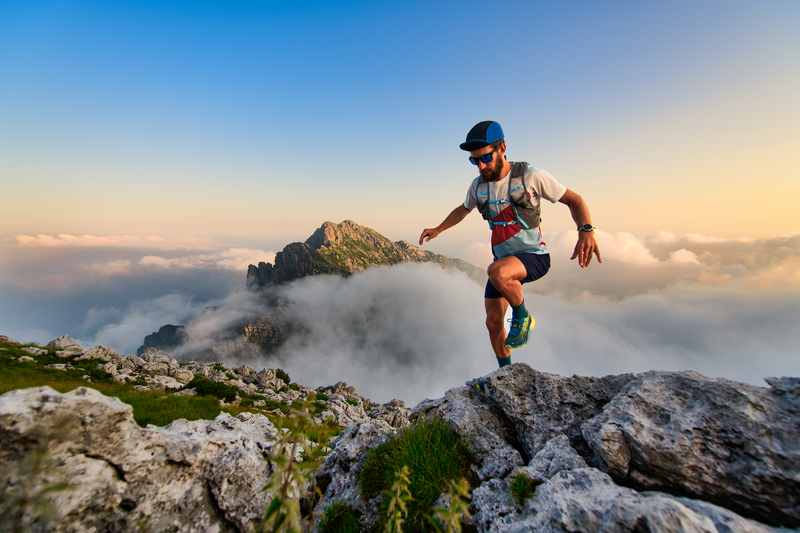
94% of researchers rate our articles as excellent or good
Learn more about the work of our research integrity team to safeguard the quality of each article we publish.
Find out more
REVIEW article
Front. Bioeng. Biotechnol. , 17 May 2017
Sec. Bionics and Biomimetics
Volume 5 - 2017 | https://doi.org/10.3389/fbioe.2017.00030
This article is part of the Research Topic Biomimetic fabrication for 3D biology View all 5 articles
Computational modeling has been increasingly applied to the field of tissue engineering and regenerative medicine. Where in early days computational models were used to better understand the biomechanical requirements of targeted tissues to be regenerated, recently, more and more models are formulated to combine such biomechanical requirements with cell fate predictions to aid in the design of functional three-dimensional scaffolds. In this review, we highlight how computational modeling has been used to understand the mechanisms behind tissue formation and can be used for more rational and biomimetic scaffold-based tissue regeneration strategies. With a particular focus on musculoskeletal tissues, we discuss recent models attempting to predict cell activity in relation to specific mechanical and physical stimuli that can be applied to them through porous three-dimensional scaffolds. In doing so, we review the most common scaffold fabrication methods, with a critical view on those technologies that offer better properties to be more easily combined with computational modeling. Finally, we discuss how modeling, and in particular finite element analysis, can be used to optimize the design of scaffolds for skeletal tissue regeneration.
Mechanical signals play an important role in cell differentiation (Engler et al., 2006), tissue development (O’Reilly and Kelly, 2016), and tissue homeostasis (Horsnell and Baldock, 2016). This is especially the case for skeletal tissue where bone remodeling is governed by the loads that the tissue experiences. In tissue engineering, mechanical stimulation can be used to enhance or control tissue development. However, in order to optimize stimulation regimes, it is important to understand and control how external stimuli, like compression and fluid perfusion, are translated to mechanical signals at the cellular level. After a brief historical perspective on bone mechanobiology and the theories and models that have been formulated over the past two centuries, we discuss scaffold fabrication methods in correlation with the use of finite element analysis (FEA) to design structures that better mimic the native environment. We elaborate on how the combination of developments in FEA to predict and design the local mechanical environment, and additive manufacturing (AM) to fabricate three-dimensional scaffolds with a high level of architectural control, has enabled the use of smart and biomimetic scaffold design for skeletal tissue regeneration.
Our current understanding of bone tissue starts with scientific research conducted as early as the 1800s when bone was investigated for its functional adaptation and internal organization. In 1832, Bourgery realized that bone complied with the maximum strength, minimum material principle, which explains the presence of cortical and trabecular bone. In 1866, the engineer Culmann and the anatomist Meyer showed the remarkable resemblance between the direction of the principle stresses in a crane and the bone architecture of the femur (Figure 1). Four years later, Wolff used the observations of Culmann and Meyer to explain the architecture of bone with mechanical stresses. According to Wolff, high stress values correspond with compact bone, less stress with trabecular bone, while no stress means a medullary cavity. In 1881, Roux postulated that bone was self-regulating. It was Wolff in 1892, however, who combined his own observations with those of Bourgery and Roux, leading to the working principles of bone adaptation to external loading, now generally known as Wolff’s law (Roesler, 1987). However, Roux realized 3 years later that functional adaptation was not only restricted to bone but was also applicable to other supportive tissues, such as cartilage and connective tissue. He postulated that the functional mechanical stimulus for bone was compression, while tension resulted in connective tissue, and shearing together with either compression or tension resulted in cartilage (Pauwels, 1960). Although Roux first described a relation between functional stimuli, cell differentiation, and tissue formation, correlating the application of mechanically functional stimuli for specific tissues formation has been a topic of debate since research on the effect of mechanical stimuli on tissue formation was initiated.
Figure 1. Stress lines in a crane as calculated and shown by Culmann (left) and the orientation of trabecular bone in a human femur as shown by Meyer (right). Reprinted from Skedros and Brand (2011) with permission.
More than 60 years later, in 1965, Pauwels postulated a new theory (Figure 2A) based on clinical observations of fracture healing, where cells in the mesenchyme tissue subjected to either pure tension, compression, or shearing elongate. Cell elongation causes the formation of collagen fibrils and, thus, connective tissue (Pauwels, 1960). Hydrostatic pressure on the cell, caused either by swelling of the cell or homogenous compressive forces on the mesenchyme tissue, results in cartilage. In Pauwels theory, bone is not associated to any mechanical stimulus as it was formed on an existing solid framework, either on existing bone, connective tissue, or cartilage.
Figure 2. Multiple mechano-regulation models that predict tissue development based on different mechanical stimuli. Panel (A) shows the model of Pauwels where an elementary sphere suspended in mesenchyme tissue subjected to compression, tension, and shear causes cells to form collagen fibrils. Hydrostatic pressure results in chondrogenesis while bone is formed on an existing solid framework. Panel (B) shows the model of Carter. With good vascularization, low distortional (octahedral shear stress), and volumetric (hydrostatic pressure) stresses results in bone formation, intermediate stresses in cartilage, and high stresses in fibrous tissue. Poor vascularization inhibits bone formation. Panel (C) shows the model of Claes and Heigele which dictates that low volumetric stress (0.15 MPa hydrostatic pressure) and low local distortional strain (5%) results in intramembranous ossification while hydrostatic pressures <−0.15 MPa with local strains between −15 and 15% results in endochondral ossification. Other hydrostatic pressures and strains results in connective tissue or fibrocartilage. Panel (D) shows the model of Prendergast where a distortional strain (tissue or octahedral shear strain) and interstitial fluid flow through mesenchyme tissue stimulates the mesenchymal cell to differentiate and form a tissue. The cell and formed tissue will be replaced by other cell types and tissue based on the perceived biophysical stimuli. Replacement of the cell and tissue population continues until the formed tissue withstands the biophysical stimuli and an equilibrium occurs. Adapted with permission from Pauwels (1960), Carter et al. (1988), Claes and Heigele (1999), and Lacroix and Prendergast (2002), respectively.
Not until 1981, Hayes and Snyder showed for the first time the highly significant correlation between bone structure and mechanical stresses with FEA. It became increasingly apparent that mechanical loading induced a local signal for cells to respond to. With the emergence of FEA, the research on mechanical loading and cell differentiation increased. Mechano-regulation theories were proposed in which biophysical stimuli were related with cell differentiation and tissue formation (Carter et al., 1988, 1998; Prendergast et al., 1997; Claes and Heigele, 1999). Carter was one of the first to support his theory based on the prediction of fracture healing with FEA (Figure 2B) (Carter et al., 1988). Besides mechanical loading, the entire history of cyclic loading on the mesenchyme tissue of the fracture callus and the vascularity of the mesenchyme tissue were equally important in the formation of tissue. Bone, cartilage, or fibrous tissues were based on two cyclic stresses, the distortional and the volumetric stress.
Claes and Heigele (1999) proposed a quantitative mechano-regulation theory also based on fracture healing (Figure 2C). Their theory distinguished itself from others by the fact that values were given for the mechanical stimuli thresholds for cell differentiation. Also, they proposed that new bone was formed on existing bone or along calcified tissue and that the local strains and stresses dictated the tissue formation. Prendergast modeled tissue formation at the bone–implant interface from a biphasic point of view (Figure 2D) (Prendergast et al., 1997; Lacroix and Prendergast, 2002). The theory assumes that cell populations can be replaced by other cell populations based on the biophysical stimuli perceived. Analyses continued until the formed tissue was able to support the applied biophysical loading. The biophysical stimuli were described by the deformation of the solid part with the octahedral shear strain, and the application of interstitial fluid flow through the mesenchyme tissue.
