- Biorex Inc., Québec, QC, Canada
There is currently a strong drive to expand aquaculture further offshore co-occurring with a rapid change of the conditions under which this activity will be practiced due to climate change. At the dawn of these profound changes a global review of the current status of technologies used commercially to grow extractive species in wave exposed environments can serve as a benchmark for future developments. Part 1 of this paper presents a systematic inventory of commercial farms in temperate exposed waters. The study area includes 5 regions in the northern hemisphere and 3 regions in the southern hemisphere and covers entirely or part of 48 countries and territories. The inventory is based on 80+ high resolution aquaculture lease maps, most of them available as Internet Web-GIS applications, that cover the entire study area with the exception of a few countries. Exposed sites are first identified from these maps using simple wave fetch criteria and this preselection is then validated using climatological data on wave height and power density (energy flux). The number of sites and the leased area are tallied by region, country, species group and production method. The longline is the production method used in more than 99% of the sites inventoried. Longline design and farm layout in 28 of these sites are reviewed. With a few exceptions, semi-submerged or fully submerged designs are used (in some cases they have been for more than 30 years) while the information on farm layout is patchy. A review of structural damage and loss of cultured biomass due to hydrodynamic forces in commercial and experimental farms confirms that surface and semi-submerged longlines are more vulnerable to large storms than fully-submerged designs.
1 Introduction
In 2013 global aquaculture production (including algae) exceeded global capture fisheries for the first time (FAO, 2022). This remarkable milestone is the result of two major long-term trends: the stagnation of capture fisheries since the mid-1990s and the 24-fold increase of eastern Asia aquaculture production from 1980 to 2020. However, the growth rate of aquaculture production peaked in 1996 and has considerably decreased since (Sumaila et al., 2022). In some cases, production has actually decreased since the mid-1990s such as bivalves in Europe (Avdelas et al., 2021), bivalves and seaweed in Japan (Watanabe and Sakami, 2021) and scallops in Chile (von Brand et al., 2016). Kelp (order Laminariales) aquaculture production is presently insignificant outside of Asia (< 1,000 t total; FAO, 2023a) despite recent developments in northern Europe and North America. The World Bank (2013) estimated that global demand for fish and seafood for human consumption would increase by 36% from 2006 to 2030 and that aquaculture needs to fill the 40 million tonnes gap. As for seaweeds, there is a global 12 billion US$ potential for new markets including biofuels and bioplastics (World Bank, 2023).
In 2013, the FAO introduced the Blue Growth Initiative to promote sustainable mariculture development in response to the growing demand for seafood and seaweed and ensure global food security. This agenda has been adopted by the European Union, OECD and World Bank (Massa et al., 2017). Several countries have implemented this initiative through marine spatial planning and the creation of allocated zones for aquaculture (AZAs) with the objectives of reserving space for mariculture, reducing user conflicts and environmental impacts and speeding-up the leasing/permitting process (FAO, 2013; Sanchez-Jerez et al., 2016; Macias et al., 2019; Morris et al., 2021; Wang et al., 2022). In temperate waters, most of the space available for mariculture in sheltered areas (estuaries, lagoons, fjords and enclosed bays) is already occupied. Expansion is only possible in more exposed sites. Moreover, in several sheltered areas the carrying capacity has been exceeded and part of the production is moving farther offshore to reduce the density of farming operations (Mille and Blachier, 2009; Komatsu et al., 2016; Wang et al., 2022). There is also increasing pressure from other coastal users and regulators to move existing nearshore farms farther offshore (Wang et al., 2022). For these reasons, newly created AZAs are mostly situated in exposed sites away from conflicting uses. Another important opportunity for mariculture expansion is its co-location with marine renewable energy farms which are, by definition, situated in high energy environments. For example, it is projected that the installed capacity of offshore wind farms will increase 15- to 24-fold between 2018 and 2040 and that these farms will occupy 47,000 to 73,000 km2 of exposed waters, mainly in China, Europe and northeastern USA (IEA, 2019).
Co-occurring with this strong drive for exposed waters, climate change will have a significant impact on the conditions in which mariculture will develop in the coming decades (Cubillo et al., 2021; Hu et al., 2021; Liu et al., 2024). More specifically, the IPCC (2022) predicts for the second half of the 21st century an increase of the average sea temperature and of the frequency, duration and intensity of marine heat waves in all regions as well as an increase of the mean wave energy and extreme wave heights in several regions. At the dawn of these profound changes, a global review of the technologies currently used by commercial farms in high energy environments can be useful for the industry and the R&D community and serve as a benchmark for future development.
