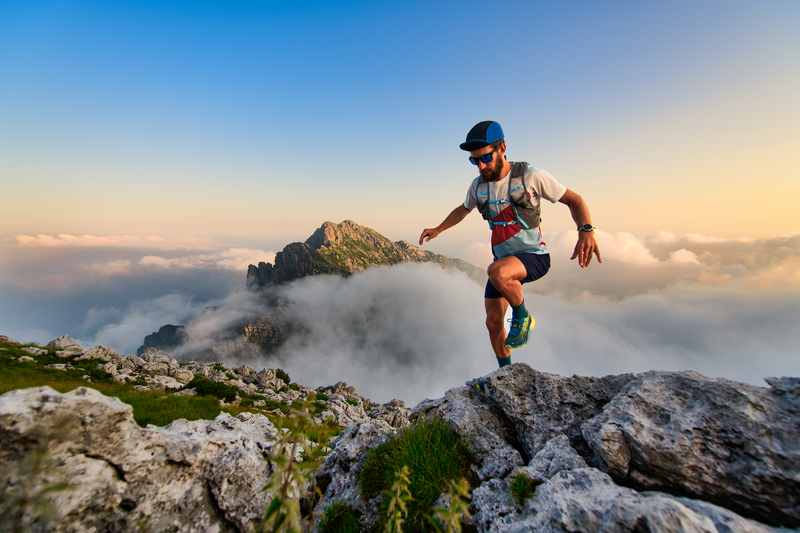
95% of researchers rate our articles as excellent or good
Learn more about the work of our research integrity team to safeguard the quality of each article we publish.
Find out more
PERSPECTIVE article
Front. Batter. Electrochem. , 05 April 2024
Sec. Next Generation Batteries and Technologies
Volume 3 - 2024 | https://doi.org/10.3389/fbael.2024.1377192
The rapid advancement of technology and the growing need for energy storage solutions have led to unprecedented research in the field of metal-ion batteries. This perspective article provides a detailed exploration of the latest developments and future directions in energy storage, particularly focusing on the promising alternatives to traditional lithium-ion batteries. With solid-state batteries, lithium-sulfur systems and other metal-ion (sodium, potassium, magnesium and calcium) batteries together with innovative chemistries, it is important to investigate these alternatives as we approach a new era in battery technology. The article examines recent breakthroughs, identifies underlying challenges, and discusses the significant impact of these new frontiers on various applications–from portable electronics to electric vehicles and grid-scale energy storage. Against the backdrop of a shifting paradigm in energy storage, where the limitations of conventional lithium-ion batteries are being addressed by cutting-edge innovations, this exploration offers insights into the transformative potential of next-generation battery technologies. The article further aims to contribute to the ongoing scientific dialogue by focusing on the environmental and economic implications of these technologies.
Lithium-ion batteries (LIBs) have been at the forefront of portable electronic devices and electric vehicles for decades, driving technological advancements that have shaped the modern era (Weiss et al., 2021). Undoubtedly, LIBs are the workhorse of energy storage, offering a delicate balance of energy density, rechargeability, and longevity (Xiang et al., 2022). They are utilized in various electronic devices, such as smartphones and electric cars, and have become a fundamental component of modern portable power. However, as devices become more advanced, and electrification of transportation accelerates, some challenges still exist. Resource scarcity, safety risks of liquid electrolytes, and theoretical limitations of lithium-ion chemistry are areas of concern (Song et al., 2023). Researchers are exploring alternative materials (Peng et al., 2016), solid-state electrolytes (Bates et al., 2022), and new chemistries/technologies, such as lithium-sulfur (Guo et al., 2024) and lithium-air batteries (Bai et al., 2023), to overcome these challenges and develop the next frontier in energy storage.
The world is shifting towards renewable energy at a fast pace, and the demand for clean energy solutions is increasing globally. This has made it imperative to innovate battery technology (Chen et al., 2012). In particular, solid-state batteries have the potential to improve safety and energy density and could revolutionize energy storage paradigms (Miyazaki, 2020). Additionally, lithium-sulfur chemistry boasts a theoretical energy density that exceeds that of conventional lithium-ion batteries, providing a glimpse into a future where energy storage is not limited by the past (Wang et al., 2023). Other alternative chemistries involving sodium, potassium, magnesium and calcium offer sustainable and scalable energy storage solutions (Zhang et al., 2021; Liu M. et al., 2022). These emerging frontiers in battery technology hold great promise for overcoming the limitations of conventional lithium-ion batteries.
To effectively explore the latest developments in battery technology, it is important to first understand the complex landscape that researchers and engineers are dealing with. The pursuit of these emerging technologies requires a comprehensive approach, taking into account not just the technical details but also the economic, environmental and societal impact. As the world faces the challenges of climate change and pursues decarbonization of various industries, the significance of advanced batteries has become increasingly apparent (Davis et al., 2018).
It is important to carefully consider both the advantages and drawbacks of emerging technologies when navigating this field. This requires a comprehensive evaluation that looks beyond laboratory advancements and considers their practical applications. This article aims to provide guidance for researchers, policymakers, and industry stakeholders by discussing the latest developments, challenges, and potential of next-generation battery technologies. Specifically, it will explore solid-state batteries, lithium-sulfur chemistry, and alternative chemistries beyond lithium. By delving into each of these areas, this article hopes to contribute to the ongoing conversation in the scientific community and offer a roadmap for the future of energy storage.
