- 1Drosophila Genetics and Epigenetics, Sorbonne Université, Centre National de la Recherche Scientifique, Biologie du Développement—Institut de Biologie Paris Seine, Paris, France
- 2Laboratory of RNA Epigenetics, Institute of Molecular Biology, Mainz, Germany
Analogous to DNA methylation and histone modifications, RNA modifications represent a novel layer of regulation of gene expression. The dynamic nature and increasing number of RNA modifications offer new possibilities to rapidly alter gene expression upon specific environmental changes. Recent lines of evidence indicate that modified RNA molecules and associated complexes regulating and “reading” RNA modifications play key roles in the nervous system of several organisms, controlling both, its development and function. Mutations in several human genes that modify transfer RNA (tRNA) have been linked to neurological disorders, in particular to intellectual disability. Loss of RNA modifications alters the stability of tRNA, resulting in reduced translation efficiency and generation of tRNA fragments, which can interfere with neuronal functions. Modifications present on messenger RNAs (mRNAs) also play important roles during brain development. They contribute to neuronal growth and regeneration as well as to the local regulation of synaptic functions. Hence, potential combinatorial effects of RNA modifications on different classes of RNA may represent a novel code to dynamically fine tune gene expression during brain function. Here we discuss the recent findings demonstrating the impact of modified RNAs on neuronal processes and disorders.
Introduction
An estimated 1–2% of all genes in a given organism contribute to nucleic acid modification systems, suggesting biological importance of modified nucleotides (Grosjean, 2009). A classic example is the methylation of cytosine on DNA, which acts as a critical epigenetic regulator of gene expression (Bird, 2002). Additionally, current advances in RNA modification research report over 140 distinct post-transcriptional RNA modifications (Cantara et al., 2011; Machnicka et al., 2013). Initial knowledge has been derived from studies on abundant non-coding RNAs (ncRNAs), such as transfer RNAs (tRNAs) and ribosomal RNAs (rRNAs), in prokaryotes and simple eukaryotes. These pioneer investigations described a diverse, chemically complex, and strongly conserved nature of RNA nucleotide modifications (Cantara et al., 2011; Machnicka et al., 2013). The most heavily modified RNAs in any cell type and organism are tRNAs. Up to 20% of nucleotides in mammalian cytoplasmic tRNAs carry modifications (Motorin and Helm, 2011; Pan, 2018). Modified nucleotides outside the anticodon loop of tRNAs occur non-randomly at conserved positions across diverse species and affect in general its stability (Helm, 2006; Motorin and Helm, 2010). In addition, modifications in the anticodon loop can contribute to optimize mRNA decoding by directly affecting codon-anticodon interactions (Agris, 2008).
Aberrant tRNA and rRNA modifications have been linked to various human disease syndromes and the phenotypes are often observed in specific tissues such as the gonads and the nervous system (Torres et al., 2014). Notably, increasing number of predicted human transfer RNA (tRNA) modification genes have been associated with neurological disorders, in particular with intellectual disability (ID) (for recent review see Bednárová et al., 2017). ID, or previously known as Mental Retardation (MR), is characterized by non-progressive cognitive impairment and affects 1–3% of the general population (Daily et al., 2000). It is presently unclear whether all observed phenotypes are caused by aberrant tRNA modifications, by effects on unidentified other RNA substrates (see below) and/or by a modification-independent function of the involved enzymes (Guo and Schimmel, 2013; Genenncher et al., 2018). Likewise, it is unknown why some tissues, in particular the brain, are more sensitive to the loss of these modifications.
Importantly, besides the heavily modified tRNAs and rRNAs, mRNAs, small and long non-coding RNAs were also found to harbor post-transcriptional modifications. Recent technological advances that allowed mapping of selected RNA modifications on a transcriptome-wide scale revealed widespread distribution of N6-methyladenosine (m6A), pseudouridine (Ψ) and ribose 2′-O-methylation (Nm) on mRNA (Dominissini et al., 2012; Meyer et al., 2012; Carlile et al., 2014; Schwartz et al., 2014a; Dai et al., 2017). The prevalence of some others, including N1-methyladenine (m1A) and 5-methylcytidine (m5C) is still debated (Dominissini et al., 2016; Li et al., 2016, 2017c; Dominissini and Rechavi, 2017; Legrand et al., 2017; Safra et al., 2017). m6A, the most abundant mRNA modification, was shown to affect almost every step of mRNA biogenesis, including splicing, export, translation, and mRNA decay (Lence et al., 2017; Roignant and Soller, 2017). It is thus not surprising that misregulation of m6A results in several physiological defects, including brain development abnormalities, obesity, cancer, and other diseases (Batista, 2017; Dai et al., 2018). In addition, the discovery of m6A RNA demethylases (Jia et al., 2011; Zheng et al., 2013; Jacob-Hirsch et al., 2018) and the identification of m6A-binding proteins (Dominissini et al., 2012) indicated that similarly to DNA modification, RNA methylation can be reversible and convey information via recognition of effector proteins.
Altogether these recent studies revealed an entire new layer of regulation of gene expression, which has been central to the development of a novel concept called “RNA epigenetics or epitranscriptomics” (He, 2010; Meyer et al., 2012). However, the exact biological function of the majority of modified RNA nucleotides remains to be discovered. In this review, we will focus on several RNA modifications and will discuss their involvement in the development of the brain and neurological disorders.
5-Methylcytosine (m5C)
Cytosine can be methylated at the 5th position of the pyrimidine ring to form 5-methylcytosine (m5C) (Figure 1). Various eukaryotic cytosine-5-RNA methyltransferases catalyze the formation of m5C at specific positions (Motorin and Grosjean, 1999; Brzezicha et al., 2006; Sharma et al., 2013; Metodiev et al., 2014; Haag et al., 2015; Schosserer et al., 2015). The analysis of genetic mutations in two particular RNA cytosine-5 methyltransferase family members (Dnmt2/Trdmt and NCL1/TRM4/NSun2) has provided important insights into the biological effects of aberrant m5C deposition.
Dnmt2
Dnmt2 is a member of the most widely conserved eukaryotic cytosine-5-DNA methyltransferase protein family (Goll and Bestor, 2005). Despite this classification, only few studies reported Dnmt2-mediated DNA methylation (Hermann et al., 2003; Kunert et al., 2003; Phalke et al., 2009) and it is today acknowledged that Dnmt2 functions mainly as a tRNA methylase (Okano et al., 1998; Schaefer and Lyko, 2010a,b; Raddatz et al., 2013). Dnmt2 methylation activity on position C38 of three tRNAs, which include tRNAAsp, tRNAVal, and tRNAGly, has been described in yeast, Drosophila, mouse, and human cells (Goll et al., 2006; Jurkowski et al., 2008; Schaefer et al., 2010). Knockdown of Dnmt2 in zebrafish embryos leads to differentiation defects in some organs, and notably, to abnormal neurogenesis in the hypothalamus and diencephalon (Rai et al., 2007). In Dnmt2 mutant flies, reduced viability under stress conditions was observed (Schaefer et al., 2010). This is in accordance with previous studies that suggest an increased tolerance for stress in Drosophila and Entamoeba upon Dnmt2 overexpression (Lin et al., 2005; Fisher et al., 2006). Nevertheless, the majority of studies suggests that Dnmt2 mutation does not trigger strong detrimental phenotypes in yeast, Drosophila and mice (Wilkinson et al., 1995; Kunert et al., 2003; Goll et al., 2006; Schaefer et al., 2010), which raises the question why zebrafish relies on Dnmt2 for proper development, whereas mice and flies do not. One possible explanation is that these organisms have redundant mechanisms that compensate for the loss of Dnmt2, which may be absent or less robust in zebrafish. Consistent with this possibility, it was shown that Dnmt2 mutant mice exhibit lethal phenotypes in the absence of a second m5C methyltransferase, NSun2 (Tuorto et al., 2012). In human, polymorphisms in DNMT2 have been associated with spina bifida, a congenital malformation of the central nervous system (Franke et al., 2009).
NSun2
Unlike Dnmt2, it has been established that mammalian NSun2 does not only modify tRNAs (Blanco et al., 2011; Tuorto et al., 2012) but also other small ncRNAs such as 7SK, vault, and Y-RNAs (Hussain et al., 2013; Khoddami and Cairns, 2013). Dnmt2 and Nsun2 double knockout mice showed a lethal phenotype. However, deletion of NSun2 alone (Blanco et al., 2011; Tuorto et al., 2012; Hussain et al., 2013) or in combination with Dnmt2 (Rai et al., 2007; Tuorto et al., 2012) in specific tissues impairs cellular differentiation pathways in mammalian skin, testes, and brain. The function in the brain appears conserved as Nsun2 mutations are associated with ID and Dubowitz-like syndrome in humans (Abbasi-Moheb et al., 2012; Khan et al., 2012; Martinez et al., 2012), as well as with microcephaly in human and mice (Blanco et al., 2014). In addition, a recent study in human and mice neuron precursor cells showed that m5C deposited by Nsun2 regulates neural stem cell (NSC) differentiation and motility (Flores et al., 2017). This study thus provides some links between the failure of RNA m5C deposition and the associated brain development diseases.
It is intriguing that patient fibroblasts and Nsun2-deficient mice (Blanco et al., 2014), as well as Dnmt2 mutant flies (Durdevic et al., 2013), exhibit increase cleavage of tRNA, and elevated production of tRNA fragments (tRFs). This accumulation of tRFs reduces protein translation rates and increases oxidative stress as well as neuronal apoptosis. Interestingly, reducing tRNA cleavage in Nsun2-deficient brains is sufficient to rescue sensitivity to oxidative stress, implying that tRFs play a role in Nsun2-mediated defects.
(2′-O)-Methylation (Nm)
2′-O-methylation (Nm) is a common nucleoside modification of RNA, where a methyl group is added to the 2′ hydroxyl of the ribose moiety (Figure 1). Nm increases hydrophobicity, protects RNAs from nuclease attacks and stabilizes helical structures (Kurth and Mochizuki, 2009; Byszewska et al., 2014; Kumar et al., 2014; Yildirim et al., 2014). Nm is predominantly found internally in ribosomal RNAs and small nuclear RNAs as well as in tRNAs and in a number of sites on mRNA (Darzacq et al., 2002; Rebane et al., 2002; Kurth and Mochizuki, 2009; Zhao et al., 2012; Somme et al., 2014; Dai et al., 2017). This modification is also present at the 3′-end of miRNAs and siRNAs in plants (Li et al., 2005; Yu et al., 2005), as well as in siRNAs and piRNAs in animals (Horwich et al., 2007; Saito et al., 2007). Nm methyltransferases acting on tRNAs are highly conserved from bacteria and archaea to humans (Somme et al., 2014) and usually target positions in the anticodon loop. For instance, TRM7 in S. cerevisiae modifies positions 32 and 34 of selected tRNA, amongst which is tRNAPhe (Pintard et al., 2002; Guy et al., 2012). Strikingly, FTSJ1, the TRM7 ortholog in human, methylates the exact same positions of the exact same tRNAs (Guy and Phizicky, 2015; Guy et al., 2015). Consistent with a conserved function, expression of human FTSJ1 can suppress the severe growth defect of S. cerevisiae Δtrm7 mutants (Pintard et al., 2002; Guy and Phizicky, 2015). Reduction of the modification level in tRNAPhe was reported in carcinoma and neuroblastoma in mice (Pergolizzi and Grunberger, 1980; Kuchino et al., 1982) and is associated with ID in human (see below).
FTSJ1
One of the best characterized associations between ID in human and mutations in a gene encoding for Nm is the one between non-syndromic X-linked ID (NSXLID) and mutations in the FTSJ1 gene (OMIM:300499) (Guy et al., 2015). One third of the X-linked ID (XLID) conditions are syndromic (S-XLID) and the other two thirds are non-syndromic (NS-XLID) (Lubs et al., 2012). NSXLID is associated with no obvious and consistent phenotype other than mental retardation (IQ < 70), indeed NSXLID disorders are clinically diverse and genetically heterogeneous. FTSJ1 loss of function causes NSXLID retardation in males (Froyen et al., 2007; Takano et al., 2008). Heterozygous loss of function mutations in females do not cause the disease, which is probably due to inactivation of the affected X chromosome. Several alleles of FTSJ1 from six independent families correlate with NSXLID. All of these alleles lead to a reduction in mRNA levels and/or protein function (Willems et al., 1993; Hamel et al., 1999; Freude et al., 2004; Ramser et al., 2004; Froyen et al., 2007; Takano et al., 2008; Guy et al., 2015; Table 1). Consistently with the 2′-O-methyltransferase activity of FTSJ1 on tRNAs, Guy and Phizicky reported that two genetically independent lymphoblastoid cell lines (LCLs) of NSXLID patients with FTSJ1 loss of function mutations nearly completely lack Cm32 and Gm34 on tRNAPhe (Guy et al., 2015). Additionally, tRNAPhe from a patient carrying an FTSJ1-p.A26P missense allele specifically lacks Gm34, but has normal levels of Cm32. tRNAPhe from the corresponding Saccharomyces cerevisiae TRM7-A26P mutant also specifically lacks Gm34. Altogether, these findings strongly suggest that the absence of Gm34, but not Cm32 modification on tRNAPhe causes NSXLID in patients carrying distinct FTSJ1 alleles. Nevertheless, the molecular consequences arising from the loss of this 2′-O-methylation are not yet determined. Furthermore, it is noteworthy to mention two additional studies involving families from the Chinese Han population (Dai et al., 2008; Gong et al., 2008), where three single nucleotide polymorphisms (SNPs) in the FTSJ1 gene were analyzed. Authors found a positive association with occurrence of NSXLID (Dai et al., 2008) as well as with general cognitive ability, verbal comprehension, and perceptual organization in male individuals (Gong et al., 2008). Although it seems tempting to link the variance of FTSJ1 gene to general human cognitive ability, more profound studies are needed to support this idea.
