- 1Universities Space Research Association at NASA Ames Research Center, Moffett Field, CA, United States
- 2Department of Solar Materials, Helmholtz Centre for Environmental Research, Leipzig, Germany
The aromatic nature of shikimate pathway intermediates gives rise to a wealth of potential bio-replacements for commonly fossil fuel-derived aromatics, as well as naturally produced secondary metabolites. Through metabolic engineering, the abundance of certain intermediates may be increased, while draining flux from other branches off the pathway. Often targets for genetic engineering lie beyond the shikimate pathway, altering flux deep in central metabolism. This has been extensively used to develop microbial production systems for a variety of compounds valuable in chemical industry, including aromatic and non-aromatic acids like muconic acid, para-hydroxybenzoic acid, and para-coumaric acid, as well as aminobenzoic acids and aromatic α-amino acids. Further, many natural products and secondary metabolites that are valuable in food- and pharma-industry are formed outgoing from shikimate pathway intermediates. (Re)construction of such routes has been shown by de novo production of resveratrol, reticuline, opioids, and vanillin. In this review, strain construction strategies are compared across organisms and put into perspective with requirements by industry for commercial viability. Focus is put on enhancing flux to and through shikimate pathway, and engineering strategies are assessed in order to provide a guideline for future optimizations.
Introduction
Importance of Bio-Derived (Aromatic) Building Blocks for the Global Chemical Market
The worldwide push to move toward a more sustainable society not only includes the goal to move from fossil fuel dependency toward renewable feedstocks but also aims to maintain and increase standards of living by facilitating the access to pharmaceuticals and securing the availability of foodstuff. Biotechnology is anticipated to be a key to fulfilling these objectives, as biochemical pathways are extremely versatile, giving rise to a wealth of diverse organic compounds (Chen and Nielsen, 2013; Becker and Wittmann, 2015; Becker et al., 2015). It has been predicted that bio-feedstocks could increase to 17% (Webster, 2012) of the global chemical business by 2025, equivalent to 425 billion USD (de Jong et al., 2013; Averesch, 2016), with global demand for biomass derived chemicals exceeding 8.5 Mt by 2023 (Insights, 2016). Unfortunately, to date, the majority of the bio-replacements is hardly cost competitive. This highlights the major hurdle for white biotechnology: outperforming existing chemical synthesis. The cost advantage will strongly depend on yield and titer (ergo the pathway), which comes down to cost of substrates vs. current oil price as well as capital and operational costs, which depend on the rate. Very generalized, the yield of a bioprocess should reach 85% of the theoretical maximum (Peralta-Yahya et al., 2012) and achieve a target product titer higher than 50 g/L (Woodley, 2017) (or close to the solubility limit) at a productivity in the single-figure g/(L × h) range (Woodley, 2017). A minimum specific rate has been defined as 0.01 mol/(gCDW × h) (Averesch et al., 2018). The competition with petrochemistry is less distinct for foodstuffs and especially pharmaceuticals, as in these industries products need to suffice (extremely) high quality standards, and are (often) natural products, which can be scarce and/or are hard to synthesize chemically, which makes them vastly more expensive. Among these, aromatics and aromatics-derived compounds are a very important class with applications in all three areas (chemical, pharmaceutical, and food industries). In biochemistry, aromatic compounds are almost exclusively obtained via the shikimate pathway, which not only leads to aromatic amino acids but also gives rise to diverse aromatic precursors allowing the biosynthesis of a great variety of secondary metabolites/natural products (Knaggs, 2003; Kayser and Averesch, 2015; Lai et al., 2017). Research efforts to engineer the shikimate pathway in various ways to establish microbial production systems for bulk and fine chemicals are strong (Thompson et al., 2015; Suástegui and Shao, 2016; Noda and Kondo, 2017), an overview of the compounds that have been bio-derived from the shikimate pathway and are currently bioavailable is given in Figure 1.
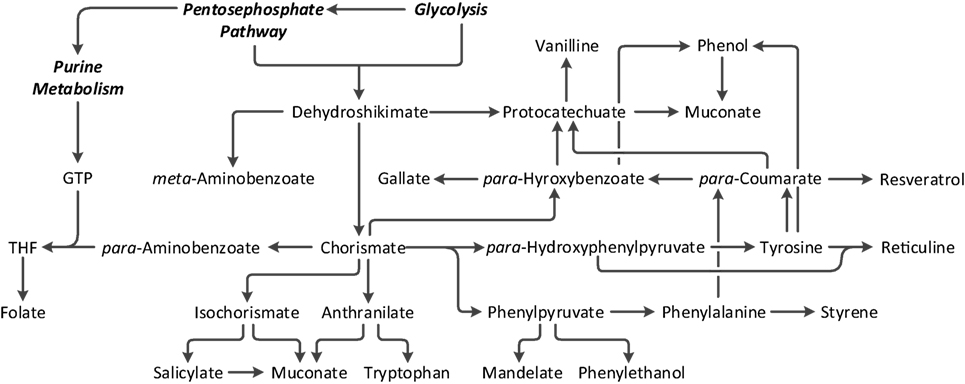
Figure 1. Major branch point intermediates and products associated with and derived from the shikimate pathway by means of metabolic engineering.
Peculiarities of the Shikimate Pathway in Model Organisms
The shikimate pathway is the central metabolic route leading to formation of tryptophan (TRP), tyrosine (TYR), and phenylalanine (PHE), this pathway exclusively exists in plants and microorganisms (Kayser and Averesch, 2015). It starts with the condensation of intermediates of glycolysis and pentosephosphate-pathway, phosphoenolpyruvate (PEP), and erythrose-4-phosphate (E4P), respectively, which enter the pathway through a series of condensation and redox reactions via 3-deoxy-d-arabino-heptulosonate-7-phosphate (DAHP), 3-dehydroquinate (DHQ), 3-dehydroshikimate (DHS) to shikimate. From there the central branch point metabolite chorismate is obtained via shikimate-3-phosphate under ATP hydrolysis and introduction of a second PEP (Herrmann, 1995). Recent approaches, especially those reconstructing more complex biosynthetic pathways, rely on Saccharomyces cerevisiae as host (Suástegui and Shao, 2016). Therefore, special attention is given to the yeasts’ shikimate pathway. In S. cerevisiae, the initial step of the shikimate pathway is catalyzed by two DAHP synthase isozymes (Meuris, 1973; Kunzler et al., 1992), encoded by ARO4 and ARO3 and regulated through feedback-inhibition by TYR and PHE, respectively. In Escherichia coli three DAHP synthase isozymes exist (aroF, aroG, aroH), which are each feedback inhibited by one of the three aromatic amino acids (TYR, PHE, TRP), in contrast the two DAHP synthases of plants are not subject to feedback-inhibition (Herrmann, 1995). In plants and bacteria the subsequent five steps are catalyzed by single enzymes, while in yeast these bioconversions are catalyzed by a pentafunctional protein, a conglomerate of monofunctional domains, which is expressed by the single gene ARO1 (Duncan et al., 1987). From the central intermediate chorismate the pathway branches off to anthranilate and prephenate leading to aromatic amino acid, para-hydroxybenzoic acid (pHBA) and para-aminobenzoic acid (pABA) synthesis, the latter being a precursor for folate metabolism (Botet et al., 2007).
In S. cerevisiae, the gene ARO7 encodes chorismate mutase which catalyzes the conversion of chorismate to prephenate where the biosynthesis route to TYR and PHE branches off, it is regulated by GCN4 (Schmidheini et al., 1990). However, this step appears to proceed also spontaneously (Winter et al., 2014; Averesch et al., 2016).
The initial step to TRP biosynthesis is catalyzed by anthranilate synthase, encoded by TRP2 which forms a multifunctional hetero–oligomeric complex with indole-3-glycerol phosphate synthase encoded by TRP3. As well TRP3 (Prantl et al., 1985) as also TRP2 (Krömer et al., 2013) may have second functions, as deletions lead to a heavy growth rate reduction regardless of the supplementation of tryptophan.
In S. cerevisiae, pABA is a precursor for ubiquinone (coenzyme Q) synthesis (Marbois et al., 2010). Outgoing from chorismate, pABA is synthesized in two subsequent reactions catalyzed by aminodeoxychorismate synthase and aminodeoxychorismate lyase. The aminodeoxychorismate synthase of S. cerevisiae, encoded by ABZ1, has similarity to E. coli pABA synthase components PabA and PabB, indicating that it is a bifunctional enzyme (Edman et al., 1993). In other organisms, like for example E. coli, the first step in ubiquinone biosynthesis is formation of pHBA in a single step bioconversion directly from the central shikimate pathway intermediate chorismate. The reaction is catalyzed by chorismate pyruvate lyase, which is encoded by the gene ubiC (Nichols and Green, 1992). It is remarkable that pHBA can also be detected in yeast though no chorismate lyase analog has been described to date (Marbois et al., 2010; Pierrel et al., 2010), indicating that either the responsible enzyme has yet to be discovered or the compound is obtained from a different precursor. It has been proposed that this precursor is rather 4-hydroxyphenylpyruvate then catechol, giving rise to a second pathway to ubiquinone synthesis in yeast (Marbois et al., 2010).
Regulation of the shikimate pathway in S. cerevisiae is manifold (Lingens et al., 1967) and yet to be fully understood. In general GCN4, the transcriptional activator of amino acid biosynthetic genes, controls aromatic amino acid biosynthesis, tightly regulating expression at transcriptional level in response to amino acid starvation (Braus, 1991).
Industrial Significance of Shikimate Pathway Derived Compounds
Among shikimate pathway derived compounds, feedstocks for polymer industry are most popular, with cis,cis-muconic acid (ccMA) as biological precursor for the nylon-6,6 building block adipic acid leading the way (Chen and Nielsen, 2013; Polen et al., 2013; Van Duuren and Wittmann, 2014; Xie et al., 2014; Bart and Cavallaro, 2015a,b; Deng et al., 2016). Biotechnological production of ccMA offers a promising alternative to chemical synthesis of adipic acid as conversion to adipic acid is easy and efficient (Polen et al., 2013; Deng et al., 2016; Vardon et al., 2016). Further, ccMA can also be used for the production of terephthalic acid (Burk et al., 2011). Other potential bio-replacements for terephthalic acid are the shikimate pathway intermediates pHBA and pABA (Farlow and Krömer, 2016). pHBA is used mainly in liquid crystal polymers like the high-strength fiber Vectran® and has an estimated market value of approx. 150 million USD per annum (Krömer et al., 2013). It is also the base material for parabens, preservatives in the cosmetic, and pharmaceutical industries (Kluczyk et al., 2002). pABA is a compound with versatile applicability, it is already being used as crosslinking agent for resins and dyes, as a precursor in the pharmaceutical industry and as a therapeutic itself (e.g., for the drug POTABA®). It is also a good UV filter as it absorbs UVB radiation (Krömer et al., 2013). Terephthalic acid (Farlow and Krömer, 2016) is a monomer in production of polyethyleneterephthalate. PET is used for packaging as well as clothing and recently also in the auto industry (Research, 2014b). The global market volume for PET packaging was nearing 16 Mt valued at 48.1 billion USD in 2014 (Pira, 2014). This is expected to rise to 19.9 Mt and 60 billion USD in 2019 (Pira, 2014). The global market volume for bio-based PET exceeded 540 kt in 2012 and is expected to rise sharply to more than the 10-fold by 2020 (Research, 2014a). Another potentially pABA-derived polymer precursor is para-phenylenediamine, which may potentially be obtained via, e.g., Kochi- or Hunsdiecker reaction followed by nucleophilic substitution, as proposed before (Averesch et al., 2016). para-Phenylenediamine is (besides terephthalic acid) the second building block of the aramid-fiber Kevlar®. Global demand for these materials, which combine high strength with low weight as well as chemical and heat resistance properties, was estimated to 74.5 kt in 2014 and is expected to rise to 110 kt in 2020 with an estimated value of 4.7 billion USD (Markets, 2014).
As a metabolic successor to pABA also production of folates has been pursued in many different bacteria, with lactic acid bacteria leading the way in microbial production of the B vitamin (Sybesma et al., 2003; Patring et al., 2006). As a dietary supplement, this underlines the other great field of application for shikimate pathway derived compounds, which is the food- and pharma-industry. Especially the class of benzylisoquinoline alkaloids (BIAs) are currently in the focus (DeLoache et al., 2015; Galanie et al., 2015), which are derived from tyrosine. BIAs are a class of alkaloids comprised of ca. 2,500 different compounds, with the opioids being the most popular among them. Opioids are indispensable in palliative care and to date still plant-derived, which makes them susceptible to supply difficulties due to environmental factors like climate or disease (Narcross et al., 2016), at the most altering quality and in the worst case resulting in total harvest loss. Further, the demand of approx. 1,000 km2 agricultural area for poppy farming to generate the annual demand of ca. 800 t opiates constitutes a potential competition for food crops (Galanie et al., 2015).
