- 1Istituto di Ricerca Genetica e Biomedica (IRGB), Consiglio Nazionale delle Ricerche (CNR), Milan Unit, Milan, Italy
- 2Human Genome Laboratory, Humanitas Clinical and Research Institute, Rozzano, Milan, Italy
- 3Department of Medical Biotechnologies and Translational Medicine, University of Milan, Milan, Italy
Mesenchymal stem cells (MSCs) are multipotent stromal cells that are identified by in vitro plastic adherence, colony-forming capacity, expression of a panel of surface molecules, and ability to differentiate at least toward osteogenic, adipogenic, and chondrogenic lineages. They also produce trophic factors with immunomodulatory, proangiogenic, and antiapoptotic functions influencing the behavior of neighboring cells. On the other hand, a reciprocal regulation takes place; in fact, MSCs can be isolated from several tissues, and depending on the original microenvironment and the range of stimuli received from there, they can display differences in their essential characteristics. Here, we focus mainly on the bone tissue and how soluble factors, such as growth factors, cytokines, and hormones, present in this microenvironment can orchestrate bone marrow-derived MSCs fate. We also briefly describe the alteration of MSCs behavior in pathological settings such as hematological cancer, bone metastasis, and bone marrow failure syndromes. Overall, the possibility to modulate MSCs plasticity makes them an attractive tool for diverse applications of tissue regeneration in cell therapy. Therefore, the comprehensive understanding of the microenvironment characteristics and components better suited to obtain a specific MSCs response can be extremely useful for clinical use.
Introduction
Great attention has been recently paid to the characterization and biomedical applications of multipotent adult stem cells present in the stromal compartment of several post-natal tissues, the “mesenchymal stem cells” (MSCs). MSCs, identified in bone tissue as precursor cells of osteoblasts\osteocytes, chondrocytes, and marrow adipocytes, are defined as multipotent cells that can be easily isolated from the stromal fraction. MSCs exhibit in vitro plastic adherence, fibroblast spindle-like shaped morphology, and expression of a panel of surface molecules that is continuously refined to identify unique markers for bona fide MSCs definition (Bourin et al., 2013; Schena et al., 2017). MSCs possess self-renewal and clonogenic capacity and highly proliferate and differentiate at least toward the osteogenic, adipogenic, and chondrogenic lineages both in vitro, by means of specific differentiation media, and in vivo in an ectopic bone formation assay (Schena et al., 2017). In bone, MSCs are located around sinusoids and along the perivascular network in the stroma (Sacchetti et al., 2007; Mendez-Ferrer et al., 2010), where they take part in the generation of the complex and heterogeneous system of the bone marrow microenviroment (BM-ME). In fact, MSCs together with pericytes, adventitial cells, endothelial cells, fibroblasts, marrow adipocytes, and hematopoietic and immune cells generate a dynamic compartment by establishing cell-to-cell interactions and producing soluble factors with autocrine and paracrine functions (Moore and Lemischka, 2006; Bianco et al., 2013). Many reports in literature deal with the MSCs’ secretome, i.e., the variety of factors released by MSCs in physiopathological conditions. For example, MSCs exert immunomodulatory properties on innate and adaptive immune cells by sensing inflammatory environments (Bernardo and Fibbe, 2013) and secreting pro- and anti-inflammatory chemokines (Keating, 2012; Le Blanc and Mougiakakos, 2012).
Moreover, MSCs organize the vascular network, since they interact with endothelial and hematopoietic cells by producing or responding to different molecules (e.g., VEGF, FGF-2, PDGF-α, and TGF-β1) (Jain, 2003; Sacchetti et al., 2007) and synthesize antiapoptotic factors (e.g., HGF and IGF1) in pathological conditions (Nagaya et al., 2005; Kennelly et al., 2016). MSCs also exert supportive functions for hematopoietic stem cells (HSC), thanks to direct cell-to-cell contact and secreted trophic molecules, e.g., jagged 1 and BMPs (Calvi et al., 2003; Zhang et al., 2003; He et al., 2017). Furthermore, they modulate osteoclast formation, survival, and resorptive activity through positive and negative regulatory molecules, among which RANKL and OPG are the iconic ones (Sharaf-Eldin et al., 2016). Finally, MSCs differentiation and secretory activities are relevant in skeletal pathologies such as multiple myeloma (MM), bone metastases, and bone marrow failure syndromes (BMFS), and their capacity to support and/or regulate hematopoiesis and cancer cells survival has been extensively described (Mundy, 2002; Kassen et al., 2014; David Roodman and Silbermann, 2015; Fairfield et al., 2016).
From the opposite perspective, neighboring cells or cells residing in other tissues in turn provide stimuli influencing MSCs properties in physiopathological conditions.
Here, we exactly aim to take this latter point of view and to provide some examples of soluble factors present in BM-ME that are able to direct MSCs fate and orchestrate their cellular response. MSCs secretome (Murphy et al., 2013) and plasticity make them an attractive tool for biomedical applications, such as tissue regeneration and cell-based therapy for several diseases. The capacity to modulate functional properties of MSCs is essential for their optimal exploitation in clinical practice. To this final goal, a wider understanding of the variety of molecular and cellular interactions in BM-ME is of paramount importance.
Microenvironment Factors Orchestrate MSCs Fate
The intense cellular interactions in the BM make this microenvironment a dynamic compartment where several soluble factors are able to modulate MSC functions. Here, we will describe some of these molecules, their signaling pathways (Figure 1), and their final effect on MSC fate (Figure 2).
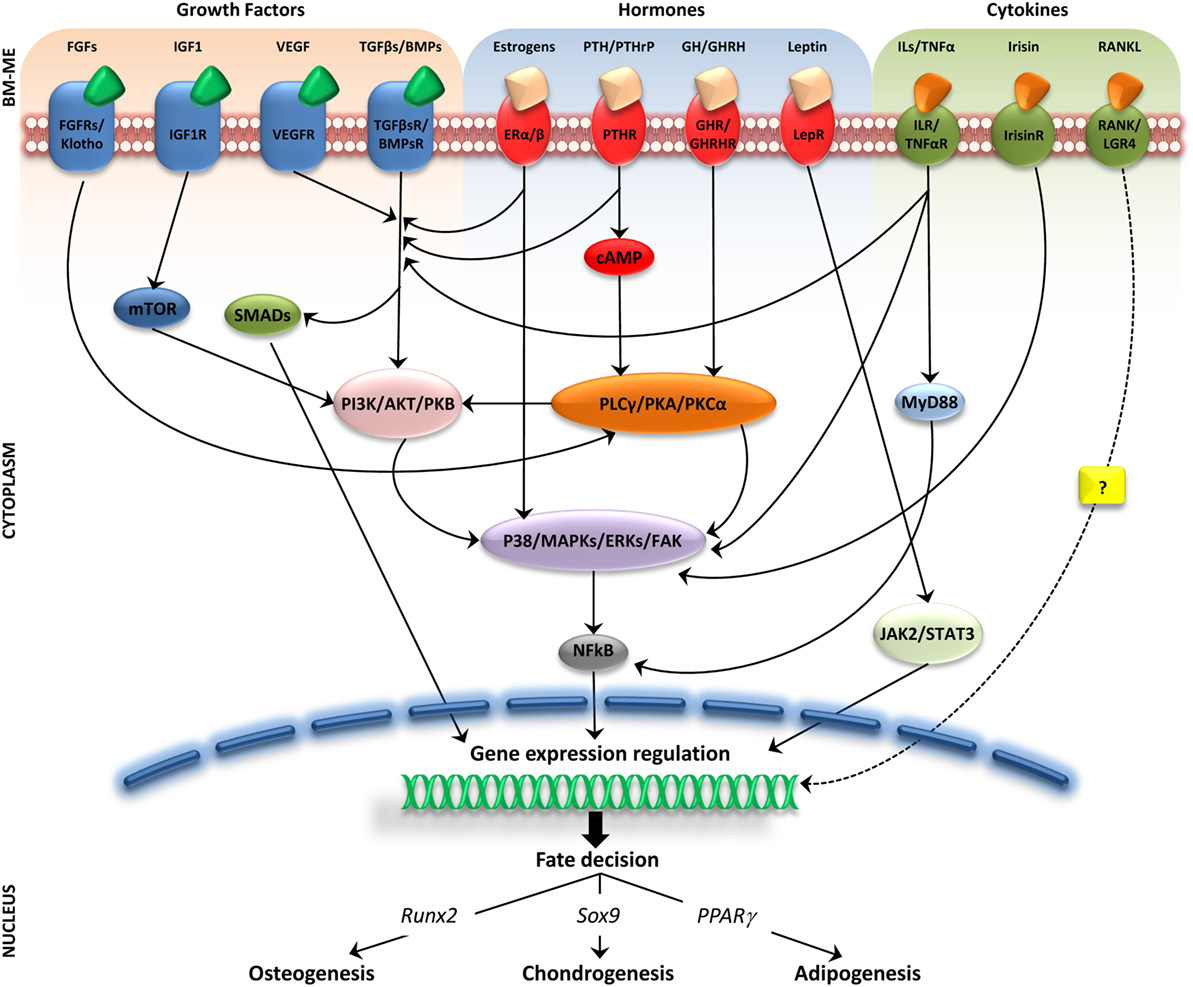
Figure 1. Molecular pathways activated by soluble factors influencing bone marrow mesenchymal stem cells (MSCs) differentiation. Simplified representation of cellular players in bone marrow microenviroment (BM-ME) showing that growth factors, hormones, and cytokines, by binding to their respective receptors on the MSC plasma membrane, trigger activation of signaling cascades that ultimately result in gene expression regulation relevant for MSCs differentiation fate.
