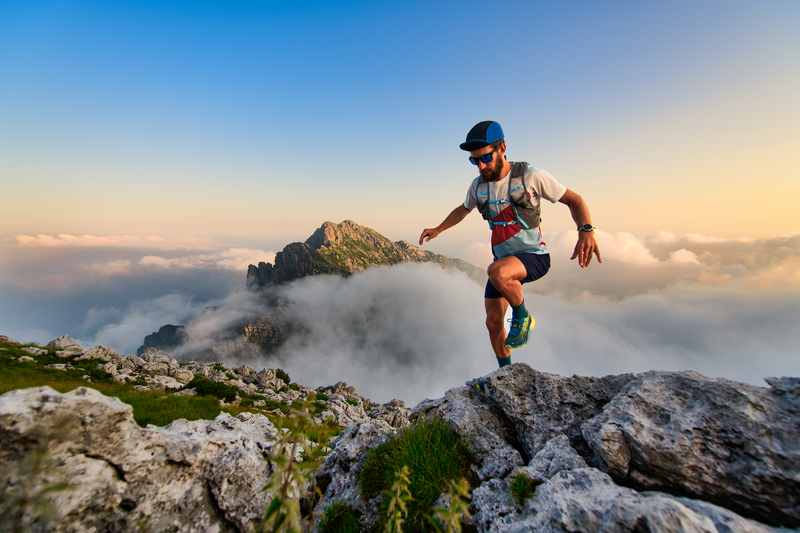
94% of researchers rate our articles as excellent or good
Learn more about the work of our research integrity team to safeguard the quality of each article we publish.
Find out more
REVIEW article
Front. Bioeng. Biotechnol. , 10 July 2018
Sec. Tissue Engineering and Regenerative Medicine
Volume 6 - 2018 | https://doi.org/10.3389/fbioe.2018.00090
Intervertebral disc (IVD) is an avascular tissue which contributes to the weight bearing, motion, and flexibility of spine. However, IVD is susceptible to damage and even failure due to injury, pathology, and aging. Annulus fibrosus (AF), the structural and functional integrity of which is critically essential to confine nucleus pulpous (NP) and maintain physiological intradiscal pressure under mechanical loading, plays a critical role in the biomechanical properties of IVD. AF degeneration commonly results in substantial deterioration of IVD. During this process, the biomechanical properties of AF and the balance between anabolism and catabolism in IVD are progressively disrupted, leading to chronic back pain, and even disability of individuals. Therefore, repairing and regenerating AF are effective treatments to degeneration-associated pains. However, they remain highly challenging due to the complexity of natural AF tissue in the aspects of cell phenotype, biochemical composition, microstructure, and mechanical properties. Tissue engineering (TE), by combining biological science and materials engineering, shed lights on AF regeneration. In this article, we review recent advances in the pro-anabolic approaches in the form of cell delivery, bioactive factors delivery, gene therapy, and TE strategies for achieving AF regeneration.
Low back pain (LBP) is one of the most common causes of activity limitations, neurological deficit, and disability in affected individuals. In the United States, more than 52 million people suffer from back pain and the clinical cost reaches more than $40 billion each year (Murray et al., 2012). Intervertebral disc (IVD) disorders contribute to LBP and neck pain in multiple ways with few available treatments. Current conservative treatments to LBP include nonpharmacological and pharmacological approaches, and surgical approaches may be considered if conservative methods fail (Finch, 2006). However, surgical interventions such as spinal fusion are very invasive and often require a long postoperative recovery, with a non-negligible risk of complications high post-surgery recurrence rate (Andersson, 1999). Therefore, new approaches are urgently needed for the treatment of degeneration disc disease (DDD).
Anatomically, the IVD is the fibrocartilaginous part of a three-component construct that consists of two posterior articulations of diarthrodial facet joints, along with a gelatinous proteoglycan-rich nucleus pulposus (NP) in the center and a multi-lamellar collagen-rich fibrocartilage annulus fibrosus (AF) in the surrounding, both of which are connected by the cartilaginous endplates (CE) to the vertebral bodies (Figure 1A). Together, these constructs contribute to the weight bearing, motion, and flexibility of spine while protecting its neural anatomy. Changes in the biomechanical and structural properties of IVD negatively impact motion, spinal alignment, flexibility, or neural anatomy, which can ultimately result in loss of normal function of IVD and further serious degenerative diseases like DDD. In the past decades, diverse strategies have been developed aiming to ameliorate IVD degeneration and promote its regeneration (Buckwalter, 1995; Adams and Dolan, 2012). While considerable progress has been achieved in treatment and regeneration of NP, much less is achieved in that of AF. As a crucial supporting component in the biomechanical constitution of IVD, the structural and mechanical integrity of AF is highly essential in confining NP, and tears or fissures in AF are closely associated with the onset and development of DDD (Nerurkar et al., 2009; Li et al., 2017). Therefore, strategies to repair or regenerate of AF are now considered necessary in DDD treatments.
Figure 1. (A) Schematic illustration of normal and degenerative IVDs. (B) Schematic illustration of the anatomic structure of an IVD. (C) 3D representation of an ovine AF using optical coherence tomography. Individual images on the right side show how collagen orientation changes along radial direction. Scale bar, 500 μm (Reprinted with permission from Han et al., 2015). (D) Forces and motion contribute to axial loading of IVD that is balanced by the hydrostatic pressure generated in the gelatinous NP. Pressure in NP causes tensile stress in the surrounding AF or “hoop stress” (Reprinted with permission from Bowles and Setton, 2017).
Recent progress in understanding of AF physiopathology from aspects of cell biology, biomaterials, and mechanics, is leading to new approaches towards AF repair and regeneration (Driscoll et al., 2013). Unlike NP, AF is a typical inhomogeneous tissue, with its cellular and biochemical characteristics varying peripherally from inner to outer zones along its radial direction, which, in turn, lead to region-specific biological and biomechanical functions (Bruehlmann et al., 2002; Cortes et al., 2013). Therefore, successful AF repair and regeneration require the recovery of biomechanical and structural properties of healthy AF and restoration of biological behaviors of resident cells within AF. In view of the cells apoptosis during AF degeneration, cell-based therapies were studied with growing interest. Recently, gene mutations or microRNA dysregulation was also regarded as a therapeutic approach for AF-associated diseases based on the delivery of genes or small interfering RNA (siRNA) to stimulate the restoration of AF functions (Clouet et al., 2018). Moreover, owing to the tremendous complexity of AF tissue, the use of biological factors targeting AF degeneration was also contemplated. Tissue engineering (TE) approaches are now emerging as promising therapy toward DDD using engineered disc and AF replacements. An ideal engineered AF tissue should recapitulate the biochemical, microstructural, and cellular complexity of native AF tissue (Chan et al., 2011). Although few reviews have been published on delivery systems for the treatment of AF defects, no systematic studies have described support structures with mechanical properties and structural features matching those of the AF (Sharifi et al., 2015; Li et al., 2016). In this review, we will first introduce the recent understanding in the pathophysiological mechanisms of AF and then focus on describing biomolecule-, gene-, cell-, and TE-based strategies for regeneration of AF.
AF holds the NP by confining its swelling pressure and resisting shear and tensile stresses from the internal pressure exerted by the NP. In contrast to the relatively simple structure of NP, AF is a highly heterogeneous tissue in terms of cellular phenotype, biomechanics, biochemistry, and microstructure, with typical gradient characteristics along the radial direction. Microscopically, AF consists of 15–25 concentric layers, which are composed of alternatingly aligned oblique collagen fibers interspersed with proteoglycans (Figures 1B,C) (Bhattacharjee et al., 2012; Li et al., 2014). The lamellas in AF intercross with each other and possess prominent mechanical nonlinearity and anisotropy, contributing to the radially gradient mechanical properties of AF. The outer layers of AF are more oriented between layers than the inner ones. Moreover, the outer zone of AF is more fibrous consisting of mainly collagen-I, while the inner zone is more cartilaginous, containing mostly collagen-II, and aggrecan (Mizuno et al., 2004; Martin et al., 2014). Accordingly, the content of collagen-I increases from the inner zone to the outer zone of AF, and the opposite trend is found in the content of collagen-II and aggrecan. The collagen fibers of AF are tensioned by intradiscal pressure through two mechanisms: direct radial pressure from NP, and cranial-caudal stretch from the separation of the two endplates (Figure 1D). Besides the microstructural heterogeneity, the mechanical properties of AF also gradually change from inner to outer layers along radial direction, leading to regional specific mechanical properties and biological functions (Bowles et al., 2011; Wismer et al., 2014). In general, the elastic modulus of AF lamellae changes from 59 MPa to 136 MPa from the inner region to outer region of AF. This unique composition and architecture of AF is crucial for IVD to sustain anisotropic, nonlinear, and viscoelastic mechanical loading and maintain homeostasis. Therefore, the repair and regeneration of AF must take into consideration the structural and mechanical characteristics of this tissue over a wide range of length scales.
