- 1Department of Biomedical Engineering, University of Texas at Austin, Austin, TX, USA
- 2Institute for Biomaterials, Drug Delivery and Regenerative Medicine, University of Texas at Austin, Austin, TX, USA
- 3Institute for Cellular and Molecular Biology, University of Texas at Austin, Austin, TX, USA
- 4Institute for Computational Engineering and Sciences, University of Texas at Austin, Austin, TX, USA
Wound healing is an intricate process that requires complex coordination between many cell types and an appropriate extracellular microenvironment. Chronic wounds often suffer from high protease activity, persistent infection, excess inflammation, and hypoxia. While there has been intense investigation to find new methods to improve cutaneous wound care, the management of chronic wounds, burns, and skin wound infection remain challenging clinical problems. Ideally, advanced wound dressings can provide enhanced healing and bridge the gaps in the healing processes that prevent chronic wounds from healing. These technologies have great potential for improving outcomes in patients with poorly healing wounds but face significant barriers in addressing the heterogeneity and clinical complexity of chronic or severe wounds. Active wound dressings aim to enhance the natural healing process and work to counter many aspects that plague poorly healing wounds, including excessive inflammation, ischemia, scarring, and wound infection. This review paper discusses recent advances in the development of biomaterials and nanoparticle therapeutics to enhance wound healing. In particular, this review focuses on the novel cutaneous wound treatments that have undergone significant preclinical development or are currently used in clinical practice.
Introduction
Cutaneous injuries are a universal aspect of medical care, with approximately 300 million chronic and 100 million traumatic wound patients worldwide. Wounds have an immense financial burden on health-care systems worldwide, accounting for over $25 billion every year in the US alone (Sen et al., 2009). In addition, the incidence of chronic wounds has rapidly increased due to the rising prevalence of type 2 diabetes, peripheral vascular disease, and metabolic syndrome. Although treatments for acute and small area traumatic wounds are effective, problems arise in the long-term care for patients with large area burns, infected wounds, and chronic wounds. Figure 1 provides a summary of the standard wound care procedures present in many wound care clinics and advanced wound care centers, which are present only in specialized wound care units. Clearly, the need for postsurgical and emergency wound care is on the rise, with new treatments being added to the advanced wound care. However, many mechanistic aspects of wound repair remain poorly understood, we direct the reader to other reviews for further information about detailed mechanisms of wound healing (Martin, 1997; Gurtner et al., 2008; Eming et al., 2014). In addition, we refer the reader to reviews of gene and stem cell therapies for wound healing (Branski et al., 2009; Heublein et al., 2015), role of mechanical forces in wound healing (Agha et al., 2011; Wong et al., 2011a, 2012), and immune response to biomaterial implants (Franz et al., 2011) for further information on those topics. In this review, we will focus on biomaterial- and nanoparticle (NP)-based wound therapeutics, in particular, those that have significant preclinical development or are in clinical trials/clinical use. To provide perspective for these therapies, we will first discuss important aspects of healing infection wounds, burn wounds, and chronic wounds, as these types of wounds would benefit most from improved therapies for wound healing.
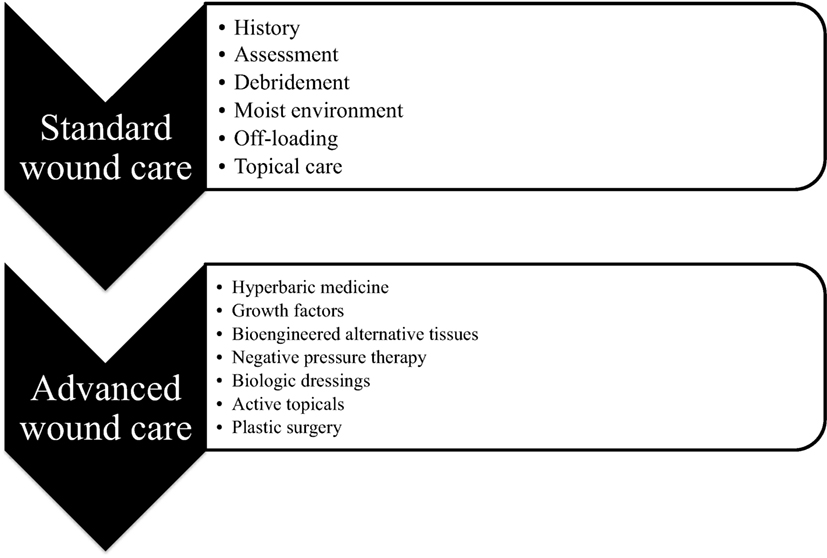
Figure 1. Clinical wound care. Schematic diagram showing the differences between standard wound care that is available at any clinic and advanced wound care that is available only in special wound care units across the country.
The prevalence of chronic wounds has increased rapidly in the past decades, creating a major escalation in health-care costs and patient morbidity (Sen et al., 2009). The chronic wounds are heterogeneous in their presentation and etiology. Many times there is high protease activity, large-scale infection and biofilm formation, ischemia or hypoxia in the tissue, recurrent injury due to neuropathy, or cellular failure leading to gangrene associated with chronic wounds. Chronic wounds most often occur as venous leg ulcers, pressure ulcers, or foot ulcers and are found at elevated rates in patients with diabetes and obesity. The prevalence of type 2 diabetes has increased dramatically in the past years and further drives the problem of chronic wound development (American Diabetes, 2013; Futrega et al., 2014). In addition, neuropathy and microvascular angiopathy are common complications of diabetes and contribute to a 12–25% lifetime risk of developing diabetic ulcers (Singh et al., 2005). Specifically, diabetic foot ulcers are responsible for 25–50% of the total cost of diabetes treatment (Armstrong et al., 2013) and are the most common cause for limb amputations in the US (Larsson et al., 1998). Diabetic ulcer is a complex clinical problem requiring a multifaceted treatment plan with standard therapeutic components, including debridement of necrotic tissue, offloading, infection control, surgical revascularization, and limb elevation/compression (Ayello, 2005; Alexiadou and Doupis, 2012; Andrews et al., 2015). Skin grafts, including bioengineered or artificial skin, autografts, allografts, or xenografts from animal tissue, have produced promising results in clinical trials for diabetic foot ulceration (Santema et al., 2016). While many bioactive approaches have been explored to improve wound care in diabetic patients (Calderini et al., 2014), many of these have been found to be ineffective in clinical trials, and these non-healing wounds remain a major clinical challenge (Steed et al., 1992; Richard et al., 1995; Wieman et al., 1998; Steed, 2006; Uchi et al., 2009; Marti-Carvajal et al., 2015).
Infection control is extremely important due to rising incidence of multidrug-resistant microbes all over the world. The widespread use of antibiotics over the past 20 years has led to the development of antibiotic resistance in bacteria and fungi (Bell et al., 2014). The situation is particularly severe in developing countries where there is little control over the sale and usage of antibiotics. Rampant use of antibiotics has selected for the introduction of bacterial genes from the soil “resistome” into human pathogens, as demonstrated by the presence of the same genes in soil bacteria and human bacteria (Blair et al., 2015). Staphylococcus is the most common infection found in burns and wounds (Dhanalakshmi et al., 2016). Methicillin-resistant Staphylococcus aureus (MRSA) is the most common hospital-borne infection affecting millions of patients daily (Dantes et al., 2013). Interestingly, the microbes affecting patients with chronic infected wounds are dependent on the geographic location as well (Banu et al., 2015; Uckay et al., 2015). In May 2015, the World Health Assembly, a subset of World Health Organization adopted a Global Action Plan against antimicrobial resistance, which shows the urgency of this problem (Assembly, 2015). In December 2015, the US congress has approved a $303 million increase in funding to fight this global epidemic of antimicrobial resistance. Antibiotic-resistant organisms present a major problem in infected wounds, and there is an immense need for engineering new therapies using nanotechnology and advanced biomaterial science to address this problem. The development of efficient antimicrobial drugs against the multidrug-resistant microbes that delay wound healing would provide a substantial benefit to patients with chronic wounds from infection.
Burn injuries also present significant challenges in restoring patient functionality and cosmetic repair. Acute burns result in a sudden influx of inflammatory cytokines and growth factors (Evers et al., 2010). Large area burns often lead to complications, including hypertrophic scarring, facial disfigurement, and loss of muscle and function. Tissue perfusion is of paramount importance to improve wound healing by bringing more nutrients and creating hyperoxyic conditions suitable for healing (Ramasastry, 2005). Current therapeutic regimes for burns are painstakingly long and often still result in scarring and contraction that must be addressed through further therapies and long-term care. Effective wound dressings that induce functional reconstruction following burn injury would have a profound impact on patients with large area burns.
The ideal advanced wound dressing would maintain the wound microenvironment and address the limitations of wound healing specific to the type of wound and patient’s accompanying disease or injury state. It is generally advantageous for wound dressings to be breathable, allowing optimal gaseous exchange and thus protect the periwound skin from maceration and assist in autolytic debridement in removing debris and necrotic tissues. The dressing must also maintain the balanced moist wound environment by donating moisture to the dry wounds and absorbing moisture and exudates from wet wounds. It must act as a barrier to protect against infections and provide thermal insulation to the wound. Finally, advanced wound dressings should aim to enhance aspects of the natural wound healing processes. These could include the promotion of angiogenesis in wounds with poor perfusion, modulation of the immune cells within the wound, enhancement of the invasion and migration of fibroblasts and keratinocytes in the healing wound, and prevention or treatment of infection.
In this review, we focus specifically on the current biomaterial or NP-based therapies that have significant preclinical development or have entered into clinical usage or trials. We discuss the Section “Fundamental Aspects of Wound Healing” briefly followed by sections devoted to “Biomaterial-Based Therapies” and “Nanoparticle-Based Wound Therapies.” We chose to focus on both biomaterials- and NPs-based therapies because with the advent of new and advanced technologies, the fields have become interdependent. In our opinion, we need to be cognizant of the advances in both the fields to be able to develop new therapies to tackle the myriad challenges in the field of wound healing.
Fundamental Aspects of Wound Healing
There are four relatively distinct phases in wound healing process that include hemostasis, inflammation, proliferation, and remodeling (Figure 2). To understand the process better, let us draw analogy with a kingdom at war. The first step during war is to close the gates of the castle that is equivalent to the hemostasis phase of wound healing, which entails clotting of blood mediated by platelets. The next step is to mount a massive attack against the enemy, which is similar to the inflammation phase of wound healing, mediated by macrophages and neutrophils. Then a huge number of repair people like plumbers, roofers, framers, and laborers are recruited to fix the damage done to the castle, which is similar to the proliferation phase of wound healing, mediated by macrophages, lymphocytes, fibroblasts, and keratinocytes. Finally, the renovation of the castle is handled by interior designers for the final redecoration similar to the remodeling phase of wound healing mediated primarily by fibroblasts. Wound healing phases are in delicate balance with each other, especially the inflammation and proliferation phases. If there is too much inflammation during healing, it leads to chronic non-healing wounds that are common in many peripheral vascular diseases and type 2 diabetes patients. On the contrary, too much proliferation during healing leads to scar formation that is not esthetically pleasing and reduces the quality of life. In addition, if there is damage to the underlying muscle tissue, the satellite cells are activated to form myoblasts to initiate the muscle healing process, which generally takes even longer than skin wound healing.
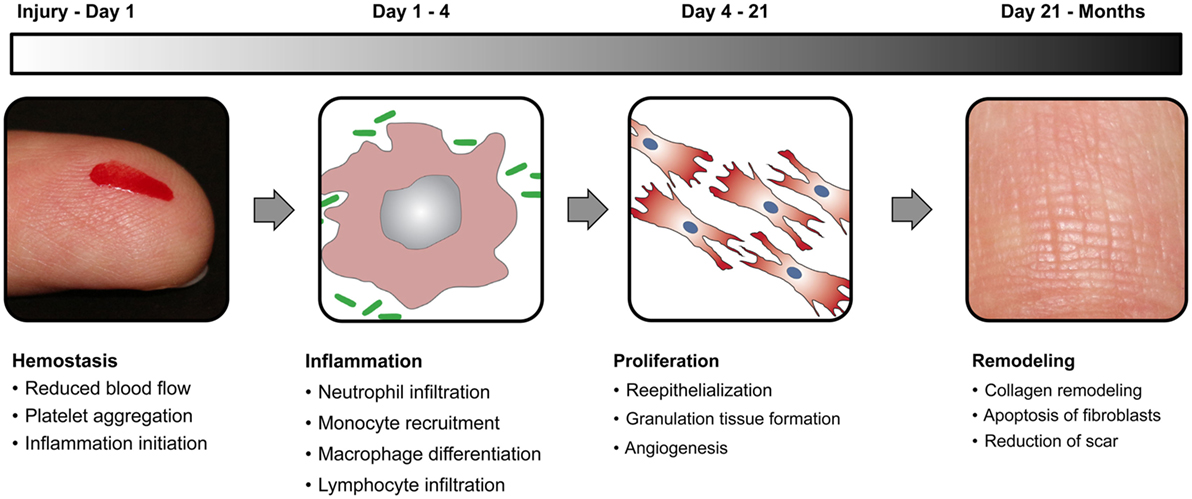
Figure 2. Wound healing phases. Schematic diagram elucidating the four distinct stages of normal wound healing, including hemostasis, inflammation, proliferation, and remodeling, along with the time scale of each phase.
Hemostasis Phase
Following the initial wounding, there is the potential for bleeding and requiring hemostasis (Figure 2). On the battlefield, blood loss or hemorrhage from acute injury is the leading cause of deaths for soldiers (Clifford, 2004). Even though tourniquets are still used in the field to stop blood flow temporarily in large wounds, it may lead to ischemia and reperfusion injury in the tissue (Percival and Rasmussen, 2012). Thus, products to enhance hemostasis are of paramount importance in preventing exsanguination and hemorrhagic shock in people with extensive wounds. The most commonly used methods to facilitate hemostasis are direct pressure on the wound and application of hemostatic materials. Hemostatics can be of three different types including clotting facilitators (e.g., kaolin) and mucoadhesive agents (e.g., chitosan) (Kozen et al., 2008).
Inflammation Phase
The natural response of the immune system to any bodily injury is to monitor the condition and illicit inflammatory reaction to tackle the foreign particles (Figure 2). The classic signs of inflammation have been described since first century AD in Rome and are referred to as dolor (pain), calor (heat), rubor (redness), and tumor (swelling) (Karimbux, 2014). The inflammatory response is mediated by the neutrophils and monocytes (that differentiate into macrophages) (Broughton et al., 2006). The neutrophils are involved in infection control, and macrophages remove cellular debris and provide soluble signals that activate fibroblasts and myofibroblasts in the proliferation phase of wound healing by releasing various kinds of cytokines, proteases, and growth factors in the wound. For patients with chronic conditions, the wounds often acquire a highly inflammatory state, necessitating the use of non-steroidal anti-inflammatory drugs (salicylates, arylalkanoic acids, 2-arylpropionic acids, N-arylanthranilic acids, pyrazolidines, oxicams, and COX-2 inhibitors) (Su et al., 2010) and antibiotics (polymyxins, macrolides, tetracyclines, aminoglycosides, lincosamides, streptogramins, and pleuromutilins) (Martin, 1997).