Another class of less controversial but nevertheless important plant-derived medical products, which originate from the phenylalanine-branch of the shikimate pathway are the tropan-alkaloids. Scopolamine, the main compound of interest, is an important bulk compound in the semi-synthesis of drugs like Buscopan® and Spiriva®. Spiriva was ranked number 13 best-selling drug in 2013 with sales reaching almost 3 billon USD (Brooks, 2014) and continues to be one of the most important medicines. Scopolamine is still exclusively derived from farmed Duboisia species (Averesch and Kayser, 2014; Ullrich et al., 2016). Therefore, interest to develop a biotechnological process is high, in order to stabilize supply (Ullrich et al., 2017). Not quite as popular, but still with some pharmaceutical relevance are metabolites of tryptophan. In particular, indole and quinoline alkaloids are used as chemotherapeutic agents (Krivoruchko and Nielsen, 2015).
Another important natural product, which naturally originates from the shikimate pathway from a different branch than the synthetic biology route, is vanillin (Hansen et al., 2009; Kayser and Averesch, 2015). Interestingly, biotechnological production derives vanillin from central shikimate pathway, different to natural production that proceeds via p-coumarate (cf. Figure 1). Extraction from vanilla beans can deliver less than 1% of the world’s annual 16 kt demand of vanillin, as it is one of the most popular food aromas with prices up to 1,500 USD/kg for high quality extracts (Evolva, 2014) and a total market value of approx. 650 million USD. It can be made petro-chemically or chemically from lignin waste; however, a fully biological process is favored, due to consumer acceptance (Kaur and Chakraborty, 2013); biotechnological production is run by Evolva.
Results—Strain Engineering Strategies for Production of Aromatics and Shikimate Pathway Derived Compounds
The full spectrum of shikimate pathway derived products is covered; however, only studies outgoing from non-petrochemistry derived carbon-sources are considered, unlike, e.g., production of ccMA in Pseudomonas putida from benzoate (Schmidt and Knackmuss, 1984; Bang and Choi, 1995; Choi et al., 1997), as benzoate can hardly be considered a sustainable feedstock. While there are too many studies on shikimate pathway derived products to be compared at once, different reviews have covered this from distinct perspectives, each focusing on certain groups of shikimate pathway derived products (Polen et al., 2013; Rodriguez et al., 2014; Xie et al., 2014; Thompson et al., 2015; Lee and Wendisch, 2017; Narcross et al., 2016; Suástegui and Shao, 2016; Noda and Kondo, 2017; Wang et al., 2018). In contrast to the existing reviews, this review also discusses bio-based production of aromatic compounds progressively by means of certain unique studies, looking at significant modifications that, e.g., increase the precursor availability, channel flux through the shikimate pathway or impact formation of aromatics on a cellular level. For this, a number of values have been calculated, which allow present strain construction strategies to be compared from an industry point of view. In Table 1 the production of different target compounds is compared by used carbon-sources, employed organism and specific strain, genes overexpressed and/or knocked out, final titer, product yield as fraction of theoretical maximum (calculated by metabolic modeling, cf. File S1 in Supplementary Material) productivity. Estimation of specific rates help to further classify the engineering strategies of the respective studies. In addition, Figure 2 gives an overview of all available modifications that enhance carbon flow to and within the shikimate pathway. Finally, a direction toward the next generation of aromatics producing microbial cell factories is given.
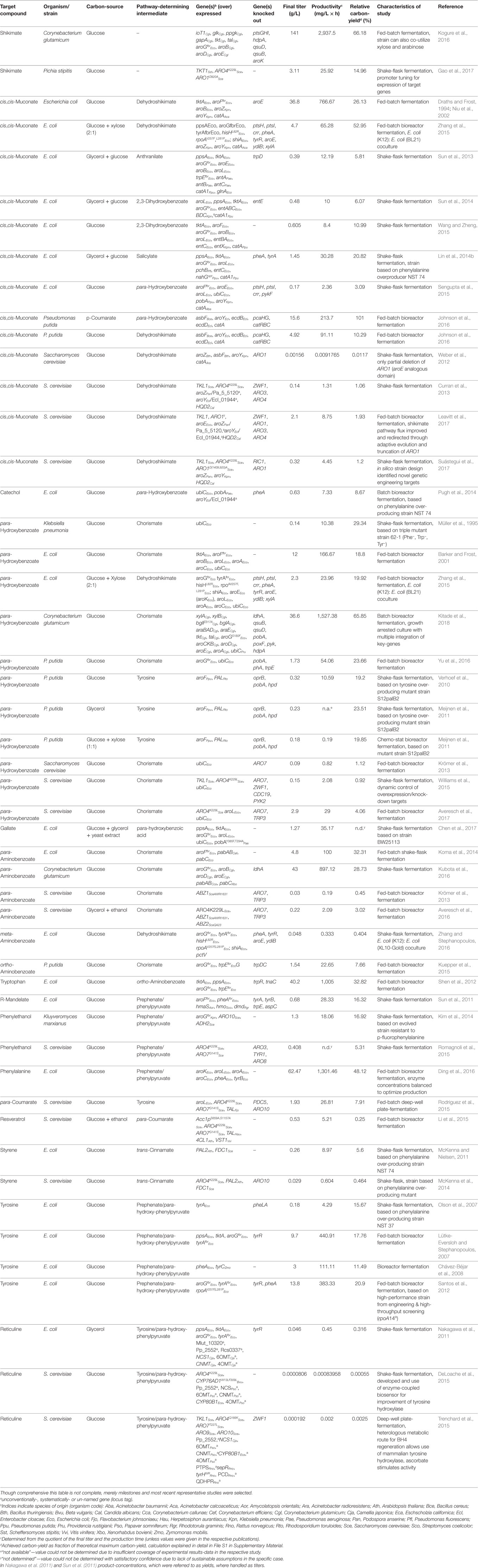
Table 1. Overview of studies on production of aromatics and aromatics-derived compounds from the shikimate pathway—comparison of target compounds, carbon-sources, organisms and strains, genes overexpressed or knocked out, final titers, and peculiarities of study.
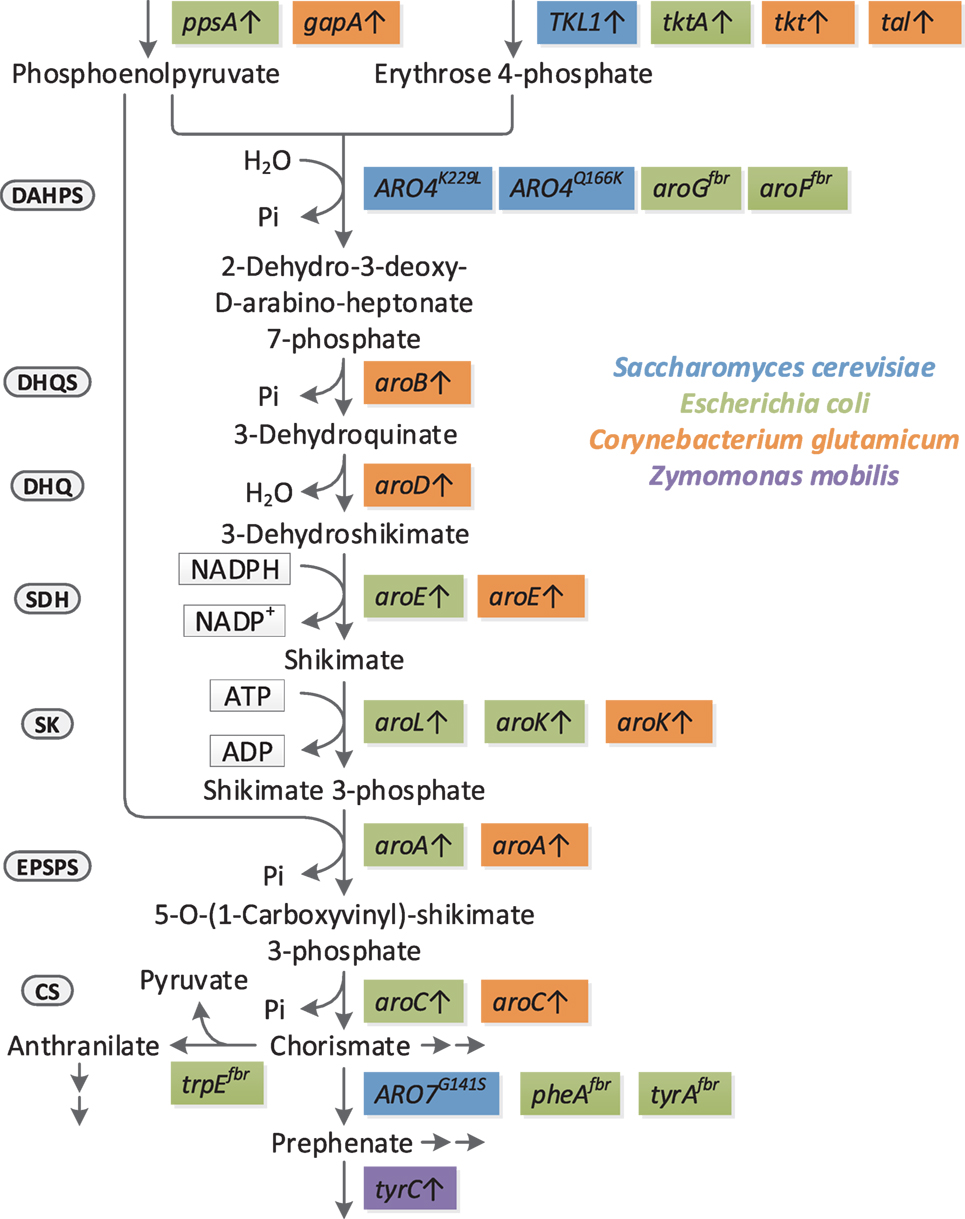
Figure 2. Reported genetic modifications that significantly improve flux to and through the shikimate pathway in the respective context. Only feedback-resistant enzymes and overexpression targets are included, knockouts have not been respected. Highlighted in blue are yeast (in most cases S. cerevisiae) genes, green E. coli genes, orange C. glutamicum genes, and purple Z. mobilis genes. With the exception of aroL (applied in S. cerevisiae), tyrC (applied in E. coli) and aroGfbr (applied in C. glutamicum) the respective overexpression targets have not been applied outside their native organism. Major enzymes are indicted to the left of the linear part of the pathway; DAHPS, 3-deoxy-d-arabinoheptulosonate 7-phosphate (DAHP) synthase; DHQS, 3-dehydroquinate synthase; DHQ, 3-dehydroquinate dehydratase; SDH, shikimate 5-dehydrogenase; SK, shikimate kinase; EPSPS, 5-enolpyruvylshikimate 3-phosphate synthase; CS, chorismate synthase.
Production of ccMA
De novo production of ccMA from glucose via the shikimate pathway has been pioneered in E. coli (Draths and Frost, 1994). The pathway was established in two steps outgoing from dehydroshikimate (DHS), via protocatechuate (PCA) and catechol by means of the aroZ gene from Klebsiella pneumoniae encoding DHS dehydratase, the aroY gene from K. pneumoniae encoding PCA decarboxylase and the catA gene from Acinetobacter calcoaceticus encoding catechol 1,2-dioxygenase. The level of the entry metabolites to shikimate pathway was increased by overexpressing tktA and carbon flux was increased by means of a feedback-inhibition resistant DAHP synthase aroFfbr alongside overexpression of the E. coli native aroB. Further, blocking of the pathway below DHS by deletion of the DHS dehydrogenase gene aroE, directed carbon flux to ccMA. The optimized process showed a high maximum titer of 36.8 g/L and its productivity, which is close to reaching g/(L × h), remains so far unrivaled (Niu et al., 2002). The yield, however, stays far behind the theoretical maximum (cf. Table 1).
