- 1Oceanography Department, Dalhousie University, Halifax, NS, Canada
- 2Aquaculture Services, Innovasea Marine Systems Canada, Bedford, NS, Canada
- 3Marine Affairs, Dalhousie University, Halifax, NS, Canada
Aquaculture farms represent a complex 3D environment and face regular seasonal challenges such as acute and chronically elevated temperatures during summer. Further, fish are exposed to the interaction between their environment and farm operations, which can cause challenging conditions. In the context of modern net-pen aquaculture and ocean warming, there is therefore a need to understand the welfare of these commercially important species under the realistic conditions they encounter. Fish were tagged with two types of biologgers measuring temperature, heart rate, external acceleration, and depth of fish as they experienced standard aquaculture operations over two periods of thermal stress, one short-term and one long-term. The fish response during the thermal stress events was compared with the periods preceding and following both events, and an additional analysis was carried out to further explore the effects of feeding and farm operations. Fish displayed signs of both secondary and potentially tertiary stress in response to the short- and long-term heat event and both heart rate and acceleration increased in response to feeding but displayed a more nuanced response to operations. As part of the broader concept of precision fish farming, this research, based on data from 7 individual fish, represents a case study that presents the potential use of biologgers as tools for recognising early signs of stress by observing the secondary stress response, thereby demonstrating the potential for informed and timely stress identification to guide farm management decisions to enhance fish welfare and production efficiency in commercial aquaculture.
1 Introduction
In the dynamic landscape of modern net-pen aquaculture and ocean warming, there is a pressing need to understand the intricate interplay between aquaculture operations and environmental conditions on fish stress, physiology, and behaviour of cultivated species (Calado et al., 2021; Froehlich et al., 2018; Stehfest et al., 2017). Temperature is both a direct and indirect driver that modulates metabolic rates, appetite, immune response, and growth rates (Barton, 2002; Farrell, 2009; Reid et al., 2019). Atlantic salmon (Salmo salar) are susceptible to thermal stress (Dwyer and Piper, 1987; Gallant et al., 2017; Gamperl et al., 2020), and production cycles regularly span two summers to maximise fast-growth periods, where, in addition to thermal stress, salmon can be exposed to other stressors (Burt et al., 2012; Jones and Price, 2022; Mardones et al., 2021). Farmers must be able to recognise granular changes in fish performance to respond with appropriate husbandry decisions to stressors, improving production cycles, growth, and fish welfare (Ashley, 2007). In Nova Scotia, maximum temperatures occur in late summer and early autumn, and this time of year is highly relevant for farmers in this region due to the likelihood of hypoxia and thermal stress events (Burke et al., 2021; Burt et al., 2012). Thermal stress can be acute or chronic in nature and it is difficult to determine absolute impacts on fish when additional sources of stress, e.g. farm operations (Iversen and Eliassen, 2014), co-occur and reduce fish ability to respond to stressors (Brijs et al., 2018; Gallant et al., 2017).
Fish stress on farms is inferred through operational welfare indicators (OWI), which serve as a straightforward and reproducible mechanism for farmers to promptly evaluate fish stress and react to suboptimal conditions to improve welfare (Noble et al., 2018). The stress response in fish is initiated by a disruption to the organism’s homeostasis, the body’s natural ability to regulate itself to maintain stability, in response to a real or perceived threat (Wendelaar Bonga, 1997). During the primary stress response, hormones are released by the adrenal glands into the blood stream, which results in secondary responses, including increased cardiorespiratory activity, increase in heat-shock protein synthesis and changes in plasma and metabolite levels (Ackerman et al., 2000). These physiological changes can modify certain biological activities and reallocate the energy of an individual to enable them to try and overcome the stressor they are experiencing (Huntingford et al., 2006). During events of prolonged stress, e.g., disease state, chronic stress may lead to tertiary responses such as reduced appetite and growth, altered swimming behaviours and a poor immune response (Barton, 2002). However, if fish are showing signs of tertiary stress responses, it is difficult to identify the initial cause of the stress and it is often too late to take action to prevent this response from occurring in the first place (Brijs et al., 2018).
Measurements of heart rate have been used in previous studies as a secondary stress-indicator in response to netting, chasing, crowding, and heat events (Boman, 2014; Brijs et al., 2018; Gamperl et al., 2021; Hvas et al., 2020) and they present a unique opportunity to identify early stress responses in cultured fish (Axelsson et al., 2007). Behavioural studies done in tanks on land have also shown that swimming activity increases in response to induced stress, such as simulated crowding (Svendsen et al., 2021), and similarly, increased swimming activity has been observed in response to crowding and delousing on commercial farms (Føre et al., 2018a). Additionally, low dissolved oxygen and elevated temperature decrease salmon swimming speed and feeding activity in net pens and recirculating aquaculture systems (Kolarevic et al., 2016; Oldham et al., 2018; Stockwell et al., 2021). Consequently, monitoring the physiology, i.e. heart rate, and behaviour, i.e. activity, of farmed fish during commercial operations offers a direct means to assess the impact of various stressors. These observations require less interpretation and provide a new potential method to assess fish welfare compared to more traditional indicators.
Modern, intensive salmon aquaculture requires monitoring and control of the farm system to ensure that fish are raised in an environmentally and ethically responsible way that prioritises fish health and efficient production systems. Using concepts from traditional land-based farming, precision fish farming (PFF) is an approach to farming that promotes enhanced monitoring, control, and databasing of all available data streams to develop support systems for enhanced decision making (O’Donncha and Grant, 2019). This includes improving previously manual or labour-intensive procedures or creating new data streams using modern, innovative technology (O’Donncha et al., 2021). Despite the progress of machine vision algorithms learning to identify individual fish (Schraml et al., 2021), these tools are not widely used in commercial settings, which results in a limited ability to examine repeated observations of the same individuals throughout the grow out cycle. Other than intermittent health checks that do not provide repeated observations for individuals, most fish-based observations are conducted at the group level (i.e, tertiary stress responses). Biologgers are a potential avenue to overcome this data gap and use the secondary stress response as an input to automated decision support systems that have been proposed for precision farming (Føre et al., 2018b). Furthermore, they provide a new data stream that enables data driven decision making and promotes repeatability in operational decisions, which are key components of PFF. There are still challenges that must be overcome before biologgers are adopted into standard operating procedures, and research undertaken on commercially operating farms is an important step to achieve this.
The objective of this work is to explore the behavioural and physiological responses of farmed Atlantic salmon to 1) thermal stress, and 2) the compounding effects of general farm operations and feeding events. Salmon farms in Nova Scotia experience acute and chronic warm temperatures during the summer periods of production cycles and with the ongoing effects of climate change impacting the North Atlantic, it is important to understand how salmon respond to elevated temperatures (Klinger et al., 2017). Biologgers provide a unique avenue to observe free swimming fish as they experience standard aquaculture operations in commercial net pens and allow for direct observations of fish exposed to compounding stressors. In this study, biologgers were used alongside an environmental monitoring system with oxygen and temperature sensors. Further, a farm log was kept to record when environmental stress overlapped with routine and exceptional farm operations. This research helps further the use of biologgers as precision aquaculture tools in commercial aquaculture and provides a mechanism to recognise earlier signs of stress in farmed salmon, which can help to inform farm management to potentially alleviate sources of stress sooner.
2 Materials and methods
2.1 Experimental animals and ethical statement
This study took place on a commercially operating Atlantic salmon farm in Nova Scotia, Canada. The data used in this study are a subset of thermal stress periods from a longer study that focussed on the thermal physiology of salmon (Korus et al., 2024). Accordingly, additional site information and detailed methods can be found in Korus et al. (2024), but in brief, two types of biologgers were implanted, an Acoustic Data Storage Tags (ADST, model V13AP, diameter: 13 mm, length: 43 mm, weight in air: 11.5 g; Innovasea Systems Inc, Bedford, Nova Scotia, Canada) that measures tri-axis acceleration and depth, logging them internally and acoustically transmitting (at 69 kHz) and a centi-HRT ACT Data Storage Tags (DST, DST centi-HRT ACT G2, diameter: 15 mm, length: 46 mm, weight in air: 19 g; STAR-ODDI, Gardabaer, Iceland), that records heart rate, temperature, and acceleration. The ADST tags, which were not used in this analysis due to a malfunction in some of the pressure sensors, limiting statistical power, and the centi-HRT ACT DST were surgically implanted (see below) into 17 fish on July 29th, 2021, on a vessel alongside the study pen. External acceleration was used as a proxy for swimming activity as it has been shown to positively increase with swimming speed (see Zrini and Gamperl, 2021 for validation and discussion of the approach. All means are reported as mean ± standard deviation. Fish were mixed-sex, diploids, originating from the Saint John River strain, stocked in May 2020 with an average weight of 78 g. The tagged fish weighed between 2.7 and 5 kg (3.76 ± 0.67 kg) and the combined tag weight was 30.5 g, resulting in a maximum tag contribution of 1.1% of body weight, well within the 2% guideline of tag to body weight ratio while minimising the ratio as much as possible (Lawrence et al., 2023). The site was located at the mouth of Liverpool Bay in Nova Scotia, protected by the presence of a small island. Fish were kept in a pen of ~8,000 conspecifics that had a circumference of 100 m and the net extended 10 m deep. All other pens on the farm had approximately 25,000 fish at the start of the study period. The depth surrounding the site was between 12 and 15 m. The surgeries were performed by the producer’s veterinarian, and all experimental protocols were approved by the Dalhousie University Committee on Laboratory Animals (UCLA) (protocol number 20-119).