AF degeneration involves complex and multifactorial physiopathology. Genetics, mechanical factors, environmental changes, aging, and toxic factors are considered as most relevant risk factors (Collin et al., 2017). Recent studies have highlighted that the changes in biomechanics and inflammation may play pivotal roles during AF degeneration (Adams et al., 2000; Urban et al., 2004).
Changes in biomechanics can lead to significant alteration of cellular physiology. Several groups showed that distinct compression on a spinal motion segment, either in vivo or ex vivo, could cause catabolic, anabolic and inflammatory cell responses in AF and IVD (Wang et al., 2007; Adams et al., 2009). As a result, cell behaviors in response to mechanical environmental variation are considered a pivotal factor determining the normal function and dysfunction of AF and IVD (Haglund et al., 2011). AF cells significantly respond to changes of hydrostatic pressure within AF. It was reported that AF cells could produce roughly 20% more proteoglycan under higher hydrostatic pressure (Le Maitre et al., 2008). On the other hand, the production of collagen I and aggrecan was found to reduce, while that of Tissue Inhibitor of Metalloproteinase-1 (TIMP-1) increased, which could affect remodeling of extracellular matrix (ECM) (Smith et al., 2004). This pressure sensing behavior appeared to be impaired in cells from degenerated AF as they responded less anabolically to physiologic intradiscal pressure (Wang et al., 2007; Wuertz et al., 2007). Shift from hydrostatic pressure to shear stress in the degenerated IVD was also reported to have distinct mechanobiological effects on AF cells. Similar to other load-bearing tissues like cartilage and bone, the increase in shear stress will stimulate the formation of fibrous tissues rich in collagen-I (Hsieh and Twomey, 2010). In addition, increased shear stress can also lead to the increasing of nitric oxide which could reduce the production of proteoglycan and increase apoptosis in the cells within IVD (Liu et al., 2001). Thus, the increased shear stresses in AF may accelerate IVD degeneration.
Biomechanical changes also induce inflammatory responses within AF and IVD (Purmessur et al., 2013). Indeed, in the altered biomechanical load model of degenerated IVD, significant increase was observed in the expression of inflammatory cytokines such as IL-1β, IL-6, TNF-α, and IL-8. These cytokines expressed by NP and AF cells remodeled the ECM from anabolism to catabolism, gradually leading to DDD (Le Maitre et al., 2005). IL-1 is up-regulated in degenerated human discs, inducing MMP-7, MMP-13, and ADAMTS, suggesting a deregulation of the normal IVD homoeostasis (Le Maitre et al., 2007). The biomechanically-induced inflammatory cytokines can also irritate the sensory nerves and cause pain response (Richardson et al., 2009). It is reported that TNF-α blockers had no effect on matrix degradation, but it might be contributing to discogenic pain in case where nerve ingrowth into IVD degenerative fissures occurs (Hess et al., 2011). By blocking p38 MAPK, it was possible to blunt the expression of factors associated with inflammation and to partially restore the synthesis of proteoglycans (Studer et al., 2008).
Overall, with the development of degeneration induced by altered biomechanical environment, there is a significant reduction in the expression of collagen-II and proteoglycans. Simultaneously, the expression of collagen-I also increases, indicating that matrix stress is changed and may in turn accelerate the progress of disc degeneration (Purmessur et al., 2013). This degenerative circle illustrates the progressive nature of DDD and serves as a backbone to improve scientific discussion and speed-up therapeutic advancement.
Regarding to the multi-factorial nature of AF, three main therapeutic strategies have been practiced in the past, i.e., biomolecular-, nucleic acid-based therapies as well as intracelluar signaling pathways target therapy. All of these approaches have an long-term effects and the pro-anabolic approaches can trigger AF regeneration while lowering pain-related symptoms.
Growth factors (GFs) are one of the indispensability effectors of the cell-cell communication. They are polypeptides that can bind with specific receptors on cells membranes and thereby influence cell behaviors such as ECM synthesis, proliferation and differentiation (Desai et al., 1999). The signaling by GFs can function in three different ways: paracrine (a signal produced by a cell to induce changes in nearby cells), autocrine (a hormone or chemical messenger secreted by a cell to induce self changes) and endocrine (a hormone secreted into the blood circulation directly by a specific organ) (Wang et al., 2003). Delivery of GFs to mobilize endogenous cells probably becomes an effective solution than allogeneic or autologous cell transplantation to reestablish homeostasis in an early degenerated AF. The studies summarized in Table 1 show promising results after injection of various GFs, such as transforming growth factor-β1 (TGF-β1), TGF-β3, bone morphogenetic protein-2 (BMP-2), BMP-7, epidermal growth factor (EGF), basic fibroblast growth factor (bFGF), insulin-like growth factor-I (IGF-I), and platelet-derived growth factor (PDGF) (Tolonen et al., 2006).
Recently, the crucial role of GFs in AF physiology was more proven, and the application of GFs as therapeutic approaches to retard, treat or reverse the process of AF degeneration could be of great potential. Studies on the effects of GFs on cell behavior showed that intradiscal injections of GFs increased ECM production. As reported, IGF-1, and TGF-β1 could stimulate the synthesis of sulphated GAGs, collagen I, collagen II, and reduce activation of matrix metalloproteinase-2 (MMP-2) and MMP-13 in AF cells, as a result of facilitating cells to present a fibrocartilagious phenotype (Moon et al., 2008). GDF-5 is a major factor involved in early IVD development, it favors cell proliferation, and stimulates the synthesis of collagen-II and proteoglycan in vitro. Intradiscal injection of GDF-5 in rabbits and rats was shown to increase disc height. In addition, clinical trials were conduct to evaluate the safety and efficacy of intradiscal injection of GDF-5 in patients with DDD (NCT01124006, NCT01158925 and NCT01182337 on clinicaltrials.gov), however, no peer-reviewed publication is currently available. While a modest improvement in clinical parameters was observed, some researchers suggested that direct intradiscal injection may not be the most suitable route (Blanquer et al., 2015).
In fact, it is likely that GFs act synergistically in promoting anabolic activity of cells. Therefore, effective clinically use of GFs may potentially be obtained by cocktails of them. To design an in vitro model of AF degeneration, Cho et al. applied AF cells that were cultured in monolayer with the stimulation of IL-1β and TNF-α. Researchers analyzed the synergistic effects of TGF-β1 and BMP-2 and compared with administration of either GF alone. Results showed that the combination of the GFs effectively increased the anabolism and decreased the catabolism of AF cells, indicating that the use of cocktails of GFs could provide better outcomes than either alone (Cho et al., 2013).
Despite the therapeutic significance of GFs, several limitations remain to be overcome. Due to the different cell density and populations within AF, it is much more difficult for AF regeneration compared with other tissues. Furthermore, the level of degeneration significantly affected the success of GF therapy as the degenerative AF may lead to down-regulation of ECM synthesis and cell apoptosis (Elmasry et al., 2016). In addition, the half-life of GFs is generally short. Therefore, multiple injections of GFs may be required in order to obtain long-term effects, which may lead to a high risk of unpredictable side effects. Undesirable side effects such as ectopic bone formation after direct injection of BMP may accelerate the degeneration progress (Michalek et al., 2010). Meanwhile, as the internal pressure of disc could bring about leakages in the adjacent tissues, injection of GFs in a liquid state is not considered to be an ideal form of delivery because of its undesirable side effects. Accordingly, gene therapy, and sustained delivery systems are being developed to improve the local delivery of GFs in order to enhance the efficacy of the outcomes.
