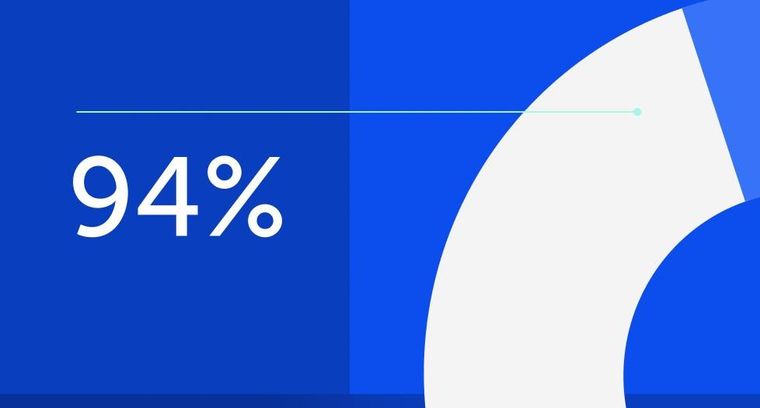
94% of researchers rate our articles as excellent or good
Learn more about the work of our research integrity team to safeguard the quality of each article we publish.
Find out more
ORIGINAL RESEARCH article
Front. Bioinform., 06 June 2022
Sec. Genomic Analysis
Volume 2 - 2022 | https://doi.org/10.3389/fbinf.2022.912795
Probiotics are health-beneficial microorganisms with mainly immunomodulatory and anti-inflammatory properties. Lactobacillus delbrueckii species is a common bacteria used in the dairy industry, and their benefits to hosting health have been reported. This study analyzed the core genome of nine strains of L. delbrueckii species with documented probiotic properties, focusing on genes related to their host health benefits. For this, a combined methodology including several software and databases (BPGA, SPAAN, BAGEL4, BioCyc, KEEG, and InterSPPI) was used to predict the most important characteristics related to L. delbrueckii strains probiose. Comparative genomics analyses revealed that L. delbrueckii probiotic strains shared essential genes related to acid and bile stress response and antimicrobial activity. Other standard features shared by these strains are surface layer proteins and extracellular proteins-encoding genes, with high adhesion profiles that interacted with human proteins of the inflammatory signaling pathways (TLR2/4-MAPK, TLR2/4-NF-κB, and NOD-like receptors). Among these, the PrtB serine protease appears to be a strong candidate responsible for the anti-inflammatory properties reported for these strains. Furthermore, genes with high proteolytic and metabolic activity able to produce beneficial metabolites, such as acetate, bioactive peptides, and B-complex vitamins were also identified. These findings suggest that these proteins can be essential in biological mechanisms related to probiotics’ beneficial effects of these strains in the host.
Lactobacillus delbrueckii is the type species of Lactobacillus genus after a new proposed taxonomic reclassification that divided this genus into 25 new, based on genetic and phylogenetic analysis associated with ecological and metabolic properties (Zheng et al., 2020). This Lactic Acid Bacteria (LAB) member comprises gram-positive, rod-shaped, facultatively anaerobic, and acid-resistant microorganisms, which occupy diverse carbohydrate-rich environments with final fermentative metabolism-derived lactic acid production (Salvetti et al., 2012; Duar et al., 2017). This species includes mainly two subspecies: bulgaricus and lactis, both with high importance in industrial fermented dairy products (primarily yogurt and cheeses production) and biotherapeutics approaches (Hao et al., 2011; El Kafsi et al., 2014; Santos Rocha et al., 2014).
Some studies have been characterizing the L. delbrueckii strains as probiotics based on their ability to resist gastrointestinal tract (GIT) stressors (Ferreira et al., 2013), pathogens inhibition (De Jesus L. C. L. et al., 2021), and anti-inflammatory effects mainly focused on GIT disease treatment, such as colorectal cancer (Wan et al., 2014), ulcerative colitis (Santos Rocha et al., 2014), and intestinal mucositis (De Jesus et al., 2019). In addition, pre-clinical therapeutical applications of these microorganisms to other pathological conditions, such as arthritis (Kano et al., 2013), depression (Qiu et al., 2021), and diabetes (Hallajzadeh et al., 2021), have also been reported. Among this species, L. delbrueckii subsp. lactis CIDCA 133 is the best-characterized probiotics strain whose beneficial characteristics and safety aspects have been widely evaluated by in vitro and in vivo, as well as in silico analysis, for example, its ability to inhibit Escherichia coli, Bacillus cereus, Citrobacter rodentium, and Salmonella Typhimurium pathogens; immunomodulation by inhibition of NF-κB signaling pathway; tolerance to high concentrations of bile salts; no hemolytic or mucin degradation activity, and no adverse effects to clinical and histopathological mice parameters (Rolny et al., 2016; Hugo et al., 2017; De Jesus et al., 2019; De Jesus L. C. L. et al., 2021; De Jesus LCL. et al., 2021; Barroso et al., 2022).
Although most studies focus on the effect and action mechanism of viable probiotic strains, there is a growing interest in applying probiotics as microbiologically non-viable but immunologically active products. This would be more viable and safer for probiotic applications in clinical practice due to safety concerns regarding this active metabolic form favoring the risk of bacterial translocation (Moradi et al., 2020; Teame et al., 2020). Some studies have evaluated the inactivation of these microorganisms or products derived from them in different inflammation models and obtained similar results to their metabolically active form (Sang et al., 2013; Nakai et al., 2021; Trindade et al., 2021).
According to Hill et al. (2014), probiotics are defined as “live microorganisms that confer a health benefit on the host when administered in adequate amounts.” However, it should also be highlighted that most of these beneficial effects attributed to probiotics are strain-dependent, revealing that individual characteristics of the strains provide relevant data for the development of effective probiotic products and facilitate individualized or personalized use for clinical applications (Bubnov et al., 2018; McFarland et al., 2018). This strain-specific property was more substantially related by Rocha et al. (2012) that, when screening 57 dairy L. delbrueckii strains, observed that the immunomodulation levels of these bacteria varied depending on the strain. Among the 37 L. delbrueckii subsp. bulgaricus and 20 L. delbrueckii subsp. lactis strains tested, the most effective immunomodulators strains belong to the subsp. lactis (Rocha et al., 2012), including CNRZ327 and CNRZ333 strains.
Individual biological properties of probiotic strains may be related to a high degree of variation in their genomic content. Thus, studies at the genomic level can provide insights into the main genetic factors and molecular mechanisms associated with the probiotic features of these microorganisms, such as GIT survival, pathogens inhibition, and immunoregulation (Ventura et al., 2012; Salvetti and O,Toole, 2018; Castro-López et al., 2021). Probiotics studies using the genome approach have been performed to identify genetic factors involved with features of different potential probiotics strains, such as Lactobacillus helveticus (Fontana et al., 2019), Lactiplantibacillus plantarum (Zhang et al., 2018), Pediococcus sp. (Wanna et al., 2021), Bifidobacterium sp. (Duar et al., 2020), Enterococcus sp. (Hussein et al., 2020), Lactococcus lactis (Oliveira et al., 2017), among others. In this context, the comparative analysis proves to be an essential tool in probiogenomics, contributing to further exploring the diversity and evolutionary relationship of species (Sun et al., 2015), and identifying and comparing the gene repertoire in different strains (Fontana et al., 2019) and the relationship of these molecules with reported probiotics effects of these bacteria on the host (Papadimitriou et al., 2015; Sun et al., 2015).
Although the importance of L. delbrueckii strains in the food industry, few studies have focused on genomic studies of L. delbrueckii probiotics strains regarding their host health benefits (El Kafsi et al., 2014; Sun et al., 2015; Kanmani et al., 2018; De Jesus L. C. L. et al., 2021). Thus, this study carried out a comprehensive functional gene characterization of L. delbrueckii species with reported probiotics effects, which may be associated with the specific host health benefits of these strains reported phenotypically, and provide a better comprehension of their probiotics features.