2.2 Surgical procedure
Individual fish were dip-netted from an aquaculture pen one at a time and placed into an induction tank with seawater and 200 mg L−1 tricaine methanesulfonate (TMS). Once fish had lost all signs of consciousness and no longer responded to physical stimulation, they were transferred to a scale to record their weight and length. Fish were then placed on a surgical v-trough, and seawater containing a lighter dose of anaesthetic (100 mg L−1) was flushed over the gills for oxygenation and sedation. The surgical procedure is detailed in Korus et al. (2024), but in brief, a 2 cm incision was made posterior to the pectoral fins to implant both the ADST tag and centi-HRT ACT tag. The ADST tag was positioned caudally, while the centi-HRT ACT tag was placed superior to the incision with electrodes oriented for optimal electrocardiogram (ECG) signals. To stabilise the tag based on recommendations from the tag manufacturer, the centi-HRT ACT tag was secured in place using the channel provided through the ceramic casing on the tag, and the incision was closed with interrupted sutures. A fluorescent T-Bar tag was injected through the dorsal fin for fish identification. Following surgery, the fish were moved to a recovery tank fitted with a hose, pumping oxygenated seawater, and monitored until they exhibited normal locomotory behaviour. Seventeen fish were tagged; however, the final three fish were unable to recover fully due to a boat safety issue caused by the unexpected increase of wind and waves, which caused the continuous overflow of the tank, compromising the engine area. The recovery tank had to be emptied unexpectedly, which resulted in much shorter recovery times for the fish undergoing recovery, surgery and the one under anaesthetic at the time of the incident. Real-time acoustic data suggested subsequent mortality for these three fish. Overall, the surgical procedure averaged 8 minutes, and recovery time in the tank averaged 55 minutes.
2.3 Tag programming
Centi-HRT ACT tags were programmed to target periods of expected thermal extremes during a standard production cycle. To capture this, a 1-hour sampling rate was used immediately following surgery for 2 weeks to observe fish recovery from July 30th to August 16th, 2021. Following this, a 4-week period from August 16th to September 16th, 2021, of 20-minute samples was used to capture high frequency data during potential warm thermal events. From the 16th of September onwards, a reduced rate of 6 hours was used to conserve battery life and memory storage. The summer period was considered finished in mid-November, after the end of the long-term heat event (see below), equating to a study period of 124 days. Although data collection continued until March 31st, 2022, winter data was outside the scope of this work and only analysed in Korus et al. (2024).
2.4 Farm data
To provide context to the biologger measurements, logs of farm operations data were collected throughout the study period. Anytime divers were on site for maintenance work or mortality assessments, a time sheet was logged, and this data was combined with other site reports recording in-situ net cleaning operations, general site maintenance and predator net removal to create an operational time series. Feeding times and amounts were also recorded and used as an hourly time series in the analysis. In addition to the farm logs, 5 real time environmental monitoring sensors (aquaMeasure DOTD, Innovasea, Halifax, Canada) measuring dissolved oxygen, temperature and depth were deployed on a vertical profile line 1.5 m apart at approximately 2, 3.5, 5, 6.5 and, 8 m. Data was transmitted acoustically every 2–3 minutes and logged internally every 5 minutes for the duration of the study.
2.5 Data processing and analysis
Fish were harvested on July 26th, 2022, but only seven fish with tags were retrieved and used in the analysis. The remaining seven fish were not found, and it is unknown what happened to them, but from the acoustic data, five of the remaining seven were reporting normal data at the end of March 2022, so they were not considered mortalities. The average weight at harvest was 7.46 ± 0.40 kg and the average length was 75.34 ± 2.91 cm representing a 98% weight increase and a 23% length increase (see individual fish biometrics in Korus et al., 2024). Fish condition was assessed using the condition factor (K):
where W and L are weight (g) and length (mm), respectively. The condition factor was recorded at the time of tagging and after harvest and all values of K (K > 1.4) indicated good or excellent condition in all fish except F14, which was classed as poor at the time of harvest only (1.0 < K < 1.2). For all analyses, data were processed using the tsclean() function in the R forecast package (Hyndman et al., 2024) and data were averaged hourly, daily or by event for each individual fish and then combined for subsequent analysis.
To explore periods of thermal stress, heat maps were generated by filling in missing data points at each depth over time and then upsampled over the water column using linear interpolation to smooth the transition across depths. In September and October, two distinct periods of elevated temperature were observed and classified by an acute increase, followed by a swift decrease in temperature throughout the entire water column. The start and end points of these heat events were manually selected based on the sharpest rate of change between subsequent points. The average response in heart rate and acceleration during the heat events was compared to non-heat event periods for each fish using a type II regression to account for two independent variables. External acceleration of all individuals followed a skewed distribution, so data was log transformed and tested for normality using the Shapiro-Wilks test prior to averaging for all subsequent analyses. The slope and intercept of each regression were statistically compared to 1 and 0, respectively, to determine the effect of the heat event. Further statistical analyses were conducted to assess the impact of the individual heat events by comparing them to the time periods immediately before and after each thermal event. The before and after periods for the short-term heat event (STHE) were selected to be the same duration as the heat event (2.5 days), however due to the nature of the second, long-term heat event (LTHE), the before and after periods were determined to be half (17 days) of the duration of the heat event (34 days) to avoid overlap with the first short-term heat event. Comparisons of heart rate, log-transformed external acceleration, and temperature were made before, during, and after each heat event using the Friedman test and post-hoc analysis was done using the Nemenyi test. The Friedman test is a non-parametric rank-sum test between three of more paired groups and the Nemenyi post-hoc was used to test significance between paired groups when the Friedman test was significant. The number of times fish were fed per day was also averaged for each period.
To analyse the effects of feeding and farm operations on behaviour and physiology, daily averages of all biologger data were calculated using daytime data only (all data between sunset and sunrise was excluded) to remove the daytime bias of feeding and operations. To remove the confounding effect of temperature in this analysis, heart rate was corrected using the Arrhenius temperature (TA) (Equation 2):
where fH is heart rate, Tobs is the observed temperature in Kelvin, TA is the Arrhenius temperature (TA = 1663, Korus et al., 2024) and T is the reference temperature that heart rate is being corrected to, in this case, 13.9°C was used because it was the average temperature observed from August to mid-November, the full extent of the period considered in this analysis. To determine whether there were any statistically significant differences in heart rate and log-transformed acceleration during all combinations of feeding vs. non-feeding periods and during operations vs non-operations periods, a Friedman test and post hoc Nemenyi test were carried out. P-values obtained from any statistical analysis were considered statistically significant if p < 0.05. All statistical analyses were performed in RStudio v. 4.2.2, RStudio Inc., Boston, MA, USA; https://www.rstudio.com/).
3 Results
3.1 Environmental conditions
Temperature had little variation throughout the water column and averaged 13.1 ± 2.8°C from August to November, with a maximum temperature of 21.5°C occurring on August 13th and a minimum temperature of 6.4°C occurring on September 14th (Figure 1A). The maximum daily average was 18.3°C on August 29th and the minimum daily average temperature was 9.0°C on September 13th. Oxygen saturation ranged from 62.3 to 147.0% throughout this period; values over 140% can be indicative of biofouling and these values were predominantly apparent at the surface where sensors are most affected (Figure 1B). Oxygen displayed high variability day to day but showed no distinct seasonal trends. When oxygen was stratified throughout the water column, oxygen was generally higher at the surface and there were only three days throughout the period where oxygen dropped below 70%. The average oxygen saturation throughout the water column was 102.7 ± 6.6% from August to November with a maximum daily average of 118.1% on August 27th and a minimum daily average of 88.5% on November 20th.