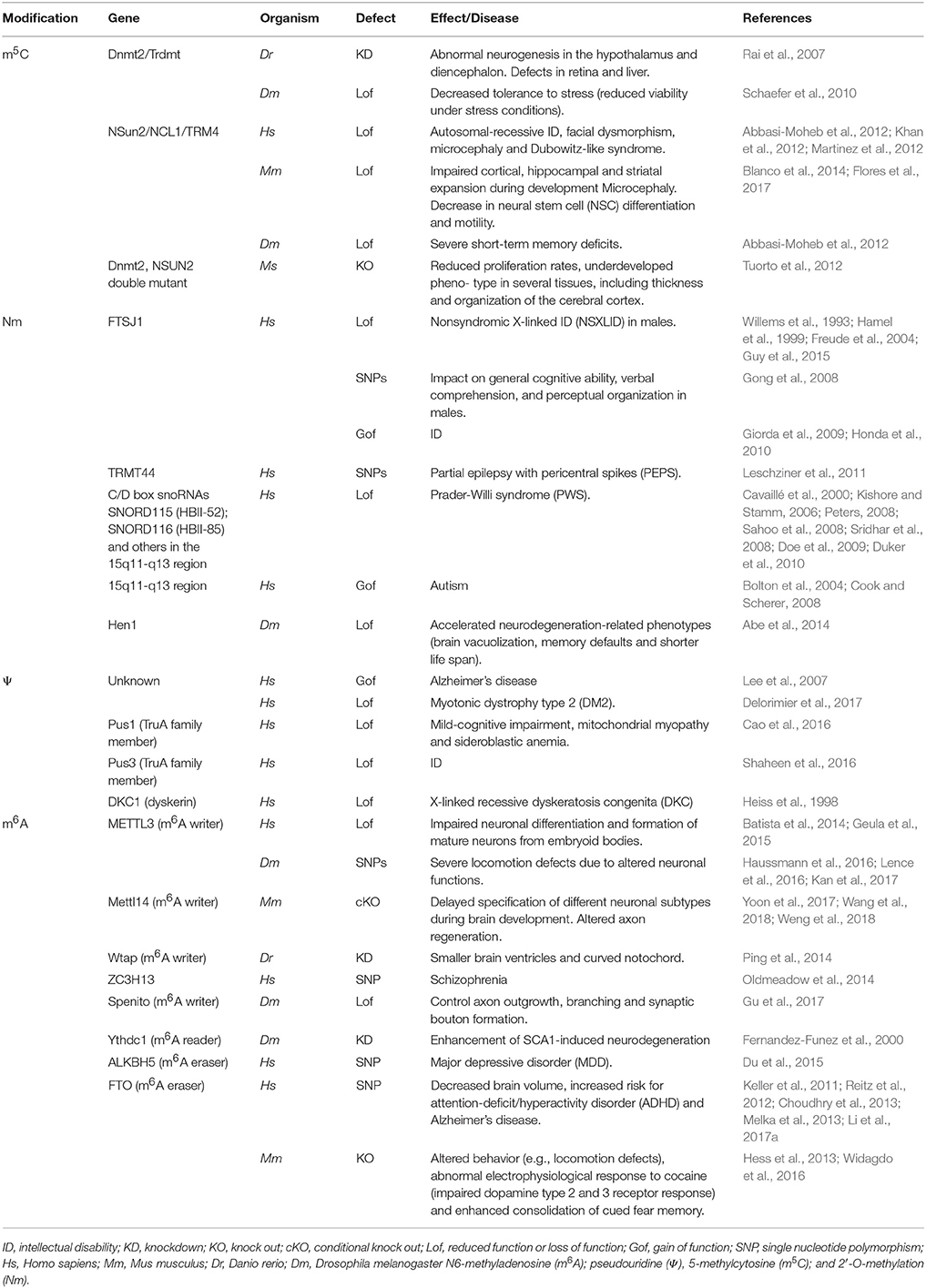
Table 1. Proteins required for writing, reading, or removal of different RNA modifications and their mutations associated with altered brain functions.
TRMT44
TRMT44 is a putative 2′-O-methyluridine methyltransferase predicted to methylate residue 44 in tRNASer (Leschziner et al., 2011). Mutations in this gene were identified as a causative mutation in partial epilepsy with pericentral spikes (PEPS), a novel mendelian idiopathic epilepsy (Leschziner et al., 2011). However, the underlying mechanisms are currently unknown.
Small Nucleolar RNAs (snoRNAs)
snoRNAs are a class of regulatory RNAs responsible for post-transcriptional modification of ribosomal RNAs (rRNAs). Two families of snoRNAs have been described, based on their structure and function: C/D box snoRNAs are responsible for 2′-O-methylation (Cavaillé et al., 1996), whereas H/ACA box snoRNAs mediate pseudouridylation (Ganot et al., 1997 and see the following chapter in this review). In zebrafish, loss of three snoRNAs results in impaired rRNA modifications, causing severe developmental defects including growth delay and deformations in the head region (Higa-Nakamine et al., 2012). In human, C/D box snoRNAs have been implicated in Prader-Willi syndrome (PWS), a complex neurological disease characterized with mental retardation, low height, obesity, and muscle hypotonia (Sridhar et al., 2008; Doe et al., 2009). In several independent studies, PWS was shown to be caused by the loss of imprinted snoRNAs in locus 15q11-q13. Large deletions of this region underlie about 70% of cases of PWS (Peters, 2008), whereas duplication of the same region is associated with autism (Belmonte et al., 2004; Bolton et al., 2004; Cook and Scherer, 2008). Locus 15q11–q13 contains numerous copies of two C/D box snoRNAs—SNORD115 (HBII-52), and SNORD116 (HBII-85) (Cavaillé et al., 2000). SNORD115 is believed to play key roles in the fine-tuning of serotonin receptor (5-HT2C) by influencing its pre-mRNA splicing (Vitali et al., 2005; Kishore and Stamm, 2006; Falaleeva et al., 2017), whereas SNORD116 loss is thought to contribute to the etiology of the PWS (Cavaillé et al., 2000; Sahoo et al., 2008; Duker et al., 2010).
Hen1/Pimet
Hen1/Pimet is a conserved enzyme, which adds 2′-O-methyl group to 3′-terminal nucleotides of miRNAs and siRNAs in plants, and of siRNAs and piRNAs in animals. Addition of this modification protects these small non-coding RNAs (sncRNAs) from 3′ → 5′ exonuclease degradation (Li et al., 2005; Horwich et al., 2007; Saito et al., 2007; Terrazas and Kool, 2009; Ross et al., 2014). In the absence of Hen1/Pimet, piRNA, and siRNA are destabilized and sncRNA silencing activities are compromised. Surprisingly, Hen1 mutant flies display neither increased lethality nor sterility under normal laboratory conditions but show however accelerated neurodegeneration (brain vacuolization), memory default, and shorter lifespan (Abe et al., 2014). This suggests a protective effect of Nm and small RNA pathways against age-associated neurodegenerative events. Accordingly, Drosophila lacking the siRNA effector, Argonaute 2 (Ago2), are viable but exhibit memory impairment and shortened lifespan (Li et al., 2013).
Pseudouridine (ψ)
Pseudouridine (also known as 5-ribosyluracil or ψ) is the first discovered (Cohn and Volkin, 1951) and most abundant RNA modification, present in a broad range of non-coding RNA, and was also recently detected in coding mRNA (Carlile et al., 2014; Lovejoy et al., 2014; Schwartz et al., 2014a; Li et al., 2015). The isomerization of uridine into ψ improves the base stacking in RNAs by the formation of additional hydrogen bonds, which influences RNA secondary structure and increases the stability of RNA duplexes (Arnez and Steitz, 1994; Davis, 1995). Pseudouridylation was shown to have a strong impact on different aspects of cellular processes, including translation efficiency, splicing, telomere maintenance, and the regulation of gene expression (Mochizuki et al., 2004; Carlile et al., 2014; Schwartz et al., 2014a). This base modification is catalyzed by pseudouridine synthases (Pus) that act on their substrates by two distinct mechanisms. One of those mechanisms is the guide RNA-dependent pseudouridylation, in which H/ACA box snoRNAs target RNAs for pseudouridylation via specific sequence interactions between the snoRNAs and the target RNA. A specific enzyme present in the snoRNP (sno-ribonucleoprotein) particule catalyzes the uridine modification (dyskerin in human, Cbf5 in yeast; Duan et al., 2009; Liang et al., 2009). Alternatively, RNA-independent pseudouridylation requires stand-alone pseudouridine synthases (Pus) that directly catalyze ψ formation at particular target RNA (Yu et al., 2011; Carlile et al., 2014; Rintala-Dempsey and Kothe, 2017). Each enzyme has a unique specificity for its target RNA and modifies uridine in a certain consensus sequence. Pus enzymes are present in all kingdoms of life, evolutionary conserved and are categorized into six families, based on their consensus sequences: TruA, TruB, TruD, RluA, and RsuA. The sixth family member, Pus10, is exclusive to eukaryotes and archaea (Koonin, 1996; Kaya and Ofengand, 2003; Fitzek et al., 2018).
Several pieces of evidence hint toward an implication of ψ in regulating neuronal functions. For instance, patients with mild-to-moderate severity of Alzheimer's disease show significantly elevated levels of urinal ψ (Lee et al., 2007) but it is currently unknown whether there is a link between this increase and the Alzheimer's disease etiology. Furthermore, it has been suggested that pseudouridylation can serve as a direct indicator of oxidative stress, which in turn has been linked to an increasing risk of neurodegeneration (Roth et al., 1999; Uttara et al., 2009). Accordingly, in cells exposed to acute oxidative stress by H2O2 treatment, Li and colleagues detected an elevation by ~40–50% in mRNA ψ levels, demonstrating that mRNA pseudouridylation acts as a direct response to cellular stress (Li et al., 2015).
A recent report demonstrated a direct implication of ψ in neuronal disorders from patients with myotonic dystrophy type 2 (DM2) (Delorimier et al., 2017). DM2 is a neuromuscular disease characterized by severe gray matter changes, including neuronal loss and global neuronal impairment (Minnerop et al., 2011; Meola and Cardani, 2015). DM2 patients have an increased binding of Muscleblind-like 1 protein (MBNL1) to CCUG repeats in an intron of the CNBP gene (Cho and Tapscott, 2007). Interestingly, it was recently reported that pseudouridylation within CCUG repeats reduces RNA flexibility and thus modestly inhibits MBNL1 binding (Delorimier et al., 2017). Similarly, ψ modification of a minimally structured model RNA resulted in an even more drastic reduction of MBNL1 binding to CCUG repeats. This study shows that ψ can reduce the disease-causing binding of MBNL1 at extended CCUG repeats and offers a basis for future research in treating neurodegenerative diseases.
Pus1
Pus1 is a member of the TruA family that typically pseudouridylates tRNA but was also recently found to act on rRNA, snRNA, and mRNA (Schwartz et al., 2014a; Carlile et al., 2015). Mutations of Pus1 in human lead to mitochondrial myopathy and sideroblastic anemia (Bykhovskaya et al., 2004; Fernandez-Vizarra et al., 2007; Bergmann et al., 2010). Recently, a mild cognitive impairment was also characterized in a long-surviving patient with two novel Pus1 mutations (Cao et al., 2016). A different study demonstrated a Pus1-dependent pseudouridylation of the steroid RNA activator (SRA). Pseudouridylated SRA acts as a co-activator of the nuclear estrogen receptor α (ERα) (Zhao et al., 2004; Leygue, 2007). Given that ERα was shown to regulate neuronal survival (Gamerdinger et al., 2006; Foster, 2012), it is conceivable that one of the functions of Pus1 in brain activity is mediated via the control of the ER pathway.
Pus3
Pus3 is another member of the TruA family, which has a strong sequence homology to Pus1 but acts on distinct target RNA. In situ hybridization showed accumulation of Pus3 mRNA in the nervous system of mice embryos, suggesting a role of Pus3 in neural development (Diez-Roux et al., 2011). Accordingly, a truncated form of Pus3 accompanied by reduced levels of ψ U39 in tRNA was detected in patients with ID (Shaheen et al., 2016). Taken together, the discovery of impaired cognition caused by mutations of TruA enzymes emphasizes their importance in neuronal development and maintenance regulation.
Dyskerin and RluA-1
The pseudouridine synthase dyskerin is essential for the H/ACA-box mediated pseudouridylation in human (Heiss et al., 1998; Lafontaine et al., 1998). Mutations of the dyskerin-encoding gene DKC1 causes X-linked recessive dyskeratosis congenita (DKC), a rare progressive congenital disorder that mostly affects highly regenerative tissues, such as the skin and bone marrow (Heiss et al., 1998; Mochizuki et al., 2004). Cells of the affected patients have decreased telomerase activity and thus reduced telomere length, which may be responsible for the disease (Mitchell et al., 1999). Interestingly, expression analysis of Dyskerin 1 showed a high level in embryonic neural tissue, as well as in specific subsets of neurons in the cerebellum and olfactory bulb of adult brains (Heiss et al., 2000). While the function of dyskerin in hematopoiesis has been studied intensively, we are yet lacking a detailed understanding about its potential nervous system function in adult brains. In Drosophila melanogaster, RluA enzymes modify uridines in rRNA and tRNA. In situ hybridization in embryos revealed a specific RluA-1 mRNA localization to dendrites of a subset of peripheral neurons, which also raises the question about the molecular function of RluA-1 and its target RNA in the peripheral nervous system during embryonic development (Wang et al., 2011).