Delivery of genes into a patient's AF cells using short fragment of DNA can potentially manipulate the synthesis and secretion of encoded proteins to enhance AF homeostasis and possibly suppress degenerative disease (Chadderdon et al., 2004). In terms of the apoptosis of cells in degenerated AF, direct gene therapy can be appropriate for early degenerative stage, while it appears that indirect method is of more interest for more severe cases. One obvious advantage of genetic modification method is the prolonged lasting effect compared with repeated injection of therapeutic GFs, which maintain a desired concentration of GFs for an extended period. However, owing to the very limited cell capacity to uptake genetic material, gene therapy needs vectors to deliver genetic material into the cells (Shimer et al., 2004). Two main methods of gene delivery can be envisioned: (i) In vivo gene therapy, the vectors conveying the modified genes can be introduced in the body directly or (ii) ex vivo gene therapy, target cells can be harvested, genetically modified in vitro, and re-implanted. Two kinds of carriers are mainly used for gene, non-viral and viral vectors delivery. For gene therapy of AF, most studies used adenovirus and adeno-associated virus (AAV) as the vectors. It was found that the use of adenoviral vectors coding for TIMP-1 and BMP-2 on human degenerated disc cells increased proteoglycan synthesis (Wallach et al., 2003b). In addition, the efficacy of AAV-mediated co-transfection of Sox-9 and OP-1 genes were tested in a rabbit disc degeneration model, and the MRI signal, disc height, gene expression, and matrix molecules were found to increase dramatically (Ren et al., 2013). A mass of viral vector delivery systems were evaluated. However, the most prominent problem of viral vehicles is the potential infection and immunological rejection (Wallach et al., 2003a). Non-viral gene therapy involves the use of cationic compounds or naked DNA including liposomes and “gene guns.” There are limited reports on non-virus mediated gene transfer applications in the IVD. Nishida et al. achieved sustained transgene expression in IVD cells in vivo through a microbubble-enhanced ultrasound gene therapy (Nishida et al., 2006). Although the therapy might be the preferred for lower infection possibility, non-viral gene delivery systems are generally of insufficient transfection efficiency. More recently, many investigators developed new more efficient protocols to overcome the limitations of non-viral gene delivery systems (Dash et al., 2011; Mahor et al., 2011). It is promising that non-viral gene delivery systems will draw increasing attention in future therapies for disc diseases.
Gene therapy based on siRNAs is a recently-developed parallel strategy to classic gene therapies (Woods et al., 2011). SiRNAs can control the gene expression by binding to target mRNA and inhibiting RNA degradation or translation. Preliminary studies that investigated the use of siRNA for transfecting disc cells have shown some promising results (Clouet et al., 2018). In an in vitro inflammation model in which AF and NP cells were activated by LPS treatment to mimic their inflammatory phenotypes in DDD, the cells were transfected with IL-1β siRNAs by a polymeric system. Such treatment significantly knocked down the expression of IL-1β. In addition, the inhibitory effect was more pronounced in AF cells than in NP cells (Newland et al., 2013).
To the best of our knowledge, there was no clinical trial based on gene or siRNA therapies for AF regeneration. Despite encouraging results, these approaches need further improvement considering harsh environment where these biological molecules were injected. Therefore, using biomaterials to protect these molecules and nucleic acids can be an effective strategy. The biologics loaded by biomaterials can be delivered in a spatiotemporally controlled manner so as to promote long-term regeneration of AF.
In the recent years, numerous intracellular signaling pathways have been focused on AF degeneration. Intracellular signaling controls most of cellular activities to maintain homeostasis in harsh environment. Studies showed that nuclear factor kappa-B (NF-κB) pathway, mitogen-activated protein kinase (MAPK), and Wnt pathway play crucial roles in adaptation of AF cells, pain mediators and IVD degeneration. Active MAPK and NF-κB signaling can lead to production of matrix degrading enzymes such as MMPs and aggrecanases. A study investigated the roles of MAPK and NF-κB pathways in regulating pro-inflammatory mediators such as TNF-α, IL-1β or IL-6 in several musculoskeletal disorders (Klawitter et al., 2012). MAPK pathway was also identified as a potential therapeutic target for control of anti-catabolic and anti-inflammatory situations in IVD. Suppression of MAPK pathway could also regulate integrin expression, matrix production, osmosis, and cell survival of IVD cells (Yang et al., 2016). Inhibition of p38 MAPK successfully suppressed secretion of pro-inflammatory cytokines while restoring proteoglycan synthesis in AF cells. Likewise, NF-κB activity has also been considered a potential therapeutic target to treat IVD hernitation and associated pain. Increased NF-κB activity was correlated with reduced proteoglycan content in IVD. Blocking NF-κB signaling pathway by inhibitor could improve the accumulation of proteoglycan and was able to counteract the apoptosis of disc cells and matrix deposition in animal models (Morwood and Nicholson, 2006).
Wnt pathway is also associated with the progression of DDD. Wnt signaling follows either the classical Wnt/β-catenin-dependent pathway or the non-classical β-catenin-independent pathway (Kondo et al., 2011). In a study examining the Wnt signaling in IVD, treatment of IVD cells with LiCl, an activator of Wnt, led to an up-regulation of β-catenin, and induced a decrease in cell proliferation (Hiyama et al., 2010). Another study investigated the DDD in β-catenin conditional mice. It seemed that the expression levels of catabolic genes such as aggrecanase and MMP-13 were up-regulated in disc cells of β-catenin knockout mice (Hiyama et al., 2011). These findings suggest that Wnt/β-catenin-dependent pathway might play a key role to maintain the function of IVD.
Recently, the Notch signaling pathway, a hypoxia sensitive transducer, was reported to be closely related to proliferation of progenitor cells in harsh environments of IVD. The proportion of Notch signaling can be activated by hypoxia in IVD cells. Inhibition of Notch signaling blocked hypoxic induction of the activity of the Notch-responsive luciferase reporters and reduced AF cell proliferation. In addition, Notch signaling may play an important role in degenerative processes mediated by pro-inflammatory cytokine (Wang et al., 2013). In degenerate discs, the expression of Notch-2 and IL-1β was significantly increased compared with non-degenerate discs.
More recently, Piezo protein was proved to be a vital mechanical regulator, which had a preponderant influence on maintaining AF tissue homeostasis. Researchers demonstrated that Piezo promoted differentiation of secretory enteroendocrine cells by inhibition of Notch signaling (Sugimoto et al., 2017). Thus, Piezo signaling might become another important factor on proliferation, differentiation, and apoptosis of AF.
Cells are the basic components of biological tissue, which largely determines the properties and functions. To encounter the decreasing cells during AF degeneration, repopulating degenerated AF with healthy cells aims to restore tissue homeostasis by transplanting cells able to secrete functional ECM. Various cells were considered and tested to achieve these clinically objectives. NP and AF cells harvested from surgically removed IVD were first used in human clinical trials with promising results (Gruber et al., 2004). However, the low number of harvested cell limited their further use. Stem cell engineering provides an alternative way to address this issue. With appropriate stimulation, stem cells can be directed to differentiate toward various specific cell phenotypes. Bone marrow derived mesenchymal stem cells (MSCs) and adipose derived stem cells (ASCs) in their undifferentiated state are currently the most widely used cell types (Yañez et al., 2006; Elsaadany et al., 2017). MSCs are considered as immune-evasive and hypo-immunogenic that could be transplanted across major histocompatibility barriers. ASCs are abundant in fat tissue, with advantages of easy accessibility, and more potent immune-modulatory effects in comparison with MSC. These cells were also reported to secrete GAGs and collagen-II when implanted in mice (Hoogendoorn et al., 2008). In animal models such as rabbit, rat and mouse, MSCs injected in the IVD successfully inhibited disc degeneration by reducing the expression of catabolic genes and promoting the synthesis of anabolic genes, resulting in the maintenance of the AF integrity. Recently, pluripotent stem cells and induced pluripotent stem (iPS) cells were also considered for AF regeneration for their theoretical potential to differentiate into AF cells (Kraus and Lufkin, 2017). In the past few years, our group isolated a population of AF-derived colony-forming cells from rabbit IVD. These cells were self-renewable and could be readily induced to differentiate into different cell types including osteocytes, chondrocytes, and adipocytes, respectively. Such AF-derived stem cells (AFSCs) could potentially be a valuable source for repair or regeneration of AF tissue (Liu et al., 2014). In addition, we found that transforming growth factor-β3-mediated BMSC had strong tendency to differentiate into various types of AF cells and presented gene expression profiles similar to AFSCs (Guo et al., 2015).
Mechanical stimulus is one of the most effective stimuli in regulating the differentiation of stem cells (Doroski et al., 2010; Li and Wang, 2010). Applied forces, including compression, tension, hydrostatic pressure, and stress, could remarkably affect the maintenance and lineage specification of MSCs (Discher et al., 2009). It is believed that a diversity of mechanical signals within in vivo niches work together to regulate the fate of stem cells. For example, tensile forces induce osteogenic differentiation while compression forces can lead to chondrogenic differentiation. External mechanical stimuli also influence the growth of cells and formation of ECM. Elsaadany et al. applied varying magnitudes of equiaxial strain at different frequencies to investigate the differentiation of ASCs (Elsaadany et al., 2017). They found that 6% strain and 1 Hz was the optimal loading modality to induce differentiation into AF-like cells and formation of AF-like matrix. Furthermore, the equiaxial load also induced region-specific differentiation of ASCs in the inner and outer regions on their scaffolds. In our recent work, we applied cyclic tensile strain (CTS) with different magnitudes (2, 5, and 12%) during AFSCs culture. The expression of anabolic genes (collagen-I, collagen-II, and aggrecan) in AFSCs was found to increase with increasing applied CTS from low (2%) to moderate (5%) at a frequency of 0.5 Hz for 4 h. In contrast, the anabolic genes expression decreased at high magnitude of CTS (12%). In rabbit injected with stimulated AFSCs (CTS = 5%) in AF, IVD degeneration was found to be significantly slowed down, and disc height and MRI signal intensity were dramatically improved (unpublished data).