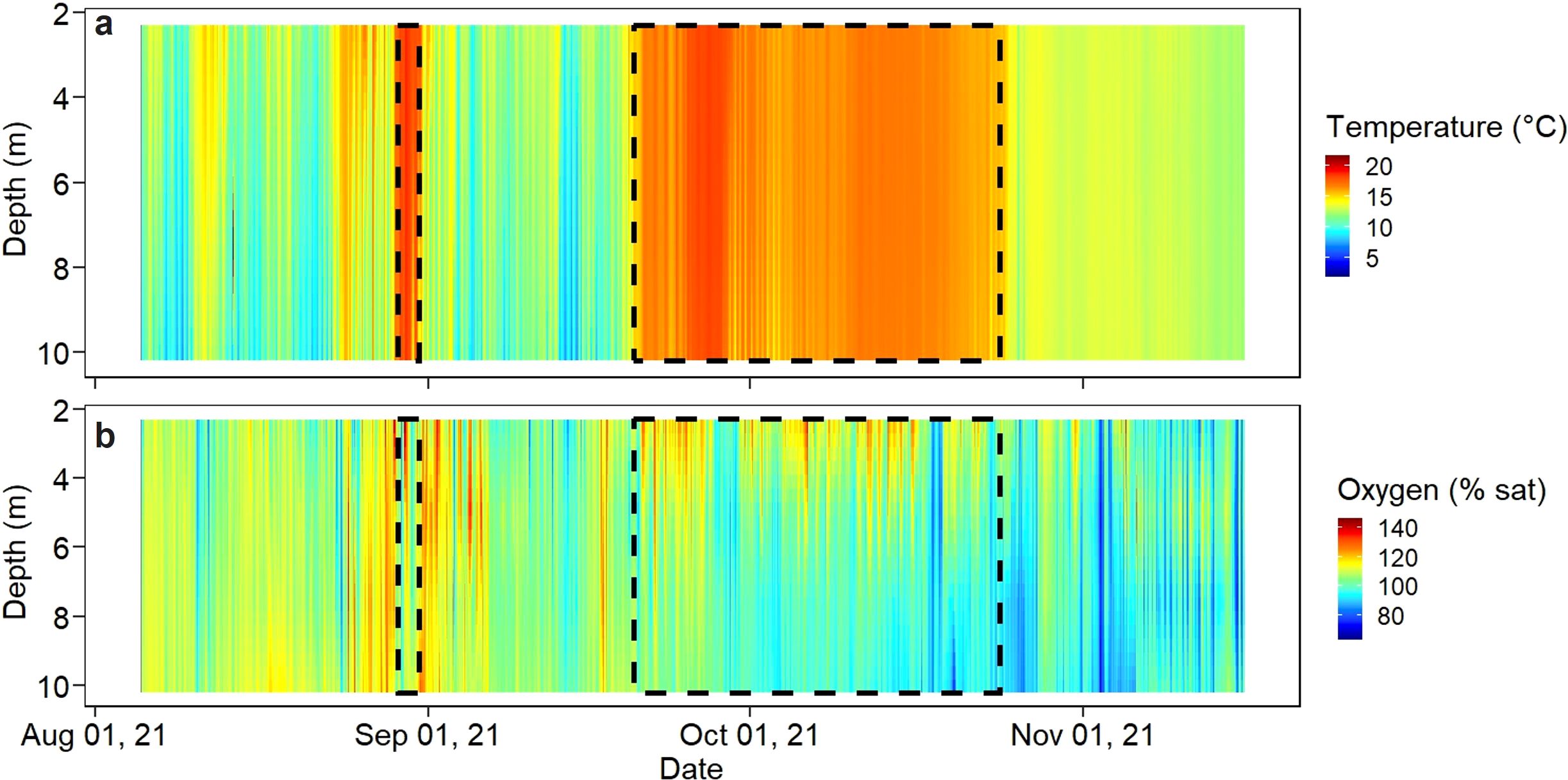
Figure 1. Environmental data from cage 14 throughout the duration of the summer period from 5 oxygen, temperature and depth sensors on a profile line separated by 1.5 m (A) Water temperature [°C]. (B) Oxygen [% Saturation].
3.2 Response to heat events
A general comparison between the average heart rate of individual fish during the periods with heat events and the rest of the study period revealed a positive linear relationship (r2 = 0.85, p < 0.01, Figure 2A), suggesting that variation between individual responses persists under the effect of thermal events. The linear relationship between the heart rate of individuals under both conditions revealed that the slope was significantly lower than 1 (p < 0.05), but the intercept was not significantly different than 0 (p = 0.08). This suggests that individuals with naturally higher heart rate could be restricted in their capacity to proportionally increase heart rate in response to thermal stress, in contrast to those with naturally lower heart rates. The same comparison between log-transformed average external acceleration during heat events and the rest of the study period was carried out and revealed a similar positive relationship (r2 = 0.80, Figure 2B). The slope of the linear relationship between the log-transformed acceleration of individuals was significantly lower than 1 (p < 0.001) and, the intercept was also statistically different than 0 (p < 0.01), suggesting that fish activity was consistently higher under normal conditions than under thermal stress but the change in activity was not proportional to normal conditions across individuals.
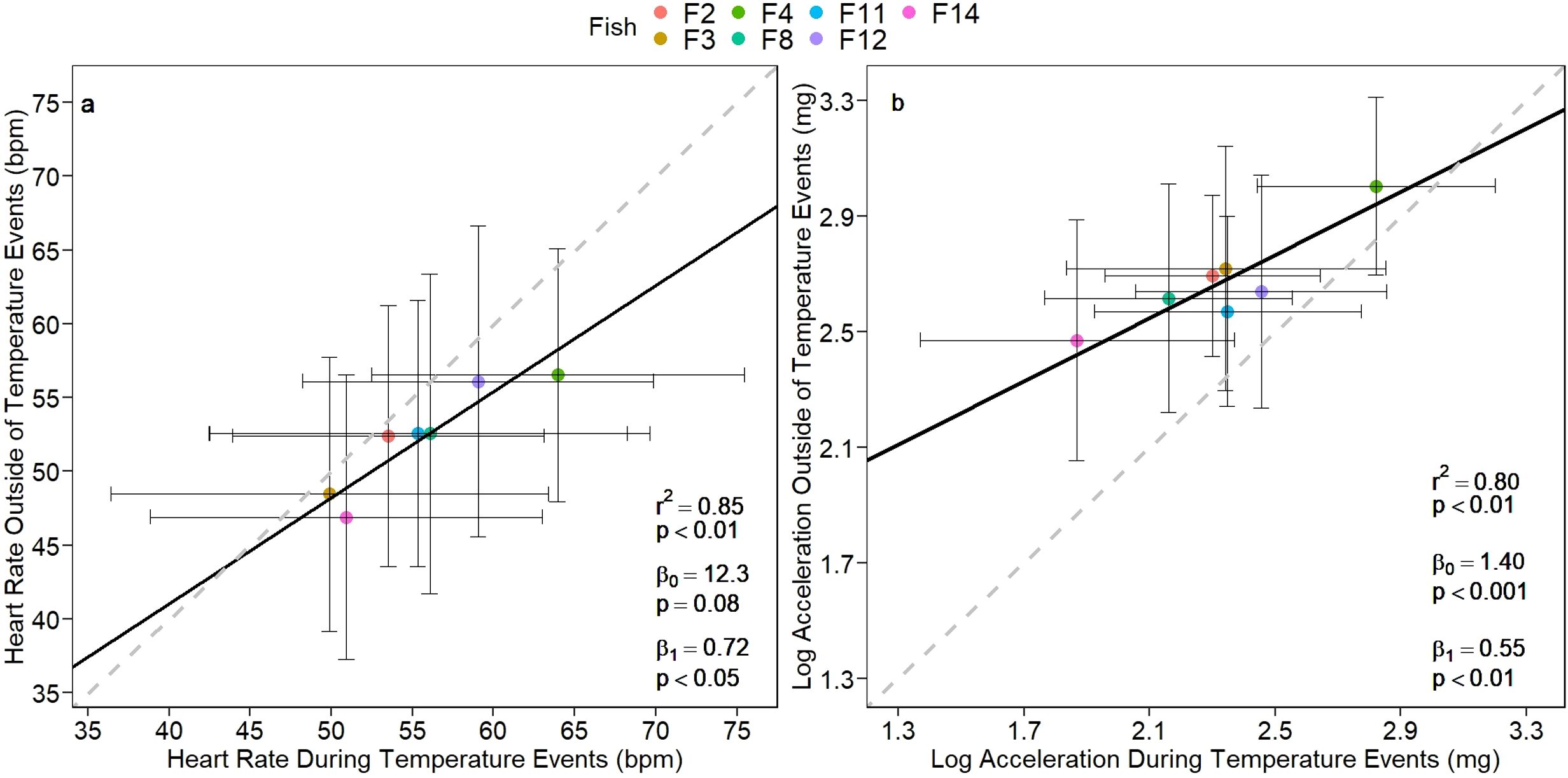
Figure 2. Type II regression analysis of (A) fH [bpm] and (B) log-transformed acceleration [mg] of individual fish during the heat events and not during the heat events. Dashed line shows 1:1 relationship. Regression slope and intercept were tested against 1 and 0, respectively, and estimates of the intercept (β0) and slope (β1) are presented on the plot.