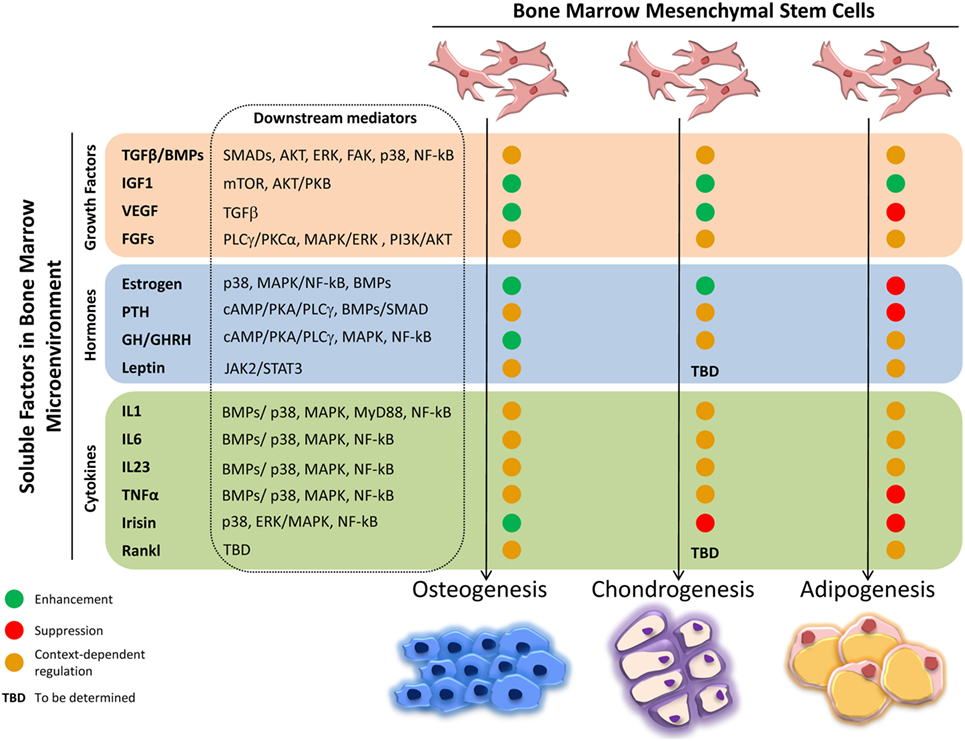
Figure 2. Effects of soluble factors on bone marrow mesenchymal stem cells (MSCs) trilineage differentiation. Schematic representation of the effect exerted by the described soluble molecules on MSCs osteogenic, chondrogenic, and adipogenic differentiation. Green circle, enhancement; red circle, suppression; orange circle, context-dependent regulation; TBD, to be determined.
Growth Factors (GFs)
In general, GFs influence MSCs acting both in a paracrine and autocrine manner; in fact, MSCs express on their surface GF receptors.
Proteins of the TGFβ/BMP superfamily are the most abundant GFs in BM-ME and originate mainly from bone matrix degradation and activated T cells (Tang et al., 2009; Croes et al., 2016; Li et al., 2016). The TGFβ family comprises three members: TGFβ1, TGFβ2, and TGFβ3. TGFβ1, released from the bone matrix by the osteoclast resorptive activity, has been demonstrated in vitro and in vivo to induce MSCs migration to the remodeling sites, thus coupling bone formation and resorption. The mechanism through which this function is exerted, whether through the canonical signaling pathway, involving SMADs, or the non-canonical one, involving AKT, ERK1/2, FAK, and p38, is debated (Tang et al., 2009; Dubon et al., 2017). Moreover, in vitro TGFβ1 drives MSCs fate toward osteoblasts generation and inhibits adipogenic differentiation; accordingly, TGFβ1 induces the switching from adipogenesis to osteogenesis when added to an adipogenic medium, acting mainly on the SMAD/C/EBPs/PPARγ signaling (Choy and Derynck, 2003; van Zoelen et al., 2016). In other reports, during in vitro MSC expansion, TGFβ1 reduces the number of osteoprogenitor cells and limits their expansion inducing a rapid terminal differentiation, suggesting that TGFβ1 effects on MSCs might depend on the commitment state of the cells (Walsh et al., 2003; Claros et al., 2014). Furthermore, TGFβ1 is a key molecule in chondrogenesis by stabilizing SOX9 via the canonical SMAD or the non-canonical p38 pathways (Coricor and Serra, 2016; Dexheimer et al., 2016). A similar potent effect on chondrogenic differentiation has been demonstrated for TGFβ2 and TGFβ3 (Vinatier et al., 2009).
Furthermore, TGFβ1 induces ADAM12 expression in MSCs/pericytes triggering myofibroblast transdifferentiation and contributing to fibrosis (Cipriani et al., 2016).
The BMP family comprises at least 15 members, which usually exert synergistic effects with TGFβs and activate SMAD transcription factors and expression of genes such as Runx2, Pparγ, or Sox9. This ultimately results in the promotion of MSCs differentiation toward the adipogenic (e.g., BMP2, BMP4, and BMP7), osteogenic (e.g., BMP2, BMP6, and BMP9), or chondrogenic (e.g., BMP2 and BMP7) lineage, depending on the microenvironmental concentrations (Kang et al., 2009; Chen et al., 2012).
IGF1, a polypeptide with a high-binding affinity to IGF1R and insulin-like features (Wang et al., 2013), is one of the most abundant GFs deposited in the bone matrix. In vitro and in vivo in mouse and rat models, IGF1 released from bone matrix degradation induces osteoblast differentiation via the mTOR pathway and enhances osteoblasts function (Xian et al., 2012; Crane and Cao, 2014). Regarding chondrogenesis, IGF1 in combination with TGFβs enhances in vitro chondrocytes proliferation and collagen II production (Fukumoto et al., 2003; Indrawattana et al., 2004), while for the adipogenic fate commitment, IGF1 activates IGF1R-dependent AKT/PKB signaling, increasing Pparγ expression and lipid accumulation (Scavo et al., 2004).
VEGF is a key soluble molecule involved in endothelial cell proliferation, migration, and tissue vascularization (Ferrara et al., 2003). MSCs/osteoblasts themselves express VEGF and its receptors, so this GF may exert both a paracrine and an autocrine regulation (Niida et al., 1999; Nakagawa et al., 2000; Kaigler et al., 2003; Yang et al., 2008; Liu and Olsen, 2014; Marsano et al., 2016). VEGF is essential for coupling angiogenesis to bone formation during skeletal development, by promoting chondrocytes survival in hypoxic regions of cartilaginous templates, vascularization of developing bones, and proliferation and differentiation of osteoblasts (Maes et al., 2004; Zelzer et al., 2004). In post-natal bone homeostasis, VEGF favors MSCs osteoblastogenesis at the expense of adipogenesis, through intracrine regulation of Runx2 and Pparγ (Liu et al., 2012), while intracellular blockade of VEGF signaling in MSCs activates TGFβ signaling, thus inducing spontaneous in vivo chondrogenesis and formation of a hypoxic microenvironment and a stable hyaline cartilage (Marsano et al., 2016).
