- Departamento de Química Orgánica, Instituto de Ciencia Molecular (ICMol), Universidad de Valencia, Valencia, Spain
Nanomaterials are proving useful for regenerative medicine in combination with stem cell therapy. Nanoparticles (NPs) can be administrated and targeted to desired tissues or organs and subsequently be used in non-invasive real-time visualization and tracking of cells by means of different imaging techniques, can act as therapeutic agent nanocarriers, and can also serve as scaffolds to guide the growth of new tissue. NPs can be of different chemical nature, such as gold, iron oxide, cadmium selenide, and carbon, and have the potential to be used in regenerative medicine. However, there are still many issues to be solved, such as toxicity, stability, and resident time. Upconversion NPs have relevant properties such as (i) low toxicity, (ii) capability to absorb light in an optical region where absorption in tissues is minimal and penetration is optimal (note they can also be designed to emit in the near-infrared region), and (iii) they can be used in multiplexing and multimodal imaging. An overview on the potentiality of upconversion materials in regenerative medicine is given.
Introduction
Regenerative medicine aims to maintain, regenerate, and replace damaged or non-functional human cells, tissues, or organs to restore normal functions (Engel et al., 2008; Harrison and Sirivisoot, 2011; Gao et al., 2015; Mitragotri et al., 2015) via stimulating the body’s own repair mechanisms. In this context, nanostructures can play an important role regarding implants or scaffolds for tissue engineering and cell therapies, e.g., nanopatterning of surfaces to elicit specific biological responses from the host tissue and organs (Engel et al., 2008; Zhang and Webster, 2009; Harrison and Sirivisoot, 2011). Thus, the generation of new surfaces, structures, and materials containing nanoparticles (NPs) can provide the possibility of mimicking the natural environment of cells and promoting certain functions, such as cell mobility, cell adhesion, and cell differentiation that would be directly related to the nanotopography of the biomaterial (Engel et al., 2008).
The capability of nanomaterials to be multifunctional, as they can consist of different functional components in a single unit, is leading to significant advances over traditional imaging, sensing, and structural technologies (Harrison and Sirivisoot, 2011). Thus, NPs are used in biomedical applications for imaging (cell tracking and visualization), therapy, drug delivery aimed at target biological functions, surface modifications of implantable materials, diagnosis (Engel et al., 2008; Harrison and Sirivisoot, 2011; Gao et al., 2015), and even in the regulation of cell behavior (adhesion, growth, and differentiation), which is of relevance in regenerative medicine (Mitragotri et al., 2015).
New smart biomaterials could be implanted to monitor and direct the regenerative process at the cellular level. For example, NPs could help to monitor a disease (e.g., via emission or magnetism) and bring about tissue repair (e.g., through light-induced targeted delivery) creating better non-invasive regenerative therapies (Harrison and Sirivisoot, 2011; Gao et al., 2015). Interestingly, stem cells have the ability to generate all types of tissues together with an unlimited self-renewal capacity; hence, research is focused on being able to locate, recruit, and mark these cells to monitor and/or trigger the regeneration process (Harrison and Sirivisoot, 2011).
Nanoparticles are also being explored as nanocarriers for theranostic applications (Grazú et al., 2012; Muthu et al., 2014). This new field integrates NP design with simultaneous imaging and therapy, aiming to offer individualized treatments based on in vivo molecular images to allow for a comprehensive diagnosis (Rai et al., 2010). Several NPs have been used as platforms for NP-based theranostics (Choi et al., 2012; Miao et al., 2016): gold nanoparticles (GNPs) (Gao and Li, 2016), carbon nanotubes (CNTs) (Tran et al., 2009; Yun et al., 2012; Fraczek-Szczypta, 2014), magnetic NPs (MNPs) (Gao et al., 2015), silica NPs (SNPs) (Santra et al., 2005; Vivero-Escoto et al., 2012), quantum dots (QDs) (Ho and Leong, 2010), and upconversion NPs (UCNPs) (Chen et al., 2014a), among others. Remarkably, it has been estimated that at least half of the drugs used in 2020 will be based on nanotechnology (Grazú et al., 2012).
Current methods of evaluating cell treatments typically involve destructive or invasive techniques, such as tissue biopsies, whereas traditional non-invasive methods, such as magnetic resonance imaging (MRI) and positron emission tomography (PET), rely heavily on contrast agents and usually lack the specificity or resident time to be a viable option for cell tracking (Engel et al., 2008; Harrison and Sirivisoot, 2011). Photonic applications for diagnostics, therapy, and interventional guidance are increasing (Jin et al., 2011; Rwei et al., 2015). Fiber-optic based catheters can be used to perform localized imaging or laser ablation of a desired target to treat, for example, coronary artery disease (van Soest et al., 2015). Moreover, irradiation density is easy to dose and can provide spatiotemporal control (Rwei et al., 2015; van Soest et al., 2015). The limitations of biophotonic technologies for imaging usually arise from limited penetration depth of light into tissues; however, penetration depth could reach the centimeter scale for applications that rely on near-infrared (NIR) wavelengths and on optical power (diffuse optics and sensing) (Rwei et al., 2015; van Soest et al., 2015). UCNPs are transparent to visible light but can absorb two or more photons in the NIR region and emit at a higher energy level via a non-linear conversion process. Therefore, these luminescent NPs enable high-contrast optical biomedical imaging by suppressing the background of tissue autofluorescence and avoiding high absorption of the tissue.
In this mini-review, an overview of the use of NPs in regenerative medicine and what UCNPs can offer to this field is given.
Nanoparticles in Biomedicine
Nanoparticles used in biomedicine must be biocompatible, water-dispersible, and stable in physiological media (Mu et al., 2014). To date, most of the ongoing nanomedicine clinical trials or those already on the market are being injected and provide passive drug targeting (Li et al., 2010; Grazú et al., 2012; Markovsky et al., 2012; Rwei et al., 2015). Therefore, the stability of nanocarriers in biological media is crucial when formulating nanomedicines (Acharya and Sahoo, 2011; Wu et al., 2011). Their aggregation and rapid clearance have to be avoided (Veiseh et al., 2010), and their pharmacokinetic profile should be studied in advance (Grazú et al., 2012). Equally important, it is extremely difficult for a nanoplatform to selectively reach its target site (Wu et al., 2011). Currently, the most universal way to improve its affinity toward a target is by attachment of ligands [RGD (Zhou et al., 2012), antigen, and folate] that selectively recognize and bind to the target site. This active targeting, which relies on specific interactions, can lead to the accumulation of the nanoplatforms preferentially in, e.g., a tumor region, an ischemic tissue, or an inflamed area as a result of their extravasation through permeable vasculature, an effect known as enhanced permeation and retention (EPR) (Maeda, 2001; Hartner et al., 2009; Albanese et al., 2012).
Multifunctional NPs have been used to design biomaterials and nanoplatforms able to entrap and deliver drugs and biomolecules (such as DNA and growth and differentiation factors, among others bioactive agents) to cells and tissues, for the effective implementation of regenerative therapies (Panyam and Labhasetwar, 2003; Solanki et al., 2008; Grazú et al., 2012). For example, GNPs conjugated with a DNA–polymer complex have been used as nanoscaffolds for delivery of DNA into hMSCs through reverse transfection (Uchimura et al., 2007). They have also been used as a reinforcing- or bioactivity-enhancement phase for polymeric matrices in 3D scaffolds for tissue engineering (Reddy et al., 2006; Engel et al., 2008).