Solid-state batteries (Figure 1A) are a new type of battery technology that aims to overcome the safety concerns associated with traditional batteries that use liquid electrolytes (Janek and Zeier, 2023). They offer higher energy density, which is a significant advantage. The recent advancements in solid electrolytes, interface engineering, and the integration of solid-state technology into practical applications make them crucial candidates for next-generation energy storage (Aziam et al., 2022). However, they face significant challenges such as manufacturing scalability, cost-effectiveness, and long-term stability that need to be addressed (Ke et al., 2020). As the demand for advanced energy storage solutions continues to increase, solid-state batteries are becoming an increasingly important area of research. The “Solid-State Revolution” presents a groundbreaking frontier that is well-positioned to tackle the significant limitations associated with conventional lithium-ion batteries (He et al., 2021).
Figure 1. Schematic representation (A) comparing conventional lithium-ion battery and its solid-state counterpart, and (B) the various interfaces of solid-state lithium-ion battery. (C) A plot comparing the inonic conductivity vs operational potential window of different solid-polymer (PEO-Polyethylene oxide; PEEC- poly (ethylene ether carbonate); PFEC- poly (fluoroethylene carbonate))/inorganic (LI/NASICON- Li/Na2+2xZn1−xGeO4; LLZO- Li7La3Zr2O12; LLTO- Li0.5La0.5TiO3) electrolytes with the conventional liquid electrolyte (LiPF6, EC:DMC).
Solid-state batteries are a game-changer in the world of energy storage, offering enhanced safety, energy density, and overall performance when compared to traditional lithium-ion batteries (Liu C. et al., 2022). The latter uses a liquid electrolyte to facilitate ion movement between the positive and negative electrodes during charge and discharge cycles. Although effective, this design poses safety risks such as leakage, thermal runaway, and flammability (Feng et al., 2020). This has been observed in high-profile incidents involving lithium-ion batteries (McKerracher et al., 2021). The solid-state battery design seeks to eliminate these risks by replacing the liquid electrolyte with a solid electrolyte, resulting in a more stable and secure energy storage solution (Figure 1A).
Developing solid-state batteries (Figure 1B) has been a major challenge, but recent advancements in materials science have allowed the attainment of solid electrolytes with enhanced conductivity (Figure 1C), making solid-state battery technology practically feasible (Shi et al., 2023). Solid ceramic electrolytes, polymer electrolytes, and composite electrolyte materials have emerged as frontrunners in the quest for suitable solid electrolytes (Figure 1C) that can match the ionic conductivity of their liquid counterparts (Lee et al., 2022; Li et al., 2023a). With these exceptional advancements, researchers are now more confident in their ability to create solid-state batteries that can revolutionize energy storage technology.
The shift towards solid-state batteries brings about significant improvements in terms of safety. By eliminating flammable liquid electrolytes, solid-state batteries reduce the risk of thermal runaway, making them inherently safer for applications that prioritize safety, such as electric vehicles. Additionally, solid electrolytes are more robust, contributing to longer battery life and addressing concerns about degradation and capacity fade often encountered in traditional lithium-ion batteries over time (Che et al., 2023). Apart from being safer, solid-state batteries also have the potential for significantly higher energy density. The inherent properties of solid electrolytes allow for the utilization of high-capacity materials without compromising safety or stability, resulting in batteries that can store more energy within a given volume or weight. This is especially important in applications that require maximized energy density, such as electric vehicles looking for extended ranges between charges (Xu et al., 2022).
Despite the promising potential of solid-state batteries, there are still challenges that need to be overcome in order for them to be widely adopted. The manufacturing of scalable solid-state batteries at a competitive cost is an obstacle that researchers and engineers are actively addressing. Another challenge is related to the interface between solid electrolytes and electrode materials (Figure 1B), as well as the mechanical stresses that occur during charge-discharge cycles. Ongoing investigations are focused on these areas (Stallard et al., 2022). Despite these challenges, the “Solid-State Revolution” is making its way into various industries. Electric vehicle manufacturers are investing in solid-state battery technology to improve safety and range. In addition, portable electronics, medical devices, and aerospace applications are exploring the potential benefits of solid-state batteries. As research progresses, the possibilities of large-scale applications, including grid-scale energy storage, are becoming more achievable.
Lithium-sulfur batteries (Figure 2), like solid-state batteries, are poised to overcome the limitations of traditional lithium-ion batteries (Wang et al., 2023). These batteries offer a high theoretical energy density and have the potential to revolutionize energy storage technologies (Wang et al., 2022). Recent developments have successfully stabilized the sulfur cathode, improved cycle life, resolved issues related to capacity fade, and ensured practical applications and scalability (Zhou et al., 2022; Bi et al., 2023). This breakthrough marks a significant upgrade in energy storage that surpasses the limitations of traditional lithium-ion systems.
Unlike traditional lithium-ion batteries that rely on intercalation chemistry, lithium-sulfur batteries operate on a fundamentally different principle (Deng et al., 2022). In Li-S chemistry, lithium metal generates multiple lithium-sulfur species, known as lithium polysulfides, during electrochemical reactions. These species move back and forth between a lithium metal (negative) and a sulfur (positive) electrodes (Song et al., 2024). The use of sulfur, an abundant and cost-effective element, is the key to achieving energy densities higher than those of lithium-ion batteries. Lithium-sulfur batteries have a remarkable theoretical energy density compared to traditional lithium-ion batteries, which typically have energy densities in the range of 150–250 Wh/kg. They have the potential to exceed 500 Wh/kg and can even approach 1,000 Wh/kg in theory (Zhou et al., 2022). This inherent high energy density positions Li-S batteries as attractive candidates for weight and volume-sensitive applications, such as electric aviation and, portable electronics.