In FGF family, many members positively regulate MSCs proliferation and osteogenic differentiation, by interacting with FGFR2 and activating PLCγ/PKCα, MAPK/ERK 1/2, and PI3K/AKT pathways (Marie, 2012). FGFs are also crucial for the regulation of MSCs chondrogenic differentiation through FGF/FGFR3, as demonstrated by their involvement in the pathogenesis of different forms of chondrodysplasia (Ornitz and Legeai-Mallet, 2017). However, also for these GFs, results in literature are discordant: recent data indicate that FGF1 and FGF2 maintain MSCs in an uncommitted state, preventing their differentiation (Le Blanc et al., 2015; Simann et al., 2017). Finally, the hormone-like FGF23, produced by bone cells and by cells of different tissues, favors osteogenic differentiation at the expenses of adipogenesis by binding to its receptor Klotho, which mediates the activation of MAPKs signaling (Li et al., 2013c).
Hormones
The skeleton is widely recognized as both an endocrine organ and a target for other endocrine tissues (Fukumoto and Martin, 2009).
The prototypical example of MSCs-regulating hormone is estrogens, the main molecules involved in post-menopausal osteoporosis. Estrogens bind their α and/or β receptors and induce MSCs proliferation and osteogenic and chondrogenic differentiation (Rodriguez et al., 2008), through the activation of BMPs/WNT/β-catenin and p38 MAPKs/NF-κB signaling pathways (Gopalakrishnan et al., 2006; Li et al., 2013b; Kim et al., 2015; Cong et al., 2016). Moreover, estrogens induce early osteoblast differentiation and inhibit adipogenesis in mice (Okazaki et al., 2002). Furthermore, they reduce LPL levels, impair adipocyte progression into hypertrophic state, and influence body adipose tissue depots distribution and glucose metabolism (Post et al., 2008). Overall, this evidence points to a role of estrogens in regulating both bone and glucose homeostasis.
PTH is one of the principal modulator of calcium homeostasis through cAMP/PKA/PLCγ signaling. It also displays both catabolic and anabolic functions in bone remodeling: the former is exerted by inducing RANKL production, which fosters osteoclasts’ generation and activity, the latter by affecting MSCs fate (Hock and Gera, 1992; Qin et al., 2004). Indeed, PTH induces in vitro osteogenic differentiation via LRP6-dependent BMP/SMAD signaling (Polo and Di Fiore, 2006; Jilka, 2007; Qiu et al., 2010; Yu et al., 2012). Accordingly, in vivo deletion of PTHR in murine MSCs reduces bone formation and increases bone resorption and marrow adiposity, while intermittent PTH administration to control mice reduces marrow adipogenesis (Fan et al., 2017). On the contrary, PTHrP affects MSCs differentiation, preventing chondrocyte hypertrophy and blocking osteogenesis through regulation of Sox9 and Runx2 gene expressions (Provot et al., 2006; Zhang et al., 2009; Fischer et al., 2014).
GH regulates linear growth during development (Gomes et al., 2013; Ma et al., 2016) and is involved in BM adiposity maintenance. It enhances adipocytes and osteoblast precursor pool size, while it induces MSCs osteogenesis and inhibits BM fat accumulation (Menagh et al., 2010). Its regulator, GHRH, has receptors (GHRHR) also on MSCs (Gomes et al., 2013; Ma et al., 2016) and through their binding promotes MSCs proliferation and survival via the cAMP/PKA/PLCγ signaling, activates MAPK signals, and induces osteogenic differentiation (Jaiswal et al., 2000; Xia et al., 2016).
Finally, the adipose tissue-derived hormone leptin contributes to guide MSCs commitment but contradictory results are reported (Ducy et al., 2000; Kontogianni et al., 2004; La Cava and Matarese, 2004). MSCs highly express leptin receptor (Zhou et al., 2014), and in vitro, leptin enhances osteogenic differentiation and reduces the adipogenic one (Thomas, 2004). On the contrary, leptin in vivo regulates MSCs, increasing marrow adipogenesis and reducing osteogenesis in response to diet and adiposity, through the JAK2/STAT3 pathway (Yue et al., 2016).
Cytokines
Among the cell populations present in BM-ME, immune cells participate in directing MSCs fate by secreting a variety of cytokines, with anabolic or anti-anabolic effects depending on the inflammatory state of the bone tissue.
For example, T cells activate bone formation by producing Wnt ligands that initiate Wnt signaling and osteoblastogenesis (Ouji et al., 2006; Terauchi et al., 2009). They also produce CD40L that binds CD40 on MSCs inducing their proliferation and survival (Ahuja et al., 2003; Gao et al., 2008; Li et al., 2013a).
Conflicting results are reported regarding the effects of proinflammatory cytokine on MSCs differentiation. In a proinflammatory environment, the interleukins IL-1β, IL-6, and IL-23 (mainly derived from Th17 cells) have been reported to increase the differentiation performance of human MSC toward the osteogenic and adipogenic lineages (Pourgholaminejad et al., 2016). Accordingly, TNFα, IL-1β, and IL-6 enhance osteoblast differentiation by triggering NF-κB signaling or modulating BMP2 pathway (Nakase et al., 1997; Hess et al., 2009; Huh and Lee, 2013; Croes et al., 2015).
On the contrary, IL-1 and TNFα inhibit MSCs osteogenesis and adipocyte generation, acting not only through the canonical NF-κB signaling (Lacey et al., 2009; Sullivan et al., 2014) but also via other mechanisms such as IL-1R1/MyD88 signal transduction, as described for murine MSCs obtained from different genetic backgrounds (Martino et al., 2016). Similarly, IL-6 can impair MSCs ability to generate adipocytes and chondrocytes and keep them in an undifferentiated state by activating ERK1/2 (Pricola et al., 2009).
Recently, great attention has been paid to the skeletal muscle-derived myokine Irisin, which is able to directly target the bone tissue, thus regulating its physiology. Specifically, Irisin can induce MSCs osteoblast differentiation through p38/ERK MAPK signaling pathways, leading to the upregulation of osteogenic marker genes, such as Atf4, Runx2, Osx, Lrp5, β-catenin, Alp, and Col1a1 (Colaianni et al., 2015; Qiao et al., 2016).
RANKL Involvement in MSCs Fate Decision
RANKL is the essential osteoclastogenic factor produced mainly by MSCs, osteoblasts, and osteocytes (Sobacchi et al., 2007, 2013; Nakashima et al., 2011) and also by T cells in the bone marrow (Pacifici, 2016a,b). The possibility that RANKL might be an additional factor in BM-ME influencing MSCs properties has been considered only lately. In fact, recent reports indicate that RANKL might have bone anabolic effects when pulsed or low doses of the cytokine are administered to ovariectomized mice (Buchwald et al., 2015; Cline-Smith et al., 2016). In line with these observations, our group has found that BM-MSCs derived from RANKL-deficient mice display a partial osteogenic differentiation defect, which is improved by restoring the production of the soluble form of the cytokine. Our data suggest that RANKL might contribute to direct MSCs fate in an autocrine/paracrine manner, likely through the interaction with either its receptor RANK (Schena et al., 2017) or the recently identified RANKL receptor LGR4 (Luo et al., 2016) (an R-spondin receptor, suggested to regulate bone formation in synergy with Wnt3a), which are both expressed in MSCs (Schena et al., 2017). On this basis, we might speculate that fine tuning, rather than completely blocking, RANKL could be relevant to regulate bone physiology.
MSCs Fate in Pathological Conditions
Multiple Myeloma
Multiple myeloma is a common hematological malignancy mainly characterized by osteolytic lesions due to increased osteoclast number and activity and strongly decreased bone formation (Kassen et al., 2014). MSCs and osteoblasts support MM cells survival, proliferation, and progression (Azab et al., 2009; Reagan et al., 2014; Roccaro et al., 2014; Fairfield et al., 2016), while osteogenic differentiation is reduced in MM patients, which might be a putative strategy of MM cells to preserve cells (e.g., MSCs) necessary for their support (Corre et al., 2007; Reagan et al., 2014). Cell-to-cell contact and production of soluble factors are likely involved in these mechanisms. For example, MM cells inhibit Runx2 and inactivate the non-canonical Wnt5a/Ror2 pathway; a putative role of IL-7 produced by MM cells can be hypothesized (Giuliani et al., 2005; D’Souza et al., 2011; Bolzoni et al., 2013). Moreover, MM cells secrete Wnt inhibitory factors, i.e., Dkk1 and sclerostin; TGFβ, which impairs osteoblast differentiation (Lee et al., 2003; Tian et al., 2003; Colucci et al., 2011); and also factors inducing MSCs growth that, in turn, produce osteoclast-activating factors (i.e., IL-6, MCSF, TNFα, and RANKL) leading to osteolysis (David Roodman and Silbermann, 2015).