Nanocarriers designed for delivery are able to bypass biological barriers, such as cell membranes and the blood–brain barrier, and can be loaded with high drug concentrations of therapeutics to be released. Once the target site is reached, therapeutic drugs must be delivered from the nanocarrier in order to become bioavailable and aid the regeneration process (Qiu and Park, 2001). Passive processes, such as diffusion, particle erosion, particle degradation, and polymer swelling, can control the release (Mudshinge et al., 2011). The nanoplatform has to be stable enough to promote controlled release of cargo exclusively when triggering (Loomis et al., 2011; Wong and Choi, 2015). Interestingly, the delivery can be activated using in vivo signals, such as pH (Bigall et al., 2011; Sato et al., 2011), ion concentration, redox potential (Kang et al., 2010; Luo et al., 2011), presence of certain enzymes (de la Rica et al., 2012), and temperature (Kim and Lee, 2004). Exogenous triggering using nanomaterials responsive to light (Byoung-chan and Kun, 2012), magnetic fields (Ge et al., 2012; Hawkins et al., 2012), or ultrasounds (Epstein-Barash et al., 2010) can be advantageous, since it can control the timing and degree of release (Ganta et al., 2008), thus minimizing the drug release at off-target sites (Rwei et al., 2015).
Bioimaging Techniques
Bioimaging gives morphological, anatomical, and physiological information of biosamples and reports on the extension of the pathology and organ dysfunction, thus giving valuable information about selecting the best administration route of the elected drug (Naumova et al., 2014; Dong et al., 2015). Different non-invasive imaging techniques, differing in terms of sensitivity, resolution, data acquisition time, penetration depth, and costs, have been explored using NPs (Table 1) (Liu, 2011; Roco et al., 2011; Gao et al., 2013; Naumova et al., 2014; Prodi et al., 2015).
Luminescence Imaging
Light of an external source (UV–VIS–IR light) is absorbed by the contrast (emissive) agent (injected into the cell, tissue, or animal) and subsequently emitted at shorter or longer wavelengths. This low-cost method presents high sensitivity and enables fast analysis. Limitations of luminescence imaging (LI) are high light scattering and autofluorescence of the biological sample when using short wavelengths (UV and VIS light) (Sharma et al., 2006; Prodi et al., 2015; Wolfbeis, 2015).
Raman Imaging
This is based on the Raman effect, which is the inelastic molecular scattering of incident light (Jokerst et al., 2013). Raman imaging (RI) has high specificity because each chemical bond has a characteristic vibrational energy. The absence of photobleaching and autofluorescence background when using NIR excitation makes this technique very promising in regenerative medicine (Gao et al., 2013). However, its weakness is its low photon efficiency (less than one in a million incident photons corresponds to Raman scattering), resulting in weak signals and/or long acquisition times (Liu, 2011). To increase the signal, the Raman active molecules are placed on a metallic plasmonic surface, usually GNPs, due to their very strong surface enhancement capabilities when illuminated at the plasmon resonance band (Jokerst et al., 2013). This technique is termed surface-enhanced Raman scattering (SERS) (Jokerst et al., 2013).
X-Ray Computed Tomography
This is one of the most widely used tools in clinical diagnosis due to its availability, efficiency, and low cost. It directs X-rays to a biosample and measures the decrease in intensity along a linear path, obtaining cross sectional images (Law and Wong, 2014). Computed tomography (CT) can provide anatomic and functional information of bones, organs, and tissues and is generally used in combination with other techniques, such as MRI and PET (Law and Wong, 2014). CT requires short acquisition time, has an unlimited penetration depth, and high spatial resolution; however, its weakness is that it has a limited soft tissue discrimination, and there are concerns on the health risks associated with X-ray radiation (McMahon and Currell, 2013).
Ultrasound Imaging
These images are generated from pulsed sound waves reflected and transmitted between tissue structures, which are eventually detected as echoes (time response to travel back) (Naumova et al., 2014). This technique allows real-time images, presents high sensitivity, and is low cost. However, its disadvantage is its limited penetration depth in tissues. In spite of that, this is one of the most commonly used techniques in clinical assays (Law and Wong, 2014).
Photoacoustic Imaging
The absorbed energy from an external source (VIS–IR light) is transformed into kinetic energy of the sample through energy exchange processes, i.e., the incident light is converted into ultrasonic emission (Gao and Li, 2016). This technique hybridizes the high contrast and spectral selectivity of optical imaging with high ultrasonic resolution. It is a low cost and rapid technique, but has a moderate penetration depth (Prodi et al., 2015).
Magnetic Resonance Imaging
It is based on the principles of nuclear magnetic resonance, which uses magnets to polarize the hydrogen nuclei in water molecules, thus obtaining a spatial distribution of signals emitted from protons in the tissue (Law and Wong, 2014). MRI is a popular technique for cellular imaging in large animals and humans due to its high temporal and spatial contrast, high specificity, absence of ionizing radiation, and unlimited penetration depth. Unfortunately, MRI is expensive and needs a long time of analysis (Yeo et al., 2014).
Nuclear Imaging
Positron emission tomography and single photon emission computed tomography (SPECT) use radionuclides (Law and Wong, 2014). PET uses biologically active positron-emitting radiotracers (such as fluorine-18) that decay and cause the annihilation of a positron and an electron producing two γ-rays. This is one of the most sensitive methods for quantitative measurement of physiologic processes in vivo (Law and Wong, 2014). SPECT consists of the emission of positrons to emit a single γ-ray, which is measured directly by rotating gamma cameras obtaining the image (Law and Wong, 2014). These methodologies possess high sensitivity and no limitations in penetration depth. The main drawbacks are the problems caused by the radiation, long acquisition times, and the presence of artifacts easily generated due to patient movement or bad distribution of the radiotracer (Law and Wong, 2014; Naumova et al., 2014).
Upconversion Nanoparticles in Bioimaging
Upconversion nanoparticles upconvert NIR light (800, 915, and 980 nm) to multi-wavelength light (narrow emission bands at UV, visible, and even NIR region) using a low-power continuous-wave diode laser. NIR excitation allows for deep tissue penetration and avoids autofluorescence of the biological samples (i.e., higher sensitivity) (Philippot and Reiss, 2012; Dong et al., 2015). Moreover, the possibility of using both NIR excitation and NIR emission (NIR-to-NIR upconversion) is particularly relevant for in vivo imaging of small animals, because it permits deep tissue penetration and less absorption and scattering of bio-tissues and organs. These properties combined with their high stability, low cytotoxicity (Gnach et al., 2015; Sun et al., 2015), good photostability, and non-photoblinking or -photobleaching make UCNPs unique optical tools for biological studies (Liu, 2011; DaCosta et al., 2014; Bünzli, 2015; Dong et al., 2015; Prodi et al., 2015). The only drawback of UCNPs in LI is their relatively low upconversion quantum yield; several strategies are being explored to enhance their emission yield (such as covering the UCNPs with an inorganic shell) (Chen et al., 2014a, 2015).
Though UCNPs have been mainly used in LI, recent studies have shown their versatility for other bioimaging techniques. PEG-capped NaYbF4:Er3+, Gd3+ NPs have been effectively internalized by HeLa cells and provided higher contrast images of a rat heart than equivalent concentrations of iobitridol (a current clinical contrast agent) (Liu et al., 2012a). Moreover, UCNPs can be conveniently functionalized with iodinated compounds (silica-coated UCNPs with an iodine compound attached that absorbs X-ray radiation) to make them suitable for CT imaging (Zhang et al., 2011). These UCNPs allowed the visualization of a liver for 30 min, thus demonstrating their long circulating time. Moreover, hybrid BaYbF5 NPs perform well in the in vivo X-ray CT angiography (Liu et al., 2012b). This binary CT agent was more efficient than iobitridol and allowed the visualization of the vasculature in an in vivo mouse model during 2 h.