Recent advances also highlighted the critical role of internal forces due to cell–matrix interaction (Engler et al., 2006). MSCs were found to be regulated by internal microscopic forces that occurred on contracting cells pulled by each other or by surrounding ECM. These internal forces can be regulated by adjusting the properties of scaffold materials, such as elasticity, microstructure, and substrate patterning (Zhu et al., 2016). The seeded cells can generate contractile forces to sense the properties of substrates and thereby perceive mechanical information that directs the fate of MSC such as proliferation, migration, and differentiation. More importantly, a moderate mechanical regulation is found to be required to stimulate the anabolic expression, which can potentially be utilized for repair and regeneration of AF.
Despite the global promise of cell therapies, the stringer conditions of DDD (low nutrient level, high osmolarity, low oxygen tension, and high intradiscal pressure) hampered the application of therapy by injection of stem cells. To overcome it, engineered scaffolds may be used to create a biomimetic environment that mimics the native structure and mechanical properties of AF. These scaffolds can additionally limit the risk of off-target effects related to cell effusion by promoting cell retention at the injection site.
Tissue engineering, by combining material science and biological engineering, is a promising way to restore the normal functions of tissues and tissue regeneration (Laschke et al., 2009). Scaffolds are one of the most important elements in AF TE by providing appropriate mechanical properties, adequate space, and biochemical cues for seeded cells to grow, differentiate, and produce ECM to regenerate AF tissue (Yeganegi et al., 2010). Ideally, the scaffold should mimic the native AF tissue that should be strong enough to sustain the complex mechanical loads after implantation. Meanwhile, the scaffolds should be fully biodegradable and allow the maintenance of cell phenotype. In addition, they should mimic the microstructure and anisotropic biochemical properties of native AF tissue, allowing an appropriate delivery of bioactive agents, and be suitable to fixation.
Various kinds of scaffolds have been designed for AF engineering. The scaffolds can be made from natural materials or synthetic materials. Nature materials include collagen, hyaluronic acid (HA), chitosan, alginate, silk fibroin, and chondroitin sulfate (CS). Some researchers also paid attention to natural biologic materials such as decellularized matrix from bone, AF, or IVD, fibrinogen isolated from bovine plasma and bone matrix gelatin (BMG) (Chan et al., 2013; Pereira et al., 2018). Obtained by bacterial fermentation or extracted from natural sources, the natural scaffolds are endowed with advantages such as low toxicity, similarity to native tissue, and easy large-scale production. Synthetic polymers are obtained from reproducible industrial products and their mechanical and physicochemical properties can be finely adjusted. The most commonly synthetic materials used for AF TE include poly(trimethylene carbonate) (PTMC), poly(lactide-co-glycolide) (PLGA), poly(ε-caprolactone) (PCL), poly(D, L-lactide) (PDLLA), poly(L-lactide) (PLLA), polyurethane, and HA-poly(ethylene glycol) (PEG) (Nerurkar et al., 2011; Vadalà et al., 2012) (Table 2). These scaffolds can be fabricated and processed using various techniques depending on the desired structure characteristics (aligned, angle-ply, hierarchical, bilayer, biphasic, etc) and mechanical properties of the final engineered tissue. Among the techniques, electrospinning is preferred for AF TE by researchers for its ability to produce micro- and nanofibers which largely recapitulate the structural characters of native AF tissue (Figure 2). In addition, diverse bioactive substances, such as proteins, antibiotics, can be encapsulated in the scaffolds to realized controlled delivery of bioactive molecules to enhance AF regeneration.
Table 2. Composite strategies for AF TE. [Reproduced with permission from (Li et al., 2017)].
Figure 2. (A) Electrospinning of polymer solutions is used to create aligned fibrous sheets that are then wound circumferentially to yield an aligned AF to encase the NP (Reprinted with permission from Bowles and Setton, 2017). (B) The schematic shows the process of building artificial AF by constructing multilamellar PCL/ PLGA/Collagen I (PPC). (C) The TE IVD implant integrates with the native vertebrae. (D) The MRI images of the control (Left) and PPC IVD (Right) after implantation. (E) H&E staining shows the interface between the PPC IVD and the surrounding tissues (Reprinted with permission from Yang et al., 2017).
The microstructure of scaffolds significantly affects the function and morphology of cells cultured on it. By replicating the key length scales and structural features of native AF tissues, aligned fibrous scaffolds are currently preferred for AF regeneration, which are expected to control cell morphology and differentiation, as well as direct the ordered deposition of new ECM. Many studies have shown that fibers orientation can affect the final mechanical properties and the matrix formation of engineered AF constructs. Our group recently found that aligned fibrous scaffolds could provide a favorable microenvironment for AFSCs to differentiate into cell phenotypes similar to AF cells in different regions (Liu et al., 2015). Nerurkar et al. fabricated aligned nanofibrous scaffolds with nonlinear distribution of modulus mimicking that of native AF. They found that AF cells grew along the fibers within scaffolds and formed ECM with considerable alignment (Nerurkar et al., 2009). In a study using silk fibroin (SF) based scaffolds with fibers alignment resembling the fibrous orientation of AF tissue, the scaffolds guided the alignment of human chondrocytes and, in turn, the alignment of the deposited ECM (Bhattacharjee et al., 2012). By offering the combined effects of cell/matrix alignment and chondrogenic re-differentiation support, these aligned SF scaffolds can potentially serve as a suitable substrate for AF regeneration. In all, the topographical and structural features of scaffolds can regulate cell behaviors such as division, matrix synthesis, and apoptosis, and can even direct stem cells to differentiate toward specific lineages.
In addition to composition and structure, the mechanical property of scaffold is also an important designing factor for AF TE (Yeganegi et al., 2010; Nerurkar et al., 2011). An ideal AF substitute should recapitulate the mechanical properties and distribution of native AF tissue. The mechanical properties such as stiffness can significantly affect the cell behaviors such as adhesion, proliferation, differentiation, and migration, and thereby play important roles in AF repair and regeneration. In particular, the stiffness of substrate was found to affect the differentiation of stem cells and direct their lineage specification. Similarly, rat AF cells were reported to be sensitive to substrate stiffness which could regulate their morphology, growth, apoptosis, and ECM metabolism (Zhang et al., 2011). In our recent studies, we fabricated a series of nanofibrous polyurethane scaffolds with elastic modulus close to that of native AF tissue and studied the behaviors of AFSCs and BMSCs seeded on the scaffolds. Depending on the elasticity of scaffold materials, AFSCs showed strong tendency to differentiate into different AF-like cells. On scaffolds with low modulus, the expression of collagen-I in both AFSCs and tBMSCs was relatively low, while the expression collagen-II, and aggrecan was relatively high. The opposite trend was observed on scaffolds with high modulus (Guo et al., 2015; Zhu et al., 2016). Since the inner region of AF mainly consists of collagen-II and PGs and outer regions mainly contains collagen I, it was likely that both AFSCs and tBMSCs tend to differentiate into cells in inner region of AF on the soft scaffolds, whereas they preferred to differentiate into cells in outer region of AF on the stiff scaffolds. Wan et al. fabricated a biphasic elastic scaffold to elastically and structurally simulate the AF. The inner phase of the scaffold was an elastic material based on poly (polycarolactone triol malate) (PPCLM), while the outer part of the scaffold was demineralized bone matrix gelation (BMG). The biphasic scaffold had an enhanced compressive strength and tensile stress than uniphasic scaffold, making it a promising candidate for AF repair (Wan et al., 2008). In all, the mechanical properties of scaffolds remarkably affects the biochemical and biomechanical properties of cultured AFSCs and the ECM they produce. These findings provide new insights toward developing engineered AF with biological characteristics and mechanical functions approximating the native AF tissues.
Scaffolds have been widely used for AF TE. However, limited studies reported the use of scaffolds in combination with the delivery of bioactive agents for AF regeneration. Vadala et al. reported a bioactive electrospun PLLA scaffold containing TGF-β1, which promoted collagen and glycosaminoglycan production and cell proliferation (Vadalà et al., 2012). The half-life activity of TGF-β1 is generally no longer than 2–3 min, and thereby repeated injections are needed which can lead to unwanted side effects like toxicity. The release profile of TGF-β1 from the PLLA electrospun matrix showed a sustained delivery, avoiding the toxic side effects. To enhance AF regeneration, Guillaume et al. developed a biomimetic poly (trimethylene carbonate) (PTMC) scaffold with controlled pore characteristics and combined it with sustained delivery of active compounds as TGF-beta 3 and FGF-2 (Guillaume et al., 2014). Bao et al. reported the incorporation of anti-microbial agents such as berberine in electrospun PCL scaffolds with a sustained strong anti-fungal and anti-bacterial activity for 33 days (Bao et al., 2013). Overall, combining scaffolds with drug delivery capacity is attracting increasing attention for AF engineering.