Production of ccMA via a different branch of the shikimate pathway in E. coli utilizing glucose and glycerol could only achieve 390 mg/L ccMA (Sun et al., 2013). There, ccMA was derived downstream of chorismate from anthranilate on the TRP synthesis branch of the shikimate pathway. This was done by cloning an anthranilate 1,2-dioxygenase from Pseudomonas aeruginosa (encoded by the genes antA antB antC, cloned as an operon) and catechol 1,2-dioxygenase from Pseudomonas putida (catA1) in E. coli. TRP biosynthesis was blocked and overexpression of glutamine synthase resulted in a strengthened glutamine regeneration system favoring anthranilate formation in combination with a feedback-inhibition resistant anthranilate synthase (trpE) and overexpression of PEP synthetase (ppsA), tktA, aroGfbr, aroE, aroB, aroL, and trpEfbr. Two more variations of this pathway for production of ccMA have been developed by this group, both involve the isomerization of chorismate to isochorismate. The first one proceeds via 2,3-dihydroxybenzoate (Sun et al., 2014), the other one via salicylate (Lin et al., 2014b). The routes delivered respective titers of 480 and 1,500 mg/L. In the first case this was achieved using a strain with a deletion in entE, and further engineering to channel flux to chorismate, as described in another study (Lin et al., 2013). Overexpression of the E. coli genes entABC (coding for 2,3-dihydro-2,3-DHBA dehydrogenase, isochorismatase, and isochorismate synthase) along with a K. pneumoniae 2,3-DHBA decarboxylase and the same catechol 1,2-dioxygenase as in the previous study (Sun et al., 2013) completed the pathway. In the second case an “off the shelf” phenylalanine over-producing E. coli strain NST 74 (ATCC31884) with deletions in pheA and tyrA was amended with the same modifications to increase precursor abundance as described previously (Lin et al., 2013). Overexpression of the genes for isochorismate synthase from E. coli (entC), isochorismate pyruvate lyase from Pseudomonas fluorescens (pchB), an optimized salicylate 1-monoxygenase from Pseudomonas putida (nahGopt) and the catechol 1,2-dioxygenase, as described before (Sun et al., 2013), lead to ccMA production. The pathway via salicylate was the only one to reach a meaningful yield around 1/5th of the theoretical maximum, which is a performance close to the record by the Frost-Lab. Also productivity was highest via salicylate, however, orders of magnitude lower than the respective benchmark (Frost-Lab).
A “hybrid” of the DHS-pathway and the routes via chorismate to ccMA leads from chorismate via pHBA to PCA, from here the steps coincide with the pathway via DHS. It was shown that in E. coli PCA and catechol can be produced this way, with the highest yield being that of catechol at a titer of 451 mg/L (Pugh et al., 2014). Here, strain construction was also based on the phenylalanine over-producing strain NST 74. Overexpression of chorismate lyase, encoded by ubiC, initiated the pathway, a pHBA hydroxylase (pobA) from P. aeruginosa and a protocatechuate decarboxylase from Enterobacter cloacae completed it. To further increase flux to the target compounds the chorismate mutase/prephenate dehydratase (pheA) was disrupted. Recently the complete pathway from glucose to ccMA was established (Sengupta et al., 2015). The engineered E. coli strain was capable of producing almost 170 mg/L ccMA. The strain had deletions in the genes ptsH, ptsI, crr, and pykF to boost PEP levels available to the shikimate pathway. Further the E. coli DAHP synthase, shikimate 5-dehydrogenase, shikimate kinase II and chorismate lyase (aroFfbr, aroE, aroL, and ubiC) were overexpressed along with a pobA from P. putida, aroY from K. pneumoniae, and catA from Acinetobacter sp. ADP1 coding for 4-hydroxybenzoate hydrolyase, protocatechuate decarboxylase, and catechol 1,2-dioxygenase, respectively. Despite the extensive genetic engineering, the yield as well as the specific rate of product formation were comparatively low (0.5 mg/gCDW × h), hinting at limitations in one or more steps of this pathway.
In a different approach to sustainable ccMA production, carbon-flux enters the shikimate pathway starting from lignin-derived species. In the respective study, a ccMA titer of 13.5 g/L was obtained (Deng et al., 2016). This was done by engineering the metabolism of a P. putida KT2440 strain: in a pathway that uses downstream aromatic amino acid metabolism intermediates like p-coumarate as substrates, a route to ccMA was constructed that partially coincides in the last steps with the route via pHBA. The same approach was followed in another study where in addition PCA decarboxylase activity was enhanced by expressing associated proteins to produce ccMA from lignin and glucose in P. putida KT2440, leading to titers of 15.6 and 4.9 g/L (Johnson et al., 2016). This way complete conversion of p-coumarate into ccMA was achieved at the highest specific rate.
Initial de novo production of ccMA in S. cerevisiae was established by following a similar strain construction strategy as in the first approach in E. coli (Draths and Frost, 1994): partial deletion of ARO1 (the aroE analogous domain) blocked the conversion of 3-dehydroshikimate into shikimate, while a DHS dehydratase from Bacillus thuringiensis (aroZ), a PCA decarboxylase from K. pneumoniae (aroY), and a catechol 1,2-dioxygenases from Acinetobacter radioresistens (catA) composed the pathway (Weber et al., 2012). The bottleneck here appeared to be the first step: PCA levels no higher than 7 mg/L could be detected, which limited the final titer to only 1.56 mg/L (Weber et al., 2012). In an analogous approach the DHS dehydratase was taken from Podospora anserina and the PCA decarboxylase from Enterobacter cloacae (Curran et al., 2013). A catechol 1,2-dioxygenase from Candida albicans completed the pathway. In addition, knockout of ARO3 and over expression of the feedback-resistant ARO4K229L increased overall flux to aromatics. Further optimizations were deletion of ZWF1 and overexpression of TKL1. The former intended to increase flux to the shikimate pathway in order to increase conversion of G6P to E4P, by blocking the channeling of G6P into the oxidative branch of the pentose phosphate pathway. The latter favored the conversion of pentoses into E4P by increased transketolase levels. In this study, a final ccMA titer of 141 mg/L could be achieved. In a follow up study the previous strain was improved using a biosensor for aromatic amino acids (Leavitt et al., 2016), performing adaptive laboratory evolution to improve flux through the shikimate pathway. Flux was then redirected, following a similar approach as in Weber et al. (2012): by deleting the ARO1 and expressing a truncated version thereof, flux was redirected from dehydroshikimate toward ccMA, while auxotrophy was prevented by attenuated expression of the E. coli aroE. With the final strain a titer of 2.1 g/L could be reached in a fed-batch bioreactor experiment (Leavitt et al., 2017), while also the yield was improved it, however, remained far (10-fold) behind the studies on E. coli, reaching only about a fiftieth of the theoretical maximum. Compared to E. coli, also productivities and specific rates are orders of magnitude lower (cf. Table 1).
Production of pHBA
Initially bio-based production of pHBA was established in plants, like N. tabacum (Siebert et al., 1996; Köhle et al., 2003) or sugarcane (McQualter et al., 2005). Microbial production of pHBA from glucose was first reported using K. pneumonia; deriving it from chorismate a final titer of 120 mg/L was reached (Müller et al., 1995). Due to the low amount of carbon-source this nevertheless corresponds to a significant yield (cf. Table 1). This was done by introducing the E. coli chorismate lyase, encoded by ubiC, on a plasmid into a K. pneumonia strain deficient in the ability to produce aromatic amino acids. While the specific rate could only be roughly estimated (cf. File S1 in Supplementary Material), it nevertheless ranks in an order of magnitude among the highest (double-digit mg/gCDW × h range), indicating the organism’s potential as producer for aromatics. As a foremost reason its pathogenicity has probably stopped its exploitation for this purpose and the research community has focused on safer microbial hosts. Bacterial production of pHBA has been enabled in Pseudomonas putida, reaching a titer of 0.32 (Verhoef et al., 2010) and 1.73 g/L (Yu et al., 2016), while both groups reached comparable yields approaching 1/4th of the theoretical maximum, the latter reached a fivefold higher productivity. The superiority of the more recent approach may be attributable to the shorter pathway, deriving pHBA directly from chorismate, rather than downstream of tyrosine. Fermentative production of pHBA in E. coli has been patented (Johnson et al., 1998) and reported (Barker and Frost, 2001) deriving the compound via chorismate from glucose, where a final concentration of 12 g/L was achieved at similar yields (approx. 1/5th of the theoretical maximum) and rates as in Pseudomonas. This was done by overexpression of the feedback-inhibition resistant DAHP synthase aroFfbr as well as elevation of expression levels of other enzymes in the pathway (tktA, aroB, aroL, aroA, and aroC). The conversion of chorismate to pHBA was accomplished by the overexpression of ubiC. It was found that the DAHP synthase was feedback inhibited by pHBA while DAHP synthase overexpression reduced chorismate lyase expression levels (Barker and Frost, 2001). An active transport of the aromatic out of the cell (Van Dyk et al., 2004) may contribute to achieving these comparatively high titers of extracellular pHBA. More recently, the same E. coli coculture system that was used for production of ccMA from glucose and xylose, was also used for production of pHBA (Zhang et al., 2015). The dehydroshikimate over-producing strain was complemented with a strain where the downstream shikimate pathway was engineered for conversion of dehydroshikimate into pHBA via chorismate. Due to the diluting nature of the fed-batch process only a titer of 2.3 g/L was reached, the yield, however, was comparable to the previous approaches. The highest production of pHBA was achieved in C. glutamicum in a growth-arrested culture with multiple integration of key-genes (Kitade et al., 2018). A titer of 36.66 g/L was reached, at almost 2/3rd of the theoretical maximum yield (which represents a new record and is on one level together with the shikimate-producing strain of the same group), with the second highest rate of aromatics production (1.5 g/h) to date. This was achieved through extensive metabolic engineering, in both, the shikimate pathway itself and throughout the central metabolism. In particular, the final production strain had deletions in the dihydroxyacetone phosphatase and pyruvate kinase (hdpA and pyk) to re-route carbon flux, as well as multiple integrations of the chorismate synthase, shikimate kinase, 3-dehydroquinate synthase and a feedback-inhibition resistant DAHP synthase (aroCKB and aroGfbr). Further, besides other modifications (deletion of ldhA, qsuB, qsuD, pobA, poxF, and overexpression of xylA, xylB, bglF317A, bglA, araBAD, araE, tkt, tal, aroD, aroE, aroA), it featured a new feedback-inhibition resistant ubiC from Providencia rustigianii.
Production of pHBA in S. cerevisiae was first demonstrated by overexpressing the ubiC gene from E. coli in a strain where drainage of flux away from chorismate was prevented by deletions of the ARO7 and TRP3 genes, abolishing the biosynthesis of aromatic amino acids. The titer of the a proof-of-principle study reached 90 mg/L (Krömer et al., 2013). Production of pHBA in S. cerevisiae was revived using a substantially different approach, where gene silencing was regulated by a dynamic circuit (Williams et al., 2015). This allowed application of in silico determined knockouts (Averesch and Krömer, 2014), which are lethal when applied constitutively. The circuit autonomously triggers gene expression at a high population density, and was linked with an RNA interference module to enable target gene silencing. This was used to control flux through the shikimate pathway for the production of pHBA. Dynamic RNA repression allowed gene knock-downs, which were identified by elementary flux mode analysis as highly productive but with low biomass formation, to be implemented after a population growth phase. In particular, silencing of ARO7, ZWF1 and the two pyruvate kinase genes CDC19 and PYK2 along with overexpression of TKL1, ARO4K229L and ubiC resulted in a pHBA titer of 0.15 g/L. In a further study an S. cerevisiae strain previously engineered to channel flux to chorismate (Averesch et al., 2016) was optimized for pHBA formation. There, constitutive deletions of ARO7 and TRP3, as well as expression of ARO4K229L and aroL were used to increase flux through the shikimate pathway. Further, ubiC was screened against the mutated version ubiCCCSS, which supposedly has enhanced solubility (Holden et al., 2002). Surprisingly ubiC still performed better (Averesch et al., 2017), also kinetic limitations appeared to be not as profound as reported (Holden et al., 2002). The strain was then used to stepwise develop a production process; in a fed-batch bioreactor a final titer of 2.9 g/L could be reached, at a productivity of almost 30 mg/(L × h) and a yield that reached 4% of the theoretical maximum.
In addition to being an intermediate for production of ccMA, pHBA can also be an intermediate for production of gallic acid. This was recently shown by engineering the p-hydroxybenzoate hydroxylases (pobA) from Pseudomonas aeruginosa (containing the mutations Y385F and T294A) in a way that enabled formation of gallic acid from pHBA. With additional overexpression of ppsA, tktA, aroGfbr, aroL, and ubiC the E. coli strain reached a product titer of 1.27 g/L (Chen et al., 2017). In another study, a pathway to phenol was established via pHBA, which is an alternative to the route via tyrosine and shortens the pathway (Thompson, 2017).
