis, B., and Krömer, J. O. (2018). Anodic electro-fermentation: anaerobic production of L-Lysine by recombinant Corynebacterium glutamicum. Biotechnol. Bioeng. 115, 1499–1508. doi: 10.1002/bit.26562
Verea, L., Oumarou, S., Campos, J., Ginez, F., Verde, A., and Sebastian, P. J. (2014). Performance of a microbial electrolysis cell (MEC) for hydrogen production with a new process for the biofilm formation. Int. J. Hydrogen Energy 39, 8938–8946. doi: 10.1016/j.ijhydene.2014.03.203
Villano, M., Monaco, G., Aulenta, F., and Mauro, M. (2011). Electrochemically assisted methane production in a biofilm reactor. J. Power Sources 196, 9467–9472. doi: 10.1016/j.jpowsour.2011.07.016
Wang, G., Guo, Y., Cai, J., Wen, H., Mao, Z., Zhang, H., et al. (2019). Electricity production and the analysis of the anode microbial community in a constructed wetland-microbial fuel cell. RSC Adv. 9, 21460–21472.
Wang, Y., Lun, J., Leng, Q., Wu, Y., He, X., and Wang, K. (2016). Electrochemical sensor for glutathione detection based on mercury ion triggered hybridization chain reaction signal amplification. Biosens. Bioelectron. 77, 914–920. doi: 10.1016/j.bios.2015.10.071
Wetser, K., Sudirjo, E., Buisman, C. J. N., and Strik, D. P. B. T. B. (2015). Electricity generation by a plant microbial fuel cell with an integrated oxygen reducing biocathode. Appl. Energy 137, 151–157. doi: 10.1016/j.apenergy.2014.10.006
Yang, L., Deng, W., Zhang, Y., Tan, Y., Ma, M., and Xie, Q. (2017). Boosting current generation in microbial fuel cells by an order of magnitude by coating an ionic liquid polymer on carbon anodes. Biosens. Bioelectron. 91, 644–649. doi: 10.1016/j.bios.2017.01.028
Yang, Y., Ding, Y., Hu, Y., Cao, B., Rice, S. A., Kjelleberg, S., et al. (2015). Enhancing bidirectional electron transfer of Shewanella oneidensis by a synthetic flavin pathway. ACS Synth. Biol. 4:815. doi: 10.1021/sb500331x
Yazdi, H., Alzate-Gaviria, L., and Ren, Z. J. (2015). Pluggable microbial fuel cell stacks for septic wastewater treatment and electricity production. Bioresour. Technol. 180, 258–263. doi: 10.1016/j.biortech.2014.12.100
Yin, Q., Zhu, X., Zhan, G., Bo, T., Yang, Y., Tao, Y., et al. (2016). Enhanced methane production in an anaerobic digestion and microbial electrolysis cell coupled system with co-cultivation of Geobacter and Methanosarcina. J. Environ. Sci. China 42, 210–214. doi: 10.1016/j.jes.2015.07.006
Yuan, M., Kummer, M. J., and Minteer, S. D. (2019). Strategies for bioelectrochemical CO2 reduction. Chemistry 25, 14258–14266. doi: 10.1002/chem.201902880
Yuan, Y., Shin, H., Kang, C., and Kim, S. (2016). Wiring microbial biofilms to the electrode by osmium redox polymer for the performance enhancement of microbial fuel cells. Bioelectrochemistry 108, 8–12. doi: 10.1016/j.bioelechem.2015.11.001
Zhang, D., Li, Z., Zhang, C., Zhou, X., Xiao, Z., Awata, T., et al. (2017). Phenol-degrading anode biofilm with high coulombic efficiency in graphite electrodes microbial fuel cell. J. Biosci. Bioeng. 123, 364–369. doi: 10.1016/j.jbiosc.2016.10.010
Zhang, Y., and Angelidaki, I. (2015). Bioelectrochemical recovery of waste-derived volatile fatty acids and production of hydrogen and alkali. Water Res. 81, 188–195. doi: 10.1016/j.watres.2015.05.058
Zhao, C., Gai, P. P., Song, R., Zhang, J. R., and Zhu, J. J. (2015). Graphene/Au composites as an anode modifier for improving electricity generation in Shewanella-inoculated microbial fuel cells. Anal. Methods 7, 4640–4644. doi: 10.1039/c5ay00976f
Zhao, F., Falk, H., Uwe, S. D., Fritz, S., Peter, B., and Iris, H. (2006). Challenges and constraints of using oxygen cathodes in microbial fuel cells. Environ. Sci. Technol. 40, 5193–5199. doi: 10.1021/es060332p
Zhao, Z., Zhang, Y., Quan, X., and Zhao, H. (2015a). Evaluation on direct interspecies electron transfer in anaerobic sludge digestion of microbial electrolysis cell. Bioresour. Technol. 200:235. doi: 10.1016/j.biortech.2015.10.021
Zhao, Z., Zhang, Y., Wang, L., and Quan, X. (2015b). Potential for direct interspecies electron transfer in an electric-anaerobic system to increase methane production from sludge digestion. Sci. Rep. 5:11094. doi: 10.1038/srep11094
Zhen, G., Lu, X., Kobayashi, T., Kumar, G., and Xu, K. (2016). Promoted electromethanosynthesis in a two-chamber microbial electrolysis cells (MECs) containing a hybrid biocathode covered with graphite felt (GF). Chem. Eng. J. 284, 1146–1155. doi: 10.1016/j.cej.2015.09.071
Zheng, S., Zhang, H., Li, Y., Zhang, H., Wang, O., Zhang, J., et al. (2015). Co-occurrence of Methanosarcina mazei and Geobacteraceae in an iron (III)-reducing enrichment culture. Front. Microbiol. 6:941. doi: 10.3389/fmicb.2015.00941
Keywords: bioelectrochemical system, electron transfer, microbial fuel cells, microbial electrolysis cells, energy generation, coenzyme metabolism
Citation: Zheng T, Li J, Ji Y, Zhang W, Fang Y, Xin F, Dong W, Wei P, Ma J and Jiang M (2020) Progress and Prospects of Bioelectrochemical Systems: Electron Transfer and Its Applications in the Microbial Metabolism. Front. Bioeng. Biotechnol. 8:10. doi: 10.3389/fbioe.2020.00010
Received: 04 September 2019; Accepted: 08 January 2020;
Published: 31 January 2020.
Edited by:
Yuan Lu, Tsinghua University, ChinaReviewed by:
Yang-Chun Yong, Jiangsu University, ChinaEnrico Marsili, Nazarbayev University, Kazakhstan
Copyright © 2020 Zheng, Li, Ji, Zhang, Fang, Xin, Dong, Wei, Ma and Jiang. This is an open-access article distributed under the terms of the Creative Commons Attribution License (CC BY). The use, distribution or reproduction in other forums is permitted, provided the original author(s) and the copyright owner(s) are credited and that the original publication in this journal is cited, in accordance with accepted academic practice. No use, distribution or reproduction is permitted which does not comply with these terms.
*Correspondence: Jiangfeng Ma, bWFqaWFuZ2ZlbmdAbmp0ZWNoLmVkdS5jbg==