Effective AF repair is a clinical demand for treating DDD. With the massive findings recently, the physiopathology of AF degeneration has been gradually unveiled. Various biotherapies have been proposed, including molecular therapies, nucleic acid-based therapies and mechano-regulated cell based therapies. These therapies, aiming at supplementing biologics including GFs, genes and cells in AF, have shown promising results in vitro and in vivo. Nevertheless, their clinical uses still remain a major concern due to the short-term efficacy and insufficient stability of them. These limitations may be, at least partially, overcome by biomaterials-based TE using a combination of cells and biomolecules to restore AF anabolism. In addition, advanced drug delivery approaches that suit specifically for AF TE will also be a promising strategy for clinical translation.
In recent years, interdisciplinary strategies addressing biologic, biomechanical, and biomaterial needs for AF regeneration were initiated. Contributions from all these areas are important to construct a better biological implantation, which is expected to replicate the properties of AF and help maintain the mechanical properties of the IVD after implantation. AF regeneration relies on a comprehensive consideration of biological, biomechanical, and biomaterial cues. Future development should pay more attention to making full use of mechanical stimulation or biomaterials-mediated delivery of biomechanics to effectively achieve AF regeneration. Continued exploration of intracellular signaling pathways of biomechanical and biomaterials-mediated mechano-transduction should provide novel approach to restoring the homeostatic mechanical properties of AF and ultimately to achieving effective treatments for DDD.
BL designed the topic and main structure and wrote the manuscript. GC, CS, HW, WZ, and HY wrote the manuscript.
The authors declare that the research was conducted in the absence of any commercial or financial relationships that could be construed as a potential conflict of interest.
This work was supported by the National Key R&D Program of China (2016YFC1100203), National Natural Science Foundation of China (81672213 and 31530024), Jiangsu Provincial Special Program of Medical Science (BL2012004), Jiangsu Provincial Clinical Orthopedic Center, Key Laboratory of Stem Cells, and Biomedical Materials of Jiangsu Province, and Chinese Ministry of Science, and Technology, the Priority Academic Program Development (PAPD) of Jiangsu Higher Education Institutions, and Postgraduate Research & Practice Innovation Program of Jiangsu Province (KYCX18_2529).
Adams, M. A., and Dolan, P. (2012). Intervertebral disc degeneration: evidence for two distinct phenotypes. J. Anat. 221, 497–506. doi: 10.1111/j.1469-7580.2012.01551.x
Adams, M. A., Dolan, P., and McNally, D. S. (2009). The internal mechanical functioning of intervertebral discs and articular cartilage, and its relevance to matrix biology. Matrix Biol. 28, 384–389. doi: 10.1016/j.matbio.2009.06.004
Adams, M. A., Freeman, B. J., Morrison, H. P., Nelson, I. W., and Dolan, P. (2000). Mechanical initiation of intervertebral disc degeneration. Spine 25, 1625–1636. doi: 10.1097/00007632-200007010-00005
Andersson, G. B. (1999). Epidemiological features of chronic low-back pain. Lancet 354, 581–585. doi: 10.1016/S0140-6736(99)01312-4
Bao, J., Lv, W., Sun, Y. Y., and Deng, Y. (2013). Electrospun antimicrobial microfibrous scaffold for annulus fibrosus tissue engineering. J. Mater. Sci. 48, 4223–4232. doi: 10.1007/s10853-013-7235-7
Bhattacharjee, M., Miot, S., Gorecka, A., Singha, K., Loparic, M., Dickinson, S., et al. (2012). Oriented lamellar silk fibrous scaffolds to drive cartilage matrix orientation: towards annulus fibrosus tissue engineering. Acta Biomater. 8, 3313–3325. doi: 10.1016/j.actbio.2012.05.023
Blanquer, S. B., Grijpma, D. W., and Poot, A. A. (2015). Delivery systems for the treatment of degenerated intervertebral discs. Adv. Drug Deliv. Rev. 84, 172–187. doi: 10.1016/j.addr.2014.10.024
Bowles, R. D., Gebhard, H. H., Hartl, R., and Bonassar, L. J. (2011). Tissue-engineered intervertebral discs produce new matrix, maintain disc height, and restore biomechanical function to the rodent spine. Proc. Natl. Acad. Sci. U.S.A. 108, 13106–13111. doi: 10.1073/pnas.1107094108
Bowles, R. D., and Setton, L. A. (2017). Biomaterials for intervertebral disc regeneration and repair. Biomaterials 129, 54–67. doi: 10.1016/j.biomaterials.2017.03.013
Bruehlmann, S. B., Rattner, J. B., Matyas, J. R., and Duncan, N. A. (2002). Regional variations in the cellular matrix of the annulus fibrosus of the intervertebral disc. J. Anat. 201, 159–171. doi: 10.1046/j.1469-7580.2002.00080.x
Buckwalter, J. A. (1995). Aging and degeneration of the human intervertebral disc. Spine 20, 1307–1314. doi: 10.1097/00007632-199506000-00022
Chadderdon, R. C., Shimer, A. L., Gilbertson, L. G., and Kang, J. D. (2004). Advances in gene therapy for intervertebral disc degeneration. Spine J. 4, 341S−347S. doi: 10.1016/j.spinee.2004.07.027
Chan, L. K., Leung, V. Y., Tam, V., Lu, W. W., Sze, K. Y., and Cheung, K. M. (2013). Decellularized bovine intervertebral disc as a natural scaffold for xenogenic cell studies. Acta Biomater. 9, 5262–5272. doi: 10.1016/j.actbio.2012.09.005
Chan, S. C., Ferguson, S. J., and Gantenbein-Ritter, B. (2011). The effects of dynamic loading on the intervertebral disc. Eur. Spine J. 20, 1796–1812. doi: 10.1007/s00586-011-1827-1
Cho, H., Lee, S., Park, S. H., Huang, J., Hasty, K. A., and Kim, S. J. (2013). Synergistic effect of combined growth factors in porcine intervertebral disc degeneration. Connect. Tissue Res. 54, 181–186. doi: 10.3109/03008207.2013.775258
Chubinskaya, S., Kawakami, M., Rappoport, L., Matsumoto, T., Migita, N., and Rueger, D. C. (2007). Anti-catabolic effect of OP-1 in chronically compressed intervertebral discs. J. Orthop. Res. 25, 517–530. doi: 10.1002/jor.20339
Clouet, J., Fusellier, M., Camus, A., Le Visage, C., and Guicheux, J. (2018). Intervertebral disc regeneration: from cell therapy to the development of novel bioinspired endogenous repair strategies. Adv. Drug Deliv. Rev. doi: 10.1016/j.addr.2018.04.017. [Epub ahead of print].
Collin, E. C., Carroll, O., Kilcoyne, M., Peroglio, M., See, E., Hendig, D., et al. (2017). Ageing affects chondroitin sulfates and their synthetic enzymes in the intervertebral disc. Signal Trans. Target. Ther. 2:17049. doi: 10.1038/sigtrans.2017.49
Cortes, D. H., Han, W. M., Smith, L. J., and Elliott, D. M. (2013). Mechanical properties of the extra-fibrillar matrix of human annulus fibrosus are location and age dependent. J. orthop. Res. 31, 1725–1732. doi: 10.1002/jor.22430
Dash, B. C., Mahor, S., Carroll, O., Mathew, A., Wang, W., Woodhouse, K. A., et al. (2011). Tunable elastin-like polypeptide hollow sphere as a high payload and controlled delivery gene depot. J. Control. Release 152, 382–392. doi: 10.1016/j.jconrel.2011.03.006
Desai, B. J., Gruber, H. E., and Hanley, E. N. Jr. (1999). The influence of Matrigel or growth factor reduced Matrigel on human intervertebral disc cell growth and proliferation. Histol. Histopathol. 14, 359–368.