N6-Methyladenosine (m6A)
m6A is an abundant mRNA modification that regulates nearly all aspects of mRNA processing including splicing, export, translation, stability, and decay (Meyer and Jaffrey, 2017; Roignant and Soller, 2017; Roundtree et al., 2017). This modification is catalyzed by a stable protein complex composed of two methyltransferases, Methyltransferase like-3 (Mettl3) and Methyltransferase like-14 (Mettl14) (Sledz and Jinek, 2016; Wang et al., 2016a,b; Schöller et al., 2018). Additional proteins required for m6A deposition are Wilms‘ tumor 1-associating protein (Wtap) (Liu et al., 2014; Ping et al., 2014; Wang et al., 2014), Vir like m6A methyltransferase associated (Virma) (Schwartz et al., 2014b; Yue et al., 2018), Zinc finger CCCH domain-containing protein 13 (Zc3h13) (Guo et al., 2018; Knuckles et al., 2018; Wen et al., 2018), RNA binding protein 15 (Rbm15) and its paralog Rbm15B (Patil et al., 2016). Mettl3 has the catalytic activity and can accommodate the SAM substrate, while Mettl14 serves to stabilize the binding to RNA (Sledz and Jinek, 2016; Wang et al., 2016a,b; Schöller et al., 2018). In vertebrates, m6A modification is dynamically regulated and can be reversed by two demethylases belonging to the family of α-ketoglutarate dependent dioxygenases, Fat mass and obesity associated protein (FTO) and ALKBH5 (Jia et al., 2011; Zheng et al., 2013). Recent advances in techniques to map m6A modification in a transcriptome wide manner enabled identification of thousands of modified mRNAs and lncRNAs (Dominissini et al., 2012; Meyer et al., 2012). While m6A has been involved in many physiological processes, increasing evidence suggests an importance of m6A modification in brain development and in the function of the nervous system.
m6A Writer Complex
m6A levels are particularly high in the nervous system, as shown in the developing mouse brain (Meyer et al., 2012), and in heads of adult flies (Lence et al., 2016). Furthermore, a recent study detected higher m6A content in the mouse cerebellum and in neurons compared to glia (Chang et al., 2017). Using in situ hybridization in zebrafish embryos, Ping et al. showed that Wtap is ubiquitously expressed at 36 h post-fertilization with enrichment in the brain region (Ping et al., 2014). Consistently, Wtap depletion using morpholino treatment resulted in severe developmental defects, including appearance of smaller brain ventricles and curved notochord at 24 h post-fertilization. Importance of m6A during neuronal development was further demonstrated by depletion of METTL3 in human embryonic stem cells (hESC), which strongly impaired neuronal differentiation (Batista et al., 2014), as well as the formation of mature neurons from embryoid bodies (Geula et al., 2015). Notably, m6A mRNA modification is essential for mouse survival as mice lacking Mettl3 die at E6.5 (Geula et al., 2015). However, two recent studies performed a conditional KO (cKO) of Mettl14 specifically in neurons and revealed an essential role of m6A in embryonic cortical neurogenesis (Yoon et al., 2017; Wang et al., 2018). Mettl14 cKO animals showed a decreased NSC proliferation and premature differentiation of NSCs (Wang et al., 2018), as well as delayed specification of different neuronal subtypes during brain development (Yoon et al., 2017). Yoon at al. further demonstrated that m6A modification is required for timely decay of transcripts involved in stem cell maintenance and cell cycle regulation in cortical neuronal progenitors. This allows accurate progression of the cell cycle and in turn induces the spatiotemporal formation of different neuronal subtypes. Interestingly, the authors also observed that many transcripts linked to mental disorders (autism, schizophrenia) are m6A modified in human, but not in mouse cultures of neuronal progenitor cells (NPC), raising the possibility that m6A regulates specifically these human diseases (Yoon et al., 2017). Consistent with this hypothesis, polymorphisms in ZC3H13 have been associated with schizophrenia (Oldmeadow et al., 2014).
Beyond the role in neuronal development, m6A modification also plays a critical role in the process of axon regeneration in mature mouse neurons (Weng et al., 2018). Weng et al. showed that upon peripheral nerve injury m6A levels of many transcripts in dorsal root ganglion (DRG) were elevated, which led to increased translation during the time of axon regeneration, via the specific m6A reader protein Ythdf1. Mettl14 and Ythdf1 conditional KO mice displayed strong reduction of sensory axon regeneration, resulting from reduced protein synthesis, revealing the critical role of m6A modification in response to injury (Weng et al., 2018).
In Drosophila, loss of components of the methyltransferase complex results in severe locomotion defects due to altered neuronal functions (Haussmann et al., 2016; Lence et al., 2016; Kan et al., 2017). Mettl3 mutants display alterations in walking speed and orientation, which can be rescued by ectopic expression of Mettl3 cDNA in neurons. Whether a particular subset of neurons is responsible for the observed alterations awaits further investigations. Interestingly, another member of the m6A methyltransferase complex, Nito (RBM15 in human), was recently shown to control axon outgrowth, branching and to regulate synaptic bouton formation via the activity of the CCAP/bursicon neurons (Gu et al., 2017), providing first insights toward addressing this question.
m6A Readers
As mentioned above, most characterized functions of m6A rely on the direct binding of the so-called m6A “reader” proteins to the modified site. The best-studied m6A readers are the YTH-domain containing proteins, which can specifically bind m6A via their YTH domain (Luo and Tong, 2014; Theler et al., 2014; Xu et al., 2014). RNA in situ hybridization of rat brain sections showed that one particular member of the YTH family, Ythdc1, is enriched in specific cells in the brain (Hartmann et al., 1999). Interestingly, in a yeast two-hybrid screen to identify Ythdc1-interacting proteins, libraries from P5 and E16 brains were screened and the rat homolog of Sam68 was found as the main interactor (Hartmann et al., 1999). Sam68 is known to regulate neuronal activity-dependent alternative splicing events (i.e., of neurexin-1) (Iijima et al., 2011). In line with this function, in situ hybridization assays showed that Drosophila Ythdc1 localizes in the ventral neurectoderm and central nervous system of Drosophila embryos (Lence et al., 2016) and a reduced level of Ythdc1 was found to enhance SCA1-induced neurodegeneration (Fernandez-Funez et al., 2000).
Apart from the YTH-protein family of conventional m6A reader proteins, a number of other proteins that bind RNA in m6A-dependent fashion has been recently identified (Edupuganti et al., 2017). Among them, Fragile X mental retardation protein (FMRP, also known as POF; FMR1; POF1; FRAXA) was shown to preferentially bind an RNA probe containing m6A sites (Edupuganti et al., 2017). FMRP plays critical roles in synaptic plasticity and neuronal development. Its loss of function in human leads to the Fragile X syndrome, which is the most prevalent form of inherited ID and the foremost monogenic cause of autism (Bardoni et al., 2001; Lubs et al., 2012; Wang et al., 2012; Hagerman and Polussa, 2015). FMRP has a central role in neuronal development and synaptic plasticity through the regulation of alternative mRNA splicing, mRNA stability, mRNA dendritic transport and postsynaptic local protein synthesis of a subset of mRNAs (Antar et al., 2006; Didiot et al., 2008; Bechara et al., 2009; Ascano et al., 2012; Guo et al., 2015). Moreover, it represses mRNA translation during the transport of dendritic mRNAs to postsynaptic dendritic spines and activates mRNA translation of a subset of dendritic mRNAs at synapses (Bechara et al., 2009; Fähling et al., 2009). Consistent with a potential interplay between FMRP and m6A, a recent study found that m6A is present on many synaptic mRNAs that are known targets of FMRP protein (Chang et al., 2017). Future research will seek to further illuminate the potential role of FMRP within the m6A pathway. It is interesting to note that Nm also appears to contribute to FMRP-mediated translation regulation at synapses. FMRP can form a complex with the non-coding RNA, brain cytoplasmic RNA (BC1), to repress translation of a subset of FMRP target mRNAs (Zalfa et al., 2003). This interaction is modulated by the Nm status of BC1 RNA. In both nucleus and cytoplasm in the cell body, Nm is present on BC1, but it is virtually absent at synapses (Lacoux et al., 2012). The authors suggested that changes in the 2′-O-methylation status of BC1 RNA contribute to the fine-tuned regulation of gene expression at synapses and consequently to neuronal plasticity by influencing FMRP local translational control. This example supports a likely combinatorial role of RNA modifications in the regulation of similar targets and/or processes during brain function.
m6A Erasers
Two proteins in humans were reported to act as m6A erasers: (FTO) and AlkB homolog 5 (ALKBH5) (Jia et al., 2011; Zheng et al., 2013). Both belong to the family of Fe2+-α-ketoglutarate-dependent deoxygenases and catalyze the removal of the methyl group of m6A by oxidation. Interestingly, even though ALKBH5 is only moderately expressed in the brain, it has been associated with mental disorders. Du et al. found that certain polymorphisms within the ALKBH5 gene correlate with the major depressive disorder (MDD), suggesting an involvement of ALKBH5 in conferring risk of MDD (Du et al., 2015).
In comparison, FTO is highly expressed in the human brain, especially in the hypothalamus and the pituitary gland and displays dynamic expression during postnatal neurodevelopment. Polymorphic alleles of FTO in human were identified to increase the risk for hyperactive disorder (Choudhry et al., 2013), for Alzheimer's disease (Keller et al., 2011; Reitz et al., 2012) and to affect the brain volume (Melka et al., 2013; Li et al., 2017a). Molecular and functional studies have shown that FTO knockout mice display altered behavior, including locomotion defects, but also influences learning and memory. For instance, fear conditioned mice showed a significant increase in m6A intensity on several neuronal targets, and knockdown of FTO further enhanced consolidation of cued fear memory (Widagdo et al., 2016). In line with this finding, FTO deficiency reduces the proliferation and neuronal differentiation of adult NSCs, which leads to impaired learning and memory (Li et al., 2017a). Electrophysiological tests in FTO knockout mice demonstrated an impaired dopamine type 2 and 3 receptor response, resulting in an abnormal response to cocaine (Hess et al., 2013). Strikingly, a recent study in mouse embryonic dorsal root ganglia found that FTO is enriched and specifically expressed in axons, influencing translation of axonal mRNAs (Yu et al., 2018). This demonstrates the dynamic role of m6A modification in regulating local translation. However, it is important to stress that FTO was also demonstrated to additionally demethylate m6Am, which is present next to the 7mG cap modification (Mauer et al., 2017). In comparison to m6A, m6Am also contains a methyl group on the ribose. As available antibodies recognize both modifications indistinguishably, it is currently difficult to assign their respective contribution in the context of brain activity.
Conclusion
The association of aberrant RNA modifications with various neurological disorders highlights the importance of these chemical moieties for proper brain development and cognition. However, today the role of RNA modifications in these processes is not completely understood. One of the current challenges lies in identifying the class and identity of RNAs that are targeted by RNA modification enzymes and are causative of the neurological defects. Common targets for many of these enzymes are tRNAs and rRNAs, thus it is likely that in many cases their dysfunction plays an important role in the etiology of the disease. Yet, this does not explain why the phenotypes observed upon mutations of these enzymes are often restricted to the brain. Some of these enzymes or snoRNAs are predominantly expressed in the nervous system, which indicates their importance in this tissue and suggests the existence of differentially modified ribosomes (also recently called specialized ribosomes) that may carry distinct functions (Briggs and Dinman, 2017; Sloan et al., 2017). However, other snoRNA or enzymes exhibit wider tissue distribution, suggesting that brain specific phenotypes may reflect a higher sensitivity to altered translation in this tissue compared to others organs. This would be consistent with other disease-causing mutations in ribosomal proteins or tRNA synthetase genes that also manifest their effect specifically in the nervous system (Antonellis et al., 2003; Antonellis and Green, 2008; Yao and Fox, 2013; Brooks et al., 2014). What could be the reason(s) behind this increased sensitivity? It has been observed that tRNA level is in general higher in the brain compared to other tissues, suggestive of a bigger translational demand (Dittmar et al., 2006). This neuronal specificity may arise from the local translation that occurs at synapses upon environmental changes. In this context, RNA modifications represent an attractive system to regulate this acute need in a dynamic and flexible manner. However, other examples point toward a translation-independent mechanism. For instance, in the case of FTSJ1 mutations and the associated NSXLID, neither the amount, nor the charging of the concerned tRNAs appear to be affected (Guy et al., 2012, 2015), seemingly ruling out a general translation defect. Perhaps in this case, the absence of modification can stimulate tRNA cleavage and generate tRFs that can also interfere with translation. This increase in tRFs would not necessarily be associated with a corresponding reduction of the uncleaved tRNA since tRNA levels are tightly regulated (Wilusz, 2015). Alternatively, the absence of modification on the tRNA may affect its interaction with the ribosome and thus influence translation efficiency or fidelity. Therefore, careful examination at different molecular levels is required to appreciate the effect of tRNA and rRNA modification enzyme mutations on translation and their consequence on the neurological phenotype.