Upconversion nanoparticles have also been studied as potential photoacoustic imaging (PAI) agents (Maji et al., 2014), using NaYF4:Yb3+, Er3+ NPs covered with α-cyclodextrin. Excitation at 980 nm of the nanosystem dispersed in water led to luminescence quenching due to non-radiative relaxation processes and brought about an enhanced photoacoustic signal. The in vivo localization of the UCNPs in mice showed their capability for PAI (Maji et al., 2014).
In addition, UCNPs containing Gd3+ as dopant and/or as host matrix have been studied as MRI contrast agents. For example, ultra-small NaGdF4 NPs have proved more efficient in MRI angiography and atherosclerotic plaque imaging than some commercial MRI contrast agents, and they were easily excreted by kidney (Xing et al., 2014). Furthermore, a chelating molecule [diethylenetriaminepentaacetic acid (DTPA)] was used to functionalize the surface of UCNPs with the aim of capturing potentially released Gd3+ ions, thus avoiding toxic effects in vivo (Xing et al., 2014). Also, Gd3+ can be incorporated in both, the NP as dopant ion and the ligand. Recent studies demonstrated that simultaneous internal and external incorporation of Gd3+ ions increase MRI sensitivity (Du et al., 2016).
Moreover, UCNPs can be used for multimodal imaging, i.e., the combination of two or more imaging techniques to benefit from their strengths and weaknesses (Chen et al., 2014a; DaCosta et al., 2014; Li et al., 2014a; Bünzli, 2015; Cheng and Lin, 2015; Christ and Schäferling, 2015; Dong et al., 2015; Park et al., 2015; Prodi et al., 2015; Rieffel et al., 2015a; Wu et al., 2015; Zhou et al., 2015). There are examples of UCNPs in bimodal imaging combining PET/LI (Sun et al., 2011), SPECT/LI (Yang et al., 2013), PAI/LI (Maji et al., 2014), MRI/LI (Zhou et al., 2010; Cheng et al., 2013a), CT/LI (Zhang et al., 2011; Liu et al., 2013a; Zheng et al., 2014), and ultrasound imaging (USI)/LI (Jin et al., 2015).
Thus, NaGdF4:Yb3+, Er3+, NPs functionalized with bovine serum albumin attached to DTPA-Gd3+ have proved useful in upconversion LI and MRI (Du et al., 2016). 18F radionuclide has been directly bound to the surface of NaYF4 UCNPs to generate a dual-model bioimaging technique, combining upconversion LI and PET imaging (Sun et al., 2011). Several prevalent diseases are associated with the lymphatic system, which is usually difficult to study by bioimaging due to the lack of techniques with adequate sensitivity and temporal resolution. Recently, NaYF4:Yb, Tm UCNPs have been labeled with 18F−, and the in vivo mouse distribution of 18F-NaYF4:Yb, Tm UCNPs was monitored by upconversion LI. In addition, the mouse lymphatic system was imaged with ultra-high sensitivity by PET (Sun et al., 2011). Moreover, a nanohybrid comprising NaYF4:Yb, Er, and Fe3O4 NPs has shown versatile for upconversion LI and MRI. Mouse MSCs (mMSCs) were labeled with this nanohybrid, and the resulting nanoplatform was injected in mice with two wounds at opposite sides on the abdominal skin. Then, a magnet was placed on one of the wounds for 6 h to target magnetically the mMSCs at one of the wounds. The mMSCs were followed in vivo by LI and MRI, thus observing that the wound treated with mMSCs presented enhanced tissue repairing (Cheng et al., 2013a).
In addition, examples of trimodal (Xing et al., 2012; Cheng et al., 2013b; Ni et al., 2014; Wang et al., 2014a; Yi et al., 2015; Zhai et al., 2015), tetramodal (Sun et al., 2013), and even hexamodal (Rieffel et al., 2015b) imaging by using UCNPs have also been reported.
Upconversion Nanoparticles in Regenerative Medicine
Near-infrared light is particularly attractive to monitor cell–surface interactions in regenerative medicine (Figure 1). NIR-controlled cell adhesion has been achieved with UCNP-based programmable substrates either by using photocaged linkers (Li et al., 2014b) or via photoswitchable substrates (Li et al., 2015). The UCNP harvested NIR light and converted it into UV light, thus inducing the cleavage of the photocaged linkers and on-demand release of adhesive cells (Li et al., 2014b). This strategy not only enables deep tissue photocontrol of the cell adhesion on substrate but also opens a new approach to design UCNP-based cell scaffolds to manipulate dynamically cell–matrix and cell–cell interactions (Li et al., 2014b).
Cell-molecular recognition can be reversibly guided in interfacial systems by using photoswitching systems based on photoisomerization reactions (Weber et al., 2014). In this context, UCNPs have been combined with photoswitchable systems such as spiropyrans (which converts to merocyanine after ring opening) (Zhang et al., 2012a,b; Chen et al., 2014b; Lai et al., 2014; Zhou et al., 2014), as well as diarylethenes (Boyer et al., 2012; Yang et al., 2014) and azobenzenes (cis/trans-isomerization) (Liu et al., 2013b; Wang et al., 2014b). Recently, nanohybrids consisting of core–shell–shell–shell UCNPs, specifically, NaYF4:Tm,Yb@NaYF4@NaYF4:Er,Yb@NaYF4 NPs were coated with silica and conjugated afterward with spiropyran (Figure 2A). This system has been described as a new generation of single-wavelength NIR-controlled photoswitches, which can control the efficient adhesion and detachment of cells reversibly and non-invasively (Li et al., 2015).
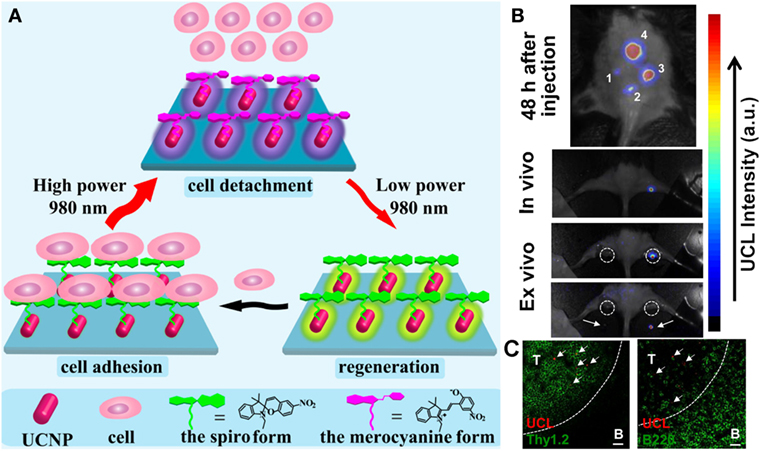
Figure 2. (A) Schematic illustration of the utilization of SP-UCNP as a NIR-triggered photoswitch for non-invasive and reversible control of cell adhesion/detachment by merely altering the power density of a single-wavelength 980-nm laser. Reprinted with permission from Li et al. (2015). Copyright (2015) American Chemical Society (Li et al., 2015). (B) Top: UCL image of a C56BL/6 mouse subcutaneously injected with various numbers of UCNPs labeled DCs (≈50–50,000). Middle: labeled DCs were injected into the rig; strong UCL signals from the draining lymph node were seen under in vivo UCL imaging. Bottom: ex vivo imaging of popliteal lymph nodes (white circles) before (top) and after (bottom) being dissected from the mouse. (C) Immunofluorescence images of the lymph nodes dissected from the mouse injected with UPP@OVA-labeled DCs. T: T-cell zone (Thy1.2+). B: B-cell zone (B220+). Scale bar = 20 μm. Arrows point to UCL signals from labeled DCs. Adapted with permission from Xiang et al. (2015). Copyright (2015) American Chemical Society (Xiang et al., 2015).