In this context a synthetic variation of the shikimate pathway for production of terephthalic acid is also worth mentioning—it utilizes the same biochemical conversion steps as for production of pHBA via chorismate, with the only difference from the natural shikimate pathway being that it originates from a different precursor: Outgoing from a compound analogous to erythrose 4-phosphate, where the –OH group at the C2 position is replaced by –CH3, namely “2-hydroxy-3-methy1-4-oxobutoxy phosphonate,” this pathway leads to the di-acid (terephthalic acid) rather than the hydroxyl-acid (pHBA) (Osterhout et al., 2013).
Production of Aminobenzoates
Production of pABA in S. cerevisiae was first demonstrated by overexpressing an ABZ1 gene coding for aminodeoxychorismate synthase from the wine yeast strain AWRI1631 in an ARO7 and TRP3 knockout strain, resulting in a final titer of 34 mg/L (Krömer et al., 2013). After the initial study in S. cerevisiae, recently also enhanced production of pABA in E. coli has been reported, reaching maximum titers of 4.8 g/L (Koma et al., 2014). This was accomplished by employing the E. coli feedback-inhibition resistant DAHP synthase aroFfbr and aminodeoxychorismate lyase pabC genes in combination with a Corynebacterium efficiens aminodeoxychorismate synthase pabAB, which combines both domains of the bifunctional pABA synthase in one protein. pABA production in S. cerevisiae was revamped by re-engineering a strain that incorporated the feedback-resistant ARO4K229L as well as deletions in the ARO7 and TRP3 genes, in order to channel flux to chorismate. This strain was used to screen different ABZ1 and ABZ2 genes for pABA production. In glucose-based shake-flask fermentations the highest titer was reached when overexpressing the ABZ1 and ABZ2 genes from the wine yeast strains AWRI1631 and QA23, respectively. In silico metabolic modeling indicated a metabolic advantage for pABA production on glycerol and combined glycerol–ethanol carbon-sources. This was confirmed experimentally and in a fed-batch bioreactor experiments pABA a titer of 237 mg/L was reached (Averesch et al., 2016). In parallel pABA production in C. glutamicum was accomplished; screening different genes for pABA formation in a similar way as in the S. cerevisiae study, pabAB from Corynebacterium callunae, pabC from Xenorhabdus bovienii were identified as most efficient (Kubota et al., 2016). In a strain incorporating an aroGfbr from E. coli and a deletion in ldhA (lactate dehydrogenase), while overexpressing the native aroB, aroD, and aroE genes (3-dehydroquinate synthase, 3-dehydroquinate dehydratase, shikimate 5-dehydrogenase) a pABA titer of 43 g/L was reached. The yield reached almost 30% of the theoretical maximum and also the rate approached the threshold for industrial feasibility, underlining the meaningfulness of this host-engineering targets combination.
Microbial production of the pABA isomer anthranilate (ortho-aminobenzoic acid, oABA) has also been established, in particular by engineering Pseudomonas putida: A feedback-resistant DAHP synthase (aroGfbr) was overexpressed in combination with a feedback-resistant anthranilate synthase (trpEfbr) while the anthranilate phosphoribosyl transferase (trpD), indole-3-glycerol phosphate synthase (trpC) and chorismate mutase (pheA) were knocked out in order to redirect carbon-flux. The highest oABA titer of 1.54 g/L was, however, obtained in a strain that still carried the chorismate mutase (Kuepper et al., 2015).
Recently also the third isomer, meta-aminobenzoic acid (mABA), has become bioavailable and a biological production system has been demonstrated (Zhang and Stephanopoulos, 2016). This was accomplished based on the previously developed E. coli–E. coli coculture system for production of ccMA and pHBA by the Stephanopoulos Lab (see above). One strain accumulates DHS while the second one converts it to the aromatic compound of interest (in this case mABA), accomplished by a PLP-dependent 3-aminobenzoate synthase (pctV). Comparing the obtained titer, yield, and most importantly rate of mABA production to the previous studies on production of ccMA and pHBA, which are, respectively, 198- and 73-fold higher, it becomes clear that PctV must be the bottleneck.
Production of Aromatic Amino Acids and Phenylpyruvate-Branch Derived Products
Although only a minor biotechnological product, the same basic engineering strategy was used for production of TRP in E. coli (Shen et al., 2012). By overexpressing tktA (transketolase) and ppsA (PEP synthase) in combination with aroGfbr and trpEfbr 40.2 g/L could be produced. The corresponding yield and productivity are approaching the defined requirements for an industrial process (approx. 1/3rd of guide values), however, the mediocre specific rate indicates shortcomings in the strain construction.
Production of mandelic acid in E. coli was established by introducing a heterologous pathway consisting of the genes hmaS, hmo, and dmd, encoding for hydroxymandelate synthase (Amycolatopsis orientalis), hydroxymandelate oxydase (Streptomyces coelicolor) and mandelate dehydrogenase (Rhodotorula graminis). In addition, flux through the shikimate pathway was redirected by deletions of tyrA (chorismate mutase/prephenate dehydrogenase), tyrB (aromatic amino acid aminotransferase), trpE (anthranilate synthase) and aspC (aspartate aminotransferase) as well as introduction of aroFfbr and pheAfbr. The resulting final titers were 0.74 g/L S-mandelic acid and 0.68 g/L R-mandelic acid, respectively (Sun et al., 2011).
Production of phenylethanol, which is mainly used as a fragrance, but has recently also been identified as a next generation biofuel, has so far mostly been established via the Ehrlich-pathway, which degrades PHE. Since PHE itself is a metabolic end product and a compound with commercial valuable, this approach can hardly be considered industrially relevant (Zhang et al., 2014b). De novo production of phenylethanol has been established in different organisms, including E. coli (Kang et al., 2014) and different yeasts (Kim et al., 2014; Romagnoli et al., 2015). Strain construction strategies mostly focus on enhancing phenylpyruvate decarboxylase and alcohol dehydrogenase activities, while inhibiting PHE formation by deletion of the respective transaminase (ARO8), thus blocking the entry of the Ehrlich-pathway. In combination with the usual feedback-resistant DHAP synthase (and chorismate mutase) respective titers in the range of 1.3 and 0.37 g/L could be reached (Kim et al., 2014; Romagnoli et al., 2015). It is noteworthy that in a study on production of pABA in S. cerevisiae [(Averesch et al., 2016), see above], in glucose batch cultures phenylethanol titers of almost 0.1 g/L were obtained, which exceeded the target product, even though the chorismate mutase (ARO7) was knocked out (Averesch, 2016). This was attributed to a flux overflow that degraded chorismate into prephenate and phenylpyruvate (Winter et al., 2014). In respect of this, it might be worthwhile for future engineering strategies to focus more on increasing overall flow to and through shikimate pathway rather than phenylethanol formation itself. In particular, in a study on production of p-coumaric acid in S. cerevisiae in addition to the same feedback-resistant DAHP synthase ARO4K229L and chorismate mutase ARO7G141S the shikimate kinase was identified as major bottleneck and overexpression of aroL in the S. cerevisiae strain significantly improved production, reaching a final titer of 1.93 g/L (Rodriguez et al., 2015). The same pathway has also been extended toward production of resveratrol, where a titer of 0.53 g/L could be obtained in a fed-batch setup from glucose and ethanol (Li et al., 2015). More recently, coumaric acid producing yeast strains were also utilized for production of different flavonoids (Rodriguez et al., 2017b). In a study on transcriptional changes in p-coumaric acid over-producing S. cerevisiae strains, downregulation of amino acid and sugar transporters was observed. Knockouts of some of these (transporters of aromatic amino acid, TAT1, and polyamines, TPO1) resulted in an over 40% increased production (Rodriguez et al., 2017a).
Extensive work has been done on the production of PHE, as it is a valuable amino acid with diverse applications in food- and pharma-industry. In the most notable recent work the optimum concentrations of six enzymes (aroK, aroL, aroA, aroC, pheA, and tyrB) along the shikimate pathway were adjusted in E. coli allowing an impressive final titer of 62.47 g/L to be reached (Ding et al., 2016), with a yield of roughly half of theoretical maximum and the third highest productivity of an aromatic product. This is even more impressive when considering how far downstream of the main pathway the compound is derived and underlines the importance for identification of bottlenecks in order to balance the pathway and tailor specific strain construction strategies.
PHE is a precursor for trans-cinnamic acid, which leads to the production of aroma compounds like cinnamaldehyde as well as cinnamyl- and hydrocinnamyl-alcohol, demonstrated in S. cerevisiae (Gottardi et al., 2017). Further, styrene can be produced via trans-cinnamate; in E. coli this was established based on the PHE over-producing strain NST 74, in which expression of PAL2 (phenylalanine ammonia lyase) from Arabidopsis thaliana and FDC1 (trans-cinnamic acid decarboxylase) from S. cerevisiae lead to styrene production of 0.26 g/L (McKenna and Nielsen, 2011). The same group also showed production in S. cerevisiae, where first a PHE over-producing mutant was developed through metabolic evolution, in which ARO1, ARO2, ARO3, and ARO8 appeared to be significantly upregulated. Then the same PAL2 and FDC1 genes were overexpressed, together with ARO4K229L, resulting in a styrene titer of 29 mg/L (McKenna et al., 2014).
Production of Tyrosine and Derived Products
Biotechnological production of benzylisoquinoline alkaloids (BIAs) and precursor thereof has become accessible with the first study reporting heterologous de novo reticuline biosynthesis in E. coli (Nakagawa et al., 2011). In the respective study, the tyrR gene was disrupted, and feedback-inhibition-resistant versions of the DAHP synthase (aroGfbr) and chorismate mutase (tyrAfbr) were introduced, while the ppsA and transketolase (tktA) were exogenously introduced to enhance tyrosine production prior to the BIA synthetic pathway.
Biosynthesis of reticuline has also been reported in S. cerevisiae by two independent laboratories, following different approaches (DeLoache et al., 2015; Trenchard et al., 2015) to establish the BIA synthetic pathway. Both studies apply a feedback-inhibition resistant DAHP synthase, while the latter also includes the ZWF1 knockout, overexpression of the transketolase (TKL1), aromatic aminotransferase II and phenylpyruvate decarboxylase (ARO9 and ARO10) and a feedback-inhibition resistant chorismate mutase (interestingly the uncommon ARO7T227L is used—also the DAHP synthase, ARO4Q166K, is a different one than used by most other researchers, cf. Box 1). The full pathway to opioids consist of more than a handful of steps downstream of chorismate, which are likely to be rate limiting at this stage; however, in future product formation may benefit from increased availability of the pathway’s substrates. Especially channeling flux to tyrosine may be important in respect of the previously discussed flux overflow in S. cerevisiae (Winter et al., 2014; Averesch et al., 2016). For example, for production of TYR in E. coli, it was reported that product formation was enhanced when expressing tyrC from Zymomonas mobilis, a feedback-inhibition-insensitive cyclohexadienyl dehydrogenase in combination with pheA, the chorismate mutase-prephenate dehydrogenase native to E. coli, rather than using tyrA (Chávez-Béjar et al., 2008). Transfer of these genes into a yeast-based production system for compounds derived from TYR (e.g., BIAs) may be worthwhile, potentially in combination with the deletion of PHA2, this may channel flux more efficiently to TYR.
Box 1. Feedback-inhibition resistant DAHP synthases.
Another point to consider when optimizing and balancing flux entering the shikimate pathway is the existence of various mutated DAHP synthases for both, E. coli (Kikuchi et al., 1997; Koma et al., 2012; Zhang et al., 2014a) and S. cerevisiae (Hartmann et al., 2003; Helmstaedt et al., 2005; Luttik et al., 2008), many of which feature altered feedback-regulation. In E. coli, AroG contributes by far the most (approx. 80%) to total DAHP synthase activity and is inhibited by PHE, while AroF (approx. 20% activity) and AroH are inhibited by TYR and TRP, respectively (Lin et al., 2014a). In S. cerevisiae ARO3p and ARO4p are tightly regulated by TYR and PHE, respectively, while the latter is also feedback-inhibited by high concentrations of PHE and TRP (Kunzler et al., 1992). For E. coli a variety of genes exist that encode feedback-inhibition resistant versions of all three isozymes (the most popular ones being aroFP148L, aroGP150L, aroGD146N, aroFD147N, and aroGF209S), while for S. cerevisiae so far only feedback-inhibition resistant versions of ARO4p have been described (ARO4K229L and the rarely applied ARO4Q166K). All of this needs to be taken into consideration when developing strain construction strategies toward, e.g., aromatic amino acid derived compounds or/and when including deletions that require the supplementation of certain aromatic amino acids. Therefore, a holistic comparison of the performance and the effects of the different feedback-inhibition resistant DAHP synthases in different organisms would be especially important. It could especially be interesting to see how E. coli genes perform in S. cerevisiae and potentially also vice-versa. This has partially been covered when comparing ARO4K229L vs. aroGL175D, aroGS180F, and aroFNST74, for production of p-coumarate in S. cerevisiae (Rodriguez et al., 2015), where ARO4K229L appeared to be most effective.
