- 1Fungal Physiology, Westerdijk Fungal Biodiversity Institute and Fungal Molecular Physiology, Utrecht University, Utrecht, Netherlands
- 2Fungal Natural Products, Westerdijk Fungal Biodiversity Institute, Utrecht, Netherlands
- 3US Department of Energy Joint Genome Institute, Walnut Creek, CA, United States
- 4Department of Microbiology, University of Helsinki, Helsinki, Finland
Cinnamic acid is an aromatic compound commonly found in plants and functions as a central intermediate in lignin synthesis. Filamentous fungi are able to degrade cinnamic acid through multiple metabolic pathways. One of the best studied pathways is the non-oxidative decarboxylation of cinnamic acid to styrene. In Aspergillus niger, the enzymes cinnamic acid decarboxylase (CdcA, formally ferulic acid decarboxylase) and the flavin prenyltransferase (PadA) catalyze together the non-oxidative decarboxylation of cinnamic acid and sorbic acid. The corresponding genes, cdcA and padA, are clustered in the genome together with a putative transcription factor previously named sorbic acid decarboxylase regulator (SdrA). While SdrA was predicted to be involved in the regulation of the non-oxidative decarboxylation of cinnamic acid and sorbic acid, this was never functionally analyzed. In this study, A. niger deletion mutants of sdrA, cdcA, and padA were made to further investigate the role of SdrA in cinnamic acid metabolism. Phenotypic analysis revealed that cdcA, sdrA and padA are exclusively involved in the degradation of cinnamic acid and sorbic acid and not required for other related aromatic compounds. Whole genome transcriptome analysis of ΔsdrA grown on different cinnamic acid related compounds, revealed additional target genes, which were also clustered with cdcA, sdrA, and padA in the A. niger genome. Synteny analysis using 30 Aspergillus genomes demonstrated a conserved cinnamic acid decarboxylation gene cluster in most Aspergilli of the Nigri clade. Aspergilli lacking certain genes in the cluster were unable to grow on cinnamic acid, but could still grow on related aromatic compounds, confirming the specific role of these three genes for cinnamic acid metabolism of A. niger.
Introduction
Cinnamic acid, an aromatic compound with a distinct aroma, is naturally found as a free compound in several plants, such as Cinnamomum verum, C. cassia, and C. zeylanicum (He et al., 2005; Gruenwald et al., 2010). Cinnamic acid is also one of the central intermediates for the biosynthesis of lignin, flavonoids and coumarins in plants (Hoskins, 1984; Chemler and Koffas, 2008; Vargas-Tah and Gosset, 2015) and it is synthesized through the deamination of phenylalanine (Yamada et al., 1981). High concentrations of cinnamic acid can accumulate in soil, especially in those soils that are continuously used for crop cultivation, where it is released by root exudation and decaying plant tissue (Xie and Dai, 2015; Latif et al., 2017). Cinnamic acid has antioxidant and antimicrobial properties, it can be used as a precursor for the synthesis of thermoplastics and flavoring agents, and it is also widely used in cosmetic and health products (Chemler and Koffas, 2008; Vargas-Tah and Gosset, 2015). Hence, recent studies have focused on metabolic engineering of the microbial shikimate pathway to produce cinnamic acid from simple sugars and complex substrates (Thompson et al., 2015; Vargas-Tah and Gosset, 2015; Averesch and Krömer, 2018).
A recent review summarized the various metabolic pathways used by microorganisms to degrade cinnamic acid, and its role as a carbon source (Figure 1) (Lubbers et al., 2019). One of the most studied pathways of cinnamic acid metabolism is the non-oxidative decarboxylation of cinnamic acid to styrene, which was reported to occur in several fungi, such as Aspergillus, Penicillium, Saccharomyces, and Trichoderma (Marth et al., 1966; Clifford et al., 1969; Milstein et al., 1983; Pinches and Apps, 2007; Lafeuille et al., 2009; Plumridge et al., 2010; Richard et al., 2015; Liewen and Marth, 2016). Two genes were identified in Aspergillus niger and Saccharomyces cerevisiae, that are involved in the non-oxidative decarboxylation of cinnamic acid using prenylated flavin mononucleotide (FMN) as a cofactor to convert cinnamic acid to styrene (Plumridge et al., 2010; Payne et al., 2015). These genes are clustered in the genome and encode a putative 3-octaprenyl-4-hydroxybenzoate carboxy-lyase, referred to as cinnamic acid decarboxylase (cdcA, formerly ferulic acid decarboxylase (fdcA), see Discussion), and a flavin prenyltransferase (padA) (Figure 1) (Plumridge et al., 2010; Payne et al., 2015). In addition, CdcA and PadA also catalyze the decarboxylation of the food preservative sorbic acid to 1,3-pentadiene. An additional pathway was suggested in A. niger in which cinnamic acid is para-hydroxylated to p-coumaric acid (Bocks, 1966), but this seems to play a minor role in A. niger since only small amounts of p-coumaric acid were detected. In Aspergillus japonicus, cinnamic acid was suggested to be reduced to cinnamaldehyde and cinnamyl alcohol (Milstein et al., 1983). Better understanding of the cinnamic acid metabolic pathway in A. niger can aid in the creation of cell factories or unlock new strategies to make valuable aromatic building blocks.
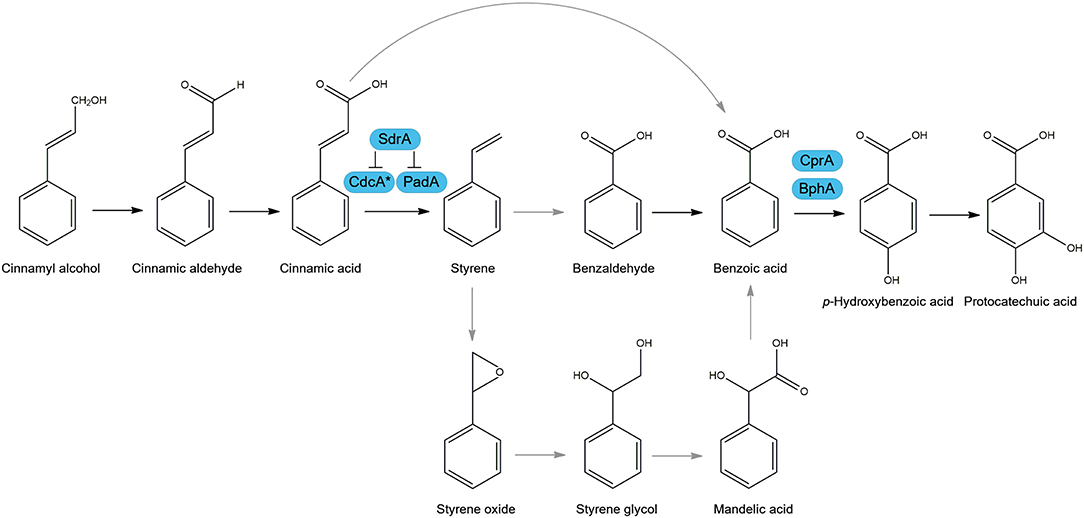
Figure 1. Suggested cinnamic acid metabolic pathways in Aspergillus niger. Arrows in black are observed metabolic conversions in literature and in gray are suggested conversions. Boxed in blue are characterized enzymes of A. niger involved in this metabolic pathway. Cinnamic acid decarboxylase (CdcA), Flavin prenyltransferase (PadA), sorbic acid decarboxylase regulator (SdrA), benzoate 4-monooxygenase (BphA), cytochrome P450 reductase (CprA). *FdcA is renamed to CdcA, see Discussion.
