- Exponent, Inc., Menlo Park, CA, United States
The field of lithium (Li)-ion batteries has entered a stage where industry is largely focusing on optimizing current cell chemistries to increase the effective energy density of commercial cells while academia is mainly driven by the development of novel materials for next-generation cell chemistries. In addition to their different research goals, industry is producing commercial cells with increasingly diversified size and shape, while the majority of academic research reports on limited small sized cell formats. Such differences in research settings are leading to a disconnect between industrial and academic perspectives. More specifically, a disconnect arises due to academic research results obtained from smaller scale cells not translating to cells with more complex designs. In this paper, we discuss where a disconnect between industry and academia exists, and demonstrate how industry relevant problems can be studied in academia by utilizing a multifaceted approach and further provide valuable insights at a practical level.
1 Introduction
Since their commercialization in 1991, lithium (Li)-ion batteries have become a vital technology in our society enabling breakthroughs in various products including consumer electronics and electric vehicles (Goodenough and Park, 2013; Winter et al., 2018; Li et al., 2020). While the applications and market size of Li-ion batteries have grown significantly over the last three decades, their underlying cell chemistry remains largely unchanged (i.e., the intercalation chemistry based on graphite anodes and transition metal oxide cathodes developed in the 1980s is still being utilized) (Whittingham, 2004; Asenbauer et al., 2020; Reddy et al., 2020). Despite utilization of a similar underlying cell chemistry, industry has continued to increase the effective energy density of commercial cells through various design modifications, such as decreasing component thickness (e.g., separator, current collectors, cell enclosure, etc.). However, these engineering and materials changes can generate new failure mechanisms, such as shorts through separators, deformed or torn current collectors, breached cell enclosures, and more. Thus, cell quality control and field failures are important challenges facing the Li-ion battery industry, and solving such problems involves understanding the complex interplay between chemical and mechanical properties of cell components ranging from the electrode level to the full cell level (Loud and Hu, 2007; Mikolajczak et al., 2012; Cai et al., 2020).
Unfortunately, these industrial problems are often not the focus in academia (Frith et al., 2023; Nature Nanotechnology, 2023) where work is largely driven by chemistry and materials research topics. Taking degradation products at the electrode as an example, the main topic of interest in academia is determining the chemical species and identifying reaction pathways whereas industry is interested in more diverse phenomena such as the physical expansion caused by those products and deformation of the cell structure that can lead to failure. One of the factors contributing to these different interests is the funding sources and their objectives. Funding opportunities in academia generally encourage fundamental research and novel ideas that can pioneer transformational technologies (e.g., next-generation cell chemistries), and hence research topics at a low technology readiness level are preferred at the expense of further understanding current technologies. On the other hand, funding opportunities for applied work often come from companies, which may have restrictions on data publication and materials accessibility. Since sharing new findings is a critical component to academic research, applied research becomes less prioritized in academic settings. However, considering the current status of Li-ion battery technology, efforts should be made to enable research that is commercially relevant.
Studying industry relevant problems does not necessarily require manufacturing or testing industrial sized cells, but rather focusing on the components that become critical in larger cell formats and understanding their fundamental properties. In this paper, we discuss where the gap between academic and industry research on Li-ion batteries lies and how the disconnect can be bridged via a multidisciplinary approach. Then, we present a case study on the degradation of cylindrical Li-ion batteries to demonstrate how fundamental understandings of mechanical and electrochemical behavior of cell components can provide insights on addressing industrial problems.
2 Batteries at different length scales
One of the major differences between academia and industrial research settings is the cell formats used to test battery performance. In academic research, 2032-type coin cells are mainly used since they can be easily fabricated with only a small amount of material and provide a good platform to compare results. However, the results obtained from these coin cells often do not translate to larger cells manufactured in the industry (e.g., cylindrical, prismatic, and pouch cells). One of the main factors causing this discrepancy is the mechanical components in batteries that can have prominent impacts on the performance of larger cells, even if the chemistry remains unchanged. Unlike coin cells which contain two small single-sided circular electrodes pressed against each other at one planar interface, cylindrical cells (such as the commonly used 18650 format) are constructed with two long strips of double-sided electrodes that are tightly wound. Due to the tension and curvature of the electrode winding of cylindrical cells, the mechanical environment of the electrodes is different even if everything else comprising the cell is the same between the two cell formats. Other cell formats will also experience unique mechanical stresses. For example, in wound pouch cells the mechanical stress at the electrode winding bend will differ from the rest of the cell where the electrodes lie flat on top of each other. An additional factor in pouch cells is their soft polymer casing compared to the rigid metal cases of cylindrical or prismatic cells, which affects the pressure applied to the electrode windings.