Nine genomes of L. delbrueckii strains with reported probiotics properties in the literature (Savino et al., 2011; Santos Rocha et al., 2014; Li et al., 2015, 2017, 2020; Kanmani et al., 2018; Usui et al., 2018; De Jesus et al., 2019; El-Khadragy et al., 2019) were downloaded from the NCBI database (Table 1). The genome assemblies were evaluated by QUAST 5.0.2 (Gurevich et al., 2013) and BUSCO v4.0.6 software (Benchmarking Universal Single-Copy Orthologs) (Simão et al., 2015). In addition, all genomes were annotated using the Prokaryotic Genome Annotation System (Prokka) v1.14.5 software (Seemann, 2014).
This study used the BPGA (Bacterial Pan Genome Analysis) pipeline for performance pan-genome (Chaudhari et al., 2016). The genome sequences were submitted in FASTA format to Orthofinder software to predict orthologs genes (Emms and Kelly, 2019), using default parameters with a p-value cut-off of 1E−5. This software bases its inference method on OrthoMCL (Li et al., 2003) through the hybrid Markov Clustering algorithm (Enright, 2002), which computes sequence similarities with BLAST and then uses the MCL clustering algorithm to identify clusters of highly connected sequences. After this process of predicting orthologous genes, through in-house scripts, these genes were classified according to the subsets of the pangenome, being divided into the core genome, shared, and singletons. For the development of the pangenome, after the classification process in its subsets, in-house scripts were used to estimate what would be the fixed parameters of the Heap Law (Soares et al., 2013; Guimaraes et al., 2015) and the Utterance of the Least Squares Principle (for core genome subsets and singletons). For genetic contexts, we can represent Heap’s Law according to the formula n = k *N γ, which (n) would be the value for the number of genes for a given number of genomes (N); and then k and γ can be considered as free parameters. By this law, γ can be calculated as α = 1—γ, so when α > 1 (γ < 0), the pangenome is called closed, which means that there is no increase, or there is no significant increase, of genes when more genomes of the studied organism are sequenced. If α < 1 (0 < γ < 1), suggests the pan-genome of the probiotic strains is open, which indicates that there is an increase in the number of genes when more genomes are sequenced. The Least Squares Principle Statement can be represented by the formula n = k * exp [−x/t] + tgθ, where (n) is also the number of genes, and k, t, and tgθ are considered as parameters free. With the result of this law, we were able to estimate, based on the number of singletons added to each new sequencing, how many genomes are still needed for the core genome of the studied group to reach stability.
Clusters of Orthologous Groups (COGs) for core genes, accessory genes, and singletons (first unique genes of the strains) were obtained using the eggNOG-mapper v2 web tool (http://eggnog-mapper.embl.de/) (E-value < 0.001) (Cantalapiedra et al., 2021). Furthermore, a complementary functional annotation analysis was carried out using the Kyoto Encyclopedia of Genes and Genomes (KEGG) through the KEGG Mapper/BLASTKOALA tool (https://www.kegg.jp/blastkoala/) (Kanehisa et al., 2016).
Proteins involved in antibacterial activity were also evaluated across the probiotics L. delbrueckii genomes. For this purpose, genes coding bacteriocins were predicted through BAGEL4 (http://bagel4.molgenrug.nl/) (van Heel et al., 2018). The bacteriocins-encoding genes’ distribution among the genomes was visualized through a heatmap of presence and absence. Furthermore, core proteins producing other antimicrobial compounds, such as hydrogen peroxide and organic acids, were investigated using the KEGG Mapper/BLASTKOALA tool (Almeida et al., 2021).
Identification of core proteins of probiotics L. delbrueckii strains related to GIT stress response (acid and bile) and proteolytic activity were manually predicted through Prokka–derived annotation, based on previous studies (Liu et al., 2010; Papadimitriou et al., 2016; De Jesus LCL. et al., 2021).
The presence of genes involved in metabolic pathways related to carbohydrate metabolism, lactate, short-chain fatty acids (SCFAs), and vitamin B biosynthesis was predicted using the BioCyc database (https://biocyc.org/) (Karp et al., 2019). The genomes of L. delbrueckii subsp. lactis DSM 20072 (Genome access: NZ_CP022988.1) and L. delbrueckii subsp. bulgaricus ATCC 11842 (Genome access: NC_008054.1) strains were used for this comparative analysis. Furthermore, the carbohydrate-active enzymes (CAZymes) families were predicted through the Carbohydrate-Active Enzyme (CAZy) database (http://www.cazy.org/) (Cantarel et al., 2009).
To evaluate the potential biological interaction between core proteins of L. delbrueckii probiotic strains and human immune proteins, first, the subcellular localization of proteins identified in the core genome was predicted using SurfG+ software (Barinov et al., 2009). Second, the core proteins were predicted for their ability to be an adhesin calculated by SPAAN software (score >0.7) (Sachdeva et al., 2005). After, immune protein sequences related to the inflammation pathways (TLR2/4-MAPK, TLR2/4-NF-κB, and NOD-like receptor signaling pathways) were mapped and obtained from KEGG pathways and UniProt (UP000005640), respectively (Supplementary Table S1). Finally, the protein-protein interaction was conducted in the InterSPPI v2 web server (http://zzdlab.com/InterSPPI/) (Lian et al., 2019). Graphical analysis of resulting interaction networks (minimum score: 0.9765; specificity: 0.99) was performed by Cytoscape v3.9.0 software (Shannon, 2003).
The probiotics group of Lactobacillus delbrueckii strains evaluated in this study is mainly formed by the subspecies bulgaricus (n = 5) (LJJ, KLDS1.0207, DSM 20080, 2038 and ATCC 11842), delbrueckii (n = 2) (TUA4408L, DSM20074), and lactis (n = 2) (CNRZ327 and CIDCA 133). The strains were mainly isolated from dairy environments, including cheeses, yogurts, and fermented milk. The genome evaluation of these nine strains revealed a genome size and GC content average of 1,951 ± 0.10 Mb and 49.69 ± 0.12%, with 1,664 ± 0.09 protein-coding sequences (CDS) (Table 1).
The pan-genome (total gene repertoire) obtained through BPGA with nine probiotics L. delbrueckii strains is composed of a total of 2,609 genes (Figure 1A), of which 1,268 (48.60%) belong to the core genome (number of genes shared by all strains), 892 genes (34.18%) to the accessory genome (genes shared by two or more strains), and 449 (17.20%) are strain-specific (uniques) (genes present in a single strain) (Figure 1B). Furthermore, the CIDCA 133, DSM20074, and CNRZ327 strains presented the highest exclusive genes, with 102, 76, and 69 genes, respectively, followed by TUA4408L (53 genes), 2038 (47 genes), ATCC11842 (39 genes), KLDS10207 (32 genes), LJJ (16 genes), and DSM20080 (15 genes).
FIGURE 1. Pan-genome of probiotics L. delbrueckii strains. (A) Curve development of pan (blue color) and core (salmon color) genome (α = 0.83). The number of gene families is plotted in function of the genome number. (B) Venn diagram illustrating the core-genome size (center part), accessory genomes (around the center), and unique genes for each strain (extreme values).
According to the curve generated for these nine genomes based on Heap’s Law and leats-square fit of the exponential regression decay, the number of genes families in the pan-genome increased with the addition of each other genome (n = 1,848.134*n^0.156), suggesting that the pan-genome of probiotics L. delbrueckii strains remains open. For the subsets of the core genome and singletons developed by the Utterance of the Least Squares Principle, it can be observed a value of tgθ of approximately 1,182 genes (n = 465.995 * exp [−x/4.839] + 1182.675) for the core genome, and a value of approximately 24 (n = 219.676 * exp [−x/4.356] + 24.813) for the strain-specific. This result shows that at each new sequencing, 24 new genes are added to this pangenome, and it is expected that the core genome will stabilize when it reaches around 1,182 genes (Figure 1A).
Analysis of the COG distribution for the pan-genome revealed that many of the proteins in the core genome are related to “translation, ribosomal structure, and biogenesis” (12%), “replication, recombination and repair” (8%), “amino acid transport and metabolism” (7%), “nucleotide transport and metabolism” (7%), and “unknown function” (18%). The accessory genome presented COG terms related to “amino acid transport and metabolism” (14%), “replication, recombination and repair” (10%), “transcription” (8%), “defense mechanisms” (7%), and “unknown function” (13%). Finally, “replication, recombination and repair” (29%), “amino acid transport and metabolism” (16%), “defense mechanisms” (8%), and “unknown function” (18%) were the most common COGs terms related to unique genes (Figure 2A).