Breast Cancer (BC) and Prostate Cancer (PC)
Breast cancer cells preferably metastasize to bone inducing purely osteolytic lesions, via the production of osteoclast-activating factors (mainly RANKL and MCSF). Furthermore, osteolytic lesions are production sites of several soluble factors derived from osteoclasts’ resorption of the bone matrix, such as TGFβ (Kang et al., 2003). These molecules can inhibit osteoblast development and functions and are able to induce BC cell proliferation and progression that, in turn, sustain the secretion of osteoblast inhibitory factors (Mundy, 2002).
Bone metastases in PC tend to be osteosclerotic, rather than osteolytic. PC cells produce soluble factors, e.g., BMPs, TGFβ, IGF1, FGFs, and VEGF, which increase MSCs osteogenic differentiation, osteoblast development, and bone deposition, leading to elevated mineral apposition, even though the newly formed bone is immature and of poor quality (Guise et al., 2006; David Roodman and Silbermann, 2015). Of note, the osteoclast-inducing hormone PTHrP, above reported as osteogenic inhibitor, is highly produced by PC cells and in this context enhances osteoblast progenitors’ proliferation and early osteogenesis (Liao et al., 2008).
Bone Marrow Failure Syndromes
Bone marrow failure syndromes are hematological disorders characterized by impaired hematopoiesis comprising different phenotypes, i.e., myelodysplastic syndromes (MDS), aplastic anemia (AA), and chronic idiopathic neutropenia (CIN). MSCs play an important role in maintaining and restoring hematopoiesis, thanks to the secretion of regulatory factors for HSC functionality (Kastrinaki et al., 2013). Scanty data are available the other way round. For example in AA, MSCs osteogenic capacity is inhibited in favor of adipogenesis (Papadaki et al., 2001; Shipounova et al., 2009; Xu et al., 2009). Recently, oncostatin M, a member of the IL-6 family, has been reported to stimulate HSC expansion, inhibiting adipogenic differentiation and enhancing osteogenic differentiation of MSCs. Upon administration in mice bearing BM injury, it decreases marrow adipogenesis and restores HSC number (Sato et al., 2014).
Conclusion
Bone marrow microenviroment is constituted by many diverse cell types, which establish an intense cross-talk among them. In particular, in recent years, MSCs have gained great attention for their trophic support to other cells, ability to secrete bioactive factors and plasticity, and for the possibility to be exploited in regenerative medicine applications. On the other hand, the outcome of much experimentation has failed to meet the forecasted expectations. The real challenge that still has to be faced is the global understanding of the cellular and molecular mechanisms, which take place in BM-ME and star MSCs as main character or as target.
This review gives just a flavor of the variety of soluble factors provided by neighboring cells, by the ECM or by other tissues influencing MSCs properties in pathophysiological settings. Many of these factors may elicit opposite MSCs behavior depending on the overall environmental conditions, as demonstrated by the controversial results reported in literature. Furthermore, the signaling pathways activated downstream each ligand/receptor interaction often intersect and then intertwine or diverge, thus generating an additional layer of complexity. The recent highlight on RANKL as a putative novel regulator of MSCs fate raises the possibility that additional factors involved in orchestrating MSCs functions have still to be recognized. A wider landscape of molecular and cellular interactions and of rules to be accomplished or modified to elicit specific cell behaviors needs to be reached. This deep understanding will improve the capacity to manipulate BM-ME and to effectively use MSCs for cell therapy.
Author Contributions
All the authors contributed to organize, draft, and revise the manuscript.
Conflict of Interest Statement
The authors declare that the research was conducted in the absence of any commercial or financial relationships that could be construed as a potential conflict of interest.
Acknowledgments
We acknowledge the many authors whose original contribution in the field could not be cited in this minireview for the sake of brevity.
Funding
This work was partially supported by the European Community’s Seventh Framework Program (FP7/2007-2013, SYBIL Project), by PRIN projects (20102M7T8X_003 and 2015F3JHMB_004) and by Programma Nazionale per la Ricerca – Consiglio Nazionale delle Ricerche Aging Project to AV, and by Ministero della Salute – Giovani Ricercatori (grants GR-2008-1134625 and GR-2011-02348266) and by Telethon (grant GGP12178) to CS, and by the Italian Society for Osteoporosis, Mineral Metabolism and Skeleton Diseases (SIOMMMS) grant to CM.
References
Ahuja, S. S., Zhao, S., Bellido, T., Plotkin, L. I., Jimenez, F., and Bonewald, L. F. (2003). CD40 ligand blocks apoptosis induced by tumor necrosis factor alpha, glucocorticoids, and etoposide in osteoblasts and the osteocyte-like cell line murine long bone osteocyte-Y4. Endocrinology 144, 1761–1769. doi: 10.1210/en.2002-221136
Azab, A. K., Runnels, J. M., Pitsillides, C., Moreau, A. S., Azab, F., Leleu, X., et al. (2009). CXCR4 inhibitor AMD3100 disrupts the interaction of multiple myeloma cells with the bone marrow microenvironment and enhances their sensitivity to therapy. Blood 113, 4341–4351. doi:10.1182/blood-2008-10-186668
Bernardo, M. E., and Fibbe, W. E. (2013). Mesenchymal stromal cells: sensors and switchers of inflammation. Cell Stem Cell 13, 392–402. doi:10.1016/j.stem.2013.09.006
Bianco, P., Cao, X., Frenette, P. S., Mao, J. J., Robey, P. G., Simmons, P. J., et al. (2013). The meaning, the sense and the significance: translating the science of mesenchymal stem cells into medicine. Nat. Med. 19, 35–42. doi:10.1038/nm.3028
Bolzoni, M., Donofrio, G., Storti, P., Guasco, D., Toscani, D., Lazzaretti, M., et al. (2013). Myeloma cells inhibit non-canonical wnt co-receptor ror2 expression in human bone marrow osteoprogenitor cells: effect of wnt5a/ror2 pathway activation on the osteogenic differentiation impairment induced by myeloma cells. Leukemia 27, 451–463. doi:10.1038/leu.2012.190
Bourin, P., Bunnell, B. A., Casteilla, L., Dominici, M., Katz, A. J., March, K. L., et al. (2013). Stromal cells from the adipose tissue-derived stromal vascular fraction and culture expanded adipose tissue-derived stromal/stem cells: a joint statement of the International Federation for Adipose Therapeutics and Science (IFATS) and the International Society for Cellular Therapy (ISCT). Cytotherapy 15, 641–648. doi:10.1016/j.jcyt.2013.02.006
Buchwald, Z. S., Yang, C., Nellore, S., Shashkova, E. V., Davis, J. L., Cline, A., et al. (2015). A bone anabolic effect of RANKL in a murine model of osteoporosis mediated through FoxP3+ CD8 T cells. J. Bone Miner. Res. 30, 1508–1522. doi:10.1002/jbmr.2472
Calvi, L. M., Adams, G. B., Weibrecht, K. W., Weber, J. M., Olson, D. P., Knight, M. C., et al. (2003). Osteoblastic cells regulate the haematopoietic stem cell niche. Nature 425, 841–846. doi:10.1038/nature02040