Transcriptional regulation is important in controlling the metabolic flux and energy usage in metabolic processes in microorganisms. Many transcription regulators involved in carbohydrate metabolism of filamentous fungi have been identified (Benocci et al., 2017), but less is known about the transcriptional regulation of aromatic metabolic pathways. It has been shown that a Zn2Cys6-finger transcription factor named sorbic acid decarboxylase regulator (SdrA), is involved in the regulation of cdcA and padA (Plumridge et al., 2010). The corresponding gene (sdrA) is located between cdcA and padA in a gene cluster. While deletion of sdrA revealed that it is essential for the non-oxidative decarboxylation of cinnamic acid, until now its regulatory targets are still unknown. In S. cerevisiae, orthologs of cdcA and padA are also clustered, but no transcription factor has been found between these genes (Mukai et al., 2010; Plumridge et al., 2010).
In this paper, we studied SdrA in more detail in order to identify its regulatory targets using transcriptomic data of the A. niger deletion strain ΔsdrA cultivated in cinnamic acid and sorbic acid. We used whole genome transcriptome analysis of ΔsdrA to identify new regulatory targets and performed a phenotypic analysis of cdcA, padA and sdrA deletion strains to determine the role of CdcA, PadA, and SdrA in other aromatic metabolic pathways. Finally, synteny analysis of the cinnamic acid decarboxylation gene cluster using the genomes of 30 Aspergilli was performed to analyze the conservation of the gene cluster. Growth tests on cinnamic acid were performed on a subset of these species to confirm predictions made on the basis of the synteny analysis.
Materials and Methods
Strains, Media, and Culture Conditions
All strains used in this study are shown in Tables 1, 2, and were grown on complete medium for Aspergillus (CM, de Vries et al., 2004) containing 1.5% (w/v) agar supplemented with 1% fructose and 1.22 g L−1 uridine at 30°C for 4 days. Spores were harvested with 10 mL N-(2-acetamido)-2-aminoethanesulfonic acid buffer. Phenotypic experiments were performed using minimal medium for Aspergillus (MM, de Vries et al., 2004) containing aromatic compounds as sole carbon source, supplemented with 1.22 g L−1 uridine and inoculated with 103 spores in 2 μl. Due to variable toxicity of the aromatic compounds different concentrations were used for the growth profile, i.e., 2 mM for ferulic acid, 3 mM for benzoic acid, benzaldehyde, styrene and 5 mM for the remaining compounds. All aromatic compounds were purchased from Sigma Aldrich.
Construction of Gene Deletion Cassettes and Transformation of A. niger
The gene deletion cassettes were constructed using 1,000 bp upstream and downstream DNA fragment of the gene containing an overlap of the selection marker hygromycin B (hph) from Escherichia coli. The hph selection marker was amplified from plasmid pAN7.1 (Punt et al., 1987). These three fragments were fused in a PCR reaction using the GoTaq Long PCR Master Mix (Promega, Madison, WI, USA). The fusion PCR mixture contained 0.4 μl of each amplified product, 0.6 μl of 10 μM upstream and downstream primers (Supplementary Table 1), 12.5 μl GoTaq Long PCR Master Mix in a total volume of 25 μl. The following PCR conditions were used: 94°C for 2 min, 35 cycles of 94°C for 30 s, 60°C for 30 s, 72°C for 5 min, and a final extension at 72°C for 10 min. A. niger N593 ΔkusA was transformed through protoplast-mediated transformation as described in Kowalczyk et al. (2017).
Transfer Conditions, RNA Extraction, and Transcriptome Analysis
The transcriptomes of the reference strain A. niger N593 and ΔsdrA induced for 2 h in cinnamic acid and sorbic acid were analyzed with RNAseq by DOE Joint Genome Institute (JGI, Walnut Creek, CA, USA). Transfer experiments and subsequent RNA-sequencing were performed in biological triplicates. A. niger strains were pre-grown in 200 mL CM with 2% fructose inoculated with 1 x 106 spores/mL and incubated overnight on rotary shakers at 30°C, 250 rpm. Freshly germinated mycelia were harvested on Miracloth and washed with MM. Equal portions of mycelia were transferred to 250 mL flasks containing 50 mL MM and 0.02% (w/v) cinnamic acid, sorbic acid, benzoic acid, cinnamyl alcohol and salicylic acid. The cultures were incubated on rotary shakers for 2 h at 30°C, 250 rpm. Mycelia were harvested, dried between tissue paper to remove excess liquid and frozen in liquid nitrogen. Frozen mycelia were ground using the tissue lyser (QIAGEN, Hilden, Germany) and total RNA was extracted using TRIzol reagent (Invitrogen, Life Technologies, Carlsbad, CA, USA) and RNA isolation kit (NucleoSpin RNA, MACHEREY-NAGEL GmbH & Co. KG, Düren, Germany) according the manufacturer's recommendation. The quality and quantity of RNA was determined by gel electrophoresis and RNA6000 Nano Assay using the Agilent 2100 Bioanalyzer (Agilent Technologies, Santa Clara, CA, USA). Purification of mRNA, synthesis of cDNA library and sequencing were conducted at the JGI. Data was processed as described in Kowalczyk et al. (2017). The transcriptome data was stored at the NCBI Sequence Read Archive (SRA) (Supplementary Table 2).
Synteny Analysis
BLAST analyses were performed using the amino acid sequence of cdcA, sdrA, padA, NRRL3_8293, NRRL3_8294, NRRL3_8295, NRRL3_8299, NRRL3_8300, and NRRL3_8301 as a query on 30 Aspergillus genomes. All genomes used in this analysis were downloaded from JGI Mycocosm (Grigoriev et al., 2014). Synteny analysis was performed on the cinnamic acid decarboxylation core cluster (cdcA, sdrA, padA) with 20.000 bp on each side. BLAST hits and protein IDs used for the synteny analysis are mentioned in Supplementary Table 3.
Results
CdcA, SdrA, and PadA Are Essential for Cinnamic Acid and Sorbic Acid Utilization
To understand the role of CdcA (NRRL3_8296), SdrA (NRRL3_8297), and PadA (NRRL3_8298) for aromatic metabolism in A. niger, deletion mutants (ΔcdcA, ΔsdrA, and ΔpadA) were made and tested on a set of related aromatic compounds as a sole carbon source. ΔcdcA and ΔpadA resulted in abolished growth on cinnamic acid and reduced growth on sorbic acid, while deletion of ΔsdrA resulted in reduced growth on both cinnamic acid and sorbic acid. This demonstrated that all three genes, cdcA, sdrA, and padA, are important for the metabolism of cinnamic acid and sorbic acid. No growth reduction was observed on other tested aromatic compounds (Figure 2), indicating that cdcA and padA are not essential for the conversion of these related compounds (Figure 2).
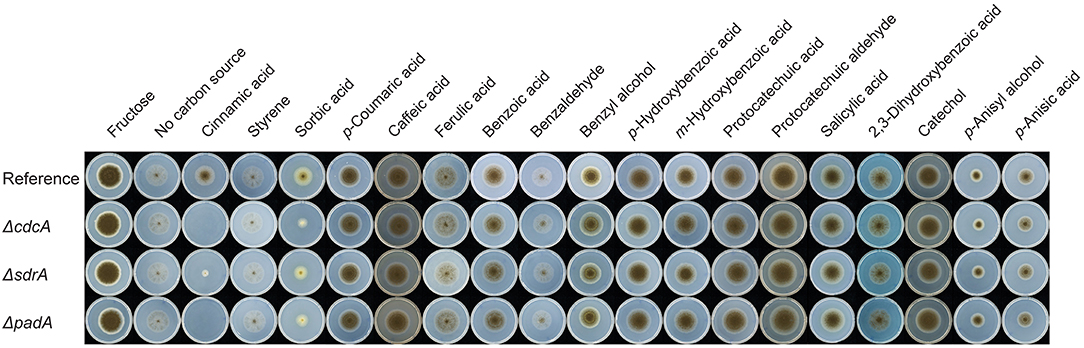
Figure 2. Phenotypic analysis of the cinnamic acid decarboxylase mutants. Strains were grown for 10 days at 30°C. The reference strain is A. niger N593 ΔkusA. Due to variable toxicity of the aromatic compounds different concentrations were used for the growth profile, i.e., 2 mM for ferulic acid, 3 mM for benzoic acid, benzaldehyde, styrene and 5 mM for the remaining compounds. Fructose and the no carbon source were used as growth controls.