A study, where tissue differentiation in a torsional loading environment was compared with the predictive models previously developed, showed that the models were not able to predict the tissue differentiation in complete agreement with in vivo observations (Isaksson et al., 2006). However, the theory of Prendergast with its octahedral shear strain and interstitial fluid velocity seemed to be the most accurate. A different study where the prediction of tissue differentiation with FEA was compared with in vivo bone formation in a bone chamber did also not result in a full validation of the model (Khayyeri et al., 2009). The variability between the in vivo samples, which were probably due to genetic variability, were not reflected in the in silico predictions. Yet, since its formulation, the theory of Prendergast has been used to develop mechano-biological models to investigate the influence of mechanical loading (Pérez and Prendergast, 2007), angiogenesis (Checa and Prendergast, 2009), cell migration and proliferation (Pérez and Prendergast, 2007; Byrne et al., 2011), and cell sensitivity to mechanical loading (Khayyeri et al., 2011) between different species (Checa et al., 2011) on tissue differentiation to explain the observed differences.
It is clear that human bone marrow mesenchymal stromal cells (hMSCs) respond to active mechanical stimulation. When hMSCs were seeded on a 2D silicone sheet, an applied 2–8% uniaxial strain through stretching resulted in osteogenic differentiation (Jagodzinski et al., 2004; Haasper et al., 2008). Another experiment applying uniaxial strain through bending, showed both osteogenic differentiation (Qi et al., 2008) and chondrogenic differentiation (Friedl et al., 2007). Coating of the surface membrane with extracellular matrix (ECM) proteins accelerated osteogenic differentiation with stretching (Huang et al., 2009). With parallel-plate flow chambers, the effect of shear stress due to fluid flow on hMSCs showed to favor osteogenic differentiation as well (Kreke et al., 2008). hMSCs showed to be more sensitive to shear stress after a longer attachment time before fluid shear stress was applied (Yourek et al., 2010).
Numerous studies have also been performed to elucidate the response of cells to mechanical stimulation in a three-dimensional environment. Demineralized bovine bone seeded with hMSCs showed to be susceptive to mechanical loading in vitro (Mauney et al., 2004). Osteogenic markers were upregulated and mineralization was increased in the stimulated samples while a further enhancement of osteogenic differentiation was seen when the medium was supplemented with dexamethasone. hMSCs seeded in a three-dimensional collagen matrix and subjected to 10 and 12% tensile strain were driven into osteogenic differentiation without the need of chemical supplements (Sumanasinghe et al., 2006). The non-stimulated controls did not show osteogenic differentiation. In a different study, hydroxyapatite ceramic scaffolds were subjected to dynamic compression of various frequencies (Dumas et al., 2009). Frequencies of 0, 25, 50, or 100 Hz were superimposed on a 3-Hz compression frequency. Compression of 3 Hz alone showed an upregulation of bone specific proteins, which was further amplified with a superimposed 25 Hz frequency. The 50 and 100 Hz reduced osteogenic differentiation showing the responsiveness of cells to compression to be dependent on the strain rate profile as well. A study performed with osteoblast-like cells showed the influence of the frequency of stimulation (Sittichockechaiwut et al., 2009). Short periods of compression were applied to cell seeded poly urethane foam scaffolds on only a few days during the entire culture period of 20 days. Three days of applying a mechanical load was sufficient to induce a faster ECM maturation.
The effect of shear stress on cell differentiation was shown using different experimental set-ups (Kreke et al., 2008). Titanium fiber meshes seeded with hMSCs and subjected to a continuous fluid perfusion in parallel-plate flow chambers, showed osteogenic differentiation (Holtorf et al., 2005). When the medium was supplemented with dexamethasone, the osteogenic differentiation was further enhanced. Synthetic foam scaffolds seeded with hMSCs and suspended in a spinner flask containing dexamethasone supplemented medium had upregulated osteogenic gene expression compared to static cultured scaffolds (Stiehler et al., 2009). Collagen scaffolds seeded with hMSCs were stimulated in either a perfusion bioreactor or spinner flask and showed osteogenic differentiation in both cases (Meinel et al., 2004). The scaffold architecture and fluid flow direction influenced cell differentiation and tissue formation. Additionally, calcium deposits were found in the inner part of the scaffold and showed different deposition orientations based on the fluid flow.
The combination of continuous fluid perfusion and cyclic compression was shown to affect osteogenic differentiation of hMSCs in a decellularized bovine matrix (Jagodzinski et al., 2008). Perfusion alone resulted in a proliferative behavior of the seeded hMSCs, while in combination with mechanical loading hMSCs differentiated toward the osteogenic lineage. Similar results were shown in a study with a collagen scaffold for a meniscus implant. Continuous fluid perfusion in combination with mechanical stimulation improved the biomechanical properties of the in vitro tissue-engineered construct (Petri et al., 2012). A porous poly urethane scaffold intended for a meniscus implant also showed the beneficial effect of fluid perfusion and mechanical stimulation on the biomechanical properties of the tissue-engineered construct (Liu et al., 2012). The continuous stimulation resulted in increased ECM deposition and, therefore, higher equilibrium modulus. However, constructs with a lower stimulation time displayed a lower equilibrium modulus than the constructs with a longer stimulation time. A PGA-mesh scaffold subjected to mechanical shear and a simultaneous static compression resulted in improved biochemical properties of the tissue-engineered construct (Shahin and Doran, 2012). After stimulation, GAG and collagen type II synthesis were enhanced when compared to perfusion alone and static cultures.
The role of the scaffold architecture is crucial in order to understand cell differentiation and tissue formation inside skeletal tissue engineering scaffolds. Several fabrication techniques exist for scaffolds used in tissue engineering and regenerative medicine. These included conventional, electrospinning, and AM techniques. Each fabrication technique is linked to specific scaffold properties that affect how the cells within the scaffold respond to external mechanical stimuli. This will be discussed in more detail in the following section.
Conventional techniques are based on fabrication technologies in which the biomaterial is mixed with a porogen agent. Some examples are particulate leaching, thermally induced phase separation (TIPS), gas-foaming, and emulsion freeze-drying (Janik and Marzec, 2015). Depending on the technology used, some control over pore size, shape, and interconnectivity is possible by adapting the processing parameters. Especially, TIPS results in highly interconnected tubular pores (Ma and Zhang, 2001). However, since pore formation in conventional scaffold fabrication techniques depends on random nucleation events or random packing of particles, the exact porosity of resulting scaffolds is hard to predict and control. As such, these scaffolds are less fit for applications where an exact scaffold geometry is needed to control the local mechanical signals that cells experience upon scaffold compression or perfusion.
Conventional scaffold fabrication techniques lack the possibility of mimicking the structural composition of bone ECM, thus limiting the implementation of the input that can be originated by FEA. With electrospinning, small diameter fibers can be produced in the micro- and nanoscale range mimicking the physical dimensions of natural fibrillar ECM (Boffito et al., 2014; Shabafrooz et al., 2014). An electrical field between the metallic nozzle and the metallic collector created by a high voltage potential ejects a thin liquid polymer solution to the collector. During the travel to the collector, the solvent of the polymer is evaporated and a dry fiber is left on the collector. Fiber properties are dependent on the applied voltage (Deitzel et al., 2001; Meechaisue et al., 2006), flow rate (Megelski et al., 2002), collector distance (Bhardwaj and Kundu, 2010), solution parameters (Henriques et al., 2009), and ambient parameters (Kumbar et al., 2008). The voltage is able to influence the fiber diameter from microns to few tens of nanometers (Deitzel et al., 2001). The flow rate influences the fiber diameter as well, along with the fiber morphology (Megelski et al., 2002). Apart from tuning the diameter of the individual fibers, process parameters can also be adapted in order to create micro- or nanoscale surface features on the fibers (Li and Xia, 2004). By controlling the evaporation of the solvent during electrospinning, a phase separation process can be initiated resulting in surface features, such as grooves (Liu et al., 2014) or nanoporosity (Wang et al., 2013).