Conclusion
Final Thoughts on Available Targets for Genetic Engineering
Considering that in general the reactions of the shikimate pathway are thermodynamically favored (Averesch, 2016), it can be concluded that limitations are mostly kinetic and/or regulatory. This is supported by a study showing the performance of the shikimiate pathway in S. cerevisiae to be greatly dependent on the type strain (Suástegui et al., 2016). In reverse this means that the shikimate pathway is most likely tightly regulated, hence the greatest challenge will be to overcome this, rather than the fine tuning of individual reactions at the final step to the target product. While many approaches have been made to increase production of certain individual products from shikimate pathway, all applying different strain engineering strategies (cf. Figure 2), only a few target optimizations beyond the reactions of the pathway. In addition to what has been described in Box 2, two commonly applied targets beyond the shikimate pathway are overexpression of the transketolase and deletion of the glucose-6-phosphate dehydrogenase. The latter, once a popular knockout target in yeast (ZWF1) is rarely applied anymore, a trend that is in accordance with our findings from in silico metabolic modeling (Averesch, 2016): we found that the knockout rarely benefits the yield (opposing to its original intention); especially in case of reducing pathways it negatively impacts redox cofactor availability (NADPH production from PPP). This is supported by our previous study on production of pHBA (described above) (Williams et al., 2015), where knock-down of ZWF1 had an adverse effect on the product titer.
Box 2. Importance of precursors for shikimate pathway derived products.
The crucial role of PEP, which is as well precursor as also cofactor of the shikimate pathway, has already been elaborated in the first study on production of ccMA in E. coli (Niu et al., 2002), where a difference in theoretical maximum yields of 43% was determined, depending on the availability of PEP. In another early study on E. coli, where the PEP synthase (pps) was overexpressed, it was found that not only PEP levels need to be increased but also E4P limits the formation of DAHP and E4P levels should, therefore, be increased too, in particular by overexpressing transketolase (tktA) (Patnaik and Liao, 1994). This was elaborated in a study combining tktA overexpression with knockout of pykA, pykF and deactivation of the PTS to increase PEP availability, thus reaching on almost 20-fold increase in carbon partitioning to the shikimate pathway (Gosset et al., 1996). A recent flux analysis on shikimate production in S. cerevisiae study even concluded that in yeast rather E4P is the rate limiting precursor (Suástegui et al., 2016). In a related study, the xylose utilizing yeast Scheffersomyces stipitis (Pichia stipitis) was employed, which was also further optimized for E4P formation by overexpressing TKL1, as well as channeling flux to and accumulating carbon in shikimate (by means of ARO4K229L and ARO1D920A). This led to a final titer of 3.11 g/L (Gao et al., 2017), which corresponds to one of the highest carbon-yields achieved in a yeast-based system. That this is a meaningful strain construction strategy is further underlined by another recent study on shikimate production in Corynebacterium glutamicum (Kogure et al., 2016). Here also the PTS was disabled to increase PEP availability through disruption of the ptsH gene, while ATP driven carbon-source uptake was strengthened by overexpressing iolT1 (myo-inositol permease), glk (glucokinase), and ppgk (polyphosphate glucokinase) and flux to PEP was increased by overexpressing gapA (glyceraldehyde-3-phosphate dehydrogenase), while loss to glycerol was inhibited by deletion of hdpA (DHAP phosphatase). E4P availability was increased by enhancing flux through the PPP by overexpressing tkt (transketolase) and tal (transaldolase), co-utilization of xylose and arabinose complemented this. Additional overexpression of the aroG, aroB, aroD, and aroE (DAHP synthase, DHQ synthase, DHQ dehydratase, and shikimate dehydrogenase) genes along the shikimate pathway as well as knockout of downstream pathways (qsuD, qsuB, and aroK) lead to an impressive shikimate titer of 141 g/L. The corresponding yield reached 2/3rd of the theoretical maximum, which is close to industrial feasibility and the current record for a compound in the biosynthesis of aromatic. The corresponding productivity was nearly 3 g/(L × h), which is the threshold for industrial standards. Growth-arrested cells even reached a productivity beyond 5 g/(L × h) (Kogure et al., 2016). Lately, the same group has diversified the C. glutamicum based microbial cell factory for aromatics—in a different product context rather the pyk was knocked out instead of disabling the PTS, besides other extensive modifications throughout the pathway (Kitade et al., 2018).
Perspectives and Recommendations for Future Strain Development
Systems Biology has already begun to revolutionize Biotechnology by replacing trial and error driven Metabolic Engineering with rational approaches (Dai and Nielsen, 2015). In this context especially Metabolic Modeling has tremendous potential for a priori development of strain construction strategies The radical in silico developed knockout strategy for minimum-efficacy production (Averesch and Krömer, 2014; Williams et al., 2015) is also applicable to other shikimate pathway derived compounds that involve the release of PYR, i.e., anthranillic acid and pABA (given it is feasible in the respective organism). In brief, deletion of the pyruvate kinase in theory rewires the central metabolism, rerouting flux through the shikimate pathway as the only remaining means for the metabolism to feed PYR into the TCA-cycle. That is, only if flux through the shikimate pathway is high enough to replace the junction between glycolysis and TCA-cycle. This may become possible by combining production of all products from the shikimate pathway, which result in PYR formation while also significantly increasing flux to and through the shikimate pathway by all available means. Thus, PYR formation from the shikimate pathway may become high enough to drive central metabolism. If successful, this might allow evolutionary adaptation of a PYK knockout strain to higher aromatics production (by selecting for the fittest organism also the highest aromatics producer will be obtained, due to coupling of growth to product formation) and could engender the next generation of an aromatics producing strain. Especially recent C. glutamicum based strains (Kogure et al., 2016; Kubota et al., 2016; Kitade et al., 2018) deliver yields high enough to potentially allow this; again only in case the knockout strategy is applicable to the organism, which is not a given and needs to be verified individually; for example it cannot be transferred to E. coli (Averesch and Krömer, 2014). In this context, the exploration of other organisms as suitable hosts for production of aromatics could be worthwhile. For example, Bacillus subtilis as one of the big four industrial microbial workhorses has so far been ignored for production of aromatics. Very exiting could be the implementation of production of aromatics with PYR as a by-product in an organism that does by nature not have a pyruvate kinase, like Acinetobacter baylyi, where it was shown that heterologous expression of a pyruvate kinase in this organism increases the growth rate (Kannisto et al., 2014).
If evolutionary adaptation allows to significantly improve aromatics production, sequencing, determination of SNPs and characterizations of, e.g., new feedback-resistant enzymes [similar to what has been done in the Stephanopoulos group (Santos et al., 2012)] of the adapted strain may lead to identification of unknown bottlenecks and help overcoming these. Further, this may enable reverse engineering, i.e., application of the novel targets to existing production strains. That way also strains or pathways, which do not allow the direct application of the PYK knockout (i.e., all pathways that proceed via the chorismate mutase), would benefit from the new platform strain.
As molecular biology tools for most model organisms used in Metabolic Engineering are advanced and streamlined, CRISPR has often limited advantage over traditional genetic engineering techniques, such as homologous recombination. However, when precise regulation is needed, such as conditional silencing, CRISPRi (Qi et al., 2013) is often superior to other techniques like RNAi (Crook et al., 2014), as CRISPRi directly inhibits transcription, opposing to RNAi, which inhibits translation and is thus less tight. Tools regulating expression on translational level also impose a higher burden on the metabolism of the organism and are not universally applicable [RNAi is limited to eukaryotes, in prokaryotes sRNAs can be utilized for posttranscriptional gene-repression (Na et al., 2013)]. Further, no equivalent to CRISPRa exists, which has the opposite function, and allows the activation and conditional upregulation of genetic targets (Dominguez et al., 2015). CRISPRi may be especially useful in case constitutive application of the developed strain construction strategy is not feasible [e.g., lethal knockout (Williams et al., 2015)]. In particular, in the context of the knockout strategy described above, CRISPRi may be used for (simultaneous) silencing of PTS and PYK while CRISPRa could be used to upregulate key-bottleneck enzymes. This would minimize technical effort, while constituting a chance to circumvent challenging genetic intervention strategies.
Author Contributions
NA and JK jointly conceived the study. NA reviewed the literature and extracted the data, drafted the manuscript, and performed supporting calculations. JK edited the manuscript and guided the calculations. Both authors read and approved the final manuscript.
Conflict of Interest Statement
The authors declare that the research was conducted in the absence of any commercial or financial relationships that could be construed as a potential conflict of interest.
Acknowledgments
Parts of this review are based on the Ph.D. thesis of NA (Averesch, 2016), in particular the introductory chapters and some descriptive parts of the strain construction strategies contain sections that have been adapted.
Funding
The study was not backed by any specific funding.
Supplementary Material
The Supplementary Material for this article can be found online at https://www.frontiersin.org/articles/10.3389/fbioe.2018.00032/full#supplementary-material.
References
Averesch, N. J. H. (2016). Engineering Yeast Shikimate Pathway Towards Production of Aromatics: Rational Design of a Chassis Cell Using Systems and Synthetic Biology. Ph.D. thesis, The University of Queensland, Brisbane.
Averesch, N. J. H., and Kayser, O. (2014). Assessing heterologous expression of hyoscyamine 6β-hydroxylase – a feasibility study. Procedia Chem. 13, 69–78. doi: 10.1016/j.proche.2014.12.008
Averesch, N. J. H., and Krömer, J. O. (2014). Tailoring strain construction strategies for muconic acid production in S. cerevisiae and E. coli. Metab. Eng. Commun. 1, 19–28. doi:10.1016/j.meteno.2014.09.001
Averesch, N. J. H., Martínez, V. S., Nielsen, L. K., and Krömer, J. O. (2018). Towards synthetic biology strategies for adipic acid production – an in-silico tool for combined thermodynamics and stoichiometric analysis of metabolic networks. ACS Synth. Biol. 7, 490–509. doi:10.1021/acssynbio.7b00304
Averesch, N. J. H., Prima, A., and Krömer, J. O. (2017). Enhanced production of para-hydroxybenzoic acid by genetically engineered Saccharomyces cerevisiae. Bioprocess Biosyst. Eng. 40, 1283–1289. doi:10.1007/s00449-017-1785-z
Averesch, N. J. H., Winter, G., and Krömer, J. O. (2016). Production of para-aminobenzoic acid from different carbon-sources in engineered Saccharomyces cerevisiae. Microb. Cell Fact. 15, 1–16. doi:10.1186/s12934-016-0485-8
Bang, S.-G., and Choi, C. Y. (1995). DO-stat fed-batch production of cis,cis-muconic acid from benzoic acid by Pseudomonas putida BM014. J. Ferment. Bioeng. 79, 381–383. doi:10.1016/0922-338X(95)94001-8
Barker, J. L., and Frost, J. W. (2001). Microbial synthesis of p-hydroxybenzoic acid from glucose. Biotechnol. Bioeng. 76, 376–390. doi:10.1002/bit.10160
Bart, J. C. J., and Cavallaro, S. (2015a). Transiting from adipic acid to bioadipic acid. 1, petroleum-based processes. Ind. Eng. Chem. Res. 54, 1–46. doi:10.1021/ie5020734
Bart, J. C. J., and Cavallaro, S. (2015b). Transiting from adipic acid to bioadipic acid. Part II. biosynthetic pathways. Ind. Eng. Chem. Res. 54, 567–576. doi:10.1021/ie502074d
Becker, J., Lange, A., Fabarius, J., and Wittmann, C. (2015). Top value platform chemicals: bio-based production of organic acids. Curr. Opin. Biotechnol. 36, 168–175. doi:10.1016/j.copbio.2015.08.022
Becker, J., and Wittmann, C. (2015). Advanced biotechnology: metabolically engineered cells for the bio-based production of chemicals and fuels, materials, and health-care products. Angew. Chem. Int. Ed. Engl. 54, 3328–3350. doi:10.1002/anie.201409033
Botet, J., Mateos, L., Revuelta, J. L., and Santos, M. A. (2007). A chemogenomic screening of sulfanilamide-hypersensitive Saccharomyces cerevisiae mutants uncovers ABZ2, the gene encoding a fungal aminodeoxychorismate lyase. Eukaryotic Cell 6, 2102–2111. doi:10.1128/EC.00266-07
Braus, G. H. (1991). Aromatic amino acid biosynthesis in the yeast Saccharomyces cerevisiae: a model system for the regulation of a eukaryotic biosynthetic pathway. Microbiol. Rev. 55, 349–370.