The lithium-sulfur chemistry shows immense potential, but its practical realization faces significant challenges. One major obstacle is the notorious issue of polysulfide dissolution. During discharge-charge cycles, sulfur tends to dissolve into intermediate polysulfide species (Figure 2), leading to the “shuttle effect.” It causes the loss of active material, reduced cycle life, and overall degradation of performance (Wang et al., 2023). However, researchers are actively pursuing strategies to mitigate the challenges posed by polysulfide dissolution.
Recent advancements in battery technology have demonstrated significant progress in stabilizing the sulfur cathode. Nanoengineering approaches, which incorporate conductive carbon materials and porous structures, have proven to be highly effective in confining sulfur and mitigating the shuttle effect (Wang et al., 2015; Lakshmi et al., 2022). These approaches have been demonstrated to be effective in recent breakthroughs (Zhou et al., 2022). Furthermore, advances in electrolyte chemistry, such as the use of high-concentration electrolytes, functional additives, and protective coatings (solid-electrolyte interface) have been shown to successfully suppress polysulfide dissolution, resulting in enhanced overall electrochemical performance of Li-S batteries. These promising developments indicate a bright future for the field of battery technology.
Despite facing challenges, lithium-sulfur batteries have been attracting attention across various industries (Liu et al., 2018). Electric vehicle manufacturers have recognized the potential of these batteries to significantly extend the range of electric cars, thus addressing a key limitation of the current lithium-ion technology. In addition, portable electronics, where lightweight and compact energy storage is crucial, are exploring the feasibility of lithium-sulfur batteries. The appeal of this technology is not just limited to incremental improvements, but it represents a potential paradigm shift in energy storage. Although there are challenges to overcome and optimization is needed for Li-S battery implementation, the technology holds the promise of reshaping energy storage landscapes.
Looking towards the future, ongoing research initiatives are crucial to unlocking the full potential of lithium-sulfur batteries. To achieve this, strategies to stabilize the sulfur cathode, innovative approaches to address polysulfide dissolution, and exploration of new materials and electrode architectures must be considered (Wang et al., 2022). Collaborative efforts between academia and industry will play a crucial role in accelerating the development and commercialization of lithium-sulfur batteries. As researchers continue to explore new possibilities, lithium-sulfur batteries hold the potential to become the most promising solution for high energy density and sustainable energy storage applications.
Researchers are currently investigating alternative materials and chemistries for batteries, such as sodium- (Liu M. et al., 2022), potassium- (Yuan et al., 2021), magnesium- (Li et al., 2023b) and calcium-ion (Gummow et al., 2018) batteries, aiming to develop next-generation energy storage solutions. These alternatives are being evaluated for their potential to offer sustainable and readily available energy storage options, considering factors such as performance, cost, and scalability. With the increasing global demand for energy storage solutions, there is a growing focus on finding alternatives to traditional lithium-ion batteries (Gao et al., 2022). While lithium-ion batteries are widely used, concerns about the availability of lithium resources and limitations in energy density have prompted efforts to diversify battery technologies.
Sodium- and potassium-ion batteries (Chen et al., 2019) offer significant advantages over traditional lithium-ion batteries, including their abundance, cost-effectiveness, and potential for higher energy density. While lithium is limited in availability and concentrated regionally, sodium and potassium are plentiful and widely dispersed globally, ensuring a more stable supply chain. Moreover, the lower cost of sodium and potassium resources makes them economically appealing for large-scale energy storage applications (Song et al., 2021). Research indicates that sodium and potassium batteries could achieve comparable or higher energy densities than lithium-ion batteries, particularly with advancements in electrode materials and electrolyte chemistry (Yin et al., 2021). For instance, recent studies have demonstrated significant progress in sodium-ion battery technology through the development of high-performance electrode materials, which could lead to enhanced energy storage capabilities.
Furthermore, sodium and potassium batteries demonstrate improved safety and stability in comparison to lithium-ion counterparts. The larger size of sodium and potassium ions helps to minimize dendrite formation, which is a common issue leading to short circuits and battery failures in lithium-ion systems. This reduced risk of dendrite formation contributes to the durability and dependability of sodium- and potassium-ion batteries, rendering them safer choices for widespread utilization. Recent advancements also highlight the superior stability of potassium-ion batteries, suggesting their potential for prolonged use in demanding applications. Overall, the abundance, cost-effectiveness, and enhanced safety profile of sodium- and potassium-ion batteries position them as promising alternatives to lithium-ion batteries for the next-generation of energy storage technologies.
Magnesium- (Li et al., 2023b) and calcium- (Gummow et al., 2018) ion batteries present clear advantages over lithium-based counterparts. Firstly, magnesium and calcium are more abundant and widely distributed in the Earth’s crust, alleviating concerns about resource availability. Additionally, magnesium- and calcium-ions carry multiple positive charges, potentially yielding higher energy densities compared to lithium-, sodium-, and potassium-ions, thus enhancing energy storage capacity. Moreover, these batteries exhibit potential for improved safety through the formation of more stable solid-electrolyte interphase (SEI) layers on electrode surfaces, reducing the risks of dendrite formation and thermal runaway. Furthermore, they can operate at higher voltages, facilitating efficient device power while ensuring stability and longevity.