FIGURE 2. Functional analysis of gene families of probiotics L. delbrueckii strains. Distribution of COG (A) and KEGG (B) functional categories in the core, accessory, and unique genes. COG categories: [A] RNA processing and modification, [B] Chromatin structure and dynamics, [C] Energy production and conversion, [D] Cell cycle control, cell division, chromosome partitioning, [E] Amino acid transport and metabolism, [F] Nucleotide transport and metabolism, [G] Carbohydrate transport and metabolism, [H] Coenzyme transport and metabolism, [I] Lipid transport and metabolism, [J] Translation, ribosomal structure and biogenesis, [K] Transcription, [L] Replication, recombination and repair, [M] Cell wall/membrane/envelope biogenesis, [N] Cell motility, [O] Post-translational modification, protein turnover, and chaperones, [P] Inorganic ion transport and metabolism, [Q] Secondary metabolites biosynthesis, transport, and catabolism, [R] General function prediction only, [S] Function unknown, [T] Signal transduction mechanisms, [U] Intracellular trafficking, secretion, and vesicular transport, [V] Defense mechanisms, [W] Extracellular structures, [Y] Nuclear structure, and [Z] Cytoskeleton.
Furthermore, the KEGG annotation revealed that most genes in the core genome are related to “genetic information processing” (35%), followed by “environmental information processing” (10%), “carbohydrates metabolism” (9%), and “signaling and cellular processes” (9%). In the accessory genome, most of the genes were related to “signaling and cellular processes” (24%), “genetic information processing” (16%), “environmental information processing” (12%), and “amino acid metabolism” (10%) function. To unique genes, “amino acid metabolism” (33%), followed by “genetic information processing” (16%), “signaling and cellular processes” (16%), and “carbohydrates metabolism” (16%) were the most frequent categories (Figure 2B).
In the core genome of L. delbrueckii probiotics strains, it was identified some genes encoding proteins that were previously reported to be involved in GIT stress response (acid and bile), including enolase, serine protease HtrA, ornithine decarboxylase, two-component sensor histidine kinase, chaperones (DnaK, DnaJ, GroeL), Na+/H+ antiporter NhaC, F0F1 ATP system genes, S-ribosylhomocysteine lyase, ATP-dependent ClpX protease, glycine/betaine ABC transporter permease, among others (Table 2).
TABLE 2. Predicted proteins identified in the core genome of probiotics Lactobacillus delbrueckii strains involved in acid and bile tolerance.
The core genome of L. delbrueckii probiotic strains encodes various proteolytic enzymes essential for their growth, survival, and organoleptic properties of dairy products manufacturing. These enzymes including oligopeptide ABC transporters system (oppD, oppC, oppF, oppA, oppB), peptidases (pepM, pepQ, pepT, pepO, pepR), and proteinases (PrtB, PrtM). The peptidases mainly cleave substrates containing casein, methionine, proline, cysteine, leucine, serine, asparagine, and glutamate-derived peptides (Supplementary Table S2).
Carbohydrate metabolism was identified as essential enzymes related to glucose, fructose, sucrose, mannose, chitobiose, and galactose. These proteins include 6-phospho-beta-glucosidase, glucokinase, mannose-6-phosphate isomerase, and phosphoglucomutase. Furthermore, some genes related to the transport of cellobiose, mannose, fructose, and glucose carbohydrates were also identified, mainly related to the PTS system, the main carbohydrate active-transport system in bacteria (Supplementary Table S3). It was also determined that the most abundant carbohydrate-active enzymes (CAZy) gene families in the core genome of L. delbrueckii probiotics strains belong to glycosyltransferases (GTs) families (GT1, GT2, GT4, GT26, GT28, GT51) (n = 10), followed by glycoside hydrolases (GHs) (GH4, GH13, GH31, GH32, GH73) (n = 7), and carbohydrate-binding modules (CBMs) (CBM48) (n = 1), respectively.
Genes encoding proteins such as glucokinase, glucose-6-phosphate isomerase, glyceraldehyde 3-phosphate dehydrogenase, phosphoglycerate kinase, ribulose-phosphate 3-epimerase, pyruvate kinase, phosphoketolase, lactate dehydrogenase, and acetate kinase were also identified in the core genome of L. delbrueckii probiotics strains. These essential proteins are involved in the homofermentative or heterofermentative pathways, producing lactate or acetate. Genes involved in the biosynthesis of complex B vitamins were also predicted, including thiamine pyrophosphokinase (thiamine or vitamin B1), a riboflavin kinase (riboflavin or vitamin B2), dihydrofolate reductase (folate or vitamin B9), and cob(I)alamin adenosyltransferase (cobalamin or vitamin B12). No propionate or butyrate-related gene was identified in the core genome (Supplementary Table S4).
The L. delbrueckii strains showed different profiles in terms of bacteriocins. Among all strains, subspecies lactis showed a greater diversity of bacteriocins in their genome, including enterolysin A, helveticin J, and bovicin_255. Few bacteriocins were found for the subspecies bulgaricus. The bacteriocins enterolysin A appears to be conserved in the species (Figure 3). Furthermore, it was identified in the core genome D-lactate dehydrogenase, L-lactate dehydrogenase, acetate kinase, L-lactate oxidase, glycolate oxidase, and pyruvate oxidase genes, which acts like crucial enzymes in the biosynthesis of organic acids (lactate and acetate), and hydrogen peroxide, respectively (Supplementary Table S5).
FIGURE 3. Bacteriocins profile across probiotics L. delbrueckii strains. The heatmap indicates the presence (dark yellow) or absence (dark blue) of bacteriocins-encoding genes.
A total of 1,268 proteins identified in the core genome of L. delbrueckii probiotics strains were classified by SurfG+ software as cytoplasmic (CYT) (n = 918), membrane (ME) (n = 204), protein surfaces exposed (PSE) (n = 105), and secreted (SE) (n = 41) (Figure 4A). Of these proteins, 22 classified as secreted, 17 PSE, eight cytoplasmic, and two membranes were predicted by SPAAN with a high probability of being an adhesin (score >0.7) (Figure 4B), including LysM peptidoglycan-binding domain-containing protein (LysM), aggregation promoting protein (Apf), proteinase B (PrtB), penicillin-binding protein (Pbp1A), oligopeptide ABC transporter (OppA), lipoteichoic acid synthase (LtsA), phosphoglycerate mutase (Pgm1), peptide methionine sulfoxide reductase (MsrA), fluoride efflux transporter CrcB (CrcB), among others (Supplementary Table S6).
FIGURE 4. Core proteins of L. delbrueckii strains interact with human immune proteins involved in inflammatory pathways. (A) Subcellular localization of core proteins. (B) The number of core proteins with adhesin profile. (C) COG categories are assigned to core proteins with adhesin profiles. (D) Subnetwork mapping of L. delbrueckii core proteins (green circles nodes) interacting with human immune proteins (yellow, dark circle nodes). Different line colors indicates the interaction degree between the proteins, based on interaction score: 0.97 (green line), 0.98 (purple line), 0.99 (blue light line), 1.0 (red line).
The functional characteristics of these 49 predicted adhesin-like proteins were determined using COG analysis. These proteins were spread over 13 COGs related to “cellular processes and signaling” (38.7%) (e.g., cell wall/membrane/envelope biogenesis—28.6%; cell motility—2%; intracellular trafficking, secretion, and vesicular transport—2%; cell cycle control, cell division, chromosome partitioning—4.1%; and post-translational modification, protein turnover, and chaperones—2%), “metabolism” (20.4%) (e.g., amino acid transport and metabolism-10.2%; nucleotide transport and metabolism—2%; carbohydrate transport and metabolism—6.2%; and inorganic ion transport and metabolism—2%), and “information, storage and processing” (6.1%) (e.g., transcription—4.1%; and translation, ribosomal structure, and biogenesis—2%). Furthermore, 33.3% of adhesin-like proteins were assigned to the “poorly characterized” category (function unknown) (Figure 4C).