Cinnamic Acid and Sorbic Acid Both Induce the Cinnamic Acid and Sorbic Acid Decarboxylation Cluster and Neighboring Genes
A. niger N593 was pre-grown in CM with fructose and transferred to MM containing cinnamic acid, sorbic acid, cinnamyl alcohol, benzoic acid, 2-hydroxybenzoic acid (salicylic acid), or a no carbon source condition and were incubated for 2 h. Cinnamyl alcohol and benzoic acid are both related to cinnamic acid as they are among conversion products of cinnamic acid (Figure 1). The cultivation without any carbon source was used as a reference control to remove background noise between the conditions. Salicylic acid has not been observed to have any connection with cinnamic acid metabolic pathways (Martins et al., 2015). Therefore, it was used as a control representing an aromatic compound, which does not induce the expression of the cinnamic acid decarboxylase cluster.
Genome-wide gene expression analysis of the samples was performed using RNA-seq. A total of 3,921 genes were upregulated in all five conditions by A. niger N593 compared to the no carbon source control (Figure 3). Two thousand four hundred and ninety and two thousand one hundred and fourty eight genes were upregulated on cinnamic acid and sorbic acid, respectively, compared to the no carbon source control, while 211 of these genes were upregulated in both conditions (Figure 3). Upregulation of cdcA, sdrA, and padA was observed on cinnamic acid, cinnamyl alcohol, sorbic acid, and benzoic acid (Table 3). The three genes (NRRL3_8293, NRRL3_8294, and NRRL3_8295) located next to cdcA and one gene (NRRL3_8299) located next to padA were induced by both cinnamic acid and sorbic acid (Table 3). On cinnamyl alcohol, cdcA, padA, NRRL3_8293, and NRRL3_8295 were also upregulated, but this was not the case for sdrA, NRRL3_8294, and NRRL3_8299. Two other genes, NRRL3_8300 and NRRL3_8301 were specifically induced by cinnamic acid. The lowest overlap in upregulated transcripts was found between salicylic acid and cinnamic acid/sorbic acid suggesting that the genes involved in the cinnamic acid/sorbic acid pathway are not involved in the conversion of salicylic acid. This was also supported by the fact that cdcA, sdrA and padA and their neighboring genes were not induced on salicylic acid (Table 3). The eukaryotic protein subcellular localization predictor software DeepLoc-1.0 was used to predict the cellular localization of the cinnamic acid and sorbic acid decarboxylation cluster genes (Table 3).
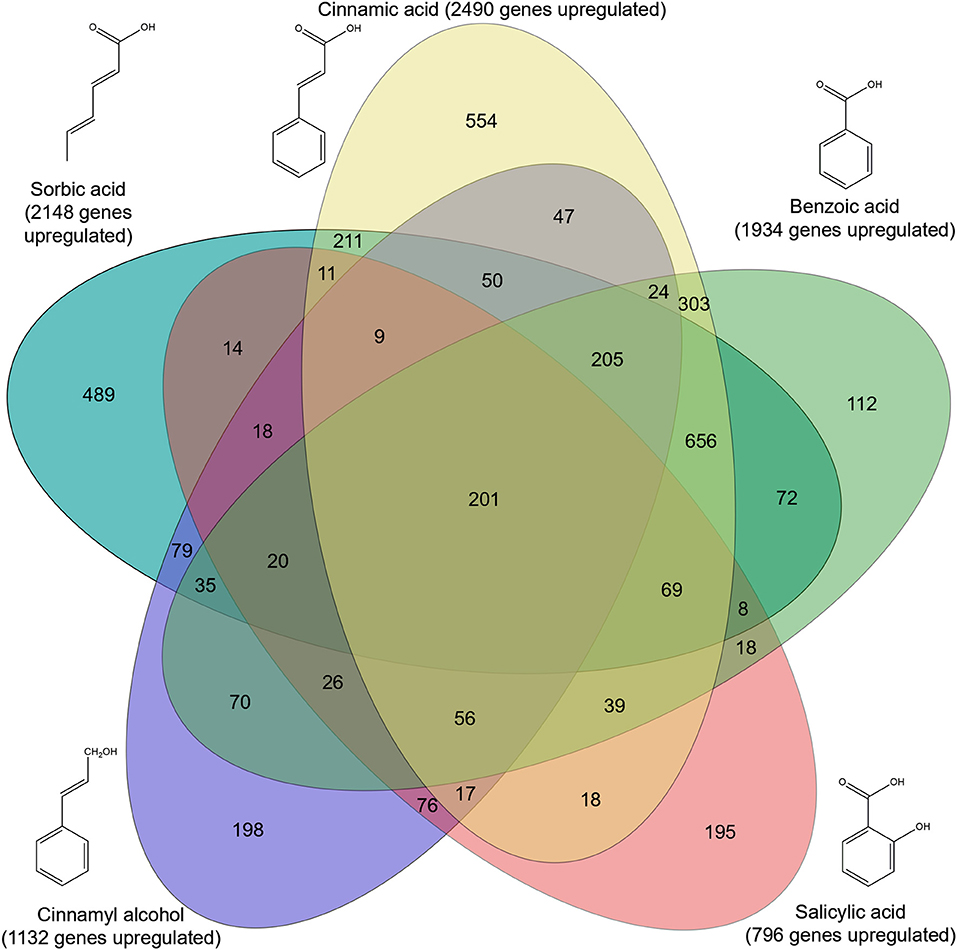
Figure 3. Venn diagram of upregulated genes in five conditions compared to a non-carbon source control. Genes were considered upregulated when the fold change ≥2, p-value ≤ 0.05 and FPKM ≥10. Significance was calculated using DESeq2 (Love et al., 2014).
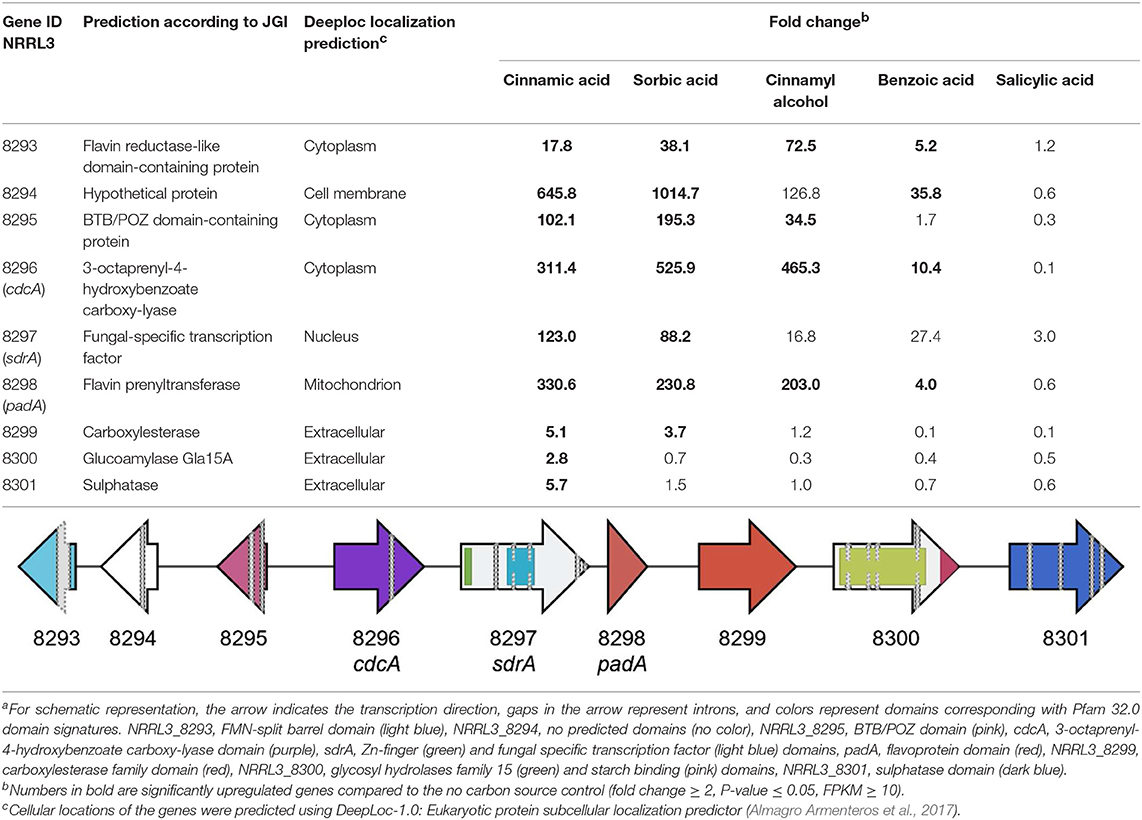
Table 3. Fold change and schematic presentationa of the cinnamic acid and sorbic acid decarboxylation gene cluster and neighboring genes.