Although electrospun scaffolds can mimic more closely the physical dimensions of native ECM, scaffolds obtained through electrospinning and conventional techniques have the common problem that the pore network configuration is hard to control. To circumvent this problem, scaffolds can also be fabricated using AM, which is defined as the process of joining materials to make objects from three-dimensional model data, usually in a layer upon layer method (Mota et al., 2015). Such a layer-by-layer method enables to construct scaffolds through the Computer-Aided Design/Computer-Aided Manufacturing (CAD/CAM) principles. This means that pore size, shape, and porosity can be designed to match the needs of a specific application. Among AM platforms are powder-, photosensitive-, and melt-extrusion-based techniques. With powder-based techniques, a powder is bound through, for example, melting [Selective Laser Sintering (SLS)] or a binding material (Printing). In SLS, powder particles of either polymer, ceramic or a composite, are bound through a high-intensity laser beam, after which new powder particles are applied for the next layer (Ciardelli et al., 2005; Williams et al., 2005; Eosoly et al., 2010; Shanjani et al., 2010). The resolution of this technique depends both on the powder and laser beam used. Printing is based on the same technique as SLS, except that the binding occurs through a binding material, such as an organic solvent, that is printed on the powder bed (Butscher et al., 2011). Here, the droplet size determines the minimal possible scaffold fiber diameter and spacing. Through sintering after scaffold fabrication, the mechanical properties can be improved (Shanjani et al., 2010).
Stereolithography (STL) is a photosensitive-based technique in which a light source polymerizes a liquid resin. Similar to printing, the beam traces the contours of the designed scaffold. Upon finishing a layer, the station with the cured resin is lowered and a new layer of liquid resin is placed on the fabricated layer (Hutmacher et al., 2004). Pore size, shape, and porosity can be designed through the CAD/CAM principle and mechanical properties can, therefore, be tuned accordingly (Melchels et al., 2011). However, besides the limitation of available biocompatible photosensitive biomaterials, fabricated scaffolds through STL needs post-processing for a better polymerization and removing unreacted resin (Melchels et al., 2010).
Fused deposition modeling (FDM), three-dimensional fiber deposition (3DF), precision extrusion deposition (PED), and bioscaffolding are examples of extrusion-based AM techniques (Mota et al., 2015). In FDM, a filament of a thermoplastic polymer is shortly heated in the nozzle and extruded on a stationary platform. The main advantage of this technique is the accurate control of the pore configuration of the scaffold. However, only thermoplastic polymers in a filament shape can be used as the base biomaterial, which limits in part the palette of biomaterials that can be processed (Hutmacher, 2000). 3DF is very similar to FDM. However, the polymer is inserted in a cartridge which is heated and subsequently pressurized for extruding the polymer melt onto the platform in a layer-by-layer fashion. The limitation of a thermoplastic polymer in a filament base shape is removed and thermoplastic polymers in any base shape could be used, thus expanding the gamma of biomaterials that can be used (Moroni et al., 2005). The disadvantage of this system is the possible thermal degradation of the polymer due to high residency time in the heated cartridge. Similar to FDM and 3DF, PED uses a screw to extrude the polymer melt on the platform (Shor et al., 2009). The combination of pressurized extrusion (3DF) and screw extrusion (PED) can be found in a commercial AM machine, the Bioscaffolder. This machine has been used to obtain scaffolds with the same pore configuration but from different biomaterials (Chaudhuri et al., 2015; Hendrikson et al., 2015).
From an FEA perspective, scaffolds should be highly reproducible and the pore configuration highly controllable. Scaffolds obtained through conventional fabrication techniques lack the possibility of complete control of the pore configuration and, therefore, also suffer from limited reproducibility in terms of producing the same identical pore network. Although electrospinning is a very interesting technique, the fiber dimensions, the control of pore configuration, and reproducibility renders electrospun scaffolds not desirable for investigating scaffold properties with FEA. Melt-electrospinning is a rising technology that has attracted a lot of attention for scaffold fabrication as it holds the potential to combine the high fidelity of AM platforms with the ECM-mimicking properties of electrospinning (Gernot et al., 2015). In this respect, it will be very interesting to combine this technology with the more rationale design of scaffolds for bone or other tissue regeneration that can be obtained through FEA. Printing is an interesting option as the pore configuration can be controlled. However, it remains challenging to obtain reproducible scaffolds when the surface topography of the fibers is concerned. Photosensitive techniques are very interesting as the reproducibility and pore configuration will be highly controllable. Despite good combination of computational modeling and design of scaffolds pore network and STL has been achieved (Melchels et al., 2010), the limited availability of biomaterials makes them a less suitable option than extrusion-based techniques. With these techniques the pore configuration is highly controllable and scaffolds are highly reproducible. Additionally, the choice of suitable biomaterials is broader than other AM techniques. Therefore, extrusion-based AM technologies seem the best scaffold fabrication platform to be combined with FEA design.
Scaffolds developed with the intention to serve as bone grafts are generally characterized for their osteogenic potential and not for their mechanical properties. However, there is a consensus that the mechanical properties of the scaffold should match the mechanical properties of bone when the scaffold will be used in a load-bearing application (Fröhlich et al., 2008; Velasco et al., 2015). Therefore, finite element models (FEMs) have been developed to capture the anisotropic mechanical behavior of trabecular bone. By tuning the porosity of the FEMs to match the hierarchical structure of trabecular bone, anisotropic mechanical behavior was predicted similar to trabecular bone (Huang et al., 2014). A different method was applied to develop a scaffold architecture matching the desired porosity and elastic properties of trabecular bone using an optimization algorithm. Scaffolds were fabricated by printing (Lin et al., 2004), casting (Hollister et al., 2002), or selective laser melting (Challis et al., 2010). However, no mechanical tests were performed on the fabricated scaffolds. More recently, a numerical optimization strategy was developed in which the mechanical properties of the scaffold could match native tissue. The novelty of this approach was that the material behavior of degradation and loss of mechanical stiffness were taken into account and used to develop a scaffold with struts of different biomaterials (Heljak et al., 2012).
The hierarchical structure of cartilage is depth dependent and is usually divided into three zones, the superficial, middle, and deep zone, in which the composition and collagen orientation delineates the zones (Pearle et al., 2005). In the superficial zone, collagen is oriented tangential, while in the deep zone it is oriented perpendicular to the articular surface and the middle zone delineates the transition in the orientation. The composition and orientation of the constituents, gives articular cartilage, and subchondral bone, a range of complex mechanical characteristics and behaviors. The orientation of collagen results in anisotropic mechanical behavior of cartilage (Treppo et al., 2000). Furthermore, collagen can resist tensile loads better than compressive loads.
The anisotropic composition and the visco-elastic behavior of cartilage results in a range of reported mechanical properties. For a human knee, a dynamic stiffness of 4.5 MPa was reported at a loading frequency of 0.1 Hz (Treppo et al., 2000), an aggregate static modulus of 0.1–2.0 MPa (Moutos et al., 2010), while a Young’s modulus of 10 MPa has been used in FEA simulations for cartilage (Lacroix et al., 2002). Electrospun scaffolds have been used to regenerate articular cartilage and meniscus, as the nanofibers supposedly mimic the collagen orientation in articular cartilage (Baker and Mauck, 2007; Wise et al., 2009; Accardi et al., 2013). It was shown that fiber orientation not only influences cell orientation (Wise et al., 2009) but also the mechanical properties of the scaffold (Baker and Mauck, 2007; Accardi et al., 2013). More recently, hydrogels have been fiber reinforced to mimic the articular cartilage even more, obtaining encouraging results compared with equine (Visser et al., 2015) and human articular cartilage (Moutos et al., 2010; Liao et al., 2013).
Swelling of cartilage due to the presence of proteoglycans is counterbalanced by the solid collagenous matrix. The presence of water gives cartilage visco-elastic properties and provides fluid load support. Upon loading of cartilage, fluid in the cartilage is pressurized and supports the load. However, in time, the fluid is expelled from the tissue and the solid matrix supports the load. Besides, this load sharing between the solid and fluid matrix proved to be important for the frictional response of articular cartilage (Caligaris and Ateshian, 2008; Ateshian, 2009). The sliding velocity, contact area, and lubrication are all influential on the friction coefficient (Caligaris and Ateshian, 2008; Gleghorn and Bonassar, 2008; Neu et al., 2008; Ateshian, 2009; Bonnevie et al., 2011; Shi et al., 2011). Combinations of contact pressure, speed, and viscosity (Hersey number) results in different lubrication modes which can be related to the friction coefficient by the Stribeck curve (Gleghorn and Bonassar, 2008). Therefore, the experimental setup has significant influence if such a fluid support is achieved and the resulting friction coefficient measured (Shi et al., 2011). Friction coefficients for bovine articular cartilage were reported between 0.02 and 0.1 (Caligaris and Ateshian, 2008; Bonnevie et al., 2011), while for human cartilage values between 0.06 and 0.15 were reported for a variety of experimental settings (Merkher et al., 2006). Non-cellular woven PCL scaffolds infused with either an alginate or poly acrylamide hydrogel showed to have friction values similar to the higher frictional coefficients of human cartilage (Liao et al., 2013). The same woven PCL scaffolds but now cultured with hMSCs and fibrin showed similar friction coefficients as the non-cellular scaffolds (Moutos and Guilak, 2010). Electrospun scaffolds subjected to physiological loads revealed that fiber orientation in the direction of the loading had lower surface damage compared to perpendicular oriented fibers. In vitro cell culture, however, did not reduce the friction coefficient in these scaffolds which were similar to the friction coefficient of a bovine knee joint explant (Accardi et al., 2013).