Brooks, M. (2014). Top 100 Selling Drugs of 2013. Medscape Medical News. Available at: http://www.medscape.com/viewarticle/820011. Accessed July 7, 2016.
Burk, M. J., Osterhout, R. E., and Sun, J. (2011). Semi-Synthetic Terephthalic Acid via Microorganisms That Produce Muconic Acid. US patent application US 12/851,478.
Chávez-Béjar, M. I., Lara, A. R., López, H., Hernández-Chávez, G., Martinez, A., Ramírez, O. T., et al. (2008). Metabolic engineering of Escherichia coli for L-tyrosine production by expression of genes coding for the chorismate mutase domain of the native chorismate mutase-prephenate dehydratase and a cyclohexadienyl dehydrogenase from Zymomonas mobilis. Appl. Environ. Microb. 74, 3284–3290. doi:10.1128/AEM.02456-07
Chen, Y., and Nielsen, J. (2013). Advances in metabolic pathway and strain engineering paving the way for sustainable production of chemical building blocks. Curr. Opin. Biotechnol. 24, 965–972. doi:10.1016/j.copbio.2013.03.008
Chen, Z., Shen, X., Wang, J., Wang, J., Yuan, Q., and Yan, Y. (2017). Rational engineering of p-hydroxybenzoate hydroxylase to enable efficient gallic acid synthesis via a novel artificial biosynthetic pathway. Biotechnol. Bioeng. 114, 2571–2580. doi:10.1002/bit.26364
Choi, W. J., Lee, E. Y., Cho, M. H., and Choi, C. Y. (1997). Enhanced production of cis,cis-muconate in a cell-recycle bioreactor. J. Ferment. Bioeng. 84, 70–76. doi:10.1016/S0922-338X(97)82789-4
Crook, N. C., Schmitz, A. C., and Alper, H. S. (2014). Optimization of a yeast RNA interference system for controlling gene expression and enabling rapid metabolic engineering. ACS Synth. Biol. 3, 307–313. doi:10.1021/sb4001432
Curran, K. A., Leavitt, J. M., Karim, A. S., and Alper, H. S. (2013). Metabolic engineering of muconic acid production in Saccharomyces cerevisiae. Metab. Eng. 15, 55–66. doi:10.1016/j.ymben.2012.10.003
Dai, Z., and Nielsen, J. (2015). Advancing metabolic engineering through systems biology of industrial microorganisms. Curr. Opin. Biotechnol. 36, 8–15. doi:10.1016/j.copbio.2015.08.006
de Jong, Ed, Higson, A., Walsh, P., and Wellisch, M. (2013). Bio-Based Chemicals – Value Added Products from Biorefineries. EA Bioenergy – Task 42 Biorefinery. Available at: http://www.ieabioenergy.com/wp-content/uploads/2013/10/Task-42-Biobased-Chemicals-value-added-products-from-biorefineries.pdf.
DeLoache, W. C., Russ, Z. N., Narcross, L., Gonzales, A. M., Martin, V. J. J., and Dueber, J. E. (2015). An enzyme-coupled biosensor enables (S)-reticuline production in yeast from glucose. Nat. Chem. Biol. 11, 465–471. doi:10.1038/nchembio.1816
Deng, Y., Ma, L., and Mao, Y. (2016). Biological production of adipic acid from renewable substrates: current and future methods. Biochem. Eng. J. 105(Part A), 16–26. doi:10.1016/j.bej.2015.08.015
Ding, D., Liu, Y., Xu, Y., Zheng, P., Li, H., Zhang, D., et al. (2016). Improving the production of L-phenylalanine by identifying key enzymes through multi-enzyme reaction system in vitro. Sci. Rep. 6, 32208. doi:10.1038/srep32208
Dominguez, A. A., Lim, W. A., and Qi, L. S. (2015). Beyond editing: repurposing CRISPR–Cas9 for precision genome regulation and interrogation. Nat. Rev. Mol. Cell Biol. 17, 5. doi:10.1038/nrm.2015.2
Draths, K. M., and Frost, J. W. (1994). Environmentally compatible synthesis of adipic acid from D-Glucose. J. Am. Chem. Soc. 116, 399–400. doi:10.1021/ja00080a057
Duncan, K., Edwards, R. M., and Coggins, J. R. (1987). The pentafunctional arom enzyme of Saccharomyces cerevisiae is a mosaic of monofunctional domains. Biochem. J. 246, 375–386. doi:10.1042/bj2460375
Edman, J. C., Goldstein, A. L., and Erbe, J. G. (1993). Para-aminobenzoate synthase gene of Saccharomyces cerevisiae encodes a bifunctional enzyme. Yeast 9, 669–675. doi:10.1002/yea.320090613
Evolva. (2014). Vanillin. Evolva. Available at: http://www.evolva.com/vanillin/. Accessed July 7, 2016.
Farlow, A., and Krömer, J. O. (2016). Reactions with aminobenzoic acids via diazonium salts open new routes to bio-derived aromatics. IJOC 6, 5. doi:10.4236/ijoc.2016.62010
Galanie, S., Thodey, K., Trenchard, I. J., Filsinger Interrante, M., and Smolke, C. D. (2015). Complete biosynthesis of opioids in yeast. Science 349, 1095–1100. doi:10.1126/science.aac9373
Gao, M., Cao, M., Suastegui, M., Walker, J. A., Rodriguez-Quiroz, N., Wu, Y., et al. (2017). Innovating a nonconventional yeast platform for producing shikimate as the building block of high-value aromatics. ACS Synth. Biol. 6, 29–38. doi:10.1021/acssynbio.6b00132
Gosset, G., Yong-Xiao, J., and Berry, A. (1996). A direct comparison of approaches for increasing carbon flow to aromatic biosynthesis in Escherichia coli. J. Ind. Microbiol. 17, 47–52. doi:10.1007/BF01570148
Gottardi, M., Knudsen, J. D., Prado, L., Oreb, M., Branduardi, P., and Boles, E. (2017). De novo biosynthesis of trans-cinnamic acid derivatives in Saccharomyces cerevisiae. Appl. Microbiol. Biotechnol. 101, 4883–4893. doi:10.1007/s00253-017-8220-x
Hansen, E. H., Møller, B. L., Kock, G. R., Bünner, C. M., Kristensen, C., Jensen, O. R., et al. (2009). De Novo biosynthesis of vanillin in fission yeast (Schizosaccharomyces pombe) and Baker’s yeast (Saccharomyces cerevisiae). Appl. Environ. Microbiol. 75, 2765–2774. doi:10.1128/AEM.02681-08
Hartmann, M., Schneider, T. R., Pfeil, A., Heinrich, G., Lipscomb, W. N., and Braus, G. H. (2003). Evolution of feedback-inhibited β/α barrel isoenzymes by gene duplication and a single mutation. Proc. Natl. Acad. Sci. U.S.A. 100, 862–867. doi:10.1073/pnas.0337566100
Helmstaedt, K., Strittmatter, A., Lipscomb, W. N., and Braus, G. H. (2005). Evolution of 3-deoxy-d-arabino-heptulosonate-7-phosphate synthase-encoding genes in the yeast Saccharomyces cerevisiae. Proc. Natl. Acad. Sci. U.S.A. 102, 9784–9789. doi:10.1073/pnas.0504238102
Herrmann, K. M. (1995). The Shikimate pathway: early steps in the biosynthesis of aromatic compounds. Plant Cell 7, 907–919. doi:10.2307/3870046
Holden, M. J., Mayhew, M. P., Gallagher, D. T., and Vilker, V. L. (2002). Chorismate lyase: kinetics and engineering for stability. Biochim. Biophys. Acta 1594, 160–167. doi:10.1016/S0167-4838(01)00302-8
Insights, G. M. (2016). Bio Based Polyethylene Terephthalate (PET) Market Size by Application (Consumer Goods, Technical, Bottles), Industry Analysis Report, Regional Outlook, Downstream Application Potential, Price Trends, Competitive Market Share & Forecast, 2016 – 2023. Global Market Insights. Available at: https://www.gminsights.com/industry-analysis/bio-based-PET-market. Accessed July 7, 2016.
Johnson, B. F., Amaratunga, M., and Lobos, J. H. (1998). Method for Increasing Total Production of 4-Hydroxybenzoic Acid by Biofermentation. US patent application US 09/161,129.
Johnson, C. W., Salvachúa, D., Khanna, P., Smith, H., Peterson, D. J., and Beckham, G. T. (2016). Enhancing muconic acid production from glucose and lignin-derived aromatic compounds via increased protocatechuate decarboxylase activity. Metab. Eng. Commun. 3, 111–119. doi:10.1016/j.meteno.2016.04.002
Kang, Z., Zhang, C., Du, G., and Chen, J. (2014). Metabolic engineering of Escherichia coli for production of 2-phenylethanol from renewable glucose. Appl. Biochem. Biotechnol. 172, 2012–2021. doi:10.1007/s12010-013-0659-3
Kannisto, M., Aho, T., Karp, M., and Santala, V. (2014). Metabolic engineering of Acinetobacter baylyi ADP1 for improved growth on gluconate and glucose. Appl. Environ. Microbiol. 80, 7021–7027. doi:10.1128/AEM.01837-14
Kaur, B., and Chakraborty, D. (2013). Biotechnological and molecular approaches for vanillin production: a review. Biotechnol. Appl. Biochem. 169, 1353–1372. doi:10.1007/s12010-012-0066-1
Kayser, O., and Averesch, N. (2015). Technische Biochemie: Die Biochemie und industrielle Nutzung von Naturstoffen. Wiesbaden: Springer-Verlag.
Kikuchi, Y., Tsujimoto, K., and Kurahashi, O. (1997). Mutational analysis of the feedback sites of phenylalanine-sensitive 3-deoxy-D-arabino-heptulosonate-7-phosphate synthase of Escherichia coli. Appl. Environ. Microbiol. 63, 761–762.