That being said, the general picture is probably more complex. For instance, recent reports show that tRFs not only interfere with translation but can also affect transposon regulation and genome stability (Durdevic et al., 2013; Martinez et al., 2017; Schorn et al., 2017; Zhang et al., 2017). This activity could in principle also contribute to neurological disorders as growing evidence suggests associations between (re)expression of transposable elements and the occurrence of neuropathies (Perrat et al., 2013; Krug et al., 2017; Zahn, 2017; Jacob-Hirsch et al., 2018). In addition, beyond tRNA and tRF, the recent studies on m6A clearly demonstrate the involvement of mRNA modification in different aspects of neuronal development and regulation. The large diversity of RNA processing events in the brain, including the high rates of alternative splicing and recursive splicing (Duff et al., 2015; Sibley et al., 2015), the inclusion of microexons (Irimia et al., 2014) and the biogenesis of circular RNAs (cirRNAs) can all in principle be affected by RNA modifications. For instance, m6A modification was recently found on circRNAs, and enables their translation (Yang et al., 2017; Zhou et al., 2017). Given that misexpression of circRNAs has been associated with neurological disorders (Shao and Chen, 2016; van Rossum et al., 2016; Li et al., 2017b), some of m6A brain functions may rely on circRNA-mediated regulation. Thus, because neurons face distinct challenges with regards to localisation of RNAs to distal processes and to localized translation it is likely that in the brain, more than in any other tissues, a combinatorial effect of RNA modifications on different classes of RNAs represents a critical informational layer that dynamically fine-tunes gene regulation (Figure 2). Exciting discoveries are lying ahead for deciphering this intricate epitranscriptomics code.
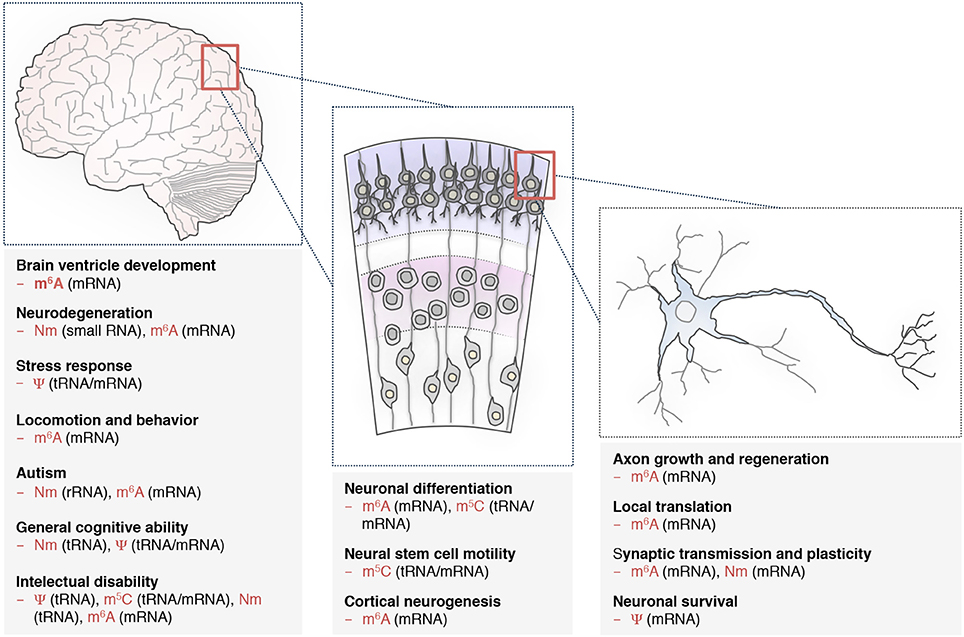
Figure 2. RNA modifications are implicated in various neuronal processes. Distinct RNA modifications of tRNAs, small RNAs and mRNAs are required for common biological processes during brain development (left), neuronal differentiation (middle), and proper functioning of individual neuron (right), (see also Table 1). N6-methyladenosine (m6A), pseudouridine (Ψ), 5-methylcytosine (m5C), and 2′-O-methylation (Nm). The RNA classes in the brackets are the ones studied so far. Additional types with important functions may be modified as well.
Author Contributions
All authors listed have made a substantial, direct and intellectual contribution to the work, and approved it for publication.
Funding
The work presented was supported by DFG (RO4681/5-1), the DIP (RO4681/6-1) to J-YR, by the IBPS Actions Incitatives 2018 to CC, the French MESR to DD and an ARC Ph.D. fellowship to MA. CC acknowledges the Réseau André Picard for its financial support. J-YR and CC are members of the European Epitranscriptomic COST Action (CA16120).
Conflict of Interest Statement
The authors declare that the research was conducted in the absence of any commercial or financial relationships that could be construed as a potential conflict of interest.
References
Abbasi-Moheb, L., Mertel, S., Gonsior, M., Nouri-Vahid, L., Kahrizi, K., Cirak, S., et al. (2012). Mutations in NSUN2 cause autosomal-recessive intellectual disability. Am. J. Hum. Genet. 90, 847–855. doi: 10.1016/j.ajhg.2012.03.021
Abe, M., Naqvi, A., Hendriks, G. J., Feltzin, V., Zhu, Y., Grigoriev, A., et al. (2014). Impact of age-associated increase in 2′-O-methylation of miRNAs on aging and neurodegeneration in Drosophila. Genes Dev. 28, 44–57. doi: 10.1101/gad.226654.113
Agris, P. F. (2008). Bringing order to translation, the contributions of transfer RNA anticodon-domain modifications. EMBO Rep. 9, 629–635. doi: 10.1038/embor.2008.104
Antar, L. N., Li, C., Zhang, H., Carroll, R. C., and Bassell, G. J. (2006). Local functions for FMRP in axon growth cone motility and activity-dependent regulation of filopodia and spine synapses. Mol. Cell. Neurosci. 32, 37–48. doi: 10.1016/j.mcn.2006.02.001
Antonellis, A., and Green, E. D. (2008). The role of aminoacyl-tRNA synthetases in genetic diseases. Annu. Rev. Genomics Hum. Genet. 9, 87–107. doi: 10.1146/annurev.genom.9.081307.164204
Antonellis, A., Ellsworth, R. E., Sambuughin, N., Puls, I., Abel, A., Lee-Lin, S. Q., et al. (2003). Glycyl tRNA synthetase mutations in Charcot-Marie-Tooth disease type 2D and distal spinal muscular atrophy type V. Am. J. Hum. Genet. 72, 1293–1299. doi: 10.1086/375039
Arnez, J. G., and Steitz, T. A. (1994). Crystal-structure of unmodified Trna(Gln) complexed with glutaminyl-transfer-rna synthetase and atp suggests a possible role for pseudo-uridines in stabilization of Rna structure. Biochemistry 33, 7560–7567. doi: 10.1021/bi00190a008
Ascano, M., Mukherjee, N., Bandaru, P., Miller, J. B., Nusbaum, J. D., Corcoran, D. L., et al. (2012). FMRP targets distinct mRNA sequence elements to regulate protein expression. Nature 492, 382–386. doi: 10.1038/nature11737
Bardoni, B., Schenck, A., and Mandel, J. L. (2001). The fragile X mental retardation protein. Brain Res. Bull. 56, 375–382. doi: 10.1016/S0361-9230(01)00647-5
Batista, P. J. (2017). The RNA modification N(6)-methyladenosine and Its implications in human disease. Genomics Proteomics Bioinformatics 15, 154–163. doi: 10.1016/j.gpb.2017.03.002
Batista, P. J., Molinie, B., Wang, J., Qu, K., Zhang, J., Li, L., et al. (2014). m(6)A RNA modification controls cell fate transition in mammalian embryonic stem cells. Cell Stem Cell 15, 707–719. doi: 10.1016/j.stem.2014.09.019
Bechara, E. G., Didiot, M. C., Melko, M., Davidovic, L., Bensaid, M., Martin, P., et al. (2009). A novel function for fragile x mental retardation protein in translational activation. PLoS Biol. 7:e16. doi: 10.1371/journal.pbio.1000016
Bednárová, A., Hanna, M., Durham, I., VanCleave, T., England, A., Chaudhuri, A., et al. (2017). Lost in translation: defects in transfer rna modifications and neurological disorders. Front. Mol. Neurosci. 10:135. doi: 10.3389/fnmol.2017.00135
Belmonte, M. K., Cook, E. H., Anderson, G. M., Rubenstein, J. L. R., Greenough, W. T., Beckel-Mitchener, A., et al. (2004). Autism as a disorder of neural information processing: directions for research and targets for therapy. Mol. Psychiatry 9, 646–663. doi: 10.1038/sj.mp.4001499
Bergmann, A. K., Campagna, D. R., McLoughlin, E. M., Agarwal, S., Fleming, M. D., Bottomley, S. S., et al. (2010). Systematic molecular genetic analysis of congenital sideroblastic anemia: evidence for genetic heterogeneity and identification of novel mutations. Pediatr. Blood Cancer 54, 273–278. doi: 10.1002/pbc.22244
Bird, A. (2002). DNA methylation patterns and epigenetic memory. Genes Dev. 16, 6–21. doi: 10.1101/gad.947102
Blanco, S., Dietmann, S., Flores, J. V., Hussain, S., Kutter, C., Humphreys, P., et al. (2014). Aberrant methylation of tRNAs links cellular stress to neuro-developmental disorders. EMBO J. 33, 2020–2039. doi: 10.15252/embj.201489282
Blanco, S., Kurowski, A., Nichols, J., Watt, F. M., Benitah, S. A., and Frye, M. (2011). The RNA-methyltransferase Misu (NSun2) poises epidermal stem cells to differentiate. PLoS Genet. 7:e1002403. doi: 10.1371/journal.pgen.1002403
Bolton, P. F., Veltman, M. W., Weisblatt, E., Holmes, J. R., Thomas, N. S., Youings, S. A., et al. (2004). Chromosome 15q11-13 abnormalities and other medical conditions in individuals with autism spectrum disorders. Psychiatr. Genet 14, 131–137. doi: 10.1097/00041444-200409000-00002
Briggs, J. W., and Dinman, J. D. (2017). Subtractional heterogeneity: a crucial step toward defining specialized ribosomes. Mol. Cell 67, 3–4. doi: 10.1016/j.molcel.2017.06.022
Brooks, S. S., Wall, A. L., Golzio, C., Reid, D. W., Kondyles, A., Willer, J. R., et al. (2014). A novel ribosomopathy caused by dysfunction of RPL10 disrupts neurodevelopment and causes X-linked microcephaly in humans. Genetics 198, 723–733. doi: 10.1534/genetics.114.168211
Brzezicha, B., Schmidt, M., Makalowska, I., Jarmolowski, A., Pienkowska, J., and Szweykowska-Kulinska, Z. (2006). Identification of human tRNA:m5C methyltransferase catalysing intron-dependent m5C formation in the first position of the anticodon of the pre-tRNA Leu (CAA). Nucleic Acids Res. 34, 6034–6043. doi: 10.1093/nar/gkl765
Bykhovskaya, Y., Casas, K., Mengesha, E., Inbal, A., and Fischel-Ghodsian, N. (2004). Missense mutation in pseudouridine synthase 1 (PUS1) causes mitochondrial myopathy and sideroblastic anemia (MLASA). Am. J. Hum. Genet. 74, 1303–1308. doi: 10.1086/421530
Byszewska, M., Smietanski, M., Purta, E., and Bujnicki, J. M. (2014). RNA methyltransferases involved in 5' cap biosynthesis. RNA Biol. 11, 1597–1607. doi: 10.1080/15476286.2015.1004955
Cantara, W. A., Crain, P. F., Rozenski, J., McCloskey, J. A., Harris, K. A., Zhang, X., et al. (2011). The RNA modification database, RNAMDB: 2011 update. Nucleic Acids Res. 39, D195–D201. doi: 10.1093/nar/gkq1028
Cao, M., Dona, M., Valentino, M. L., Semplicini, C., Maresca, A., Cassina, M., et al. (2016). Clinical and molecular study in a long-surviving patient with MLASA syndrome due to novel PUS1 mutations. Neurogenetics 17, 143–143. doi: 10.1007/s10048-016-0475-3
Carlile, T. M., Rojas-Duran, M. F., and Gilbert, W. V. (2015). Transcriptome-wide identification of pseudouridine modifications using Pseudo-seq. Curr. Protoc. Mol. Biol. 112, 4. 25. 21-24. doi: 10.1002/0471142727.mb0425s112
Carlile, T. M., Rojas-Duran, M. F., Zinshteyn, B., Shin, H., Bartoli, K. M., and Gilbert, W. V. (2014). Pseudouridine profiling reveals regulated mRNA pseudouridylation in yeast and human cells. Nature 515, 143–146. doi: 10.1038/nature13802
Cavaillé, J., Buiting, K., Kiefmann, M., Lalande, M., Brannan, C. I., Horsthemke, B., et al. (2000). Identification of brain-specific and imprinted small nucleolar RNA genes exhibiting an unusual genomic organization. Proc. Natl. Acad. Sci. U.S.A. 97, 14311–14316. doi: 10.1073/pnas.250426397
Cavaillé, J., Nicoloso, M., and Bachellerie, J. P. (1996). Targeted ribose methylation of RNA in vivo directed by tailored antisense RNA guides. Nature 383, 732–735. doi: 10.1038/383732a0
Chang, M., Lv, H., Zhang, W., Ma, C., He, X., Zhao, S., et al. (2017). Region-specific RNA m(6)A methylation represents a new layer of control in the gene regulatory network in the mouse brain. Open Biol. 7:170166. doi: 10.1098/rsob.170166
Cho, D. H., and Tapscott, S. J. (2007). Myotonic dystrophy: emerging mechanisms for DM1 and DM2. Biochim Biophys. Acta 1772, 195–204. doi: 10.1016/j.bbadis.2006.05.013
Choudhry, Z., Sengupta, S. M., Grizenko, N., Thakur, G. A., Fortier, M. E., Schmitz, N., et al. (2013). Association between obesity-related gene FTO and ADHD. Obesity 21, E738–E744. doi: 10.1002/oby.20444
Cohn, W. E., and Volkin, E. (1951). Nucleoside-5′-phosphates from ribonucleic acid. Nature 167, 483–484. doi: 10.1038/167483a0