For osteochondral replacements, scaffold designs are classified upon their structural design. In order to succeed in regenerating osteochondral tissue, a part of the scaffold has to be dedicated to cartilage regeneration and the other part to bone regeneration. Therefore, a scaffold for osteochondral constructs can be classified as either mono-, bi-, or multiphasic from either the scaffold architecture, biomaterial, or composition. In a monophasic scaffold design, there is only one scaffold design for the whole construct and no distinction is made between the cartilage and bone region. A biphasic scaffold can have for example two architectures, two biomaterials, or two porosities, specifically for bone and cartilage. A multiphasic scaffold has more than two different architectures, biomaterials, or porosities in order to obtain an osteochondral construct. The easiest method of obtaining a biphasic scaffold is to combine a scaffold for cartilage regeneration, usually a hydrogel, with a scaffold for bone regeneration, usually a polymer or a ceramic (Gao et al., 2001). However, separation of the constructs in vivo is a point of concern (Schek et al., 2004). Ideally, the bond between the different scaffolds should be mimicking the tidemark region between articular cartilage and subchondral bone as seen in native tissue. This implies the need of a multiphasic scaffold in order to provide a stable construct (Jiang et al., 2010). A hydrogel region for cartilage regeneration could be coupled with PLGA microspheres to a region of composite PLGA microspheres for bone regeneration. Mechanical characterization of the construct, however, showed lower values for the construct compared to native tissue (Jiang et al., 2010). Another method of obtaining multiphasic scaffold design is through scaffolds with distinct gradients or porosities for cartilage and bone regeneration. Microspheres loaded with growth factors specific for cartilage or bone regeneration were used to obtain a scaffold with a functional growth factor gradient. Histological analysis showed good results although no mechanical analyses have been performed (Mohan et al., 2011). Combining microspheres with a porogen, a gradient in porosity was achieved for the scaffold (Dorcemus and Nukavarapu, 2014). Alternatively, microspheres with different diameters were used to obtain a scaffold with a gradient in pore size (Tang et al., 2012). While there was a difference in proliferation rate between the different pore sizes, osteogenic differentiation was not statistically different. These studies, therefore, show how challenging it is still to design a functional scaffold for osteochondral tissue regeneration where several physical, chemical, mechanical, and biological different features have to be taken into account. It is also for these more complex tissue engineering and regenerative medicine strategies where multiple tissues are targeted that FEA can be of great help in optimizing the scaffolds design.
Additive manufacturing provides a tool in developing scaffolds with tailored design that can help better matching musculoskeletal tissue regeneration requirements. Pore configuration can be completely controlled and, therefore, tuned to mechanically match native tissue (Moroni et al., 2006a,b). A database has been developed with different structural configurations, in which the combination of configuration and porosity can be used to obtain scaffolds with desirable mechanical properties through SLS (Sudarmadji et al., 2011). Besides the structural configuration to match the mechanical properties with native tissue, cells in the scaffold should be able to migrate throughout the whole scaffold with a sufficient nutrient and waste exchange provided for them to from tissue (Hollister et al., 2002; Shipley et al., 2009). To satisfy these requirements, the porosity and interconnectivity of the pores play an important role (Sengers et al., 2007). Additionally, the pore shape alongside the porosity and interconnectivity play an important role in the stress and strain distribution when mechanically loaded (Dias et al., 2014). Mathematical models have been developed to optimize the scaffolds internal architecture for trabecular bone to obtain the desired mechanical properties and porosity (Hollister et al., 2002; Lin et al., 2004), and fabricated scaffolds displayed a reasonable match with the computational design (Dias et al., 2014).
Using FEMs, the mechanical behavior of a representative pore of a regularly structured titanium scaffold was reproduced (Ryan et al., 2009). FEMs based on μCT scans proved to be a good indicator for the apparent stiffness, but the CAD-based FEMs were not able to capture the experimental results. Similar for the permeability of AM scaffolds, CAD-based FEMs could not predict the permeability of the scaffold accurately compared to the experimental values, while the μCT-based FEMs did (Truscello et al., 2012).
Despite the lack of accurately reproducing experimental results, CAD-based models were used to investigate the influence of scaffold designs on cell differentiation and tissue formation. For this, coupling of the mechano-regulation of Prendergast (Prendergast et al., 1997) with the stress and strain distributions in the scaffold was applied. By using a representative pore of a (theoretical) scaffold as unit-cells, the influence of biomaterial stiffness, porosity, and pore size was determined (Sanz-Herrera et al., 2009). A faster bone formation was seen in a scaffold with a stiffer biomaterial, increased pore sizes, or porosity with the limit of early collapsing with a too large pore size or porosity. Similar behavior was seen in an idealized scaffold whereas also the dissolution rate showed to be important as a too fast dissolution rate collapsed the scaffold (Byrne et al., 2011).
A μCT-scanned calcium phosphate scaffold was used to calculate the stress and strain distribution throughout the scaffold with FEA (Sandino et al., 2008). It not only showed the influence of pore morphology on the stress and strain distribution but also on the stress and strain magnitudes. The heterogeneous pore configuration of the scaffold resulted in areas with high stresses and strains. A later study investigated the effect of loading on cell differentiation and tissue formation in the same μCT-scanned scaffold (Sandino and Lacroix, 2011), showing that the current stimulation values used in in vitro studies were higher than necessary. A FEM of a salt-leached scaffold highlighted the effect of irregular pore size and distribution by predicting a heterogeneous stress and strain distribution and subsequently a heterogeneous tissue formation (Milan et al., 2009, 2010). CAD-based FEMs were developed to investigate the influence of pore shape, size, and porosity with various biophysical loadings (Olivares et al., 2009). Similar to the irregular structured FEMs, these FEMs showed the critical influence on the stress and strain distribution and the cell differentiation stimulus. Fluid flow simulations evidenced the influence of the pore shape on shear stress in which small pore sizes resulted in high shear stresses. FEMs based on μCT-scanned AM scaffolds with different pore configurations also showed the influence of pore configuration on the stress and strain distribution and cell differentiation prediction (Hendrikson et al., 2016). Pore size and shape influenced fluid flow and shear stress distribution, while the presence of a supporting column influenced the strain distribution from mechanical compression. For cell differentiation prediction, the fluid flow and mechanical loading each had a distinctive influence based on the pore configuration. Large pore sizes had less influence for fluid flow, while with smaller pore sizes the fluid flow had a stronger influence.
The field of AM is developing fast. Resolution, as well as the amount of compatible biomaterials, is increasing. This allows for an ever increasing control over the geometry and surface structure, as well as the mechanical properties and the surface chemistry of scaffolds that are produced using AM. Combined with an increasing complexity and computational power of FEMs, and a better understanding of how mechanical signals result in specific cellular behavior and differentiation, this will allow for the design and manufacturing of scaffolds that result in predictable and optimal tissue development upon active mechanical stimulation and fluid perfusion. Apart from that, recent developments regarding the AM of scaffolds using shape memory or conductive materials, results in a further increase of the control over cellular differentiation (Figure 3A) (Gladman et al., 2016). A particular appealing option would be to develop reconfigurable three-dimensional systems able to change structural properties depending on the applied deformation (Figure 3B) (Coulais et al., 2016). On the modeling side, further improvement of current models developed to predict cell activity could combine cellular with genetic information (Bolander et al., 2016). Furthermore, current models focus on a specific tissue formation, without taking into account associated tissues like vascular and neural networks. Whereas, more recent models have also started to look into vasculature predictions (Carlier et al., 2015), innervation remains a field poorly studied in tissue engineering and regenerative medicine.
Figure 3. Examples of current developments where computational modeling is combined with additive manufacturing to acquire advanced functionalities. Panel (A) shows an example of biomimetic 4D printing, where composite hydrogel architectures that are encoded with localized, anisotropic swelling behavior, are printed in designs that result in a predictable and controllable shape change when the objects are hydrated. Panel (B) shows an example where anisotropically deforming cubic building blocks are combined using three-dimensional printing. By computationally designing the relative placement of multiple building blocks, predictable surface textures can be created when exposed to uniaxial compression or extension. Adapted with permission from Gladman et al. (2016) and Coulais et al. (2016), respectively.