Chen, G., Deng, C., and Li, Y. P. (2012). TGF-beta and BMP signaling in osteoblast differentiation and bone formation. Int. J. Biol. Sci. 8, 272–288. doi:10.7150/ijbs.2929
Choy, L., and Derynck, R. (2003). Transforming growth factor-beta inhibits adipocyte differentiation by Smad3 interacting with CCAAT/enhancer-binding protein (C/EBP) and repressing C/EBP transactivation function. J. Biol. Chem. 278, 9609–9619. doi:10.1074/jbc.M212259200
Cipriani, P., Di Benedetto, P., Ruscitti, P., Liakouli, V., Berardicurti, O., Carubbi, F., et al. (2016). Perivascular cells in diffuse cutaneous systemic sclerosis overexpress activated ADAM12 and are involved in myofibroblast transdifferentiation and development of fibrosis. J. Rheumatol. 43, 1340–1349. doi:10.3899/jrheum.150996
Claros, S., Rico-Llanos, G. A., Becerra, J., and Andrades, J. A. (2014). A novel human TGF-beta1 fusion protein in combination with rhBMP-2 increases chondro-osteogenic differentiation of bone marrow mesenchymal stem cells. Int. J. Mol. Sci. 15, 11255–11274. doi:10.3390/ijms150711255
Cline-Smith, A., Gibbs, J., Shashkova, E., Buchwald, Z. S., Novack, D. V., and Aurora, R. (2016). Pulsed low-dose RANKL as a potential therapeutic for postmenopausal osteoporosis. JCI Insight 1, e88839. doi:10.1172/jci.insight.88839
Colaianni, G., Cuscito, C., Mongelli, T., Pignataro, P., Buccoliero, C., Liu, P., et al. (2015). The myokine irisin increases cortical bone mass. Proc. Natl. Acad. Sci. U.S.A. 112, 12157–12162. doi:10.1073/pnas.1516622112
Colucci, S., Brunetti, G., Oranger, A., Mori, G., Sardone, F., Specchia, G., et al. (2011). Myeloma cells suppress osteoblasts through sclerostin secretion. Blood Cancer J. 1, e27. doi:10.1038/bcj.2011.22
Cong, Q., Jia, H., Biswas, S., Li, P., Qiu, S., Deng, Q., et al. (2016). p38alpha MAPK regulates lineage commitment and OPG synthesis of bone marrow stromal cells to prevent bone loss under physiological and pathological conditions. Stem Cell Reports 6, 566–578. doi:10.1016/j.stemcr.2016.02.001
Coricor, G., and Serra, R. (2016). TGF-beta regulates phosphorylation and stabilization of Sox9 protein in chondrocytes through p38 and Smad dependent mechanisms. Sci. Rep. 6, 38616. doi:10.1038/srep38616
Corre, J., Mahtouk, K., Attal, M., Gadelorge, M., Huynh, A., Fleury-Cappellesso, S., et al. (2007). Bone marrow mesenchymal stem cells are abnormal in multiple myeloma. Leukemia 21, 1079–1088. doi:10.1038/sj.leu.2404621
Crane, J. L., and Cao, X. (2014). Function of matrix IGF-1 in coupling bone resorption and formation. J. Mol. Med. (Berl) 92, 107–115. doi:10.1007/s00109-013-1084-3
Croes, M., Oner, F. C., Kruyt, M. C., Blokhuis, T. J., Bastian, O., Dhert, W. J., et al. (2015). Proinflammatory mediators enhance the osteogenesis of human mesenchymal stem cells after lineage commitment. PLoS ONE 10:e0132781. doi:10.1371/journal.pone.0132781
Croes, M., Oner, F. C., van Neerven, D., Sabir, E., Kruyt, M. C., Blokhuis, T. J., et al. (2016). Proinflammatory T cells and IL-17 stimulate osteoblast differentiation. Bone 84, 262–270. doi:10.1016/j.bone.2016.01.010
David Roodman, G., and Silbermann, R. (2015). Mechanisms of osteolytic and osteoblastic skeletal lesions. Bonekey Rep. 4, 753. doi:10.1038/bonekey.2015.122
Dexheimer, V., Gabler, J., Bomans, K., Sims, T., Omlor, G., and Richter, W. (2016). Differential expression of TGF-beta superfamily members and role of Smad1/5/9-signalling in chondral versus endochondral chondrocyte differentiation. Sci. Rep. 6, 36655. doi:10.1038/srep36655
D’Souza, S., del Prete, D., Jin, S., Sun, Q., Huston, A. J., Kostov, F. E., et al. (2011). Gfi1 expressed in bone marrow stromal cells is a novel osteoblast suppressor in patients with multiple myeloma bone disease. Blood 118, 6871–6880. doi:10.1182/blood-2011-04-346775
Dubon, M. J., Yu, J., Choi, S., and Park, K. S. (2017). Transforming growth factor beta induces bone marrow mesenchymal stem cell migration via noncanonical signals and N-cadherin. J. Cell. Physiol. doi:10.1002/jcp.25863
Ducy, P., Amling, M., Takeda, S., Priemel, M., Schilling, A. F., Beil, F. T., et al. (2000). Leptin inhibits bone formation through a hypothalamic relay: a central control of bone mass. Cell 100, 197–207. doi:10.1016/S0092-8674(00)81558-5
Fairfield, H., Falank, C., Avery, L., and Reagan, M. R. (2016). Multiple myeloma in the marrow: pathogenesis and treatments. Ann. N. Y. Acad. Sci. 1364, 32–51. doi:10.1111/nyas.13038
Fan, Y., Hanai, J. I., Le, P. T., Bi, R., Maridas, D., DeMambro, V., et al. (2017). Parathyroid hormone directs bone marrow mesenchymal cell fate. Cell Metab. 25, 661–672. doi:10.1016/j.cmet.2017.01.001
Ferrara, N., Gerber, H. P., and LeCouter, J. (2003). The biology of VEGF and its receptors. Nat. Med. 9, 669–676. doi:10.1038/nm0603-669
Fischer, J., Aulmann, A., Dexheimer, V., Grossner, T., and Richter, W. (2014). Intermittent PTHrP(1-34) exposure augments chondrogenesis and reduces hypertrophy of mesenchymal stromal cells. Stem Cells Dev. 23, 2513–2523. doi:10.1089/scd.2014.0101
Fukumoto, S., and Martin, T. J. (2009). Bone as an endocrine organ. Trends Endocrinol. Metab. 20, 230–236. doi:10.1016/j.tem.2009.02.001
Fukumoto, T., Sperling, J. W., Sanyal, A., Fitzsimmons, J. S., Reinholz, G. G., Conover, C. A., et al. (2003). Combined effects of insulin-like growth factor-1 and transforming growth factor-beta1 on periosteal mesenchymal cells during chondrogenesis in vitro. Osteoarthr. Cartil. 11, 55–64. doi:10.1053/joca.2002.0869
Gao, Y., Wu, X., Terauchi, M., Li, J. Y., Grassi, F., Galley, S., et al. (2008). T cells potentiate PTH-induced cortical bone loss through CD40L signaling. Cell Metab. 8, 132–145. doi:10.1016/j.cmet.2008.07.001
Giuliani, N., Colla, S., Morandi, F., Lazzaretti, M., Sala, R., Bonomini, S., et al. (2005). Myeloma cells block RUNX2/CBFA1 activity in human bone marrow osteoblast progenitors and inhibit osteoblast formation and differentiation. Blood 106, 2472–2483. doi:10.1182/blood-2004-12-4986
Gomes, S. A., Rangel, E. B., Premer, C., Dulce, R. A., Cao, Y., Florea, V., et al. (2013). S-nitrosoglutathione reductase (GSNOR) enhances vasculogenesis by mesenchymal stem cells. Proc. Natl. Acad. Sci. U.S.A. 110, 2834–2839. doi:10.1073/pnas.1220185110
Gopalakrishnan, V., Vignesh, R. C., Arunakaran, J., Aruldhas, M. M., and Srinivasan, N. (2006). Effects of glucose and its modulation by insulin and estradiol on BMSC differentiation into osteoblastic lineages. Biochem. Cell Biol. 84, 93–101. doi:10.1139/o05-163
Guise, T. A., Mohammad, K. S., Clines, G., Stebbins, E. G., Wong, D. H., Higgins, L. S., et al. (2006). Basic mechanisms responsible for osteolytic and osteoblastic bone metastases. Clin. Cancer Res. 12(20 Pt 2), 6213s–6216s. doi:10.1158/1078-0432.CCR-06-1007
He, Q., Scott Swindle, C., Wan, C., Flynn, R. J., Oster, R. A., Chen, D., et al. (2017). Enhanced hematopoietic stem cell self-renewal-promoting ability of clonal primary mesenchymal stromal/stem cells versus their osteogenic progeny. Stem Cells 35, 473–484. doi:10.1002/stem.2481