Proliferation Phase
This is the rebuilding phase of the wound healing process (Figure 2). During the inflammatory phase, the macrophages and neutrophils release various cytokines and chemokines that attract cells into the wound microenvironment, including other lymphocytes, endothelial cells, fibroblasts, myofibroblasts, and keratinocytes (DiPietro, 1995). The keratinocytes migrate from the wound edge, cover the wound bed, and restore barrier function in the skin. The fibroblasts proliferate and secrete various extracellular matrix (ECM) proteins including fibrin, fibronectin, collagen and other ECM proteins that provide a provisional matrix for tissue remodeling and angiogenesis. This forms the granulation tissue of the wound, which is imperative for the proper wound healing (Mayet et al., 2014). Lymphocytes and other immune cells provide additional responses to infectious agents, continuing the processes initiated by the early influx of neutrophils. The endothelial cells under the stimulation of soluble factors released by platelets, macrophages, and other cells initiate neovascularization in the wound bed to improve nutrient and oxygen exchange. The new vessels also aid in the transport of other cells into the affected area facilitating the wound healing process. This step can last anywhere from 4 days to 3 weeks.
Remodeling Phase
The final phase of the wound healing process is the remodeling of the wound and surrounding tissue by the fibroblasts, which start in about 3 weeks after injury and can continue until as long as 2 years (Figure 2). The fibroblasts and myofibroblasts put down a network of collagen fibers and other ECM proteins in an orderly manner while using proteases to degrade existing disordered tissue. The granulation tissue formed during proliferation phase is made up of immature type III collagen and is relatively weak. During remodeling, the fibroblasts gradually replace the type III collagen with mature type I collagen (Hantash et al., 2008). The final goal is to restore the tissue to pre-injury conditions during which the wound becomes gradually less vascularized.
Animal Models for Wound Healing
To study wound healing effectively by mimicking the human wound healing process, a number of wound healing animal models (Kim et al., 2015) have been developed in mouse (Wong et al., 2011b), rat (Dorsett-Martin and Wysocki, 2008), rabbit (Chien, 2007; Aksoy et al., 2009; Pelizzo et al., 2015), and pig (Sullivan et al., 2001). Small mammals such as rats, mice, and rabbits are relatively inexpensive, require fewer resources, have multiple mutant models for delayed wound healing, and thus are easily obtainable. Furthermore, the wound healing process in small mammals is completed in 1–2 weeks instead of weeks or months in human clinical studies. However, a major limitation of these models is the differences in the mechanisms of wound healing in comparison to human wound healing and, in particular, the complexities of a chronic non-healing wound (Ansell et al., 2012). The human skin anatomy is significantly different from that of rats, mice, or rabbits (Ansell et al., 2012). Therefore, the wound healing process is vastly different and hence difficult to compare. In addition, rodents primarily use wound contraction using the underlying thin muscle layer, panniculus carnosus to heal wound, while humans heal wounds by granulation tissue formation (Dunn et al., 2013). Among large animal models, porcine skin has a close structural resemblance to human skin in terms of epidermal thickness and dermal-to-epidermal thickness ratio (Sullivan et al., 2001). They also share similar patterns of hair follicles and vasculature in the skin. Moreover, the dermal collagen and dermal elastic content in porcine skin is more similar to humans than other commonly used mammals (Sullivan et al., 2001). However, a major limitation of wound healing models in pigs is the significant costs of housing and care of the animals, variable wound contraction in pigs depending on the location, and the high rate of growth of pigs that can skew the wound healing process.
Biomaterial-Based Wound Therapies
Biomaterials have been used to enhance wound healing since the earliest medicine-related writings. Ancient writings from the Egyptians include references to the usage of honey, grease, and vegetable fiber for enhancing wound healing (Shah, 2011). Biomaterials have become an integral part of the medical industry since twentieth century. The global biomaterials industry is estimated to be $150 billion and has been growing steadily (Ratner, 2007). After World War II, restricted classes of new polymers were released to the public and were adopted into medical devices. These materials included polyesters, silicones, fluoropolymers, polyurethanes, nylons, and methacrylates (Ratner, 2007). These polymers were then used for biomedical applications, such as vascular grafts, intraocular lenses, hip prostheses, hydrocephalus shunts, kidney dialysis systems, and other medical devices. Currently, the biomaterials used in the medical industry can be broadly classified into three categories: metals (stents, dental implants, etc.), ceramics (orthopedic and dental implants), and polymers (sutures, vascular grafts, joint tissue, soft tissue in general) (Ratner, 1993). Biomaterials have been tremendously important in the wound care industry specifically for wound dressings, cell encapsulation therapies, and NP encapsulation therapies. We will discuss the role of biomaterials in the wound healing applications in this section.
Biomaterials Currently in Development
A summary of the biomaterials currently in development is shown in Table 1.
Standalone Biomaterials
There has been significant rise in engineering biomaterials for the wound care industry (Salamone et al., 2016). An advanced liquid adhesive bandage has been developed that provides a liquid, amphiphilic, siloxysilane polymer-containing coating material, with or without an antimicrobial agent, that can act as a bandage or coating on skin, on a device or on a dressing to prevent damage to wounds, skin, or mucosal tissue resulting from applied pressure, friction, and shear forces (Salamone et al., 2016). Dextran-based hydrogels have been used in third-degree porcine burn wound model and showed enhanced wound closure, reepithelialization, and nerve reinnervation compared to the control group (Sun et al., 2011; Shen et al., 2015). The most promising feature of dextran-based hydrogels for burn wounds is the efficient nerve regeneration compared to the non-adhesive Curity dressing treatment (Figure 3). Biomaterials that are selectively degraded by reactive oxygen species (ROS) have been used to create wound healing scaffolds with matched rates of tissue in growth- and cell-mediated scaffold biodegradation (Martin et al., 2014). These poly-thioketal urethane-based scaffolds were stable for 25 weeks in aqueous conditions but were degraded by tissue ROS in 7 weeks resulting in enhanced wound closure compared to polyester urethane dressings (Martin et al., 2014). Biomaterials based on bioglasses have recently also shown potential in enhancing wound healing and angiogenesis in animal model of wound healing (Mao et al., 2015; Xu et al., 2015; Zhao et al., 2015; Yu et al., 2016; Zhou et al., 2016). For instance, copper-doped borate bioactive glass microfibers were shown to increase both the rate of collagen deposition and angiogenesis in full-thickness wounds in rats (Zhao et al., 2015). In addition, artificial dermal constructs are also important in healing wound defects. Previous methods of preparation resulted in residual aldehydes left over from the manufacturing process. UV cross-linking has been used to create efficient artificial dermal constructs of collagen and glycosaminoglycan mixed in a hydrogel for wound healing applications (Lew et al., 2007). They tested it in vitro with human keratinocytes and found higher cell proliferation and biocompatibility compared to chemically cross-linked constructs. Synthetic hydrogels with variable lengths of polypeptides linked to polyethylene glycol (PEG) have been shown to have both antibacterial and cell adhesive properties (Song et al., 2012). Standalone hydrogels for wound healing is a promising approach and provide a starting point for delivering bioactive agents through direct conjugation or encapsulation as discussed below.
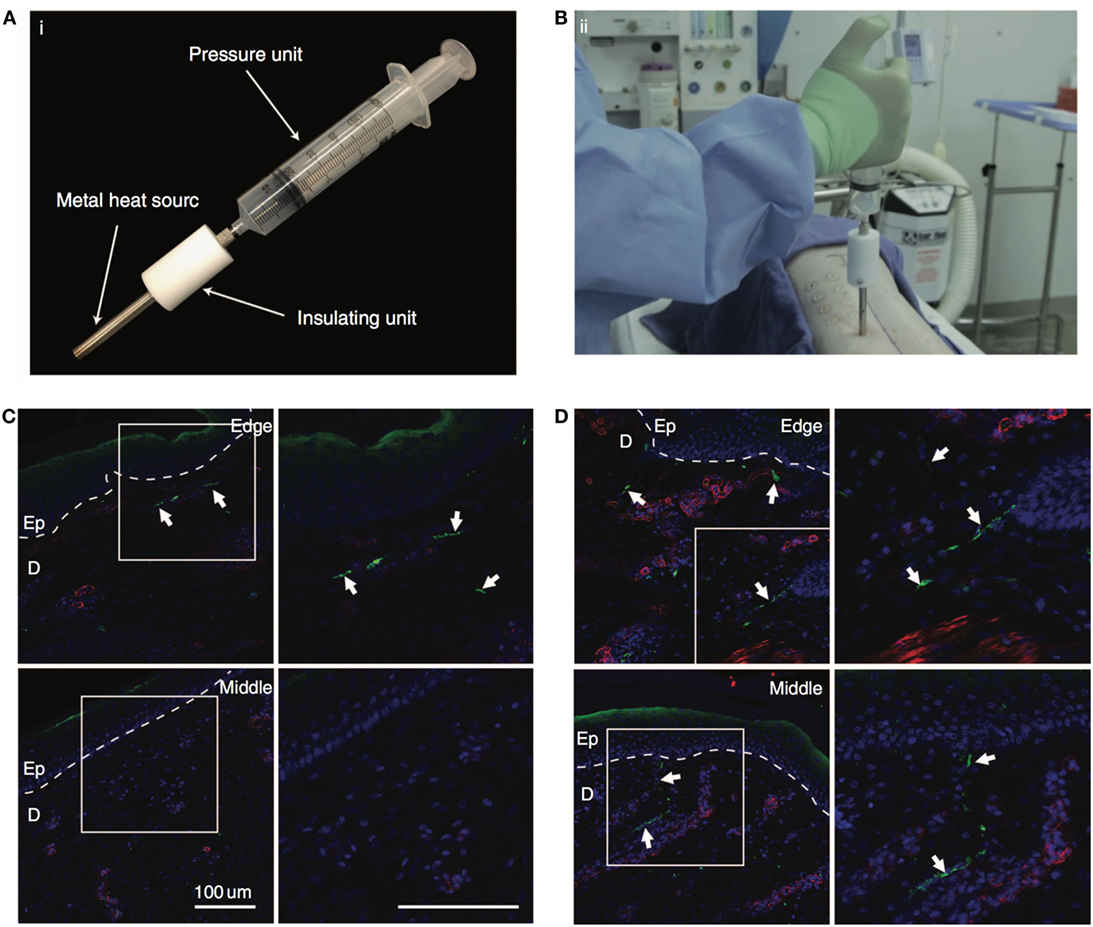
Figure 3. Nerve regeneration in porcine wound model. (A) The custom-made device with pressure unit to create the burn wounds on the dorsal region of porcine skin. (B) The surgeon creating the burn wounds on the thoracic paravertebral region using the custom device. (C,D) Immunostaining of the wound tissue at day 40 treated with non-adhesive Curity dressing (Covidien) and dextran hydrogel, respectively. Upper panel shows the tissue edge, and lower panel shows the middle of the tissue. White arrows show the neuronal fibers. Staining for neurons (PGP9.5) is shown in green, blood vessels (α-smooth muscle actin) in red, and nuclei (DAPI) in blue. Bar = 100 μm. Reproduced with permission from Shen et al. (2015).
Biomaterials Encapsulating Bioactive Components
In the past decades, growth factors were tested as topical treatments to enhance wound healing. However, these growth factor therapies have met with only limited success in clinical trials, and the majority of studies are small- to mid-sized clinical trials that often track only complete wound closure but not percent closure or time to closure, often leaving the clinical recommendation for these therapies unclear. The only approved clinical growth factor treatment for chronic wounds is recombinant platelet-derived growth factor-BB (PDGF-BB) (Becaplermin), and, while approved by the FDA, it has shown mixed results in clinical trials on chronic ulcers and has only seen limited clinical adoption (Fang and Galiano, 2008; Papanas and Maltezos, 2008; Buchberger et al., 2011). Other growth factors, including FGF-2 and epidermal growth factor (EGF), have either shown no improvement or shown only moderate benefits in small clinical trials (Richard et al., 1995; Acosta et al., 2006; Fernandez-Montequin et al., 2009). A structural domain in placenta growth factor-2 (PlGF-2) has been shown to bind strongly to ECM proteins, including fibronectin, vitronectin, tenascin, osteopontin, fibrinogen, and collagen I. By fusing this domain to other growth factors like vascular endothelial growth factor-A (VEGF-A), PDGF-BB, and bone morphogenetic protein-2 (BMP-2), super-affinity growth factors were created (Martino et al., 2014). These engineered growth factors were shown to significantly enhance diabetic wound healing in mice compared to native growth factors. A recent study delivered amniotic fluid-derived stem (AFS) cells, which are known to secrete cytokines and growth factors, in full-thickness skin wounds in a nu/nu murine model (Skardal et al., 2016). The AFS cells have only a limited lifetime in vivo, but a photo-crosslinkable heparin-conjugated hyaluronic acid hydrogel preserved the growth factors and cytokines produced by the cells, allowing these paracrine factors to be released into the wound. The treatment improved wound closure with enhanced reepithelialization and increased vascularization and production of ECM (Skardal et al., 2016). Hyaluronic acid-based wound dressings that release α-tocopherols (vitamin E) have been shown to improve wound healing (Pereira et al., 2016). Since the tissue microenvironment has a plethora of chemical cues, delivering of one bioactive component may not be enough to address all the issues of impaired wound healing. In addition, temporal control in release of factors, correlating with the different phases of wound healing, would likely be an important aspect for promoting healing in complex wounds.
Biomaterials Encapsulating Cell Therapies
Stem cells or progenitor cells have great regenerative potential especially during injury to the body. In order to target chronic wounds, mesenchymal stem cells (MSCs) were engineered to overexpress the vascular endothelial growth factor (VEGF) gene and encapsulated in a poly(β-amino ester) hydrogel (Yang et al., 2010). Fibrin and PEG gels encapsulating adipose-derived stem cells (ASCs) from discarded burn skin samples were tested in a rat excisional wound model (Zamora et al., 2013). The ASCs significantly improved wound healing outcomes by day 16 compared to the controls. Stem cell therapies delivered in biomaterials have also shown promise for burn wound healing (Ozturk and Karagoz, 2015), diabetic ulcers (Heublein et al., 2015), and cutaneous wound healing (Branski et al., 2009). Microporous annealed particle (MAP) gels were created by mixing pre-gel mixture including a PEG-VS containing an RGD peptide region and matrix metalloproteinase (MMP) substrate with MMP crosslinker solution in a microfluidic channel to make gels that were annealed using FXIIIa into a final microporous scaffold (Griffin et al., 2015). The gels were loaded with dermal fibroblasts and MSCs during in vitro studies and found to have robust tubular network formation within 48 h. MAP gels combine the important wound dressing properties of injectability and microporosity that provide mechanical support for rapid cell migration, molecular cues to direct cell adhesion, and resorption after tissue regeneration (Griffin et al., 2015). MAP gels have shown to be effective in wound healing in both in vitro and mouse model of excisional wound healing. Encapsulation of newly developed induced pluripotent stem cells (Li and Li, 2014), reprogrammed adult cells, and other stem cells in hydrogels will be interesting while modulating the hydrogel properties to mimic the tissue microenvironment.