Now take it one step further and consider cycling of these cells. Both graphite and transition metal oxide active materials experience volume changes during intercalation and de-intercalation of Li+ ions (Schweidler et al., 2018; Spingler et al., 2021) that are extended to the electrode level. The dynamic evolution of mechanical stress on the electrodes during cycling will show different profiles in different cell formats. With the increasing number of custom shaped batteries targeted for various applications, understanding the mechanical aspects of batteries becomes more important. Moreover, this is an extremely relevant topic for next-generation materials research. Silicon (Si), for example, experiences severe volume changes (∼300%) during lithiation and delithiation (Obrovac and Krause, 2007; Chae et al., 2020). At the particle level, this behavior can lead to pulverization resulting in loss of Si active material as well as continuous electrolyte decomposition and loss of Li inventory at the freshly exposed surfaces. At a larger scale, the volume change can lead to deformation of the copper (Cu) current collector (Veith, 2020; Rodrigues et al., 2023). The rippling, or shredding in extreme cases, of the Cu foil observed in coin cells and single layer pouch cells will have a more complex response in larger cell formats.
Broadly speaking, while the mechanical properties of various anodes and cathodes have been well studied in academia (Mukhopadhyay and Sheldon, 2014; Tavassol et al., 2016; Bassett et al., 2019; Yoon et al., 2019; Tanim et al., 2022), the majority of observations are limited to microscopic scale phenomena, such as interfacial and bulk stresses of the active material particles. Electrode-level studies are limited to a relatively small scale (typically around a few square centimeters) due to the sample size constraints of the measurements or difficulties in preparing larger size electrodes with reliable quality.
The question then becomes—how can the results obtained from limited dimensions and fundamental understandings therein be applied to more complex systems? To answer this, researchers should seek to understand how each cell component, including the current collector and separator that are often treated as inert, influences the overall performance of a full cell under different geometries and mechanical constraints. While there are a number of different ways to study this, one approach is to directly evaluate commercial batteries and observe their response to different test conditions. In the next section, we demonstrate how this can be done by presenting a case study on commercial 18650 format batteries.
3 Mechanical and electrochemical interplay in batteries
One of the common failure modes in cylindrical type Li-ion batteries is collapse of the cell core due to the mechanical stress induced by volume changes of the electrodes during cycling. This phenomenon is not present in coin cells, which only have a single planar layer, and as a result is not well studied in academic literature. The few available studies on this phenomenon have shown that core collapse is accelerated when the batteries are cycled at extreme charging conditions (e.g., higher C-rate and low temperature) (Waldmann et al., 2014; Carter et al., 2019), and can occur regardless of the presence of a center tube, which is a hollow metal tube that is inserted at the center of cylindrical cells to allow a path for gas release (Willenberg et al., 2020). These findings align with Exponent’s experience as well. While this previous work very nicely demonstrates how cells respond to the applied test conditions as well as the mechanisms behind the evolution of the cell core collapse, there have been no discussions on a proactive approach to this problem—can the cells be screened in advance to mitigate failures associated with core collapse? Moreover, based on the findings can the cells be designed to avoid this issue?
To determine parameters that can be used to predict core collapse behavior, we evaluated commercially available 18650 batteries (2.5 Ah). 21 cells of the same type were tested to ensure enough data points for observing trends in their degradation mechanisms. All cells were cycled by charging at 4 A to 4.2 V followed by a voltage hold at 4.2 V with a 0.1 A taper current limit and then discharging at 1.5 A to 2.5 V. A 10-min rest was applied between charge and discharge, and the cells were cycled 300 times at 23 °C ± 3 °C. To monitor the structural evolution of the cells, X-ray computed tomography (CT) scans were performed prior to cycling, and after 100, 200, and 300 cycles. Core collapse was then evaluated by performing image analysis to quantify the void area in the core of the windings using two-dimensional (2D) CT cross section images of the center of the cell. In an uncycled cell, the core of the windings initially appears as a dark circular region as shown in the CT image in Figure 1A, and decreases in area upon cycling as collapse of the innermost wraps begins to occur. The “estimated core collapse onset” was determined using a liner interpolation of the void area measured in the CT scans performed every 100 cycles to calculate the approximate cycle when the void area would have decreased by 10% from the original area.