With the fast development of modern technologies, CAD/CAM approaches are very realistic methods with which scaffolds can be prepared that possess properties at several multidimensional scales and are usable in tissue engineering and regenerative medicine. Smart use of the combination of computational power and scaffold design can provide valuable and deep insights in the process of tissue formation. FEA could be used to design a priori scaffolds with optimal structural, mechanical, and physical properties to direct specific cell function. FEAs could also be used to predict the best culture conditions, in terms of nutrient perfusion and active mechanical stimulation, to further influence cell differentiation. Such combination has the potential to accelerate research in addressing the ultimate goal of tissue engineering: the replacing, engineering, or regenerating human cells, tissues, or organs to restore or establish normal function. Furthermore, the resulting improved engineered constructs would also find broad use in studying skeletal biology in more reliable three-dimensional in vitro models, thus holding the potential to offer a replacement to animal studies both for fundamental studies to better understand pathological mechanisms behind skeletal diseases as well as for applied research to find more efficient advanced therapies.
WH, JR, and LM structured the literature to be reviewed. WH, JR, CB, and LM wrote the manuscript. WH selected figures and asked for reprint permission.
The authors declare that the research was conducted in the absence of any commercial or financial relationships that could be construed as a potential conflict of interest.
The authors would like to acknowledge funding for WH from the Netherlands Institute of Regenerative Medicine, contract grant number: FES0908. This project/research has been also made possible with the support of the Dutch Province of Limburg.
Accardi, M. A., McCullen, S. D., Callanan, A., Chung, S., Cann, P. M., Stevens, M. M., et al. (2013). Effects of fiber orientation on the frictional properties and damage of regenerative articular cartilage surfaces. Tissue Eng. Part A 19, 2300–2310. doi: 10.1089/ten.TEA.2012.0580
Ateshian, G. A. (2009). The role of interstitial fluid pressurization in articular cartilage lubrication. J. Biomech. 42, 1163–1176. doi:10.1016/j.jbiomech.2009.04.040
Baker, B. M., and Mauck, R. L. (2007). The effect of nanofiber alignment on the maturation of engineered meniscus constructs. Biomaterials 28, 1967–1977. doi:10.1016/j.biomaterials.2007.01.004
Bhardwaj, N., and Kundu, S. C. (2010). Electrospinning: a fascinating fiber fabrication technique. Biotechnol. Adv. 28, 325–347. doi:10.1016/j.biotechadv.2010.01.004
Boffito, M., Sartori, S., and Ciardelli, G. (2014). Polymeric scaffolds for cardiac tissue engineering: requirements and fabrication technologies. Polym. Int. 63, 2–11. doi:10.1002/pi.4608
Bolander, J., Chai, Y. C., Geris, L., Schrooten, J., Lambrechts, D., Roberts, S. J., et al. (2016). Early BMP, Wnt and Ca(2+)/PKC pathway activation predicts the bone forming capacity of periosteal cells in combination with calcium phosphates. Biomaterials 86, 106–118. doi:10.1016/j.biomaterials.2016.01.059
Bonnevie, E. D., Baro, V. J., Wang, L., and Burris, D. L. (2011). In situ studies of cartilage microtribology: roles of speed and contact area. Tribol. Lett. 41, 83–95. doi:10.1007/s11249-010-9687-0
Butscher, A., Bohner, M., Hofmann, S., Gauckler, L., and Müller, R. (2011). Structural and material approaches to bone tissue engineering in powder-based three-dimensional printing. Acta Biomater. 7, 907–920. doi:10.1016/j.actbio.2010.09.039
Byrne, D. P., Lacroix, D., and Prendergast, P. J. (2011). Simulation of fracture healing in the tibia: mechanoregulation of cell activity using a lattice modeling approach. J. Orthop. Res. 29, 1496–1503. doi:10.1002/jor.21362
Caligaris, M., and Ateshian, G. A. (2008). Effects of sustained interstitial fluid pressurization under migrating contact area, and boundary lubrication by synovial fluid, on cartilage friction. Osteoarthr. Cartil. 16, 1220–1227. doi:10.1016/j.joca.2008.02.020
Carlier, A., Geris, L., van Gastel, N., Carmeliet, G., and Van Oosterwyck, H. (2015). Oxygen as a critical determinant of bone fracture healing – a multiscale model. J. Theor. Biol. 365, 247–264. doi:10.1016/j.jtbi.2014.10.012
Carter, D. R., Beaupré, G. S., Giori, N. J., and Helms, J. A. (1998). Mechanobiology of skeletal regeneration. Clin. Orthop. 355, S41–S55. doi:10.1097/00003086-199810001-00006
Carter, D. R., Blenman, P. R., and Beaupré, G. S. (1988). Correlations between mechanical stress history and tissue differentiation in initial fracture healing. J. Orthop. Res. 6, 736–748. doi:10.1002/jor.1100060517
Challis, V. J., Roberts, A. P., Grotowski, J. F., Zhang, L. C., and Sercombe, T. B. (2010). Prototypes for bone implant scaffolds designed via topology optimization and manufactured by solid freeform fabrication. Adv. Eng. Mater. 12, 1106–1110. doi:10.1002/adem.201000154
Chaudhuri, O., Gu, L., Darnell, M., Klumpers, D., Bencherif, S. A., Weaver, J. C., et al. (2015). Substrate stress relaxation regulates cell spreading. Nat. Commun. 6, 6364. doi:10.1038/ncomms7365
Checa, S., and Prendergast, P. J. (2009). A mechanobiological model for tissue differentiation that includes angiogenesis: a lattice-based modeling approach. Ann. Biomed. Eng. 37, 129–145. doi:10.1007/s10439-008-9594-9
Checa, S., Prendergast, P. J., and Duda, G. N. (2011). Inter-species investigation of the mechano-regulation of bone healing: comparison of secondary bone healing in sheep and rat. J. Biomech. 44, 1237–1245. doi:10.1016/j.jbiomech.2011.02.074
Ciardelli, G., Chiono, V., Vozzi, G., Pracella, M., Ahluwalia, A., Barbani, N., et al. (2005). Blends of poly-(ε-caprolactone) and polysaccharides in tissue engineering applications. Biomacromolecules 6, 1961–1976. doi:10.1021/bm0500805
Claes, L. E., and Heigele, C. A. (1999). Magnitudes of local stress and strain along bony surfaces predict the course and type of fracture healing. J. Biomech. 32, 255–266. doi:10.1016/S0021-9290(98)00153-5
Coulais, C., Teomy, E., de Reus, K., Shokef, Y., and van Hecke, M. (2016). Combinatorial design of textured mechanical metamaterials. Nature 535, 529–532. doi:10.1038/nature18960
Deitzel, J. M., Kleinmeyer, J., Harris, D., and Beck Tan, N. C. (2001). The effect of processing variables on the morphology of electrospun nanofibers and textiles. Polymer 42, 261–272. doi:10.1016/S0032-3861(00)00250-0
Dias, M. R., Guedes, J. M., Flanagan, C. L., Hollister, S. J., and Fernandes, P. R. (2014). Optimization of scaffold design for bone tissue engineering: a computational and experimental study. Med. Eng. Phys. 36, 448–457. doi:10.1016/j.medengphy.2014.02.010
Dorcemus, D. L., and Nukavarapu, S. P. (2014). Novel and unique matrix design for osteochondral tissue engineering. MRS Proc. 1621, 17–23. doi:10.1557/opl.2014.285