Nuclei Acid Delivering Biomaterials
A comprehensive genetic study mapping the gene expression profiles during the cutaneous wound healing has motivated the development of gene delivery strategies in facilitating wound healing (Deonarine et al., 2007). Biomaterial-based nucleic acid delivery systems reduce degradation, enhance uptake, and control the treatment dose. Such gene delivery systems can be formulated from biocompatible, biodegradable, and FDA-approved polymers such as poly-l-lactic acid and poly-d,l-lactide-co-glycolide (Kim et al., 2005). Collagen hydrogels encapsulating DNA encoding the PDGF-BB gene is effective in accelerating wound healing in ischemic dermal ulcers in New Zealand white rabbits (Tyrone et al., 2000). Hyaluronic acid-based porous hydrogels containing an MMP-degradable linker that encapsulates the VEGF plasmid DNA results in pro-angiogenic effects and improved diabetic wound healing in mice compared to non-porous hydrogels (Tokatlian et al., 2015). Since PDGF-BB is the only FDA-approved growth factor for diabetic foot ulcers, there have been numerous attempts in enhancing the gene delivery methods for PDGF (Petrie et al., 2005).
The skin microenvironment is teeming with proteases including MMPs, which are significantly upregulated during the wound healing process (Madlener et al., 1998; Steffensen et al., 2001; Rohani and Parks, 2015). These proteases degrade therapeutic proteins like growth factors, cytokines, and chemokines; and ECM proteins including collagen, fibronectin, and vitronectin. Therefore, silencing these proteases has become an important goal in wound healing research. Small interfering RNA (siRNA) can provide gene-specific silencing and present a safe and effective route for knockdown of inflammatory or other target proteins in chronic skin wounds. To modulate the release kinetics and injectability, pH-responsive smart polymer nanoparticles (SPN) are loaded with an injectable polyurethane scaffold (Nelson et al., 2013). The SPNs feature electrostatic loading, nuclease protection of siRNA, and pH-dependent membrane disruptive activity. Polyurethane formulations can be directly injected into a wound or defect where they cure into mechanically robust, biodegradable scaffolds that conform precisely to the shape and size of the wound. In this study, they delivered siRNA against GAPDH along with PDGF-BB encapsulated in the scaffold and showed enhanced excisional wound healing mice (Nelson et al., 2013). Another family of self-assembled wound dressings silence MMP-9 and improve wound healing in diabetic mice (Castleberry et al., 2015). Cationic star-shaped polymers have been used as siRNA carrier for reducing MMP-9 expression in skin fibroblast cells and promoting wound healing in diabetic rats (Li et al., 2014). There are many appealing aspects of delivering RNA-based therapeutics including those using miRNA, lncRNA, piRNA, or shRNA; however, delivering these in an effective manner remains a major objective in the field.
Animal Product-Based Biomaterials
Biomaterials derived from natural products can provide materials with greater complexity and composition. In order to mimic the ECM conditions of the wound and to provide a scaffold for the fibroblasts for collagen deposition, ECM-based therapies have gained popularity. The porcine small intestine submucosa that has been lyophilized and sterilized has been recently shown to provide similar benefits to those provided by fish skin-based products for enhancing healing dermal wounds (Mostow et al., 2005). Similarly, lyophilized bovine amniotic membrane has been effective in wound healing applications (Kang et al., 2013). The silk protein, fibroin, is an effective scaffolding material providing a fine mesh for the cells to grow and enhance wound healing (Hasatsri et al., 2015). A phase I clinical trial using fibroin to enhance wound healing is currently underway. Finally, there have been numerous marine polysaccharide hydrogels like marine collagen from Stomolophus nomurai meleagris, Oncorhynchus keta, Lates calcarifer, Stichopus japonicas, and Salmo salar, alginate from Macrocystis pyrifera, chitosan from crabs and shrimps, which are bioactive and increase wound healing rates in mice (Chandika et al., 2015). There is rising need for quality control to prevent transmission of diseases and pathogens from animal products. Lyophilization, pasteurization, and sterilization are important techniques to reduce cross contamination. The major concerns with natural products include batch-to-batch variability, long-term immunogenicity, and safety (Pashuck and Stevens, 2012; Prestwich et al., 2012). Animal product-based biomaterials have been explored only in a limited manner until for chronic wounds, leaving tremendous potential for the development of new therapies in future studies.
Antibacterial and Drug-Loaded Biomaterials
A better understanding of the molecular mechanisms underlying the antibiotic resistance will help engineer efficient drugs to target these resistant organisms (Blair et al., 2015). Potential modes of resistance include reduced permeability to antibiotics, increased efflux of antibiotics, alterations in antibiotic targets through mutations, modification or protection of targets, and direct chemical modifications of the antibiotics. Bacterial resistance to antibiotics is inversely correlated with the rate of metabolism, with lower metabolism leading to higher resistance and vice versa (Bryan and Van Den Elzen, 1977; Kohanski et al., 2007; Allison et al., 2011; Martinez and Rojo, 2011). Recently, it has been shown that both Gram-negative bacteria and Gram-positive bacteria were killed by kanamycin when the microbes were pretreated with alanine or glucose that promotes the TCA cycle by substrate activation (Peng et al., 2015). Finally, increasing the microbial ROS production makes the Escherichia coli susceptible to antibiotics (Brynildsen et al., 2013).
Biomaterial-based wound dressings are ideal for loading drugs or antibiotics due to their tunable properties and release kinetics. Chitosan microspheres loaded with silver sulfadiazine encapsulated in PEG fibrin gels showed robust antimicrobial activity against S. aureus and Pseudomonas aeruginosa (Seetharaman et al., 2011). Streptomycin has been loaded into polyethylene oxide (PEO) polymer composite films (Pawar et al., 2013), PEO with carrageenan composite films (Boateng et al., 2013), and PEO with alginate composite films (Pawar et al., 2014) for improving wound healing. Similarly, ciprofloxacin has been loaded into electrospun polyurethane and dextran dressings (Unnithan et al., 2012) and PEG–chitosan scaffold (Sinha et al., 2012). Many other antibiotic agents have been loaded into various natural, synthetic, or composite wound dressings. There is a revived interest, and recent academic and industrial research spending has increased with the goal of developing efficient and targeted antimicrobial drugs that have enhanced uptake, reduced degradation, and metabolic mimicry to increase uptake. Apart from antimicrobials, many small molecule drugs and intermediates have been delivered using biomaterial-based systems (Hubbell, 1996). The drug 1,4-dihydrophenonthrolin-4-one-3-carboxylic acid (1,4-DPCA), which is a prolyl hydroxylase (PHD) inhibitor was loaded in a locally injectable hydrogel to achieve controlled delivery of the drug over 4–10 days (Zhang et al., 2015b). This stabilized the constitutive expression of hypoxia-inducible factor-1α (HIF-1α) protein, an important factor in wound healing and angiogenesis.
Biomaterials in Clinical Usage
A summary of the biomaterials currently in clinical usage is shown in Table 2.
Thin Films, Foams, and Wafers
The most commonly used wound dressings are thin flexible sheets of transparent polyurethane with adhesive backing. These dressings are transparent, allowing clinicians to visualize the skin and are also permeable to water vapor, O2, CO2, but impermeable to bacteria and water. Thus apart from wound healing, they are widely used in sealing the vascular access devices, especially in catheters and saline drip since they are highly elastic and conform to the body contours. The widespread usage of these thin films, impregnated with chlorhexidine, has reduced the incidence of central line infections significantly (Jeanes and Bitmead, 2015). They are also used in superficial wounds, partial-thickness wounds, sutured wounds, donor graft sites, granular wounds, slough-covered wounds with minimal drainage and lacerations or abrasions (Stashak et al., 2004). However, these dressings are ineffective in wounds with high moisture and exudate content since they have minimum absorptive capacity and thus can cause tissue maceration. They should not be used in highly infected wounds and places where the skin is sensitive or fragile because the skin might tear while removing the dressing.
In many cases, excessive exudate secretion is detrimental to the wound healing process. Polyurethane absorptive foam dressings have been developed with a hydrophilic surface to interface with the wound, while the hydrophobic surface faced outside environment. These dressings are permeable to gas, but not to bacteria and other pathogens. Unlike the films, these dressings are highly absorptive and are used in wounds with minimal to heavy exudates, granulating or slough-covered partial-thickness wounds, donor sites, minor burns, diabetic ulcers, and venous insufficiency ulcers (Banks et al., 1997). Foam dressings impregnated with methylene blue have also been used for a bacteriostatic effect (Coutts et al., 2014). The advantages of foam dressings are their ease of use, remarkable absorptive capacity, and availability in various degrees of adhesivity and occlusivity. However, the absorptive aspect of polyurethane foams makes them inappropriate for dry or eschar-covered wounds and arterial ulcers.
Hydrocolloids such as pectin, gelatin, and carboxymethyl cellulose along with adhesives and polymers are used to prepare wafers in thin dressings. These dressings contain hydrophilic colloidal particles with a strong adhesive backing that only need a small area of intact skin to secure, eliminating the need for taping over the dressing (Hutchinson and McGuckin, 1990). These dressings have moderate absorptive capacity but are highly occlusive and are effective barrier against urine, stool, and microbes. Thus, they are used in partial and full-thickness wounds, granular and necrotic wounds, sacral and coccygeal pressure ulcers, minor burns, and venous insufficiency ulcers. However, the hydrocolloidal dressings are contraindicated for heavily draining wounds, infected wounds, arterial ulcers, third-degree burns, and exposed tendons/fascia (Kannon and Garrett, 1995).
Glycerin and Alginate Hydrogels
Hydrogels are extensively used in preparing wound dressings. There are many hydrogels in clinical use both in wet and dry (lyophilized) forms. Glycerin-based wound dressings with high water content are available in sheets, gels, or impregnated gauzes (Baum and Busuito, 1998). These are highly moistened and thus absorb minimal amount of fluid but donate moisture to dry wounds. These dressings are permeable to gas and water and are almost always non-adhesive and require secondary bandages. Therefore, these are mainly used for minimally draining wounds, superficial and partial-thickness wounds, softening eschared wounds by moisture and provide padding to decrease shear forces on the wounds (Kirsner, 2016). However, the glycerin dressings are contraindicated in heavily draining wounds and infected wounds.
Another class of hydrogel wound dressings that are widely used is the alginate-based dressings. Alginic acid is extracted from seaweed, converted into sodium salts, and cross-linked with calcium. These dressings are hydrophilic to provide a moist wound environment and are highly absorptive if delivered in a lyophilized form. Since these dressings are highly permeable and non-occlusive, a secondary dressing is needed to keep them in place (Gu et al., 2014). Alginate hydrogels can be fashioned as both sheets for the surface wounds and ropes for the deep wounds. They also are versatile in providing a delivery platform and can be impregnated with silver, honey, and sodium chloride for additional antimicrobial and hyperosmotic properties. Thus, these dressings are used in moderate to highly draining wounds, partial- and full-thickness draining wounds, and infected wounds (Kirsner, 2016). However, they are contraindicated in dry or minimally draining or eschar-covered wounds, arterial ulcers, and exposed deeper structures tendon, joint capsule, or bone.
Hemostatics
The most common structural protein in the animal world, collagen, has been used extensively to create hemostatic biosynthetic dressings. The collagen fragments in the dressings induce cell proliferation and chemotaxis while reducing matrix MMP activity (Ruszczak, 2003). MMPs are tissue proteases or endopeptidases that are zinc containing, calcium dependent, and are crucial for wound remodeling phase because they preferentially break down ECM components in the skin (Birkedal-Hansen et al., 1993). Despite the limited studies, and the need for improved study designs and increased number of randomized controlled trials, wound dressings containing collagen appear to have some benefit in the treatment of diabetic foot ulcers and should be carefully considered by clinicians that manage wounds (Holmes et al., 2013). Carboxymethyl cellulose or oxidized regenerated cellulose (ORC) combined with collagen leads to decreased MMP activity, increased cell proliferation, and chemotaxis (Cullen et al., 2002).
Composites
To combine the benefits of different kinds of biomaterial dressings, composite dressings have been designed with multiple layers of different biomaterials (Pillay et al., 2015). The bottom or innermost layer is generally composed of a semi or non-adhesive material that allows the wound exudate to flow to the next layer, and it also conforms to the wound’s granulation tissue. This layer is thin, non-adherent/adherent, and non-toxic woven/non-woven mesh. They are often made of polyurethane or polyester, PTFE, and sometimes contain silicone and petroleum complements. They are applied directly to the wound bed and allow the drainage to pass through. The middle layer comprises of highly absorptive material that pulls the wound exudate away from the wound but keeps the environment moist. This reduces skin maceration due to excess moisture and reduces bacterial growth and improves autolytic debridement. The top most or outermost layer is highly occlusive in nature and protects the wound from infection (Wittaya-areekul and Prahsarn, 2006; Elsner et al., 2010). These multilayer dressings can be used as both primary and secondary dressings.
Other Biomaterial-Based Wound Dressings
Apart from standard wound dressings, biomaterials have been used to develop skin protectants (Hoggarth et al., 2005), skin sealants (Kemp, 1994), moisture barriers (Zehrer et al., 2005), and keratolytics (Zehrer et al., 2005) that can be delivered as a cream. Skin protectants are often applied to the wound and periwound skin. They prevent maceration of the periwound skin by wound fluid and also prevent rashes and skin breakdown in areas of leakage (Hoggarth et al., 2005). Skin sealants are liquid-based poly-vinyl-methyl (PVM) polymers that form a protective waterproof, breathable, transparent layer on the skin on drying (Kemp, 1994). This protects the periwound skin from moisture, adhesives, and shear stress. Skin sealants work well with adhesive dressing application. The moisture barriers comprise of creams or ointments containing petrolatum, dimethicone, and zinc oxide. Some also contain antifungal miconazole especially useful in treating intertrigo (inflammation of skin folds) (Zehrer et al., 2005). Moisturizers are more hydrophilic creams, lotions, or gels that donate moisture to the wound or periwound skin. Keratolytics soften the hard scales and calluses and hyperkeratotic lesions and include salicylic acid, urea, ammonium lactate creams (Zehrer et al., 2005). However, these keratolytics can cause skin maceration if overused.
Nanoparticle-Based Wound Therapies
The global nanotechnology industry reached over $1.5 trillion in 2014, becoming a major economic force (Sahoo et al., 2007; Zhou et al., 2014). A central constituent of the nanotechnology industry is engineered NPs. The number of consumer products containing NPs is growing at a rapid pace and is expected to reach 10,000 by the year 2020. NPs have emerged as a new class of therapeutics in the last couple of decades due to their ability to be targeted and low toxicity. NPs are generally defined as particles ranging from 1 to 100 nm in size. These small particles often have different physical and chemical properties from bulk materials. These properties may include alterations in melting points, specific surface areas, specific optical properties, mechanical strengths, and specific magnetizations. These unique properties make them attractive for various industrial and medical applications.
Nanoparticles have become significant in the regenerative medicine field in the last two decades (McLaughlin et al., 2016). Many biological processes happen at through mechanisms that fundamentally act at the nanometer scale. Thus, materials such as NPs can be used as unique tools for drug delivery, imaging, sensing, and probing biological processes (Wang and Wang, 2014). In the context of wound healing, the special properties of NPs like electric conductivity, antimicrobial activity, high surface to volume ratio, swelling, and contraction make NPs versatile resources. In the following sections, we will specifically talk about various NP-based therapeutics that are either undergoing preclinical development or in current clinical use.
Nanoparticles Currently in Development
The NP-based wound therapies under development are summarized in Table 3.