Hess, K., Ushmorov, A., Fiedler, J., Brenner, R. E., and Wirth, T. (2009). TNFalpha promotes osteogenic differentiation of human mesenchymal stem cells by triggering the NF-kappaB signaling pathway. Bone 45, 367–376. doi:10.1016/j.bone.2009.04.252
Hock, J. M., and Gera, I. (1992). Effects of continuous and intermittent administration and inhibition of resorption on the anabolic response of bone to parathyroid hormone. J. Bone Miner. Res. 7, 65–72. doi:10.1002/jbmr.5650070110
Huh, J. E., and Lee, S. Y. (2013). IL-6 is produced by adipose-derived stromal cells and promotes osteogenesis. Biochim. Biophys. Acta 1833, 2608–2616. doi:10.1016/j.bbamcr.2013.06.025
Indrawattana, N., Chen, G., Tadokoro, M., Shann, L. H., Ohgushi, H., Tateishi, T., et al. (2004). Growth factor combination for chondrogenic induction from human mesenchymal stem cell. Biochem. Biophys. Res. Commun. 320, 914–919. doi:10.1016/j.bbrc.2004.06.029
Jain, R. K. (2003). Molecular regulation of vessel maturation. Nat. Med. 9, 685–693. doi:10.1038/nm0603-685
Jaiswal, R. K., Jaiswal, N., Bruder, S. P., Mbalaviele, G., Marshak, D. R., and Pittenger, M. F. (2000). Adult human mesenchymal stem cell differentiation to the osteogenic or adipogenic lineage is regulated by mitogen-activated protein kinase. J. Biol. Chem. 275, 9645–9652. doi:10.1074/jbc.275.13.9645
Jilka, R. L. (2007). Molecular and cellular mechanisms of the anabolic effect of intermittent PTH. Bone 40, 1434–1446. doi:10.1016/j.bone.2007.03.017
Kaigler, D., Krebsbach, P. H., Polverini, P. J., and Mooney, D. J. (2003). Role of vascular endothelial growth factor in bone marrow stromal cell modulation of endothelial cells. Tissue Eng. 9, 95–103. doi:10.1089/107632703762687573
Kang, Q., Song, W. X., Luo, Q., Tang, N., Luo, J., Luo, X., et al. (2009). A comprehensive analysis of the dual roles of BMPs in regulating adipogenic and osteogenic differentiation of mesenchymal progenitor cells. Stem Cells Dev. 18, 545–559. doi:10.1089/scd.2008.0130
Kang, Y., Siegel, P. M., Shu, W., Drobnjak, M., Kakonen, S. M., Cordon-Cardo, C., et al. (2003). A multigenic program mediating breast cancer metastasis to bone. Cancer Cell 3, 537–549. doi:10.1016/S1535-6108(03)00132-6
Kassen, D., Moore, S., Percy, L., Herledan, G., Bounds, D., Rodriguez-Justo, M., et al. (2014). The bone marrow stromal compartment in multiple myeloma patients retains capability for osteogenic differentiation in vitro: defining the stromal defect in myeloma. Br. J. Haematol. 167, 194–206. doi:10.1111/bjh.13020
Kastrinaki, M. C., Pavlaki, K., Batsali, A. K., Kouvidi, E., Mavroudi, I., Pontikoglou, C., et al. (2013). Mesenchymal stem cells in immune-mediated bone marrow failure syndromes. Clin. Dev. Immunol. 2013, 265608. doi:10.1155/2013/265608
Keating, A. (2012). Mesenchymal stromal cells: new directions. Cell Stem Cell 10, 709–716. doi:10.1016/j.stem.2012.05.015
Kennelly, H., Mahon, B. P., and English, K. (2016). Human mesenchymal stromal cells exert HGF dependent cytoprotective effects in a human relevant pre-clinical model of COPD. Sci. Rep. 6, 38207. doi:10.1038/srep38207
Kim, R. Y., Yang, H. J., Song, Y. M., Kim, I. S., and Hwang, S. J. (2015). Estrogen modulates bone morphogenetic protein-induced sclerostin expression through the Wnt signaling pathway. Tissue Eng. Part A 21, 2076–2088. doi:10.1089/ten.TEA.2014.0585
Kontogianni, M. D., Dafni, U. G., Routsias, J. G., and Skopouli, F. N. (2004). Blood leptin and adiponectin as possible mediators of the relation between fat mass and BMD in perimenopausal women. J. Bone Miner. Res. 19, 546–551. doi:10.1359/JBMR.040107
La Cava, A., and Matarese, G. (2004). The weight of leptin in immunity. Nat. Rev. Immunol. 4, 371–379. doi:10.1038/nri1350
Lacey, D. C., Simmons, P. J., Graves, S. E., and Hamilton, J. A. (2009). Proinflammatory cytokines inhibit osteogenic differentiation from stem cells: implications for bone repair during inflammation. Osteoarthr. Cartil. 17, 735–742. doi:10.1016/j.joca.2008.11.011
Le Blanc, K., and Mougiakakos, D. (2012). Multipotent mesenchymal stromal cells and the innate immune system. Nat. Rev. Immunol. 12, 383–396. doi:10.1038/nri3209
Le Blanc, S., Simann, M., Jakob, F., Schutze, N., and Schilling, T. (2015). Fibroblast growth factors 1 and 2 inhibit adipogenesis of human bone marrow stromal cells in 3D collagen gels. Exp. Cell Res. 338, 136–148. doi:10.1016/j.yexcr.2015.09.009
Lee, M. H., Kim, Y. J., Kim, H. J., Park, H. D., Kang, A. R., Kyung, H. M., et al. (2003). BMP-2-induced Runx2 expression is mediated by Dlx5, and TGF-beta 1 opposes the BMP-2-induced osteoblast differentiation by suppression of Dlx5 expression. J. Biol. Chem. 278, 34387–34394. doi:10.1074/jbc.M211386200
Li, J., Liu, X., Zuo, B., and Zhang, L. (2016). The role of bone marrow microenvironment in governing the balance between osteoblastogenesis and adipogenesis. Aging Dis. 7, 514–525. doi:10.14336/AD.2015.1206
Li, J. Y., Adams, J., Calvi, L. M., Lane, T. F., Weitzmann, M. N., and Pacifici, R. (2013a). Ovariectomy expands murine short-term hemopoietic stem cell function through T cell expressed CD40L and Wnt10B. Blood 122, 2346–2357. doi:10.1182/blood-2013-03-487801
Li, L., Yao, X. L., He, X. L., Liu, X. J., Wu, W. C., Kuang, W., et al. (2013b). Role of mechanical strain and estrogen in modulating osteogenic differentiation of mesenchymal stem cells (MSCs) from normal and ovariectomized rats. Cell. Mol. Biol. (Noisy-le-grand) 59, OL1889–OL1893.
Li, Y., He, X., Olauson, H., Larsson, T. E., and Lindgren, U. (2013c). FGF23 affects the lineage fate determination of mesenchymal stem cells. Calcif. Tissue Int. 93, 556–564. doi:10.1007/s00223-013-9795-6
Liao, J., Li, X., Koh, A. J., Berry, J. E., Thudi, N., Rosol, T. J., et al. (2008). Tumor expressed PTHrP facilitates prostate cancer-induced osteoblastic lesions. Int. J. Cancer 123, 2267–2278. doi:10.1002/ijc.23602
Liu, Y., Berendsen, A. D., Jia, S., Lotinun, S., Baron, R., Ferrara, N., et al. (2012). Intracellular VEGF regulates the balance between osteoblast and adipocyte differentiation. J. Clin. Invest. 122, 3101–3113. doi:10.1172/JCI61209
Liu, Y., and Olsen, B. R. (2014). Distinct VEGF functions during bone development and homeostasis. Arch. Immunol. Ther. Exp. (Warsz.) 62, 363–368. doi:10.1007/s00005-014-0285-y
Luo, J., Yang, Z., Ma, Y., Yue, Z., Lin, H., Qu, G., et al. (2016). LGR4 is a receptor for RANKL and negatively regulates osteoclast differentiation and bone resorption. Nat. Med. 22, 539–546. doi:10.1038/nm.4076
Ma, Q., Xia, X., Tao, Q., Lu, K., Shen, J., Xu, Q., et al. (2016). Profound actions of an agonist of growth hormone-releasing hormone on angiogenic therapy by mesenchymal stem cells. Arterioscler. Thromb. Vasc. Biol. 36, 663–672. doi:10.1161/ATVBAHA.116.307126
Maes, C., Stockmans, I., Moermans, K., Van Looveren, R., Smets, N., Carmeliet, P., et al. (2004). Soluble VEGF isoforms are essential for establishing epiphyseal vascularization and regulating chondrocyte development and survival. J. Clin. Invest. 113, 188–199. doi:10.1172/JCI19383
Marie, P. J. (2012). Fibroblast growth factor signaling controlling bone formation: an update. Gene 498, 1–4. doi:10.1016/j.gene.2012.01.086