Cook, E. H., and Scherer, S. W. (2008). Copy-number variations associated with neuropsychiatric conditions. Nature 455, 919–923. doi: 10.1038/nature07458
Dai, D., Wang, H., Zhu, L., Jin, H., and Wang, X. (2018). N6-methyladenosine links RNA metabolism to cancer progression. Cell Death Dis. 9:124. doi: 10.1038/s41419-017-0129-x
Dai, L., Xing, L., Gong, P., Zhang, K., Gao, X., Zheng, Z., et al. (2008). Positive association of the FTSJ1 gene polymorphisms with nonsyndromic X-linked mental retardation in young Chinese male subjects. J. Hum. Genet. 53, 592–597. doi: 10.1007/s10038-008-0287-x
Dai, Q., Moshitch-Moshkovitz, S., Han, D., Kol, N., Amariglio, N., Rechavi, G., et al. (2017). Nm-seq maps 2'-O-methylation sites in human mRNA with base precision. Nat. Methods 14, 695–698. doi: 10.1038/nmeth.4294
Daily, D. K., Ardinger, H. H., and Holmes, G. E. (2000). Identification and evaluation of mental retardation. Am. Fam. Physician 61, 1059–1067. Available online at: https://www.aafp.org/afp/2000/0215/p1059.html
Darzacq, X., Jády, B. E., Verheggen, C., Kiss, A. M., Bertrand, E., and Kiss, T. (2002). Cajal body-specific small nuclear RNAs: a novel class of 2'-O-methylation and pseudouridylation guide RNAs. EMBO J. 21, 2746–2756. doi: 10.1093/emboj/21.11.2746
Davis, D. R. (1995). Stabilization of RNA stacking by pseudouridine. Nucleic Acids Res. 23, 5020–5026. doi: 10.1093/nar/23.24.5020
Delorimier, E., Hinman, M. N., Copperman, J., Datta, K., Guenza, M., and Berglund, J. A. (2017). Pseudouridine modification inhibits muscleblind-like 1 (MBNL1) binding to CCUG repeats and minimally structured RNA through reduced RNA flexibility. J. Biol. Chem. 292, 4350–4357. doi: 10.1074/jbc.M116.770768
Didiot, M. C., Tian, Z., Schaeffer, C., Subramanian, M., Mandel, J. L., and Moine, H. (2008). The G-quartet containing FMRP binding site in FMR1 mRNA is a potent exonic splicing enhancer. Nucleic Acids Res. 36, 4902–4912. doi: 10.1093/nar/gkn472
Diez-Roux, G., Banfi, S., Sultan, M., Geffers, L., Anand, S., Rozado, D., et al. (2011). A high-resolution anatomical atlas of the transcriptome in the mouse embryo. PLoS Biol. 9:e1000582. doi: 10.1371/journal.pbio.1000582
Dittmar, K. A., Goodenbour, J. M., and Pan, T. (2006). Tissue-specific differences in human transfer RNA expression. PLoS Genet. 2:e221. doi: 10.1371/journal.pgen.0020221
Doe, C. M., Relkovic, D., Garfield, A. S., Dalley, J. W., Theobald, D. E. H., Humby, T., et al. (2009). Loss of the imprinted snoRNA mbii-52 leads to increased 5htr2c pre-RNA editing and altered 5HT(2C)R-mediated behaviour. Hum. Mol. Genet. 18, 2140–2148. doi: 10.1093/hmg/ddp137
Dominissini, D., and Rechavi, G. (2017). Loud and clear epitranscriptomic m(1)A signals: now in single-base resolution. Mol. Cell 68, 825–826. doi: 10.1016/j.molcel.2017.11.029
Dominissini, D., Moshitch-Moshkovitz, S., Schwartz, S., Salmon-Divon, M., Ungar, L., Osenberg, S., et al. (2012). Topology of the human and mouse m6A RNA methylomes revealed by m6A-seq. Nature 485, 201–206. doi: 10.1038/nature11112
Dominissini, D., Nachtergaele, S., Moshitch-Moshkovitz, S., Peer, E., Kol, N., Ben-Haim, M. S., et al. (2016). The dynamic N(1)-methyladenosine methylome in eukaryotic messenger RNA. Nature 530, 441–446. doi: 10.1038/nature16998
Du, T., Rao, S., Wu, L., Ye, N., Liu, Z., Hu, H., et al. (2015). An association study of the m6A genes with major depressive disorder in Chinese Han population. J. Affect. Disord. 183, 279–286. doi: 10.1016/j.jad.2015.05.025
Duan, J., Li, L., Lu, J., Wang, W., and Ye, K. (2009). Structural mechanism of substrate RNA recruitment in H/ACA RNA-guided pseudouridine synthase. Mol. Cell 34, 427–439. doi: 10.1016/j.molcel.2009.05.005
Duff, M. O., Olson, S., Wei, X., Garrett, S. C., Osman, A., Bolisetty, M., et al. (2015). Genome-wide identification of zero nucleotide recursive splicing in Drosophila. Nature 521, 376–379. doi: 10.1038/nature14475
Duker, A. L., Ballif, B. C., Bawle, E. V., Person, R. E., Mahadevan, S., Alliman, S., et al. (2010). Paternally inherited microdeletion at 15q11.2 confirms a significant role for the SNORD116 C/D box snoRNA cluster in Prader-Willi syndrome. Eur. J. Hum. Genet. 18, 1196–1201. doi: 10.1038/ejhg.2010.102
Durdevic, Z., Mobin, M. B., Hanna, K., Lyko, F., and Schaefer, M. (2013). The RNA methyltransferase Dnmt2 is required for efficient Dicer-2-dependent siRNA pathway activity in Drosophila. Cell Rep. 4, 931–937. doi: 10.1016/j.celrep.2013.07.046
Edupuganti, R. R., Geiger, S., Lindeboom, R. G. H., Shi, H., Hsu, P. J., Lu, Z., et al. (2017). N(6)-methyladenosine (m(6)A) recruits and repels proteins to regulate mRNA homeostasis. Nat. Struct. Mol. Biol. 24, 870–878. doi: 10.1038/nsmb.3462
Fähling, M., Mrowka, R., Steege, A., Kirschner, K. M., Benko, E., Förstera, B., et al. (2009). Translational regulation of the human achaete-scute homologue-1 by fragile X mental retardation protein. J. Biol. Chem. 284, 4255–4266. doi: 10.1074/jbc.M807354200
Falaleeva, M., Welden, J. R., Duncan, M. J., and Stamm, S. (2017). C/D-box snoRNAs form methylating and non-methylating ribonucleoprotein complexes: old dogs show new tricks. BioEssays 39:1600264. doi: 10.1002/bies.201600264
Fernandez-Funez, P., Nino-Rosales, M. L., de Gouyon, B., She, W. C., Luchak, J. M., Martinez, P., et al. (2000). Identification of genes that modify ataxin-1-induced neurodegeneration. Nature 408, 101–106. doi: 10.1038/35040584
Fernandez-Vizarra, E., Berardinelli, A., Valente, L., Tiranti, V., and Zeviani, M. (2007). Nonsense mutation in pseudouridylate synthase 1 (PUS1) in two brothers affected by myopathy, lactic acidosis and sideroblastic anaemia (MLASA). J. Med. Genet. 44, 173–180. doi: 10.1136/jmg.2006.045252
Fisher, O., Siman-Tov, R., and Ankri, S. (2006). Pleiotropic phenotype in Entamoeba histolytica overexpressing DNA methyltransferase (Ehmeth). Mol. Biochem. Parasitol. 147, 48–54. doi: 10.1016/j.molbiopara.2006.01.007
Fitzek, E., Joardar, A., Gupta, R., and Geisler, M. (2018). Evolution of eukaryal and archaeal pseudouridine synthase pus10. J. Mol. Evol. 86, 77–89. doi: 10.1007/s00239-018-9827-y
Flores, J. V., Cordero-Espinoza, L., Oeztuerk-Winder, F., Andersson-Rolf, A., Selmi, T., Blanco, S., et al. (2017). Cytosine-5 RNA methylation regulates neural stem cell differentiation and motility. Stem Cell Rep. 8, 112–124. doi: 10.1016/j.stemcr.2016.11.014
Foster, T. C. (2012). Role of estrogen receptor alpha and beta expression and signaling on cognitive function during aging. Hippocampus 22, 656–669. doi: 10.1002/hipo.20935
Franke, B., Vermeulen, S. H., Steegers-Theunissen, R. P., Coenen, M. J., Schijvenaars, M. M., Scheffer, H., et al. (2009). An association study of 45 folate-related genes in spina bifida: Involvement of cubilin (CUBN) and tRNA aspartic acid methyltransferase 1 (TRDMT1). Birth Defects Res. A Clin. Mol. Teratol. 85, 216–226. doi: 10.1002/bdra.20556
Freude, K., Hoffmann, K., Jensen, L. R., Delatycki, M. B., des Portes, V., Moser, B., et al. (2004). Mutations in the FTSJ1 gene coding for a novel S-adenosylmethionine- binding protein cause nonsyndromic X-linked mental retardation. Am. J. Hum. Genet. 75, 305–309. doi: 10.1086/422507
Froyen, G., Bauters, M., Boyle, J., Van Esch, H., Govaerts, K., van Bokhoven, H., et al. (2007). Loss of SLC38A5 and FTSJ1 at Xp11.23 in three brothers with non-syndromic mental retardation due to a microdeletion in an unstable genomic region. Hum. Genet. 121, 539–547. doi: 10.1007/s00439-007-0343-1
Gamerdinger, M., Manthey, D., and Behl, C. (2006). Oestrogen receptor subtype-specific repression of calpain expression and calpain enzymatic activity in neuronal cells - implications for neuroprotection against Ca2+−mediated excitotoxicity. J. Neurochem. 97, 57–68. doi: 10.1111/j.1471-4159.2006.03675.x
Ganot, P., Bortolin, M. L., and Kiss, T. (1997). Site-specific pseudouridine formation in preribosomal RNA is guided by small nucleolar RNAs. Cell 89, 799–809. doi: 10.1016/S0092-8674(00)80263-9
Genenncher, B., Durdevic, Z., Hanna, K., Zinkl, D., Mobin, M. B., Senturk, N., et al. (2018). Mutations in cytosine-5 tRNA methyltransferases impact mobile element expression and genome stability at specific DNA repeats. Cell Rep. 22, 1861–1874. doi: 10.1016/j.celrep.2018.01.061
Geula, S., Moshitch-Moshkovitz, S., Dominissini, D., Mansour, A. A., Kol, N., Salmon-Divon, M., et al. (2015). m(6)A mRNA methylation facilitates resolution of naive pluripotency toward differentiation. Science 347, 1002–1006. doi: 10.1126/science.1261417
Giorda, R., Bonaglia, M. C., Beri, S., Fichera, M., Novara, F., Magini, P., et al. (2009). Complex segmental duplications mediate a recurrent dup(X)(p11.22-p11.23) associated with mental retardation, speech delay, and EEG anomalies in males and females. Am. J. Hum. Genet. 85, 394–400. doi: 10.1016/j.ajhg.2009.08.001
Goll, M. G., and Bestor, T. H. (2005). Eukaryotic cytosine methyltransferases. Annu. Rev. Biochem. 74, 481–514. doi: 10.1146/annurev.biochem.74.010904.153721
Goll, M. G., Kirpekar, F., Maggert, K. A., Yoder, J. A., Hsieh, C. L., Zhang, X., et al. (2006). Methylation of tRNAAsp by the DNA methyltransferase homolog Dnmt2. Science 311, 395–398. doi: 10.1126/science.1120976
Gong, P., Li, J., Dai, L., Zhang, K., Zheng, Z., Gao, X., et al. (2008). Genetic variations in FTSJ1 influence cognitive ability in young males in the chinese han population. J. Neurogenet. 22, 277–287. doi: 10.1080/01677060802337299
Gu, T., Zhao, T., Kohli, U., and Hewes, R. S. (2017). The large and small SPEN family proteins stimulate axon outgrowth during neurosecretory cell remodeling in Drosophila. Dev. Biol. 431, 226–238. doi: 10.1016/j.ydbio.2017.09.013
Guo, J., Tang, H. W., Li, J., Perrimon, N., and Yan, D. (2018). Xio is a component of the Drosophila sex determination pathway and RNA N6-methyladenosine methyltransferase complex. Proc. Natl. Acad. Sci. U.S.A. 115, 3674–3679. doi: 10.1073/pnas.1720945115
Guo, M., and Schimmel, P. (2013). Essential nontranslational functions of tRNA synthetases. Nat. Chem. Biol. 9, 145–153. doi: 10.1038/nchembio.1158
Guo, W., Polich, E. D., Su, J., Gao, Y., Christopher, D. M., Allan, A. M., et al. (2015). Fragile X proteins FMRP and FXR2P control synaptic GluA1 expression and neuronal maturation via distinct mechanisms. Cell Rep. 11, 1651–1666. doi: 10.1016/j.celrep.2015.05.013
Guy, M. P., and Phizicky, E. M. (2015). Conservation of an intricate circuit for crucial modifications of the tRNAPhe anticodon loop in eukaryotes. RNA 21, 61–74. doi: 10.1261/rna.047639.114
Guy, M. P., Podyma, B. M., Preston, M. A., Shaheen, H. H., Krivos, K. L., Limbach, P. A., et al. (2012). Yeast Trm7 interacts with distinct proteins for critical modifications of the tRNAPhe anticodon loop. RNA 18, 1921–1933. doi: 10.1261/rna.035287.112