Kim, T.-Y., Lee, S.-W., and Oh, M.-K. (2014). Biosynthesis of 2-phenylethanol from glucose with genetically engineered Kluyveromyces marxianus. Enzyme Microb. Technol. 6, 44–47. doi:10.1016/j.enzmictec.2014.04.011
Kitade, Y., Hashimoto, R., Suda, M., Hiraga, K., and Inui, M. (2018). Production of 4-hydroxybenzoic acid by an aerobic growth-arrested bioprocess using metabolically engineered Corynebacterium glutamicum. Appl. Environ. Microbiol. 84, e02587–17. doi:10.1128/AEM.02587-17
Kluczyk, A., Popek, T., Kiyota, T., De Macedo, P., Stefanowicz, P., Lazar, C., et al. (2002). Drug evolution: p-aminobenzoic acid as a building block. Curr. Med. Chem. 9, 1871–1892. doi:10.2174/0929867023368872
Knaggs, A. R. (2003). The biosynthesis of shikimate metabolites. Nat. Prod. Rep. 20, 119–136. doi:10.1039/b100399m
Kogure, T., Kubota, T., Suda, M., Hiraga, K., and Inui, M. (2016). Metabolic engineering of Corynebacterium glutamicum for shikimate overproduction by growth-arrested cell reaction. Metab. Eng. 38, 204–216. doi:10.1016/j.ymben.2016.08.005
Köhle, A., Sommer, S., Li, S.-M., Schilde-Rentschler, L., Ninnemann, H., and Heide, L. (2003). Secondary metabolites in transgenic tobacco and potato: high accumulation of 4-hydroxybenzoic acid glucosides results from high expression of the bacterial gene ubiC. Mol. Breed. 11, 15–24. doi:10.1023/A:1022211521390
Koma, D., Yamanaka, H., Moriyoshi, K., Ohmoto, T., and Sakai, K. (2012). A convenient method for multiple insertions of desired genes into target loci on the Escherichia coli chromosome. Appl. Microbiol. Biotechnol. 93, 815–829. doi:10.1007/s00253-011-3735-z
Koma, D., Yamanaka, H., Moriyoshi, K., Sakai, K., Masuda, T., Sato, Y., et al. (2014). Production of P-aminobenzoic acid by metabolically engineered Escherichia coli. Biosci. Biotechnol. Biochem. 78, 350–357. doi:10.1080/09168451.2014.878222
Krivoruchko, A., and Nielsen, J. (2015). Production of natural products through metabolic engineering of Saccharomyces cerevisiae. Curr. Opin. Biotechnol. 35, 7–15. doi:10.1016/j.copbio.2014.12.004
Krömer, J. O., Nunez-Bernal, D., Averesch, N. J. H., Hampe, J., Varela, J., and Varela, C. (2013). Production of aromatics in Saccharomyces cerevisiae—a feasibility study. J. Biotechnol. 163, 184–193. doi:10.1016/j.jbiotec.2012.04.014
Kubota, T., Watanabe, A., Suda, M., Kogure, T., Hiraga, K., and Inui, M. (2016). Production of para-aminobenzoate by genetically engineered Corynebacterium glutamicum and non-biological formation of an N-glucosyl byproduct. Metab. Eng. 38, 322–330. doi:10.1016/j.ymben.2016.07.010
Kuepper, J., Dickler, J., Biggel, M., Behnken, S., Jaeger, G., Wierckx, N., et al. (2015). Metabolic engineering of Pseudomonas putida KT2440 to produce anthranilate from glucose. Front. Microbiol. 6:1310. doi:10.3389/fmicb.2015.01310
Kunzler, M., Paravicini, G., Egli, C. M., Irniger, S., and Braus, G. H. (1992). Cloning, primary structure and regulation of the ARO4 gene, encoding the tyrosine-inhibited 3-deoxy-D-arabino-heptulosonate-7-phosphate synthase from Saccharomyces cerevisiae. Gene 113, 67–74. doi:10.1016/0378-1119(92)90670-K
Lai, B., Plan, M. R., Averesch, N. J. H., Yu, S., Kracke, F., Lekieffre, N., et al. (2017). Quantitative analysis of aromatics for synthetic biology using liquid chromatography. Biotechnol. J. 12, 1600269. doi:10.1002/biot.201600269
Leavitt, J. M., Tong, A., Tong, J., Pattie, J., and Alper, H. S. (2016). Coordinated transcription factor and promoter engineering to establish strong expression elements in Saccharomyces cerevisiae. Biotechnol. J. 11, 866–876. doi:10.1002/biot.201600029
Leavitt, J. M., Wagner, J. M., Tu, C. C., Tong, A., Liu, Y., and Alper, H. S. (2017). Biosensor-enabled directed evolution to improve muconic acid production in Saccharomyces cerevisiae. Biotechnol. J. 12, 1600687. doi:10.1002/biot.201600687
Lee, J.-H., and Wendisch, V. F. (2017). Biotechnological production of aromatic compounds of the extended shikimate pathway from renewable biomass. J. Biotechnol. 257, 211–221. doi:10.1016/j.jbiotec.2016.11.016
Li, M., Kildegaard, K. R., Chen, Y., Rodriguez, A., Borodina, I., and Nielsen, J. (2015). De novo production of resveratrol from glucose or ethanol by engineered Saccharomyces cerevisiae. Metab. Eng. 32, 1–11. doi:10.1016/j.ymben.2015.08.007
Lin, S., Liang, R., Meng, X., Ouyang, H., Yan, H., Wang, Y., et al. (2014a). Construction and expression of mutagenesis strain of aroG gene from Escherichia coli K-12. Int. J. Biol. Macromol. 68, 173–177. doi:10.1016/j.ijbiomac.2014.04.034
Lin, Y., Sun, X., Yuan, Q., and Yan, Y. (2014b). Extending shikimate pathway for the production of muconic acid and its precursor salicylic acid in Escherichia coli. Metab. Eng. 23, 62–69. doi:10.1016/j.ymben.2014.02.009
Lin, Y., Shen, X., Yuan, Q., and Yan, Y. (2013). Microbial biosynthesis of the anticoagulant precursor 4-hydroxycoumarin. Nat. Commun. 4, 2603. doi:10.1038/ncomms3603
Lingens, F., Goebel, W., and Uesseler, H. (1967). Regulation der biosynthese der aromatischen aminosauren in Saccharomyces cerevisiae. Eur. J. Biochem. 1, 363–374. doi:10.1007/978-3-662-25813-2_50
Lütke-Eversloh, T., and Stephanopoulos, G. (2007). L-Tyrosine production by deregulated strains of Escherichia coli. Appl. Microbiol. Biotechnol. 75, 103–110. doi:10.1007/s00253-006-0792-9
Luttik, M. A., Vuralhan, Z., Suir, E., Braus, G. H., Pronk, J. T., and Daran, J. M. (2008). Alleviation of feedback inhibition in Saccharomyces cerevisiae aromatic amino acid biosynthesis: quantification of metabolic impact. Metab. Eng. 10, 141–153. doi:10.1016/j.ymben.2008.02.002
Marbois, B., Xie, L. X., Choi, S., Hirano, K., Hyman, K., and Clarke, C. F. (2010). para-Aminobenzoic acid is a precursor in coenzyme Q6 biosynthesis in Saccharomyces cerevisiae. J. Biol. Chem. 285, 27827–27838. doi:10.1074/jbc.M110.151894
Markets, R. A. (2014). Aramid Fibers (Para and Meta) – A Global Market Overview. Available at: http://www.researchandmarkets.com/research/5nwxtj/aramid_fibers. Accessed June, 2015.
McKenna, R., and Nielsen, D. R. (2011). Styrene biosynthesis from glucose by engineered E. coli. Metab. Eng. 13, 544–554. doi:10.1016/j.ymben.2011.06.005
McKenna, R., Thompson, B., Pugh, S., and Nielsen, D. R. (2014). Rational and combinatorial approaches to engineering styrene production by Saccharomyces cerevisiae. Microb. Cell Fact. 13, 123. doi:10.1186/s12934-014-0123-2
McQualter, R. B., Chong, B. F., Meyer, K., Van Dyk, D. E., O’shea, M. G., Walton, N. J., et al. (2005). Initial evaluation of sugarcane as a production platform for p-hydroxybenzoic acid. Plant Biotechnol. J. 3, 29–41. doi:10.1111/j.1467-7652.2004.00095.x
Meijnen, J.-P., Verhoef, S., Briedjlal, A. A., Winde, J. H., and Ruijssenaars, H. J. (2011). Improved p-hydroxybenzoate production by engineered Pseudomonas putida S12 by using a mixed-substrate feeding strategy. Appl. Microbiol. Biotechnol. 90, 885–893. doi:10.1007/s00253-011-3089-6
Meuris, P. (1973). Feedback inhibition of the DAHP synthetases by tRNA in Saccharomyces cerevisiae. Mol. Gen. Genet. 121, 207–218. doi:10.1007/BF00267048
Müller, R., Wagener, A., Schmidt, K., and Leistner, E. (1995). Microbial production of specifically ring-13C-labelled 4-hydroxybenzoic acid. Appl. Microbiol. Biotechnol. 43, 985–988. doi:10.1007/BF00166913
Na, D., Yoo, S. M., Chung, H., Park, H., Park, J. H., and Lee, S. Y. (2013). Metabolic engineering of Escherichia coli using synthetic small regulatory RNAs. Nat. Biotechnol. 31, 170. doi:10.1038/nbt.2461
Nakagawa, A., Minami, H., Kim, J.-S., Koyanagi, T., Katayama, T., Sato, F., et al. (2011). A bacterial platform for fermentative production of plant alkaloids. Nat. Commun. 2, 326. doi:10.1038/ncomms1327
Narcross, L., Fossati, E., Bourgeois, L., Dueber, J. E., and Martin, V. J. J. (2016). Microbial factories for the production of benzylisoquinoline alkaloids. Trends Biotechnol. 34, 228–241. doi:10.1016/j.tibtech.2015.12.005
Nichols, B. P., and Green, J. M. (1992). Cloning and sequencing of Escherichia coli ubiC and purification of chorismate lyase. J. Bacteriol. 174, 5309–5316. doi:10.1128/jb.174.16.5309-5316.1992
Niu, W., Draths, K. M., and Frost, J. W. (2002). Benzene-free synthesis of adipic acid. Biotechnol. Prog. 18, 201–211. doi:10.1021/bp010179x
Noda, S., and Kondo, A. (2017). Recent advances in microbial production of aromatic chemicals and derivatives. Trends Biotechnol. 35, 785–796. doi:10.1016/j.tibtech.2017.05.006
Olson, M. M., Templeton, L. J., Suh, W., Youderian, P., Sariaslani, F. S., Gatenby, A. A., et al. (2007). Production of tyrosine from sucrose or glucose achieved by rapid genetic changes to phenylalanine-producing Escherichia coli strains. Appl. Microbiol. Biotechnol. 74, 1031–1040. doi:10.1007/s00253-006-0746-2
Osterhout, R. E., Burgard, A. P., and Burk, M. J. (2013). Microorganisms and Processes for Producing Terephthalic Acid and Its Salts. US patent application PCT/US2013/022113.
Patnaik, R., and Liao, J. C. (1994). Engineering of Escherichia coli central metabolism for aromatic metabolite production with near theoretical yield. Appl. Environ. Microbiol. 60, 3903–3908.
Patring, J., Hjortmo, S., Jastrebova, J., Svensson, U., Andlid, T., and Jägerstad, I. M. (2006). Characterization and quantification of folates produced by yeast strains isolated from kefir granules. Eur. Food Res. Technol. 223, 633–637. doi:10.1007/s00217-005-0245-1
Peralta-Yahya, P. P., Zhang, F., Del Cardayre, S. B., and Keasling, J. D. (2012). Microbial engineering for the production of advanced biofuels. Nature 488, 320–328. doi:10.1038/nature11478
Pierrel, F., Hamelin, O., Douki, T., Kieffer-Jaquinod, S., Mühlenhoff, U., Ozeir, M., et al. (2010). Involvement of mitochondrial ferredoxin and para-aminobenzoic acid in yeast coenzyme Q biosynthesis. Chem. Biol. 17, 449–459. doi:10.1016/j.chembiol.2010.03.014
Pira, S. (2014). PET Packaging Industry News. Available at: http://www.smitherspira.com/news/2014/april/demand-for-pet-packaging-material-in-2019. Accessed June, 2015.
Polen, T., Spelberg, M., and Bott, M. (2013). Toward biotechnological production of adipic acid and precursors from biorenewables. J. Biotechnol. 167, 75–84. doi:10.1016/j.jbiotec.2012.07.008
Prantl, F., Strasser, A., Aebi, M., Furter, R., Niederberger, P., Kirschner, K., et al. (1985). Purification and characterization of the indole-3-glycerolphosphate synthase/anthranilate synthase complex of Saccharomyces cerevisiae. Eur. J. Biochem. 146, 95–100. doi:10.1111/j.1432-1033.1985.tb08624.x
Pugh, S., Mckenna, R., Osman, M., Thompson, B., and Nielsen, D. R. (2014). Rational engineering of a novel pathway for producing the aromatic compounds p-hydroxybenzoate, protocatechuate, and catechol in Escherichia coli. Process Biochem. doi:10.1016/j.procbio.2014.08.011
Qi, L. S., Larson, M. H., Gilbert, L. A., Doudna, J. A., Weissman, J. S., Arkin, A. P., et al. (2013). Repurposing CRISPR as an RNA-guided platform for sequence-specific control of gene expression. Cell 152, 1173–1183. doi:10.1016/j.cell.2013.02.022
Research, G. V. (2014a). Bio-Based Polyethylene Terephthalate (PET) Market by Application (Packaging (Bottles), Technical, Consumer Goods) and Segment Forecasts to 2020 Expected to Reach 5,800 Kilo Tons by 2020. Grand View Research. Available at: https://www.grandviewresearch.com/press-release/global-bio-based-polyethylene-terephthalate-pet. Accessed July 7, 2016.
Research, G. V. (2014b). Global Bio-Based Polyethylene Terephthalate (PET) Market. Available at: http://www.grandviewresearch.com/industry-analysis/bio-based-polyethylene-terephthalate-pet-industry. Accessed June, 2014.