The core proteins with a high adhesion profile were also evaluated to interact with human immune proteins. InterSPPI software predicted 44 interactions (Supplementary Table S7). The proteinase PrtB was the most frequent interaction among the core proteins. Other immunomodulatory proteins were also predicted, such as LysM peptidoglycan-binding domain-containing protein (LysM), lipoteichoic acid synthase (LtsA), penicillin-binding protein (Pbp1b), N-acetylmuramidase (Acm), putative lipoprotein A-antigen (TcsA), among others (Figure 4D), demonstrating that these proteins can be involved with immunoregulatory ability of the L. delbrueckii probiotics strains. Regarding human proteins, the nuclear factor NF-κB p105 subunit (NFKB1), engaged in TLR/NF-κB signaling pathway, was the most frequent interaction. However, other human immune proteins involved in TLR2/4-MAPK, TLR2/4-NF-κB, and NOD-like receptor signaling pathways also interacted with immunoregulatory proteins of L. delbrueckii strains, such as TLR4, TRAF6, RELA, NFKBIA, NOD1, NOD2, FOS, JUN, and MAPK10, among others (Figure 4D; Supplementary Table S7).
Comparative genomics revealed a high variation level in the genome of nine L. delbrueckii probiotics strains, with the subspecies lactis presenting a larger genome size (Mb) than subspecies bulgaricus, corroborating the findings of El Kafsi et al. (2014). This genomic variation can be related to the differences in the number of unique genes observed across the strains, in which the subspecies lactis had the highest number. The pangenome analysis of Lactobacillus delbrueckii species has already been carried out by Inglin et al. (2018) and Kim et al. (2021). However, the above authors did not perform a functional analysis related to the probiosis of these strains. Thus, in our work, the performance of a combined analysis of pan-genome data of nine potential L. delbrueckii probiotics strains allowed us to obtain more robust data related to the most relevant characteristics of the probiose of these strains, mainly related to their ability to survive the TGI, adhesion, antibacterial activity, and immunomodulation.
Functional analysis of the core genome revealed that the proteins of nine L. delbrueckii probiotic strains are mainly involved in genetic and environmental information processing and metabolic activities, which suggests the importance of these genes in conserved cellular processes of these microorganisms to survive and adapt to specific environments or host.
One of the first adaptation steps of probiotics to the host involved molecular/cellular mechanisms related to their response to GIT stressors (stomach acidity and bile salt) (Papadimitriou et al., 2016). The core genome of L. delbrueckii probiotics strains harbors genes related to these stress response mechanisms, mainly including transcriptional regulators expression (e.g., two-component sensor histidine kinase), proton extrusions, and bile efflux (e.g., Na+/H+ antiporter, F0F1 ATPase genes, glycine/betaine ABC transporter permease), metabolic response (e.g., acetate kinase, pyruvate oxidase, ornithine decarboxylase), and heat shock/chaperones proteins production (e.g., GroEL, GroES, DnaK, DnaJ, ClpX). The expression of these genetic factors can be essential to the survival strategy of these bacteria on the GIT, allowing them to arrive in viable amounts sufficient to promote their interactions and beneficial effects with the specific-host sites of action. Genome and phenotype-scale studies demonstrated that these survival and adaptation mechanisms were observed in L. delbrueckii LJJ (Li et al., 2020) strain and are also shared with others, such as UFV H2b20 (Ferreira et al., 2013), 2038 (Hao et al., 2011), ATCC 11842, and CNRZ327 (El Kafsi et al., 2014), and CIDCA 133 (De Jesus LCL. et al., 2021).
This study´s probiotics L. delbrueckii strains were mainly isolated from dairy products, supporting the prediction of core genome enzymes related to a conserved proteolytic and metabolic sugar system. This high metabolic property enhances the fermentation ability of these strains with the production of essential metabolites (e.g., bioactive peptides, lactate, SCFA, and vitamins). These compound’s synthesis requires specific enzymes (e.g., proteinases and peptidases, glyceraldehyde-3-phosphate dehydrogenase, pyruvate kinase, phosphoketolase, acetate kinase, lactate dehydrogenase, riboflavin kinase, thiamine pyrophosphokinase, among others) of these strains, involved in proteolysis, and both phosphoketolase or Embden-Meyerhof (EMP) metabolic pathways (Kandler, 1983; Ye et al., 2021).
A vital feature derived from the fermentation process by probiotic strains is their antimicrobial activity due to organic acids, hydrogen peroxide, and bacteriocins production. The genome of L. delbrueckii probiotics strains has genes coding for these antimicrobial compounds (e.g., bacteriocin enterolysin A, D-lactate dehydrogenase, L-lactate dehydrogenase, acetate kinase, L-lactate oxidase, glycolate oxidase, and pyruvate oxidase), which makes them highly relevant in the food industry, since when used in the fermentation of food dairy products, it can control and preserve these products against the food spoilage of pathogens. The antibacterial effect associated with these L. delbrueckii-producing compounds against some pathogens, such as Salmonella sp., Enterococcus faecalis, Escherichia coli, Gardnerella vaginalis, Listeria monocytogens, Pseudomonas aeruginosa, has been previously reported (Evivie et al., 2020; De Jesus LCL. et al., 2021; Qiu et al., 2021).
It is essential to identify genes/metabolic pathways and characterize bioproducts produced by probiotic bacteria with high fermentative capacity since studies have demonstrated the beneficial effects of fermented products derived from these microorganisms in GIT inflammatory diseases. For example, milk fermented by L. delbrueckii CNRZ327 (2 × 109 CFU/mL) attenuated TNBS-induced colitis in a murine model, improving the epithelial architecture, and reducing inflammatory parameters (IL6, TNFα, MPO) and oxidative markers (COX2 and Hmox) (Plé et al., 2016). Similar effects were reported in L. delbrueckii CIDCA 133, whose milk fermented by the strain preserved the intestinal epithelium from the inflammatory damage caused by the chemotherapy drug 5-FU (300 mg/kg) (De Jesus et al., 2019). Another study demonstrated that intake of yogurt fermented with L. delbrueckii 2038 improves aging by metabolites production and microbiota and intestinal epithelial regulation (Usui et al., 2018). It is suggested that these effects can be associated with the production of organic acids (lactate, SCFA) and bioactive metabolites (vitamins) produced by these bacteria, although these studies did not assess their concentration. However, it is important to highlight that the ability of L. delbrueckii species to produce SCFA or vitamins with host health benefits has been previously reported (Laiño et al., 2012; Levit et al., 2018; Dan et al., 2019), makes them promise to be used as an adjuvant for the treatment of inflammatory GIT diseases and other pathological conditions due to their reported antioxidants, anti-inflammatory, and immunomodulatory properties.
Immunomodulatory and anti-inflammatory properties or probiotics bacterial can also be related to the surface layer proteins or extracellular proteins (Hidalgo-Cantabrana et al., 2020; Chandhni et al., 2021) due to the ability of these proteins to interact with the host cells via pattern recognition receptors (e.g., Toll-like receptors-TLR, NOD-like receptors-NLR) inducing specific signalization pathways responses, as nuclear factor kappa B (NF-κB) and mitogen-activated protein kinase (MAPK) (Delgado et al., 2020). This hypothesis is corroborated by Rocha et al. (2012) when they showed that surface-exposed proteins of the L. delbrueckii CNRZ333 strain played a role in NF-κB immune modulation (Rocha et al., 2012).
L. delbrueckii strains characterized as probiotics shared genes with potential interaction with inflammatory pathways-related human immune proteins, including proteinase B (PrtB), penicillin-binding protein (Pbp1A), and lipoteichoic acid synthase (LtsA), among others, with PrtB being the most interacting protein. PrtB is a cell envelope-associated serine protease essential to milk casein degradation (Gilbert et al., 1996). The expression of this protein and its analogs producing bioactive health-beneficial peptides has been suggested to be crucial to the immunomodulatory properties of L. delbrueckii strains (De Jesus LCL. et al., 2021). For example, De Jesus LCL. et al. (2021) showed that predicted proteins of L. delbrueckii CIDCA 133 with a high adhesin profile, including PrtB protein, interacted with human immune proteins involved with NF-κB signaling pathway activation. These findings can be related to their in vivo results. It was demonstrated that consumption of this probiotic strain presented an anti-inflammatory profile by activating TLRs receptors (Tlr2, Tlr4), decreasing Nfkb1 and enhancing immunoregulatory markers Il10 and Tgfb gene expression (De Jesus LCL. et al., 2021). These results are also supported by Espeche Turbay et al. (2012). They demonstrated that milk β-casein degradation by L. delbrueckii CRL581 ameliorates TNBS-induced acute intestinal inflammation by increasing immunoregulatory IL10 and decreasing leukocytes infiltrate and the IFNγ pro-inflammatory marker (Espeche Turbay et al., 2012). It is believed that these effects can be attributed to its cell envelope-associated proteinase PrtL activity (Villegas et al., 2015).