Choosing among sodium-, potassium-, magnesium-, and calcium-ions, and other potential alternatives involves balancing factors like material abundance, cost-effectiveness, and electrochemical properties. Sodium-ion batteries could work well for grid-scale energy storage. Additionally, potassium-ion batteries might be a good fit for applications requiring both high energy density and cost-effectiveness. Conversely, magnesium- and calcium-ion batteries, known for their stability, could be ideal for safety-critical applications.
Exploring alternative chemistries ‘Beyond Lithium’ presents various challenges, encompassing technical hurdles such as ion mobility, electrode materials, and manufacturing scalability (Zhang et al., 2021). These challenges are actively addressed by the research community, leveraging advancements in materials science, nanotechnology, and computational modeling to understand the complexities of alternative battery chemistries. The successful integration of alternative battery chemistries into real-world applications, spanning from portable electronics to electric vehicles and grid-scale energy storage, is paramount. Therefore, research initiatives aimed at bridging the gap between laboratory-scale breakthroughs and practical, scalable implementations play a crucial role in facilitating the successful adoption of alternative battery technologies (Gao et al., 2022).
The growing interest in sustainable energy has created a need for advanced batteries that can contribute to grid stability, peak shaving, and overall efficiency (Larcher and Tarascon, 2015; Newton et al., 2021). Practical scenarios and real-world examples have demonstrated how improved energy storage technology can boost the use of renewable energy. Integrating renewable energy into the power grid is critical as we shift towards a sustainable future. However, the intermittent nature of renewable energy poses significant challenges. This is where next-generation battery technologies become indispensable in addressing these challenges and highlighting the transformative potential of advanced energy storage solutions. Prioritizing the development and implementation of advanced battery technologies is essential to ensure a seamless integration of renewable energy sources into the power grid (Hakimi and Moghaddas-Tafreshi, 2012).
Renewable energy sources like wind and solar power have the potential to provide reliable and eco-friendly energy. However, their intermittent nature poses a significant challenge to the stability of power grids, which creates a mismatch between the timing of renewable energy generation and the demand for electricity. Therefore, it is essential to store the excess energy during periods of abundance and release it when needed. This approach is critical to unlocking the full potential of renewable energy.
The limitations of traditional energy storage solutions, such as pumped hydroelectric storage, due to geographic constraints, are widely recognized. Additionally, these solutions often lack the necessary agility and scalability to address the dynamic nature of renewable energy. However, emerging batteries, with their advanced capabilities such as higher energy density, faster response times, and improved cycle life, are indisputably considered to be crucial components for effectively integrating renewable energy into the grid.
One of the main functions of next-generation batteries is to mitigate the variability of renewable energy generation, especially in the context of ‘Integrating Renewables’ (Shahnazian et al., 2018). They are designed to store excess energy during periods of high renewable output and release it during periods of low or no output, ensuring a stable and dependable power supply. This capability is particularly important in situations where a significant portion of the energy mix is derived from intermittent renewable sources. Advanced batteries not only address intermittency, but also contribute to grid stability and resilience by quickly responding to fluctuations in energy demand and supply, which helps maintain a balance between generation and consumption.
Renewable energy sources are not limited to traditional grid systems. They also include distributed energy resources like residential solar panels and wind turbines. Next-generation batteries play a crucial role in enabling these resources by allowing households and businesses to store excess energy locally. This reduces their reliance on the central power grid, creating a more decentralized and resilient energy infrastructure. In addition, they make it possible to create microgrids, which are smaller localized grids that can function independently or in conjunction with the primary grid. This technology can improve energy security in remote or off-grid areas.
The transportation industry heavily relies on next-generation battery technologies, especially in electric vehicles (EVs). These batteries not only enable clean and sustainable mobility, but they also function as mobile energy storage units. When parked, they can contribute excess energy back to the grid. As a result, they are becoming an indispensable component of the renewable energy landscape.
Although the integration of renewable energy with next-generation batteries has many potential benefits, it is essential to recognize the obstacles that currently exist (Benavides et al., 2022). Advancements in technology are imperative to enhance the capabilities, productivity, and durability of these batteries. Additionally, economic considerations such as production expenses and scalability will significantly impact the broad acceptance of these innovations.
The integration of renewable energy sources into the energy system is interconnected with the development and implementation of advanced energy storage solutions. The potential of next-generation batteries not only lies in electricity storage, but it also involves transforming the entire energy infrastructure. This transformation can make it more sustainable, resilient, and flexible to adapt to the challenges of a dynamic and renewable-based future. The collaboration between renewable energy and advanced energy storage is considered to be a key factor in creating a cleaner and more sustainable energy future.
The development of advanced battery technologies is gaining momentum, and it is vital to examine both their technical capabilities and their broader effects on the environment and the economy. (Blecua de Pedro et al., 2023). The environmental and economic implications of new developments in energy storage include their effect on sustainability, resource usage, and economic viability (Harper et al., 2023). The environmental concerns start with the materials used in these batteries (Wentker et al., 2019). Traditional lithium-ion batteries have been criticized for their use of lithium, cobalt, and nickel, which require significant mining and processing (Llamas-Orozco et al., 2023). However, new battery technologies that use sodium, potassium, magnesium and calcium may offer more sustainable alternatives that are more abundant and widely distributed. Additionally, advancements in sustainable electrode materials and recycling technologies may help reduce the environmental impact of battery production and disposal (Gonzales-Calienes et al., 2023).