Guy, M. P., Shaw, M., Weiner, C. L., Hobson, L., Stark, Z., Rose, K., et al. (2015). Defects in tRNA Anticodon Loop 2'-O-methylation are implicated in nonsyndromic X-linked intellectual disability due to mutations in FTSJ1. Hum. Mutat. 36, 1176–1187. doi: 10.1002/humu.22897
Haag, S., Warda, A. S., Kretschmer, J., Günnigmann, M. A., Höbartner, C., and Bohnsack, M. T. (2015). NSUN6 is a human RNA methyltransferase that catalyzes formation of m5C72 in specific tRNAs. RNA 21, 1532–1543. doi: 10.1261/rna.051524.115
Hagerman, R. J., and Polussa, J. (2015). Treatment of the psychiatric problems associated with fragile X syndrome. Curr. Opin. Psychiatry 28, 107–112. doi: 10.1097/YCO.0000000000000131
Hamel, B. C., Smits, A. P., van den Helm, B., Smeets, D. F., Knoers, N. V., van Roosmalen, T., et al. (1999). Four families (MRX43, MRX44, MRX45, MRX52) with nonspecific X-linked mental retardation: clinical and psychometric data and results of linkage analysis. Am. J. Med. Genet. 85, 290–304. doi: 10.1002/(SICI)1096-8628(19990730)85:3<290::AID-AJMG21>3.0.CO;2-H
Hartmann, A. M., Nayler, O., Schwaiger, F. W., Obermeier, A., and Stamm, S. (1999). The interaction and colocalization of Sam68 with the splicing-associated factor YT521-B in nuclear dots is regulated by the Src family kinase p59(fyn). Mol. Biol. Cell 10, 3909–3926. doi: 10.1091/mbc.10.11.3909
Haussmann, I. U., Bodi, Z., Sanchez-Moran, E., Mongan, N. P., Archer, N., Fray, R. G., et al. (2016). m(6)A potentiates Sxl alternative pre-mRNA splicing for robust Drosophila sex determination. Nature 540, 301–304. doi: 10.1038/nature20577
He, C. (2010). Grand challenge commentary: RNA epigenetics? Nat. Chem. Biol. 6, 863–865. doi: 10.1038/nchembio.482
Heiss, N. S., Bächner, D., Salowsky, R., Kolb, A., Kioschis, P., and Poustka, A. (2000). Gene structure and expression of the mouse dyskeratosis congenita gene, Dkc1. Genomics 67, 153–163. doi: 10.1006/geno.2000.6227
Heiss, N. S., Knight, S. W., Vulliamy, T. J., Klauck, S. M., Wiemann, S., Mason, P. J., et al. (1998). X-linked dyskeratosis congenita is caused by mutations in a highly conserved gene encoding a protein with putative nucleolar functions. Nat. Genet. 19, 32–38. doi: 10.1038/ng0598-32
Helm, M. (2006). Post-transcriptional nucleotide modification and alternative folding of RNA. Nucleic Acids Res. 34, 721–733. doi: 10.1093/nar/gkj471
Hermann, A., Schmitt, S., and Jeltsch, A. (2003). The human Dnmt2 has residual DNA-(cytosine-C5) methyltransferase activity. J. Biol. Chem. 278, 31717–31721. doi: 10.1074/jbc.M305448200
Hess, M. E., Hess, S., Meyer, K. D., Verhagen, L. A., Koch, L., Brönneke, H. S., et al. (2013). The fat mass and obesity associated gene (Fto) regulates activity of the dopaminergic midbrain circuitry. Nat. Neurosci. 16, 1042–1048. doi: 10.1038/nn.3449
Higa-Nakamine, S., Suzuki, T., Uechi, T., Chakraborty, A., Nakajima, Y., Nakamura, M., et al. (2012). Loss of ribosomal RNA modification causes developmental defects in zebrafish. Nucleic Acids Res. 40, 391–398. doi: 10.1093/nar/gkr700
Honda, S., Hayashi, S., Imoto, I., Toyama, J., Okazawa, H., Nakagawa, E., et al. (2010). Copy-number variations on the X chromosome in Japanese patients with mental retardation detected by array-based comparative genomic hybridization analysis. J. Hum. Genet. 55, 590–599. doi: 10.1038/jhg.2010.74
Horwich, M. D., Li, C., Matranga, C., Vagin, V., Farley, G., Wang, P., et al. (2007). The Drosophila RNA methyltransferase, DmHen1, modifies germline piRNAs and single-stranded siRNAs in RISC. Curr. Biol. 17, 1265–1272. doi: 10.1016/j.cub.2007.06.030
Hussain, S., Sajini, A. A., Blanco, S., Dietmann, S., Lombard, P., Sugimoto, Y., et al. (2013). NSun2-mediated cytosine-5 methylation of vault noncoding RNA determines its processing into regulatory small RNAs. Cell Rep. 4, 255–261. doi: 10.1016/j.celrep.2013.06.029
Iijima, T., Wu, K., Witte, H., Hanno-Iijima, Y., Glatter, T., Richard, S., et al. (2011). SAM68 regulates neuronal activity-dependent alternative splicing of neurexin-1. Cell 147, 1601–1614. doi: 10.1016/j.cell.2011.11.028
Irimia, M., Weatheritt, R. J., Ellis, J. D., Parikshak, N. N., Gonatopoulos-Pournatzis, T., Babor, M., et al. (2014). A highly conserved program of neuronal microexons is misregulated in autistic brains. Cell 159, 1511–1523. doi: 10.1016/j.cell.2014.11.035
Jacob-Hirsch, J., Eyal, E., Knisbacher, B. A., Roth, J., Cesarkas, K., Dor, C., et al. (2018). Whole-genome sequencing reveals principles of brain retrotransposition in neurodevelopmental disorders. Cell Res. 28, 187–203. doi: 10.1038/cr.2018.8
Jia, G., Fu, Y., Zhao, X., Dai, Q., Zheng, G., Yang, Y., et al. (2011). N6-methyladenosine in nuclear RNA is a major substrate of the obesity-associated FTO. Nat. Chem. Biol. 7, 885–887. doi: 10.1038/nchembio.687
Jurkowski, T. P., Meusburger, M., Phalke, S., Helm, M., Nellen, W., Reuter, G., et al. (2008). Human DNMT2 methylates tRNA(Asp) molecules using a DNA methyltransferase-like catalytic mechanism. RNA 14, 1663–1670. doi: 10.1261/rna.970408
Kan, L., Grozhik, A. V., Vedanayagam, J., Patil, D. P., Pang, N., Lim, K. S., Huang, Y. C., et al. (2017). The m(6)A pathway facilitates sex determination in Drosophila. Nat. Commun. 8:15737. doi: 10.1038/ncomms15737
Kaya, Y., and Ofengand, J. (2003). A novel unanticipated type of pseudouridine synthase with homologs in bacteria, archaea, and eukarya. RNA 9, 711–721. doi: 10.1261/rna.5230603
Keller, L., Xu, W., Wang, H. X., Winblad, B., Fratiglioni, L., and Graff, C. (2011). The obesity related gene, FTO, interacts with APOE, and is associated with Alzheimer's disease risk: a prospective cohort study. J. Alzheimer's Dis. 23, 461–469. doi: 10.3233/JAD-2010-101068
Khan, M. A., Rafiq, M. A., Noor, A., Hussain, S., Flores, J. V., Rupp, V., et al. (2012). Mutation in NSUN2, which encodes an RNA methyltransferase, causes autosomal-recessive intellectual disability. Am. J. Hum. Genet. 90, 856–863. doi: 10.1016/j.ajhg.2012.03.023
Khoddami, V., and Cairns, B. R. (2013). Identification of direct targets and modified bases of RNA cytosine methyltransferases. Nat. Biotechnol. 31, 458–464. doi: 10.1038/nbt.2566
Kishore, S., and Stamm, S. (2006). The snoRNA HBII-52 regulates alternative splicing of the serotonin receptor 2C. Science 311, 230–232. doi: 10.1126/science.1118265
Knuckles, P., Lence, T., Haussmann, I. U., Jacob, D., Kreim, N., Carl, S. H., Masiello, I., et al. (2018). Zc3h13/Flacc is required for adenosine methylation by bridging the mRNA-binding factor Rbm15/Spenito to the m(6)A machinery component Wtap/Fl(2)d. Genes Dev. 32, 415–429. doi: 10.1101/gad.309146.117
Koonin, E. V. (1996). Pseudouridine synthases: four families of enzymes containing a putative uridine-binding motif also conserved in dUTPases and dCTP deaminases. Nucleic Acids Res. 24, 2411–2415. doi: 10.1093/nar/24.12.2411
Krug, L., Chatterjee, N., Borges-Monroy, R., Hearn, S., Liao, W. W., Morrill, K., et al. (2017). Retrotransposon activation contributes to neurodegeneration in a Drosophila TDP-43 model of ALS. PLoS Genet. 13:e1006635. doi: 10.1371/journal.pgen.1006635
Kuchino, Y., Borek, E., Grunberger, D., Mushinski, J. F., and Nishimura, S. (1982). Changes of post-transcriptional modification of wye base in tumor-specific tRNAPhe. Nucleic Acids Res. 10, 6421–6432. doi: 10.1093/nar/10.20.6421
Kumar, S., Mapa, K., and Maiti, S. (2014). Understanding the effect of locked nucleic acid and 2'-O-methyl modification on the hybridization thermodynamics of a miRNA-mRNA pair in the presence and absence of AfPiwi protein. Biochemistry 53, 1607–1615. doi: 10.1021/bi401677d
Kunert, N., Marhold, J., Stanke, J., Stach, D., and Lyko, F. (2003). A Dnmt2-like protein mediates DNA methylation in Drosophila. Development 130, 5083–5090. doi: 10.1242/dev.00716
Kurth, H. M., and Mochizuki, K. (2009). 2'-O-methylation stabilizes Piwi-associated small RNAs and ensures DNA elimination in Tetrahymena. RNA 15, 675–685. doi: 10.1261/rna.1455509
Lacoux, C., Di Marino, D., Boyl, P. P., Zalfa, F., Yan, B., Ciotti, M. T., et al. (2012). BC1-FMRP interaction is modulated by 2'-O-methylation: RNA-binding activity of the tudor domain and translational regulation at synapses. Nucleic Acids Res. 40, 4086–4096. doi: 10.1093/nar/gkr1254
Lafontaine, D. L., Bousquet-Antonelli, C., Henry, Y., Caizergues-Ferrer, M., and Tollervey, D. (1998). The box H + ACA snoRNAs carry Cbf5p, the putative rRNA pseudouridine synthase. Genes Dev. 12, 527–537. doi: 10.1101/gad.12.4.527
Lee, S. H., Kim, I., and Chung, B. C. (2007). Increased urinary level of oxidized nucleosides in patients with mild-to-moderate Alzheimer's disease. Clin. Biochem. 40, 936–938. doi: 10.1016/j.clinbiochem.2006.11.021
Legrand, C., Tuorto, F., Hartmann, M., Liebers, R., Jacob, D., Helm, M., et al. (2017). Statistically robust methylation calling for whole-transcriptome bisulfite sequencing reveals distinct methylation patterns for mouse RNAs. Genome Res. 27, 1589–1596. doi: 10.1101/gr.210666.116
Lence, T., Akhtar, J., Bayer, M., Schmid, K., Spindler, L., Ho, C. H., et al. (2016). m(6)A modulates neuronal functions and sex determination in Drosophila. Nature 540, 242–247. doi: 10.1038/nature20568
Lence, T., Soller, M., and Roignant, J. Y. (2017). A fly view on the roles and mechanisms of the m(6)A mRNA modification and its players. RNA Biol. 14, 1232–1240. doi: 10.1080/15476286.2017.1307484
Leschziner, G. D., Coffey, A. J., Andrew, T., Gregorio, S. P., Dias-Neto, E., Calafato, M., et al. (2011). Q8IYL2 is a candidate gene for the familial epilepsy syndrome of partial epilepsy with pericentral spikes (PEPS). Epilepsy Res. 96, 109–115. doi: 10.1016/j.eplepsyres.2011.05.010
Leygue (2007). Steroid receptor RNA activator (SRA1): unusual bifaceted gene products with suspected relevance to breast cancer. Nucl. Recept. Signal. 5:e006. doi: 10.1621/nrs.05006
Li, J., Yang, Z., Yu, B., Liu, J., and Chen, X. (2005). Methylation protects miRNAs and siRNAs from a 3'-end uridylation activity in Arabidopsis. Curr. Biol. 15, 1501–1507. doi: 10.1016/j.cub.2005.07.029
Li, L., Zang, L., Zhang, F., Chen, J., Shen, H., Shu, L., et al. (2017a). Fat mass and obesity-associated (FTO) protein regulates adult neurogenesis. Hum. Mol. Genet. 26, 2398–2411. doi: 10.1093/hmg/ddx128
Li, T. R., Jia, Y. J., Wang, Q., Shao, X. Q., and Lv, R. J. (2017b). Circular RNA: a new star in neurological diseases. Int. J. Neurosci. 127, 726–734. doi: 10.1080/00207454.2016.1236382
Li, W., Prazak, L., Chatterjee, N., Grüninger, S., Krug, L., Theodorou, D., et al. (2013). Activation of transposable elements during aging and neuronal decline in Drosophila. Nat Neurosci 16, 529–531. doi: 10.1038/nn.3368
Li, X., Zhu, P., Ma, S., Song, J., Bai, J., Sun, F., et al. (2015). Chemical pulldown reveals dynamic pseudouridylation of the mammalian transcriptome. Nat. Chem. Biol. 11, 592–597. doi: 10.1038/nchembio.1836
Li, X., Xiong, X., Wang, K., Wang, L., Shu, X., Ma, S., et al. (2016). Transcriptome-wide mapping reveals reversible and dynamic N(1)-methyladenosine methylome. Nat. Chem. Biol. 12, 311–316. doi: 10.1038/nchembio.2040