Anti-inflammatory properties of other surface layer components of L. delbrueckii strains have also been reported as extracellular polysaccharides of L. delbrueckii TUA4408L, which presented antiviral activity against rotavirus infection in porcine cells by modulating TLR2/4, interferon regulatory factor (IRF)-3, and the antiviral factors IFN-β, MxA, and RNase L expression (Kanmani et al., 2018). Altogether, these findings reveal that these bacteria factors are essential to leading the biological process of the host, mainly immune regulation. Therefore, based on these findings, the knowledge at the genomic level of the individual characteristics of probiotics, as well as the genetic factors associated with their immunoregulatory capacity, can facilitate individualized or personalized use of them for clinical applications, thus being an alternative approach to the problems arising from the use of live beneficial microorganisms in clinical practice. Furthermore, the exploration of genetic factors can contribute to validating the role of these probiotics-derived bioactive molecules in different pathological conditions, including their beneficial effects on those that affect distant sites and organs (e.g., skin, respiratory and urogenital tracts, brain, bones, among others) (Reid et al., 2017; Bubnov et al., 2018). Thus, we reinforce that further studies, including knockout genes or heterologous production of these proteins, must be performed to validate these genotypic findings with the phenotypic reported results described for these strains and elucidate the underlying mechanisms involved in their immunomodulatory activities.
In summary, this first probiotic genomic characterization study for potential L. delbrueckii probiotics species shows that these bacteria share a broad gene repertoire that functionally may be responsible for phenotypic features attributed to these strains on the host. The data presented support other studies that aim to identify genetic factors and mechanisms related to the beneficial effects of new probiotic targets from the Lactobacillus species with high commercial and biotechnological relevance. Furthermore, these data open perspectives for new studies to be carried out to evaluate the predicted interacting bacteria proteins with human immune proteins as possible anti-inflammatory molecules to be tested in therapeutic approaches to different inflammatory conditions.
Conceptualization: LJ and FA; Methodology: LJ, TS, AF, and FA; Formal analysis and investigation: LJ and FA; Writing-original draft preparation: LJ; Writing-review and editing: FA, TS, SS, LA, and VA. All authors read and approved the final manuscript.
This work was supported by the National Council for Scientific and Technological Development (CNPq) (Grant number 312045/2020-4).
The authors declare that the research was conducted in the absence of any commercial or financial relationships that could be construed as a potential conflict of interest.
All claims expressed in this article are solely those of the authors and do not necessarily represent those of their affiliated organizations, or those of the publisher, the editors and the reviewers. Any product that may be evaluated in this article, or claim that may be made by its manufacturer, is not guaranteed or endorsed by the publisher.
The authors would like to acknowledge the Pró-Reitoria de Pesquisa—UFMG, Instituto Oswaldo Cruz-FIOCRUZ, and funding agencies FAPEMIG, CAPES, and CNPq.
The Supplementary Material for this article can be found online at: https://www.frontiersin.org/articles/10.3389/fbinf.2022.912795/full#supplementary-material
Barinov, A., Loux, V., Hammani, A., Nicolas, P., Langella, P., Ehrlich, D., et al. (2009). Prediction of Surface Exposed Proteins in Streptococcus pyogenes, with a Potential Application to Other Gram-Positive Bacteria. Proteomics 9, 61–73. doi:10.1002/pmic.200800195
Barroso, F. A. L., de Jesus, L. C. L., da Silva, T. F., Batista, V. L., Laguna, J., Coelho-Rocha, N. D., et al. (2022). Lactobacillus delbrueckii CIDCA 133 Ameliorates Chemotherapy-Induced Mucositis by Modulating Epithelial Barrier and TLR2/4/Myd88/NF-Κb Signaling Pathway. Front. Microbiol. 13, 858036. doi:10.3389/fmicb.2022.858036
Bubnov, R. V., Babenko, L. P., Lazarenko, L. M., Mokrozub, V. V., and Spivak, M. Y. (2018). Specific Properties of Probiotic Strains: Relevance and Benefits for the Host. EPMA J. 9, 205–223. doi:10.1007/s13167-018-0132-z
Cantalapiedra, C. P., Hernández-Plaza, A., Letunic, I., Bork, P., and Huerta-Cepas, J. (2021). eggNOG-Mapper V2: Functional Annotation, Orthology Assignments, and Domain Prediction at the Metagenomic Scale. Mol. Biol. Evol. 38, 5825–5829. doi:10.1093/molbev/msab293
Cantarel, B. L., Coutinho, P. M., Rancurel, C., Bernard, T., Lombard, V., and Henrissat, B. (2009). The Carbohydrate-Active EnZymes Database (CAZy): an Expert Resource for Glycogenomics. Nucleic Acids Res. 37, D233–D238. doi:10.1093/nar/gkn663
Castro-López, C., García, H. S., Guadalupe Martínez-Ávila, G. C., González-Córdova, A. F., Vallejo-Cordoba, B., and Hernández-Mendoza, A. (2021). Genomics-based Approaches to Identify and Predict the Health-Promoting and Safety Activities of Promising Probiotic Strains - A Probiogenomics Review. Trends Food Sci. Technol. 108, 148–163. doi:10.1016/j.tifs.2020.12.017
Chandhni, P. R., Pradhan, D., Sowmya, K., Gupta, S., Kadyan, S., Choudhary, R., et al. (2021). Ameliorative Effect of Surface Proteins of Probiotic Lactobacilli in Colitis Mouse Models. Front. Microbiol. 12, 679773. doi:10.3389/fmicb.2021.679773
Chaudhari, N. M., Gupta, V. K., and Dutta, C. (2016). BPGA- an Ultra-fast Pan-Genome Analysis Pipeline. Sci. Rep. 6, 24373. doi:10.1038/srep24373
Dan, T., Ren, W., Liu, Y., Tian, J., Chen, H., Li, T., et al. (2019). Volatile Flavor Compounds Profile and Fermentation Characteristics of Milk Fermented by Lactobacillus delbrueckii subsp. bulgaricus. Front. Microbiol. 10, 2183. doi:10.3389/fmicb.2019.02183
De Jesus, L. C. L., Drumond, M. M., Aburjaile, F. F., Sousa, T. J., Coelho-Rocha, N. D., Profeta, R., et al. (2021b). Probiogenomics of Lactobacillus delbrueckii subsp. lactis CIDCA 133: In Silico, In Vitro, and In Vivo Approaches. Microorganisms 9, 829. doi:10.3390/microorganisms9040829
De Jesus, L. C. L., de Jesus Sousa, T., Coelho-Rocha, N. D., Profeta, R., Barroso, F. A. L., Drumond, M. M., et al. (2021a). Safety Evaluation of Lactobacillus delbrueckii subsp. lactis CIDCA 133: a Health-Promoting Bacteria. Probiotics Antimicro. Prot. doi:10.1007/s12602-021-09826-z