In order to assess the environmental impact of batteries, it is important to consider their entire life cycle - from the extraction of raw materials to their proper disposal when they are no longer useable. Life cycle assessments (LCAs) provide a thorough understanding of the environmental effects of batteries and help to ensure that they align with sustainability objectives (Popien et al., 2023). This assessment is essential in making decisions about materials selection, manufacturing processes, and recycling strategies. Improving the recyclability of batteries is a key factor in reducing their environmental impact. Although the recycling infrastructure for lithium-ion batteries has improved significantly, it is imperative that the next-generation batteries focus on achieving even better recyclability, in order to establish a circular economy. This will help in the efficient recovery and reuse of materials, minimize waste, and reduce dependence on finite resources.
It is crucial to consider the energy and carbon footprint of battery production and operation. Manufacturing batteries is an energy-intensive process, and energy losses occur during charging and discharging cycles. Therefore, optimizing energy efficiency is essential. To reduce the carbon footprint, it is necessary to use renewable energy sources for manufacturing and charging batteries, as well as making improvements in battery efficiency. While environmental concerns are crucial, economic feasibility is also vital for widespread adoption. The cost of manufacturing, scalability of production, and overall affordability are essential factors in determining economic viability. To make energy storage more affordable, it is necessary to make advancements in manufacturing processes, achieve economies of scale, and establish supportive regulatory frameworks.
The economic implications of next-generation batteries go beyond just the cost of the batteries themselves. These batteries have the potential to transform energy markets and industries by improving grid stability, enabling peak shaving, and promoting efficient use of renewable energy (Harper et al., 2023). As a result, this can bring economic benefits by reducing the need for expensive peaker plants, improving overall grid efficiency, and contributing to a more resilient energy infrastructure. Furthermore, the shift towards these batteries presents opportunities for job creation and fostering economic growth while also promoting a sustainable energy future.
A striking balance between environmental and economic considerations is crucial when approaching the intersection of these two fields (Blecua de Pedro et al., 2023). As we transition to innovative, ‘Beyond Lithium’ batteries, a comprehensive approach that considers both technical capabilities and alignment with environmental sustainability goals and economic feasibility is essential. Effective policies and regulations are crucial for encouraging sustainable practices, circular economies, and responsible manufacturing. These measures will guide the industry towards a future where next-generation batteries can efficiently store energy and contribute to a cleaner, greener, and economically sound energy landscape.
In the pursuit of next-generation battery technologies that go beyond the limitations of lithium-ion, it is important to look into the future and predict the trajectory of these advancements. By doing so, we can grasp the transformational potential these technologies hold for the global energy scenario.
The “Solid-State Revolution” appears to set for significant advancement in near future. Upon overcoming challenges related to manufacturing scalability and cost-effectiveness, solid-state batteries are likely to transition from laboratory breakthroughs to commercial viability. Enhanced safety, higher energy density, and potential for diverse applications make solid-state batteries compelling candidates for powering the next-generation of electric vehicles, portable electronics, and grid-scale energy storage systems. Collaboration across industries along with ongoing research and development efforts will be vital for unlocking the full potential of solid-state battery technology.
With its promise of unprecedented energy density, lithium-sulfur chemistry stands at the threshold of transformative applications. Despite the existing challenges associated with polysulfide dissolution, recent breakthroughs in stabilizing the sulfur cathodes and addressing degradation issues suggest a bright future. As research has focused on refining electrode materials, optimizing electrolyte formulations, and advancing manufacturing processes, lithium-sulfur batteries may become the energy storage solution of choice for applications demanding lightweight, high-energy-density systems. In particular, electric vehicles could undergo a paradigm shift as lithium-sulfur batteries overcome technological barriers and enter the mainstream.
The exploration of alternative chemistries beyond lithium, such as sodium-, potassium-, magnesium- and calcium-ion batteries, presents a wide range of potential avenues. Sodium-ion batteries, due to their abundance and cost-effectiveness, could be utilized for grid-scale energy storage, lessening reliance on scarce lithium resources. Potassium-ion batteries, offering a balance between energy density and cost, may play a crucial role in portable electronics and electric vehicles. Meanwhile, magnesium- and calcium-ion batteries, capitalizing on stability advantages, could have applications in safety-critical scenarios. The future viability of these technologies depends on overcoming their unique challenges, optimizing their performance, and tailoring them to specific use cases.
The combination of renewable energy sources and advanced energy storage is essential for creating a sustainable energy future. As renewable energy becomes more prevalent worldwide, next-generation batteries play a crucial role in maintaining grid stability, managing peak energy demand, and enhancing overall energy efficiency. Predictions for the future include widespread adoption of advanced batteries on both large-scale utility systems and smaller distributed networks, leading to a more robust, decentralized, and environmentally friendly energy infrastructure. This integration of renewables with energy storage is anticipated to transform the overall processes of power generation, consumption, and distribution.
Predictions for future advancements in next-generation batteries emphasize the importance of balancing environmental sustainability and economic viability. Progress in materials science, recycling methods, and manufacturing techniques is anticipated to decrease the environmental impact of batteries, aligning with global initiatives for a circular economy and sustainable resource management. Additionally, factors such as economies of scale, novel business strategies, and favorable regulations are expected to lower the cost of next-generation batteries, making them economically feasible. This development is likely to stimulate the growth of a robust industry, generating employment opportunities and contributing to economic prosperity.
In summary, the exploration of ‘Beyond Lithium-ion’ signifies a crucial era in the advancement of energy storage technologies. The combination of solid-state batteries, lithium-sulfur batteries, alternative chemistries, and renewable energy integration holds promise for reshaping energy generation, storage, and utilization. However, there are significant challenges to overcome, necessitating collaborative efforts from researchers, industries, and policymakers. The potential of next-generation batteries extends beyond scientific inquiry; it offers a pathway to a sustainable, efficient, and resilient energy future. As research progresses and innovations materialize, the narrative of ‘Beyond Lithium-ion’ is poised to have a profound and lasting impact on global energy systems for generations to come.