Dezawa, M. (2008). Systematic neuronal and muscle induction systems in bone marrow stromal cells: the potential for tissue reconstruction in neurodegenerative and muscle degenerative diseases. Med. Mol. Morphol. 41, 14–19. doi: 10.1007/s00795-007-0389-0
Discher, D. E., Mooney, D. J., and Zandstra, P. W. (2009). Growth factors, matrices, and forces combine and control stem cells. Science 324, 1673–1677. doi: 10.1126/science.1171643
Doroski, D. M., Levenston, M. E., and Temenoff, J. S. (2010). Cyclic tensile culture promotes fibroblastic differentiation of marrow stromal cells encapsulated in poly(ethylene glycol)-based hydrogels. Tissue Eng. 16, 3457–3466. doi: 10.1089/ten.tea.2010.0233
Driscoll, T. P., Nakasone, R. H., Szczesny, S. E., Elliott, D. M., and Mauck, R. L. (2013). Biaxial mechanics and inter-lamellar shearing of stem-cell seeded electrospun angle-ply laminates for annulus fibrosus tissue engineering. J. Orthop. Res. 31, 864–870. doi: 10.1002/jor.22312
Elmasry, S., Asfour, S., de Rivero Vaccari, J. P., and Travascio, F. (2016). A computational model for investigating the effects of changes in bioavailability of insulin-like growth factor-1 on the homeostasis of the intervertebral disc. Comput. Biol. Med. 78, 126–137. doi: 10.1016/j.compbiomed.2016.09.016
Elsaadany, M., Winters, K., Adams, S., Stasuk, A., Ayan, H., and Yildirim-Ayan, E. (2017). Equiaxial strain modulates adipose-derived stem cell differentiation within 3D biphasic scaffolds towards annulus fibrosus. Sci. Rep. 7:12868. doi: 10.1038/s41598-017-13240-3
Engler, A. J., Sen, S., Sweeney, H. L., and Discher, D. E. (2006). Matrix elasticity directs stem cell lineage specification. Cell 126, 677–689. doi: 10.1016/j.cell.2006.06.044
Finch, P. (2006). Technology Insight: imaging of low back pain. Nat. Clin. Pract. Rheumatol. 2, 554–561. doi: 10.1038/ncprheum0293
Gan, Y. B., Li, S. K., Li, P., Xu, Y., Wang, L. Y., Zhao, C., et al. (2016). A controlled release codelivery system of mscs encapsulated in dextran/gelatin hydrogel with TGF-beta 3-loaded nanoparticles for nucleus pulposus regeneration. Stem Cells Int. 2016:9042019. doi: 10.1155/2016/9042019
Gruber, H. E., Leslie, K., Ingram, J., Norton, H. J., and Hanley, E. N. (2004). Cell-based tissue engineering for the intervertebral disc: in vitro studies of human disc cell gene expression and matrix production within selected cell carriers. Spine J. 4, 44–55. doi: 10.1016/S1529-9430(03)00425-X
Guillaume, O., Daly, A., Lennon, K., Gansau, J., Buckley, S. F., and Buckley, C. T. (2014). Shape-memory porous alginate scaffolds for regeneration of the annulus fibrosus: effect of TGF-beta3 supplementation and oxygen culture conditions. Acta Biomater. 10, 1985–1995. doi: 10.1016/j.actbio.2013.12.037
Guo, Q. P., Liu, C., Li, J., Zhu, C. H., Yang, H. L., and Li, B. (2015). Gene expression modulation in TGF-3-mediated rabbit bone marrow stem cells using electrospun scaffolds of various stiffness. J. Cell. Mol. Med. 19, 1582–1592. doi: 10.1111/jcmm.12533
Haglund, L., Moir, J., Beckman, L., Mulligan, K. R., Jim, B., Ouellet, J. A., et al. (2011). Development of a bioreactor for axially loaded intervertebral disc organ culture. Tissue Eng. Methods 17, 1011–1019. doi: 10.1089/ten.tec.2011.0025
Han, S. K., Chen, C. W., Wierwille, J., Chen, Y., and Hsieh, A. H. (2015). Three dimensional mesoscale analysis of translamellar cross-bridge morphologies in the annulus fibrosus using optical coherence tomography. J. Orthop. Res. 33, 304–311. doi: 10.1002/jor.22778
Hegewald, A. A., Medved, F., Feng, D., Tsagogiorgas, C., Beierfuss, A., Schindler, G. A., et al. (2013). Enhancing tissue repair in annulus fibrosus defects of the intervertebral disc: analysis of a bio-integrative annulus implant in an in-vivo ovine model. J. Tissue Eng. Regen. Med. 9, 405–414. doi: 10.1002/term.1831
Henry, N., Clouet, J., Le Visage, C., Weiss, P., Gautron, E., Renard, D., et al. (2017). Silica nanofibers as a new drug delivery system: a study of the protein-silica interactions. J. Mater. Chem B. 5, 2908–2920. doi: 10.1039/C7TB00332C
Hess, A., Axmann, R., Rech, J., Finzel, S., Heindl, C., Kreitz, S., et al. (2011). Blockade of TNF-alpha rapidly inhibits pain responses in the central nervous system. Proc. Natl. Acad. Sci. U.S.A. 108, 3731–3736. doi: 10.1073/pnas.1011774108
Hiyama, A., Sakai, D., Risbud, M. V., Tanaka, M., Arai, F., Abe, K., et al. (2010). Enhancement of intervertebral disc cell senescence by WNT/beta-catenin signaling-induced matrix metalloproteinase expression. Arthritis Rheum. 62, 3036–3047. doi: 10.1002/art.27599
Hiyama, A., Sakai, D., Tanaka, M., Arai, F., Nakajima, D., Abe, K., et al. (2011). The relationship between the Wnt/beta-catenin and TGF-beta/BMP signals in the intervertebral disc cell. J. Cell. Physiol. 226, 1139–1148. doi: 10.1002/jcp.22438
Hoogendoorn, R. J., Lu, Z. F., Kroeze, R. J., Bank, R. A., Wuisman, P. I., and Helder, M. N. (2008). Adipose stem cells for intervertebral disc regeneration: current status and concepts for the future. J. Cell. Mol. Med. 12, 2205–2216. doi: 10.1111/j.1582-4934.2008.00291.x
Hsieh, A. H., and Twomey, J. D. (2010). Cellular mechanobiology of the intervertebral disc: new directions and approaches. J. Biomech. 43, 137–145. doi: 10.1016/j.jbiomech.2009.09.019
Kim, D. J., Moon, S. H., Kim, H., Kwon, U. H., Park, M. S., Han, K. J., et al. (2003). Bone morphogenetic protein-2 facilitates expression of chondrogenic, not osteogenic, phenotype of human intervertebral disc cells. Spine 28, 2679–2684. doi: 10.1097/01.BRS.0000101445.46487.16
Klawitter, M., Quero, L., Klasen, J., Liebscher, T., Nerlich, A., Boos, N., et al. (2012). Triptolide exhibits anti-inflammatory, anti-catabolic as well as anabolic effects and suppresses TLR expression and MAPK activity in IL-1beta treated human intervertebral disc cells. Eur. Spine J. 21 (Suppl. 6), S850–S859. doi: 10.1007/s00586-011-1919-y
Koepsell, L., Zhang, L., Neufeld, D., Fong, H., and Deng, Y. (2011). Electrospun nanofibrous polycaprolactone scaffolds for tissue engineering of annulus fibrosus. Macromol. Biosci. 11, 391–399. doi: 10.1002/mabi.201000352
Kondo, N., Yuasa, T., Shimono, K., Tung, W., Okabe, T., Yasuhara, R., et al. (2011). Intervertebral disc development is regulated by Wnt/beta-catenin signaling. Spine 36, E513–E518. doi: 10.1097/BRS.0b013e3181f52cb5
Kraus, P., and Lufkin, T. (2017). Implications for a stem cell regenerative medicine based approach to human intervertebral disk degeneration. Front. Cell Dev. Biol. 5:17. doi: 10.3389/fcell.2017.00017
Laschke, M. W., Strohe, A., Scheuer, C., Eglin, D., Verrier, S., Alini, M., et al. (2009). In vivo biocompatibility and vascularization of biodegradable porous polyurethane scaffolds for tissue engineering. Acta Biomater. 5, 1991–2001. doi: 10.1016/j.actbio.2009.02.006
Le Maitre, C. L., Frain, J., Fotheringham, A. P., Freemont, A. J., and Hoyland, J. A. (2008). Human cells derived from degenerate intervertebral discs respond differently to those derived from non-degenerate intervertebral discs following application of dynamic hydrostatic pressure. Biorheology 45, 563–575. doi: 10.3233/BIR-2008-0498
Le Maitre, C. L., Freemont, A. J., and Hoyland, J. A. (2005). The role of interleukin-1 in the pathogenesis of human intervertebral disc degeneration. Arthritis Res. Ther. 7, R732–R745. doi: 10.1186/ar1732
Le Maitre, C. L., Pockert, A., Buttle, D. J., Freemont, A. J., and Hoyland, J. A. (2007). Matrix synthesis and degradation in human intervertebral disc degeneration. Biochem. Soc. Trans. 35, 652–655. doi: 10.1042/BST0350652
Li, B., Li, J., Zhou, P., and Yang, H. (2017). “Annulus fibrosus tissue engineering: achievements and future development,” in Handbook of Intelligent Scaffolds for Tissue Engineering and Regenerative Medicine, 2nd Edn., ed G. Khang (Singapore: Pan Stanford Publishing Pte. Ltd.), 1013–1054.