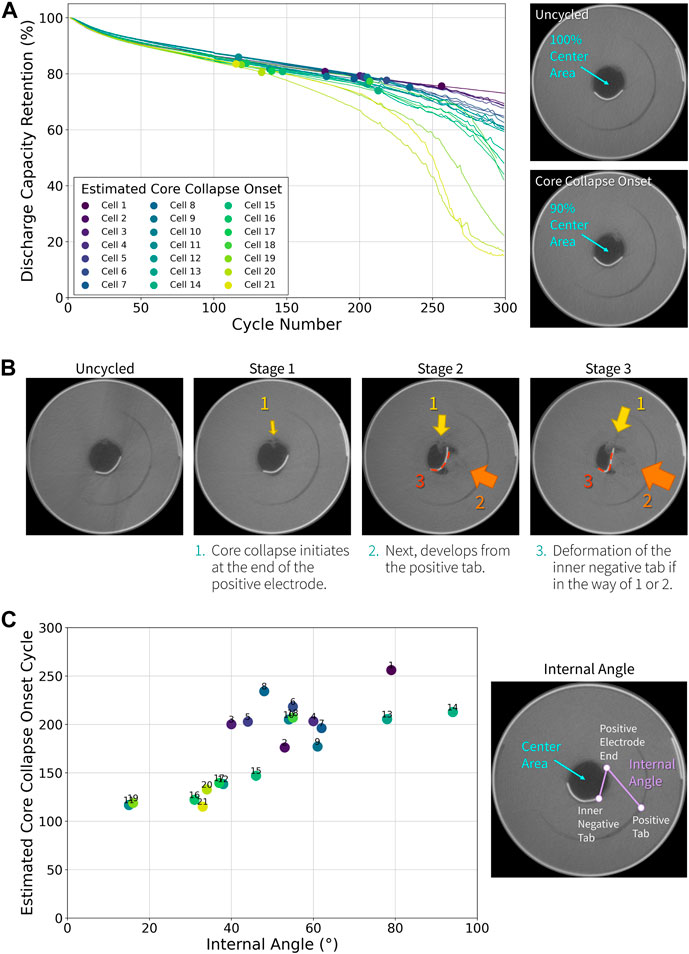
FIGURE 1. Structural evolution and electrochemical performance of 18650-type Li-ion batteries upon cycling. 21 cells were tested under the same condition. (A) Discharge capacity retention plotted versus cycle number. The estimated core collapse onset of each cell (defined as the point where the center area is decreased by 10% of the original area) is indicated with markers. Example CT cross sections of an 18650 cell before cycling and at the core collapse onset are shown on the right. (B) Stages of the core collapse observed from all cells. (C) Estimated core collapse onset cycle plotted versus the angle between the positive electrode end, inner negative tab, and embedded positive tab. The internal angle is described in the CT cross section shown on the right.
Discharge capacity retention of the 21 cells are show in Figure 1A and the estimated core collapse onset of each cell is indicated with markers. A widely varying cycle performance is observed (capacity retention ranging from 73% to 15% after 300 cycles), despite being the same design. This result emphasizes the importance of testing sufficient number of cells to be able to accurately evaluate their performance. Another noticeable trend is that the core collapse begins prior to the rapid decrease in the capacity, which is often referred to as a “knee” in literature (Attia et al., 2022). In most cases, the steady decrease in capacity continues for another 50 to 100 cycles before rapid fade is observed. That core collapse precedes the knee in cycling data could suggest a possible cause and effect, with core collapse accelerating electrical performance degradation; however insufficient data exists to rule out core collapse as an early symptom of degradation. Despite the uncertainty in the sequence of events, this phenomenon clearly demonstrates the interconnected structural deformation and electrochemical degradation within the cell leading to changes in the electrical response.