De Jesus, L. C. L., Drumond, M. M., de Carvalho, A., Santos, S. S., Martins, F. S., Ferreira, Ê., et al. (2019). Protective Effect of Lactobacillus delbrueckii subsp. lactis CIDCA 133 in a Model of 5 Fluorouracil-Induced Intestinal Mucositis. J. Funct. Foods 53, 197–207. doi:10.1016/j.jff.2018.12.027
Delgado, S., Sánchez, B., Margolles, A., Ruas-Madiedo, P., and Ruiz, L. (2020). Molecules Produced by Probiotics and Intestinal Microorganisms with Immunomodulatory Activity. Nutrients 12, 391. doi:10.3390/nu12020391
Duar, R. M., Casaburi, G., Mitchell, R. D., Scofield, L. N. C., Ortega Ramirez, C. A., Barile, D., et al. (2020). Comparative Genome Analysis of Bifidobacterium longum subsp. infantis Strains Reveals Variation in Human Milk Oligosaccharide Utilization Genes Among Commercial Probiotics. Nutrients 12, 3247. doi:10.3390/nu12113247
Duar, R. M., Lin, X. B., Zheng, J., Martino, M. E., Grenier, T., Pérez-Muñoz, M. E., et al. (2017). Lifestyles in Transition: Evolution and Natural History of the Genus Lactobacillus. FEMS Microbiol. Rev. 41, S27–S48. doi:10.1093/femsre/fux030
El Kafsi, H., Binesse, J., Loux, V., Buratti, J., Boudebbouze, S., Dervyn, R., et al. (2014). Lactobacillus delbrueckii ssp. lactis and ssp. bulgaricus: a Chronicle of Evolution in Action. BMC Genomics 15, 407. doi:10.1186/1471-2164-15-407
El-Khadragy, M. F., Al-Olayan, E. M., Elmallah, M. I. Y., Alharbi, A. M., Yehia, H. M., and Abdel Moneim, A. E. (2019). Probiotics and Yogurt Modulate Oxidative Stress and Fibrosis in Livers of Schistosoma mansoni-infected Mice. BMC Complement. Altern. Med. 19, 3. doi:10.1186/s12906-018-2406-3
Emms, D. M., and Kelly, S. (2019). OrthoFinder: Phylogenetic Orthology Inference for Comparative Genomics. Genome Biol. 20, 238. doi:10.1186/s13059-019-1832-y
Enright, A. J., Van Dongen, S., and Ouzounis, C. A. (2002). An Efficient Algorithm for Large-Scale Detection of Protein Families. Nucleic Acids Res. 30, 1575–1584. doi:10.1093/nar/30.7.1575
Espeche Turbay, M. B., de Moreno de LeBlanc, A., Perdigón, G., Savoy de Giori, G., and Hebert, E. M. (2012). β-Casein Hydrolysate Generated by the Cell Envelope-Associated Proteinase of Lactobacillus delbrueckii ssp. lactis CRL 581 Protects against Trinitrobenzene Sulfonic Acid-Induced Colitis in Mice. J. Dairy Sci. 95, 1108–1118. doi:10.3168/jds.2011-4735
Evivie, S. E., Abdelazez, A., Li, B., Lu, S., Liu, F., and Huo, G. (2020). Lactobacillus delbrueckii subsp. bulgaricus KLDS 1.0207 Exerts Antimicrobial and Cytotoxic Effects In Vitro and Improves Blood Biochemical Parameters In Vivo against Notable Foodborne Pathogens. Front. Microbiol. 11, 583070. doi:10.3389/fmicb.2020.583070
Ferreira, A. B., De Oliveira, M. N., Freitas, F. S., Alfenas-Zerbini, P., Da Silva, D. F., De Queiroz, M. V., et al. (2013). Increased Expression of Clp Genes in Lactobacillus delbrueckii UFV H2b20 Exposed to Acid Stress and Bile Salts. Benef. Microbes 4, 367–374. doi:10.3920/BM2013.0022
Fontana, A., Falasconi, I., Molinari, P., Treu, L., Basile, A., Vezzi, A., et al. (2019). Genomic Comparison of Lactobacillus helveticus Strains Highlights Probiotic Potential. Front. Microbiol. 10, 1380. doi:10.3389/fmicb.2019.01380
Gilbert, C., Atlan, D., Blanc, B., Portailer, R., Germond, J. E., Lapierre, L., et al. (1996). A New Cell Surface Proteinase: Sequencing and Analysis of the prtB Gene from Lactobacillus delbruekii subsp. bulgaricus. J. Bacteriol. 178, 3059–3065. doi:10.1128/jb.178.11.3059-3065.1996
Guimarães, L. C., Florczak-Wyspianska, J., de Jesus, L. B., Viana, M. V., Silva, A., Ramos, R. T., et al. (2015). Inside the Pan-Genome - Methods and Software Overview. Curr. Genomics 16, 245–252. doi:10.2174/1389202916666150423002311
Gurevich, A., Saveliev, V., Vyahhi, N., and Tesler, G. (2013). QUAST: Quality Assessment Tool for Genome Assemblies. Bioinformatics 29, 1072–1075. doi:10.1093/bioinformatics/btt086
Hallajzadeh, J., Eslami, R. D., and Tanomand, A. (2021). Effect of Lactobacillus delbrueckii subsp. lactis PTCC1057 on Serum Glucose, Fetuin-A ,and Sestrin 3 Levels in Streptozotocin-Induced Diabetic Mice. Probiotics Antimicrob. Proteins 13, 383–389. doi:10.1007/s12602-020-09693-0
Hao, P., Zheng, H., Yu, Y., Ding, G., Gu, W., Chen, S., et al. (2011). Complete Sequencing and Pan-Genomic Analysis of Lactobacillus delbrueckii subsp. bulgaricus Reveal its Genetic Basis for Industrial Yogurt Production. PLoS One 6, e15964. doi:10.1371/journal.pone.0015964
Hidalgo-Cantabrana, C., Moro-García, M. A., Blanco-Míguez, A., Fdez-Riverola, F., Oliván, M., Royo, L. J., et al. (2020). The Extracellular Proteins of Lactobacillus acidophilus DSM 20079T Display Anti-inflammatory Effect in Both in Piglets, Healthy Human Donors and Crohn's Disease Patients. J. Funct. Foods 64, 103660. doi:10.1016/j.jff.2019.103660
Hill, C., Guarner, F., Reid, G., Gibson, G. R., Merenstein, D. J., Pot, B., et al. (2014). Expert Consensus Document. The International Scientific Association for Probiotics and Prebiotics Consensus Statement on the Scope and Appropriate Use of the Term Probiotic. Nat. Rev. Gastroenterol. Hepatol. 11, 506–514. doi:10.1038/nrgastro.2014.66
Hugo, A. A., Rolny, I. S., Romanin, D., and Pérez, P. F. (2017). Lactobacillus delbrueckii subsp. lactis (Strain CIDCA 133) Stimulates Murine Macrophages Infected with Citrobacter rodentium. World J. Microbiol. Biotechnol. 33, 48. doi:10.1007/s11274-017-2219-4
Hussein, W. E., Abdelhamid, A. G., Rocha-Mendoza, D., García-Cano, I., and Yousef, A. E. (2020). Assessment of Safety and Probiotic Traits of Enterococcus durans OSY-EGY, Isolated from Egyptian Artisanal Cheese, Using Comparative Genomics and Phenotypic Analyses. Front. Microbiol. 11, 608314. doi:10.3389/fmicb.2020.608314
Inglin, R. C., Meile, L., and Stevens, M. J. A. (2018). Clustering of Pan- and Core-Genome of Lactobacillus Provides Novel Evolutionary Insights for Differentiation. BMC Genomics 19, 284. doi:10.1186/s12864-018-4601-5
Kandler, O. (1983). Carbohydrate Metabolism in Lactic Acid Bacteria. Ant. Van Leeuwenhoek 49, 209–224. doi:10.1007/BF00399499
Kanehisa, M., Sato, Y., and Morishima, K. (2016). BlastKOALA and GhostKOALA: KEGG Tools for Functional Characterization of Genome and Metagenome Sequences. J. Mol. Biol. 428, 726–731. doi:10.1016/j.jmb.2015.11.006
Kanmani, P., Albarracin, L., Kobayashi, H., Hebert, E. M., Saavedra, L., Komatsu, R., et al. (2018). Genomic Characterization of Lactobacillus delbrueckii TUA4408L and Evaluation of the Antiviral Activities of its Extracellular Polysaccharides in Porcine Intestinal Epithelial Cells. Front. Immunol. 9, 2178. doi:10.3389/fimmu.2018.02178