Upconversion nanoparticles are unique for live cell tracking because of their optical properties (Vetrone et al., 2010; Tian et al., 2012; Li et al., 2013). Thus, silica/NaYF4:Yb, Er NPs loaded into cells were applied in cell migratory tracking for 5 h by a time-lapse confocal microscope. The direction, speed, and cell–cell interaction of migrating cells were clearly visualized. Consequently, the nanoplatform could be used to track live myoblast cells in a living mouse model with cryoinjured hind limb (Idris et al., 2009). Indeed polymer-coated UCNPs with different emission colors, aimed to multicolor in vivo upconversion LI, well performed in multiplexed lymph node mapping, and multicolor in vivo cancer cell tracking (Cheng et al., 2010).
Even though UCNPs have relatively low upconversion efficiencies, they have shown ultrahigh sensitivity in vivo stem cell tracking (Solanki et al., 2008; Cheng et al., 2013a; Gao et al., 2013). In addition, antigen-loaded UCNPs have recently been used to label and stimulate dendritic cells (DCs) to induce antigen-specific immune response in vivo animals (Figures 2B,C). The homing of DCs to draining lymph nodes was monitored by impressively sensitive in vivo tracking of the NP-labeled DCs by upconversion LI (Xiang et al., 2015); a few DCs were enough to be clearly seen, while, comparatively, thousands of cells are usually needed to enable in vivo tracking in mice when using QDs and MNPs (Kraitchman et al., 2005; Yukawa et al., 2010).
It is worth noting that UCNPs can be used as multifunctional nanoplatforms for light-driven therapies, such as photothermal and photodynamic therapy, and spatiocontrolled drug delivery (Chen et al., 2014a; Shanmugam et al., 2014; Rwei et al., 2015). Therefore, it is presumed that they will become relevant in cell therapy, cell therapy combined with bioimaging, and by extension in regenerative medicine.
Conclusion
Upconversion NPs have unique properties for their application in regenerative medicine. Specifically, they are considered non-toxic; can exhibit NIR-to-visible upconversion luminescence and, more importantly, NIR-to-NIR upconversion luminescence; possess extraordinary (photo)stability; and can be designed with additional features to make them useful tools for other bioimaging techniques or for multimodal imaging. Moreover, UCNPs are excellent candidates for multifunctional NP therapeutics, which can be triggered locally or remotely by NIR light. Thus, UCNPs can be relatively easily visualized in the body and can monitor the biological components and events, and/or may behave as functional scaffolds in the regeneration of damaged tissues and/or organs. Undoubtedly, UCNPs can be used for selective treatment of the affected area using photonics with simultaneous visualization of the process due to their multi-wavelength emission.
Author Contributions
All authors listed, have made substantial, direct and intellectual contribution to the work, and approved it for publication.
Conflict of Interest Statement
The authors declare that the research was conducted in the absence of any commercial or financial relationships that could be construed as a potential conflict of interest.
Funding
We thank the Spanish Ministry of Economy and Competitiveness (CTQ2014-60174-P, FPU grant to LF-S and RyC contract to MG-B).
References
Acharya, S., and Sahoo, S. K. (2011). PLGA nanoparticles containing various anticancer agents and tumour delivery by EPR effect. Adv. Drug Deliv. Rev. 63, 170–183. doi:10.1016/j.addr.2010.10.008
Albanese, A., Tang, P. S., and Chan, W. C. W. (2012). The effect of nanoparticle size, shape, and surface chemistry on biological systems. Annu. Rev. Biomed. Eng. 14, 1–16. doi:10.1146/annurev-bioeng-071811-150124
Bigall, N. C., Curcio, A., Leal, M. P., Falqui, A., Palumberi, D., Di Corato, R., et al. (2011). Magnetic nanocarriers with tunable pH dependence for controlled loading and release of cationic and anionic payloads. Adv. Mater. 23, 5645–5650. doi:10.1002/adma.201103505
Boyer, J.-C., Carling, C.-J., Chua, S. Y., Wilson, D., Johnsen, B., Baillie, D., et al. (2012). Photomodulation of fluorescent upconverting nanoparticle markers in live organisms by using molecular switches. Chemistry 18, 3122–3126. doi:10.1002/chem.201103767
Bünzli, J.-C. G. (2015). Lanthanide light for biology and medical diagnosis. J. Lumin. 170, 866–878. doi:10.1016/j.jlumin.2015.07.033
Byoung-chan, B., and Kun, N. (2012). Development of polymeric cargo for delivery of photosensitizer in photodynamic therapy. Int. J. Photoenergy 2012, 1–14. doi:10.1155/2012/431975
Chen, G., Agren, H., Ohulchanskyy, T. Y., and Prasad, P. N. (2015). Light upconverting core-shell nanostructures: nanophotonic control for emerging applications. Chem. Soc. Rev. 44, 1680–1713. doi:10.1039/c4cs00170b
Chen, G., Qiu, H., Prasad, P. N., and Chen, X. (2014a). Upconversion nanoparticles: design, nanochemistry, and applications in theranostics. Chem. Rev. 114, 5161–5214. doi:10.1021/cr400425h
Chen, Z., Zhou, L., Bing, W., Zhang, Z., Li, Z., Ren, J., et al. (2014b). Light controlled reversible inversion of nanophosphor-stabilized pickering emulsions for biphasic enantioselective biocatalysis. J. Am. Chem. Soc. 136, 7498–7504. doi:10.1021/ja503123m
Cheng, L., Wang, C., Ma, X., Wang, Q., Cheng, Y., Wang, H., et al. (2013a). Multifunctional upconversion nanoparticles for dual-modal imaging-guided stem cell therapy under remote magnetic control. Adv. Funct. Mater. 23, 272–280. doi:10.1002/adfm.201201733
Cheng, L., Wang, C., and Liu, Z. (2013b). Upconversion nanoparticles and their composite nanostructures for biomedical imaging and cancer therapy. Nanoscale 5, 23–37. doi:10.1039/c2nr32311g
Cheng, L., Yang, K., Zhang, S., Shao, M., Lee, S., and Liu, Z. (2010). Highly-sensitive multiplexed in vivo imaging using pegylated upconversion nanoparticles. Nano Res. 3, 722–732. doi:10.1007/s12274-010-0036-2
Cheng, Z., and Lin, J. (2015). Synthesis and application of nanohybrids based on upconverting nanoparticles and polymers. Macromol. Rapid Commun. 36, 790–827. doi:10.1002/marc.201400588