All cells exhibited essentially the same initial electrical performance, indicating the core collapse onset is not governed by (nor can be predicted from) the initial discharge capacity or cell impedance. While the core collapse onset differed between cells, a similar structural evolution was observed in all cells. Figure 1B demonstrates the three stages of core collapse observed in these cells. In the first stage, the collapse starts at the innermost edge of the positive electrode. In the second stage, another initiating point develops radially aligned with the positive tab, which is embedded in the electrode winding. In the third stage, deformation of the inner negative tab occurs if it is in the way of the progression of the core collapse. From this observation, we see that the core collapse involves three stress concentrators—the positive electrode end, the embedded positive tab, and the inner negative tab.
In an effort to understand the relationship between these three components, the angle between them was measured and defined as the “internal angle” of unaged cells. This angle can be used as a proxy for the internal structure of the cell. Since the dimensions of the electrodes are the same, the variations in the internal angle correspond to variations in winding parameters used during the manufacturing process (i.e., the angle changes if one cell is wound tighter than the other). When the estimated core collapse onset cycle number is plotted as a function of the internal angle, a correlation is observed where an earlier core collapse onset occurs with a narrower internal angle (Figure 1C). While the exact same three stages of structural evolution may not be observed from other cylindrical cells with different designs, the winding parameters will still play a critical role in determining the core collapse behavior. Thus, this study suggests that the mechanical deformations and resulting chemical degradations triggered by the variation in tension can be reduced by implementing stronger controls during the electrode winding of the cell manufacturing process.
4 Discussion
The core collapse study is an excellent example demonstrating how battery research needs a multidisciplinary approach. Core collapse caused by variation in the initial cell structure leading to capacity fade of the cell during cycling is an intertwined problem of mechanical and electrochemical phenomena. While there are manufacturing components associated with this problem to a certain degree, it also raises fundamental questions that can provide valuable insights and guide industry toward advancing cell performance. For example, how does the electrode coating respond to the mechanical stress applied during cycling when changing its composition (i.e., different active material or binder) or when changing its geometry (i.e., on a curved surface versus a planar configuration)? Developing methods to accurately measure such properties and how they change under different conditions (e.g., different temperatures) will have a significant impact at a practical level for designing new cells. The methods used to study this can be either experimental, computational, or a combination of both. Moreover, a more holistic multifaceted approach involving mechanical, thermal, and electrical fields in addition to electrochemical and materials subjects is necessary to understand the complex interactions between the cell components.
While this may seem like a daunting challenge, it is imperative that industry and academia work together as the problems that commercial Li-ion batteries are currently facing are also applicable to next-generation battery chemistries, such as Li-S and Na-ion systems. Emerging chemistries often use similar cell formats and construction methods as existing Li-ion cells, and hence similar issues exist. For example, expansion of electrodes, whether it comes from accumulation of degradation products or expansion of active materials, will result in mechanical deformation leading to cell failure. High energy density materials (e.g., Si and Li metal) tend to have more complex volume changes upon cycling and the failure mechanisms will likely be exacerbated. Lately the Li-ion battery industry has been releasing more information regarding their experience and findings, and although it may not be the most recent technology, academia can leverage the reported results as guidelines for applied research ideas. Considering the practical factors early on in the development stage will benefit the next-generation technologies beyond the proof-of-concept level and provide valuable information to industry for future commercialization efforts.
Obtaining a deeper understanding of mechanisms behind industrial problems by leveraging fundamental research in academia will combine the strength of each field and bridge the gap between academic and industry Li-ion battery research.
Data availability statement
The datasets presented in this article are not readily available because properties of the batteries tested need to remain confidential. Requests to access the datasets should be directed to YH, eWhhQGV4cG9uZW50LmNvbQ==.
Author contributions
YH: Conceptualization, Writing–original draft. RL: Data curation, Formal Analysis, Writing–review and editing. TB: Formal Analysis, Writing–review and editing. KB: Conceptualization, Supervision, Writing–review and editing.