The original contributions presented in the study are included in the article/Supplementary material, further inquiries can be directed to the corresponding authors.
BV: Writing–review and editing, Writing–original draft, Visualization, Validation, Supervision, Conceptualization. KS: Writing–review and editing, Writing–original draft, Visualization, Validation, Supervision, Conceptualization.
The author(s) declare that no financial support was received for the research, authorship, and/or publication of this article.
BV thanks Japan Society for the Promotion of Science for the JSPS Research Fellowship (P21035).
The authors declare that the research was conducted in the absence of any commercial or financial relationships that could be construed as a potential conflict of interest.
All claims expressed in this article are solely those of the authors and do not necessarily represent those of their affiliated organizations, or those of the publisher, the editors and the reviewers. Any product that may be evaluated in this article, or claim that may be made by its manufacturer, is not guaranteed or endorsed by the publisher.
Aziam, H., Larhrib, B., Hakim, C., Sabi, N., Ben Youcef, H., and Saadoune, I. (2022). Solid-state electrolytes for beyond lithium-ion batteries: a review. Renew. Sustain. Energy Rev. 167, 112694. doi:10.1016/j.rser.2022.112694
Bai, T., Li, D., Xiao, S., Ji, F., Zhang, S., Wang, C., et al. (2023). Recent progress on single-atom catalysts for lithium–air battery applications. Energy and Environ. Sci. 16, 1431–1465. doi:10.1039/d2ee02949a
Bates, A. M., Preger, Y., Torres-Castro, L., Harrison, K. L., Harris, S. J., and Hewson, J. (2022). Are solid-state batteries safer than lithium-ion batteries? Joule 6, 742–755. doi:10.1016/j.joule.2022.02.007
Benavides, D., Arévalo, P., Tostado-Véliz, M., Vera, D., Escamez, A., Aguado, J. A., et al. (2022). An experimental study of power smoothing methods to reduce renewable sources fluctuations using supercapacitors and lithium-ion batteries. Batteries 8, 228. doi:10.3390/batteries8110228
Bi, C.-X., Hou, L.-P., Li, Z., Zhao, M., Zhang, X.-Q., Li, B.-Q., et al. (2023). Protecting lithium metal anodes in lithium–sulfur batteries: a review. Energy Mater. Adv. 4, 0010. doi:10.34133/energymatadv.0010
Blecua De Pedro, M., Ponce, C. H., De Meatza, I., Frax, L. M., Peidro, C. S., Boyano, I., et al. (2023). Environmental and economic assessment of a higher energy density and safer operation lithium-ion cell for stationary applications. Sustain. Mater. Technol. 37, e00704. doi:10.1016/j.susmat.2023.e00704
Che, Y., Hu, X., Lin, X., Guo, J., and Teodorescu, R. (2023). Health prognostics for lithium-ion batteries: mechanisms, methods, and prospects. Energy and Environ. Sci. 16, 338–371. doi:10.1039/d2ee03019e
Chen, M., Wang, E., Liu, Q., Guo, X., Chen, W., Chou, S.-L., et al. (2019). Recent progress on iron- and manganese-based anodes for sodium-ion and potassium-ion batteries. Energy Storage Mater. 19, 163–178. doi:10.1016/j.ensm.2019.03.030
Chen, X., Li, C., Grätzel, M., Kostecki, R., and Mao, S. S. (2012). Nanomaterials for renewable energy production and storage. Chem. Soc. Rev. 41, 7909–7937. doi:10.1039/c2cs35230c
Davis, S. J., Lewis, N. S., Shaner, M., Aggarwal, S., Arent, D., Azevedo, I. L., et al. (2018). Net-zero emissions energy systems. Science 360, eaas9793. doi:10.1126/science.aas9793
Deng, R., Wang, M., Yu, H., Luo, S., Li, J., Chu, F., et al. (2022). Recent advances and applications toward emerging lithium–sulfur batteries: working principles and opportunities. ENERGY and Environ. Mater. 5, 777–799. doi:10.1002/eem2.12257
Feng, X., Ren, D., He, X., and Ouyang, M. (2020). Mitigating thermal runaway of lithium-ion batteries. Joule 4, 743–770. doi:10.1016/j.joule.2020.02.010
Gao, Y., Pan, Z., Sun, J., Liu, Z., and Wang, J. (2022). High-energy batteries: beyond lithium-ion and their long road to commercialisation. Nano-Micro Lett. 14, 94. doi:10.1007/s40820-022-00844-2
Gonzales-Calienes, G., Kannangara, M., and Bensebaa, F. (2023). Economic and environmental viability of lithium-ion battery recycling—case study in two Canadian regions with different energy mixes. Batteries 9, 375. doi:10.3390/batteries9070375
Gummow, R. J., Vamvounis, G., Kannan, M. B., and He, Y. (2018). Calcium-ion batteries: current state-of-the-art and future perspectives. Adv. Mater. 30, 1801702. doi:10.1002/adma.201801702
Guo, Y., Niu, Q., Pei, F., Wang, Q., Zhang, Y., Du, L., et al. (2024). Interface engineering toward stable lithium–sulfur batteries. Energy and Environ. Sci. 17, 1330–1367. doi:10.1039/d3ee04183b