A detailed analysis of the heat events with the periods immediately before and after the event revealed statistical differences between heart rate for both the LTHE and the STHE event (p < 0.001, Table 1). The STHE occurred at the end of August over a period of 2.5 days starting August 28th, and it was defined by an acute temperature change (>2°C) compared to the 2.5 days before and after the event (during vs. after: p <0.001, Figure 3A). The LTHE started on September 20th and lasted until October 24th, totalling 34 days, and was similarly defined by a temperature difference >2°C during the event, which was significantly different to the temperature before the event (p < 0.001, Figure 3B). The severity of the LTHE was less than the STHE (16.9°C vs 18.1°C); however, the 34-day duration made it noteworthy. Heart rate increased during the STHE from 59 to 69 bpm and dropped to 53 bpm following the event (p < 0.001, Table 1) and post hoc analysis revealed that the decrease following the event was significant (p < 0.001, Figure 3C). Similarly, there were significant differences in heart rate over the LTHE (p < 0.05, Table 1); however, in contrast to the response to the STHE, post hoc analysis showed heart rate increased significantly following the LTHE from 49 to 55 bpm (p < 0.05, Figure 3D). Regarding log-transformed external acceleration, there were significant differences throughout both the STHE and the LTHE (p < 0.01, Table 1). Post hoc analysis revealed that it significantly increased from 2.4 mg before and during the STHE to 2.7 mg after the event (p < 0.05 and p < 0.01, Figure 3E) and significantly decreased from 2.7 mg before the LTHE to 2.3 mg during the event (p < 0.01, Figure 3F). Daily feed regimens were recorded throughout the duration of both events and were reported as an average for each period, and in both cases, the daily rate was lower during the events compared to the periods before and after (Figures 3G, H).
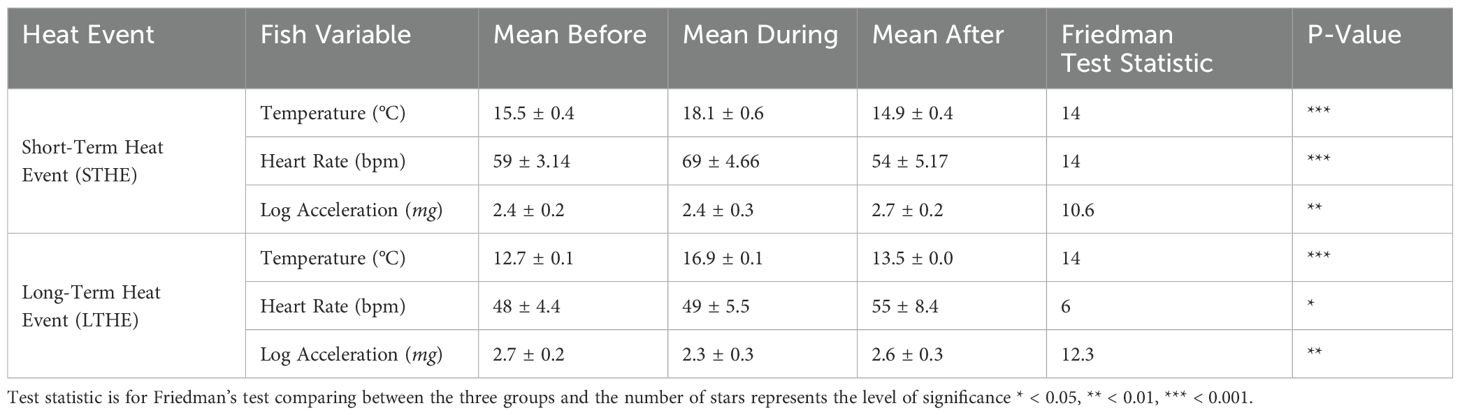
Table 1. Average temperature, heart rate and log-transformed acceleration during both the STHE and the LTHE.
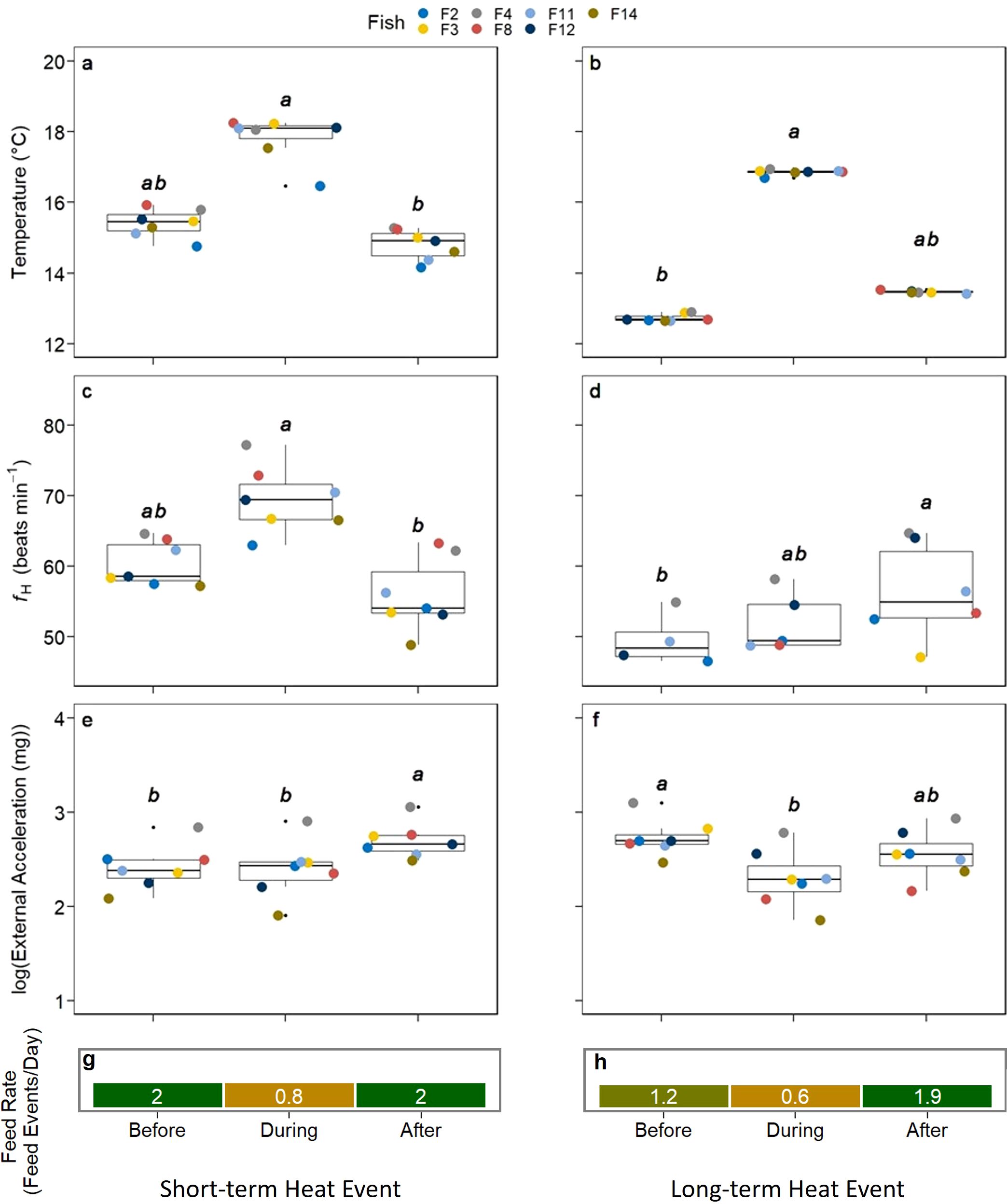
Figure 3. Comparison of fish observations before, during and after a short- and long-term heat event. Coloured points indicate the individual fish average. All comparisons were made with the Friedman test and post-hoc analysis was done using the Nemenyi test. Significant differences are indicated by lowercase lettering. All comparisons were made between the three periods before, during, and after (A) STHE temperature [°C] (B) LTHE temperature [°C]. (C) STHE fH [bpm] (D) LTHE fH [bpm]. (E) STHE log-transformed external acceleration [mg] (F) LTHE log-transformed external acceleration [mg] (G) STHE feed rate [feed events/day] (H) LTHE feed rate [feed events/day].
3.3 Response to feeding and operations
Temperature corrected heart rate was used to compare the effect of feeding and other farm operations on heart rate. Significant statistical differences emerged when comparing the four categories of feeding and operations, feeding and no operations, operations and no feeding and no feeding and no operations (Friedman chi-squared: 19.97, p < 0.001). The combined effect of feeding and operations increased heart rate significantly compared to periods when no feeding was occurring regardless of operations (p < 0.001 when neither feeding nor operations were occurring and p < 0.05 for non-feeding periods when operations were occurring, Figure 4A). When operations were not occurring, heart rate was significantly elevated during periods of feeding compared to non-feeding periods (p < 0.05). Similarly, significant statistical differences emerged when comparing the log-transformed external acceleration between the same four categories combining feeding and operations (Friedman chi-squared: 15.51, p < 0.001). Logged acceleration was higher during feeding periods only when there were no operations co-occurring compared to non-feeding periods when operations were occurring (p < 0.01) and when no operations were occurring (p < 0.05, Figure 4B).