Funding
The author(s) declare financial support was received for the research, authorship, and/or publication of this article. This study received funding from Exponent, Inc. Exponent’s financial support of this work was limited to the purchase of the tested cells and the use of its equipment and labs. Outside of the authors’ employment at Exponent and the resources provided by Exponent, there was no involvement from Exponent in the study design, collection, analysis, interpretation of data, the writing of this article, or the decision to submit it for publication. All authors declare no other competing interests.
Acknowledgments
The authors thank Dr. Adam Cohn and Dr. Colin Burke at Exponent for their help with the core collapse study.
Conflict of interest
YH, RL, TB, and KB were employed by Exponent, Inc.
Publisher’s note
All claims expressed in this article are solely those of the authors and do not necessarily represent those of their affiliated organizations, or those of the publisher, the editors and the reviewers. Any product that may be evaluated in this article, or claim that may be made by its manufacturer, is not guaranteed or endorsed by the publisher.
References
Asenbauer, J., Eisenmann, T., Kuenzel, M., Kazzazi, A., Chen, Z., and Bresser, D. (2020). The success story of graphite as a lithium-ion anode material–fundamentals, remaining challenges, and recent developments including silicon (oxide) composites. Sustain. Energy & Fuels 4 (11), 5387–5416. doi:10.1039/d0se00175a
Attia, P. M., Bills, A., Brosa Planella, F., Dechent, P., Dos Reis, G., Dubarry, M., et al. (2022). Review—“Knees” in lithium-ion battery aging trajectories. J. Electrochem. Soc. 169 (6), 060517. doi:10.1149/1945-7111/ac6d13
Bassett, K. L., Özgür Çapraz, Ö., Özdogru, B., Gewirth, A. A., and Sottos, N. R. (2019). Cathode/electrolyte interface-dependent changes in stress and strain in lithium iron phosphate composite cathodes. J. Electrochem. Soc. 166 (12), A2707–A2714. doi:10.1149/2.1391912jes
Cai, Z., Mendoza, S., Goodman, J., McGann, J., Han, B., Sanchez, H., et al. (2020). The influence of cycling, temperature, and electrode gapping on the safety of prismatic lithium-ion batteries. J. Electrochem. Soc. 167 (16), 160515. doi:10.1149/1945-7111/abcabc
Carter, R., Klein, E. J., Atkinson, R. W., and Love, C. T. (2019). Mechanical collapse as primary degradation mode in mandrel-free 18650 Li-ion cells operated at 0 °C. J. Power Sources 437, 226820. doi:10.1016/j.jpowsour.2019.226820
Chae, S., Choi, S. H., Kim, N., Sung, J., and Cho, J. (2020). Integration of graphite and silicon anodes for the commercialization of high-energy lithium-ion batteries. Angew. Chem. Int. Ed. 59 (1), 110–135. doi:10.1002/anie.201902085
Frith, J. T., Lacey, M. J., and Ulissi, U. (2023). A non-academic perspective on the future of lithium-based batteries. Nat. Commun. 14 (1), 420. doi:10.1038/s41467-023-35933-2
Goodenough, J. B., and Park, K.-S. (2013). The Li-ion rechargeable battery: a perspective. J. Am. Chem. Soc. 135 (4), 1167–1176. doi:10.1021/ja3091438
Li, W., Erickson, E. M., and Manthiram, A. (2020). High-nickel layered oxide cathodes for lithium-based automotive batteries. Nat. Energy 5 (1), 26–34. doi:10.1038/s41560-019-0513-0
Loud, J., and Hu, X. (2007). “Failure analysis methodology for Li-ion incidents,” in ISTFA 2007, San Jose, California, USA, November 4–8, 2007.
Mikolajczak, C., Kahn, M., White, K., and Long, R. T. (2012). Lithium-ion batteries hazard and use assessment. Berlin, Heidelberg: Springer Science & Business Media.