Li, B., Lin, M., Tang, Y., Wang, B., and Wang, J. H. (2008). A novel functional assessment of the differentiation of micropatterned muscle cells. J. Biomech. 41, 3349–3353. doi: 10.1016/j.jbiomech.2008.09.025
Li, B., and Wang, J. H. (2010). Application of sensing techniques to cellular force measurement. Sensors 10, 9948–9962. doi: 10.3390/s101109948
Li, J., Liu, C., Guo, Q., Yang, H., and Li, B. (2014). Regional variations in the cellular, biochemical, and biomechanical characteristics of rabbit annulus fibrosus. PLoS ONE 9:e91799. doi: 10.1371/journal.pone.0091799
Li, X., Dou, Q., and Kong, Q. (2016). Repair and regenerative therapies of the annulus fibrosus of the intervertebral disc. J. Coll. Phys. Surg. Pak. 26, 138–144.
Liu, C., Guo, Q., Li, J., Wang, S., Wang, Y., Li, B., et al. (2014). Identification of rabbit annulus fibrosus-derived stem cells. PLoS ONE 9:e108239. doi: 10.1371/journal.pone.0108239
Liu, C., Zhu, C., Li, J., Zhou, P., Chen, M., Yang, H., et al. (2015). The effect of the fibre orientation of electrospun scaffolds on the matrix production of rabbit annulus fibrosus-derived stem cells. Bone Res. 3:15012. doi: 10.1038/boneres.2015.12
Liu, G. Z., Ishihara, H., Osada, R., Kimura, T., and Tsuji, H. (2001). Nitric oxide mediates the change of proteoglycan synthesis in the human lumbar intervertebral disc in response to hydrostatic pressure. Spine 26, 134–141. doi: 10.1097/00007632-200101150-00005
Mahor, S., Collin, E., Dash, B. C., and Pandit, A. (2011). Controlled release of plasmid DNA from hyaluronan nanoparticles. Curr. Drug Deliv. 8, 354–362. doi: 10.2174/156720111795768031
Martin, J. T., Milby, A. H., Chiaro, J. A., Kim, D. H., Hebela, N. M., Smith, L. J., et al. (2014). Translation of an engineered nanofibrous disc-like angle-ply structure for intervertebral disc replacement in a small animal model. Acta Biomater. 10, 2473–2481. doi: 10.1016/j.actbio.2014.02.024
Michalek, A. J., Buckley, M. R., Bonassar, L. J., Cohen, I., and Iatridis, J. C. (2010). The effects of needle puncture injury on microscale shear strain in the intervertebral disc annulus fibrosus. Spine J. 10, 1098–1105. doi: 10.1016/j.spinee.2010.09.015
Mizuno, H., Roy, A. K., Vacanti, C. A., Kojima, K., Ueda, M., and Bonassar, L. J. (2004). Tissue-engineered composites of anulus fibrosus and nucleus pulposus for intervertebral disc replacement. Spine 29, 1290–1297; discussion 7–8. doi: 10.1097/01.BRS.0000128264.46510.27
Moon, S. H., Nishida, K., Gilbertson, L. G., Lee, H. M., Kim, H., Hall, R. A., et al. (2008). Biologic response of human intervertebral disc cells to gene therapy cocktail. Spine 33, 1850–1855. doi: 10.1097/BRS.0b013e31817e1cd7
Morwood, S. R., and Nicholson, L. B. (2006). Modulation of the immune response by extracellular matrix proteins. Arch. Immunol. Ther. Exp. 54, 367–374. doi: 10.1007/s00005-006-0043-x
Murray, C. J., Vos, T., Lozano, R., Naghavi, M., Flaxman, A. D., Michaud, C., et al. (2012). Disability-adjusted life years (DALYs) for 291 diseases and injuries in 21 regions, 1990-2010: a systematic analysis for the Global Burden of Disease Study 2010. Lancet 380, 2197–2223. doi: 10.1016/S0140-6736(12)61689-4
Nerurkar, N. L., Baker, B. M., Sen, S., Wible, E. E., Elliott, D. M., and Mauck, R. L. (2009). Nanofibrous biologic laminates replicate the form and function of the annulus fibrosus. Nat. Mater. 8, 986–992. doi: 10.1038/nmat2558
Nerurkar, N. L., Sen, S., Baker, B. M., Elliott, D. M., and Mauck, R. L. (2011). Dynamic culture enhances stem cell infiltration and modulates extracellular matrix production on aligned electrospun nanofibrous scaffolds. Acta Biomater. 7, 485–491. doi: 10.1016/j.actbio.2010.08.011
Newland, B., Moloney, T. C., Fontana, G., Browne, S., Abu-Rub, M. T., Dowd, E., et al. (2013). The neurotoxicity of gene vectors and its amelioration by packaging with collagen hollow spheres. Biomaterials 34, 2130–2141. doi: 10.1016/j.biomaterials.2012.11.049
Nishida, K., Doita, M., Takada, T., Kakutani, K., Miyamoto, H., Shimomura, T., et al. (2006). Sustained transgene expression in intervertebral disc cells in vivo mediated by microbubble-enhanced ultrasound gene therapy. Spine 31, 1415–1419. doi: 10.1097/01.brs.0000219945.70675.dd
Pan, Y., Chu, T., Dong, S., Hao, Y., Ren, X., Wang, J., et al. (2012). Cells scaffold complex for Intervertebral disc Anulus Fibrosus tissue engineering: in vitro culture and product analysis. Mol. Biol. Rep. 39, 8581–8594. doi: 10.1007/s11033-012-1710-0
Park, S. H., Gil, E. S., Cho, H., Mandal, B. B., Tien, L. W., Min, B. H., et al. (2012a). Intervertebral disk tissue engineering using biphasic silk composite scaffolds. Tissue Eng. 18, 447–458. doi: 10.1089/ten.tea.2011.0195
Park, S. H., Gil, E. S., Mandal, B. B., Cho, H., Kluge, J. A., Min, B. H., et al. (2012b). Annulus fibrosus tissue engineering using lamellar silk scaffolds. J. Tissue Eng. Regen. Med. 6 (Suppl. 3), s24–s33. doi: 10.1002/term.541
Paul, R., Haydon, R. C., Cheng, H., Ishikawa, A., Nenadovich, N., Jiang, W., et al. (2003). Potential use of Sox9 gene therapy for intervertebral degenerative disc disease. Spine 28, 755–763. doi: 10.1097/01.BRS.0000058946.64222.92
Pereira, D. R., Silva-Correia, J., Oliveira, J. M., Reis, R. L., Pandit, A., and Biggs, M. J. (2018). Nanocellulose reinforced gellan-gum hydrogels as potential biological substitutes for annulus fibrosus tissue regeneration. Nanomedicine 14, 897–908. doi: 10.1016/j.nano.2017.11.011
Purmessur, D., Walter, B. A., Roughley, P. J., Laudier, D. M., Hecht, A. C., and Iatridis, J. (2013). A role for TNFalpha in intervertebral disc degeneration: a non-recoverable catabolic shift. Biochem. Biophys. Res. Commun. 433, 151–156. doi: 10.1016/j.bbrc.2013.02.034
Ren, S., Liu, Y., Ma, J., Liu, Y., Diao, Z., Yang, D., et al. (2013). Treatment of rabbit intervertebral disc degeneration with co-transfection by adeno-associated virus-mediated SOX9 and osteogenic protein-1 double genes in vivo. Int. J. Mol. Med. 32, 1063–1068. doi: 10.3892/ijmm.2013.1497
Richardson, S. M., Doyle, P., Minogue, B. M., Gnanalingham, K., and Hoyland, J. A. (2009). Increased expression of matrix metalloproteinase-10, nerve growth factor and substance P in the painful degenerate intervertebral disc. Arthritis Res. Ther. 11:R126. doi: 10.1186/ar2793
Sharifi, S., Bulstra, S. K., Grijpma, D. W., and Kuijer, R. (2015). Treatment of the degenerated intervertebral disc; closure, repair and regeneration of the annulus fibrosus. J. Tissue Eng. Regen. Med. 9, 1120–1132. doi: 10.1002/term.1866
Shimer, A. L., Chadderdon, R. C., Gilbertson, L. G., and Kang, J. D. (2004). Gene therapy approaches for intervertebral disc degeneration. Spine 29, 2770–2778. doi: 10.1097/01.brs.0000146455.11253.08
Smith, R. L., Carter, D. R., and Schurman, D. J. (2004). Pressure and shear differentially alter human articular chondrocyte metabolism: a review. Clin. Orthop. Relat. Res. 427 (Suppl.), S89–S95. doi: 10.1097/01.blo.0000143938.30681.9d
Studer, R. K., Gilbertson, L. G., Georgescu, H., Sowa, G., Vo, N., and Kang, J. D. (2008). p38 MAPK inhibition modulates rabbit nucleus pulposus cell response to IL-1. J. Orthop. Res. 26, 991–998. doi: 10.1002/jor.20604