Deletion of sdrA Reveals Tight Regulation of the Cinnamic Acid and Sorbic Acid Decarboxylation Cluster
To further study the regulatory targets of SdrA, genome-wide transcriptome profiles of ΔsdrA was performed. The expression of 420 genes was reduced in ΔsdrA compared to A. niger N593 on cinnamic acid and 362 genes were reduced on sorbic acid, with only 50 genes that were reduced in both conditions (Supplementary Table 4). Transcript levels of cdcA and padA were significantly reduced in ΔsdrA confirming that cdcA and padA are both regulated by SdrA (Table 4). Interestingly, the transcript levels of cdcA were less affected by the deletion of sdrA on cinnamic acid than those of padA, which could indicate involvement of an additional regulator. The transcript levels of the four clustered genes (NRRL3_8293, NRRL3_8294, NRRL3_8295, and NRRL3_8299) were also reduced in ΔsdrA and therefore likely regulated by SdrA (Table 4). Transcription levels of NRRL3_8300 and NRRL3_8301 were reduced in ΔsdrA on cinnamic acid compared to A. niger N593, but not on sorbic acid. Therefore, we speculate that these genes are not part of the cinnamic acid and sorbic acid decarboxylation cluster. Similar expression profiles were observed in cinnamyl alcohol with high fold changes, but on this compound the FPKM values of NRRL3_8294 and sdrA were below 10 (Table 4) which makes it difficult to draw conclusions.
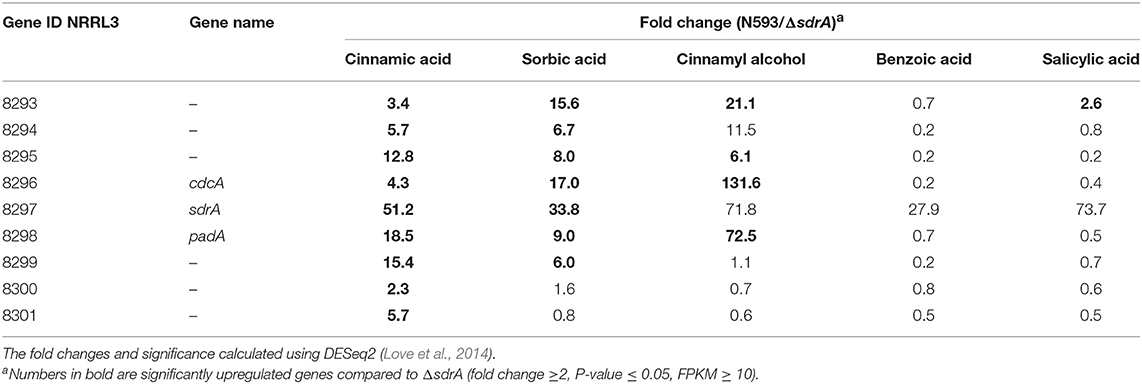
Table 4. Transcriptome data of the cinnamic acid and sorbic acid decarboxylation cluster genes of A. niger N593 compared to ΔsdrA in both cinnamic acid, sorbic acid, cinnamyl alcohol, benzoic acid, and sorbic acid.
The Cinnamic Acid and Sorbic Acid Decarboxylation Cluster Is Conserved in Aspergilli From the Nigri-Biseriates Clade
To study whether the cinnamic acid and sorbic acid decarboxylation cluster is conserved in Aspergilli, a synteny study using 30 Aspergillus genomes was performed (Supplementary Figure 1). In A. niger, two homologs of cdcA (NRRL3_3 and NRRL3_3023, 51.5 and 47.7% identity, respectively) and two homologs of padA (NRRL3_2 and NRRL3_3024, 74.6 and 55.8% identity, respectively) were observed and both of these are also clustered but in a different genomic location. However, they are not separated by a transcription factor. In addition, the expression of these genes was not induced by any of the tested compounds. BLAST analyses with the amino acid sequence of cdcA and padA revealed that most Aspergilli had on average two homologs of cdcA and padA in their genomes (Table 5). To identify the true orthologs of the cinnamic acid decarboxylation cluster we used the following criteria. First, either cdcA or padA is located next to a transcription factor. If no transcription factor is found next to one of the orthologs, the cdcA or padA ortholog with the highest E-value is used. From the 30 Aspergilli genomes, 27 genomes had a transcription factor next to the homolog of padA while the remaining three genomes did not have cdcA, sdrA, and padA orthologs in their genome (Table 5).
Most Aspergilli from the Nigri-Biserates clade had a conserved cinnamic/sorbic acid gene cluster, except A. ibericus, A. sclerotiicarbonarius (no ortholog of NRRL3_8293), and A. carbonarius and A. sclerotioniger (no ortholog of cdcA and NRRL3_8293) (Figure 3, Table 5). However, orthologs of NRRL3_8293 and cdcA that were located elsewhere in their genomes were found for these species. NRRL3_8294 is only present in Aspergilli that are closely related to A. niger (Supplementary Figure 1). Species from the Nigri-Uniseriates clade do not have cdcA homolog ortholog in their cluster or were missing cdcA and NRRL3_8300 orthologs in their cluster. The cinnamic/sorbic acid cluster of A. japonicus and A. sydowii only contained orthologs of NRRL3_8293, cdcA, sdrA, and padA, while orthologs of the remaining genes were scattered over different chromosomes. Similar observations were found for A. nidulans, however the padA homolog appears to be truncated (Figure 3). Two species from the section Flavi, A. flavus and A. oryzea, had orthologs of cdcA, sdrA, and padA, while NRRL3_8293 was separated by multiple genes from the core cluster (Figure 4). The orthologs of NRRL3_8299, NRRL3_8300, and NRRL3_8301 were clustered, but located on a different scaffold. It appeared that the sdrA and padA orthologs of A. terreus are fused and the zinc finger domain of sdrA is missing (Figure 4). A. fumigatus, A. clavatus, and A. campestris do not have an ortholog of cdcA, sdrA, and padA. In addition, only low e-value BLAST hits of sdrA were found in these Aspergilli.