There is no consensus on the definition of “open-ocean”, “offshore”, “off-the-coast” or “exposed” mariculture (Kapetsky and Aguilar-Manjarrez, 2007; Lovatelli et al., 2013; Froehlich et al., 2017; Bak et al., 2020; Howarth et al., 2022; ICES, 2023). The criteria used to classify sites are usually a combination of the distance to nearest coastline or port, water depth, current velocity, wave height and wind speed with various thresholds. Consequently, the published lists of such sites (Cheney et al., 2010; Ögmundarson et al., 2011; Buck and Langan, 2017; Galparsoro et al., 2020; Howarth et al., 2022; ICES, 2012, 2023; Fujita et al., 2023) vary considerably. Several reviews of the technological aspects of offshore/exposed extractive species aquaculture have been published since 2010 (Cheney et al., 2010; Ögmundarson et al., 2011; Fernand et al., 2017; Buck et al., 2017, 2018; Goseberg et al., 2017; Bak et al., 2020; Heasman et al., 2020; Tullberg et al., 2022; Saether et al., 2024). Most of these reviews focus on case studies or on experimental/pilot technology as opposed to commercial practice.
Extractive species are those that do not require nutrient/feed input during the at-sea grow-out phase. In temperate waters almost 100% of mariculture production of these non-fed species is for the following three groups: kelps (order Laminariales), bivalve molluscs (mussels, oysters and scallops) and tunicates (FAO, 2023a). They are prime candidates for offshore expansion and their grow-out has much lower adverse effects on the environment than fish farms (Buck et al., 2017; Mascorda Cabre et al., 2021; Fujita et al., 2023). Clawson et al. (2022) carried out a global inventory of commercial mariculture farms. They estimated the number of farms per country based on aquaculture lease maps or, when not available, by dividing the national production by the estimated average production per farm. This study excluded kelp and tunicate farms and made no distinction between sheltered and exposed farms and the production methods used. Harvey et al. (2024) compared the density of longline and raft farming (presumably bivalves and macroalgae) between parts of China, Chile, Japan, South Korea and Vietnam based on the random sampling of Google Earth imagery. This study was limited to nearshore areas with a water depth of less than 15 m. At the national and sub-national levels, aquaculture geographic information systems (Supplementary Table S3) make no distinction between sheltered and exposed sites. This is also the case for China-wide mariculture mapping exercises based on satellite imagery recently published (Liu et al., 2022a; Jin et al., 2023).
In this paper, I carry-out a systematic global inventory of extractive species commercial farms in exposed temperate waters based on high resolution aquaculture lease maps (HRALMs). The inventory is limited to temperate marine waters for the following reasons: there is no aquaculture in polar/sub-polar regions (Oyinlola et al., 2018; Clawson et al., 2022); temperate open waters are characterized by much higher wave energy than tropical/subtropical waters (Arinaga and Cheung, 2012); and information on the exact location of farms in the tropical/subtropical regions is lacking for most countries (Clawson et al., 2022) while, as we will see below, coverage is almost complete in temperate waters. I then review longline design and farm layout for the exposed sites for which the information is available. Finally, I review the information available on structural damage and cultured biomass loss in longline farms caused by hydrodynamic forces.
2 Methodology
2.1 Study area
The study area is limited to brackish and marine waters where the mean annual sea surface temperature (SST) is between 5 and 20°C. These limits correspond roughly to the global distribution of blue mussels (Mytilus sp.; Gaitan-Espitia et al., 2016; Hilbish et al., 2000) and kelps (order Laminariales; Steneck et al., 2002). The study area was subdivided into eight large regions (Figure 1): Atlantic Northeast (ANE), Atlantic Northwest (ANW), Mediterranean and Black seas (MBS), Pacific Northeast (PNE), Pacific Northwest (PNW), Temperate South America (TSAM), Temperate South Africa (TSAF) and Temperate Australasia (TAA). The list of countries and country subdivisions included in each region is given in Supplementary Table S1. The sources of the SST climatologies used to delimit the study area and of other global oceanic variables used to characterize each region are given in Supplementary Table S2.