Choi, K. Y., Liu, G., Lee, S., and Chen, X. (2012). Theranostic nanoplatforms for simultaneous cancer imaging and therapy: current approaches and future perspectives. Nanoscale 4, 330–342. doi:10.1039/c1nr11277e
Christ, S., and Schäferling, M. (2015). Chemical sensing and imaging based on photon upconverting nano- and microcrystals: a review. Methods Appl. Fluoresc. 3, 034004. doi:10.1088/2050-6120/3/3/034004
DaCosta, M. V., Doughan, S., Han, Y., and Krull, U. J. (2014). Lanthanide upconversion nanoparticles and applications in bioassays and bioimaging: a review. Anal. Chim. Acta 832, 1–33. doi:10.1016/j.aca.2014.04.030
de la Rica, R., Aili, D., and Stevens, M. M. (2012). Enzyme-responsive nanoparticles for drug release and diagnostics. Adv. Drug Deliv. Rev. 64, 967–978. doi:10.1016/j.addr.2012.01.002
Dong, H., Du, S. R., Zheng, X. Y., Lyu, G. M., Sun, L. D., Li, L. D., et al. (2015). Lanthanide nanoparticles: from design toward bioimaging and therapy. Chem. Rev. 115, 10725–10815. doi:10.1021/acs.chemrev.5b00091
Du, H., Yu, J., Guo, D., Yang, W., Wang, J., and Zhang, B. (2016). Improving the MR imaging sensitivity of upconversion nanoparticles by an internal and external incorporation of the Gd3+ strategy for in vivo tumor-targeted imaging. Langmuir 32, 1155–1165. doi:10.1021/acs.langmuir.5b04186
Engel, E., Michiardi, A., Navarro, M., Lacroix, D., and Planell, J. A. (2008). Nanotechnology in regenerative medicine: the materials side. Trends Biotechnol. 26, 39–47. doi:10.1016/j.tibtech.2007.10.005
Epstein-Barash, H., Orbey, G., Polat, B. E., Ewoldt, R. H., Feshitan, J., Langer, R., et al. (2010). A microcomposite hydrogel for repeated on-demand ultrasound-triggered drug delivery. Biomaterials 31, 5208–5217. doi:10.1016/j.biomaterials.2010.03.008
Fraczek-Szczypta, A. (2014). Carbon nanomaterials for nerve tissue stimulation and regeneration. Mater. Sci. Eng. C 34, 35–49. doi:10.1016/j.msec.2013.09.038
Ganta, S., Devalapally, H., Shahiwala, A., and Amiji, M. (2008). A review of stimuli-responsive nanocarriers for drug and gene delivery. J. Control Release 126, 187–204. doi:10.1016/j.jconrel.2007.12.017
Gao, Y., Cui, Y., Chan, J. K. Y., and Xu, C. (2013). Stem cell tracking with optically active nanoparticles. Am. J. Nucl. Med. Mol. Imaging 3, 232–246.
Gao, Y., and Li, Y. (2016). “Gold nanostructures for cancer imaging and therapy,” in Advances in Nanotheranostics I: Design and Fabrication of Theranosic Nanoparticles, ed. Z. Dai (Berlin; Heidelberg: Springer), 53–101.
Gao, Y., Lim, J., Teoh, S.-H., and Xu, C. (2015). Emerging translational research on magnetic nanoparticles for regenerative medicine. Chem. Soc. Rev. 44, 6306–6329. doi:10.1039/c4cs00322e
Ge, J., Neofytou, E., Cahill, T. J., Beygui, R. E., and Zare, R. N. (2012). Drug release from electric-field-responsive nanoparticles. ACS Nano 6, 227–233. doi:10.1021/nn203430m
Gnach, A., Lipinski, T., Bednarkiewicz, A., Rybka, J., and Capobianco, J. A. (2015). Upconverting nanoparticles: assessing the toxicity. Chem. Soc. Rev. 44, 1561–1584. doi:10.1039/c4cs00177j
Grazú, V., Moros, M., and Sánchez-Espinel, C. (2012). “Nanocarriers as nanomedicines: design concepts and recent advances,” in Frontiers of Nanoscience, Vol. 4, eds J. M. de la Fuente and V. Grazú (Oxford: Elsevier), 337–440.
Harrison, B. S., and Sirivisoot, S. (2011). “Applications of nanotechnology for regenerative medicine,” in Principles of Regenerative Medicine (Second edition), ed. A. A. L. A. T. Nerem (San Diego: Academic Press), 529–540.
Hartner, W. C., Verma, D. D., Levchenko, T. S., Bernstein, E. A., and Torchilin, V. P. (2009). ATP-loaded liposomes for treatment of myocardial ischemia. Wiley Interdiscip. Rev. Nanomed. Nanobiotechnol. 1, 530–539. doi:10.1002/wnan.46
Hawkins, A. M., Bottom, C. E., Liang, Z., Puleo, D. A., and Hilt, J. Z. (2012). Magnetic nanocomposite sol–gel systems for remote controlled drug release. Adv. Healthc. Mater. 1, 96–100. doi:10.1002/adhm.201100013
Ho, Y.-P., and Leong, K. W. (2010). Quantum dot-based theranostics. Nanoscale 2, 60–68. doi:10.1039/b9nr00178f
Idris, N. M., Li, Z., Ye, L., Wei Sim, E. K., Mahendran, R., Ho, P. C.-L., et al. (2009). Tracking transplanted cells in live animal using upconversion fluorescent nanoparticles. Biomaterials 30, 5104–5113. doi:10.1016/j.biomaterials.2009.05.062
Jin, B., Lin, M., You, M., Zong, Y., Wan, M., Xu, F., et al. (2015). Microbubble embedded with upconversion nanoparticles as a bimodal contrast agent for fluorescence and ultrasound imaging. Nanotechnology 26, 345601. doi:10.1088/0957-4484/26/34/345601
Jin, G., Prabhakaran, M. P., Liao, S., and Ramakrishna, S. (2011). Photosensitive materials and potential of photocurrent mediated tissue regeneration. J. Photochem. Photobiol. B Biol. 102, 93–101. doi:10.1016/j.jphotobiol.2010.09.010
Jokerst, J. V., Pohling, C., and Gambhir, S. S. (2013). Molecular imaging with surface-enhanced Raman spectroscopy nanoparticle reporters. MRS Bull. 38. doi:10.1557/mrs.2013.157
Kang, K. W., Chun, M.-K., Kim, O., Subedi, R. K., Ahn, S.-G., Yoon, J.-H., et al. (2010). Doxorubicin-loaded solid lipid nanoparticles to overcome multidrug resistance in cancer therapy. Nanomed. Nanotechnol. 6, 210–213. doi:10.1016/j.nano.2009.12.006
Kim, J.-H., and Lee, T. R. (2004). Thermo- and pH-responsive hydrogel-coated gold nanoparticles. Chem. Mater. 16, 3647–3651. doi:10.1021/cm049764u
Kraitchman, D. L., Tatsumi, M., Gilson, W. D., Ishimori, T., Kedziorek, D., Walczak, P., et al. (2005). Dynamic imaging of allogeneic mesenchymal stem cells trafficking to myocardial infarction. Circulation 112, 1451–1461. doi:10.1161/circulationaha.105.537480
Lai, J., Zhang, Y., Pasquale, N., and Lee, K.-B. (2014). An upconversion nanoparticle with orthogonal emissions using dual NIR excitations for controlled two-way photoswitching. Angew. Chem. Int. Ed. 53, 14419–14423. doi:10.1002/anie.201408219
Law, G.-L., and Wong, W.-T. (2014). “An introduction to molecular imaging,” in The Chemistry of Molecular Imaging, eds N. Long and W.-T. Wong (Hoboken, NJ: John Wiley & Sons, Inc.), 1–24.