Metal Nanoparticles
Silver nanoparticles (AgNPs) are the most widely studied among metal NPs. These NPs have been shown to enhance healing in a full-thickness excisional wound model in mice (Liu et al., 2010). Dressings impregnated with AgNPs have also been shown to be effective in wound healing in normal and diabetic mice (Tian et al., 2007). The antimicrobial properties of silver have been exploited in toxicity evaluation in human ASCs (Samberg et al., 2012), human cancer lines (Arora et al., 2008), human keratinocytes (Samberg et al., 2010), and other human cell lines (AshaRani et al., 2009). In addition, AgNPs have also been shown to be anti-inflammatory in a peritoneal adhesion model (Wong et al., 2009). Recently, the safety and efficacy of collagen-coated AgNPs encapsulated in collagen hydrogels was shown in primary human skin fibroblasts and keratinocytes; while antimicrobial properties were shown against S. aureus, Staphylococcus epidermidis, E. coli, and P. aeruginosa (Alarcon et al., 2015). To gain insight on the health and environmental impact of AgNPs, they were tested on zebrafish models (Asharani et al., 2008) and found that the NPs induce a dose-dependent toxicity in embryos. This may support a non-specific action of AgNPs on all cell types including the wounded host cells. Magnesium fluoride (MgF2) NPs (Lellouche et al., 2009) made using the standard microwave method (Jacob et al., 2006) are highly effective against nosocomial microbes including E. coli and S. aureus. Topical application of water-soluble cerium oxide NPs (Nanoceria) accelerates the healing of full-thickness dermal wounds in mice (Chigurupati et al., 2013). The mechanism of action is thought to be the strong antioxidant properties of cerium oxide NPs. Similarly, copper NPs have also been shown to enhance wound healing in excisional wounds of mice (Rakhmetova et al., 2010). Iron oxide NPs conjugated to thrombin have been used to enhance wound healing compared to free thrombin (Ziv-Polat et al., 2010). This was achieved by increasing the stability of thrombin via conjugation to the iron oxide. Gold NPs co-delivered with epigallocatechin gallate and α-lipoic acid significantly accelerated mouse cutaneous wound healing through anti-inflammatory and anti-oxidation effects (Leu et al., 2012). Gold NPs conjugated to siRNA-based spherical nucleic acids (SNAs) have been used for diabetic wounds with ganglioside–monosialic acid 3 synthase (GM3S) knockdown (Randeria et al., 2015). GM3S is an enzyme that is overexpressed in diabetic mice and may cause insulin resistance and reduced wound healing. In vivo studies with diet-induced obese diabetic mice showed decreases in local GM3S expression by >80% at the wound edge through an siRNA pathway and fully heals wounds clinically and histologically within 12 days, whereas the control-treated wounds were only about half of the wounds were closed (Figure 4). Gold NPs have also been used with de-cellularized porcine diaphragm as a scaffold for migrating wound cells (Cozad et al., 2011). Among all the metal NPs, we think that the most promising therapeutic options are the gold and silver NPs because of their versatility. While silver is antimicrobial and anti-inflammatory, gold can be easily functionalized for precise delivery of drug or cargo.
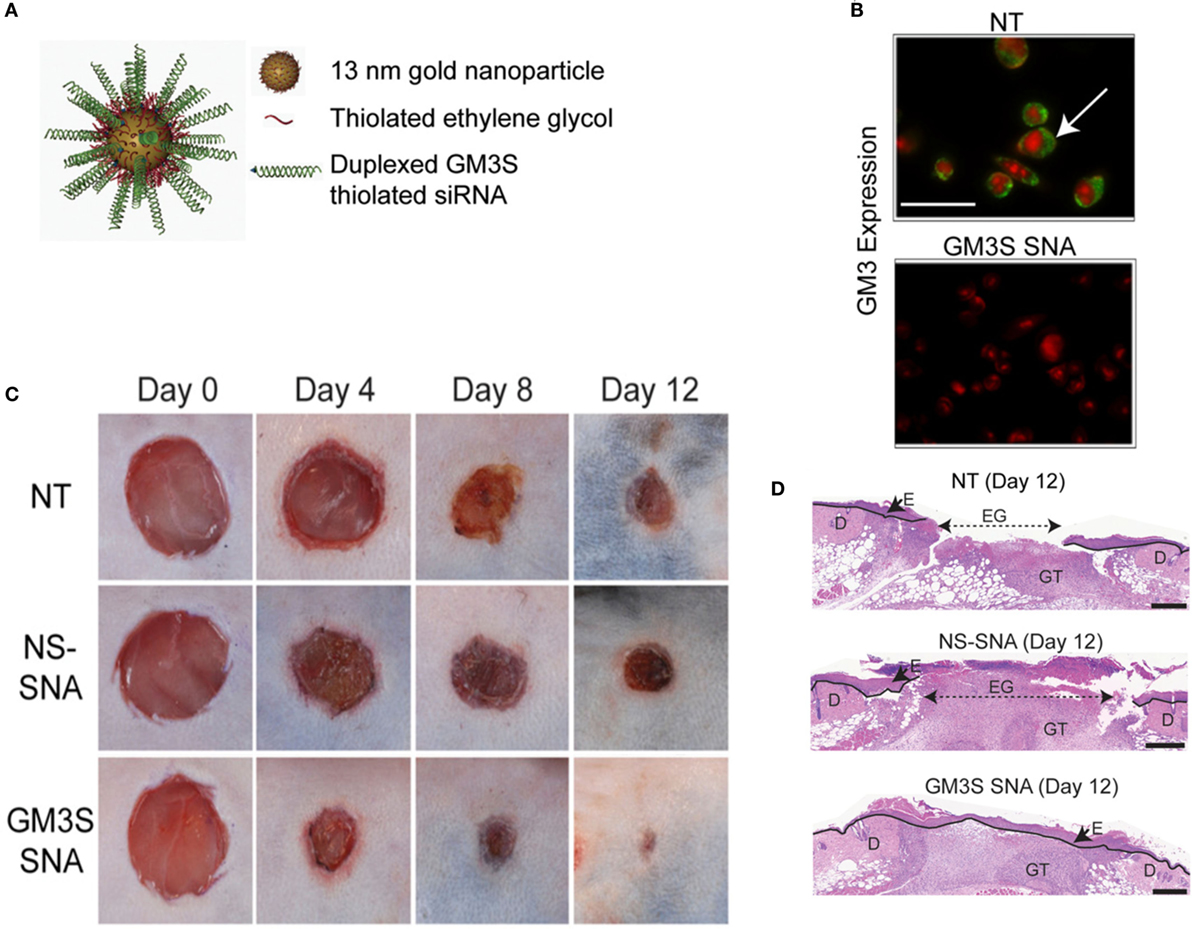
Figure 4. Overcoming insulin resistance to efficiently heal wounds in a diabetic mouse model. (A) Schematic representation of the gold nanoparticles conjugated to ganglioside–monosialic acid 3 synthase (GM3S) siRNA called the spherical nucleic acid (SNA). The SNA surface is passivated with oligoethylene glycol for colloidal stability. GM3S is a known target that is overexpressed in diabetic mice and responsible for causing insulin resistance and impeding wound healing. (B) Confocal images show elimination of GM3 in keratinocytes treated with GM3S SNA (lower) relative to no treatment NT (upper). Green stained GM3; red stained nuclei. Bar = 50 μm. (C) Macroscopic clinical images of the wounds in a diabetic diet-induced obesity mouse model over the course of 2 weeks with three different treatments. (D) Representative histologic images of the treated wounds at day 12. D, dermis; E, epidermis; EG, epidermal gap; GT, granulation tissue. Bar = 500 μm. NS, non-sense; NT, non-treated. Reproduced with permission from Randeria et al. (2015).
Antibiotic-Loaded Nanoparticles
There has been a recent surge in advanced therapeutics targeting the multidrug-resistant microbes using antibiotics linked to NPs, commonly referred to as nanobiotics. New classes of polyacrylate NPs that are conjugated to antibiotics were created to treat MRSA (Turos et al., 2007). They consist of water-insoluble N-thiolated beta-lactam antibiotics covalently conjugated to the nanopolymer. These nanobiotics significantly increased antimicrobial activity of the antibiotics in comparison to the non-conjugated antibiotic formulation. Similarly, poly(butyl acrylate-styrene) NPs conjugated to N-thiolated beta-lactam antibiotic have been prepared with conventional and polymerizable surfactants have showed higher antimicrobial activity while maintaining low toxicity (Garay-Jimenez et al., 2009). Gelatin, chitosan, and epigallocatechin gallate NPs have also been incorporated in a polyglutamic acid and gelatin hydrogels containing activated carbon fibers with gentamicin, to create a wound dressing to enhance regeneration and inhibit microbial growth (Lin et al., 2015). Vancomycin-modified NPs produced by magnetic confinement are also highly effective against both Gram-positive and Gram-negative bacteria (Kell et al., 2008). In addition, folic acid-tagged chitosan NPs have been used as “Trojan horses” to deliver vancomycin into bacterial cells and efficiently kill them (Chakraborty et al., 2010). With the rise in antibiotic-resistant bugs, the need for therapies targeting Gram-negative bacteria has become urgent matter, and NP delivery systems may provide a means to enhance the activity of conventional antibiotics in the wound environment.
Nitric Oxide Releasing Nanoparticles
Nitric oxide (NO) plays numerous roles in wound healing and can regulate deposition of ECM proteins, cell proliferation, and endothelial function. Incorporation of a functional group of diazeniumdiolate into materials results in the release of biologically active NO when exposed to an aqueous environment (DeRosa et al., 2007). There have been several studies showing the increased wound healing rate due to delivery of NO in a wound microenvironment (Blecher et al., 2012; Han et al., 2012). Biofilms of P. aeruginosa, E. coli, S. aureus, S. epidermidis, and Candida albicans were formed in vitro and exposed to NO-releasing silica NPs, which showed greater than 99% kill rate (Hetrick et al., 2009). It is interesting to note that endogenous NO may actually protect bacteria against antibiotics and other microorganisms (Gusarov et al., 2009). NO-mediated resistance is achieved through both the chemical modification of toxic compounds and the alleviation of the oxidative stress imposed by many antibiotics. Thus, inhibition of bacterial NO synthase might be a suitable future target to enhance antimicrobial therapy.
Green Synthesized Nanoparticles
Green synthesis of NPs involves using plant products or extracts that are less expensive and less harmful to the environment than the standard physicochemical methods that are generally used (Makarov et al., 2014). Genipin is prepared from geniposide by using the enzyme β-glucosidase, which is extracted from Penicillium nigricans. Genipin cross-linked with chitosan along with PEG and silver NPs are blended into a nanocomposite for enhanced wound healing and high antimicrobial activity (Liu et al., 2012). The formulated silver NPs using Coleus forskohlii root extract has been shown to be effective in healing full-thickness excision wounds in albino Wistar male rats (Naraginti et al., 2016). Silver NPs were produced in octadecylamine-modified montmorillonite clay that was mixed with extracts from Homalomena aromatica then mixed with hyper branched epoxy to create silver nanocomposite for wound healing applications (Barua et al., 2015). This nanocomposite served as an efficient wound healing scaffold with inherent antimicrobial properties.
Lipid Nanoparticles
Lipid-based NPs have given rise to an entire subfield of lipid nanotechnology (Mashaghi et al., 2013). Liposomes are versatile drug delivery system due to their ease of protein delivery, biocompatibility, intracellular delivery, modulation of size, charge, and surface properties (Safinya and Ewert, 2012). It has been shown that the loss of growth factor co-receptors in diabetic diseased state leads to growth factor resistance (Das et al., 2014), which may prevent the effectiveness of growth factor treatments to induce angiogenesis and wound healing. One method to overcome this resistance is to deliver co-receptors in a proteoliposome along with the growth factors. This was tested in a diabetic mouse model and showed improved diabetic wound healing (Das et al., 2016a,c) and enhanced ischemic revascularization (Jang et al., 2012; Das et al., 2014, 2016b; Monteforte et al., 2016)(Figure 5). There are various other lipid NPs, which have shown promise for treating peripheral vascular disease and critical limb ischemia, reviewed elsewhere (Tu et al., 2015). Solid lipid nanoparticles (SLN) are a new pharmaceutical delivery system with a solid lipid core stabilized by surfactants, which can solubilize lipophilic molecules. These SLNs have been tested for delivering bioactive molecules such as opioids like morphine (Kuchler et al., 2010b), resveratrol (Gokce et al., 2012), and silver sulfadiazine (Sandri et al., 2013) for wound healing (Kuchler et al., 2009, 2010a). Exosomes are another form of lipid NPs produced by cells and have been shown to be effective for wound healing (Rani and Ritter, 2015).
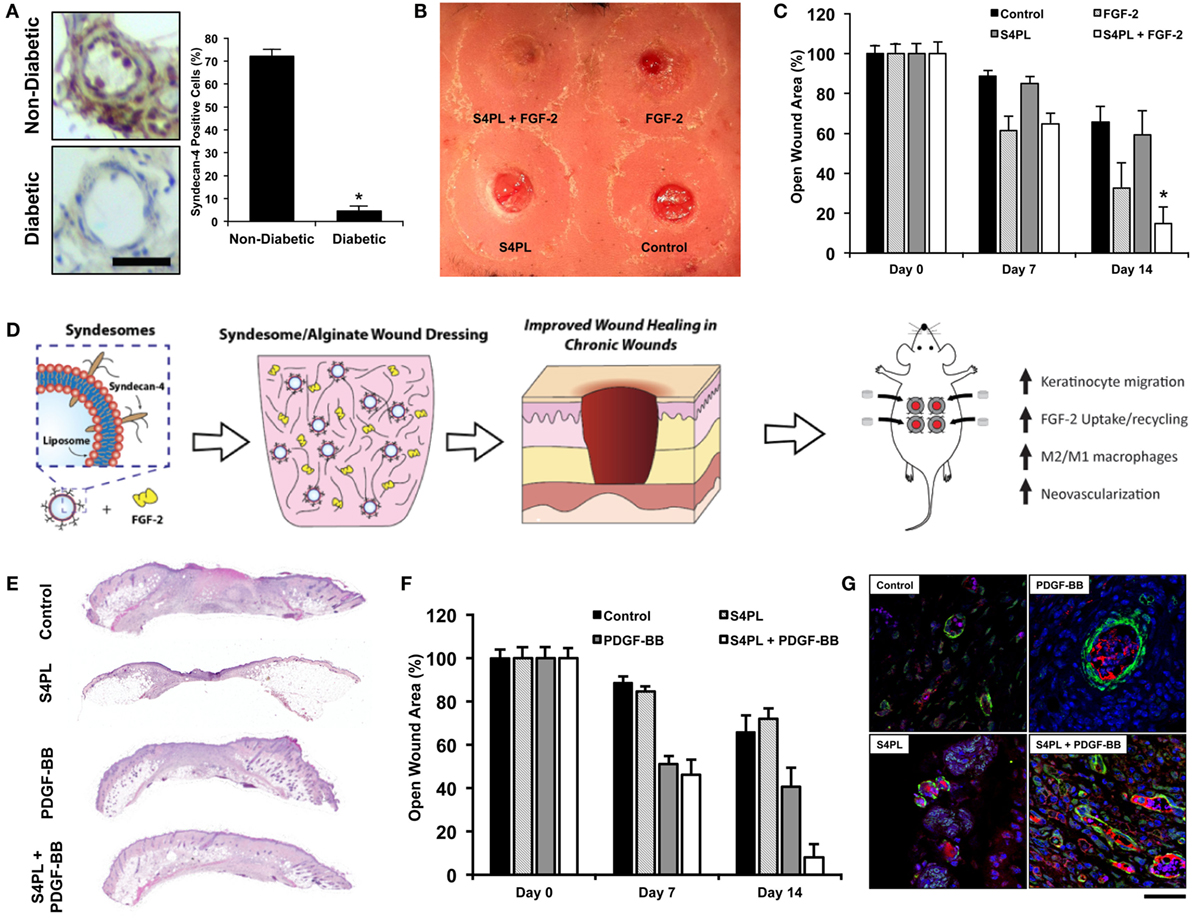
Figure 5. Delivery of co-receptors with growth factors in a lipid nanoliposome to enhance diabetic wound healing. (A) Protein expression of syndecan-4 in diabetic and non-diabetic human tissue. Bar = 25 μm. (B) Macroscopic image of the dorsal surface of the mouse with excisional wounds treated for 2 weeks with the treatments. (C) Quantification of the open wound area over the course of 2 weeks after surgery with the different treatments. (D) Schematic representation of the co-delivery of syndecan-4 in a nanoliposome with FGF-2 encapsulated in alginate wound dressings and the findings of the study. (A–D) are reproduced with permission from Das et al. (2016a). (E) Histological sections of wounds with various treatments stained with Hematoxylin and Eosin stain. (F) Quantification of the open wound area over the course of 2 weeks after surgery with the different treatments. (G) Immunofluorescent images of sections of the wound bed stained with alpha smooth muscle actin (green), PECAM (red), and DAPI (blue). (E–G) are reproduced with permission from Das et al. (2016c).