Hakimi, S. M., and Moghaddas-Tafreshi, S. M. (2012). Optimization of smart microgrid considering domestic flexible loads. J. Renew. Sustain. Energy 4. doi:10.1063/1.4739301
Harper, G. D. J., Kendrick, E., Anderson, P. A., Mrozik, W., Christensen, P., Lambert, S., et al. (2023). Roadmap for a sustainable circular economy in lithium-ion and future battery technologies. J. Phys. Energy 5, 021501. doi:10.1088/2515-7655/acaa57
He, W., Guo, W., Wu, H., Lin, L., Liu, Q., Han, X., et al. (2021). Challenges and recent advances in high capacity Li-rich cathode materials for high energy density lithium-ion batteries. Adv. Mater. 33, 2005937. doi:10.1002/adma.202005937
Janek, J., and Zeier, W. G. (2023). Challenges in speeding up solid-state battery development. Nat. Energy 8, 230–240. doi:10.1038/s41560-023-01208-9
Ke, X., Wang, Y., Ren, G., and Yuan, C. (2020). Towards rational mechanical design of inorganic solid electrolytes for all-solid-state lithium ion batteries. Energy Storage Mater. 26, 313–324. doi:10.1016/j.ensm.2019.08.029
Lakshmi, K. C. S., Vedhanarayanan, B., Shen, H.-H., and Lin, T.-W. (2022). Encapsulating chalcogens as the rate accelerator into MoS2 with expanded interlayer spacing to boost the capacity and cyclic stability of Li–S batteries. 2D Mater. 9, 034002. doi:10.1088/2053-1583/ac7056
Larcher, D., and Tarascon, J. M. (2015). Towards greener and more sustainable batteries for electrical energy storage. Nat. Chem. 7, 19–29. doi:10.1038/nchem.2085
Lee, M. J., Han, J., Lee, K., Lee, Y. J., Kim, B. G., Jung, K.-N., et al. (2022). Elastomeric electrolytes for high-energy solid-state lithium batteries. Nature 601, 217–222. doi:10.1038/s41586-021-04209-4
Li, Z., Fu, J., Zhou, X., Gui, S., Wei, L., Yang, H., et al. (2023a). Ionic conduction in polymer-based solid electrolytes. Adv. Sci. 10, 2201718. doi:10.1002/advs.202201718
Li, Z., Häcker, J., Fichtner, M., and Zhao-Karger, Z. (2023b). Cathode materials and chemistries for magnesium batteries: challenges and opportunities. Adv. Energy Mater. 13, 2300682. doi:10.1002/aenm.202300682
Liu, B., Fang, R., Xie, D., Zhang, W., Huang, H., Xia, Y., et al. (2018). Revisiting scientific issues for industrial applications of lithium–sulfur batteries. ENERGY and Environ. Mater. 1, 196–208. doi:10.1002/eem2.12021
Liu, C., Sun, J., Zheng, P., Jiang, L., Liu, H., Chai, J., et al. (2022a). Recent advances of non-lithium metal anode materials for solid-state lithium-ion batteries. J. Mater. Chem. A 10, 16761–16778. doi:10.1039/d2ta03905b
Liu, M., Wang, Y., Wu, F., Bai, Y., Li, Y., Gong, Y., et al. (2022b). Advances in carbon materials for sodium and potassium storage. Adv. Funct. Mater. 32, 2203117. doi:10.1002/adfm.202203117
Llamas-Orozco, J. A., Meng, F., Walker, G. S., Abdul-Manan, A. F. N., Maclean, H. L., Posen, I. D., et al. (2023). Estimating the environmental impacts of global lithium-ion battery supply chain: a temporal, geographical, and technological perspective. PNAS Nexus 2, pgad361. doi:10.1093/pnasnexus/pgad361
Mckerracher, R. D., Guzman-Guemez, J., Wills, R. G. A., Sharkh, S. M., and Kramer, D. (2021). Advances in prevention of thermal runaway in lithium-ion batteries. Adv. Energy Sustain. Res. 2, 2000059. doi:10.1002/aesr.202000059
Miyazaki, R. (2020). High-capacity anode materials for all-solid-state lithium batteries. Front. Energy Res. 8. doi:10.3389/fenrg.2020.00171
Newton, G. N., Johnson, L. R., Walsh, D. A., Hwang, B. J., and Han, H. (2021). Sustainability of battery technologies: today and tomorrow. ACS Sustain. Chem. Eng. 9, 6507–6509. doi:10.1021/acssuschemeng.1c02909
Peng, L., Zhu, Y., Chen, D., Ruoff, R. S., and Yu, G. (2016). Two-dimensional materials for beyond-lithium-ion batteries. Adv. Energy Mater. 6, 1600025. doi:10.1002/aenm.201600025
Popien, J.-L., Thies, C., Barke, A., and Spengler, T. S. (2023). Comparative sustainability assessment of lithium-ion, lithium-sulfur, and all-solid-state traction batteries. Int. J. Life Cycle Assess. 28, 462–477. doi:10.1007/s11367-023-02134-4
Shahnazian, F., Adabi, J., Pouresmaeil, E., and Catalão, J. P. S. (2018). Interfacing modular multilevel converters for grid integration of renewable energy sources. Electr. Power Syst. Res. 160, 439–449. doi:10.1016/j.epsr.2018.03.014
Shi, P., Ma, J., Liu, M., Guo, S., Huang, Y., Wang, S., et al. (2023). A dielectric electrolyte composite with high lithium-ion conductivity for high-voltage solid-state lithium metal batteries. Nat. Nanotechnol. 18, 602–610. doi:10.1038/s41565-023-01341-2
Song, K., Liu, C., Mi, L., Chou, S., Chen, W., and Shen, C. (2021). Recent progress on the alloy-based anode for sodium-ion batteries and potassium-ion batteries. Small 17, 1903194. doi:10.1002/smll.201903194