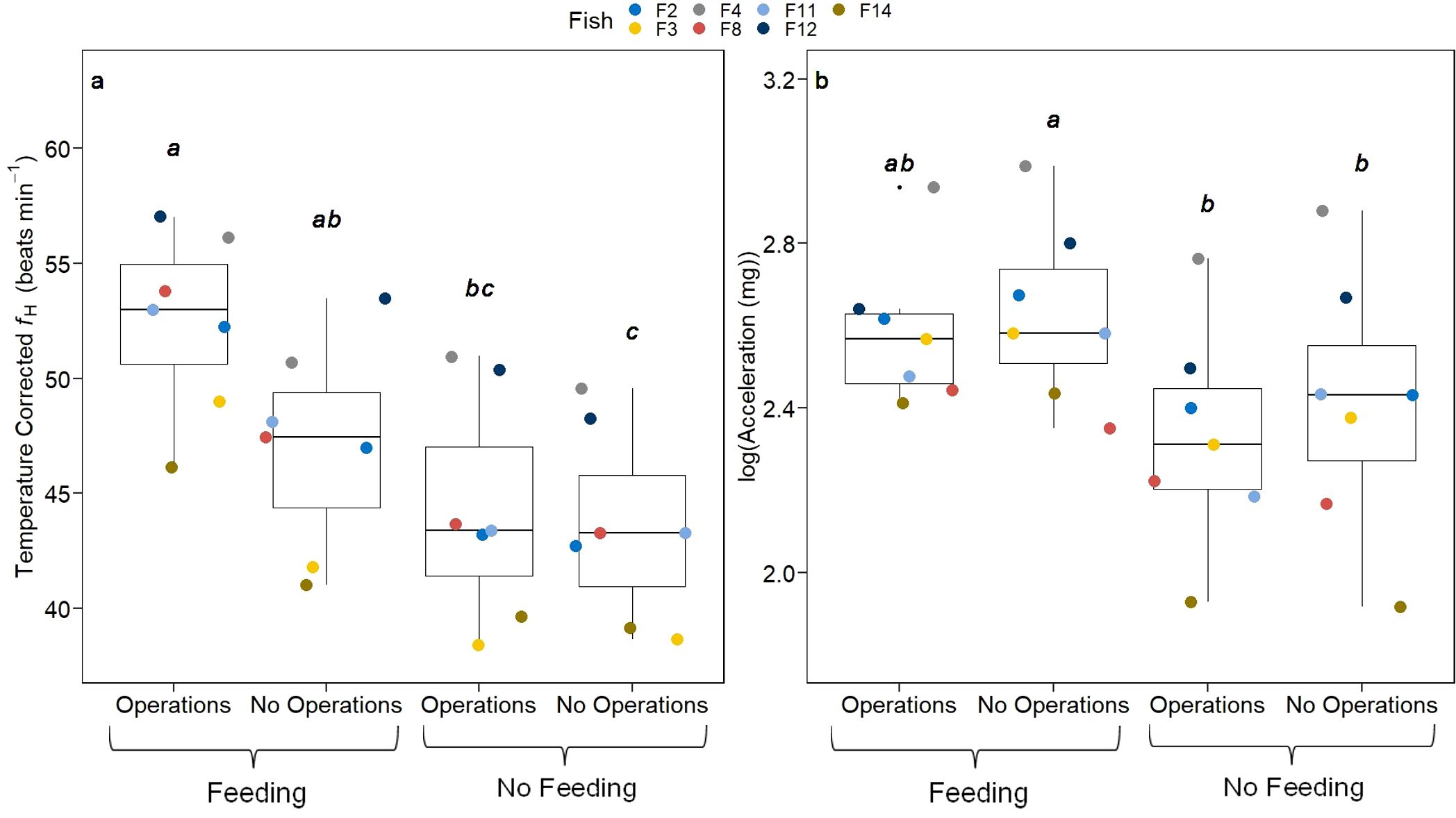
Figure 4. Comparison of fish observations between all combinations of when operations and feeding events are and are not occurring. Coloured points indicate the individual fish averages. All comparisons were made with the Friedman test and post-hoc analysis was done using the Nemenyi test. Significant differences among groups are presented by lowercase lettering. (A) fH [bpm] (B) Log-transformed external acceleration [mg].
4 Discussion
The analysis of the physiology and behaviour of Atlantic salmon using biologgers enabled an examination of the complex interactions between thermal heat events and commercial farm operations. Fish exhibited distinct responses in heart rate and log-transformed external acceleration (hereafter activity) to a short-term heat event (STHE) compared to a long-term heat event (LTHE), and fish showed physiological and behavioural responses consistent with secondary stress response during the STHE (i.e. increased heart rate) and potentially a tertiary response during the LTHE (i.e. decreased activity). Generally, feeding had a stronger effect than operations and caused both heart rate and activity to increase, and operations increased heart rate but caused a decrease in activity; however, the interaction between feeding and operations on heart rate and activity was more complex, and caused varied responses.
During the STHE, temperature reached a maximum of 19.2°C and averaged 18.1 ± 0.6°C. From a physiological perspective, heart rate followed the expected response to the thermal fluctuations over the course of the STHE, increasing cardiac output via heart rate to meet increased metabolic demands at higher temperatures (Gamperl et al., 2011). In a separate analysis of these data over the full temperature range, i.e. including the winter months, heart rate scope was reduced substantially at temperatures greater than 18°C, suggesting proximity to thresholds of thermal stress during the STHE (Korus et al., 2024). These temperatures are also comparable to those that have been cited as near the upper thermal tolerance for Atlantic salmon, specifically those used for commercial aquaculture in Canada, above which negative effects on their appetite, growth and stress have been observed (Gamperl et al., 2020). The efficiency of salmon growth at or above 19°C varies across studies in different regions, underpinning the importance of research being carried out on specific stocks to inform aquaculture management in different regions (Hevrøy et al., 2015; Nuez-Ortín et al., 2018; Tromp et al., 2018). Therefore, the temperature regime observed during the STHE exposed the individuals to their upper thermal limit.
During the LTHE, temperature reached a maximum of 18.8°C and averaged 16.9 ± 0.6°C over a 34-day period. Contrary to STHE, heart rate did not follow the expected response with temperature and, instead, heart rate increased after the event, when temperatures dropped. Observations of heart rate under chronic thermal stress are limited in the literature; however, long-term exposure (over 1 month) to elevated temperatures (19–20°C) has shown a reduction in energy body reserves, reduced feed intake and increased feed conversion ratio (Gamperl et al., 2020; Hevrøy et al., 2012; Koskela et al., 1997). The effects of chronic exposure to elevated temperature on growth vary depending on the length and severity of the exposure, size of fish and development stage and notably, larger, post-smolt salmon show reduced growth at chronically high temperatures compared to juveniles (Handeland et al., 2008; Hevrøy et al., 2012; Norambuena et al., 2016). During periods of long-term thermal stress, heart rate cannot be sustained at elevated levels, and the reallocation of energy can become detrimental to fish, resulting in these tertiary level stress responses (Handeland et al., 2008; Opinion et al., 2023). Accordingly, the chronic exposure to temperatures approaching stressful limits during the LTHE could cause the observed reduction in heart rate; however, this reduction could also be a consequence of the daily feed rate, which was lower throughout the LTHE compared to the periods before and after. During both the short-term and long-term heat events, management decisions were taken to reduce feeding frequency to reduce stress; a common response in salmon farming to heat stress (e.g. Oppedal et al., 2011). Feeding periods were associated with increased heart rate when direct comparisons were made between the different combinations of operations and feeding. Despite the increase in heart rate as a response to feeding, heart rate increased during the STHE when feed rates were reduced, suggesting that thermal response at temperatures close to their thermal limit had a stronger influence than the absence of feeding. Contrarily, at tolerable yet elevated temperatures during the LTHE, feeding rates had a greater impact on regulating heart rate. As sea surface temperatures continue to increase due to climate change, the severity and frequency of chronic exposure to elevated temperatures is also expected to increase (Klinger et al., 2017) and having a holistic understanding of the implications on stress and growth, particularly as they relate to confounding variables such as feeding, are necessary to inform farming practices.
Fish activity also displayed distinct responses to the STHE and the LTHE. Before and during the STHE, activity was consistent and significantly increased after the event. When exposed to acute temperature shock (greater than 28°C for up to 5 minutes), Atlantic salmon exhibited intense swimming speed and activity, which was interpreted as a panic response (Nilsson et al., 2019). Although swimming speed and activity are not the same measurement, many studies have shown a positive correlation between them (Kawabe et al., 2003; Zrini and Gamperl, 2021). Swimming activity has also exhibited an increased response to other stressors common to aquaculture such as crowding and delousing procedures (Føre et al., 2018a; Svendsen et al., 2021). Temperature is a unique stressor as it has both direct and indirect effects (Segner et al., 2012) and while critical swimming speed has been shown to increase when experiencing acute temperature shock, it is possible that the change in temperature was not intense enough to elicit that behavioural response, or other confounding factors such as the reduced feeding rate during the heat event, may have impacted their behavioural response. Further, operations occurred before the STHE, which also may have decreased their activity. Similarly, it is also possible that the increase in activity following the STHE was driven by an increase in the daily feed rate as activity was shown to be higher during feeding periods.