Polymer Nanoparticles
Wound dressings loaded with titanium dioxide NPs that are coated with chitosan and pectin are antimicrobial and have been shown to have great wound healing properties (Archana et al., 2013). The synergistic effects with the dressing such as antibacterial activity, high swelling properties, high moisture vapor transmission rate, hydrophilic nature, biocompatibility, wound appearance, and enhanced wound closure rate make titanium NPs a suitable candidate for wound healing applications. Growth factors are important in ensuring healthy wound healing. However, the half-life of the growth factors in the wound microenvironment is significantly reduced because of the presence of various proteolytic enzymes including MMPs (Murphy and Nagase, 2008). Encapsulation of growth factors in polymer NPs increased stability, preserved bioactivity, and promoted sustained release of the growth factors. Currently, PDGF-BB is the only growth factor that is FDA approved for diabetic foot ulcers, which makes PDGF-based therapies even more translational. Hyaluronan-based porous NPs enriched with PDGF-BB have been shown to be highly effective for the treatment of ulcers in a placebo-controlled study in rats (Zavan et al., 2009). Fibroblast growth factor-2 (FGF-2) has been successfully microencapsulated in gelatin preserving biological activity and thus allows for their use in tissue engineering, therapeutic angiogenesis, gene therapy, and drug delivery applications (Young et al., 2005). EGF is a potent mitogen for keratinocytes, which has been shown to be effective in healing gastric ulcers when delivered through a poly-l-lactic acid-based wound dressing (Han et al., 2001).
Nanoparticles in Clinical Usage
Silver Nanoparticles
Nanoparticle-based therapies in wound care are relatively new compared to conventional biomaterials that have been used for decades now. Silver has been used since ancient Roman times and now used in biomedical devices (de Alwis Weerasekera et al., 2015). Silver NP-based ointments/creams are perhaps the most widely used primarily because of the antimicrobial properties of nanocrystalline silver (Griffith et al., 2015). Silver NPs or nanocrystals in a topical gel have been used for moist wound care and promote cosmetic healing, have effective antibacterial properties, and play a role in cytokine modulation and suppress inflammation (Tian et al., 2007; Jain et al., 2009; Rigo et al., 2013). They are generally indicated for minor cuts, abrasions, lacerations, skin irritations, and first- or second-degree burns. Although the mechanisms underlying the antibacterial actions of silver are still not fully understood, several previous reports showed that the interaction between silver and the constituents of the bacterial membrane caused structural changes and damage to the membranes and intracellular metabolic activity, which might be the cause or consequence of cell death (McDonnell and Russell, 1999; Sondi and Salopek-Sondi, 2004; Pal et al., 2007; Eckhardt et al., 2013). However, prolonged exposure to colloidal silver can result in argyria where the skin attains blue gray color from accumulated silver (Rice, 2009). There are several variations of silver containing creams or gels or ointments that are available from different companies. Silver NP-based treatments are inexpensive, have low systemic toxicity, and are effective against viral and bacterial infections but have limited effects on enhancing the wound healing process in chronic wound environment (Gunasekaran et al., 2011).
Conclusion
Biomaterials have been used in wound healing since the rise of Egyptian civilization, but NPs have become tremendously important in engineering an effective treatment strategy, only in the last two decades. Biomaterials have been successfully used in manufacturing clinically approved products for aiding wound healing like films, foams, wafers, hydrogels, hemostatics, sealants, and composite dressings. However, there are no biomaterials currently approved that release bioactive components (like growth factors, cytokines, chemokines, plasmids, recombinant proteins, small molecules, cellular therapy, etc.) that directly influence the wound healing cascade. Here, we reviewed biomaterials used in the clinic and those under preclinical development. We are excited about the potential of the biomaterials undergoing development, specifically those that encapsulate bioactive compounds or cell therapies. NP therapies on the other hand have not been used widely in clinic barring silver NPs. However, there is a lot of compelling NP therapies that have shown great potential in animal models as we discussed in the paper.
With the advent of CRISPR-Cas9 technology, it would be interesting to see how scientists apply this remarkable gene editing technology to engineer the wound microenvironment (Jinek et al., 2012; Cho et al., 2013; Cong et al., 2013; Mali et al., 2013; Sander and Joung, 2014). There are many genes that are involved in the regulation of the wound healing process, and wound healing models have been tested only on a few mutant mouse models. CRISPR-Cas9 technology reduces the time to create a knockout mouse from several months to few weeks, thus enabling researchers to ask various questions. The overall goal would be to achieve fetal wound healing properties in adult wound healing with complete regeneration of hairs and glands, without delay and scarring.
Wound care is a significant economic and social burden on both the patient population and the health-care system at large. In this review, we have discussed the different biomaterial and NP-based wound therapies, which are either in current clinical usage or in preclinical development. Since there is significant variability of presentation of symptoms in the patients, effective wound care therapies need to have a multipronged approach to tackle the complex problems of pain, inflammation, infection caused by resistant bacteria, delayed healing, and associated costs to health systems and populations worldwide. The precipitous rise in multidrug-resistant bacteria is going to be the biggest challenge for wound care professionals all over the world in this decade. Emerging treatments using biomaterials or NPs to target multiple aspects have great promise for enhancing wound care and will add to the clinical armamentarium to address poorly healing wounds.
Author Contributions
SD and ABB wrote and edited the paper.
Conflict of Interest Statement
The authors declare that the research was conducted in the absence of any commercial or financial relationships that could be construed as a potential conflict of interest.
Funding
The authors gratefully acknowledge support through the Welch Foundation (F-1836) and the NIH Director’s New Innovator Grant (1DP2 OD008716-01) to AB.
References
Acosta, J. B., Savigne, W., Valdez, C., Franco, N., Alba, J. S., Del Rio, A., et al. (2006). Epidermal growth factor intralesional infiltrations can prevent amputation in patients with advanced diabetic foot wounds. Int. Wound J. 3, 232–239. doi:10.1111/j.1742-481X.2006.00237.x
Agha, R., Ogawa, R., Pietramaggiori, G., and Orgill, D. P. (2011). A review of the role of mechanical forces in cutaneous wound healing. J. Surg. Res. 171, 700–708. doi:10.1016/j.jss.2011.07.007
Aksoy, B., Aksoy, H. M., Civas, E., Ustun, H., and Atakan, N. (2009). A new experimental delayed wound healing model in rabbits. Eur. J. Dermatol. 19, 565–569. doi:10.1684/ejd.2009.0788
Alarcon, E. I., Udekwu, K. I., Noel, C. W., Gagnon, L. B., Taylor, P. K., Vulesevic, B., et al. (2015). Safety and efficacy of composite collagen-silver nanoparticle hydrogels as tissue engineering scaffolds. Nanoscale 7, 18789–18798. doi:10.1039/c5nr03826j
Alexiadou, K., and Doupis, J. (2012). Management of diabetic foot ulcers. Diabetes Ther. 3, 4. doi:10.1007/s13300-012-0004-9
Allison, K. R., Brynildsen, M. P., and Collins, J. J. (2011). Metabolite-enabled eradication of bacterial persisters by aminoglycosides. Nature 473, 216–220. doi:10.1038/nature10069
American Diabetes, A. (2013). Economic costs of diabetes in the U.S. in 2012. Diabetes Care 36, 1033–1046. doi:10.2337/dc12-2625
Andrews, K. L., Houdek, M. T., and Kiemele, L. J. (2015). Wound management of chronic diabetic foot ulcers: from the basics to regenerative medicine. Prosthet. Orthot. Int. 39, 29–39. doi:10.1177/0309364614534296
Ansell, D. M., Holden, K. A., and Hardman, M. J. (2012). Animal models of wound repair: are they cutting it? Exp. Dermatol. 21, 581–585. doi:10.1111/j.1600-0625.2012.01540.x
Archana, D., Dutta, J., and Dutta, P. K. (2013). Evaluation of chitosan nano dressing for wound healing: characterization, in vitro and in vivo studies. Int. J. Biol. Macromol. 57, 193–203. doi:10.1016/j.ijbiomac.2013.03.002
Armstrong, D. G., Kanda, V. A., Lavery, L. A., Marston, W., Mills, J. L. Sr., and Boulton, A. J. (2013). Mind the gap: disparity between research funding and costs of care for diabetic foot ulcers. Diabetes Care 36, 1815–1817. doi:10.2337/dc12-2285
Arora, S., Jain, J., Rajwade, J. M., and Paknikar, K. M. (2008). Cellular responses induced by silver nanoparticles: in vitro studies. Toxicol. Lett. 179, 93–100. doi:10.1016/j.toxlet.2008.04.009
Asharani, P. V., Lian Wu, Y., Gong, Z., and Valiyaveettil, S. (2008). Toxicity of silver nanoparticles in zebrafish models. Nanotechnology 19, 255102. doi:10.1088/0957-4484/19/25/255102
AshaRani, P. V., Low Kah Mun, G., Hande, M. P., and Valiyaveettil, S. (2009). Cytotoxicity and genotoxicity of silver nanoparticles in human cells. ACS Nano 3, 279–290. doi:10.1021/nn800596w
Assembly, W. H. (2015). Global Action Plan on Antimicrobial Resistance. Geneva: World Health Organization.
Ayello, E. A. (2005). What does the wound say? Why determining etiology is essential for appropriate wound care. Adv. Skin Wound Care 18, 98–109; quiz 110–111.
Banks, V., Bale, S., Harding, K., and Harding, E. F. (1997). Evaluation of a new polyurethane foam dressing. J. Wound Care 6, 266–269.
Banu, A., Noorul Hassan, M. M., Rajkumar, J., and Srinivasa, S. (2015). Spectrum of bacteria associated with diabetic foot ulcer and biofilm formation: a prospective study. Australas Med. J. 8, 280–285. doi:10.4066/AMJ.2015.2422
Barua, S., Chattopadhyay, P., Aidew, L., Buragohain, A. K., and Karak, N. (2015). Infection-resistant hyperbranched epoxy nanocomposite as a scaffold for skin tissue regeneration. Polym. Int. 64, 303–311. doi:10.1002/pi.4790
Baum, T. M., and Busuito, M. J. (1998). Use of a glycerin-based gel sheeting in scar management. Adv. Wound Care 11, 40–43.
Bell, B. G., Schellevis, F., Stobberingh, E., Goossens, H., and Pringle, M. (2014). A systematic review and meta-analysis of the effects of antibiotic consumption on antibiotic resistance. BMC Infect. Dis. 14:13. doi:10.1186/1471-2334-14-13
Birkedal-Hansen, H., Moore, W. G., Bodden, M. K., Windsor, L. J., Birkedal-Hansen, B., Decarlo, A., et al. (1993). Matrix metalloproteinases: a review. Crit. Rev. Oral Biol. Med. 4, 197–250.
Blair, J. M., Webber, M. A., Baylay, A. J., Ogbolu, D. O., and Piddock, L. J. (2015). Molecular mechanisms of antibiotic resistance. Nat. Rev. Microbiol. 13, 42–51. doi:10.1038/nrmicro3380
Blecher, K., Martinez, L. R., Tuckman-Vernon, C., Nacharaju, P., Schairer, D., Chouake, J., et al. (2012). Nitric oxide-releasing nanoparticles accelerate wound healing in NOD-SCID mice. Nanomedicine 8, 1364–1371. doi:10.1016/j.nano.2012.02.014
Boateng, J. S., Pawar, H. V., and Tetteh, J. (2013). Polyox and carrageenan based composite film dressing containing anti-microbial and anti-inflammatory drugs for effective wound healing. Int. J. Pharm. 441, 181–191. doi:10.1016/j.ijpharm.2012.11.045
Branski, L. K., Gauglitz, G. G., Herndon, D. N., and Jeschke, M. G. (2009). A review of gene and stem cell therapy in cutaneous wound healing. Burns 35, 171–180. doi:10.1016/j.burns.2008.03.009
Broughton, G. II, Janis, J. E., and Attinger, C. E. (2006). The basic science of wound healing. Plast. Reconstr. Surg. 117, 12S–34S. doi:10.1097/01.prs.0000225430.42531.c2
Bryan, L. E., and Van Den Elzen, H. M. (1977). Effects of membrane-energy mutations and cations on streptomycin and gentamicin accumulation by bacteria: a model for entry of streptomycin and gentamicin in susceptible and resistant bacteria. Antimicrob. Agents Chemother. 12, 163–177. doi:10.1128/AAC.12.2.163
Brynildsen, M. P., Winkler, J. A., Spina, C. S., Macdonald, I. C., and Collins, J. J. (2013). Potentiating antibacterial activity by predictably enhancing endogenous microbial ROS production. Nat. Biotechnol. 31, 160–165. doi:10.1038/nbt.2458
Buchberger, B., Follmann, M., Freyer, D., Huppertz, H., Ehm, A., and Wasem, J. (2011). The evidence for the use of growth factors and active skin substitutes for the treatment of non-infected diabetic foot ulcers (DFU): a health technology assessment (HTA). Exp. Clin. Endocrinol. Diabetes 119, 472–479. doi:10.1055/s-0031-1279713
Calderini, C., Cioni, F., Haddoub, S., Maccanelli, F., Magotti, M. G., and Tardio, S. (2014). Therapeutic approach to “diabetic foot” complications. Acta Biomed. 85, 189–204.