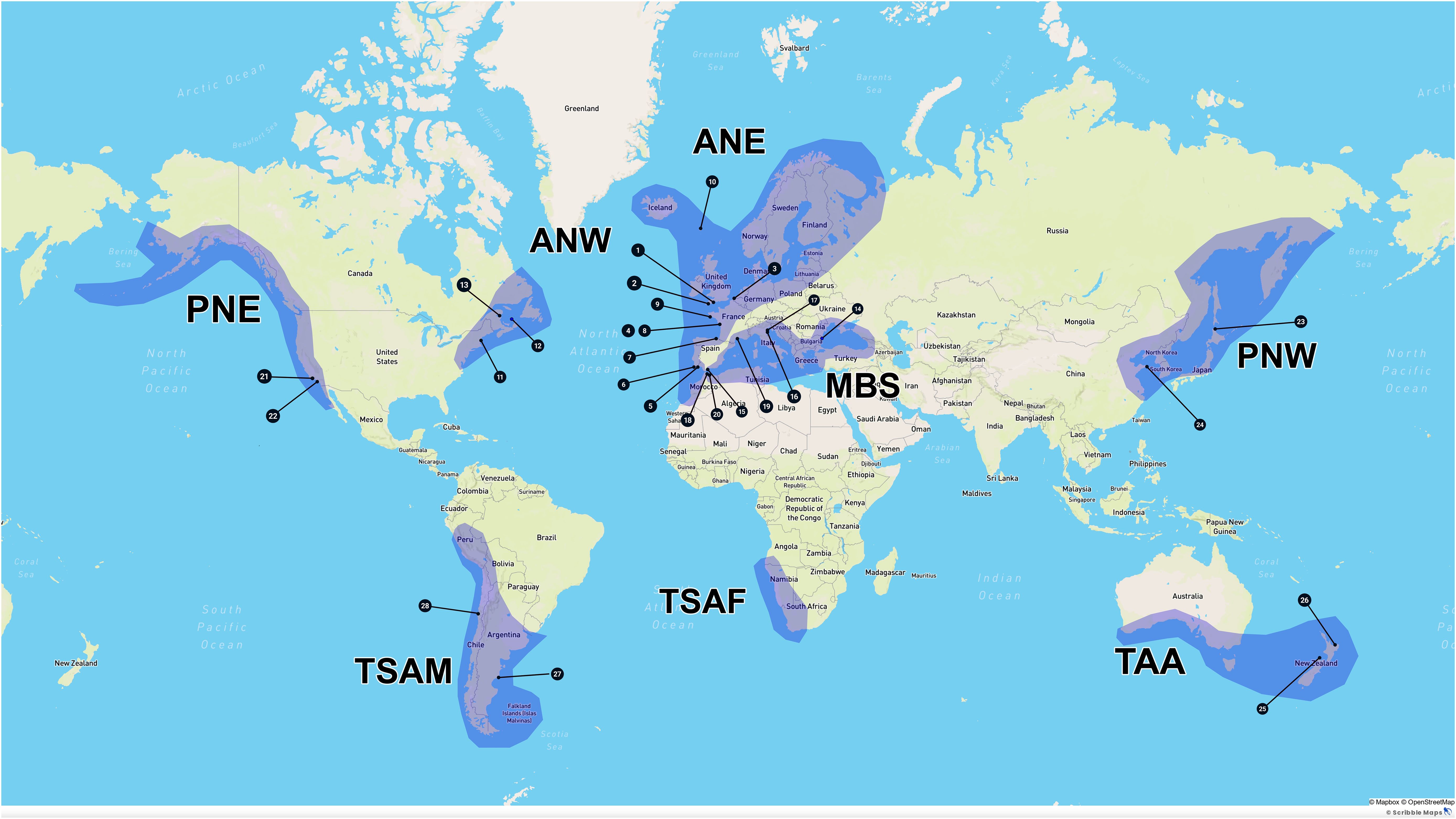
Figure 1. Limits of the eight large regions (shaded areas) which make up the study area and position of the 28 exposed sites for which detailed information on longline design and farm layout is available.
2.2 Exposed farm identification
Identification of exposed farms was based on high-resolution interactive or static aquaculture lease maps (HRALMs) available on the Internet. The extended list of the 80+ HRALMs which cover roughly 95% of the study area is provided in Supplementary Table S3. A large majority of the HRALMs are interactive Web-GIS applications or KML files readable on Google Earth that provide more or less details on individual leases. The criteria used to screen the thousands of aquaculture leases appearing on these HRALMs are 1) the type of lease (commercial and active), 2) the cultured species (extractive, non-fed), 3) the culture method (suspended, off-bottom) and 4) wave exposure (exposed sites). Inactive, proposed, under review, experimental and pilot leases were not retained. The main mariculture extractive species in temperate waters are listed in Table 1. Abalone, sea cucumbers and urchins farms were excluded from this category. Intertidal, pole, trestle, table and on-bottom (sea ranching) farms were not retained.