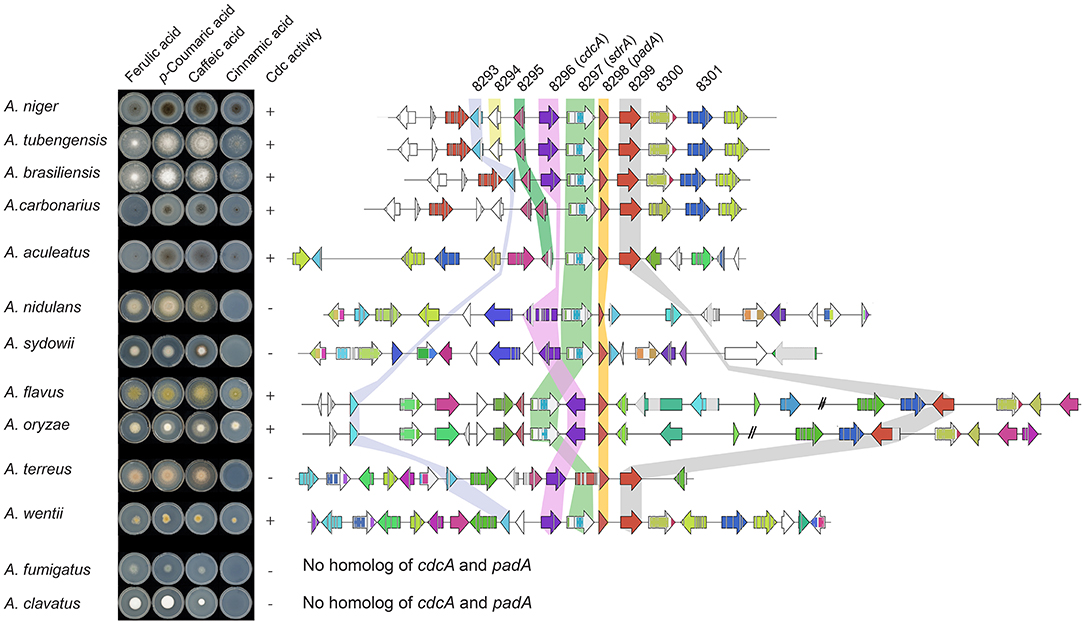
Figure 4. Synteny analysis and growth test of 13 Aspergilli. No orthologs of cdcA or padA were found in A. fumigatus and A. clavatus, therefore no cluster was added. Genes clustered on another scaffold are marked with a double dash.
Correlation Between the Ability to Grow on Cinnamic Acid and the Occurrence of an Intact Cinnamic Acid and Sorbic Acid Decarboxylation Gene Cluster
To confirm our findings and the correlation between the cinnamic acid and sorbic acid decarboxylation gene cluster and the ability to convert cinnamic acid, a growth test was performed with selected Aspergillus species based on the following criteria:
1. Species having an intact cinnamic acid and sorbic acid decarboxylation gene cluster containing cdcA, sdrA and padA homologs (A. brasiliensis, A. niger, and A. tubengensis).
2. Species containing a gene cluster without a cdcA homolog, but the homolog is present on a different scaffold (A. aculeatus and A. carbonarius).
3. Species containing no cdcA homolog (A. clavatus and A. fumigatus).
4. Species with miscellaneous gene clusters. A. oryzae and A. flavus were chosen since the location of cdcA and sdrA are swapped. A. terreus was selected because of the fused sdrA and padA genes. A. nidulans has a truncated padA. A. sydowii lacks an ortholog of NRRL3_8293 in its cluster. A. wentii lacks NRRL3_8295 and NRRL3_8301 orthologs in its cluster.
The Aspergilli of criteria one (A. niger A. brasiliensis, A. tubengensis, and A. wentii) and two (A. carbonarius and A. aculeatus) were all able to grow on cinnamic acid and the distinct smell of styrene was present. This indicates that A. carbonarius and A. aculeatus both have a functional cdcA despite its different genomic location. The Aspergilli of criteria three (A. fumigatus and A. clavatus) were not able to grow on cinnamic acid, which corresponds to the absence of cdcA, sdrA and padA homologs. Three Aspergilli of criteria four, A. flavus, A. oryzae, and A. wentii, were able to grow on cinnamic acid, while A. nidulans, A. sydowii, and A. terreus were not. In addition, all strains tested were able to grow on ferulic acid, p-coumaric acid and caffeic acid indicating that the cinnamic acid decarboxylation cluster in not required for ferulic acid, p-coumaric acid and caffeic acid utilization.
Discussion
This paper provides new insights into the regulation by the transcription factor SdrA of the cinnamic acid and sorbic acid decarboxylation cluster in Aspergilli and its regulatory targets, which also determines their ability to grow on cinnamic acid. Based on phenotypic screening of the deletion mutants of cdcA, sdrA, and padA, it is clear that the decarboxylation of cinnamic acid and sorbic acid represents a relevant step in the metabolic pathway for A. niger to utilize these compounds since the deletion of cdcA or padA resulted in abolished or reduced growth on cinnamic acid and sorbic acid (Figure 2). This also indicates that the suggested alternative cinnamic acid pathway toward p-coumaric acid is not sufficient to counteract the toxicity of cinnamic acid. Growth was not completely abolished on cinnamic acid when sdrA was deleted, which could be explained by the fact that cdcA and padA were still lowly expressed (Table 3). Deletion of either cdcA, sdrA, or padA did not affect the growth on ferulic and p-coumaric acid, which supports the outcome of a previous study showing that cdcA and padA were not induced by ferulic acid or p-coumaric acid (Stratford et al., 2012). This indicates that cdcA, sdrA, and padA are exclusively involved in cinnamic acid and sorbic acid metabolism.
Transcriptome analysis of A. niger revealed that sdrA was lowly expressed by cinnamic acid, cinnamyl alcohol and sorbic acid (Supplementary Table 4), which is in agreement with a previous study which showed this by qRT-PCR (Plumridge et al., 2010). However, its expression was significantly upregulated during growth on cinnamic acid and sorbic acid (Table 3), which indicates that low levels of SdrA are sufficient for the regulation of the cinnamic acid and sorbic acid decarboxylation cluster. Deletion of sdrA revealed a significant reduction of the expression of 50 genes in both cinnamic acid and sorbic acid. These 50 genes included cdcA and padA, revealing that SdrA regulates these two genes either directly or indirectly (Supplementary Table 4). It was reported earlier that the genes, flanking either side of the cluster, are not induced by sorbic acid (Plumridge et al., 2010). However, our transcriptome data revealed that SdrA tightly regulates six of these genes. It remains unknown which function the corresponding enzymes have in the cinnamic/sorbic acid decarboxylation pathway. NRRL3_8293 encode a flavin reductase and could be involved in the reduction of prenylated FMN. NRRL3_8295 contains a BTB/POZ domain which are known to be involved in transcriptional regulation (Zollman et al., 1994; Daniel and Reynolds, 1999; Li et al., 1999). NRRL3_8299 encode a carboxylesterase which could be involved in the hydrolysis of cinnamyl esters. Several genes of the cinnamic acid and sorbic acid decarboxylation cluster were also affected by cinnamyl alcohol with the exception of sdrA, NRRL3_8294, and NRRL3_8299. Both sdrA and NRRL3_8294 have a high fold change and were significantly different but the FPKM value were below 10 and therefore we cannot state with certainty that these genes are affected (Tables 3, 4). The deletion of sdrA resulted in reduced expression of cdcA, padA, NRRL3_8293, and NRRL3_8295 on cinnamyl alcohol, indicating that cinnamyl alcohol is part of the cinnamic acid metabolic pathway. The remaining 43 down-regulated genes are currently not connected to the cinnamic acid metabolic pathway. However, the expression of three putative transporters was reduced, which could be important for cinnamic acid or styrene transport (Supplementary Table 4).