Sugimoto, A., Miyazaki, A., Kawarabayashi, K., Shono, M., Akazawa, Y., Hasegawa, T., et al. (2017). Piezo type mechanosensitive ion channel component 1 functions as a regulator of the cell fate determination of mesenchymal stem cells. Sci. Rep. 7:17696. doi: 10.1038/s41598-017-18089-0
Tolonen, J., Gronblad, M., Vanharanta, H., Virri, J., Guyer, R. D., Rytomaa, T., et al. (2006). Growth factor expression in degenerated intervertebral disc tissue. An immunohistochemical analysis of transforming growth factor beta, fibroblast growth factor and platelet-derived growth factor. Eur. SPine J. 15, 588–596. doi: 10.1007/s00586-005-0930-6
Turner, K. G., Ahmed, N., Santerre, J. P., and Kandel, R. A. (2014). Modulation of annulus fibrosus cell alignment and function on oriented nanofibrous polyurethane scaffolds under tension. Spine J. 14, 424–434. doi: 10.1016/j.spinee.2013.08.047
Urban, J. P., Smith, S., and Fairbank, J. C. (2004). Nutrition of the intervertebral disc. Spine 29, 2700–2709. doi: 10.1097/01.brs.0000146499.97948.52
Vadalà, G., Mozetic, P., Rainer, A., Centola, M., Loppini, M., Trombetta, M., et al. (2012). Bioactive electrospun scaffold for annulus fibrosus repair and regeneration. Eur. Spine J. 21 (Suppl. 1), S20–S26. doi: 10.1007/s00586-012-2235-x
Wallach, C. J., Gilbertson, L. G., and Kang, J. D. (2003a). Gene therapy applications for intervertebral disc degeneration. Spine 28, S93–S98. doi: 10.1097/01.BRS.0000076905.31596.DF
Wallach, C. J., Sobajima, S., Watanabe, Y., Kim, J. S., Georgescu, H. I., Robbins, P., et al. (2003b). Gene transfer of the catabolic inhibitor TIMP-1 increases measured proteoglycans in cells from degenerated human intervertebral discs. Spine 28, 2331–2337. doi: 10.1097/01.BRS.0000085303.67942.94
Wan, Y., Feng, G., Shen, F. H., Laurencin, C. T., and Li, X. (2008). Biphasic scaffold for annulus fibrosus tissue regeneration. Biomaterials 29, 643–652. doi: 10.1016/j.biomaterials.2007.10.031
Wang, D. L., Jiang, S. D., and Dai, L. Y. (2007). Biologic response of the intervertebral disc to static and dynamic compression in vitro. Spine 32, 2521–2528. doi: 10.1097/BRS.0b013e318158cb61
Wang, F., Qu, D. B., and Jin, D. D. (2003). Growth factor and regeneration of intervertebral disc. Zhongguo Xiu Fu Chong Jian Wai Ke Za Zhi. 17, 73–75.
Wang, H., Tian, Y., Wang, J., Phillips, K. L., Binch, A. L., Dunn, S., et al. (2013). Inflammatory cytokines induce NOTCH signaling in nucleus pulposus cells: implications in intervertebral disc degeneration. J. Biol. Chem. 288, 16761–16774. doi: 10.1074/jbc.M112.446633
Wismer, N., Grad, S., Fortunato, G., Ferguson, S. J., Alini, M., and Eglin, D. (2014). Biodegradable electrospun scaffolds for annulus fibrosus tissue engineering: effect of scaffold structure and composition on annulus fibrosus cells in vitro. Tissue Eng. 20, 672–682. doi: 10.1089/ten.tea.2012.0679
Woods, B. I., Vo, N., Sowa, G., and Kang, J. D. (2011). Gene therapy for intervertebral disk degeneration. Orthop Clin. North Am. 42, 563–574. doi: 10.1016/j.ocl.2011.07.002
Wu, S. C., Chang, J. K., Wang, C. K., Wang, G. J., and Ho, M. L. (2010). Enhancement of chondrogenesis of human adipose derived stem cells in a hyaluronan-enriched microenvironment. Biomaterials 31, 631–640. doi: 10.1016/j.biomaterials.2009.09.089
Wuertz, K., Urban, J. P., Klasen, J., Ignatius, A., Wilke, H. J., Claes, L., et al. (2007). Influence of extracellular osmolarity and mechanical stimulation on gene expression of intervertebral disc cells. J. Orthop. Res. 25, 1513–1522. doi: 10.1002/jor.20436
Yañez, R., Lamana, M. L., Garcia-Castro, J., Colmenero, I., Ramirez, M., and Bueren, J. A. (2006). Adipose tissue-derived mesenchymal stem cells have in vivo immunosuppressive properties applicable for the control of the graft-versus-host disease. Stem Cells 24, 2582–2591. doi: 10.1634/stemcells.2006-0228
Yang, C., Cao, P., Gao, Y., Wu, M., Lin, Y., Tian, Y., et al. (2016). Differential expression of p38 MAPK alpha, beta, gamma, delta isoforms in nucleus pulposus modulates macrophage polarization in intervertebral disc degeneration. Sci. Rep. 6:22182. doi: 10.1038/srep22182
Yang, J., Yang, X., Wang, L., Zhang, W., Yu, W., Wang, N., et al. (2017). Biomimetic nanofibers can construct effective tissue-engineered intervertebral discs for therapeutic implantation. Nanoscale 9, 13095–13103. doi: 10.1039/C7NR03944A
Yeganegi, M., Kandel, R. A., and Santerre, J. P. (2010). Characterization of a biodegradable electrospun polyurethane nanofiber scaffold: mechanical properties and cytotoxicity. Acta Biomater. 6, 3847–3855. doi: 10.1016/j.actbio.2010.05.003
Yoshimura, H., Muneta, T., Nimura, A., Yokoyama, A., Koga, H., and Sekiya, I. (2007). Comparison of rat mesenchymal stem cells derived from bone marrow, synovium, periosteum, adipose tissue, and muscle. Cell Tissue Res. 327, 449–462. doi: 10.1007/s00441-006-0308-z
Zhang, Y., Anderson, D. G., Phillips, F. M., Thonar, E. J., He, T. C., Pietryla, D., et al. (2007). Comparative effects of bone morphogenetic proteins and Sox9 overexpression on matrix accumulation by bovine anulus fibrosus cells: implications for anular repair. Spine 32, 2515–2520. doi: 10.1097/BRS.0b013e318158cc09
Zhang, Y. H., Zhao, C. Q., Jiang, L. S., and Dai, L. Y. (2011). Substrate stiffness regulates apoptosis and the mRNA expression of extracellular matrix regulatory genes in the rat annular cells. Matrix Biol. 30, 135–144. doi: 10.1016/j.matbio.2010.10.008
Keywords: intervertebral disc, annulus fibrosus, tissue regeneration, biomolecular therapy, gene therapy, mechano-regulation, scaffolds, tissue engineering
Citation: Chu G, Shi C, Wang H, Zhang W, Yang H and Li B (2018) Strategies for Annulus Fibrosus Regeneration: From Biological Therapies to Tissue Engineering. Front. Bioeng. Biotechnol. 6:90. doi: 10.3389/fbioe.2018.00090
Received: 04 April 2018; Accepted: 18 June 2018;
Published: 10 July 2018.
Edited by:
Martijn van Griensven, Technische Universität München, GermanyReviewed by:
Dimitrios I. Zeugolis, National University of Ireland Galway, IrelandCopyright © 2018 Chu, Shi, Wang, Zhang, Yang and Li. This is an open-access article distributed under the terms of the Creative Commons Attribution License (CC BY). The use, distribution or reproduction in other forums is permitted, provided the original author(s) and the copyright owner(s) are credited and that the original publication in this journal is cited, in accordance with accepted academic practice. No use, distribution or reproduction is permitted which does not comply with these terms.
*Correspondence: Bin Li, YmlubGlAc3VkYS5lZHUuY24=
†These authors have contributed equally to this work.
Disclaimer: All claims expressed in this article are solely those of the authors and do not necessarily represent those of their affiliated organizations, or those of the publisher, the editors and the reviewers. Any product that may be evaluated in this article or claim that may be made by its manufacturer is not guaranteed or endorsed by the publisher.
Research integrity at Frontiers
Learn more about the work of our research integrity team to safeguard the quality of each article we publish.