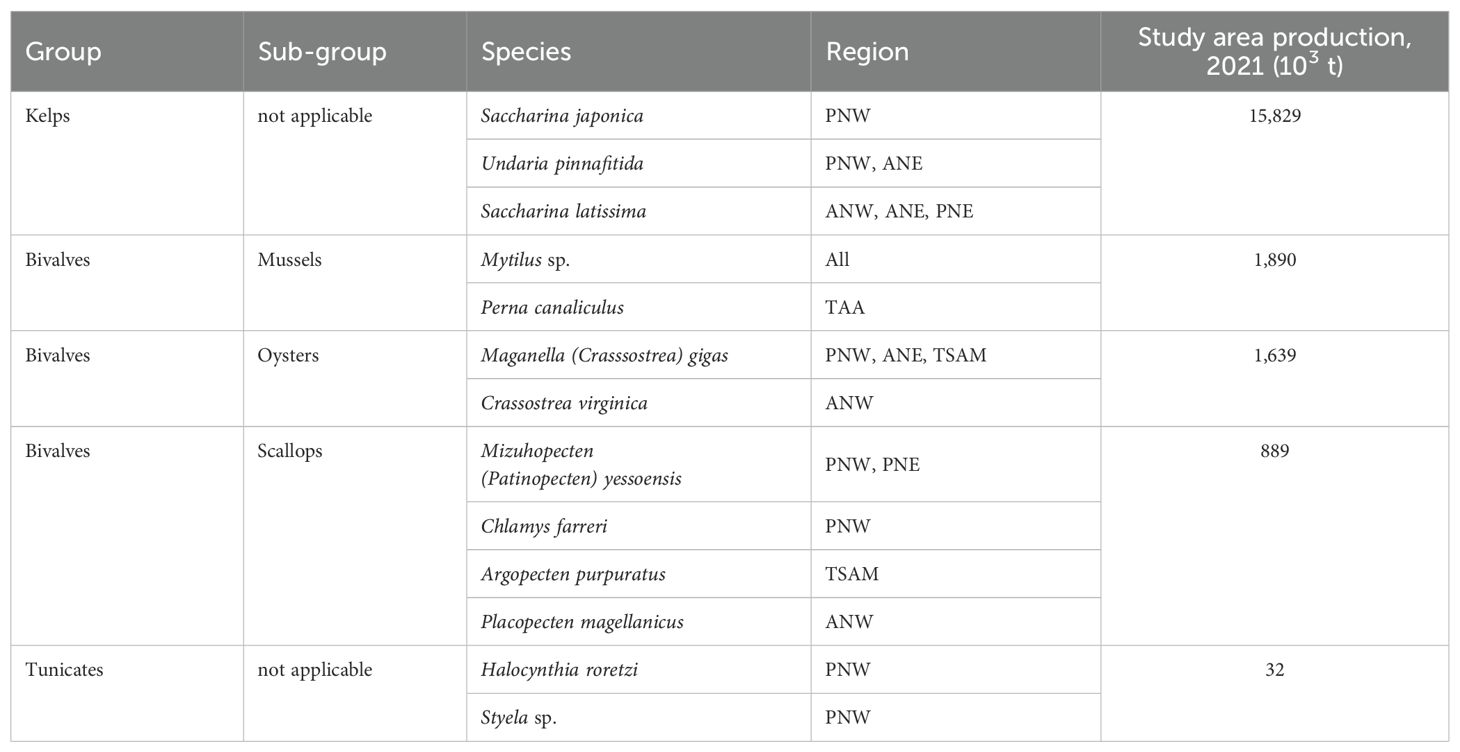
Table 1. Main temperate marine extractive species cultured off-bottom (FAO, 2023a).
Wave exposure was the only criteria used to distinguish between exposed and sheltered sites. This selection was made in two steps. In a first step, the following fetch criteria were used to preselect sites: 1) the maximum fetch of the site is longer than 150 km; and 2) the window of continuous fetch longer than 20 km is wider than 45° and includes the maximum fetch direction. This was easily evaluated and, for sites near the thresholds, measured directly on the maps. The fetch criteria used above provide only a rough estimate of wave exposure because they do not take into account the direction of the prevailing winds and swells. In a second step, wave climatologies (Supplementary Table S4) were used to validate the preselection. Examination of these maps indicated that the above fetch thresholds corresponded with one or more of the following wave thresholds: 1) annual mean significant wave height (SWH) > 0.5 m; 2) 99th percentile of SWH > 2.2 m; 3) 50-year-return-period SWH > 4.0 m; and 4) annual mean wave power density (WPD; synonym: wave energy flux) > 1.5 kW/m. The 50-year-return-period SWH is the standard extreme wave height used to design floating aquaculture facilities (Norway NS9415 Standard, 2009). The hourly WPD is proportional to SWH2T (where T is the wave period). For a given site, when the classification given by the four variables was contradictory, the one given by the variable with the highest spatial resolution was retained. For a small number of preselected sites the wave exposure thresholds were not exceeded and these sites were deleted from the compilation (e.g. Thermaikos Gulf, Greece and northeastern Adriatic Sea, Italy). One well documented site in the Faroe Islands where the maximum fetch is only 10 km was not preselected but was added to the final tally because the 50-year-return-period SWH exceeds 4 m. Preselected sites that could not be confirmed for lack of high-resolution wave climatologies were kept in the compilation.
Countries or country subdivisions for which comprehensive HRALMs were not available are: Albania, China, Falkland Islands, Georgia, Monaco, North Korea, Romania, Russian Black Sea, Tunisia, Turkey and Ukraine (outside Crimea). In addition, the Russian Far East presented a special case discussed in Section 3.2.1. In all other cases the identification of exposed sites was carried out using the following approach. First, the FishStatJ database (FAO, 2023a) was consulted and countries/country subdivisions with less than 50 t of bivalve, tunicate and kelp production were eliminated (Falkland Islands, Georgia, Monaco, Romania and Ukraine, outside Crimea). Secondly, for the remaining countries and country subdivisions, National Aquaculture Sector Overviews (NASO; FAO, 2023b) were consulted and those where all kelp, bivalve or tunicate farms were determined as sheltered after checking the wave fetch criteria on Google Earth and wave climatologies where eliminated (Albania and Tunisia). For the remaining countries/subdivisions, governmental documents and technical and academic literature that relate to the geographic position of existing farms were consulted. This allowed an estimation of the number of exposed farms in Krasnodar Krai (Russia) and Turkey. At this stage, information was missing for China and North Korea. The method used to estimate the extent (km2) of exposed farms in these two countries is described in Section 3.2.1.