During the LTHE, activity was significantly lower than it was before the event suggesting that chronic thermal stress may have a dampening effect on the overall activity of salmon. When Atlantic salmon were acclimated to warmer temperatures over multiple weeks, swimming speed was shown to increase up to a thermal optimum of 18°C but started to decrease at higher temperatures (Hvas et al., 2017). A long-term study that recorded swimming speed in response to seasonal changes in temperatures over multiple years, observed a similar swimming speed response curve, with maximum speeds occurring at 10.5°C and an 80% reduction in swimming speed at temperatures lower than 4°C and greater than 17°C (Martin et al., 2012). However, a similar study that measured external acceleration using the same biologgers on a commercial farm observed the opposite trend. Activity was elevated during a heat event in Newfoundland in 2019 that occurred over a similar time duration of ~4 weeks where temperatures increased from 10–12°C to 18–19.5°C (Gamperl et al., 2021). In addition to the opposing trends at similar temperatures, the average activity values observed by Gamperl et al. (2021) during the heat event were lower than the values observed in this study, suggesting that additional factors other than temperature influence fish activity. Further, the average fish temperatures recorded during the heat event were lower, possibly due to the depth of the net pen, which extended twice as deep in the water column allowing fish to seek out cooler temperatures through behavioural thermoregulation. Non-feeding periods were also associated with decreased activity and the reduction of feeding during the LTHE may have contributed further to lower activity. Under chronic stress, the tertiary stress response can impose a reduction in swimming capacity (Barton, 2002), caused by the increased energy allocation towards maintaining homeostasis and reducing the available energy for regular swimming activity, but it is difficult to determine that this response was definitively part of the tertiary stress response. This emphasises the difficulty of identifying stress conditions in fish using behavioural indicators and is a limitation of tools such as accelerometers in identifying stress. Although changes in behaviour can be identified and classified based on other factors, a change in behaviour does not necessarily indicate a stress condition. The effects of chronic stress can become cumulative and limit the ability of salmon to cope with additional stressors (Brijs et al., 2019; Madaro et al., 2015). Accordingly, long-term monitoring of swimming activity can provide insights into behavioural changes over time and identify when tertiary stress may be occurring.
The potential effects of temperature on Atlantic salmon behaviour and physiology have been examined above; however, it is important to recognise that thermal responses are usually confounded with farm operations such as feeding or other environmental conditions. Hypoxic conditions can occur due to oxygen consumption of fish (Solstorm et al., 2018), reduced water exchange across the farm driven by tidal cycles (Burke et al., 2021) and temperature induced hypoxia due to the combined effect of increased metabolic demands and the reduction in oxygen solubility of seawater at elevated temperatures (Neubauer and Andersen, 2019). Hypoxic conditions were not observed in the pen during the study period, but they regularly occur in this region during the late summer and fall, and many laboratory studies have examined the combined negative effects of these stressors on growth and survival (Anttila et al., 2014; Gamperl et al., 2020; Remen et al., 2012, 2016). Farm operations is another variable, unique to aquaculture that is often overlooked as a factor to consider in studies exploring the behavioural and physiological response of farmed salmon. The effect of operations had a more nuanced effect on physiology and behaviour, in that it amplified the increase in heart rate when feeding was co-occurring, but there was no effect on acceleration. Operations is a broad term that was used to encapsulate diver activities, net cleaning, predator net removal and other general maintenance so it is reasonable to assume that fish have varied responses to each activity. This analysis suggests that operations are less relevant than feeding but a (non-significant) trend of reduced activity when operations were occurring seemed to emerge.
The comparisons between heat events, feeding and operations are limited by the small sample size (n=7). Unfortunately, 7 individuals were not recovered at the time of harvest, which resulted in a loss of half of the potential data. Despite observing significant differences across many comparisons, the extrapolation of conclusions to group-level patterns should be taken with caution, and further research should aim to increase the statistical power of the analyses. Although loss of tags and small samples sizes are not uncommon in field studies where fish must be recaptured to access tag data (Gamperl et al., 2021, tagged 12 fish but only analysed 8 individuals due to mortality and inability to recover tags). Land based studies often tag similar numbers of fish but subset individuals into smaller groups for analysis of different experiments (Svendsen et al., 2021 tagged 12 fish, but only 6 individuals had accelerometers for activity analysis). This farming system is unlike many larger production systems in Norway; pens are situated in shallower water, with large tidal influence, and individuals were tagged in the pen containing 8,000 conspecifics to maximise potential of tag recovery. Accordingly, the observed behavioural response is representative of the experimental rearing conditions, and extrapolations to other systems should be approached thoughtfully. Large individual variability in behaviour has been demonstrated, e.g., after the onset of underwater lights (Ulvund et al., 2021), and consequently, the application of results should be cautiously applied when extrapolating to group-level dynamics, and future studies would benefit from a larger number of fish. The status of the remaining 7 tagged fish is unconfirmed. It is unknown whether they died prior to harvest and were not found or whether they were simply missed during tag recovery efforts. The T-Bar tags were not an effective method to externally identify tagged individuals. Other studies have used PIT tags which can be found using a metal detector at the processing plant. However, the setup of the processing facility and their experience using these types of tags would determine how effective and/or disruptive this effort is and, although it likely ensures a larger number of tags found, it may be more appropriate in non-commercial settings. Further studies should be carried out in production cages at higher densities closer to industry standards and the determination of how tags will be retrieved should be carefully considered.
This examination of Atlantic salmon physiology and behaviour investigates the complex interplay between thermal conditions, feeding patterns, and farm operations, offering insights with broader implications for precision fish farming. The sensitivity of heart rate fluctuations is a useful indicator for potentially recognising the secondary stress response in fish. However, it requires careful consideration within the proper context and in conjunction with other variables to accurately determine whether the observed increase in heart rate is due to stress or other drivers. Notably, the findings highlight that feeding and farm operations influence heart rate and acceleration, recognising that there is nuance in how the irregularity and the type of operation can have various impacts on fish. The findings also demonstrate that there is potential in the use of biologgers as precision farming tools. Further research should focus on the development of industry tools using additional observations and monitoring in commercial net pens to mitigate potentially confounding effects such as land-based environments, stocking densities, and the interaction between environmental stressors and farm operations. This could lead to the development of new fish based OWI’s that could promote better recognition of fish stress and welfare determinations in commercial aquaculture. As we better understand how fish respond to various and complex stressors, these insights pave the way for more informed and tailored approaches in aquaculture management, contributing to the ongoing development of efficient and responsible practices in commercial fish farming.
Data availability statement
The raw data supporting the conclusions of this article will be made available by the authors, without undue reservation.
Ethics statement
The animal studies were approved by Dalhousie University Committee on Laboratory Animals. The studies were conducted in accordance with the local legislation and institutional requirements. Written informed consent was obtained from the owners for the participation of their animals in this study.
Author contributions
JK: Conceptualization, Data curation, Formal analysis, Investigation, Methodology, Visualization, Writing – original draft, Writing – review & editing. RF: Funding acquisition, Investigation, Supervision, Validation, Visualization, Writing – original draft, Writing – review & editing. JG: Conceptualization, Funding acquisition, Project administration, Supervision, Validation, Writing – original draft, Writing – review & editing.
Funding
The author(s) declare financial support was received for the research, authorship, and/or publication of this article. This work was supported by the Atlantic Fisheries Fund project “Big Data and Precision Fish Farming in Nova Scotia” (AFF-NS-1544) and Innovasea Marine Systems Canada.
Acknowledgments
The authors would like to thank Cooke Aquaculture for their collaboration and support of this project by use of their facilities and effort in coordinating and carrying out this research, in particular Dr. Andrew Swanson, Scott Leslie, Jennifer Hewitt, and Eoin McInnes. The authors would also like to thank Innovasea, especially Dale Webber, Tim Stone, Cyril Dempsey, Matthew Holland, Jeremy Kuehner, Ricky Sawler and Emily Napier as well as Ásgeir Bjarnason at Starr Oddi for their technical expertise and support throughout the project. This publication is based on the Master’s thesis of Jennie Korus submitted to Dalhousie University (March 2024).
Conflict of interest
The authors declare that the research was conducted in the absence of any commercial or financial relationships that could be construed as a potential conflict of interest.
Publisher’s note
All claims expressed in this article are solely those of the authors and do not necessarily represent those of their affiliated organizations, or those of the publisher, the editors and the reviewers. Any product that may be evaluated in this article, or claim that may be made by its manufacturer, is not guaranteed or endorsed by the publisher.