Li, F., Feng, W., Zhou, J., and Sun, Y. (2014a). “Lanthanide-based upconversion nanophosphors for bioimaging,” in The Chemistry of Molecular Imaging, eds N. Long and W.-T. Wong (Hoboken, NJ: John Wiley & Sons, Inc.), 299–319.
Li, W., Wang, J., Ren, J., and Qu, X. (2014b). Near-infrared upconversion controls photocaged cell adhesion. J. Am. Chem. Soc. 136, 2248–2251. doi:10.1021/ja412364m
Li, M., Al-Jamal, K. T., Kostarelos, K., and Reineke, J. (2010). Physiologically based pharmacokinetic modeling of nanoparticles. ACS Nano 4, 6303–6317. doi:10.1021/nn1018818
Li, W., Chen, Z., Zhou, L., Li, Z., Ren, J., and Qu, X. (2015). Noninvasive and reversible cell adhesion and detachment via single-wavelength near-infrared laser mediated photoisomerization. J. Am. Chem. Soc. 137, 8199–8205. doi:10.1021/jacs.5b03872
Li, W., Liu, R., Wang, Y., Zhao, Y., and Gao, X. (2013). Temporal techniques: dynamic tracking of nanomaterials in live cells. Small 9, 1585–1594. doi:10.1002/smll.201201508
Liu, H., Lu, W., Wang, H., Rao, L., Yi, Z., Zeng, S., et al. (2013a). Simultaneous synthesis and amine-functionalization of single-phase BaYF5:Yb/Er nanoprobe for dual-modal in vivo upconversion fluorescence and long-lasting X-ray computed tomography imaging. Nanoscale 5, 6023–6029. doi:10.1039/c3nr00999h
Liu, J., Bu, W., Pan, L., and Shi, J. (2013b). NIR-triggered anticancer drug delivery by upconverting nanoparticles with integrated azobenzene-modified mesoporous silica. Angew. Chem. Int. Ed. 52, 4375–4379. doi:10.1002/anie.201300183
Liu, Y., Ai, K., Liu, J., Yuan, Q., He, Y., and Lu, L. (2012a). A high-performance ytterbium-based nanoparticulate contrast agent for in vivo X-Ray computed tomography imaging. Angew. Chem. Int. Ed. 51, 1437–1442. doi:10.1002/anie.201106686
Liu, Y., Ai, K., Liu, J., Yuan, Q., He, Y., and Lu, L. (2012b). Hybrid BaYbF5 nanoparticles: novel binary contrast agent for high-resolution in vivo X-ray computed tomography angiography. Adv. Healthc. Mater. 1, 461–466. doi:10.1002/adhm.201200028
Liu, Z. (2011). “Nanoplatforms for Raman molecular imaging in biological systems,” in Nanoplatform-Based Molecular Imaging, eds X. Chen (Hoboken, NJ: John Wiley & Sons, Inc.), 197–216.
Loomis, K., McNeeley, K., and Bellamkonda, R. V. (2011). Nanoparticles with targeting, triggered release, and imaging functionality for cancer applications. Soft Matter 7, 839–856. doi:10.1039/c0sm00534g
Luo, Z., Cai, K., Hu, Y., Zhao, L., Liu, P., Duan, L., et al. (2011). Mesoporous silica nanoparticles end-capped with collagen: redox-responsive nanoreservoirs for targeted drug delivery. Angew. Chem. Int. Ed. 50, 640–643. doi:10.1002/anie.201005061
Maeda, H. (2001). The enhanced permeability and retention (EPR) effect in tumor vasculature: the key role of tumor-selective macromolecular drug targeting. Adv. Enzyme Regul. 41, 189–207. doi:10.1016/S0065-2571(00)00013-3
Maji, S. K., Sreejith, S., Joseph, J., Lin, M., He, T., Tong, Y., et al. (2014). Upconversion nanoparticles as a contrast agent for photoacoustic imaging in live mice. Adv. Mater. 26, 5633–5638. doi:10.1002/adma.201400831
Markovsky, E., Baabur-Cohen, H., Eldar-Boock, A., Omer, L., Tiram, G., Ferber, S., et al. (2012). Administration, distribution, metabolism and elimination of polymer therapeutics. J. Control Release 161, 446–460. doi:10.1016/j.jconrel.2011.12.021
McMahon, S. J., and Currell, F. J. (2013). “Gold nanoparticles for imaging and radiotherapy,” in Frontiers of Nanoscience, Vol. 5, ed. S. Huw Oxford: (Elsevier), 65–93.
Miao, T., Zhang, Y., Zeng, Y., Tian, R., and Liu, G. (2016). “Functional nanoparticles for molecular imaging-guided gene delivery and therapy,” in Advances in Nanotheranostics II: Cancer Theranostic Nanomedicine, ed. Z. Dai (Singapore: Springer), 273–305.
Mitragotri, S., Anderson, D. G., Chen, X., Chow, E. K., Ho, D., Kabanov, A. V., et al. (2015). Accelerating the translation of nanomaterials in biomedicine. ACS Nano 9, 6644–6654. doi:10.1021/acsnano.5b03569
Mu, Q., Jiang, G., Chen, L., Zhou, H., Fourches, D., Tropsha, A., et al. (2014). Chemical basis of interactions between engineered nanoparticles and biological systems. Chem. Rev. 114, 7740–7781. doi:10.1021/cr400295a
Mudshinge, S. R., Deore, A. B., Patil, S., and Bhalgat, C. M. (2011). Nanoparticles: emerging carriers for drug delivery. Saudi Pharm. J. 19, 129–141. doi:10.1016/j.jsps.2011.04.001
Muthu, M. S., Leong, D. T., Mei, L., and Feng, S.-S. (2014). Nanotheranostics – application and further development of nanomedicine strategies for advanced theranostics. Theranostics 4, 660–677. doi:10.7150/thno.8698
Naumova, A. V., Modo, M., Moore, A., Murry, C. E., and Frank, J. A. (2014). Clinical imaging in regenerative medicine. Nat. Biotechnol. 32, 804–818. doi:10.1038/nbt.2993
Ni, D., Bu, W., Zhang, S., Zheng, X., Li, M., Xing, H., et al. (2014). Single Ho3+-doped upconversion nanoparticles for high-performance T2-weighted brain tumor diagnosis and MR/UCL/CT multimodal imaging. Adv. Funct. Mater. 24, 6613–6620. doi:10.1002/adfm.201401609
Niu, X., Chen, H., Wang, Y., Wang, W., Sun, X., and Chen, L. (2014). Upconversion fluorescence-SERS dual-mode tags for cellular and in vivo imaging. ACS Appl. Mater. Interfaces 6, 5152–5160. doi:10.1021/am500411m
Panyam, J., and Labhasetwar, V. (2003). Biodegradable nanoparticles for drug and gene delivery to cells and tissue. Adv. Drug Deliv. Rev. 55, 329–347. doi:10.1016/S0169-409X(02)00228-4
Park, Y. I., Lee, K. T., Suh, Y. D., and Hyeon, T. (2015). Upconverting nanoparticles: a versatile platform for wide-field two-photon microscopy and multi-modal in vivo imaging. Chem. Soc. Rev. 44, 1302–1317. doi:10.1039/c4cs00173g
Philippot, C., and Reiss, P. (2012). “Synthesis of inorganic nanocrystals for biological fluorescence imaging,” in Frontiers of Nanoscience, eds J. M. de la Fuente and V. Grazu (Oxford: Elsevier), 4.