2.3 Inventory metrics
Results are summarized per country or territory in each of the 8 regions of the study area using three metrics: 1) number of sites (total and per species group), 2) total leased area (ha), and 3) percentage of sites that use longlines. A “site” refers to a single isolated lease or a group of several active leases in an allocated zone for aquaculture (AZA). The leased area includes the actual space occupied by the production structures, navigational channels and buffer zones around the structures and any undeveloped part of the lease. When more than one species group was listed for a site, the site was assigned to the first group listed. Bivalve sub-groups (oysters, mussels and scallops) were not distinguished because many sites grow more than one sub-group. Longlines consist of long horizontal ropes supported by buoys (floats) individually anchored to the sea bed at both ends or in arrays of several parallel ropes anchored by a grid of anchors.
2.4 Longline design and farm layout characterization
Details on longline (LL) design and farm layout was available for some of the exposed sites inventoried. The environment of each site was characterized by the following variables: region, location, water body, year established, leased area, distance to nearest coastline, water depth and wave exposure. For the latter, the criteria and thresholds presented in Table 2 were used to classify each site as moderately exposed, fully exposed or very exposed. The variables used to characterize LL design and farm layout are: LL type, mainline length and depth, mooring and anchoring configuration, LL (for bivalves) or kelp-line (for kelps) orientation relative to currents and waves, and farm density. Farm density (m of mainline/ha) was calculated as the planned/allowed maximum number of LLs multiplied by average mainline length (m) and divided by leased area (ha). Description of LL design is limited to those used for the grow-out phase; spat catching LLs are not covered. The terminology used in this part and the rest of the paper is given in Tables 3–5 and, in the cases of Tables 3 and 4, illustrated in Figure 2. The major sources of information are leasing or permitting documents, technical reports, academic literature and company websites (Supplementary Table S5). For more details on the various LL components and designs, see Bompais (1991), Langan et al. (2010), Ögmundarson et al. (2011), Bonardelli (2013), Flavin et al. (2013), Goseberg et al. (2017) and Bonardelli et al. (2019).
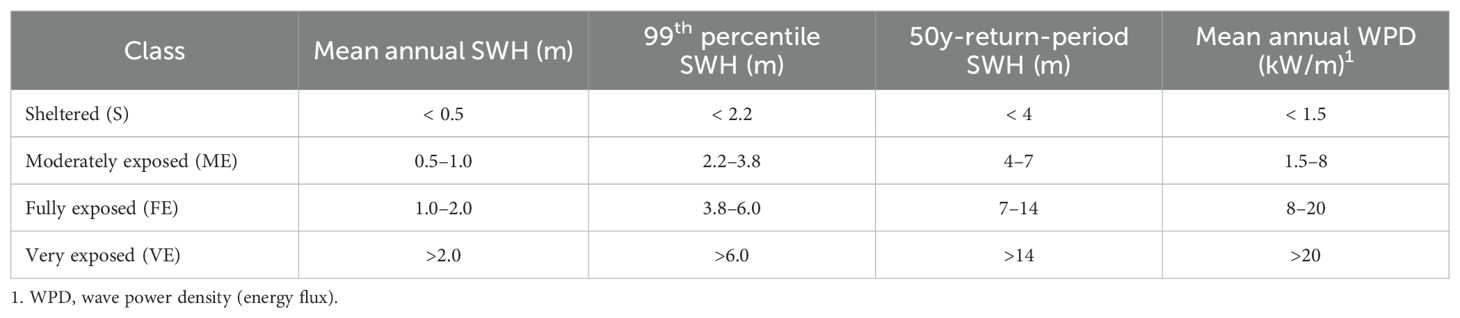
Table 2. Wave exposure classification. A site is assigned to the highest wave exposure class for which at least one of the four criteria is met.