References
Ackerman P. A., Forsyth R. B., Mazur C. F., Iwama G. K. (2000). Stress hormones and the cellular stress response in salmonids. Fish. Physiol. Biochem. 23, 327–336. doi: 10.1023/A:1011107610971
Anttila K., Jørgensen S. M., Casselman M. T., Timmerhaus G., Farrell A. P., Takle H. (2014). Association between swimming performance, cardiorespiratory morphometry, and thermal tolerance in Atlantic salmon (Salmo salar L.). Front. Mar. Sci. 1. doi: 10.3389/fmars.2014.00076
Ashley P. J. (2007). Fish welfare: Current issues in aquaculture. Appl. Anim. Behav. Sci. 104, 199–235. doi: 10.1016/j.applanim.2006.09.001
Axelsson M., Dang Q., Pitsillides K., Munns S., Hicks J., Kassab G. S. (2007). A novel, fully implantable, multichannel biotelemetry system for measurement of blood flow, pressure, ECG, and temperature. J. Appl. Physiol. 102, 1220–1228. doi: 10.1152/japplphysiol.00887.2006
Barton B. A. (2002). Stress in fishes: A diversity of responses with particular reference to changes in circulating corticosteroids. Integr. Comp. Biol. 42, 517–525. doi: 10.1093/icb/42.3.517
Boman D. (2014). Monitoring heart rate and body temperature in rainbow trout, Oncorhynchus mykiss, using a bio-logging system. Available online at: http://hdl.handle.net/2077/40926 (accessed September 14, 2023).
Brijs J., Sandblom E., Axelsson M., Sundell K., Sundh H., Huyben D., et al. (2018). The final countdown: Continuous physiological welfare evaluation of farmed fish during common aquaculture practices before and during harvest. Aquaculture 495, 903–911. doi: 10.1016/j.aquaculture.2018.06.081
Brijs J., Sandblom E., Axelsson M., Sundell K., Sundh H., Kiessling A., et al. (2019). Remote physiological monitoring provides unique insights on the cardiovascular performance and stress responses of freely swimming rainbow trout in aquaculture. Sci. Rep. 9, 9090. doi: 10.1038/s41598-019-45657-3
Burke M., Grant J., Filgueira R., Stone T. (2021). Oceanographic processes control dissolved oxygen variability at a commercial Atlantic salmon farm: Application of a real-time sensor network. Aquaculture 533, 736143. doi: 10.1016/j.aquaculture.2020.736143
Burt K., Hamoutene D., Mabrouk G., Lang C., Puestow T., Drover D., et al. (2012). Environmental conditions and occurrence of hypoxia within production cages of Atlantic salmon on the south coast of Newfoundland. Aquacult. Res. 43, 607–620. doi: 10.1111/j.1365-2109.2011.02867.x
Calado R., Mota V. C., Madeira D., Leal M. C. (2021). Summer is coming! Tackling ocean warming in atlantic salmon cage farming. Animals 11 (6), 1800. doi: 10.3390/ani11061800. Article 6.
Dwyer W. P., Piper R. G. (1987). Atlantic salmon growth efficiency as affected by temperature. Prog. Fish. Cult. 49, 57–59. doi: 10.1577/1548-8640
Farrell A. P. (2009). Environment, antecedents and climate change: Lessons from the study of temperature physiology and river migration of salmonids. J. Exp. Biol. 212, 3771–3780. doi: 10.1242/jeb.023671
Føre M., Frank K., Norton T., Svendsen E., Alfredsen J. A., Dempster T., et al. (2018a). Precision fish farming: A new framework to improve production in aquaculture. Biosyst. Eng. 173, 176–193. doi: 10.1016/j.biosystemseng.2017.10.014
Føre M., Svendsen E., Alfredsen J. A., Uglem I., Bloecher N., Sveier H., et al. (2018b). Using acoustic telemetry to monitor the effects of crowding and delousing procedures on farmed Atlantic salmon (Salmo salar). Aquaculture 495, 757–765. doi: 10.1016/j.aquaculture.2018.06.060
Froehlich H. E., Gentry R. R., Halpern B. S. (2018). Global change in marine aquaculture production potential under climate change. Nat. Ecol. Evol. 2 (6), 1745–150. doi: 10.1038/s41559-018-0669-1. Article 11.
Gallant M. J., LeBlanc S., MacCormack T. J., Currie S. (2017). Physiological responses to a short-term, environmentally realistic, acute heat stress in Atlantic salmon, Salmo salar. FACETS 2, 330–341. doi: 10.1139/facets-2016-0053
Gamperl A. K., Ajiboye O. O., Zanuzzo F. S., Sandrelli R. M., Peroni E., de F. C., et al. (2020). The impacts of increasing temperature and moderate hypoxia on the production characteristics, cardiac morphology and haematology of Atlantic Salmon (Salmo salar). Aquaculture 519, 734874. doi: 10.1016/j.aquaculture.2019.734874
Gamperl A. K., Swafford B. L., Rodnick K. J. (2011). Elevated temperature, per se, does not limit the ability of rainbow trout to increase stroke volume. J. Therm. Biol. 36, 7–14. doi: 10.1016/j.jtherbio.2010.08.007
Gamperl A. K., Zrini Z. A., Sandrelli R. M. (2021). Atlantic salmon (Salmo salar) cage-site distribution, behavior, and physiology during a newfoundland heat wave. Front. Physiol. 12. doi: 10.3389/fphys.2021.719594
Handeland S. O., Imsland A. K., Stefansson S. O. (2008). The effect of temperature and fish size on growth, feed intake, food conversion efficiency and stomach evacuation rate of Atlantic salmon post-smolts. Aquaculture 283, 36–42. doi: 10.1016/j.aquaculture.2008.06.042
Hevrøy E. M., Tipsmark C. K., Remø S. C., Hansen T., Fukuda M., Torgersen T., et al. (2015). Role of the GH-IGF-1 system in Atlantic salmon and rainbow trout postsmolts at elevated water temperature. Comp. Biochem. Physiol. A. Mol. Integr. Physiol. 188, 127–138. doi: 10.1016/j.cbpa.2015.06.030
Hevrøy E. M., Waagbø R., Torstensen B. E., Takle H., Stubhaug I., Jørgensen S. M., et al. (2012). Ghrelin is involved in voluntary anorexia in Atlantic salmon raised at elevated sea temperatures. Gen. Comp. Endocrinol. 175, 118–134. doi: 10.1016/j.ygcen.2011.10.007
Huntingford F. A., Adams C., Braithwaite V. A., Kadri S., Pottinger T. G., Sandoe P., et al. (2006). Current issues in fish welfare. J. Fish. Biol. 68, 332–372. doi: 10.1111/j.0022-1112.2006.001046.x
Hvas M., Folkedal O., Imsland A., Oppedal F. (2017). The effect of thermal acclimation on aerobic scope and critical swimming speed in Atlantic salmon, Salmo salar. J. Exp. Biol. 220, 2757–2764. doi: 10.1242/jeb.154021
Hvas M., Folkedal O., Oppedal F. (2020). Heart rate bio-loggers as welfare indicators in Atlantic salmon (Salmo salar) aquaculture. Aquaculture 529, 735630. doi: 10.1016/j.aquaculture.2020.735630
Hyndman R., Athanasopoulos G., Bergmeir C., Caceres G., Chhay L., O’Hara-Wild M., et al. (2024). forecast: Forecasting functions for time series and linear models. R package version 8.23.0. Available online at: https://pkg.robjhyndman.com/forecast/ (accessed September 14, 2023).
Iversen M. H., Eliassen R. A. (2014). The effect of allostatic load on hypothalamic–pituitary–interrenal (HPI) axis before and after secondary vaccination in Atlantic salmon postsmolts (Salmo salar L.). Fish. Physiol. Biochem. 40, 527–538. doi: 10.1007/s10695-013-9863-x
Jones S. R. M., Price D. (2022). Elevated Seawater Temperature and Infection with Neoparamoeba Perurans Exacerbate Complex Gill Disease in Farmed Atlantic Salmon (Salmo salar) in British Columbia, Canada. Microorganism 10 (5), 1039. doi: 10.3390/microorganisms10051039. Article 5.