Dumas, V., Perrier, A., Malaval, L., Laroche, N., Guignandon, A., Vico, L., et al. (2009). The effect of dual frequency cyclic compression on matrix deposition by osteoblast-like cells grown in 3D scaffolds and on modulation of VEGF variant expression. Biomaterials 30, 3279–3288. doi:10.1016/j.biomaterials.2009.02.048
Engler, A. J., Sen, S., Sweeney, H. L., and Discher, D. E. (2006). Matrix elasticity directs stem cell lineage specification. Cell 126, 677–689. doi:10.1016/j.cell.2006.06.044
Eosoly, S., Brabazon, D., Lohfeld, S., and Looney, L. (2010). Selective laser sintering of hydroxyapatite/poly-ε-caprolactone scaffolds. Acta Biomater. 6, 2511–2517. doi:10.1016/j.actbio.2009.07.018
Friedl, G., Schmidt, H., Rehak, I., Kostner, G., Schauenstein, K., and Windhager, R. (2007). Undifferentiated human mesenchymal stem cells (hMSCs) are highly sensitive to mechanical strain: transcriptionally controlled early osteo-chondrogenic response in vitro. Osteoarthr. Cartil. 15, 1293–1300. doi:10.1016/j.joca.2007.04.002
Fröhlich, M., Grayson, W. L., Wan, L. Q., Marolt, D., Drobnic, M., and Vunjak-Novakovic, G. (2008). Tissue engineered bone grafts: biological requirements, tissue culture and clinical relevance. Curr. Stem Cell Res. Ther. 3, 254–264. doi:10.2174/157488808786733962
Gao, J., Dennis, J. E., Solchaga, L. A., Awadallah, A. S., Goldberg, V. M., and Caplan, A. I. (2001). Tissue-engineered fabrication of an osteochondral composite graft using rat bone marrow-derived mesenchymal stem cells. Tissue Eng. 7, 363–371. doi:10.1089/10763270152436427
Gernot, H., Tomasz, J., Toby, D. B., Kathrin, H., Claus, M., Franz, J., et al. (2015). Additive manufacturing of scaffolds with sub-micron filaments via melt electrospinning writing. Biofabrication 7, 035002. doi:10.1088/1758-5090/7/3/035002
Gladman, A. S., Matsumoto, E. A., Nuzzo, R. G., Mahadevan, L., and Lewis, J. A. (2016). Biomimetic 4D printing. Nat. Mater. 15, 413–418. doi:10.1038/nmat4544
Gleghorn, J. P., and Bonassar, L. J. (2008). Lubrication mode analysis of articular cartilage using Stribeck surfaces. J. Biomech. 41, 1910–1918. doi:10.1016/j.jbiomech.2008.03.043
Haasper, C., Jagodzinski, M., Drescher, M., Meller, R., Wehmeier, M., Krettek, C., et al. (2008). Cyclic strain induces FosB and initiates osteogenic differentiation of mesenchymal cells. Exp. Toxicol. Pathol. 59, 355–363. doi:10.1016/j.etp.2007.11.008
Heljak, M. K., Świeszkowski, W., Lam, C. X. F., Hutmacher, D. W., and Kurzydlowski, K. J. (2012). Evolutionary design of bone scaffolds with reference to material selection. Int. J. Numer. Method. Biomed. Eng. 28, 789–800. doi:10.1002/cnm.2487
Hendrikson, W. J., Deegan, A. J., Yang, Y., van Blitterswijk, C. A., Verdonschot, N., Moroni, L., et al. (2017). Influence of Additive Manufactured Scaffold Architecture on the Distribution of Surface Strains and Fluid Flow Shear Stresses and Expected Osteochondral Cell Differentiation. Front. Bioeng. Biotechnol. 5:6. doi:10.3389/fbioe.2017.00006
Hendrikson, W. J., Rouwkema, J., van Blitterswijk, C. A., and Moroni, L. (2015). Influence of PCL molecular weight on mesenchymal stromal cell differentiation. RSC Adv. 5, 54510–54516. doi:10.1039/C5RA08048G
Henriques, C., Vidinha, R., Botequim, D., Borges, J. P., and Silva, J. A. M. C. (2009). A systematic study of solution and processing parameters on nanofiber morphology using a new electrospinning apparatus. J. Nanosci. Nanotechnol. 9, 3535–3545. doi:10.1166/jnn.2009.NS27
Hollister, S. J., Maddox, R. D., and Taboas, J. M. (2002). Optimal design and fabrication of scaffolds to mimic tissue properties and satisfy biological constraints. Biomaterials 23, 4095–4103. doi:10.1016/S0142-9612(02)00148-5
Holtorf, H. L., Jansen, J. A., and Mikos, A. G. (2005). Flow perfusion culture induces the osteoblastic differentiation of marrow stromal cell-scaffold constructs in the absence of dexamethasone. J. Biomed. Mater. Res. A 72, 326–334. doi:10.1002/jbm.a.30251
Horsnell, H., and Baldock, P. A. (2016). Osteoblastic actions of the neuropeptide Y system to regulate bone and energy homeostasis. Curr. Osteoporos. Rep. 14, 26–31. doi:10.1007/s11914-016-0300-9
Huang, C. H., Chen, M. H., Young, T. H., Jeng, J. H., and Chen, Y. J. (2009). Interactive effects of mechanical stretching and extracellular matrix proteins on initiating osteogenic differentiation of human mesenchymal stem cells. J. Cell. Biochem. 108, 1263–1273. doi:10.1002/jcb.22356
Huang, S., Chen, Z., Pugno, N., Chen, Q., and Wang, W. (2014). A novel model for porous scaffold to match the mechanical anisotropy and the hierarchical structure of bone. Mater. Lett. 122, 315–319. doi:10.1016/j.matlet.2014.02.057
Hutmacher, D. W. (2000). Scaffolds in tissue engineering bone and cartilage. Biomaterials 21, 2529–2543. doi:10.1016/S0142-9612(00)00121-6
Hutmacher, D. W., Sittinger, M., and Risbud, M. V. (2004). Scaffold-based tissue engineering: rationale for computer-aided design and solid free-form fabrication systems. Trends Biotechnol. 22, 354–362. doi:10.1016/j.tibtech.2004.05.005
Isaksson, H., van Donkelaar, C., Huiskes, R., and Ito, K. (2006). Corroboration of mechanoregulatory algorithms for tissue differentiation during fracture healing: comparison with in vivo results. J. Orthop. Res. 24, 898–907. doi:10.1002/jor.20118
Jagodzinski, M., Breitbart, A., Wehmeier, M., Hesse, E., Haasper, C., Krettek, C., et al. (2008). Influence of perfusion and cyclic compression on proliferation and differentiation of bone marrow stromal cells in 3-dimensional culture. J. Biomech. 41, 1885–1891. doi:10.1016/j.jbiomech.2008.04.001
Jagodzinski, M., Drescher, M., Zeichen, J., Hankemeier, S., Krettek, C., Bosch, U., et al. (2004). Effects of cyclic longitudinal mechanical strain and dexamethasone on osteogenic differentiation of human bone marrow stromal cells. Eur. Cell. Mater. 7, 35–41; discussion 41. doi:10.22203/eCM.v007a04
Janik, H., and Marzec, M. (2015). A review: fabrication of porous polyurethane scaffolds. Mater. Sci. Eng. C 48, 586–591. doi:10.1016/j.msec.2014.12.037
Jiang, J., Tang, A., Ateshian, G. A., Edward Guo, X., Hung, C. T., and Lu, H. H. (2010). Bioactive stratified polymer ceramic-hydrogel scaffold for integrative osteochondral repair. Ann. Biomed. Eng. 38, 2183–2196. doi:10.1007/s10439-010-0038-y
Khayyeri, H., Checa, S., Tägil, M., Aspenberg, P., and Prendergast, P. J. (2011). Variability observed in mechano-regulated in vivo tissue differentiation can be explained by variation in cell mechano-sensitivity. J. Biomech. 44, 1051–1058. doi:10.1016/j.jbiomech.2011.02.003
Khayyeri, H., Checa, S., Tägil, M., and Prendergast, P. J. (2009). Corroboration of mechanobiological simulations of tissue differentiation in an in vivo bone chamber using a lattice-modeling approach. J. Orthop. Res. 27, 1659–1666. doi:10.1002/jor.20926
Kreke, M. R., Sharp, L. A., Lee, Y. W., and Goldstein, A. S. (2008). Effect of intermittent shear stress on mechanotransductive signaling and osteoblastic differentiation of bone marrow stromal cells. Tissue Eng. Part A 14, 529–537. doi:10.1089/tea.2007.0068