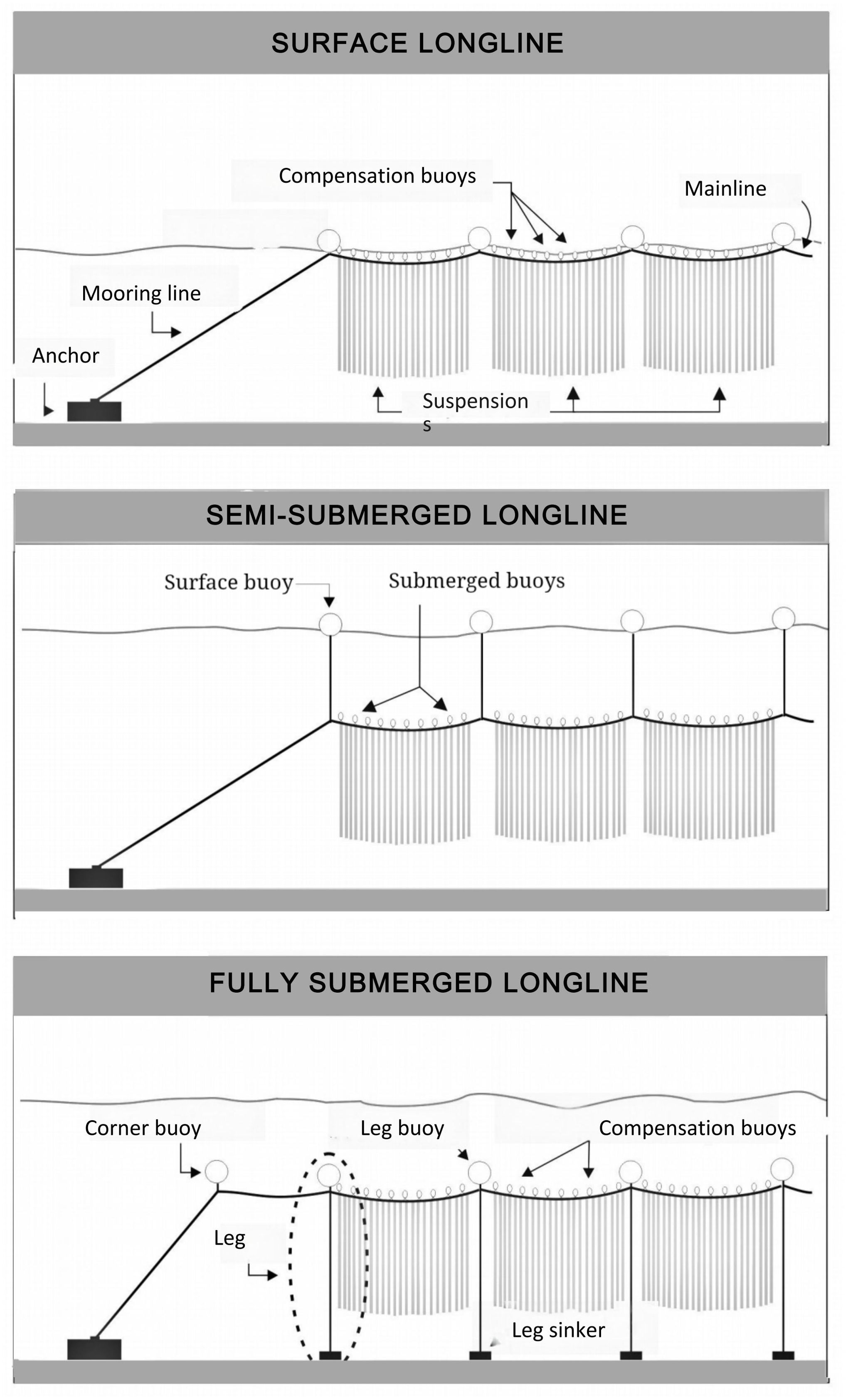
Figure 2. Schematic presentation of the three main types of longlines and their components (not to scale).
2.5 Structural damage and cultured biomass loss characterization
A review of available information on structural damage and cultured biomass loss due to hydrodynamic forces for commercial and experimental LLs was carried-out in order to compare the actual suitability of the various types of LLS relative to their level of exposure. The sources of this type of information were technical reports, academic literature and the media.
3 Results
3.1 Regional oceanic conditions
Large marginal seas in the PNW (Bohai, Yellow, Japan and Okhotsk seas), ANW (Gulf of St. Lawrence), ANE (White, North, Baltic, Irish and Celtic seas) and the MBS Region have relatively reduced wave exposure compared to areas were the coasts overlook directly the Pacific, Atlantic or Indian oceans. The pole-ward part of all regions except MBS is situated in the global extra-tropical storm belts where wave energy is at its maximum. The west facing coasts in these belts (e.g. Alaska, Ireland, southern Chile, Tasmania and New Zealand) are the most exposed areas to winter storms in the world. Late summer tropical cyclones (typhoons and hurricanes) are more frequent in the southern part of the PNW and ANW regions. All the Pacific Ocean coasts (PNW, PNE, Peru, Chile and New Zealand) are vulnerable to tsunamis.