Rodriguez, A., Chen, Y., Khoomrung, S., Özdemir, E., Borodina, I., and Nielsen, J. (2017a). Comparison of the metabolic response to over-production of p-coumaric acid in two yeast strains. Metab. Eng. 44, 265–272. doi:10.1016/j.ymben.2017.10.013
Rodriguez, A., Strucko, T., Stahlhut, S. G., Kristensen, M., Svenssen, D. K., Forster, J., et al. (2017b). Metabolic engineering of yeast for fermentative production of flavonoids. Bioresour. Technol. doi:10.1016/j.biortech.2017.06.043
Rodriguez, A., Kildegaard, K. R., Li, M., Borodina, I., and Nielsen, J. (2015). Establishment of a yeast platform strain for production of p-coumaric acid through metabolic engineering of aromatic amino acid biosynthesis. Metab. Eng. 31, 181–188. doi:10.1016/j.ymben.2015.08.003
Rodriguez, A., Martnez, J. A., Flores, N., Escalante, A., Gosset, G., and Bolivar, F. (2014). Engineering Escherichia coli to overproduce aromatic amino acids and derived compounds. Microb. Cell Fact. 13, 1–15. doi:10.1186/s12934-014-0126-z
Romagnoli, G., Knijnenburg, T. A., Liti, G., Louis, E. J., Pronk, J. T., and Daran, J.-M. (2015). Deletion of the Saccharomyces cerevisiae ARO8 gene, encoding an aromatic amino acid transaminase, enhances phenylethanol production from glucose. Yeast 32, 29–45. doi:10.1002/yea.3015
Santos, C. N. S., Xiao, W., and Stephanopoulos, G. (2012). Rational, combinatorial, and genomic approaches for engineering L-tyrosine production in Escherichia coli. Proc. Natl. Acad. Sci. U.S.A. 109, 13538–13543. doi:10.1073/pnas.1206346109
Schmidheini, T., Mosch, H. U., Graf, R., and Braus, G. H. (1990). A GCN4 protein recognition element is not sufficient for GCN4-dependent regulation of transcription in the ARO7 promoter of Saccharomyces cerevisiae. Mol. Gen. Genet. 224, 57–64. doi:10.1007/BF00259451
Schmidt, E., and Knackmuss, H.-J. (1984). Production of cis,cis-muconate from benzoate and 2-fluoro-cis,cis-muconate from 3-fluorobenzoate by 3-chlorobenzoate degrading bacteria. Appl. Microbiol. Biotechnol. 20, 351–355. doi:10.1007/BF00270599
Sengupta, S., Jonnalagadda, S., Goonewardena, L., and Juturu, V. (2015). Metabolic engineering of a novel muconic acid biosynthesis pathway via 4-hydroxybenzoic acid in Escherichia coli. Appl. Environ. Microbiol. 81, 8037–8043. doi:10.1128/AEM.01386-15
Shen, T., Liu, Q., Xie, X., Xu, Q., and Chen, N. (2012). Improved production of tryptophan in genetically engineered Escherichia coli with TktA and PpsA overexpression. J. Biomed. Biotechnol. 2012, 8. doi:10.1155/2012/605219
Siebert, M., Sommer, S., Li, S.-M., Wang, Z.-X., Severin, K., and Heide, L. (1996). Accumulation of 4-hydroxybenzoate glucosides as a result of the expression of the bacterial ubiC gene in tobacco. Plant Physiol. 112, 811–819. doi:10.1104/pp.112.2.811
Suástegui, M., Guo, W., Feng, X., and Shao, Z. (2016). Investigating strain dependency in the production of aromatic compounds in Saccharomyces cerevisiae. Biotechnol. Bioeng. 113, 2676–2685. doi:10.1002/bit.26037
Suástegui, M., and Shao, Z. (2016). Yeast factories for the production of aromatic compounds: from building blocks to plant secondary metabolites. J. Ind. Microbiol. Biotechnol. 43, 1611–1624. doi:10.1007/s10295-016-1824-9
Suástegui, M., Yu Ng, C., Chowdhury, A., Sun, W., Cao, M., House, E., et al. (2017). Multilevel engineering of the upstream module of aromatic amino acid biosynthesis in Saccharomyces cerevisiae for high production of polymer and drug precursors. Metab. Eng. 42, 134–144. doi:10.1016/j.ymben.2017.06.008
Sun, X., Lin, Y., Huang, Q., Yuan, Q., and Yan, Y. (2013). A novel muconic acid biosynthetic approach by shunting tryptophan biosynthesis via anthranilate. Appl. Environ. Microbiol. 79, 4024–4030. doi:10.1128/AEM.00859-13
Sun, X., Lin, Y., Yuan, Q., and Yan, Y. (2014). Biological production of muconic acid via a prokaryotic 2,3-dihydroxybenzoic acid decarboxylase. ChemSusChem 7, 2478–2481. doi:10.1002/cssc.201402092
Sun, Z., Ning, Y., Liu, L., Liu, Y., Sun, B., Jiang, W., et al. (2011). Metabolic engineering of the L-phenylalanine pathway in Escherichia coli for the production of S- or R-mandelic acid. Microb. Cell Fact. 10, 71–71. doi:10.1186/1475-2859-10-71
Sybesma, W., Starrenburg, M., Tijsseling, L., Hoefnagel, M. H., and Hugenholtz, J. (2003). Effects of cultivation conditions on folate production by lactic acid bacteria. Appl. Environ. Microbiol. 69, 4542–4548. doi:10.1128/AEM.69.8.4542-4548.2003
Thompson, B. (2017). Engineering Escherichia coli for the Novel and Enhanced Biosynthesis of Phenol, Catechol, and Muconic Acid. Tempe: Arizona State University.
Thompson, B., Machas, M., and Nielsen, D. R. (2015). Creating pathways towards aromatic building blocks and fine chemicals. Curr. Opin. Biotechnol. 36, 1–7. doi:10.1016/j.copbio.2015.07.004
Trenchard, I. J., Siddiqui, M. S., Thodey, K., and Smolke, C. D. (2015). De novo production of the key branch point benzylisoquinoline alkaloid reticuline in yeast. Metab. Eng. 31, 74–83. doi:10.1016/j.ymben.2015.06.010
Ullrich, S. F., Averesch, N. J. H., Castellanos, L., Choi, Y. H., Rothauer, A., and Kayser, O. (2016). Discrimination of wild types and hybrids of Duboisia myoporoides and Duboisia leichhardtii at different growth stages using 1H NMR-based metabolite profiling and tropane alkaloids-targeted HPLC-MS analysis. Phytochem. doi:10.1016/j.phytochem.2016.08.008
Ullrich, S. F., Hagels, H., and Kayser, O. (2017). Scopolamine: a journey from the field to clinics. Phytochem. Rev. 16, 333–353. doi:10.1007/s11101-016-9477-x
Van Duuren, J. B. J. H., and Wittmann, C. (2014). “First and second generation production of bio-adipic acid,” in Bioprocessing of Renewable Resources to Commodity Bioproducts, eds V. S. Bisaria, and A. Kondo (John Wiley & Sons, Inc), 584.
Van Dyk, T. K., Templeton, L. J., Cantera, K. A., Sharpe, P. L., and Sariaslani, F. S. (2004). Characterization of the Escherichia coli AaeAB efflux pump: a metabolic relief valve? J. Bacteriol. 186, 7196–7204. doi:10.1128/JB.186.21.7196-7204.2004
Vardon, D. R., Rorrer, N. A., Salvachua, D., Settle, A. E., Johnson, C. W., Menart, M. J., et al. (2016). cis,cis-Muconic acid: separation and catalysis to bio-adipic acid for nylon-6,6 polymerization. Green Chem. doi:10.1039/C5GC02844B
Verhoef, S., Ballerstedt, H., Volkers, R. J. M., Winde, J. H., and Ruijssenaars, H. J. (2010). Comparative transcriptomics and proteomics of p-hydroxybenzoate producing Pseudomonas putida S12: novel responses and implications for strain improvement. Appl. Microbiol. Biotechnol. 87, 679–690. doi:10.1007/s00253-010-2626-z
Wang, J., Shen, X., Rey, J., Yuan, Q., and Yan, Y. (2018). Recent advances in microbial production of aromatic natural products and their derivatives. Appl. Microbiol. Biotechnol. 102, 47–61. doi:10.1007/s00253-017-8599-4
Wang, J., and Zheng, P. (2015). Muconic acid production from glucose using enterobactin precursors in Escherichia coli. J. Ind. Microbiol. Biotechnol. 42, 701–709. doi:10.1007/s10295-014-1581-6
Weber, C., Bruckner, C., Weinreb, S., Lehr, C., Essl, C., and Boles, E. (2012). Biosynthesis of cis,cis-muconic acid and its aromatic precursors, catechol and protocatechuic acid, from renewable feedstocks by Saccharomyces cerevisiae. Appl. Environ. Microbiol. 78, 8421–8430. doi:10.1128/AEM.01983-12
Webster, P. (2012). A Market Overview on Bio-Based Fuels and Chemicals. CPI, Wilton Centre: Arthur D Little. Available at: http://www.soci.org/~/media/Files/Conference%20Downloads/2012/Processing%20Lignocellulosic%20Biomass%20Nov%202012/Phil_Webster__ADL_biofuels_and_biobased_chemicals_CPI_081112.ashx
Williams, T. C., Averesch, N. J., Winter, G., Plan, M. R., Vickers, C. E., Nielsen, L. K., et al. (2015). Quorum-sensing linked RNA interference for dynamic metabolic pathway control in Saccharomyces cerevisiae. Metab. Eng. 29, 124–134. doi:10.1016/j.ymben.2015.03.008
Winter, G., Averesch, N. J., Nunez-Bernal, D., and Krömer, J. O. (2014). In vivo instability of chorismate causes substrate loss during fermentative production of aromatics. Yeast 31, 333–341. doi:10.1002/yea.3025
Woodley, J. M. (2017). Bioprocess intensification for the effective production of chemical products. Comput. Chem. Eng. 105, 297–307. doi:10.1016/j.compchemeng.2017.01.015
Xie, N.-Z., Liang, H., Huang, R.-B., and Xu, P. (2014). Biotechnological production of muconic acid: current status and future prospects. Biotechnol. Adv. 32, 615–622. doi:10.1016/j.biotechadv.2014.04.001
Yu, S., Plan, M. R., Winter, G., and Krömer, J. O. (2016). Metabolic engineering of Pseudomonas putida KT2440 for the production of para-hydroxy benzoic acid. Front. Bioeng. Biotechnol. 4:90. doi:10.3389/fbioe.2016.00090
Zhang, C., Kang, Z., Zhang, J., Du, G., Chen, J., and Yu, X. (2014a). Construction and application of novel feedback-resistant 3-deoxy-d-arabino-heptulosonate-7-phosphate synthases by engineering the N-terminal domain for l-phenylalanine synthesis. FEMS Microbiol. Lett. 353, 11–18. doi:10.1111/1574-6968.12397
Zhang, H., Cao, M., Jiang, X., Zou, H., Wang, C., Xu, X., et al. (2014b). De-novo synthesis of 2-phenylethanol by Enterobacter sp. CGMCC 5087. BMC Biotechnol. 14:1–7. doi:10.1186/1472-6750-14-30
Zhang, H., Pereira, B., Li, Z., and Stephanopoulos, G. (2015). Engineering Escherichia coli coculture systems for the production of biochemical products. Proc. Natl. Acad. Sci. U.S.A. 112, 8266–8271. doi:10.1073/pnas.1506781112
Keywords: Shikimate pathway, metabolic engineering, aromatics, metabolic modelling, strain construction
Citation: Averesch NJH and Krömer JO (2018) Metabolic Engineering of the Shikimate Pathway for Production of Aromatics and Derived Compounds—Present and Future Strain Construction Strategies. Front. Bioeng. Biotechnol. 6:32. doi: 10.3389/fbioe.2018.00032
Received: 14 December 2017; Accepted: 12 March 2018;
Published: 26 March 2018
Edited by:
Xiao-Jun Ji, Nanjing Tech University, ChinaReviewed by:
Quanli Liu, Chalmers University of Technology, SwedenXixian Xie, Tianjin University of Science and Technology, China
Copyright: © 2018 Averesch and Krömer. This is an open-access article distributed under the terms of the Creative Commons Attribution License (CC BY). The use, distribution or reproduction in other forums is permitted, provided the original author(s) and the copyright owner are credited and that the original publication in this journal is cited, in accordance with accepted academic practice. No use, distribution or reproduction is permitted which does not comply with these terms.
*Correspondence: Nils J. H. Averesch, bmlscy5hdmVyZXNjaEB1cS5uZXQuYXU=