Li, X., Xiong, X., Zhang, M., Wang, K., Chen, Y., Zhou, J., et al. (2017c). Base-resolution mapping reveals distinct m(1)A methylome in nuclear- and mitochondrial-encoded transcripts. Mol. Cell 68, 993.e9–1005.e9. doi: 10.1016/j.molcel.2017.10.019
Liang, B., Zhou, J., Kahen, E., Terns, R. M., Terns, M. P., and Li, H. (2009). Structure of a functional ribonucleoprotein pseudouridine synthase bound to a substrate RNA. Nat. Struct. Mol. Biol. 16, 740–785. doi: 10.1038/nsmb.1624
Lin, M. J., Tang, L. Y., Reddy, M. N., and Shen, C. K. (2005). DNA methyltransferase gene dDnmt2 and longevity of Drosophila. J. Biol. Chem. 280, 861–864. doi: 10.1074/jbc.C400477200
Liu, J. Z., Yue, Y. N., Han, D. L., Wang, X., Fu, Y., Zhang, L., et al. (2014). A METTL3-METTL14 complex mediates mammalian nuclear RNA N-6-adenosine methylation. Nat. Chem. Biol. 10, 93–95. doi: 10.1038/nchembio.1432
Lovejoy, A. F., Riordan, D. P., and Brown, P. O. (2014). Transcriptome-wide mapping of pseudouridines: pseudouridine synthases modify specific mRNAs in S. cerevisiae. PLoS ONE 9:e110799. doi: 10.1371/journal.pone.0110799
Lubs, H. A., Stevenson, R. E., and Schwartz, C. E. (2012). Fragile X and X-linked intellectual disability: four decades of discovery. Am. J. Hum. Genet. 90, 579–590. doi: 10.1016/j.ajhg.2012.02.018
Luo, S., and Tong, L. (2014). Molecular basis for the recognition of methylated adenines in RNA by the eukaryotic YTH domain. Proc. Natl. Acad. Sci. U.S.A. 111, 13834–13839. doi: 10.1073/pnas.1412742111
Machnicka, M. A., Milanowska, K., Osman Oglou, O., Purta, E., Kurkowska, M., Olchowik, A., et al. (2013). MODOMICS: a database of RNA modification pathways−2013 update. Nucleic Acids Res. 41, D262–D267. doi: 10.1093/nar/gks1007
Martinez, F. J., Lee, J. H., Lee, J. E., Blanco, S., Nickerson, E., Gabriel, S., et al. (2012). Whole exome sequencing identifies a splicing mutation in NSUN2 as a cause of a Dubowitz-like syndrome. J. Med. Genet. 49, 380–385. doi: 10.1136/jmedgenet-2011-100686
Martinez, G., Choudury, S. G., and Slotkin, R. K. (2017). tRNA-derived small RNAs target transposable element transcripts. Nucleic Acids Res. 45, 5142–5152. doi: 10.1093/nar/gkx103
Mauer, J., Luo, X., Blanjoie, A., Jiao, X., Grozhik, A. V., Patil, D. P., et al. (2017). Reversible methylation of m(6)Am in the 5' cap controls mRNA stability. Nature 541, 371–375. doi: 10.1038/nature21022
Melka, M. G., Gillis, J., Bernard, M., Abrahamowicz, M., Chakravarty, M. M., Leonard, G. T., et al. (2013). FTO, obesity and the adolescent brain. Hum. Mol. Genet. 22, 1050–1058. doi: 10.1093/hmg/dds504
Meola, G., and Cardani, R. (2015). Myotonic dystrophies: an update on clinical aspects, genetic, pathology, and molecular pathomechanisms. Biochim. Biophys. Acta 1852, 594–606. doi: 10.1016/j.bbadis.2014.05.019
Metodiev, M. D., Spåhr, H., Loguercio Polosa, P., Meharg, C., Becker, C., Altmueller, J., et al. (2014). NSUN4 is a dual function mitochondrial protein required for both methylation of 12S rRNA and coordination of mitoribosomal assembly. PLoS Genet. 10:e1004110. doi: 10.1371/journal.pgen.1004110
Meyer, K. D., and Jaffrey, S. R. (2017). Rethinking m(6)A readers, writers, and erasers. Annu. Rev. Cell Dev. Biol. 33, 319–342. doi: 10.1146/annurev-cellbio-100616-060758
Meyer, K. D., Saletore, Y., Zumbo, P., Elemento, O., Mason, C. E., and Jaffrey, S. R. (2012). Comprehensive analysis of mRNA methylation reveals enrichment in 3' UTRs and near stop codons. Cell 149, 1635–1646. doi: 10.1016/j.cell.2012.05.003
Minnerop, M., Weber, B., Schoene-Bake, J. C., Roeske, S., Mirbach, S., Anspach, C., et al. (2011). The brain in myotonic dystrophy 1 and 2: evidence for a predominant white matter disease. Brain 134, 3527–3543. doi: 10.1093/brain/awr299
Mitchell, J. R., Wood, E., and Collins, K. (1999). A telomerase component is defective in the human disease dyskeratosis congenita. Nature 402, 551–555. doi: 10.1038/990141
Mochizuki, Y., He, J., Kulkarni, S., Bessler, M., and Mason, P. J. (2004). Mouse dyskerin mutations affect accumulation of telomerase RNA and small nucleolar RNA, telomerase activity, and ribosomal RNA processing. Proc. Natl. Acad. Sci. U.S.A. 101, 10756–10761. doi: 10.1073/pnas.0402560101
Motorin, Y., and Grosjean, H. (1999). Multisite-specific tRNA:m5C-methyltransferase (Trm4) in yeast Saccharomyces cerevisiae: identification of the gene and substrate specificity of the enzyme. RNA 5, 1105–1118. doi: 10.1017/S1355838299982201
Motorin, Y., and Helm, M. (2010). tRNA stabilization by modified nucleotides. Biochemistry 49, 4934–4944. doi: 10.1021/bi100408z
Motorin, Y., and Helm, M. (2011). RNA nucleotide methylation. Wiley Interdiscip. Rev. RNA 2, 611–631. doi: 10.1002/wrna.79
Okano, M., Xie, S., and Li, E. (1998). Dnmt2 is not required for de novo and maintenance methylation of viral DNA in embryonic stem cells. Nucleic Acids Res. 26, 2536–2540. doi: 10.1093/nar/26.11.2536
Oldmeadow, C., Mossman, D., Evans, T.-J., Holliday, E. G., Tooney, P. A., Cairns, M. J., et al. (2014). Combined analysis of exon splicing and genome wide polymorphism data predict schizophrenia risk loci. J. Psychiatr. Res. 52, 44–49. doi: 10.1016/j.jpsychires.2014.01.011
Pan, T. (2018). Modifications and functional genomics of human transfer RNA. Cell Res. doi: 10.1038/s41422-018-0013-y. [Epub ahead of print].
Patil, D. P., Chen, C. K., Pickering, B. F., Chow, A., Jackson, C., Guttman, M., et al. (2016). m(6)A RNA methylation promotes XIST-mediated transcriptional repression. Nature 537, 369–373. doi: 10.1038/nature19342
Pergolizzi, R. G., and Grunberger, D. (1980). The effect of exogenous nutrients on the biosynthesis of Y base in tRNAPHe from Ehrlich ascites carcinoma. Cancer Lett. 8, 329–333. doi: 10.1016/0304-3835(80)90149-4
Perrat, P. N., DasGupta, S., Wang, J., Theurkauf, W., Weng, Z., Rosbash, M., et al. (2013). Transposition-driven genomic heterogeneity in the Drosophila brain. Science 340, 91–95. doi: 10.1126/science.1231965
Phalke, S., Nickel, O., Walluscheck, D., Hortig, F., Onorati, M. C., and Reuter, G. (2009). Retrotransposon silencing and telomere integrity in somatic cells of Drosophila depends on the cytosine-5 methyltransferase DNMT2. Nat. Genet. 41, 696–702. doi: 10.1038/ng.360
Ping, X. L., Sun, B. F., Wang, L., Xiao, W., Yang, X., Wang, W. J., et al. (2014). Mammalian WTAP is a regulatory subunit of the RNA N6-methyladenosine methyltransferase. Cell Res. 24, 177–189. doi: 10.1038/cr.2014.3
Pintard, L., Lecointe, F., Bujnicki, J. M., Bonnerot, C., Grosjean, H., and Lapeyre, B. (2002). Trm7p catalyses the formation of two 2'-O-methylriboses in yeast tRNA anticodon loop. EMBO J. 21, 1811–1820. doi: 10.1093/emboj/21.7.1811
Raddatz, G., Guzzardo, P. M., Olova, N., Fantappié, M. R., Rampp, M., Schaefer, M., et al. (2013). Dnmt2-dependent methylomes lack defined DNA methylation patterns. Proc. Natl. Acad. Sci. U.S.A. 110, 8627–8631. doi: 10.1073/pnas.1306723110
Rai, K., Chidester, S., Zavala, C. V., Manos, E. J., James, S. R., Karpf, A. R., et al. (2007). Dnmt2 functions in the cytoplasm to promote liver, brain, and retina development in zebrafish. Genes Dev. 21, 261–266. doi: 10.1101/gad.1472907
Ramser, J., Winnepenninckx, B., Lenski, C., Errijgers, V., Platzer, M., Schwartz, C. E., et al. (2004). A splice site mutation in the methyltransferase gene FTSJ1 in Xp11.23 is associated with non-syndromic mental retardation in a large Belgian family (MRX9). J. Med. Genet. 41, 679–683. doi: 10.1136/jmg.2004.019000
Rebane, A., Roomere, H., and Metspalu, A. (2002). Locations of several novel 2'-O-methylated nucleotides in human 28S rRNA. BMC Mol. Biol. 3:1. doi: 10.1186/1471-2199-3-1
Reitz, C., Tosto, G., Mayeux, R., Luchsinger, J. A., Group, N.-L. N. F. S., and Alzheimer's Disease Neuroimaging, I. (2012). Genetic variants in the Fat and Obesity Associated (FTO) gene and risk of Alzheimer's disease. PLoS ONE 7:e50354. doi: 10.1371/journal.pone.0050354
Rintala-Dempsey, A. C., and Kothe, U. (2017). Eukaryotic stand-alone pseudouridine synthases - RNA modifying enzymes and emerging regulators of gene expression? RNA Biol. 14, 1185–1196. doi: 10.1080/15476286.2016.1276150
Roignant, J. Y., and Soller, M. (2017). m(6)A in mRNA: an ancient mechanism for fine-tuning gene expression. Trends Genet. 33, 380–390. doi: 10.1016/j.tig.2017.04.003
Ross, R. J., Weiner, M. M., and Lin, H. (2014). PIWI proteins and PIWI-interacting RNAs in the soma. Nature 505, 353–359. doi: 10.1038/nature12987
Roth, A., Schaffner, W., and Hertel, C. (1999). Phytoestrogen kaempferol (3,4',5,7-tetrahydroxyflavone) protects PC12 and T47D cells from beta-amyloid-induced toxicity. J. Neurosci. Res. 57, 399–404. doi: 10.1002/(SICI)1097-4547(19990801)57:3<399::AID-JNR12>3.0.CO;2-W
Roundtree, I. A., Evans, M. E., Pan, T., and He, C. (2017). Dynamic RNA modifications in gene expression regulation. Cell 169, 1187–1200. doi: 10.1016/j.cell.2017.05.045
Safra, M., Sas-Chen, A., Nir, R., Winkler, R., Nachshon, A., Bar-Yaacov, D., et al. (2017). The m1A landscape on cytosolic and mitochondrial mRNA at single-base resolution. Nature 551, 251–255. doi: 10.1038/nature24456
Sahoo, T., del Gaudio, D., German, J. R., Shinawi, M., Peters, S. U., Person, R. E., et al. (2008). Prader-Willi phenotype caused by paternal deficiency for the HBII-85C/D box small nucleolar RNA cluster. Nat. Genet. 40, 719–721. doi: 10.1038/ng.158
Saito, K., Sakaguchi, Y., Suzuki, T., Suzuki, T., Siomi, H., and Siomi, M. C. (2007). Pimet, the Drosophila homolog of HEN1, mediates 2 '-O-methylation of PIWI-interacting RNAs at their 3 ' ends. Genes Dev. 21, 1603–1608. doi: 10.1101/gad.1563607
Schaefer, M., and Lyko, F. (2010a). Lack of evidence for DNA methylation of Invader4 retroelements in Drosophila and implications for Dnmt2-mediated epigenetic regulation. Nat. Genet. 42, 920–921. doi: 10.1038/ng1110-920
Schaefer, M., and Lyko, F. (2010b). Solving the Dnmt2 enigma. Chromosoma 119, 35–40. doi: 10.1007/s00412-009-0240-6
Schaefer, M., Pollex, T., Hanna, K., Tuorto, F., Meusburger, M., Helm, M., et al. (2010). RNA methylation by Dnmt2 protects transfer RNAs against stress-induced cleavage. Genes Dev. 24, 1590–1595. doi: 10.1101/gad.586710
Schöller, E., Weichmann, F., Treiber, T., Ringle, S., Treiber, N., Flatley, A., et al. (2018). Interactions, localization and phosphorylation of the m6A generating METTL3-METTL14-WTAP complex. RNA 24, 499–512. doi: 10.1261/rna.064063.117
Schorn, A. J., Gutbrod, M. J., LeBlanc, C., and Martienssen, R. (2017). LTR-retrotransposon control by tRNA-derived small RNAs. Cell 170, 61.e11–71.e11. doi: 10.1016/j.cell.2017.06.013
Schosserer, M., Minois, N., Angerer, T. B., Amring, M., Dellago, H., Harreither, E., et al. (2015). Methylation of ribosomal RNA by NSUN5 is a conserved mechanism modulating organismal lifespan. Nat. Commun. 6, 6158. doi: 10.1038/ncomms7158