Marsano, A., Medeiros da Cunha, C. M., Ghanaati, S., Gueven, S., Centola, M., Tsaryk, R., et al. (2016). Spontaneous in vivo chondrogenesis of bone marrow-derived mesenchymal progenitor cells by blocking vascular endothelial growth factor signaling. Stem Cells Transl. Med. 5, 1730–1738. doi:10.5966/sctm.2015-0321
Martino, M. M., Maruyama, K., Kuhn, G. A., Satoh, T., Takeuchi, O., Muller, R., et al. (2016). Inhibition of IL-1R1/MyD88 signalling promotes mesenchymal stem cell-driven tissue regeneration. Nat. Commun. 7, 11051. doi:10.1038/ncomms11051
Menagh, P. J., Turner, R. T., Jump, D. B., Wong, C. P., Lowry, M. B., Yakar, S., et al. (2010). Growth hormone regulates the balance between bone formation and bone marrow adiposity. J. Bone Miner. Res. 25, 757–768. doi:10.1359/jbmr.091015
Mendez-Ferrer, S., Michurina, T. V., Ferraro, F., Mazloom, A. R., Macarthur, B. D., Lira, S. A., et al. (2010). Mesenchymal and haematopoietic stem cells form a unique bone marrow niche. Nature 466, 829–834. doi:10.1038/nature09262
Moore, K. A., and Lemischka, I. R. (2006). Stem cells and their niches. Science 311, 1880–1885. doi:10.1126/science.1110542
Mundy, G. R. (2002). Metastasis to bone: causes, consequences and therapeutic opportunities. Nat. Rev. Cancer 2, 584–593. doi:10.1038/nrc867
Murphy, M. B., Moncivais, K., and Caplan, A. I. (2013). Mesenchymal stem cells: environmentally responsive therapeutics for regenerative medicine. Exp. Mol. Med. 45, e54. doi:10.1038/emm.2013.94
Nagaya, N., Kangawa, K., Itoh, T., Iwase, T., Murakami, S., Miyahara, Y., et al. (2005). Transplantation of mesenchymal stem cells improves cardiac function in a rat model of dilated cardiomyopathy. Circulation 112, 1128–1135. doi:10.1161/CIRCULATIONAHA.104.500447
Nakagawa, M., Kaneda, T., Arakawa, T., Morita, S., Sato, T., Yomada, T., et al. (2000). Vascular endothelial growth factor (VEGF) directly enhances osteoclastic bone resorption and survival of mature osteoclasts. FEBS Lett. 473, 161–164. doi:10.1016/S0014-5793(00)01520-9
Nakase, T., Takaoka, K., Masuhara, K., Shimizu, K., Yoshikawa, H., and Ochi, T. (1997). Interleukin-1 beta enhances and tumor necrosis factor-alpha inhibits bone morphogenetic protein-2-induced alkaline phosphatase activity in MC3T3-E1 osteoblastic cells. Bone 21, 17–21. doi:10.1016/S8756-3282(97)00038-0
Nakashima, T., Hayashi, M., Fukunaga, T., Kurata, K., Oh-Hora, M., Feng, J. Q., et al. (2011). Evidence for osteocyte regulation of bone homeostasis through RANKL expression. Nat. Med. 17, 1231–1234. doi:10.1038/nm.2452
Niida, S., Kaku, M., Amano, H., Yoshida, H., Kataoka, H., Nishikawa, S., et al. (1999). Vascular endothelial growth factor can substitute for macrophage colony-stimulating factor in the support of osteoclastic bone resorption. J. Exp. Med. 190, 293–298. doi:10.1084/jem.190.2.293
Okazaki, R., Inoue, D., Shibata, M., Saika, M., Kido, S., Ooka, H., et al. (2002). Estrogen promotes early osteoblast differentiation and inhibits adipocyte differentiation in mouse bone marrow stromal cell lines that express estrogen receptor (ER) alpha or beta. Endocrinology 143, 2349–2356. doi:10.1210/endo.143.6.8854
Ornitz, D. M., and Legeai-Mallet, L. (2017). Achondroplasia: development, pathogenesis, and therapy. Dev. Dyn. 246, 291–309. doi:10.1002/dvdy.24479
Ouji, Y., Yoshikawa, M., Shiroi, A., and Ishizaka, S. (2006). Wnt-10b secreted from lymphocytes promotes differentiation of skin epithelial cells. Biochem. Biophys. Res. Commun. 342, 1063–1069. doi:10.1016/j.bbrc.2006.02.028
Pacifici, R. (2016a). The role of IL-17 and TH17 cells in the bone catabolic activity of PTH. Front. Immunol. 7:57. doi:10.3389/fimmu.2016.00057
Pacifici, R. (2016b). T cells, osteoblasts, and osteocytes: interacting lineages key for the bone anabolic and catabolic activities of parathyroid hormone. Ann. N. Y. Acad. Sci. 1364, 11–24. doi:10.1111/nyas.12969
Papadaki, H. A., Palmblad, J., and Eliopoulos, G. D. (2001). Non-immune chronic idiopathic neutropenia of adult: an overview. Eur. J. Haematol. 67, 35–44. doi:10.1034/j.1600-0609.2001.00473.x
Polo, S., and Di Fiore, P. P. (2006). Endocytosis conducts the cell signaling orchestra. Cell 124, 897–900. doi:10.1016/j.cell.2006.02.025
Post, S., Abdallah, B. M., Bentzon, J. F., and Kassem, M. (2008). Demonstration of the presence of independent pre-osteoblastic and pre-adipocytic cell populations in bone marrow-derived mesenchymal stem cells. Bone 43, 32–39. doi:10.1016/j.bone.2008.03.011
Pourgholaminejad, A., Aghdami, N., Baharvand, H., and Moazzeni, S. M. (2016). The effect of pro-inflammatory cytokines on immunophenotype, differentiation capacity and immunomodulatory functions of human mesenchymal stem cells. Cytokine 85, 51–60. doi:10.1016/j.cyto.2016.06.003
Pricola, K. L., Kuhn, N. Z., Haleem-Smith, H., Song, Y., and Tuan, R. S. (2009). Interleukin-6 maintains bone marrow-derived mesenchymal stem cell stemness by an ERK1/2-dependent mechanism. J. Cell. Biochem. 108, 577–588. doi:10.1002/jcb.22289
Provot, S., Kempf, H., Murtaugh, L. C., Chung, U. I., Kim, D. W., Chyung, J., et al. (2006). Nkx3.2/Bapx1 acts as a negative regulator of chondrocyte maturation. Development 133, 651–662. doi:10.1242/dev.02258
Qiao, X., Nie, Y., Ma, Y., Chen, Y., Cheng, R., Yin, W., et al. (2016). Irisin promotes osteoblast proliferation and differentiation via activating the MAP kinase signaling pathways. Sci. Rep. 6, 18732. doi:10.1038/srep18732
Qin, L., Raggatt, L. J., and Partridge, N. C. (2004). Parathyroid hormone: a double-edged sword for bone metabolism. Trends Endocrinol. Metab. 15, 60–65. doi:10.1016/j.tem.2004.01.006
Qiu, T., Wu, X., Zhang, F., Clemens, T. L., Wan, M., and Cao, X. (2010). TGF-beta type II receptor phosphorylates PTH receptor to integrate bone remodelling signalling. Nat. Cell Biol. 12, 224–234. doi:10.1038/ncb2022
Reagan, M. R., Mishima, Y., Glavey, S. V., Zhang, Y., Manier, S., Lu, Z. N., et al. (2014). Investigating osteogenic differentiation in multiple myeloma using a novel 3D bone marrow niche model. Blood 124, 3250–3259. doi:10.1182/blood-2014-02-558007
Roccaro, A. M., Sacco, A., Purschke, W. G., Moschetta, M., Buchner, K., Maasch, C., et al. (2014). SDF-1 inhibition targets the bone marrow niche for cancer therapy. Cell Rep. 9, 118–128. doi:10.1016/j.celrep.2014.08.042
Rodriguez, J. P., Astudillo, P., Rios, S., and Pino, A. M. (2008). Involvement of adipogenic potential of human bone marrow mesenchymal stem cells (MSCs) in osteoporosis. Curr. Stem Cell Res. Ther. 3, 208–218. doi:10.2174/157488808785740325
Sacchetti, B., Funari, A., Michienzi, S., Di Cesare, S., Piersanti, S., Saggio, I., et al. (2007). Self-renewing osteoprogenitors in bone marrow sinusoids can organize a hematopoietic microenvironment. Cell 131, 324–336. doi:10.1016/j.cell.2007.08.025
Sato, F., Miyaoka, Y., Miyajima, A., and Tanaka, M. (2014). Oncostatin M maintains the hematopoietic microenvironment in the bone marrow by modulating adipogenesis and osteogenesis. PLoS ONE 9:e116209. doi:10.1371/journal.pone.0116209