Deletion of sdrA did not result in abolished growth on cinnamic acid or sorbic acid. In addition, cdcA and padA were still expressed in ΔsdrA indicating that a second transcription factor is likely involved. One candidate for regulation of cdcA and padA is NRRL3_8295, present in the cluster, but also three putative fungal-specific transcription factors (NRRL3_7314, NRRL3_9846, and NRRL3_7351) were upregulated by cinnamic acid, sorbic acid and cinnamyl alcohol in N593 and were not affected by the deletion of sdrA (data not shown). Fungal specific transcription factors containing Zinc fingers are known to bind directly to regulatory motifs in promoters. Such regulatory motifs have been suggested in the promoters of cdcA and padA (Plumridge et al., 2010), but these did not occur in the neighboring genes.
Synteny analysis of the cinnamic acid and sorbic acid decarboxylation cluster in 30 Aspergillus genomes revealed that the cluster is highly conserved in Aspergilli of the Nigri-Biseriates clade. Other Aspergilli, such as A. fumigatus and A. clavatus that lack orthologs of cdcA and padA were unable to grow on cinnamic acid (Figure 4, Table 5). It has been reported that A. nidulans is unable to decarboxylate cinnamic acid, which could be caused by the truncated PadA protein (Plumridge et al., 2010). In addition, analysis of RNAseq data of A. nidulans revealed that the predicted intron and exon boundaries of A. nidulans cdcA were incorrect (Supplementary Figure 1). Ahen comparing the corrected gene model to A. niger cdcA, two internal deletions, one insertion and one substitution were identified in the sequence of A. nidulans cdcA. The second deletion results in a premature stop codon. This could be an alternative explanation why A. nidulans is unable to grow on cinnamic acid. A. flavus, A. oryzae, and A. wentii were able to grow on cinnamic acid and all had orthologs of cdcA, sdrA, padA, and NRRL3_8293 in their genome. In agreement with this, two A. oryzae strains were able to grow on cinnamic acid in a previous study (Plumridge et al., 2010).
Transcriptome analysis also revealed that an enzyme converting benzoic acid to p-hydroxybenzoic acid (Figure 1), benzoate 4-monooxygenase A (BphA, Van Gorcom et al., 1990), is highly upregulated (170.6 fold) by benzoic acid compared to no carbon source control. In addition, bphA is 24.7- and 59.6-fold upregulated by cinnamic acid and cinnamyl alcohol, respectively, while it was not induced by sorbic acid and salicylic acid, confirming that cinnamic acid and cinnamyl alcohol are converted toward benzoic acid. Our results suggest that SdrA is specifically involved in cinnamic acid conversion and not in the downstream regulation of this pathway since no phenotypic effect was observed on styrene, benzoic acid or p-hydroxybenzoic acid (Figure 2). In addition, there is strong induction of bphA by benzoic acid, cinnamic acid and cinnamyl alcohol in both A. niger N593 and ΔsdrA, supporting the finding that SdrA is not involved in the expression of genes of the downstream pathway. This induction of bphA by cinnamic acid and benzoic acid corresponds with a previous study (de Vries et al., 2002). It is clear that the decarboxylation of cinnamic acid to styrene is the main pathway in A. niger. Styrene, styrene glycol and two unidentified compounds have been detected from A. niger grown on cinnamic acid (Clifford et al., 1969). In the basidiomycete Pleurotus ostreatus, styrene is converted to phenyl-1,2-ethanediol (styrene glycol) and benzoic acid and it has been suggested that styrene oxide and mandelic acid are intermediates in this metabolic pathway (Braun-Lüllemann et al., 1997). Another pathway has been observed in the ascomycete Phomopsis liquidambari, where cinnamic acid is decarboxylated to styrene after which it is oxidized to benzaldehyde followed by the oxidation to benzoic acid (Xie and Dai, 2015).
Recently, an extensive substrate analysis of CdcA from A. niger showed that the main substrates for CdcA are cinnamic acid and sorbic acid since ≥99% was decarboxylated, while ≤70% of the ferulic acid or p-coumaric acid was decarboxylated (Aleku et al., 2018). No phenotypic difference on ferulic acid, p-coumaric acid and caffeic acid were observed (Figure 1). In addition, Aspergilli that were unable to grow on cinnamic acid could grow on ferulic acid, p-coumaric acid and caffeic acid. This indicates that the biological function of CdcA is not the decarboxylation of ferulic acid since no induction or phenotype was observed by ferulic acid (Figure 4). We propose, based on the substrate preference, the induction pattern and the phenotypic and transcriptomic analysis, to change the name of ferulic acid decarboxylase (FdcA) to cinnamic acid decarboxylase (CdcA) which fits with its biological function. In addition, CdcA also corresponds better with its enzymatic function since ferulic acid (3-methoxy-4-hydroxycinnamic acid) and p-coumaric acid (4-hydroxycinnamic acid) both have a cinnamic acid-based chemical structure. In Aspergillus luchuensis, which is closely related to A. niger, a phenolic acid decarboxylase (AlPad) has been characterized which is able to decarboxylate ferulic acid, p-coumaric acid and caffeic acid (Maeda et al., 2018). In A. niger, a 99% identical ortholog of this gene is present and therefore it is more likely that this enzyme is the ferulic acid decarboxylase.
Conclusion
In summary, we identified new regulatory targets of SdrA through the whole genome transcriptome analysis. These genes are clustered in the A. niger genome and highly conserved in Aspergilli closely related to A. niger. In addition, phenotypic analysis revealed that CdcA (formerly FdcA) and PadA are required exclusively for the decarboxylation of cinnamic acid and sorbic acid, but not for the decarboxylation of other aromatic compounds. Our results provide a better understanding of the aromatic metabolic pathways and their regulation in filamentous fungi. This can unlock strategies to design new fungal cell factories, e.g., to obtain higher yields of cinnamic acid by blocking the degradation pathway, or to increase the synthesis of styrene by overexpressing cdcA.
Data Availability Statement
The datasets generated for this study can be found in the Gene Expression Omnibus (GEO) under accession numbers SRR8275478, SRR8275427, SRR8275426, SRR8275534, SRR8275532, SRR8275533, SRR8275539, SRR8275424, SRR8275535, SRR8275425, SRR8275431, SRR8275436, SRR8275434, SRR8275437, SRR8275435, SRR8275432, SRR8275406, SRR8275433, SRR8275414, SRR8275470, SRR8275471, SRR8275472, SRR8275446, SRR8275447, SRR8275468, SRR8275469, SRR8275467, SRR8275449, SRR8275448, SRR8275450, SRR8275439, SRR8275523, SRR8275522, SRR8275404, SRR8275498, and SRR8275413.
Author Contributions
RL conducted the experiments, analyzed the data, and wrote the manuscript. JN contributed to the synteny analysis and visualization. MP processed and analyzed the RNA sequencing data. MW, AL, VN, and IG performed the RNA sequencing. AD, JV, and KH contributed to data interpretation and commented on the manuscript. RV conceived and supervised the overall project. All authors commented on the manuscript.
Funding
This project was supported through FALCON by the European Union's Horizon 2020 research and innovation programme under grant agreement No. 720918. The work conducted by the U.S. Department of Energy Joint Genome Institute, a DOE Office of Science User Facility, was supported by the Office of Science of the U.S. Department of Energy under Contract No. DE-AC02-05CH11231.
Conflict of Interest
The authors declare that the research was conducted in the absence of any commercial or financial relationships that could be construed as a potential conflict of interest.
Acknowledgments
We want to thank DOE Joint Genome Institute for their RNAseq service and David B. Archer, Jos Houbraken and Willem J. H. van Berkel for helpful discussion.