Schwartz, S., Bernstein, D. A., Mumbach, M. R., Jovanovic, M., Herbst, R. H., León-Ricardo, B. X., et al. (2014a). Transcriptome-wide mapping reveals widespread dynamic-regulated pseudouridylation of ncRNA and mRNA. Cell 159, 148–162. doi: 10.1016/j.cell.2014.08.028
Schwartz, S., Mumbach, M. R., Jovanovic, M., Wang, T., Maciag, K., Bushkin, G. G., et al. (2014b). Perturbation of m6A writers reveals two distinct classes of mRNA methylation at internal and 5' sites. Cell Rep. 8, 284–296. doi: 10.1016/j.celrep.2014.05.048
Shaheen, R., Han, L., Faqeih, E., Ewida, N., Alobeid, E., Phizicky, E. M., et al. (2016). A homozygous truncating mutation in PUS3 expands the role of tRNA modification in normal cognition. Hum. Genet. 135, 707–713. doi: 10.1007/s00439-016-1665-7
Shao, Y., and Chen, Y. (2016). Roles of circular RNAs in neurologic disease. Front. Mol. Neurosci. 9:25. doi: 10.3389/fnmol.2016.00025
Sharma, S., Yang, J., Watzinger, P., Kötter, P., and Entian, K. D. (2013). Yeast Nop2 and Rcm1 methylate C2870 and C2278 of the 25S rRNA, respectively. Nucleic Acids Res. 41, 9062–9076. doi: 10.1093/nar/gkt679
Sibley, C. R., Emmett, W., Blazquez, L., Faro, A., Haberman, N., Briese, M., et al. (2015). Recursive splicing in long vertebrate genes. Nature 521, 371–375. doi: 10.1038/nature14466
Sledz, P., and Jinek, M. (2016). Structural insights into the molecular mechanism of the m(6)A writer complex. Elife 5:e18434. doi: 10.7554/eLife.18434
Sloan, K. E., Warda, A. S., Sharma, S., Entian, K. D., Lafontaine, D. L. J., and Bohnsack, M. T. (2017). Tuning the ribosome: the influence of rRNA modification on eukaryotic ribosome biogenesis and function. RNA Biol. 14, 1138–1152. doi: 10.1080/15476286.2016.1259781
Somme, J., Van Laer, B., Roovers, M., Steyaert, J., Versées, W., and Droogmans, L. (2014). Characterization of two homologous 2'-O-methyltransferases showing different specificities for their tRNA substrates. RNA 20, 1257–1271. doi: 10.1261/rna.044503.114
Sridhar, P., Gan, H. H., and Schlick, T. (2008). A computational screen for C/D box snoRNAs in the human genomic region associated with Prader-Willi and Angelman syndromes. J. Biomed. Sci. 15, 697–705. doi: 10.1007/s11373-008-9271-x
Takano, K., Nakagawa, E., Inoue, K., Kamada, F., Kure, S., Goto, Y., et al. (2008). A loss-of-function mutation in the FTSJ1 gene causes nonsyndromic X-linked mental retardation in a Japanese family. Am. J. Med. Genet. B Neuropsychiatr. Genet. 147B, 479–484. doi: 10.1002/ajmg.b.30638
Terrazas, M., and Kool, E. T. (2009). RNA major groove modifications improve siRNA stability and biological activity. Nucleic Acids Res. 37, 346–353. doi: 10.1093/nar/gkn958
Theler, D., Dominguez, C., Blatter, M., Boudet, J., and Allain, F. H. (2014). Solution structure of the YTH domain in complex with N6-methyladenosine RNA: a reader of methylated RNA. Nucleic Acids Res. 42, 13911–13919. doi: 10.1093/nar/gku1116
Torres, A. G., Batlle, E., and Ribas de Pouplana, L. (2014). Role of tRNA modifications in human diseases. Trends Mol. Med. 20, 306–314. doi: 10.1016/j.molmed.2014.01.008
Tuorto, F., Liebers, R., Musch, T., Schaefer, M., Hofmann, S., Kellner, S., et al. (2012). RNA cytosine methylation by Dnmt2 and NSun2 promotes tRNA stability and protein synthesis. Nat. Struct. Mol. Biol. 19, 900–905. doi: 10.1038/nsmb.2357
Uttara, B., Singh, A. V., Zamboni, P., and Mahajan, R. T. (2009). Oxidative stress and neurodegenerative diseases: a review of upstream and downstream antioxidant therapeutic options. Curr. Neuropharmacol. 7, 65–74. doi: 10.2174/157015909787602823
van Rossum, D., Verheijen, B. M., and Pasterkamp, R. J. (2016). Circular RNAs: novel regulators of neuronal development. Front. Mol. Neurosci. 9:74. doi: 10.3389/fnmol.2016.00074
Vitali, P., Basyuk, E., Le Meur, E., Bertrand, E., Muscatelli, F., Cavaillé, J., et al. (2005). ADAR2-mediated editing of RNA substrates in the nucleolus is inhibited by C/D small nucleolar RNAs. J. Cell Biol. 169, 745–753. doi: 10.1083/jcb.200411129
Wang, C. C., Lo, J. C., Chien, C. T., and Huang, M. L. (2011). Spatially controlled expression of the Drosophila pseudouridine synthase RluA-1. Int. J. Dev. Biol. 55, 223–227. doi: 10.1387/ijdb.103112cw
Wang, P., Doxtader, K. A., and Nam, Y. (2016a). Structural basis for cooperative function of Mettl3 and Mettl14 methyltransferases. Mol. Cell 63, 306–317. doi: 10.1016/j.molcel.2016.05.041
Wang, T., Bray, S. M., and Warren, S. T. (2012). New perspectives on the biology of fragile X syndrome. Curr. Opin. Genet. Dev. 22, 256–263. doi: 10.1016/j.gde.2012.02.002
Wang, X., Feng, J., Xue, Y., Guan, Z., Zhang, D., Liu, Z., et al. (2016b). Structural basis of N-6-adenosine methylation by the METTL3-METTL14 complex. Nature 534, 575–578. doi: 10.1038/nature18298
Wang, Y., Li, Y., Toth, J. I., Petroski, M. D., Zhang, Z. L., and Zhao, J. C. (2014). N-6-methyladenosine modification destabilizes developmental regulators in embryonic stem cells. Nat. Cell Biol. 16, 191–198. doi: 10.1038/ncb2902
Wang, Y., Li, Y., Yue, M., Wang, J., Kumar, S., Wechsler-Reya, R. J., et al. (2018). N(6)-methyladenosine RNA modification regulates embryonic neural stem cell self-renewal through histone modifications. Nat. Neurosci. 21, 195–206. doi: 10.1038/s41593-017-0057-1
Wen, J., Lv, R., Ma, H., Shen, H., He, C., Wang, J., et al. (2018). Zc3h13 regulates nuclear RNA m(6)A methylation and mouse embryonic stem cell self-renewal. Mol. Cell 69, 1028.e6–1038.e6. doi: 10.1016/j.molcel.2018.02.015
Weng, Y. L., Wang, X., An, R., Cassin, J., Vissers, C., Liu, Y., et al. (2018). Epitranscriptomic m(6)A regulation of axon regeneration in the adult mammalian nervous system. Neuron 97, 313.e6–325.e6. doi: 10.1016/j.neuron.2017.12.036
Widagdo, J., Zhao, Q. Y., Kempen, M. J., Tan, M. C., Ratnu, V. S., Wei, W., et al. (2016). Experience-dependent accumulation of N6-methyladenosine in the prefrontal cortex is associated with memory processes in mice. J. Neurosci. Off. J. Soc. Neurosci. 36, 6771–6777. doi: 10.1523/JNEUROSCI.4053-15.2016
Wilkinson, C. R., Bartlett, R., Nurse, P., and Bird, A. P. (1995). The fission yeast gene pmt1+ encodes a DNA methyltransferase homologue. Nucleic Acids Res. 23, 203–210. doi: 10.1093/nar/23.2.203
Willems, P., Vits, L., Buntinx, I., Raeymaekers, P., Van Broeckhoven, C., and Ceulemans, B. (1993). Localization of a gene responsible for nonspecific mental retardation (MRX9) to the pericentromeric region of the X chromosome. Genomics 18, 290–294. doi: 10.1006/geno.1993.1468
Wilusz, J. E. (2015). Controlling translation via modulation of tRNA levels. Wiley Interdiscipl. Rev. RNA 6, 453–470. doi: 10.1002/wrna.1287
Xu, C., Wang, X., Liu, K., Roundtree, I. A., Tempel, W., Li, Y., et al. (2014). Structural basis for selective binding of m6A RNA by the YTHDC1 YTH domain. Nat. Chem. Biol. 10, 927–929. doi: 10.1038/nchembio.1654
Yang, Y., Fan, X., Mao, M., Song, X., Wu, P., Zhang, Y., et al. (2017). Extensive translation of circular RNAs driven by N(6)-methyladenosine. Cell Res. 27, 626–641. doi: 10.1038/cr.2017.31
Yao, P., and Fox, P. L. (2013). Aminoacyl-tRNA synthetases in medicine and disease. EMBO Mol. Med. 5, 332–343. doi: 10.1002/emmm.201100626
Yildirim, I., Kierzek, E., Kierzek, R., and Schatz, G. C. (2014). Interplay of LNA and 2'-O-methyl RNA in the structure and thermodynamics of RNA hybrid systems: a molecular dynamics study using the revised AMBER force field and comparison with experimental results. J. Phys. Chem. B 118, 14177–14187. doi: 10.1021/jp506703g
Yoon, K. J., Ringeling, F. R., Vissers, C., Jacob, F., Pokrass, M., Jimenez-Cyrus, D., et al. (2017). Temporal control of mammalian cortical neurogenesis by m(6)A methylation. Cell 171, 877–899. doi: 10.1016/j.cell.2017.09.003
Yu, A. T., Ge, J., and Yu, Y. T. (2011). Pseudouridines in spliceosomal snRNAs. Protein Cell 2, 712–725. doi: 10.1007/s13238-011-1087-1
Yu, B., Yang, Z., Li, J., Minakhina, S., Yang, M., Padgett, R. W., et al. (2005). Methylation as a crucial step in plant microRNA biogenesis. Science 307, 932–935. doi: 10.1126/science.1107130
Yu, J., Chen, M., Huang, H., Zhu, J., Song, H., Zhu, J., et al. (2018). Dynamic m6A modification regulates local translation of mRNA in axons. Nucleic Acids Res. 46, 1412–1423. doi: 10.1093/nar/gkx1182
Yue, Y., Liu, J., Cui, X., Cao, J., Luo, G., Zhang, Z., et al. (2018). VIRMA mediates preferential m(6)A mRNA methylation in 3'UTR and near stop codon and associates with alternative polyadenylation. Cell Discov. 4:10. doi: 10.1038/s41421-018-0019-0
Zahn, L. M. (2017). ERVs affect brain gene expression. Science 355, 491–492. doi: 10.1126/science.355.6324.491-d
Zalfa, F., Giorgi, M., Primerano, B., Moro, A., Di Penta, A., Reis, S., et al. (2003). The fragile X syndrome protein FMRP associates with BC1 RNA and regulates the translation of specific mRNAs at synapses. Cell 112, 317–327. doi: 10.1016/S0092-8674(03)00079-5
Zhang, Y., Shi, J., and Chen, Q. (2017). tsRNAs: new players in mammalian retrotransposon control. Cell Res. 27, 1307–1308. doi: 10.1038/cr.2017.109
Zhao, X., Patton, J. R., Davis, S. L., Florence, B., Ames, S. J., and Spanjaard, R. A. (2004). Regulation of nuclear receptor activity by a pseudouridine synthase through posttranscriptional modification of steroid receptor RNA activator. Mol. Cell 15, 549–558. doi: 10.1016/j.molcel.2004.06.044
Zhao, Y., Mo, B., and Chen, X. (2012). Mechanisms that impact microRNA stability in plants. RNA Biol. 9, 1218–1223. doi: 10.4161/rna.22034
Zheng, G., Dahl, J. A., Niu, Y., Fedorcsak, P., Huang, C. M., Li, C. J., et al. (2013). ALKBH5 is a mammalian RNA demethylase that impacts RNA metabolism and mouse fertility. Mol. Cell 49, 18–29. doi: 10.1016/j.molcel.2012.10.015
Keywords: RNA modification, m5C, Nm, pseudouridine, m6A, neurons, disease
Citation: Angelova MT, Dimitrova DG, Dinges N, Lence T, Worpenberg L, Carré C and Roignant J-Y (2018) The Emerging Field of Epitranscriptomics in Neurodevelopmental and Neuronal Disorders. Front. Bioeng. Biotechnol. 6:46. doi: 10.3389/fbioe.2018.00046
Received: 07 March 2018; Accepted: 29 March 2018;
Published: 13 April 2018.
Edited by:
Giovanni Nigita, The Ohio State University, United StatesReviewed by:
Jernej Ule, University College London, United KingdomMartin Kos, Universität Heidelberg, Germany
Copyright © 2018 Angelova, Dimitrova, Dinges, Lence, Worpenberg, Carré and Roignant. This is an open-access article distributed under the terms of the Creative Commons Attribution License (CC BY). The use, distribution or reproduction in other forums is permitted, provided the original author(s) and the copyright owner are credited and that the original publication in this journal is cited, in accordance with accepted academic practice. No use, distribution or reproduction is permitted which does not comply with these terms.
*Correspondence: Clément Carré, Y2xlbWVudC5jYXJyZUB1cG1jLmZy
Jean-Yves Roignant, ai5yb2lnbmFudEBpbWItbWFpbnouZGU=
†These authors have contributed equally to this work.