Kano, H., Kita, J., Makino, S., Ikegami, S., and Itoh, H. (2013). Oral Administration of Lactobacillus delbrueckii subspecies bulgaricus OLL1073R-1 Suppresses Inflammation by Decreasing Interleukin-6 Responses in a Murine Model of Atopic Dermatitis. J. Dairy Sci. 96, 3525–3534. doi:10.3168/jds.2012-6514
Karp, P. D., Billington, R., Caspi, R., Fulcher, C. A., Latendresse, M., Kothari, A., et al. (2019). The BioCyc Collection of Microbial Genomes and Metabolic Pathways. Brief. Bioinform. 20, 1085–1093. doi:10.1093/bib/bbx085
Kim, E., Cho, E. J., Yang, S. M., and Kim, H. Y. (2021). Identification and Monitoring of Lactobacillus delbrueckii Subspecies Using Pangenomic-Based Novel Genetic Markers. J. Microbiol. Biotechnol. 31, 280–289. doi:10.4014/jmb.2009.09034
Laiño, J. E., LeBlanc, J. G., and Savoy de Giori, G. (2012). Production of Natural Folates by Lactic Acid Bacteria Starter Cultures Isolated from Artisanal Argentinean Yogurts. Can. J. Microbiol. 58, 581–588. doi:10.1139/w2012-026
Levit, R., Savoy de Giori, G., de Moreno de LeBlanc, A., and LeBlanc, J. G. (2018). Effect of Riboflavin-Producing Bacteria against Chemically Induced Colitis in Mice. J. Appl. Microbiol. 124, 232–240. doi:10.1111/jam.13622
Li, B., Jin, D., Yu, S., Etareri Evivie, S., Muhammad, Z., Huo, G., et al. (2017). In Vitro and In Vivo Evaluation of Lactobacillus delbrueckii subsp. bulgaricus KLDS1.0207 for the Alleviative Effect on Lead Toxicity. Nutrients 9, 845. doi:10.3390/nu9080845
Li, C., Sun, J., Qi, X., and Liu, L. (2015). NaCl Stress Impact on the Key Enzymes in Glycolysis from Lactobacillus bulgaricus during Freeze-Drying. Braz J. Microbiol. 46, 1193–1199. doi:10.1590/S1517-838246420140595
Li, L., Stoeckert, C. J., and Roos, D. S. (2003). OrthoMCL: Identification of Ortholog Groups for Eukaryotic Genomes. Genome Res. 13, 2178–2189. doi:10.1101/gr.1224503
Li, W., Yang, L., Nan, W., Lu, J., Zhang, S., Ujiroghene, O. J., et al. (2020). Whole-genome Sequencing and Genomic-Based Acid Tolerance Mechanisms of Lactobacillus delbrueckii subsp. bulgaricus LJJ. Appl. Microbiol. Biotechnol. 104, 7631–7642. doi:10.1007/s00253-020-10788-5
Lian, X., Yang, S., Li, H., Fu, C., and Zhang, Z. (2019). Machine-Learning-Based Predictor of Human-Bacteria Protein-Protein Interactions by Incorporating Comprehensive Host-Network Properties. J. Proteome Res. 18, 2195–2205. doi:10.1021/acs.jproteome.9b00074
Liu, M., Bayjanov, J. R., Renckens, B., Nauta, A., and Siezen, R. J. (2010). The Proteolytic System of Lactic Acid Bacteria Revisited: a Genomic Comparison. BMC Genomics 11, 36. doi:10.1186/1471-2164-11-36
McFarland, L. V., Evans, C. T., and Goldstein, E. J. C. (2018). Strain-Specificity and Disease-Specificity of Probiotic Efficacy: A Systematic Review and Meta-Analysis. Front. Med. (Lausanne) 5, 124. doi:10.3389/fmed.2018.00124
Moradi, M., Kousheh, S. A., Almasi, H., Alizadeh, A., Guimarães, J. T., Yılmaz, N., et al. (2020). Postbiotics Produced by Lactic Acid Bacteria: The Next Frontier in Food Safety. Compr. Rev. Food Sci. Food Saf. 19, 3390–3415. doi:10.1111/1541-4337.12613
Nakai, H., Murosaki, S., Yamamoto, Y., Furutani, M., Matsuoka, R., and Hirose, Y. (2021). Safety and Efficacy of Using Heat-Killed Lactobacillus plantarum L-137: High-Dose and Long-Term Use Effects on Immune-Related Safety and Intestinal Bacterial Flora. J. Immunotoxicol. 18, 127–135. doi:10.1080/1547691X.2021.1979698
Oliveira de Almeida, M., Carvalho, R., Figueira Aburjaile, F., Malcher Miranda, F., Canário Cerqueira, J., Brenig, B., et al. (2021). Characterization of the First Vaginal Lactobacillus crispatus Genomes Isolated in Brazil. PeerJ 9, e11079. doi:10.7717/peerj.11079
Oliveira, L. C., Saraiva, T. D., Silva, W. M., Pereira, U. P., Campos, B. C., Benevides, L. J., et al. (2017). Analyses of the Probiotic Property and Stress Resistance-Related Genes of Lactococcus lactis subsp. lactis NCDO 2118 through Comparative Genomics and In Vitro Assays. PLoS One 12, e0175116. doi:10.1371/journal.pone.0175116
Papadimitriou, K., Alegría, Á., Bron, P. A., de Angelis, M., Gobbetti, M., Kleerebezem, M., et al. (2016). Stress Physiology of Lactic Acid Bacteria. Microbiol. Mol. Biol. Rev. 80, 837–890. doi:10.1128/MMBR.00076-15
Papadimitriou, K., Zoumpopoulou, G., Foligné, B., Alexandraki, V., Kazou, M., Pot, B., et al. (2015). Discovering Probiotic Microorganisms: In Vitro, In Vivo, Genetic and Omics Approaches. Front. Microbiol. 6, 58. doi:10.3389/fmicb.2015.00058
Plé, C., Breton, J., Richoux, R., Nurdin, M., Deutsch, S. M., Falentin, H., et al. (2016). Combining Selected Immunomodulatory Propionibacterium freudenreichii and Lactobacillus delbrueckii Strains: Reverse Engineering Development of an Anti-inflammatory Cheese. Mol. Nutr. Food Res. 60, 935–948. doi:10.1002/mnfr.201500580
Qiu, X., Wu, G., Wang, L., Tan, Y., and Song, Z. (2021). Lactobacillus delbrueckii Alleviates Depression-like Behavior through Inhibiting Toll-like Receptor 4 (TLR4) Signaling in Mice. Ann. Transl. Med. 9, 366. doi:10.21037/atm-20-4411
Reid, G., Abrahamsson, T., Bailey, M., Bindels, L. B., Bubnov, R., Ganguli, K., et al. (2017). How Do Probiotics and Prebiotics Function at Distant Sites?. Benef. Microbes 8, 521–533. doi:10.3920/BM2016.0222
Rolny, I. S., Tiscornia, I., Racedo, S. M., Pérez, P. F., and Bollati-Fogolín, M. (2016). Lactobacillus delbrueckii subsp lactis CIDCA 133 Modulates Response of Human Epithelial and Dendritic Cells Infected with Bacillus cereus. Benef. Microbes 7, 749–760. doi:10.3920/BM2015.0191
Sachdeva, G., Kumar, K., Jain, P., and Ramachandran, S. (2005). SPAAN: a Software Program for Prediction of Adhesins and Adhesin-like Proteins Using Neural Networks. Bioinformatics 21, 483–491. doi:10.1093/bioinformatics/bti028
Salvetti, E., Torriani, S., and Felis, G. E. (2012). The Genus Lactobacillus: A Taxonomic Update. Probiotics Antimicrob. Proteins 4, 217–226. doi:10.1007/s12602-012-9117-8
Salvetti, E., and O'Toole, P. W. (2018). The Genomic Basis of Lactobacilli as Health-Promoting Organisms. Microbiol. Spectr. 5, 49–71. doi:10.1128/microbiolspec.BAD-0011-201610.1128/9781555819705.ch2
Sang, L. X., Chang, B., Dai, C., Gao, N., Liu, W. X., and Jiang, M. (2013). Heat-killed VSL#3 Ameliorates Dextran Sulfate Sodium (DSS)-Induced Acute Experimental Colitis in Rats. Int. J. Mol. Sci. 15, 15–28. doi:10.3390/ijms15010015
Santos Rocha, C., Gomes-Santos, A. C., Garcias Moreira, T., de Azevedo, M., Diniz Luerce, T., Mariadassou, M., et al. (2014). Local and Systemic Immune Mechanisms Underlying the Anti-colitis Effects of the Dairy Bacterium Lactobacillus delbrueckii. PLoS One 9, e85923. doi:10.1371/journal.pone.0085923