Supplementary Material
The Supplementary Material for this article can be found online at: https://www.frontiersin.org/articles/10.3389/fbioe.2019.00249/full#supplementary-material
References
Aguilar-Pontes, M. V., Brandl, J., McDonnell, E., Strasser, K., Nguyen, T. T. M., Riley, R., et al. (2018). The gold-standard genome of Aspergillus niger NRRL 3 enables a detailed view of the diversity of sugar catabolism in fungi. Stud. Mycol. 91, 61–78. doi: 10.1016/j.simyco.2018.10.001
Aleku, G. A., Prause, C., Bradshaw-allen, R. T., and Plasch, K. (2018). Terminal alkenes from acrylic acid derivatives via non-oxidative enzymatic decarboxylation by ferulic acid decarboxylases. ChemCatChem 10, 4043–4052. doi: 10.1002/cctc.201800643
Almagro Armenteros, J. J., Sønderby, C. K., Sønderby, S. K., Nielsen, H., and Winther, O. (2017). DeepLoc: prediction of protein subcellular localization using deep learning. Bioinformatics 33, 3387–3395. doi: 10.1093/bioinformatics/btx431
Arnaud, M. B., Cerqueira, G. C., Inglis, D. O., Skrzypek, M. S., Binkley, J., Chibucos, M. C., et al. (2012). The Aspergillus Genome Database (AspGD): recent developments in comprehensive multispecies curation, comparative genomics and community resources. Nucleic Acids Res. 40, 653–659. doi: 10.1093/nar/gkr875
Averesch, N. J. H., and Krömer, J. O. (2018). Metabolic engineering of the shikimate pathway for production of aromatics and derived compounds—present and future strain construction strategies. Front. Bioeng. Biotechnol. 6:32. doi: 10.3389/fbioe.2018.00032
Benocci, T., Aguilar-Pontes, M. V., Zhou, M., Seiboth, B., and De Vries, R. P. (2017). Regulators of plant biomass degradation in ascomycetous fungi. Biotechnol. Biofuels 10, 1–25. doi: 10.1186/s13068-017-0841-x
Bocks, S. M. (1966). Fungal metabolism I the transformation of coumarin, o-coumaric acid and trans-cinnamic acid by Aspergillus niger. Phytochemistry 398, 127–130. doi: 10.1016/0031-9422(67)85017-9
Bos, C. J., Debets, A. J. M., Swart, K., Huybers, A., Kobus, G., and Slakhorst, S. M. (1988). Genetic analysis and the construction of master strains for assignment of genes to six linkage groups in Aspergillus niger. Curr. Genet. 14, 437–443. doi: 10.1007/BF00521266
Braun-Lüllemann, A., Majcherczyk, A., and Hüttermann, A. (1997). Degradation of styrene by white-rot fungi. Appl. Microbiol. Biotechnol. 47, 150–155. doi: 10.1007/s002530050904
Chemler, J. A., and Koffas, M. A. (2008). Metabolic engineering for plant natural product biosynthesis in microbes. Curr. Opin. Biotechnol. 19, 597–605. doi: 10.1016/j.copbio.2008.10.011
Clifford, D. R., Faulkner, J. K., Walker, J. R. L., and Woodcock, D. (1969). Metabolism of cinnamic acid by Aspergillus niger. Phytochemistry 8, 549–552. doi: 10.1016/S0031-9422(00)85398-4
Daniel, J. M., and Reynolds, A. B. (1999). The catenin p120 ctn interacts with Kaiso, a novel BTB/POZ domain zinc finger transcription factor. Mol. Cell. Biol. 19, 3614–3623. doi: 10.1128/MCB.19.5.3614
de Vries, R. P., Burgers, K., Samson, R. A., and Visser, J. (2004). A new black Aspergillus species, A. vadensis, is a promising host for homologous and heterologous protein production. Appl. Environ. Microbiol. 70, 3954–3959. doi: 10.1128/AEM.70.7.3954-3959.2004
de Vries, R. P., Riley, R., Wiebenga, A., Aguilar-Osorio, G., Amillis, S., Uchima, C. A., et al. (2017). Comparative genomics reveals high biological diversity and specific adaptations in the industrially and medically important fungal genus Aspergillus. Genome Biol. 18:28. doi: 10.1186/s13059-017-1151-0
de Vries, R. P., Van Kuyk, P. A., Kester, H. C. M., and Visser, J. (2002). The Aspergillus niger faeB gene encodes a second feruloyl esterase involved in pectin and xylan degradation and is specifically induced in the presence of aromatic compounds. Biochem. J. 363, 377–386. doi: 10.1042/bj3630377
Fedorova, N. D., Khaldi, N., Joardar, V. S., Maiti, R., Amedeo, P., Anderson, M. J., et al. (2008). Genomic islands in the pathogenic filamentous fungus Aspergillus fumigatus. PLoS Genet. 4:e1000046. doi: 10.1371/journal.pgen.1000046
Galagan, J. E., Calvo, S. E., Cuomo, C., Ma, L. J., Wortman, J. R., Batzoglou, S., et al. (2005). Sequencing of Aspergillus nidulans and comparative analysis with A. fumigatus and A. oryzae. Nature 438, 1105–1115. doi: 10.1038/nature04341
Grigoriev, I. V., Nikitin, R., Haridas, S., Kuo, A., Ohm, R., Otillar, R., et al. (2014). MycoCosm portal: gearing up for 1000 fungal genomes. Nucleic Acids Res. 42, 699–704. doi: 10.1093/nar/gkt1183
Gruenwald, J., Freder, J., and Armbruester, N. (2010). Cinnamon and health. Crit. Rev. Food Sci. Nutr. 50, 822–834. doi: 10.1080/10408390902773052
He, Z. D., Qiao, C. F., Han, Q. B., Cheng, C. L., Xu, H. X., Jiang, R. W., et al. (2005). Authentication and quantitative analysis on the chemical profile of Cassia Bark (Cortex Cinnamomi) by high-pressure liquid chromatography. J. Agric. Food Chem. 53, 2424–2428. doi: 10.1021/jf048116s
Hoskins, J. A. (1984). The occurrence, metabolism and toxicity of cinnamic acid and related compounds. J. Appl. Toxicol. 4, 283–292. doi: 10.1002/jat.2550040602
Kowalczyk, J. E., Lubbers, R. J. M., Peng, M., Battaglia, E., Visser, J., and De Vries, R. P. (2017). Combinatorial control of gene expression in Aspergillus niger grown on sugar beet pectin. Sci. Rep. 7, 1–12. doi: 10.1038/s41598-017-12362-y
Lafeuille, J. L., Buniak, M. L., Vioujas, M. C., and Lefevre, S. (2009). Natural formation of styrene by cinnamon mold flora. J. Food Sci. 74:M276–M283. doi: 10.1111/j.1750-3841.2009.01206.x
Latif, S., Chiapusio, G., and Weston, L. A. (2017). Allelopathy and the Role of Allelochemicals in Plant Defence. Oxford: Elsevier Ltd.