Kumbar, S. G., James, R., Nukavarapu, S. P., and Laurencin, C. T. (2008). Electrospun nanofiber scaffolds: engineering soft tissues. Biomed. Mater. 3:034002. doi:10.1088/1748-6041/3/3/034002
Lacroix, D., Prendergast, P., Li, G., and Marsh, D. (2002). Biomechanical model to simulate tissue differentiation and bone regeneration: application to fracture healing. Med. Biol. Eng. Comput. 40, 14–21. doi:10.1007/BF02347690
Lacroix, D., and Prendergast, P. J. (2002). A mechano-regulation model for tissue differentiation during fracture healing: analysis of gap size and loading. J. Biomech. 35, 1163–1171. doi:10.1016/S0021-9290(02)00086-6
Li, D., and Xia, Y. (2004). Electrospinning of nanofibers: reinventing the wheel? Adv. Mater. Weinheim 16, 1151–1170. doi:10.1002/adma.200400719
Liao, I. C., Moutos, F. T., Estes, B. T., Zhao, X., and Guilak, F. (2013). Composite three-dimensional woven scaffolds with interpenetrating network hydrogels to create functional synthetic articular cartilage. Adv. Funct. Mater. 23, 5833–5839. doi:10.1002/adfm.201370250
Lin, C. Y., Kikuchi, N., and Hollister, S. J. (2004). A novel method for biomaterial scaffold internal architecture design to match bone elastic properties with desired porosity. J. Biomech. 37, 623–636. doi:10.1016/j.jbiomech.2003.09.029
Liu, C., Abedian, R., Meister, R., Haasper, C., Hurschler, C., Krettek, C., et al. (2012). Influence of perfusion and compression on the proliferation and differentiation of bone mesenchymal stromal cells seeded on polyurethane scaffolds. Biomaterials 33, 1052–1064. doi:10.1016/j.biomaterials.2011.10.041
Liu, W., Huang, C., and Jin, X. (2014). Tailoring the grooved texture of electrospun polystyrene nanofibers by controlling the solvent system and relative humidity. Nanoscale Res. Lett. 9, 350. doi:10.1186/1556-276X-9-350
Ma, P. X., and Zhang, R. (2001). Microtubular architecture of biodegradable polymer scaffolds. J. Biomed. Mater. Res. 56, 469–477. doi:10.1002/1097-4636(20010915)56:4<469::AID-JBM1118>3.0.CO;2-H
Mauney, J. R., Sjostorm, S., Blumberg, J., Horan, R., O’Leary, J. P., Vunjak-Novakovic, G., et al. (2004). Mechanical stimulation promotes osteogenic differentiation of human bone marrow stromal cells on 3-D partially demineralized bone scaffolds in vitro. Calcif. Tissue Int. 74, 458–468. doi:10.1007/s00223-003-0104-7
Meechaisue, C., Dubin, R., Supaphol, P., Hoven, V. P., and Kohn, J. (2006). Electrospun mat of tyrosine-derived polycarbonate fibers for potential use as tissue scaffolding material. J. Biomater. Sci. Polym. Ed. 17, 1039–1056. doi:10.1163/156856206778365988
Megelski, S., Stephens, J. S., Bruce Chase, D., and Rabolt, J. F. (2002). Micro- and nanostructured surface morphology on electrospun polymer fibers. Macromolecules 35, 8456–8466. doi:10.1021/ma020444a
Meinel, L., Karageorgiou, V., Fajardo, R., Snyder, B., Shinde-Patil, V., Zichner, L., et al. (2004). Bone tissue engineering using human mesenchymal stem cells: effects of scaffold material and medium flow. Ann. Biomed. Eng. 32, 112–122. doi:10.1023/B:ABME.0000007796.48329.b4
Melchels, F. P. W., Bertoldi, K., Gabbrielli, R., Velders, A. H., Feijen, J., and Grijpma, D. W. (2010). Mathematically defined tissue engineering scaffold architectures prepared by stereolithography. Biomaterials 31, 6909–6916. doi:10.1016/j.biomaterials.2010.05.068
Melchels, F. P. W., Tonnarelli, B., Olivares, A. L., Martin, I., Lacroix, D., Feijen, J., et al. (2011). The influence of the scaffold design on the distribution of adhering cells after perfusion cell seeding. Biomaterials 32, 2878–2884. doi:10.1016/j.biomaterials.2011.01.023
Merkher, Y., Sivan, S., Etsion, I., Maroudas, A., Halperin, G., and Yosef, A. (2006). A rational human joint friction test using a human cartilage-on-cartilage arrangement. Tribol. Lett. 22, 29–36. doi:10.1007/s11249-006-9069-9
Milan, J.-L., Planell, J. A., and Lacroix, D. (2009). Computational modelling of the mechanical environment of osteogenesis within a polylactic acid–calcium phosphate glass scaffold. Biomaterials 30, 4219–4226. doi:10.1016/j.biomaterials.2009.04.026
Milan, J. L., Planell, J. A., and Lacroix, D. (2010). Simulation of bone tissue formation within a porous scaffold under dynamic compression. Biomech. Model. Mechanobiol. 9, 583–596. doi:10.1007/s10237-010-0199-5
Mohan, N., Dormer, N. H., Caldwell, K. L., Key, V. H., Berkland, C. J., and Detamore, M. S. (2011). Continuous gradients of material composition and growth factors for effective regeneration of the osteochondral interface. Tissue Eng. Part A 17, 2845–2855. doi:10.1089/ten.tea.2011.0135
Moroni, L., de Wijn, J. R., and van Blitterswijk, C. A. (2005). Three-dimensional fiber-deposited PEOT/PBT copolymer scaffolds for tissue engineering: influence of porosity, molecular network mesh size, and swelling in aqueous media on dynamic mechanical properties. J. Biomed. Mater. Res. A 75, 957–965. doi:10.1002/jbm.a.30499
Moroni, L., de Wijn, J. R., and van Blitterswijk, C. A. (2006a). 3D fiber-deposited scaffolds for tissue engineering: influence of pores geometry and architecture on dynamic mechanical properties. Biomaterials 27, 974–985. doi:10.1016/j.biomaterials.2005.07.023
Moroni, L., Poort, G., Van Keulen, F., de Wijn, J. R., and van Blitterswijk, C. A. (2006b). Dynamic mechanical properties of 3D fiber-deposited PEOT/PBT scaffolds: an experimental and numerical analysis. J. Biomed. Mater. Res. A 78, 605–614. doi:10.1002/jbm.a.30716
Mota, C., Puppi, D., Chiellini, F., and Chiellini, E. (2015). Additive manufacturing techniques for the production of tissue engineering constructs. J. Tissue Eng. Regen. Med. 9, 174–190. doi:10.1002/term.1635
Moutos, F. T., Estes, B. T., and Guilak, F. (2010). Multifunctional hybrid three-dimensionally woven scaffolds for cartilage tissue engineering. Macromol. Biosci. 10, 1355–1364. doi:10.1002/mabi.201000124
Moutos, F. T., and Guilak, F. (2010). Functional properties of cell-seeded three-dimensionally woven poly(epsilon-caprolactone) scaffolds for cartilage tissue engineering. Tissue Eng. Part A 16, 1291–1301. doi:10.1089/ten.TEA.2009.0480
Neu, C. P., Komvopoulos, K., and Reddi, A. H. (2008). The interface of functional biotribology and regenerative medicine in synovial joints. Tissue Eng. Part B Rev. 14, 235–247. doi:10.1089/ten.teb.2008.0047
Olivares, A. L., Marsal, È, Planell, J. A., and Lacroix, D. (2009). Finite element study of scaffold architecture design and culture conditions for tissue engineering. Biomaterials 30, 6142–6149. doi:10.1016/j.biomaterials.2009.07.041
O’Reilly, A., and Kelly, D. J. (2016). Unravelling the role of mechanical stimuli in regulating cell fate during osteochondral defect repair. Ann. Biomed. Eng. 44, 3446–3459. doi:10.1007/s10439-016-1664-9
Pauwels, F. (1960). Eine neue Theorie über den Einfluß mechanischer Reize auf die Differenzierung der Stützgewebe. Anat. Embryol. 121, 478–515.