Mukhopadhyay, A., and Sheldon, B. W. (2014). Deformation and stress in electrode materials for Li-ion batteries. Prog. Mater. Sci. 63, 58–116. doi:10.1016/j.pmatsci.2014.02.001
Nature Nanotechnology (2023). Battery researchers in academia and industry should collaborate more effectively. Nat. Nanotechnol. 18. doi:10.1038/s41565-023-01338-x
Obrovac, M., and Krause, L. (2007). Reversible cycling of crystalline silicon powder. J. Electrochem. Soc. 154 (2), A103. doi:10.1149/1.2402112
Reddy, M. V., Mauger, A., Julien, C. M., Paolella, A., and Zaghib, K. (2020). Brief history of early lithium-battery development. Materials 13 (8), 1884. doi:10.3390/ma13081884
Rodrigues, M.-T. F., Rajendran, S., Trask, S. E., Dunlop, A. R., Singh, A., Allen, J. M., et al. (2023). Cell-format-dependent mechanical damage in silicon anodes. ACS Appl. Energy Mater. 18, 9243–9248. doi:10.1021/acsaem.3c01531
Schweidler, S., de Biasi, L., Schiele, A., Hartmann, P., Brezesinski, T., and Janek, J. (2018). Volume changes of graphite anodes revisited: a combined operando X-ray diffraction and in situ pressure analysis study. J. Phys. Chem. C 122 (16), 8829–8835. doi:10.1021/acs.jpcc.8b01873
Spingler, F. B., Kücher, S., Phillips, R., Moyassari, E., and Jossen, A. (2021). Electrochemically stable in situ dilatometry of NMC, NCA and graphite electrodes for lithium-ion cells compared to XRD measurements. J. Electrochem. Soc. 168 (4), 040515. doi:10.1149/1945-7111/abf262
Tanim, T. R., Weddle, P. J., Yang, Z., Colclasure, A. M., Charalambous, H., Finegan, D. P., et al. (2022). Enabling extreme fast-charging: challenges at the cathode and mitigation strategies. Adv. Energy Mater. 12 (46), 2202795. doi:10.1002/aenm.202202795
Tavassol, H., Jones, E. M., Sottos, N. R., and Gewirth, A. A. (2016). Electrochemical stiffness in lithium-ion batteries. Nat. Mater. 15 (11), 1182–1187. doi:10.1038/nmat4708
Veith, G. M. (2020). Silicon consortium project: science of manufacturing for silicon anodes. Washington DC (United States): US DOE Office of Energy Efficiency and Renewable Energy.
Waldmann, T., Gorse, S., Samtleben, T., Schneider, G., Knoblauch, V., and Wohlfahrt-Mehrens, M. (2014). A mechanical aging mechanism in lithium-ion batteries. J. Electrochem. Soc. 161 (10), A1742–A1747. doi:10.1149/2.1001410jes
Whittingham, M. S. (2004). Lithium batteries and cathode materials. Chem. Rev. 104 (10), 4271–4302. doi:10.1021/cr020731c
Willenberg, L., Dechent, P., Fuchs, G., Teuber, M., Eckert, M., Graff, M., et al. (2020). The development of jelly roll deformation in 18650 lithium-ion batteries at low state of charge. J. Electrochem. Soc. 167 (12), 120502. doi:10.1149/1945-7111/aba96d
Winter, M., Barnett, B., and Xu, K. (2018). Before Li ion batteries. Chem. Rev. 118 (23), 11433–11456. doi:10.1021/acs.chemrev.8b00422
Keywords: Li-ion batteries, mechanical, electrochemical, interplay, core collapse
Citation: Ha Y, Licht RB, Bogart TD and Beers KM (2024) Bridging the gap between academic and industry Li-ion battery research. Front. Batteries Electrochem. 2:1287887. doi: 10.3389/fbael.2023.1287887
Received: 02 September 2023; Accepted: 08 December 2023;
Published: 04 January 2024.
Edited by:
Josefine McBrayer, Sandia National Laboratories, United StatesReviewed by:
Marco-Tulio Fonseca Rodrigues, Argonne National Laboratory (DOE), United StatesNoah Schorr, Sandia National Laboratories (DOE), United States
Copyright © 2024 Ha, Licht, Bogart and Beers. This is an open-access article distributed under the terms of the Creative Commons Attribution License (CC BY). The use, distribution or reproduction in other forums is permitted, provided the original author(s) and the copyright owner(s) are credited and that the original publication in this journal is cited, in accordance with accepted academic practice. No use, distribution or reproduction is permitted which does not comply with these terms.
*Correspondence: Yeyoung Ha, eWhhQGV4cG9uZW50LmNvbQ==