Prodi, L., Rampazzo, E., Rastrelli, F., Speghini, A., and Zaccheroni, N. (2015). Imaging agents based on lanthanide doped nanoparticles. Chem. Soc. Rev. 44, 4922–4952. doi:10.1039/c4cs00394b
Qiu, Y., and Park, K. (2001). Environment-sensitive hydrogels for drug delivery. Adv. Drug Deliv. Rev. 53, 321–339. doi:10.1016/S0169-409X(01)00203-4
Rai, P., Mallidi, S., Zheng, X., Rahmanzadeh, R., Mir, Y., Elrington, S., et al. (2010). Development and applications of photo-triggered theranostic agents. Adv. Drug Deliv. Rev. 62, 1094–1124. doi:10.1016/j.addr.2010.09.002
Reddy, S. T., Rehor, A., Schmoekel, H. G., Hubbell, J. A., and Swartz, M. A. (2006). In vivo targeting of dendritic cells in lymph nodes with poly(propylene sulfide) nanoparticles. J. Control Release 112, 26–34. doi:10.1016/j.jconrel.2006.01.006
Rieffel, J., Chitgupi, U., and Lovell, J. F. (2015a). Recent advances in higher-order, multimodal, biomedical imaging agents. Small 11, 4445–4461. doi:10.1002/smll.201500735
Rieffel, J., Chen, F., Kim, J., Chen, G., Shao, W., Shao, S., et al. (2015b). Hexamodal imaging with porphyrin-phospholipid-coated upconversion nanoparticles. Adv. Mater. 27, 1785–1790. doi:10.1002/adma.201404739
Roco, M. C., Hersam, M. C., and Mirkin, C. A. (2011). Nanotechnology Research Directions for Societal Needs in 2020. Retrospective and Outlooks. Berlin; Boston: Springer, 690.
Rwei, A. Y., Wang, W., and Kohane, D. S. (2015). Photoresponsive nanoparticles for drug delivery. Nano Today 10, 451–467. doi:10.1016/j.nantod.2015.06.004
Santra, S., Dutta, D., and Moudgil, B. M. (2005). Functional dye-doped silica nanoparticles for bioimaging, diagnostics and therapeutics. Food Bioprod. Process. 83, 136–140. doi:10.1205/fbp.04400
Sato, K., Yoshida, K., Takahashi, S., and Anzai, J.-I. (2011). pH- and sugar-sensitive layer-by-layer films and microcapsules for drug delivery. Adv. Drug Deliv. Rev. 63, 809–821. doi:10.1016/j.addr.2011.03.015
Shanmugam, V., Selvakumar, S., and Yeh, C.-S. (2014). Near-infrared light-responsive nanomaterials in cancer therapeutics. Chem. Soc. Rev. 43, 6254–6287. doi:10.1039/c4cs00011k
Sharma, P., Brown, S., Walter, G., Santra, S., and Moudgil, B. (2006). Nanoparticles for bioimaging. Adv. Colloid Interface Sci. 123–126, 471–485. doi:10.1016/j.cis.2006.05.026
Solanki, A., Kim, J. D., and Lee, K.-B. (2008). Nanotechnology for regenerative medicine: nanomaterials for stem cell imaging. Nanomedicine (Lond) 3, 567–578. doi:10.2217/17435889.3.4.567
Sun, Y., Feng, W., Yang, P., Huang, C., and Li, F. (2015). The biosafety of lanthanide upconversion nanomaterials. Chem. Soc. Rev. 44, 1509–1525. doi:10.1039/c4cs00175c
Sun, Y., Yu, M., Liang, S., Zhang, Y., Li, C., Mou, T., et al. (2011). Fluorine-18 labeled rare-earth nanoparticles for positron emission tomography (PET) imaging of sentinel lymph node. Biomaterials 32, 2999–3007. doi:10.1016/j.biomaterials.2011.01.011
Sun, Y., Zhu, X., Peng, J., and Li, F. (2013). Core-shell lanthanide upconversion nanophosphors as four-modal probes for tumor angiogenesis imaging. ACS Nano 7, 11290–11300. doi:10.1021/nn405082y
Tian, G., Gu, Z., Zhou, L., Yin, W., Liu, X., Yan, L., et al. (2012). Mn2+dopant-controlled synthesis of NaYF4:Yb/Er upconversion nanoparticles for in vivo imaging and drug delivery. Adv. Mater. 24, 1226–1231. doi:10.1002/adma.201104741
Tran, P. A., Zhang, L., and Webster, T. J. (2009). Carbon nanofibers and carbon nanotubes in regenerative medicine. Adv. Drug Deliv. Rev. 61, 1097–1114. doi:10.1016/j.addr.2009.07.010
Uchimura, E., Yamada, S., Uebersax, L., Fujita, S., Miyake, M., and Miyake, J. (2007). Method for reverse transfection using gold colloid as a nano-scaffold. J. Biosci. Bioeng. 103, 101–103. doi:10.1263/jbb.103.101
van Soest, G., Regar, E., and van der Steen, A. F. W. (2015). Photonics in cardiovascular medicine. Nat. Photon. 9, 626–629. doi:10.1038/nphoton.2015.177
Veiseh, O., Gunn, J. W., and Zhang, M. (2010). Design and fabrication of magnetic nanoparticles for targeted drug delivery and imaging. Adv. Drug Deliv. Rev. 62, 284–304. doi:10.1016/j.addr.2009.11.002
Vetrone, F., Naccache, R., Juarranz de la Fuente, A., Sanz-Rodriguez, F., Blazquez-Castro, A., Rodriguez, E. M., et al. (2010). Intracellular imaging of HeLa cells by non-functionalized NaYF4:Er3+, Yb3+ upconverting nanoparticles. Nanoscale 2, 495–498. doi:10.1039/b9nr00236g
Vivero-Escoto, J. L., Huxford-Phillips, R. C., and Lin, W. (2012). Silica-based nanoprobes for biomedical imaging and theranostic applications. Chem. Soc. Rev. 41, 2673–2685. doi:10.1039/c2cs15229k
Wang, L., Liu, J., Dai, Y., Yang, Q., Zhang, Y., Yang, P., et al. (2014a). Efficient gene delivery and multimodal imaging by lanthanide-based upconversion nanoparticles. Langmuir 30, 13042–13051. doi:10.1021/la503444g
Wang, L., Dong, H., Li, Y., Xue, C., Sun, L.-D., Yan, C.-H., et al. (2014b). Reversible near-infrared light directed reflection in a self-organized helical superstructure loaded with upconversion nanoparticles. J. Am. Chem. Soc. 136, 4480–4483. doi:10.1021/ja500933h
Weber, T., Chandrasekaran, V., Stamer, I., Thygesen, M. B., Terfort, A., and Lindhorst, T. K. (2014). Switching of bacterial adhesion to a glycosylated surface by reversible reorientation of the carbohydrate ligand. Angew. Chem. Int. Ed. 53, 14583–14586. doi:10.1002/anie.201409808
Wolfbeis, O. S. (2015). An overview of nanoparticles commonly used in fluorescent bioimaging. Chem. Soc. Rev. 44, 4743–4768. doi:10.1039/c4cs00392f
Wong, P. T., and Choi, S. K. (2015). Mechanisms of drug release in nanotherapeutic delivery systems. Chem. Rev. 115, 3388–3432. doi:10.1021/cr5004634
Wu, L., Zhang, J., and Watanabe, W. (2011). Physical and chemical stability of drug nanoparticles. Adv. Drug Deliv. Rev. 63, 456–469. doi:10.1016/j.addr.2011.02.001