Pearle, A. D., Warren, R. F., and Rodeo, S. A. (2005). Basic science of articular cartilage and osteoarthritis. Clin. Sports Med. 24, 1–12. doi:10.1016/j.csm.2004.08.007
Pérez, M. A., and Prendergast, P. J. (2007). Random-walk models of cell dispersal included in mechanobiological simulations of tissue differentiation. J. Biomech. 40, 2244–2253. doi:10.1016/j.jbiomech.2006.10.020
Petri, M., Ufer, K., Toma, I., Becher, C., Liodakis, E., Brand, S., et al. (2012). Effects of perfusion and cyclic compression on in vitro tissue engineered meniscus implants. Knee Surg. Sports Traumatol. Arthrosc. 20, 223–231. doi:10.1007/s00167-011-1600-3
Prendergast, P. J., Huiskes, R., and Søballe, K. (1997). Biophysical stimuli on cells during tissue differentiation at implant interfaces. J. Biomech. 30, 539–548. doi:10.1016/S0021-9290(96)00140-6
Qi, M. C., Hu, J., Zou, S. J., Chen, H. Q., Zhou, H. X., and Han, L. C. (2008). Mechanical strain induces osteogenic differentiation: Cbfa1 and Ets-1 expression in stretched rat mesenchymal stem cells. Int. J. Oral Maxillofac. Surg. 37, 453–458. doi:10.1016/j.ijom.2007.12.008
Roesler, H. (1987). The history of some fundamental concepts in bone biomechanics. J. Biomech. 20, 1025–1034. doi:10.1016/0021-9290(87)90020-0
Ryan, G., McGarry, P., Pandit, A., and Apatsidis, D. (2009). Analysis of the mechanical behavior of a titanium scaffold with a repeating unit-cell substructure. J. Biomed. Mater. Res. B Appl. Biomater. 90, 894–906. doi:10.1002/jbm.b.31361
Sandino, C., and Lacroix, D. (2011). A dynamical study of the mechanical stimuli and tissue differentiation within a CaP scaffold based on micro-CT finite element models. Biomech. Model. Mechanobiol. 10, 565–576. doi:10.1007/s10237-010-0256-0
Sandino, C., Planell, J. A., and Lacroix, D. (2008). A finite element study of mechanical stimuli in scaffolds for bone tissue engineering. J. Biomech. 41, 1005–1014. doi:10.1016/j.jbiomech.2007.12.011
Sanz-Herrera, J. A., García-Aznar, J. M., and Doblaré, M. (2009). On scaffold designing for bone regeneration: a computational multiscale approach. Acta Biomater. 5, 219–229. doi:10.1016/j.actbio.2008.06.021
Schek, R. M., Taboas, J. M., Segvich, S. J., Hollister, S. J., and Krebsbach, P. H. (2004). Engineered osteochondral grafts using biphasic composite solid free-form fabricated scaffolds. Tissue Eng. 10, 1376–1385. doi:10.1089/ten.2004.10.1376
Sengers, B. G., Taylor, M., Please, C. P., and Oreffo, R. O. C. (2007). Computational modelling of cell spreading and tissue regeneration in porous scaffolds. Biomaterials 28, 1926–1940. doi:10.1016/j.biomaterials.2006.12.008
Shabafrooz, V., Mozafari, M., Vashaee, D., and Tayebi, L. (2014). Electrospun nanofibers: from filtration membranes to highly specialized tissue engineering scaffolds. J. Nanosci. Nanotechnol. 14, 522–534. doi:10.1166/jnn.2014.9195
Shahin, K., and Doran, P. M. (2012). Tissue engineering of cartilage using a mechanobioreactor exerting simultaneous mechanical shear and compression to simulate the rolling action of articular joints. Biotechnol. Bioeng. 109, 1060–1073. doi:10.1002/bit.24372
Shanjani, Y., Amritha De Croos, J. N., Pilliar, R. M., Kandel, R. A., and Toyserkani, E. (2010). Solid freeform fabrication and characterization of porous calcium polyphosphate structures for tissue engineering purposes. J. Biomed. Mater. Res. Part B Appl. Biomater. 93, 510–519. doi:10.1002/jbm.b.31610
Shi, L., Sikavitsas, V. I., and Striolo, A. (2011). Experimental friction coefficients for bovine cartilage measured with a pin-on-disk tribometer: testing configuration and lubricant effects. Ann. Biomed. Eng. 39, 132–146. doi:10.1007/s10439-010-0167-3
Shipley, R. J., Jones, G. W., Dyson, R. J., Sengers, B. G., Bailey, C. L., Catt, C. J., et al. (2009). Design criteria for a printed tissue engineering construct: a mathematical homogenization approach. J. Theor. Biol. 259, 489–502. doi:10.1016/j.jtbi.2009.03.037
Shor, L., Güçeri, S., Chang, R., Gordon, J., Kang, Q., Hartsock, L., et al. (2009). Precision extruding deposition (PED) fabrication of polycaprolactone (PCL) scaffolds for bone tissue engineering. Biofabrication 1, 015003. doi:10.1088/1758-5082/1/1/015003
Sittichockechaiwut, A., Scutt, A. M., Ryan, A. J., Bonewald, L. F., and Reilly, G. C. (2009). Use of rapidly mineralising osteoblasts and short periods of mechanical loading to accelerate matrix maturation in 3D scaffolds. Bone 44, 822–829. doi:10.1016/j.bone.2008.12.027
Skedros, J. G., and Brand, R. A. (2011). Biographical sketch: Georg Hermann von Meyer (1815–1892). Clin. Orthop. Relat. Res. 469, 3072–3076. doi:10.1007/s11999-011-2040-6
Stiehler, M., Bünger, C., Baatrup, A., Lind, M., Kassem, M., and Mygind, T. (2009). Effect of dynamic 3-D culture on proliferation, distribution, and osteogenic differentiation of human mesenchymal stem cells. J. Biomed. Mater. Res. A 89, 96–107. doi:10.1002/jbm.a.31967
Sudarmadji, N., Tan, J. Y., Leong, K. F., Chua, C. K., and Loh, Y. T. (2011). Investigation of the mechanical properties and porosity relationships in selective laser-sintered polyhedral for functionally graded scaffolds. Acta Biomater. 7, 530–537. doi:10.1016/j.actbio.2010.09.024
Sumanasinghe, R. D., Bernacki, S. H., and Loboa, E. G. (2006). Osteogenic differentiation of human mesenchymal stem cells in collagen matrices: effect of uniaxial cyclic tensile strain on bone morphogenetic protein (BMP-2) mRNA expression. Tissue Eng. 12, 3459–3465. doi:10.1089/ten.2006.12.3459
Tang, G., Zhang, H., Zhao, Y., Zhang, Y., Li, X., and Yuan, X. (2012). Preparation of PLGA scaffolds with graded pores by using a gelatin-microsphere template as porogen. J. Biomater. Sci. Polym. Ed. 23, 2241–2257. doi:10.1163/156856211X614185
Treppo, S., Koepp, H., Quan, E. C., Cole, A. A., Kuettner, K. E., and Grodzinsky, A. J. (2000). Comparison of biomechanical and biochemical properties of cartilage from human knee and ankle pairs. J. Orthop. Res. 18, 739–748. doi:10.1002/jor.1100180510
Truscello, S., Kerckhofs, G., Van Bael, S., Pyka, G., Schrooten, J., and Van Oosterwyck, H. (2012). Prediction of permeability of regular scaffolds for skeletal tissue engineering: a combined computational and experimental study. Acta Biomater. 8, 1648–1658. doi:10.1016/j.actbio.2011.12.021
Velasco, M. A., Narváez-Tovar, C. A., and Garzón-Alvarado, D. A. (2015). Design, materials, and mechanobiology of biodegradable scaffolds for bone tissue engineering. Biomed Res. Int. 2015, 729076. doi:10.1155/2015/729076
Visser, J., Melchels, F. P. W., Jeon, J. E., Van Bussel, E. M., Kimpton, L. S., Byrne, H. M., et al. (2015). Reinforcement of hydrogels using three-dimensionally printed microfibres. Nat. Commun. 6, 6933. doi:10.1038/ncomms7933
Wang, T., Feng, Z.-Q., Leach, M. K., Wu, J., and Jiang, Q. (2013). Nanoporous fibers of type-I collagen coated poly(l-lactic acid) for enhancing primary hepatocyte growth and function. J. Mater. Chem. B 1, 339–346. doi:10.1039/C2TB00195K
Williams, J. M., Adewunmi, A., Schek, R. M., Flanagan, C. L., Krebsbach, P. H., Feinberg, S. E., et al. (2005). Bone tissue engineering using polycaprolactone scaffolds fabricated via selective laser sintering. Biomaterials 26, 4817–4827. doi:10.1016/j.biomaterials.2004.11.057
Wise, J. K., Yarin, A. L., Megaridis, C. M., and Cho, M. (2009). Chondrogenic differentiation of human mesenchymal stem cells on oriented nanofibrous scaffolds: engineering the superficial zone of articular cartilage. Tissue Eng. Part A 15, 913–921. doi:10.1089/ten.tea.2008.0109
Keywords: finite element analysis, additive manufacturing, skeletal regeneration, scaffolds, mechano-regulation
Citation: Hendrikson WJ, van Blitterswijk CA, Rouwkema J and Moroni L (2017) The Use of Finite Element Analyses to Design and Fabricate Three-Dimensional Scaffolds for Skeletal Tissue Engineering. Front. Bioeng. Biotechnol. 5:30. doi: 10.3389/fbioe.2017.00030
Received: 17 February 2017; Accepted: 25 April 2017;
Published: 17 May 2017
Edited by:
Giovanni Vozzi, University of Pisa, ItalyReviewed by:
Bahattin Koc, Sabancı University, TurkeyCopyright: © 2017 Hendrikson, van Blitterswijk, Rouwkema and Moroni. This is an open-access article distributed under the terms of the Creative Commons Attribution License (CC BY). The use, distribution or reproduction in other forums is permitted, provided the original author(s) or licensor are credited and that the original publication in this journal is cited, in accordance with accepted academic practice. No use, distribution or reproduction is permitted which does not comply with these terms.
*Correspondence: Lorenzo Moroni, bC5tb3JvbmlAbWFhc3RyaWNodHVuaXZlcnNpdHkubmw=
†These authors have contributed equally to this work.
Disclaimer: All claims expressed in this article are solely those of the authors and do not necessarily represent those of their affiliated organizations, or those of the publisher, the editors and the reviewers. Any product that may be evaluated in this article or claim that may be made by its manufacturer is not guaranteed or endorsed by the publisher.
Research integrity at Frontiers
Learn more about the work of our research integrity team to safeguard the quality of each article we publish.