Castleberry, S. A., Almquist, B. D., Li, W., Reis, T., Chow, J., Mayner, S., et al. (2015). Self-assembled wound dressings silence MMP-9 and improve diabetic wound healing in vivo. Adv. Mater. 28, 1809–1817. doi:10.1002/adma.201503565
Chakraborty, S. P., Sahu, S. K., Mahapatra, S. K., Santra, S., Bal, M., Roy, S., et al. (2010). Nanoconjugated vancomycin: new opportunities for the development of anti-VRSA agents. Nanotechnology 21, 105103. doi:10.1088/0957-4484/21/10/105103
Chandika, P., KO, S. C., and Jung, W. K. (2015). Marine-derived biological macromolecule-based biomaterials for wound healing and skin tissue regeneration. Int. J. Biol. Macromol. 77, 24–35. doi:10.1016/j.ijbiomac.2015.02.050
Chien, S. (2007). Ischemic rabbit ear model created by minimally invasive surgery. Wound Repair Regen. 15, 928–935. doi:10.1111/j.1524-475X.2007.00285.x
Chigurupati, S., Mughal, M. R., Okun, E., Das, S., Kumar, A., McCaffery, M., et al. (2013). Effects of cerium oxide nanoparticles on the growth of keratinocytes, fibroblasts and vascular endothelial cells in cutaneous wound healing. Biomaterials 34, 2194–2201. doi:10.1016/j.biomaterials.2012.11.061
Cho, S. W., Kim, S., Kim, J. M., and Kim, J. S. (2013). Targeted genome engineering in human cells with the Cas9 RNA-guided endonuclease. Nat. Biotechnol. 31, 230–232. doi:10.1038/nbt.2507
Clifford, C. C. (2004). Treating traumatic bleeding in a combat setting. Mil. Med. 169, 4. doi:10.7205/milmed.169.12s.8
Cong, L., Ran, F. A., Cox, D., Lin, S., Barretto, R., Habib, N., et al. (2013). Multiplex genome engineering using CRISPR/Cas systems. Science 339, 819–823. doi:10.1126/science.1231143
Coutts, P. M., Ryan, J., and Sibbald, R. G. (2014). Case series of lower-extremity chronic wounds managed with an antibacterial foam dressing bound with gentian violet and methylene blue. Adv. Skin Wound Care 27, 9–13. doi:10.1097/01.ASW.0000443270.71030.71
Cozad, M. J., Bachman, S. L., and Grant, S. A. (2011). Assessment of decellularized porcine diaphragm conjugated with gold nanomaterials as a tissue scaffold for wound healing. J. Biomed. Mater. Res. A. 99, 426–434. doi:10.1002/jbm.a.33182
Cullen, B., Watt, P. W., Lundqvist, C., Silcock, D., Schmidt, R. J., Bogan, D., et al. (2002). The role of oxidised regenerated cellulose/collagen in chronic wound repair and its potential mechanism of action. Int. J. Biochem. Cell Biol. 34, 1544–1556. doi:10.1016/S1357-2725(02)00054-7
Dantes, R., Mu, Y., Belflower, R., Aragon, D., Dumyati, G., Harrison, L. H., et al. (2013). National burden of invasive methicillin-resistant Staphylococcus aureus infections, United States, 2011. JAMA Intern. Med. 173, 1970–1978. doi:10.1001/jamainternmed.2013.10423
Das, S., Majid, M., and Baker, A. B. (2016a). Syndecan-4 enhances PDGF-BB activity in diabetic wound healing. Acta Biomater. 42, 56–65. doi:10.1016/j.actbio.2016.07.001
Das, S., Monteforte, A. J., Singh, G., Majid, M., Sherman, M. B., Dunn, A. K., et al. (2016b). Syndecan-4 enhances therapeutic angiogenesis after hind limb ischemia in mice with type 2 diabetes. Adv. Healthc. Mater. 5, 1008–1013. doi:10.1002/adhm.201500993
Das, S., Singh, G., Majid, M., Sherman, M. B., Mukhopadhyay, S., Wright, C. S., et al. (2016c). Syndesome therapeutics for enhancing diabetic wound healing. Adv. Healthc. Mater. 5, 2248–2260. doi:10.1002/adhm.201600285
Das, S., Singh, G., and Baker, A. B. (2014). Overcoming disease-induced growth factor resistance in therapeutic angiogenesis using recombinant co-receptors delivered by a liposomal system. Biomaterials 35, 196–205. doi:10.1016/j.biomaterials.2013.09.105
de Alwis Weerasekera, H., Griffith, M., and Alarcon, E. I. (2015). “Biomedical uses of silver nanoparticles: from roman wine cups to biomedical devices,” in Silver Nanoparticle Applications: In the Fabrication and Design of Medical and Biosensing Devices, eds I. E. Alarcon, M. Griffith and I. K. Udekwu (Cham: Springer International Publishing), 93–125.
Deonarine, K., Panelli, M. C., Stashower, M. E., Jin, P., Smith, K., Slade, H. B., et al. (2007). Gene expression profiling of cutaneous wound healing. J. Transl. Med. 5, 11. doi:10.1186/1479-5876-5-11
DeRosa, F., Kibbe, M. R., Najjar, S. F., Citro, M. L., Keefer, L. K., and Hrabie, J. A. (2007). Nitric oxide-releasing fabrics and other acrylonitrile-based diazeniumdiolates. J. Am. Chem. Soc. 129, 3786–3787. doi:10.1021/ja0686864
Dhanalakshmi, V., Nimal, T. R., Sabitha, M., Biswas, R., and Jayakumar, R. (2016). Skin and muscle permeating antibacterial nanoparticles for treating Staphylococcus aureus infected wounds. J. Biomed. Mater. Res. Part B Appl. Biomater. 104, 797–807. doi:10.1002/jbm.b.33635
DiPietro, L. A. (1995). Wound healing: the role of the macrophage and other immune cells. Shock 4, 233–240. doi:10.1097/00024382-199510000-00001
Dorsett-Martin, W. A., and Wysocki, A. B. (2008). “Rat models of skin wound healing,” in Sourcebook of Models for Biomedical Research, ed. P. M. Conn (Totowa, NJ: Humana Press), 631–638.
Dunn, L., Prosser, H. C., Tan, J. T., Vanags, L. Z., NG, M. K., and Bursill, C. A. (2013). Murine model of wound healing. J. Vis. Exp. 75, e50265. doi:10.3791/50265
Eckhardt, S., Brunetto, P. S., Gagnon, J., Priebe, M., Giese, B., and Fromm, K. M. (2013). Nanobio silver: its interactions with peptides and bacteria, and its uses in medicine. Chem. Rev. 113, 4708–4754. doi:10.1021/cr300288v
Elsner, J. J., Shefy-Peleg, A., and Zilberman, M. (2010). Novel biodegradable composite wound dressings with controlled release of antibiotics: microstructure, mechanical and physical properties. J. Biomed. Mater. Res. Part B Appl. Biomater. 93, 425–435. doi:10.1002/jbm.b.31599
Eming, S. A., Martin, P., and Tomic-Canic, M. (2014). Wound repair and regeneration: mechanisms, signaling, and translation. Sci. Transl. Med. 6, 265sr6. doi:10.1126/scitranslmed.3009337
Evers, L. H., Bhavsar, D., and Mailander, P. (2010). The biology of burn injury. Exp. Dermatol. 19, 777–783. doi:10.1111/j.1600-0625.2010.01105.x
Fang, R. C., and Galiano, R. D. (2008). A review of becaplermin gel in the treatment of diabetic neuropathic foot ulcers. Biologics 2, 1–12. doi:10.2147/BTT.S1338
Fernandez-Montequin, J. I., Betancourt, B. Y., Leyva-Gonzalez, G., Mola, E. L., Galan-Naranjo, K., Ramirez-Navas, M., et al. (2009). Intralesional administration of epidermal growth factor-based formulation (Heberprot-P) in chronic diabetic foot ulcer: treatment up to complete wound closure. Int. Wound J. 6, 67–72. doi:10.1111/j.1742-481X.2008.00561.x
Franz, S., Rammelt, S., Scharnweber, D., and Simon, J. C. (2011). Immune responses to implants – a review of the implications for the design of immunomodulatory biomaterials. Biomaterials 32, 6692–6709. doi:10.1016/j.biomaterials.2011.05.078
Futrega, K., King, M., Lott, W. B., and Doran, M. R. (2014). Treating the whole not the hole: necessary coupling of technologies for diabetic foot ulcer treatment. Trends Mol. Med. 20, 137–142. doi:10.1016/j.molmed.2013.12.004
Garay-Jimenez, J. C., Gergeres, D., Young, A., Lim, D. V., and Turos, E. (2009). Physical properties and biological activity of poly(butyl acrylate-styrene) nanoparticle emulsions prepared with conventional and polymerizable surfactants. Nanomedicine 5, 443–451. doi:10.1016/j.nano.2009.01.015
Gokce, E. H., Korkmaz, E., Dellera, E., Sandri, G., Bonferoni, M. C., and Ozer, O. (2012). Resveratrol-loaded solid lipid nanoparticles versus nanostructured lipid carriers: evaluation of antioxidant potential for dermal applications. Int. J. Nanomedicine 7, 1841–1850. doi:10.2147/IJN.S29710
Griffin, D. R., Weaver, W. M., Scumpia, P. O., DI Carlo, D., and Segura, T. (2015). Accelerated wound healing by injectable microporous gel scaffolds assembled from annealed building blocks. Nat. Mater. 14, 737–744. doi:10.1038/nmat4294
Griffith, M., Udekwu, K. I., Gkotzis, S., Mah, T.-F., and Alarcon, E. I. (2015). “Anti-microbiological and anti-infective activities of silver,” in Silver Nanoparticle Applications: In the Fabrication and Design of Medical and Biosensing Devices, eds I. E. Alarcon, M. Griffith and I. K. Udekwu (Cham: Springer International Publishing), 127–146.
Gu, Q., Wang, S., Wang, Q., and Wei, X. (2014). [Application status and research progress of alginate dressings]. Zhongguo Xiu Fu Chong Jian Wai Ke Za Zhi 28, 255–258.
Gunasekaran, T., Nigusse, T., and Dhanaraju, M. D. (2011). Silver nanoparticles as real topical bullets for wound healing. J. Am. Coll. Clin. Wound Spec. 3, 82–96. doi:10.1016/j.jcws.2012.05.001
Gurtner, G. C., Werner, S., Barrandon, Y., and Longaker, M. T. (2008). Wound repair and regeneration. Nature 453, 314–321. doi:10.1038/nature07039
Gusarov, I., Shatalin, K., Starodubtseva, M., and Nudler, E. (2009). Endogenous nitric oxide protects bacteria against a wide spectrum of antibiotics. Science 325, 1380–1384. doi:10.1126/science.1175439
Han, G., Nguyen, L. N., Macherla, C., Chi, Y., Friedman, J. M., Nosanchuk, J. D., et al. (2012). Nitric oxide-releasing nanoparticles accelerate wound healing by promoting fibroblast migration and collagen deposition. Am. J. Pathol. 180, 1465–1473. doi:10.1016/j.ajpath.2011.12.013
Han, K., Lee, K. D., Gao, Z. G., and Park, J. S. (2001). Preparation and evaluation of poly(l-lactic acid) microspheres containing rhEGF for chronic gastric ulcer healing. J. Control Release 75, 259–269. doi:10.1016/S0168-3659(01)00400-X
Hantash, B. M., Zhao, L., Knowles, J. A., and Lorenz, H. P. (2008). Adult and fetal wound healing. Front. Biosci. 13:51–61. doi:10.2741/2559
Hasatsri, S., Angspatt, A., and Aramwit, P. (2015). Randomized clinical trial of the innovative bilayered wound dressing made of silk and gelatin: safety and efficacy tests using a split-thickness skin graft model. Evid. Based Complement. Altern. Med. 2015, 206871. doi:10.1155/2015/206871
Hetrick, E. M., Shin, J. H., Paul, H. S., and Schoenfisch, M. H. (2009). Anti-biofilm efficacy of nitric oxide-releasing silica nanoparticles. Biomaterials 30, 2782–2789. doi:10.1016/j.biomaterials.2009.01.052
Heublein, H., Bader, A., and Giri, S. (2015). Preclinical and clinical evidence for stem cell therapies as treatment for diabetic wounds. Drug Discov. Today 20, 703–717. doi:10.1016/j.drudis.2015.01.005
Hoggarth, A., Waring, M., Alexander, J., Greenwood, A., and Callaghan, T. (2005). A controlled, three-part trial to investigate the barrier function and skin hydration properties of six skin protectants. Ostomy Wound Manage. 51, 30–42.
Holmes, C., Wrobel, J. S., Maceachern, M. P., and Boles, B. R. (2013). Collagen-based wound dressings for the treatment of diabetes-related foot ulcers: a systematic review. Diabetes Metab. Syndr. Obes. 6, 17–29. doi:10.2147/DMSO.S36024
Hubbell, J. A. (1996). Hydrogel systems for barriers and local drug delivery in the control of wound healing. J. Control. Release 39, 305–313. doi:10.1016/0168-3659(95)00162-X
Hutchinson, J. J., and McGuckin, M. (1990). Occlusive dressings: a microbiologic and clinical review. Am. J. Infect. Control 18, 257–268. doi:10.1016/0196-6553(90)90167-Q
Jacob, D. S., Bitton, L., Grinblat, J., Felner, I., Koltypin, Y., and Gedanken, A. (2006). Are ionic liquids really a boon for the synthesis of inorganic materials? A general method for the fabrication of nanosized metal fluorides. Chem. Mater. 18, 3162–3168. doi:10.1021/cm060782g
Jain, J., Arora, S., Rajwade, J. M., Omray, P., Khandelwal, S., and Paknikar, K. M. (2009). Silver nanoparticles in therapeutics: development of an antimicrobial gel formulation for topical use. Mol. Pharm. 6, 1388–1401. doi:10.1021/mp900056g
Jang, E., Albadawi, H., Watkins, M. T., Edelman, E. R., and Baker, A. B. (2012). Syndecan-4 proteoliposomes enhance fibroblast growth factor-2 (FGF-2)-induced proliferation, migration, and neovascularization of ischemic muscle. Proc. Natl. Acad. Sci. U.S.A. 109, 1679–1684. doi:10.1073/pnas.1117885109
Jeanes, A., and Bitmead, J. (2015). Reducing bloodstream infection with a chlorhexidine gel IV dressing. Br. J. Nurs. 24, S14–S19. doi:10.12968/bjon.2015.24.Sup19.S14
Jinek, M., Chylinski, K., Fonfara, I., Hauer, M., Doudna, J. A., and Charpentier, E. (2012). A programmable dual-RNA-guided DNA endonuclease in adaptive bacterial immunity. Science 337, 816–821. doi:10.1126/science.1225829
Kang, M., Choi, S., and Cho Lee, A. R. (2013). Effect of freeze dried bovine amniotic membrane extract on full thickness wound healing. Arch. Pharm. Res. 36, 472–478. doi:10.1007/s12272-013-0079-5
Kannon, G. A., and Garrett, A. B. (1995). Moist wound healing with occlusive dressings. Dermatol. Surg. 21, 583–590. doi:10.1016/1076-0512(94)00114-6
Kell, A. J., Stewart, G., Ryan, S., Peytavi, R., Boissinot, M., Huletsky, A., et al. (2008). Vancomycin-modified nanoparticles for efficient targeting and preconcentration of Gram-positive and Gram-negative bacteria. ACS Nano 2, 1777–1788. doi:10.1021/nn700183g
Kemp, M. G. (1994). Protecting the skin from moisture and associated irritants. J. Gerontol. Nurs. 20, 8–14. doi:10.3928/0098-9134-19940901-04
Kim, D. J., Mustoe, T., and Clark, R. A. (2015). Cutaneous wound healing in aging small mammals: a systematic review. Wound Repair Regen. 23, 318–339. doi:10.1111/wrr.12290
Kim, I. S., Lee, S. K., Park, Y. M., Lee, Y. B., Shin, S. C., Lee, K. C., et al. (2005). Physicochemical characterization of poly(l-lactic acid) and poly(d,l-lactide-co-glycolide) nanoparticles with polyethylenimine as gene delivery carrier. Int. J. Pharm. 298, 255–262. doi:10.1016/j.ijpharm.2005.04.017
Kirsner, R. S. (2016). The wound healing society chronic wound ulcer healing guidelines update of the 2006 guidelines-blending old with new. Wound Repair Regen. 24, 110–111. doi:10.1111/wrr.12393
Kohanski, M. A., Dwyer, D. J., Hayete, B., Lawrence, C. A., and Collins, J. J. (2007). A common mechanism of cellular death induced by bactericidal antibiotics. Cell 130, 797–810. doi:10.1016/j.cell.2007.06.049
Kozen, B. G., Kircher, S. J., Henao, J., Godinez, F. S., and Johnson, A. S. (2008). An alternative hemostatic dressing: comparison of Celox, HemCon, and QuikClot. Acad. Emerg. Med. 15, 74–81. doi:10.1111/j.1553-2712.2007.00009.x
Kuchler, S., Herrmann, W., Panek-Minkin, G., Blaschke, T., Zoschke, C., Kramer, K. D., et al. (2010a). SLN for topical application in skin diseases – characterization of drug-carrier and carrier-target interactions. Int. J. Pharm. 390, 225–233. doi:10.1016/j.ijpharm.2010.02.004
Kuchler, S., Wolf, N. B., Heilmann, S., Weindl, G., Helfmann, J., Yahya, M. M., et al. (2010b). 3D-wound healing model: influence of morphine and solid lipid nanoparticles. J. Biotechnol. 148, 24–30. doi:10.1016/j.jbiotec.2010.01.001
Kuchler, S., Radowski, M. R., Blaschke, T., Dathe, M., Plendl, J., Haag, R., et al. (2009). Nanoparticles for skin penetration enhancement – a comparison of a dendritic core-multishell-nanotransporter and solid lipid nanoparticles. Eur. J. Pharm. Biopharm. 71, 243–250. doi:10.1016/j.ejpb.2008.08.019
Larsson, J., Agardh, C. D., Apelqvist, J., and Stenstrom, A. (1998). Long-term prognosis after healed amputation in patients with diabetes. Clin. Orthop. Relat. Res. 350, 149–158.