The tidal range does not exceed 4 m except in limited macro-tidal areas in the PNW (Jiangsu, China and western Korea), PNE (Alaska), ANW (Bay of Fundy), ANE (White, Celtic and Irish seas, English Channel and Brittany) and TSAM (southern Argentina). The largest micro-tidal areas (tidal range < 2m) are the Sea of Japan (PNW) and the MBS Region. Maximum tidal currents do not exceed 0.6 m/s except in the macro-tidal areas listed above and in straits (e.g. Gibraltar, (Spain and Morocco), Cook, (NZ)). There is no sea ice present in the PNE, TSAM, TSAF and TAA regions but it is usually present during winter in the Bohai Sea, northern Sea of Japan and the Sea of Okhotsk (PNW), Gulf of St. Lawrence and northern Newfoundland (ANW), White and Baltic seas (ANE) and northern Black Sea (MBS). The four major global coastal upwelling systems (CUS) are situated in the study area: along the southern coast of PNE (California Current) and ANE (Canary-Iberia CUS), the Pacific coast of the TSAM region (Peruvian-Chilean CUS) and in the TSAF Region (Benguela Current).
3.2 Global inventory
A summary of the global inventory is presented in Table 6. Information is missing for North Korea, Russian Far East and Russian Black Sea and only a rough estimate of the exposed farmed area was possible for China. Excluding these four countries and country subdivisions, a total of 392 kelp, 299 bivalve and 172 tunicate sites were inventoried. In the case of sites for which the culture method is known, 99.4% use longlines, only 3 sites use surface rigid rafts and one site uses surface long-tubes. There are currently no exposed farms in countries, states or provinces where hundreds of sheltered farms exist. These include Ireland, Scotland (UK), western Sweden, Norway, Tasmania (Australia), southern Chile, Alaska and Maine (USA), British Columbia, Newfoundland, Nova Scotia and Prince Edward Island (Canada). Each region is reviewed separately below.
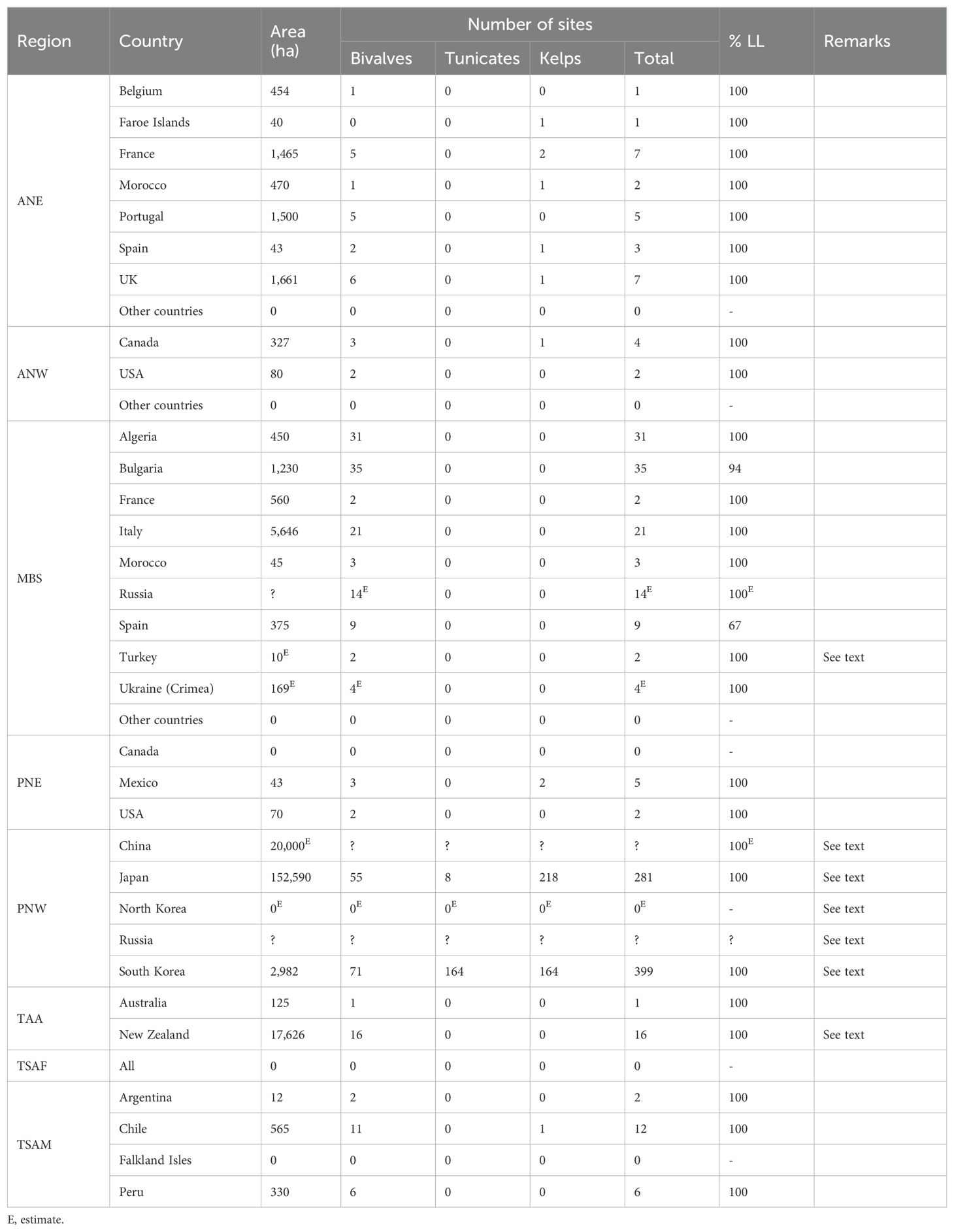
Table 6. Total area and number of exposed sites in the study area and percentage of sites using longlines (% LL) per region and country.