Wu, X., Chen, G., Shen, J., Li, Z., Zhang, Y., and Han, G. (2015). Upconversion nanoparticles: a versatile solution to multiscale biological imaging. Bioconjug. Chem. 26, 166–175. doi:10.1021/bc5003967
Xiang, J., Xu, L., Gong, H., Zhu, W., Wang, C., Xu, J., et al. (2015). Antigen-loaded upconversion nanoparticles for dendritic cell stimulation, tracking, and vaccination in dendritic cell-based immunotherapy. ACS Nano 9, 6401–6411. doi:10.1021/acsnano.5b02014
Xing, H., Bu, W., Zhang, S., Zheng, X., Li, M., Chen, F., et al. (2012). Multifunctional nanoprobes for upconversion fluorescence, MR and CT trimodal imaging. Biomaterials 33, 1079–1089. doi:10.1016/j.biomaterials.2011.10.039
Xing, H., Zhang, S., Bu, W., Zheng, X., Wang, L., Xiao, Q., et al. (2014). Ultrasmall NaGdF4 nanodots for efficient MR angiography and atherosclerotic plaque imaging. Adv. Mater. 26, 3867–3872. doi:10.1002/adma.201305222
Yang, T., Liu, Q., Li, J., Pu, S., Yang, P., and Li, F. (2014). Photoswitchable upconversion nanophosphors for small animal imaging in vivo. RSC Adv. 4, 15613–15619. doi:10.1039/c3ra47529h
Yang, Y., Sun, Y., Cao, T., Peng, J., Liu, Y., Wu, Y., et al. (2013). Hydrothermal synthesis of NaLuF4:153Sm,Yb,Tm nanoparticles and their application in dual-modality upconversion luminescence and SPECT bioimaging. Biomaterials 34, 774–783. doi:10.1016/j.biomaterials.2012.10.022
Yeo, D. C., Minocha, R., and Xu, C. (2014). “Cell engineering with nanoparticles for cell imaging,” in Micro- and Nanoengineering of the Cell Surface, ed. J. M. K. Zhao (Oxford: William Andrew Publishing), 241–251.
Yi, Z., Lu, W., Liu, H., and Zeng, S. (2015). High quality polyacrylic acid modified multifunction luminescent nanorods for tri-modality bioimaging, in vivo long-lasting tracking and biodistribution. Nanoscale 7, 542–550. doi:10.1039/c4nr05161k
Yukawa, H., Kagami, Y., Watanabe, M., Oishi, K., Miyamoto, Y., Okamoto, Y., et al. (2010). Quantum dots labeling using octa-arginine peptides for imaging of adipose tissue-derived stem cells. Biomaterials 31, 4094–4103. doi:10.1016/j.biomaterials.2010.01.134
Yun, Y., Pixley, S., Cui, X. T., Dong, Z., Collins, B., Shanov, V., et al. (2012). Carbon nanomaterials: from therapeutics to regenerative medicine. J. Nanomed. Biother. Discov. 2. doi:10.4172/2155-983x.1000104
Zhai, X., Lei, P., Zhang, P., Wang, Z., Song, S., Xu, X., et al. (2015). Growth of lanthanide-doped LiGdF4 nanoparticles induced by LiLuF4 core as tri-modal imaging bioprobes. Biomaterials 65, 115–123. doi:10.1016/j.biomaterials.2015.06.023
Zhang, B. F., Frigoli, M., Angiuli, F., Vetrone, F., and Capobianco, J. A. (2012a). Photoswitching of bis-spiropyran using near-infrared excited upconverting nanoparticles. Chem. Commun. 48, 7244–7246. doi:10.1039/c2cc33052k
Zhang, C., Xu, C.-H., Sun, L.-D., and Yan, C.-H. (2012b). Photoswitchable upconversion luminescence of rare-earth nanophosphors with covalently grafted spiropyran. Chem. Asian J. 7, 2225–2229. doi:10.1002/asia.201200446
Zhang, G., Liu, Y., Yuan, Q., Zong, C., Liu, J., and Lu, L. (2011). Dual modal in vivo imaging using upconversion luminescence and enhanced computed tomography properties. Nanoscale 3, 4365–4371. doi:10.1039/c1nr10736d
Zhang, L., and Webster, T. J. (2009). Nanotechnology and nanomaterials: promises for improved tissue regeneration. Nano Today 4, 66–80. doi:10.1016/j.nantod.2008.10.014
Zheng, X., Zhou, L., Bu, Y., Yin, W., Hu, Z., Li, M., et al. (2014). Er3+-doped YbPO4 up-conversion porous nanospheres for UCL/CT bimodal imaging in vivo and chemotherapy. J. Mater. Chem. B 2, 6508–6516. doi:10.1039/c4tb00880d
Zhou, A., Wei, Y., Wu, B., Chen, Q., and Xing, D. (2012). Pyropheophorbide A and c(RGDyK) comodified chitosan-wrapped upconversion nanoparticle for targeted near-infrared photodynamic therapy. Mol. Pharm. 9, 1580–1589. doi:10.1021/mp200590y
Zhou, J., Liu, Q., Feng, W., Sun, Y., and Li, F. (2015). Upconversion luminescent materials: advances and applications. Chem. Rev. 115, 395–465. doi:10.1021/cr400478f
Zhou, J., Sun, Y., Du, X., Xiong, L., Hu, H., and Li, F. (2010). Dual-modality in vivo imaging using rare-earth nanocrystals with near-infrared to near-infrared (NIR-to-NIR) upconversion luminescence and magnetic resonance properties. Biomaterials 31, 3287–3295. doi:10.1016/j.biomaterials.2010.01.040
Zhou, L., Chen, Z., Dong, K., Yin, M., Ren, J., and Qu, X. (2014). DNA-mediated construction of hollow upconversion nanoparticles for protein harvesting and near-infrared light triggered release. Adv. Mater. 26, 2424–2430. doi:10.1002/adma.201304437
Keywords: transparency, NIR excitation, upconverted (UV–VIS–NIR) emission, non-toxic nanoparticles, multi-wavelength/multimodal bioimaging, cell behavior regulation
Citation: González-Béjar M, Francés-Soriano L and Pérez-Prieto J (2016) Upconversion Nanoparticles for Bioimaging and Regenerative Medicine. Front. Bioeng. Biotechnol. 4:47. doi: 10.3389/fbioe.2016.00047
Received: 30 March 2016; Accepted: 23 May 2016;
Published: 13 June 2016
Edited by:
Brian McNeill, University of Ottawa Heart Institute, CanadaReviewed by:
Michael Ming-Yuan Wei, University of Texas at Arlington, USASabrina Simoncelli, Ludwig-Maximilians-Universität Munchen, Germany
Copyright: © 2016 González-Béjar, Francés-Soriano and Pérez-Prieto. This is an open-access article distributed under the terms of the Creative Commons Attribution License (CC BY). The use, distribution or reproduction in other forums is permitted, provided the original author(s) or licensor are credited and that the original publication in this journal is cited, in accordance with accepted academic practice. No use, distribution or reproduction is permitted which does not comply with these terms.
*Correspondence: María González-Béjar, bWFyaWEuZ29uemFsZXpAdXYuZXM=;
Julia Pérez-Prieto, anVsaWEucGVyZXpAdXYuZXM=