Lellouche, J., Kahana, E., Elias, S., Gedanken, A., and Banin, E. (2009). Antibiofilm activity of nanosized magnesium fluoride. Biomaterials 30, 5969–5978. doi:10.1016/j.biomaterials.2009.07.037
Leu, J. G., Chen, S. A., Chen, H. M., Wu, W. M., Hung, C. F., Yao, Y. D., et al. (2012). The effects of gold nanoparticles in wound healing with antioxidant epigallocatechin gallate and alpha-lipoic acid. Nanomedicine 8, 767–775. doi:10.1016/j.nano.2011.08.013
Lew, D. H., Liu, P. H., and Orgill, D. P. (2007). Optimization of UV cross-linking density for durable and nontoxic collagen GAG dermal substitute. J. Biomed. Mater. Res. Part B Appl. Biomater. 82, 51–56. doi:10.1002/jbm.b.30704
Li, N., Luo, H. C., Yang, C., Deng, J. J., Ren, M., Xie, X. Y., et al. (2014). Cationic star-shaped polymer as an siRNA carrier for reducing MMP-9 expression in skin fibroblast cells and promoting wound healing in diabetic rats. Int. J. Nanomedicine 9, 3377–3387. doi:10.2147/IJN.S66368
Li, S., and Li, Q. (2014). A promising approach to iPSC-based cell therapy for diabetic wound treatment: direct lineage reprogramming. Mol. Cell. Endocrinol. 393, 8–15. doi:10.1016/j.mce.2014.05.025
Lin, Y. H., Lin, J. H., Li, T. S., Wang, S. H., Yao, C. H., Chung, W. Y., et al. (2015). Dressing with epigallocatechin gallate-nanoparticles for wound regeneration. Wound Repair Regen. 24, 287–301. doi:10.1111/wrr.12372
Liu, X., Lee, P. Y., HO, C. M., Lui, V. C., Chen, Y., Che, C. M., et al. (2010). Silver nanoparticles mediate differential responses in keratinocytes and fibroblasts during skin wound healing. ChemMedChem 5, 468–475. doi:10.1002/cmdc.200900502
Liu, Y., Chen, W., and Kim, H.-I. (2012). Antibacterial activity of pH-sensitive genipin cross-linked chitosan/poly(ethylene glycol)/silver nanocomposites. Polym. Adv. Technol. 23, 8–14. doi:10.1002/pat.1818
Madlener, M., Parks, W. C., and Werner, S. (1998). Matrix metalloproteinases (MMPs) and their physiological inhibitors (TIMPs) are differentially expressed during excisional skin wound repair. Exp. Cell Res. 242, 201–210. doi:10.1006/excr.1998.4049
Makarov, V. V., Love, A. J., Sinitsyna, O. V., Makarova, S. S., Yaminsky, I. V., Taliansky, M. E., et al. (2014). “Green” nanotechnologies: synthesis of metal nanoparticles using plants. Acta Nat. 6, 35–44.
Mali, P., Yang, L., Esvelt, K. M., Aach, J., Guell, M., Dicarlo, J. E., et al. (2013). RNA-guided human genome engineering via Cas9. Science 339, 823–826. doi:10.1126/science.1232033
Mao, C., Chen, X., Miao, G., and Lin, C. (2015). Angiogenesis stimulated by novel nanoscale bioactive glasses. Biomed. Mater. 10, 025005. doi:10.1088/1748-6041/10/2/025005
Marti-Carvajal, A. J., Gluud, C., Nicola, S., Simancas-Racines, D., Reveiz, L., Oliva, P., et al. (2015). Growth factors for treating diabetic foot ulcers. Cochrane Database Syst. Rev. 10, CD008548. doi:10.1002/14651858.CD008548.pub2
Martin, J. R., Gupta, M. K., Page, J. M., Yu, F., Davidson, J. M., Guelcher, S. A., et al. (2014). A porous tissue engineering scaffold selectively degraded by cell-generated reactive oxygen species. Biomaterials 35, 3766–3776. doi:10.1016/j.biomaterials.2014.01.026
Martin, P. (1997). Wound healing – aiming for perfect skin regeneration. Science 276, 75–81. doi:10.1126/science.276.5309.75
Martinez, J. L., and Rojo, F. (2011). Metabolic regulation of antibiotic resistance. FEMS Microbiol. Rev. 35, 768–789. doi:10.1111/j.1574-6976.2011.00282.x
Martino, M. M., Briquez, P. S., Guc, E., Tortelli, F., Kilarski, W. W., Metzger, S., et al. (2014). Growth factors engineered for super-affinity to the extracellular matrix enhance tissue healing. Science 343, 885–888. doi:10.1126/science.1247663
Mashaghi, S., Jadidi, T., Koenderink, G., and Mashaghi, A. (2013). Lipid nanotechnology. Int. J. Mol. Sci. 14, 4242–4282. doi:10.3390/ijms14024242
Mayet, N., Choonara, Y. E., Kumar, P., Tomar, L. K., Tyagi, C., Du Toit, L. C., et al. (2014). A comprehensive review of advanced biopolymeric wound healing systems. J. Pharm. Sci. 103, 2211–2230. doi:10.1002/jps.24068
McDonnell, G., and Russell, A. D. (1999). Antiseptics and disinfectants: activity, action, and resistance. Clin. Microbiol. Rev. 12, 147–179.
McLaughlin, S., Podrebarac, J., Ruel, M., Suuronen, E. J., McNeill, B., and Alarcon, E. I. (2016). Nano-engineered biomaterials for tissue regeneration: what has been achieved so far? Front. Mater. 3:27. doi:10.3389/fmats.2016.00027
Monteforte, A. J., Lam, B., Das, S., Mukhopadhyay, S., Wright, C. S., Martin, P. E., et al. (2016). Glypican-1 nanoliposomes for potentiating growth factor activity in therapeutic angiogenesis. Biomaterials 94, 45–56. doi:10.1016/j.biomaterials.2016.03.048
Mostow, E. N., Haraway, G. D., Dalsing, M., Hodde, J. P., King, D., and Group, O. V. U. S. (2005). Effectiveness of an extracellular matrix graft (OASIS Wound Matrix) in the treatment of chronic leg ulcers: a randomized clinical trial. J. Vasc. Surg. 41, 837–843. doi:10.1016/j.jvs.2005.01.042
Murphy, G., and Nagase, H. (2008). Progress in matrix metalloproteinase research. Mol. Aspects Med. 29, 290–308. doi:10.1016/j.mam.2008.05.002
Naraginti, S., Kumari, P. L., Das, R. K., Sivakumar, A., Patil, S. H., and Andhalkar, V. V. (2016). Amelioration of excision wounds by topical application of green synthesized, formulated silver and gold nanoparticles in albino Wistar rats. Mater. Sci. Eng. C Mater. Biol. Appl. 62, 293–300. doi:10.1016/j.msec.2016.01.069
Nelson, C. E., Gupta, M. K., Adolph, E. J., Guelcher, S. A., and Duvall, C. L. (2013). siRNA delivery from an injectable scaffold for wound therapy. Adv. Wound Care (New Rochelle) 2, 93–99. doi:10.1089/wound.2011.0327
Ozturk, S., and Karagoz, H. (2015). Experimental stem cell therapies on burn wound: do source, dose, timing and method matter? Burns 41, 1133–1139. doi:10.1016/j.burns.2015.01.005
Pal, S., Tak, Y. K., and Song, J. M. (2007). Does the antibacterial activity of silver nanoparticles depend on the shape of the nanoparticle? A study of the Gram-negative bacterium Escherichia coli. Appl. Environ. Microbiol. 73, 1712–1720. doi:10.1128/AEM.02218-06
Papanas, N., and Maltezos, E. (2008). Becaplermin gel in the treatment of diabetic neuropathic foot ulcers. Clin. Interv. Aging 3, 233–240. doi:10.2147/CIA.S1106
Pashuck, E. T., and Stevens, M. M. (2012). Designing regenerative biomaterial therapies for the clinic. Sci. Transl. Med. 4, 160sr4. doi:10.1126/scitranslmed.3002717
Pawar, H. V., Boateng, J. S., Ayensu, I., and Tetteh, J. (2014). Multifunctional medicated lyophilised wafer dressing for effective chronic wound healing. J. Pharm. Sci. 103, 1720–1733. doi:10.1002/jps.23968
Pawar, H. V., Tetteh, J., and Boateng, J. S. (2013). Preparation, optimisation and characterisation of novel wound healing film dressings loaded with streptomycin and diclofenac. Colloids Surf. B Biointerfaces 102, 102–110. doi:10.1016/j.colsurfb.2012.08.014
Pelizzo, G., Avanzini, M. A., Icaro Cornaglia, A., Osti, M., Romano, P., Avolio, L., et al. (2015). Mesenchymal stromal cells for cutaneous wound healing in a rabbit model: pre-clinical study applicable in the pediatric surgical setting. J. Transl. Med. 13, 219. doi:10.1186/s12967-015-0580-3
Peng, B., Su, Y. B., Li, H., Han, Y., Guo, C., Tian, Y. M., et al. (2015). Exogenous alanine and/or glucose plus kanamycin kills antibiotic-resistant bacteria. Cell Metab. 21, 249–261. doi:10.1016/j.cmet.2015.01.008
Percival, T. J., and Rasmussen, T. E. (2012). Reperfusion strategies in the management of extremity vascular injury with ischaemia. Br. J. Surg. 99(Suppl. 1), 66–74. doi:10.1002/bjs.7790
Pereira, G. G., Detoni, C. B., Balducci, A. G., Rondelli, V., Colombo, P., Guterres, S. S., et al. (2016). Hyaluronate nanoparticles included in polymer films for the prolonged release of vitamin E for the management of skin wounds. Eur. J. Pharm. Sci. 83, 203–211. doi:10.1016/j.ejps.2016.01.002
Petrie, N. C., Vranckx, J. J., Hoeller, D., Yao, F., and Eriksson, E. (2005). Gene delivery of PDGF for wound healing therapy. J. Tissue Viability 15, 16–21. doi:10.1016/S0965-206X(05)54002-6
Pillay, V., Kumar, P., and Choonara, E. Y. (2015). “Integrated biomaterial composites for accelerated wound healing,” in Biomaterials in Regenerative Medicine and the Immune System, ed. L. Santambrogio (Cham: Springer International Publishing), 209–223.