Santos Rocha, C., Lakhdari, O., Blottière, H. M., Blugeon, S., Sokol, H., Bermúdez-Humarán, L. G., et al. (2012). Anti-inflammatory Properties of Dairy Lactobacilli. Inflamm. Bowel Dis. 18, 657–666. doi:10.1002/ibd.21834
Savino, F., Cordisco, L., Tarasco, V., Locatelli, E., Di Gioia, D., Oggero, R., et al. (2011). Antagonistic Effect of Lactobacillus Strains against Gas-Producing Coliforms Isolated from Colicky Infants. BMC Microbiol. 11, 157. doi:10.1186/1471-2180-11-157
Seemann, T. (2014). Prokka: Rapid Prokaryotic Genome Annotation. Bioinformatics 30, 2068–2069. doi:10.1093/bioinformatics/btu153
Shannon, P., Markiel, A., Ozier, O., Baliga, N. S., Wang, J. T., Ramage, D., et al. (2003). Cytoscape: A Software Environment for Integrated Models of Biomolecular Interaction Networks. Genome Res. 13, 2498–2504. doi:10.1101/gr.1239303
Simão, F. A., Waterhouse, R. M., Ioannidis, P., Kriventseva, E. V., and Zdobnov, E. M. (2015). BUSCO: Assessing Genome Assembly and Annotation Completeness with Single-Copy Orthologs. Bioinformatics 31, 3210–3212. doi:10.1093/bioinformatics/btv351
Soares, S. C., Silva, A., Trost, E., Blom, J., Ramos, R., Carneiro, A., et al. (2013). The Pan-Genome of the Animal Pathogen Corynebacterium pseudotuberculosis Reveals Differences in Genome Plasticity between the Biovar ovis and Equi Strains. PLoS One 8, e53818. doi:10.1371/journal.pone.0053818
Sun, Z., Harris, H. M., McCann, A., Guo, C., Argimón, S., Zhang, W., et al. (2015). Expanding the Biotechnology Potential of Lactobacilli through Comparative Genomics of 213 Strains and Associated Genera. Nat. Commun. 6, 8322. doi:10.1038/ncomms9322
Teame, T., Wang, A., Xie, M., Zhang, Z., Yang, Y., Ding, Q., et al. (2020). Paraprobiotics and Postbiotics of Probiotic Lactobacilli, Their Positive Effects on the Host and Action Mechanisms: A Review. Front. Nutr. 7, 570344. doi:10.3389/fnut.2020.570344
Trindade, L. M., Torres, L., Matos, I. D., Miranda, V. C., de Jesus, L. C. L., Cavalcante, G., et al. (2021). Paraprobiotic Lacticaseibacillus rhamnosus Protects Intestinal Damage in an Experimental Murine Model of Mucositis. Probiotics Antimicro. Prot. doi:10.1007/s12602-021-09842-z
Usui, Y., Kimura, Y., Satoh, T., Takemura, N., Ouchi, Y., Ohmiya, H., et al. (2018). Effects of Long-Term Intake of a Yogurt Fermented with Lactobacillus delbrueckii subsp. bulgaricus 2038 and Streptococcus Thermophilus 1131 on Mice. Int. Immunol. 30, 319–331. doi:10.1093/intimm/dxy035
van Heel, A. J., de Jong, A., Song, C., Viel, J. H., Kok, J., and Kuipers, O. P. (2018). BAGEL4: a User-Friendly Web Server to Thoroughly Mine RiPPs and Bacteriocins. Nucleic Acids Res. 46, W278–W281. doi:10.1093/nar/gky383
Ventura, M., Turroni, F., and van Sinderen, D. (2012). Probiogenomics as a Tool to Obtain Genetic Insights into Adaptation of Probiotic Bacteria to the Human Gut. Bioeng. Bugs 3, 73–79. doi:10.4161/bbug.18540
Villegas, J. M., Brown, L., Savoy de Giori, G., and Hebert, E. M. (2015). Characterization of the Mature Cell Surface Proteinase of Lactobacillus delbrueckii subsp. lactis CRL 581. Appl. Microbiol. Biotechnol. 99, 4277–4286. doi:10.1007/s00253-014-6258-6
Wan, Y., Xin, Y., Zhang, C., Wu, D., Ding, D., Tang, L., et al. (2014). Fermentation Supernatants of Lactobacillus delbrueckii Inhibit Growth of Human Colon Cancer Cells and Induce Apoptosis through a Caspase 3-dependent Pathway. Oncol. Lett. 7, 1738–1742. doi:10.3892/ol.2014.1959
Wanna, W., Surachat, K., Kaitimonchai, P., and Phongdara, A. (2021). Evaluation of Probiotic Characteristics and Whole Genome Analysis of Pediococcus pentosaceus MR001 for Use as Probiotic Bacteria in Shrimp Aquaculture. Sci. Rep. 11, 18334. doi:10.1038/s41598-021-96780-z
Ye, H., Zhang, X., Jiang, Y., Guo, M., Liu, X., Zhao, J., et al. (2021). Comparative Peptidomics Analysis of Fermented Milk by Lactobacillus delbrueckii ssp. bulgaricus and Lactobacillus delbrueckii ssp. lactis. Foods 10, 3028. doi:10.3390/foods10123028
Zhang, W., Ji, H., Zhang, D., Liu, H., Wang, S., Wang, J., et al. (2018). Complete Genome Sequencing of Lactobacillus plantarum ZLP001, a Potential Probiotic that Enhances Intestinal Epithelial Barrier Function and Defense against Pathogens in Pigs. Front. Physiol. 9, 1689. doi:10.3389/fphys.2018.01689
Zheng, J., Wittouck, S., Salvetti, E., Franz, C. M. A. P., Harris, H. M. B., Mattarelli, P., et al. (2020). A Taxonomic Note on the Genus Lactobacillus: Description of 23 Novel Genera, Emended Description of the Genus Lactobacillus Beijerinck 1901, and Union of Lactobacillaceae and Leuconostocaceae. Int. J. Syst. Evol. Microbiol. 70, 2782–2858. doi:10.1099/ijsem.0.004107
Keywords: comparative genomics, core genome, probiogenomics, GIT stress response, bacteriocins, immunoregulatory proteins
Citation: De Jesus LCL, Aburjaile FF, Sousa TDJ, Felice AG, Soares SDC, Alcantara LCJ and Azevedo VADC (2022) Genomic Characterization of Lactobacillus delbrueckii Strains with Probiotics Properties. Front. Bioinform. 2:912795. doi: 10.3389/fbinf.2022.912795
Received: 04 April 2022; Accepted: 16 May 2022;
Published: 06 June 2022.
Edited by:
Richard Allen White III, University of North Carolina at Charlotte, United StatesReviewed by:
Rostyslav V. Bubnov, National Academy of Sciences of Ukraine, UkraineCopyright © 2022 De Jesus, Aburjaile, Sousa, Felice, Soares, Alcantara and Azevedo. This is an open-access article distributed under the terms of the Creative Commons Attribution License (CC BY). The use, distribution or reproduction in other forums is permitted, provided the original author(s) and the copyright owner(s) are credited and that the original publication in this journal is cited, in accordance with accepted academic practice. No use, distribution or reproduction is permitted which does not comply with these terms.
*Correspondence: Luiz Carlos Junior Alcantara, bHVpei5hbGNhbnRhcmFAaW9jLmZpb2NydXouYnI=; Vasco Ariston De Carvalho Azevedo, dmFzY29AaWNiLnVmbWcuYnI=
Disclaimer: All claims expressed in this article are solely those of the authors and do not necessarily represent those of their affiliated organizations, or those of the publisher, the editors and the reviewers. Any product that may be evaluated in this article or claim that may be made by its manufacturer is not guaranteed or endorsed by the publisher.
Research integrity at Frontiers
Learn more about the work of our research integrity team to safeguard the quality of each article we publish.