Kawabe R., Kawano T., Nakano N., Yamashita N., Hiraishi T., Naito Y. (2003). Simultaneous measurement of swimming speed and tail beat activity of free-swimming rainbow trout Oncorhynchus mykiss using an acceleration data-logger. Fish. Sci. 69, 959–965. doi: 10.1046/j.1444-2906.2003.00713.x
Klinger D. H., Levin S. A., Watson J. R. (2017). The growth of finfish in global open-ocean aquaculture under climate change. Proc. R. Soc B. Biol. Sci. 284, 20170834. doi: 10.1098/rspb.2017.0834
Kolarevic J., Aas-Hansen Ø., Espmark Å., Baeverfjord G., Terjesen B. F., Damsgård B. (2016). The use of acoustic acceleration transmitter tags for monitoring of Atlantic salmon swimming activity in recirculating aquaculture systems (RAS). Aquacult. Eng. 72–73, 30–39. doi: 10.1016/j.aquaeng.2016.03.002
Korus J., Filgueira R., Grant J. (2024). Influence of temperature on the behaviour and physiology of Atlantic salmon (Salmo Salar) on a commercial farm. Aquaculture 589, 740978. doi: 10.1016/j.aquaculture.2024.740978
Koskela J., Pirhonen J., Jobling M. (1997). Feed intake, growth rate and body composition of juvenile Baltic salmon exposed to different constant temperatures. Aquacult. Internat. 5, 351–360. doi: 10.1023/A:1018316224253
Lawrence M. J., Wilson B. M., Reid G. K., Hawthorn C., English G., Black M., et al. (2023). The fate of intracoelomic acoustic transmitters in Atlantic salmon (Salmo salar) post-smolts and wider considerations for causal factors driving tag retention and mortality in fishes. Anim. Biotelemetry. 11, 40. doi: 10.1186/s40317-023-00351-0
Madaro A., Olsen R. E., Kristiansen T. S., Ebbesson L. O. E., Nilsen T. O., Flik G., et al. (2015). Stress in Atlantic salmon: Response to unpredictable chronic stress. J. Exp. Biol. 218, 2538–2550. doi: 10.1242/jeb.120535
Mardones J. I., Paredes J., Godoy M., Suarez R., Norambuena L., Vargas V., et al. (2021). Disentangling the environmental processes responsible for the world’s largest farmed fish-killing harmful algal bloom: Chil. Sci. Total. Environ. 766, 144383. doi: 10.1016/j.scitotenv.2020.144383
Martin P., Rancon J., Segura G., Laffont J., Boeuf G., Dufour S. (2012). Experimental study of the influence of photoperiod and temperature on the swimming behaviour of hatchery-reared Atlantic salmon (Salmo salar L.) smolts. Aquaculture 362–363, 200–208. doi: 10.1016/j.aquaculture.2011.11.047
Neubauer P., Andersen K. H. (2019). Thermal performance of fish is explained by an interplay between physiology, behaviour and ecology. Conserv. Physiol. 7, coz025. doi: 10.1093/conphys/coz025
Nilsson J., Moltumyr L., Madaro A., Kristiansen T. S., Gåsnes S. K., Mejdell C. M., et al. (2019). Sudden exposure to warm water causes instant behavioural responses indicative of nociception or pain in Atlantic salmon. Vet. Anim. Sci. 8, 100076. doi: 10.1016/j.vas.2019.100076
Noble C., Gismervik K., Iversen M. H., Kolarevic J., Nilsson J., Stien L. H., et al. (2018). Welfare Indicators for farmed Atlantic salmon: Tools for assessing fish welfare (Tromsø, Norway: Nofima). Available at: http://hdl.handle.net/11250/2575780.
Norambuena F., Rombenso A., Turchini G. M. (2016). Towards the optimization of performance of Atlantic salmon reared at different water temperatures via the manipulation of dietary ARA/EPA ratio. Aquaculture 450, 48–57. doi: 10.1016/j.aquaculture.2015.06.044
Nuez-Ortín W. G., Carter C. G., Nichols P. D., Cooke I. R., Wilson R. (2018). Liver proteome response of pre-harvest Atlantic salmon following exposure to elevated temperature. BMC Genomics 19, 133. doi: 10.1186/s12864-018-4517-0
O’Donncha F., Grant J. (2019). Precision aquaculture. IEEE Internet Things. Magazine. 2, 26–30. doi: 10.1109/IOTM.0001.1900033
O’Donncha F., Stockwell C. L., Planellas S. R., Micallef G., Palmes P., Webb C., et al. (2021). Data driven insight into fish behaviour and their use for precision aquaculture. Front. Anim. Sci. 2. doi: 10.3389/fanim.2021.695054
Oldham T., Oppedal F., Dempster T. (2018). Cage size affects dissolved oxygen distribution in salmon aquaculture. Aquacult. Environ. Interact. 10, 149–156. doi: 10.3354/aei00263
Opinion A. G. R., Vanhomwegen M., De Boeck G., Aerts J. (2023). Long-term stress induced cortisol downregulation, growth reduction and cardiac remodeling in Atlantic salmon. J. Exp. Biol. 226, jeb246504. doi: 10.1242/jeb.246504
Oppedal F., Dempster T., Stien L. H. (2011). Environmental drivers of Atlantic salmon behaviour in sea-cages: A review. Aquaculture 311, 1–18. doi: 10.1016/j.aquaculture.2010.11.020
Reid G. K., Gurney-Smith H. J., Marcogliese D. J., Knowler D., Benfey T., Garber A. F., et al. (2019). Climate change and aquaculture: Considering biological response and resources. Aquacult. Environ. Interact. 11, 569–602. doi: 10.3354/aei00332
Remen M., Oppedal F., Torgersen T., Imsland A. K., Olsen R. E. (2012). Effects of cyclic environmental hypoxia on physiology and feed intake of post-smolt Atlantic salmon: Initial responses and acclimation. Aquaculture 326–329, 148–155. doi: 10.1016/j.aquaculture.2011.11.036
Remen M., Sievers M., Torgersen T., Oppedal F. (2016). The oxygen threshold for maximal feed intake of Atlantic salmon post-smolts is highly temperature-dependent. Aquaculture 464, 582–592. doi: 10.1016/j.aquaculture.2016.07.037
Schraml R., Hofbauer H., Jalilian E., Bekkozhayeva D., Saberioon M., Cisar P., et al. (2021). Towards fish individuality-based aquaculture. IEEE Trans. Ind. Informatics. 17, 4356–4366. doi: 10.1109/TII.2020.3006933
Segner H., Sundh H., Buchmann K., Douxfils J., Sundell K. S., Mathieu C., et al. (2012). Health of farmed fish: Its relation to fish welfare and its utility as welfare indicator. Fish. Physiol. Biochem. 38, 85–105. doi: 10.1007/s10695-011-9517-9
Solstorm D., Oldham T., Solstorm F., Klebert P., Stien L. H., Vågseth T., et al. (2018). Dissolved oxygen variability in a commercial sea-cage exposes farmed Atlantic salmon to growth limiting conditions. Aquaculture 486, 122–129. doi: 10.1016/j.aquaculture.2017.12.008
Stehfest K. M., Carter C. G., McAllister J. D., Ross J. D., Semmens J. M. (2017). Response of Atlantic salmon Salmo salar to temperature and dissolved oxygen extremes established using animal-borne environmental sensors. Sci. Rep. 7, 4545. doi: 10.1038/s41598-017-04806-2
Stockwell C. L., Filgueira R., Grant J. (2021). Determining the effects of environmental events on cultured atlantic salmon behaviour using 3-dimensional acoustic telemetry. Front. Anim. Sci. 2. doi: 10.3389/fanim.2021.701813
Svendsen E., Føre M., Økland F., Gräns A., Hedger R. D., Alfredsen J. A., et al. (2021). Heart rate and swimming activity as stress indicators for Atlantic salmon (Salmo salar). Aquaculture 531, 735804. doi: 10.1016/j.aquaculture.2020.735804
Tromp J. J., Jones P. L., Brown M. S., Donald J. A., Biro P. A., Afonso L. O. B. (2018). Chronic exposure to increased water temperature reveals few impacts on stress physiology and growth responses in juvenile Atlantic salmon. Aquaculture 495, 196–204. doi: 10.1016/j.aquaculture.2018.05.042
Ulvund J. B., Engebretsen S., Alfredsen J. A., Kristensen T., Urke H. A., Jansen P. A. (2021). Behavioural response of farmed Atlantic salmon (Salmo salar L.) to artificial underwater lights: Wavelet analysis of acoustic telemetry data. Aquacult. Eng. 95, 102196. doi: 10.1016/j.aquaeng.2021.102196
Wendelaar Bonga S. E. (1997). The stress response in fish. Physiol. Rev. 77, 591–625. doi: 10.1152/physrev.1997.77.3.591
Keywords: temperature, heart rate, physiology, behaviour, Atlantic salmon, biologger, operations
Citation: Korus J, Filgueira R and Grant J (2024) A case study on the effect of aquaculture operations on the physiology and behaviour of Atlantic salmon (Salmo salar) during two heat events on a commercial farm. Front. Aquac. 3:1428684. doi: 10.3389/faquc.2024.1428684
Received: 06 May 2024; Accepted: 18 November 2024;
Published: 17 December 2024.
Edited by:
Øivind Bergh, Norwegian Institute of Marine Research (IMR), NorwayCopyright © 2024 Korus, Filgueira and Grant. This is an open-access article distributed under the terms of the Creative Commons Attribution License (CC BY). The use, distribution or reproduction in other forums is permitted, provided the original author(s) and the copyright owner(s) are credited and that the original publication in this journal is cited, in accordance with accepted academic practice. No use, distribution or reproduction is permitted which does not comply with these terms.
*Correspondence: Jennie Korus, amVubmllLmtvcnVzQGRhbC5jYQ==