Prestwich, G. D., Bhatia, S., Breuer, C. K., Dahl, S. L., Mason, C., McFarland, R., et al. (2012). What is the greatest regulatory challenge in the translation of biomaterials to the clinic? Sci. Transl. Med. 4, 160cm14. doi:10.1126/scitranslmed.3004915
Rakhmetova, A. A., Alekseeva, T. P., Bogoslovskaya, O. A., Leipunskii, I. O., Ol’khovskaya, I. P., Zhigach, A. N., et al. (2010). Wound-healing properties of copper nanoparticles as a function of physicochemical parameters. Nanotechnol. Russia 5, 271–276. doi:10.1134/S199507801003016X
Ramasastry, S. S. (2005). Acute wounds. Clin. Plast. Surg. 32, 195–208. doi:10.1016/j.cps.2004.12.001
Randeria, P. S., Seeger, M. A., Wang, X. Q., Wilson, H., Shipp, D., Mirkin, C. A., et al. (2015). siRNA-based spherical nucleic acids reverse impaired wound healing in diabetic mice by ganglioside GM3 synthase knockdown. Proc. Natl. Acad. Sci. U.S.A. 112, 5573–5578. doi:10.1073/pnas.1505951112
Rani, S., and Ritter, T. (2015). The exosome – a naturally secreted nanoparticle and its application to wound healing. Adv. Mater. 28, 5542–5552. doi:10.1002/adma.201504009
Ratner, B. D. (1993). New ideas in biomaterials science – a path to engineered biomaterials. J. Biomed. Mater. Res. 27, 837–850. doi:10.1002/jbm.820270702
Ratner, B. D. (2007). A paradigm shift: biomaterials that heal. Polym. Int. 56, 1183–1185. doi:10.1002/pi.2319
Rice, L. B. (2009). The clinical consequences of antimicrobial resistance. Curr. Opin. Microbiol. 12, 476–481. doi:10.1016/j.mib.2009.08.001
Richard, J. L., Parer-Richard, C., Daures, J. P., Clouet, S., Vannereau, D., Bringer, J., et al. (1995). Effect of topical basic fibroblast growth factor on the healing of chronic diabetic neuropathic ulcer of the foot. A pilot, randomized, double-blind, placebo-controlled study. Diabetes Care 18, 64–69. doi:10.2337/diacare.18.1.64
Rigo, C., Ferroni, L., Tocco, I., Roman, M., Munivrana, I., Gardin, C., et al. (2013). Active silver nanoparticles for wound healing. Int. J. Mol. Sci. 14, 4817–4840. doi:10.3390/ijms14034817
Rohani, M. G., and Parks, W. C. (2015). Matrix remodeling by MMPs during wound repair. Matrix Biol. 4, 113–121. doi:10.1016/j.matbio.2015.03.002
Ruszczak, Z. (2003). Effect of collagen matrices on dermal wound healing. Adv. Drug Deliv. Rev. 55, 1595–1611. doi:10.1016/j.addr.2003.08.003
Safinya, C. R., and Ewert, K. K. (2012). Materials chemistry: liposomes derived from molecular vases. Nature 489, 372–374. doi:10.1038/489372b
Sahoo, S. K., Parveen, S., and Panda, J. J. (2007). The present and future of nanotechnology in human health care. Nanomedicine 3, 20–31. doi:10.1016/j.nano.2006.11.008
Salamone, J. C., Salamone, A. B., Swindle-Reilly, K., Leung, K. X., and McMahon, R. E. (2016). Grand challenge in biomaterials-wound healing. Regen. Biomater. 3, 127–128. doi:10.1093/rb/rbw015
Samberg, M. E., Loboa, E. G., Oldenburg, S. J., and Monteiro-Riviere, N. A. (2012). Silver nanoparticles do not influence stem cell differentiation but cause minimal toxicity. Nanomedicine (Lond.) 7, 1197–1209. doi:10.2217/nnm.12.18
Samberg, M. E., Oldenburg, S. J., and Monteiro-Riviere, N. A. (2010). Evaluation of silver nanoparticle toxicity in skin in vivo and keratinocytes in vitro. Environ. Health Perspect. 118, 407–413. doi:10.1289/ehp.0901398
Sander, J. D., and Joung, J. K. (2014). Crispr-Cas systems for editing, regulating and targeting genomes. Nat. Biotechnol. 32, 347–355. doi:10.1038/nbt.2842
Sandri, G., Bonferoni, M. C., D’Autilia, F., Rossi, S., Ferrari, F., Grisoli, P., et al. (2013). Wound dressings based on silver sulfadiazine solid lipid nanoparticles for tissue repairing. Eur. J. Pharm. Biopharm. 84, 84–90. doi:10.1016/j.ejpb.2012.11.022
Santema, T. B., Poyck, P. P., and Ubbink, D. T. (2016). Skin grafting and tissue replacement for treating foot ulcers in people with diabetes. Cochrane Database Syst. Rev. 2, CD011255. doi:10.1002/14651858.CD011255.pub2
Seetharaman, S., Natesan, S., Stowers, R. S., Mullens, C., Baer, D. G., Suggs, L. J., et al. (2011). A PEGylated fibrin-based wound dressing with antimicrobial and angiogenic activity. Acta Biomater. 7, 2787–2796. doi:10.1016/j.actbio.2011.04.003
Sen, C. K., Gordillo, G. M., Roy, S., Kirsner, R., Lambert, L., Hunt, T. K., et al. (2009). Human skin wounds: a major and snowballing threat to public health and the economy. Wound Repair Regen. 17, 763–771. doi:10.1111/j.1524-475X.2009.00543.x
Shah, J. B. (2011). The history of wound care. J. Am. Col. Certif. Wound Spec. 3, 65–66. doi:10.1016/j.jcws.2012.04.002
Shen, Y. I., Song, H. H., Papa, A. E., Burke, J. A., Volk, S. W., and Gerecht, S. (2015). Acellular hydrogels for regenerative burn wound healing: translation from a porcine model. J. Invest. Dermatol. 135, 2519–2529. doi:10.1038/jid.2015.182
Singh, N., Armstrong, D. G., and Lipsky, B. A. (2005). Preventing foot ulcers in patients with diabetes. JAMA 293, 217–228. doi:10.1001/jama.293.2.217
Sinha, M., Banik, R. M., Haldar, C., and Maiti, P. (2012). Development of ciprofloxacin hydrochloride loaded poly(ethylene glycol)/chitosan scaffold as wound dressing. J. Porous Mater. 20, 799–807. doi:10.1007/s10934-012-9655-1
Skardal, A., Murphy, S. V., Crowell, K., Mack, D., Atala, A., and Soker, S. (2016). A tunable hydrogel system for long-term release of cell-secreted cytokines and bioprinted in situ wound cell delivery. J. Biomed. Mater. Res. Part B Appl. Biomater. doi:10.1002/jbm.b.33736
Sondi, I., and Salopek-Sondi, B. (2004). Silver nanoparticles as antimicrobial agent: a case study on E. coli as a model for Gram-negative bacteria. J. Colloid Interface Sci. 275, 177–182. doi:10.1016/j.jcis.2004.02.012
Song, A., Rane, A. A., and Christman, K. L. (2012). Antibacterial and cell-adhesive polypeptide and poly(ethylene glycol) hydrogel as a potential scaffold for wound healing. Acta Biomater. 8, 41–50. doi:10.1016/j.actbio.2011.10.004
Stashak, T. S., Farstvedt, E., and Othic, A. (2004). Update on wound dressings: indications and best use. Clin. Tech. Equine Pract. 3, 148–163. doi:10.1053/j.ctep.2004.08.006
Steed, D. L. (2006). Clinical evaluation of recombinant human platelet-derived growth factor for the treatment of lower extremity ulcers. Plast. Reconstr. Surg. 117, 143S–149S. doi:10.1097/01.prs.0000222526.21512.4c
Steed, D. L., Goslen, J. B., Holloway, G. A., Malone, J. M., Bunt, T. J., and Webster, M. W. (1992). Randomized prospective double-blind trial in healing chronic diabetic foot ulcers. CT-102 activated platelet supernatant, topical versus placebo. Diabetes Care 15, 1598–1604. doi:10.2337/diacare.15.11.1598
Steffensen, B., Hakkinen, L., and Larjava, H. (2001). Proteolytic events of wound-healing – coordinated interactions among matrix metalloproteinases (MMPs), integrins, and extracellular matrix molecules. Crit. Rev. Oral Biol. Med. 12, 373–398. doi:10.1177/10454411010120050201
Su, W. H., Cheng, M. H., Lee, W. L., Tsou, T. S., Chang, W. H., Chen, C. S., et al. (2010). Nonsteroidal anti-inflammatory drugs for wounds: pain relief or excessive scar formation? Mediators Inflamm. 2010, 413238. doi:10.1155/2010/413238
Sullivan, T. P., Eaglstein, W. H., Davis, S. C., and Mertz, P. (2001). The pig as a model for human wound healing. Wound Repair Regen. 9, 66–76. doi:10.1046/j.1524-475x.2001.00066.x
Sun, G., Zhang, X., Shen, Y. I., Sebastian, R., Dickinson, L. E., Fox-Talbot, K., et al. (2011). Dextran hydrogel scaffolds enhance angiogenic responses and promote complete skin regeneration during burn wound healing. Proc. Natl. Acad. Sci. U.S.A. 108, 20976–20981. doi:10.1073/pnas.1115973108
Tian, J., Wong, K. K., Ho, C. M., Lok, C. N., Yu, W. Y., Che, C. M., et al. (2007). Topical delivery of silver nanoparticles promotes wound healing. ChemMedChem 2, 129–136. doi:10.1002/cmdc.200600171
Tokatlian, T., Cam, C., and Segura, T. (2015). Porous hyaluronic acid hydrogels for localized nonviral DNA delivery in a diabetic wound healing model. Adv. Healthc. Mater. 4, 1084–1091. doi:10.1002/adhm.201400783
Tu, C., Das, S., Baker, A. B., Zoldan, J., and Suggs, L. J. (2015). Nanoscale strategies: treatment for peripheral vascular disease and critical limb ischemia. ACS Nano 9, 3436–3452. doi:10.1021/nn507269g
Turos, E., Shim, J. Y., Wang, Y., Greenhalgh, K., Reddy, G. S., Dickey, S., et al. (2007). Antibiotic-conjugated polyacrylate nanoparticles: new opportunities for development of anti-MRSA agents. Bioorg. Med. Chem. Lett. 17, 53–56. doi:10.1016/j.bmcl.2006.09.098
Tyrone, J. W., Mogford, J. E., Chandler, L. A., Ma, C., Xia, Y., Pierce, G. F., et al. (2000). Collagen-embedded platelet-derived growth factor DNA plasmid promotes wound healing in a dermal ulcer model. J. Surg. Res. 93, 230–236. doi:10.1006/jsre.2000.5912
Uchi, H., Igarashi, A., Urabe, K., Koga, T., Nakayama, J., Kawamori, R., et al. (2009). Clinical efficacy of basic fibroblast growth factor (bFGF) for diabetic ulcer. Eur. J. Dermatol. 19, 461–468. doi:10.1684/ejd.2009.0750
Uckay, I., Aragon-Sanchez, J., Lew, D., and Lipsky, B. A. (2015). Diabetic foot infections: what have we learned in the last 30 years? Int. J. Infect. Dis. 40, 81–91. doi:10.1016/j.ijid.2015.09.023
Unnithan, A. R., Barakat, N. A., Pichiah, P. B., Gnanasekaran, G., Nirmala, R., Cha, Y. S., et al. (2012). Wound-dressing materials with antibacterial activity from electrospun polyurethane-dextran nanofiber mats containing ciprofloxacin HCl. Carbohydr. Polym. 90, 1786–1793. doi:10.1016/j.carbpol.2012.07.071
Wang, E. C., and Wang, A. Z. (2014). Nanoparticles and their applications in cell and molecular biology. Integr. Biol. (Camb.) 6, 9–26. doi:10.1039/C3IB40165K
Wieman, T. J., Smiell, J. M., and SU, Y. (1998). Efficacy and safety of a topical gel formulation of recombinant human platelet-derived growth factor-BB (becaplermin) in patients with chronic neuropathic diabetic ulcers. A phase III randomized placebo-controlled double-blind study. Diabetes Care 21, 822–827. doi:10.2337/diacare.21.5.822
Wittaya-areekul, S., and Prahsarn, C. (2006). Development and in vitro evaluation of chitosan-polysaccharides composite wound dressings. Int. J. Pharm. 313, 123–128. doi:10.1016/j.ijpharm.2006.01.027
Wong, K. K., Cheung, S. O., Huang, L., Niu, J., Tao, C., HO, C. M., et al. (2009). Further evidence of the anti-inflammatory effects of silver nanoparticles. ChemMedChem 4, 1129–1135. doi:10.1002/cmdc.200900049
Wong, V. W., Akaishi, S., Longaker, M. T., and Gurtner, G. C. (2011a). Pushing back: wound mechanotransduction in repair and regeneration. J. Invest. Dermatol. 131, 2186–2196. doi:10.1038/jid.2011.212
Wong, V. W., Sorkin, M., Glotzbach, J. P., Longaker, M. T., and Gurtner, G. C. (2011b). Surgical approaches to create murine models of human wound healing. J. Biomed. Biotechnol. 2011, 969618. doi:10.1155/2011/969618
Wong, V. W., Longaker, M. T., and Gurtner, G. C. (2012). Soft tissue mechanotransduction in wound healing and fibrosis. Semin. Cell Dev. Biol. 23, 981–986. doi:10.1016/j.semcdb.2012.09.010
Xu, H., Lv, F., Zhang, Y., Yi, Z., Ke, Q., Wu, C., et al. (2015). Hierarchically micro-patterned nanofibrous scaffolds with a nanosized bio-glass surface for accelerating wound healing. Nanoscale 7, 18446–18452. doi:10.1039/c5nr04802h
Yang, F., Cho, S. W., Son, S. M., Bogatyrev, S. R., Singh, D., Green, J. J., et al. (2010). Genetic engineering of human stem cells for enhanced angiogenesis using biodegradable polymeric nanoparticles. Proc. Natl. Acad. Sci. U.S.A. 107, 3317–3322. doi:10.1073/pnas.0905432106
Young, S., Wong, M., Tabata, Y., and Mikos, A. G. (2005). Gelatin as a delivery vehicle for the controlled release of bioactive molecules. J. Control Release 109, 256–274. doi:10.1016/j.jconrel.2005.09.023
Yu, H., Peng, J., Xu, Y., Chang, J., and Li, H. (2016). Bioglass activated skin tissue engineering constructs for wound healing. ACS Appl. Mater. Interfaces 8, 703–715. doi:10.1021/acsami.5b09853
Zamora, D. O., Natesan, S., Becerra, S., Wrice, N., Chung, E., Suggs, L. J., et al. (2013). Enhanced wound vascularization using a dsASCs seeded FPEG scaffold. Angiogenesis 16, 745–757. doi:10.1007/s10456-013-9352-y
Zavan, B., Vindigni, V., Vezzu, K., Zorzato, G., Luni, C., Abatangelo, G., et al. (2009). Hyaluronan based porous nano-particles enriched with growth factors for the treatment of ulcers: a placebo-controlled study. J. Mater. Sci. Mater. Med. 20, 235–247. doi:10.1007/s10856-008-3566-3
Zehrer, C. L., Newman, D. K., Grove, G. L., and Lutz, J. B. (2005). Assessment of diaper-clogging potential of petrolatum moisture barriers. Ostomy Wound Manage. 51, 54–58.
Zhang, B., Wang, M., Gong, A., Zhang, X., Wu, X., Zhu, Y., et al. (2015a). HucMSC-Exosome Mediated-Wnt4 signaling is required for cutaneous wound healing. Stem Cells 33, 2158–2168. doi:10.1002/stem.1771
Zhang, Y., Strehin, I., Bedelbaeva, K., Gourevitch, D., Clark, L., Leferovich, J., et al. (2015b). Drug-induced regeneration in adult mice. Sci. Transl. Med. 7, 290ra92. doi:10.1126/scitranslmed.3010228
Zhao, S., Li, L., Wang, H., Zhang, Y., Cheng, X., Zhou, N., et al. (2015). Wound dressings composed of copper-doped borate bioactive glass microfibers stimulate angiogenesis and heal full-thickness skin defects in a rodent model. Biomaterials 53, 379–391. doi:10.1016/j.biomaterials.2015.02.112
Zhou, E. H., Watson, C., Pizzo, R., Cohen, J., Dang, Q., Ferreira de Barros, P. M., et al. (2014). Assessing the impact of engineered nanoparticles on wound healing using a novel in vitro bioassay. Nanomedicine (Lond.) 9, 2803–2815. doi:10.2217/nnm.14.40
Zhou, J., Wang, H., Zhao, S., Zhou, N., Li, L., Huang, W., et al. (2016). In vivo and in vitro studies of borate based glass micro-fibers for dermal repairing. Mater. Sci. Eng. C Mater. Biol. Appl. 60, 437–445. doi:10.1016/j.msec.2015.11.068
Keywords: biomaterials, nanoparticles, nanotherapeutics, regenerative medicine, wound care, wound healing, wound dressings, wounds
Citation: Das S and Baker AB (2016) Biomaterials and Nanotherapeutics for Enhancing Skin Wound Healing. Front. Bioeng. Biotechnol. 4:82. doi: 10.3389/fbioe.2016.00082
Received: 11 May 2016; Accepted: 11 October 2016;
Published: 31 October 2016
Edited by:
Francesca Taraballi, University of Milano-Bicocca, USAReviewed by:
Mohamed N. Rahaman, Missouri University of Science and Technology, USAEmilio Isaac Alarcon, University of Ottawa, Canada
Copyright: © 2016 Das and Baker. This is an open-access article distributed under the terms of the Creative Commons Attribution License (CC BY). The use, distribution or reproduction in other forums is permitted, provided the original author(s) or licensor are credited and that the original publication in this journal is cited, in accordance with accepted academic practice. No use, distribution or reproduction is permitted which does not comply with these terms.
*Correspondence: Aaron B. Baker, YWJiYWtlciYjeDAwMDQwO2F1c3Rpbi51dGV4YXMuZWR1