Scavo, L. M., Karas, M., Murray, M., and Leroith, D. (2004). Insulin-like growth factor-I stimulates both cell growth and lipogenesis during differentiation of human mesenchymal stem cells into adipocytes. J. Clin. Endocrinol. Metab. 89, 3543–3553. doi:10.1210/jc.2003-031682
Schena, F., Menale, C., Caci, E., Diomede, L., Palagano, E., Recordati, C., et al. (2017). Murine Rankl-/- mesenchymal stromal cells display an osteogenic differentiation defect improved by a RANKL-expressing lentiviral vector. Stem Cells 35, 1365–1377. doi:10.1002/stem.2574
Sharaf-Eldin, W. E., Abu-Shahba, N., Mahmoud, M., and El-Badri, N. (2016). The modulatory effects of mesenchymal stem cells on osteoclastogenesis. Stem Cells Int. 2016, 1908365. doi:10.1155/2016/1908365
Shipounova, I. N., Petrova, T. V., Svinareva, D. A., Momotuk, K. S., Mikhailova, E. A., and Drize, N. I. (2009). Alterations in hematopoietic microenvironment in patients with aplastic anemia. Clin. Transl. Sci. 2, 67–74. doi:10.1111/j.1752-8062.2008.00074.x
Simann, M., Le Blanc, S., Schneider, V., Zehe, V., Ludemann, M., Schutze, N., et al. (2017). Canonical FGFs prevent osteogenic lineage commitment and differentiation of human bone marrow stromal cells via ERK1/2 signaling. J. Cell. Biochem. 118, 263–275. doi:10.1002/jcb.25631
Sobacchi, C., Frattini, A., Guerrini, M. M., Abinun, M., Pangrazio, A., Susani, L., et al. (2007). Osteoclast-poor human osteopetrosis due to mutations in the gene encoding RANKL. Nat. Genet. 39, 960–962. doi:10.1038/ng2076
Sobacchi, C., Schulz, A., Coxon, F. P., Villa, A., and Helfrich, M. H. (2013). Osteopetrosis: genetics, treatment and new insights into osteoclast function. Nat. Rev. Endocrinol. 9, 522–536. doi:10.1038/nrendo.2013.137
Sullivan, C. B., Porter, R. M., Evans, C. H., Ritter, T., Shaw, G., Barry, F., et al. (2014). TNFalpha and IL-1beta influence the differentiation and migration of murine MSCs independently of the NF-kappaB pathway. Stem Cell Res Ther. 5, 104. doi:10.1186/scrt492
Tang, Y., Wu, X., Lei, W., Pang, L., Wan, C., Shi, Z., et al. (2009). TGF-beta1-induced migration of bone mesenchymal stem cells couples bone resorption with formation. Nat. Med. 15, 757–765. doi:10.1038/nm.1979
Terauchi, M., Li, J. Y., Bedi, B., Baek, K. H., Tawfeek, H., Galley, S., et al. (2009). T lymphocytes amplify the anabolic activity of parathyroid hormone through Wnt10b signaling. Cell Metab. 10, 229–240. doi:10.1016/j.cmet.2009.07.010
Thomas, T. (2004). The complex effects of leptin on bone metabolism through multiple pathways. Curr. Opin. Pharmacol. 4, 295–300. doi:10.1016/j.coph.2004.01.009
Tian, E., Zhan, F., Walker, R., Rasmussen, E., Ma, Y., Barlogie, B., et al. (2003). The role of the Wnt-signaling antagonist DKK1 in the development of osteolytic lesions in multiple myeloma. N. Engl. J. Med. 349, 2483–2494. doi:10.1056/NEJMoa030847
van Zoelen, E. J., Duarte, I., Hendriks, J. M., and van der Woning, S. P. (2016). TGFβ-induced switch from adipogenic to osteogenic differentiation of human mesenchymal stem cells: identification of drug targets for prevention of fat cell differentiation. Stem Cell Res Ther. 7, 123. doi:10.1186/s13287-016-0375-3
Vinatier, C., Mrugala, D., Jorgensen, C., Guicheux, J., and Noel, D. (2009). Cartilage engineering: a crucial combination of cells, biomaterials and biofactors. Trends Biotechnol. 27, 307–314. doi:10.1016/j.tibtech.2009.02.005
Walsh, S., Jefferiss, C., Stewart, K., and Beresford, J. N. (2003). TGFβ1 limits the expansion of the osteoprogenitor fraction in cultures of human bone marrow stromal cells. Cell Tissue Res. 311, 187–198. doi:10.1007/s00441-002-0679-8
Wang, Y., Bikle, D. D., and Chang, W. (2013). Autocrine and paracrine actions of IGF-I signaling in skeletal development. Bone Res. 1, 249–259. doi:10.4248/BR201303003
Xia, X., Tao, Q., Ma, Q., Chen, H., Wang, J., and Yu, H. (2016). Growth hormone-releasing hormone and its analogues: significance for MSCs-mediated angiogenesis. Stem Cells Int. 2016, 8737589. doi:10.1155/2016/8737589
Xian, L., Wu, X., Pang, L., Lou, M., Rosen, C. J., Qiu, T., et al. (2012). Matrix IGF-1 maintains bone mass by activation of mTOR in mesenchymal stem cells. Nat. Med. 18, 1095–1101. doi:10.1038/nm.2793
Xu, Y., Takahashi, Y., Wang, Y., Hama, A., Nishio, N., Muramatsu, H., et al. (2009). Downregulation of GATA-2 and overexpression of adipogenic gene-PPARgamma in mesenchymal stem cells from patients with aplastic anemia. Exp. Hematol. 37, 1393–1399. doi:10.1016/j.exphem.2009.09.005
Yang, Q., McHugh, K. P., Patntirapong, S., Gu, X., Wunderlich, L., and Hauschka, P. V. (2008). VEGF enhancement of osteoclast survival and bone resorption involves VEGF receptor-2 signaling and beta3-integrin. Matrix Biol. 27, 589–599. doi:10.1016/j.matbio.2008.06.005
Yu, B., Zhao, X., Yang, C., Crane, J., Xian, L., Lu, W., et al. (2012). Parathyroid hormone induces differentiation of mesenchymal stromal/stem cells by enhancing bone morphogenetic protein signaling. J. Bone Miner. Res. 27, 2001–2014. doi:10.1002/jbmr.1663
Yue, R., Zhou, B. O., Shimada, I. S., Zhao, Z., and Morrison, S. J. (2016). Leptin receptor promotes adipogenesis and reduces osteogenesis by regulating mesenchymal stromal cells in adult bone marrow. Cell Stem Cell 18, 782–796. doi:10.1016/j.stem.2016.02.015
Zelzer, E., Mamluk, R., Ferrara, N., Johnson, R. S., Schipani, E., and Olsen, B. R. (2004). VEGFA is necessary for chondrocyte survival during bone development. Development 131, 2161–2171. doi:10.1242/dev.01053
Zhang, J., Niu, C., Ye, L., Huang, H., He, X., Tong, W. G., et al. (2003). Identification of the haematopoietic stem cell niche and control of the niche size. Nature 425, 836–841. doi:10.1038/nature02041
Zhang, M., Xie, R., Hou, W., Wang, B., Shen, R., Wang, X., et al. (2009). PTHrP prevents chondrocyte premature hypertrophy by inducing cyclin-D1-dependent Runx2 and Runx3 phosphorylation, ubiquitylation and proteasomal degradation. J. Cell. Sci. 122(Pt 9), 1382–1389. doi:10.1242/jcs.040709
Keywords: mesenchymal stem cells, growth factors, hormones, cytokines, RANKL, bone marrow microenvironment
Citation: Sobacchi C, Palagano E, Villa A and Menale C (2017) Soluble Factors on Stage to Direct Mesenchymal Stem Cells Fate. Front. Bioeng. Biotechnol. 5:32. doi: 10.3389/fbioe.2017.00032
Received: 06 March 2017; Accepted: 27 April 2017;
Published: 17 May 2017
Edited by:
Carla Cunha, i3S – Instituto de Investigação e Inovação em Saúde, PortugalReviewed by:
Julie Fradette, Centre LOEX de l’Université Laval, CHU de Québec, CanadaPhilippe Bourin, Univercell Biosolutions, France
Copyright: © 2017 Sobacchi, Palagano, Villa and Menale. This is an open-access article distributed under the terms of the Creative Commons Attribution License (CC BY). The use, distribution or reproduction in other forums is permitted, provided the original author(s) or licensor are credited and that the original publication in this journal is cited, in accordance with accepted academic practice. No use, distribution or reproduction is permitted which does not comply with these terms.
*Correspondence: Cristina Sobacchi, Y3Jpc3RpbmEuc29iYWNjaGlAaHVtYW5pdGFzcmVzZWFyY2guaXQ=