Song, Y., Wang, L., Sheng, L., Ren, D., Liang, H., Li, Y., et al. (2023). The significance of mitigating crosstalk in lithium-ion batteries: a review. Energy and Environ. Sci. 16, 1943–1963. doi:10.1039/d3ee00441d
Song, Z., Jiang, W., Li, B., Qu, Y., Mao, R., Jian, X., et al. (2024). Advanced polymers in cathodes and electrolytes for lithium–sulfur batteries: progress and prospects. Small, 2308550. doi:10.1002/smll.202308550
Stallard, J. C., Wheatcroft, L., Booth, S. G., Boston, R., Corr, S. A., De Volder, M. F. L., et al. (2022). Mechanical properties of cathode materials for lithium-ion batteries. Joule 6, 984–1007. doi:10.1016/j.joule.2022.04.001
Wang, J., He, Y.-S., and Yang, J. (2015). Sulfur-based composite cathode materials for high-energy rechargeable lithium batteries. Adv. Mater. 27, 569–575. doi:10.1002/adma.201402569
Wang, M., Bai, Z., Yang, T., Nie, C., Xu, X., Wang, Y., et al. (2022). Advances in high sulfur loading cathodes for practical lithium-sulfur batteries. Adv. Energy Mater. 12, 2201585. doi:10.1002/aenm.202201585
Wang, T., He, J., Zhu, Z., Cheng, X.-B., Zhu, J., Lu, B., et al. (2023). Heterostructures regulating lithium polysulfides for advanced lithium-sulfur batteries. Adv. Mater. 35, 2303520. doi:10.1002/adma.202303520
Weiss, M., Ruess, R., Kasnatscheew, J., Levartovsky, Y., Levy, N. R., Minnmann, P., et al. (2021). Fast charging of lithium-ion batteries: a review of materials aspects. Adv. Energy Mater. 11, 2101126. doi:10.1002/aenm.202101126
Wentker, M., Greenwood, M., Asaba, M. C., and Leker, J. (2019). A raw material criticality and environmental impact assessment of state-of-the-art and post-lithium-ion cathode technologies. J. Energy Storage 26, 101022. doi:10.1016/j.est.2019.101022
Xiang, J., Wei, Y., Zhong, Y., Yang, Y., Cheng, H., Yuan, L., et al. (2022). Building practical high-voltage cathode materials for lithium-ion batteries. Adv. Mater. 34, 2200912. doi:10.1002/adma.202200912
Xu, G., Luo, L., Liang, J., Zhao, S., Yang, R., Wang, C., et al. (2022). Origin of high electrochemical stability of multi-metal chloride solid electrolytes for high energy all-solid-state lithium-ion batteries. Nano Energy 92, 106674. doi:10.1016/j.nanoen.2021.106674
Yin, H., Han, C., Liu, Q., Wu, F., Zhang, F., and Tang, Y. (2021). Recent advances and perspectives on the polymer electrolytes for sodium/potassium-ion batteries. Small 17, 2006627. doi:10.1002/smll.202006627
Yuan, F., Zhang, W., Zhang, D., Wang, Q., Li, Z., Li, W., et al. (2021). Recent progress in electrochemical performance of binder-free anodes for potassium-ion batteries. Nanoscale 13, 5965–5984. doi:10.1039/d1nr00077b
Zhang, L., Wang, H., Zhang, X., and Tang, Y. (2021). A review of emerging dual-ion batteries: fundamentals and recent advances. Adv. Funct. Mater. 31, 2010958. doi:10.1002/adfm.202010958
Keywords: lithium-ion batteries, solid-state electrolyte, lithium-sulfur electrode, renewable sources, environmental-economic implications
Citation: Vedhanarayanan B and Seetha Lakshmi KC (2024) Beyond lithium-ion: emerging frontiers in next-generation battery technologies. Front. Batteries Electrochem. 3:1377192. doi: 10.3389/fbael.2024.1377192
Received: 26 January 2024; Accepted: 14 March 2024;
Published: 05 April 2024.
Edited by:
Ali Ahmadian, University of Waterloo, CanadaReviewed by:
Lei Zhou, Jiangsu University, ChinaCopyright © 2024 Vedhanarayanan and Seetha Lakshmi. This is an open-access article distributed under the terms of the Creative Commons Attribution License (CC BY). The use, distribution or reproduction in other forums is permitted, provided the original author(s) and the copyright owner(s) are credited and that the original publication in this journal is cited, in accordance with accepted academic practice. No use, distribution or reproduction is permitted which does not comply with these terms.
*Correspondence: Balaraman Vedhanarayanan, YnZlZGhhbmFyYXlhbmFuQGdtYWlsLmNvbQ==; K. C. Seetha Lakshmi, c2VldGhha2NAZ21haWwuY29t
†These authors have contributed equally to this work and share first authorship
Disclaimer: All claims expressed in this article are solely those of the authors and do not necessarily represent those of their affiliated organizations, or those of the publisher, the editors and the reviewers. Any product that may be evaluated in this article or claim that may be made by its manufacturer is not guaranteed or endorsed by the publisher.
Research integrity at Frontiers
Learn more about the work of our research integrity team to safeguard the quality of each article we publish.