Li, X., Peng, H., Schultz, D. C., Lopez-Guisa, J. M., Rauscher, F. J., and Marmorstein, R. (1999). Structure-function studies of the BTB/POZ transcriptional repression domain from the promyelocytic leukemia zinc finger oncoprotein. Cancer Res. 59, 5275–5282. doi: 10.2210/pdb1cs3/pdb
Liewen, M. B., and Marth, E. H. (2016). Growth and inhibition of microorganisms in the presence of sorbic acid: a review. J. Food Prot. 48, 364–375. doi: 10.4315/0362-028X-48.4.364
Love, M. I., Huber, W., and Anders, S. (2014). Moderated estimation of fold change and dispersion for RNA-seq data with DESeq2. Genome Biol. 15, 1–21. doi: 10.1186/s13059-014-0550-8
Lubbers, R. J. M., Dilokpimol, A., Visser, J., Mäkelä, M. R., Hildén, K. S., and de Vries, R. P. (2019). A comparison between the homocyclic aromatic metabolic pathways from plant-derived compounds by bacteria and fungi. Biotechnol. Adv. doi: 10.1016/j.biotechadv.2019.05.002
Machida, M., Asai, K., Sano, M., Tanaka, T., Kumagai, T., Terai, G., et al. (2005). Genome sequencing and analysis of Aspergillus oryzae. Nature 438, 1157–1161. doi: 10.1038/nature04300
Maeda, M., Tokashiki, M., Tokashiki, M., Uechi, K., Ito, S., and Taira, T. (2018). Characterization and induction of phenolic acid decarboxylase from Aspergillus luchuensis. J. Biosci. Bioeng. 126, 162–168. doi: 10.1016/j.jbiosc.2018.02.009
Marth, E. H., Capp, C. M., Hasenzahl, L., Jackson, H. W., and Hussong, R. V. (1966). Degradation of potassium sorbate by Penicillium species. J. Dairy Sci. 49, 1197–1205. doi: 10.3168/jds.S0022-0302(66)88053-0
Martins, T. M., Hartmann, D. O., Planchon, S., Martins, I., Renaut, J., and Silva Pereira, C. (2015). The old 3-oxoadipate pathway revisited: new insights in the catabolism of aromatics in the saprophytic fungus Aspergillus nidulans. Fungal Genet. Biol. 74, 32–44. doi: 10.1016/j.fgb.2014.11.002
Meyer, V., Arentshorst, M., El-Ghezal, A., Drews, A. C., Kooistra, R., van den Hondel, C. A. M. J. J., et al. (2007). Highly efficient gene targeting in the Aspergillus niger kusA mutant. J. Biotechnol. 128, 770–775. doi: 10.1016/j.jbiotec.2006.12.021
Milstein, O., Vered, Y., Shragina, L., Gressel, J., Flowers, H. M., and Hüttermann, A. (1983). Metabolism of lignin related aromatic compounds by Aspergillus japonicus. Arch. Microbiol. 135, 147–154. doi: 10.1007/BF00408025
Mukai, N., Masaki, K., Fujii, T., Kawamukai, M., and Iefuji, H. (2010). PAD1 and FDC1 are essential for the decarboxylation of phenylacrylic acids in Saccharomyces cerevisiae. J. Biosci. Bioeng. 109, 564–569. doi: 10.1016/j.jbiosc.2009.11.011
Nierman, W. C., Pain, A., Anderson, M. J., Wortman, J. R., Kim, H. S., Arroyo, J., et al. (2005). Genomic sequence of the pathogenic and allergenic filamentous fungus Aspergillus fumigatus. Nature 438, 1151–1156. doi: 10.1038/nature04332
Payne, G. A., Nierman, W. C., Wortman, J. R., Pritchard, B. L., Brown, D., Dean, R. A., et al. (2006). Whole genome comparison of Aspergillus flavus and A. oryzae. Med. Mycol. 44, 9–11. doi: 10.1080/13693780600835716
Payne, K. A. P., White, M. D., Fisher, K., Khara, B., Bailey, S. S., Parker, D., et al. (2015). New cofactor supports α,β-unsaturated acid decarboxylation via 1,3-dipolar cycloaddition. Nature 522, 497–501. doi: 10.1038/nature14560
Pinches, S. E., and Apps, P. (2007). Production in food of 1,3-pentadiene and styrene by Trichoderma species. Int. J. Food Microbiol. 116, 182–185. doi: 10.1016/j.ijfoodmicro.2006.12.001
Plumridge, A., Melin, P., Stratford, M., Novodvorska, M., Shunburne, L., Dyer, P. S., et al. (2010). The decarboxylation of the weak-acid preservative, sorbic acid, is encoded by linked genes in Aspergillus spp. Fungal Genet. Biol. 47, 683–692. doi: 10.1016/j.fgb.2010.04.011
Punt, P. J., Oliver, R. P., Dingemanse, M. A., Pouwels, P. H., and van den Hondel, C. A. (1987). Transformation of Aspergillus based on the hygromycin B resistance marker from Escherichia coli. Gene 56, 117–124. doi: 10.1016/0378-1119(87)90164-8
Richard, P., Viljanen, K., and Penttilä, M. (2015). Overexpression of PAD1 and FDC1 results in significant cinnamic acid decarboxylase activity in Saccharomyces cerevisiae. AMB Express 5, 1–5. doi: 10.1186/s13568-015-0103-x
Stratford, M., Plumridge, A., Pleasants, M. W., Novodvorska, M., Baker-Glenn, C. A. G., Pattenden, G., et al. (2012). Mapping the structural requirements of inducers and substrates for decarboxylation of weak acid preservatives by the food spoilage mould Aspergillus niger. Int. J. Food Microbiol. 157, 375–383. doi: 10.1016/j.ijfoodmicro.2012.06.007
Thompson, B., Machas, M., and Nielsen, D. R. (2015). Creating pathways towards aromatic building blocks and fine chemicals. Curr. Opin. Biotechnol. 36, 1–7. doi: 10.1016/j.copbio.2015.07.004
Van Gorcom, R. F. M., Boschloo, J. G., Kuijvenhoven, A., Lange, J., Vanvark, A. J., Bos, C. J., et al. (1990). Isolation and molecular characterization of the benzoate-para-hydroxylase gene (Bpha) of Aspergillus-niger- a member of a new gene family of the cytochrome-P450 superfamily. Mol. Gen. Genet. 223, 192–197. doi: 10.1007/BF00265053
Vargas-Tah, A., and Gosset, G. (2015). Production of cinnamic and p-hydroxycinnamic acids in engineered microbes. Front. Bioeng. Biotechnol. 3, 1–10. doi: 10.3389/fbioe.2015.00116
Xie, X. G., and Dai, C. C. (2015). Biodegradation of a model allelochemical cinnamic acid by a novel endophytic fungus Phomopsis liquidambari. Int. Biodeterior. Biodegrad. 104, 498–507. doi: 10.1016/j.ibiod.2015.08.004
Yamada, S., Nabe, K., and Izuo, N. (1981). Production of L-phenylalanine from trans-cinnamic acid with Rhodotorula glutinis containing L-phenylalanine ammonia-lyase activity. Appl. Environ. Microbiol. 42, 773–778.
Zollman, S., Godt, D., Privé, G. G., Couderc, J. L., and Laski, F. A. (1994). The BTB domain, found primarily in zinc finger proteins, defines an evolutionarily conserved family that includes several developmentally regulated genes in Drosophila. Proc. Natl. Acad. Sci. U.S.A. 91, 10717–10721. doi: 10.1073/pnas.91.22.10717
Keywords: fungal aromatic metabolism, Aspergilli, synteny analysis, transcription factor, flavoprotein, cinnamic acid decarboxylase
Citation: Lubbers RJM, Dilokpimol A, Navarro J, Peng M, Wang M, Lipzen A, Ng V, Grigoriev IV, Visser J, Hildén KS and de Vries RP (2019) Cinnamic Acid and Sorbic acid Conversion Are Mediated by the Same Transcriptional Regulator in Aspergillus niger. Front. Bioeng. Biotechnol. 7:249. doi: 10.3389/fbioe.2019.00249
Received: 13 May 2019; Accepted: 16 September 2019;
Published: 27 September 2019.
Edited by:
Nils Jonathan Helmuth Averesch, Stanford University, United StatesReviewed by:
Dirk Tischler, Ruhr University Bochum, GermanyMiguel Cacho Teixeira, University of Lisbon, Portugal
Copyright © 2019 Lubbers, Dilokpimol, Navarro, Peng, Wang, Lipzen, Ng, Grigoriev, Visser, Hildén and de Vries. This is an open-access article distributed under the terms of the Creative Commons Attribution License (CC BY). The use, distribution or reproduction in other forums is permitted, provided the original author(s) and the copyright owner(s) are credited and that the original publication in this journal is cited, in accordance with accepted academic practice. No use, distribution or reproduction is permitted which does not comply with these terms.
*Correspondence: Ronald P. de Vries, ci5kZXZyaWVzQHdpLmtuYXcubmw=