3.2.1 Northwest Pacific
At least 74% of the total farming area (ha) inventoried are situated in the PNW region. The overwhelming importance of this region is not surprising knowing that it accounts for over 99% of the kelp, 72% of the bivalve and 100% of the tunicate production (sheltered + exposed) of all temperate countries (FAO, 2023a). Due to this overwhelming importance, each country is examined separately below.
In the case of China, HRALMs at the national or provincial levels are not available. The Chinese Statistical Fishery Yearbook provides the area farmed by species and province but does not distinguish between sheltered and exposed sites (Wang et al., 2022). For these reasons, it was not possible to obtain a precise estimate of the total area of exposed farms in temperate China. An indirect approach was used for the country subdivisions included in the study area. Almost 100% of the kelp production in China comes from the culture of kombu (S. japonica) and wakame (U. pinnatifida). The total area occupied by farms in China in 2015 for these two species was 436 km2 and 69 km2, respectively (Zheng et al., 2019). Liu et al. (2019) estimates that 30% of the kombu farming area (133 km2 in 2015) is located more than 11 km from the coastline in more than 20 m water depth, mostly in the following three counties: Rongcheng and Shangdao (Shandong) and Lushun (Liaoning). According to Zheng et al. (2019) almost all the wakame production in China comes from the study area. Rongcheng County at the eastern tip of the Shandong Peninsula is the only zone in the Chinese part of the study area where the thresholds for wave height and wave power are exceeded within 10 km of the coast (He and Xu, 2016; Jiang et al., 2016; Dong et al., 2020). This 300 km long peninsula juts into the center of the Yellow Sea. In Rongcheng County there is a succession of open bays (Rongcheng, Yangyuchi, Ailian, Heini) and the semi-enclosed Sanggou Bay that constitute the epicenter of kombu longline farming in China (Liu et al., 2022a; Jin et al., 2023). The total area of exposed farming in these open bays and in the area offshore Sanggou Bay can be estimated at roughly 200 km2. It is likely that 100% of this area is used solely for longline kombu farming from fall to following spring. The high-density longline fields clearly visible on Google Earth extend up to 14.5 km from the inner bay shore into the Yellow Sea. In the southern half of Rongcheng County including Sanggou Bay, remote sensing based mapping shows that the exposed culture area increased roughly eight-fold between 1990 and 2018 (Wang et al., 2022).
In Japan the estimated total area of exposed sites amounts to over 1,500 km2 which is by far the largest area of any country. It is likely that nearly 100% of these sites use LLs. Three exposed zones can be distinguished based on the main cultured species: 1) Hokkaido dominated by scallop culture (M. yessoensis), 2) Aomori Prefecture dominated by tunicate culture (H. roretzi), and 3) the rest of northern Honshu dominated by wakame (U. pinnatifida) and kombu (S. japonica) cultivation. In the case of scallop culture around Hokkaido, there are two exposed sub-zones based on the scallop culture technique: 1a) Sea of Okhotsk where longlines are used for spat catching and the intermediate culture in large leases before juveniles are sowed on the bottom and harvested by dredges (bottom culture areas are not included in the inventory) and 1b) the rest of Hokkaido where scallops are grown on LLs for all phases (Andrews et al., 2013).
In South Korea exposed sites are concentrated along the eastern coast (Sea of Japan). The average size of the farms is quite small. In terms of total area occupied, they are dominated by scallops (northeast), tunicates (central) and kelp (southeast).
HRALMs are not available for North Korea and other information on the location of aquaculture farms is very scarce. Available statistics on production (FAO, 2023a) are unreliable estimates but they indicate that extractive species culture in this country is only a very small fraction of that of South Korea, Japan and China. It is likely that the number and area of exposed farms is negligible in this country.
In the Russian Far East, according to the aquaculture leasing web application Aquavostok (2023), over 700 km2 of exposed aquaculture space have been leased and is “in use”. Most of this space was leased after 2015 and is located along the coasts of the Primorsky Krai (Sea of Japan). As a result, the total production of kelp, mollusks, echinoderms and salmon in this zone increased ten-fold between 2015 and 2021 (FAO, 2023a). Information on the cultured species and production method is not available for individual sites. Since these sites can be used to grow non-extractive species (salmon, abalone, sea urchin) and for on-bottom scallop culture, it was not possible to obtain a reliable estimate of the number and extent of sites in this sub-region.
3.2.2 Northeast Pacific
The farms are situated in the Southern California Bight (USA) and along the Pacific coast of the state of Baja California (Mexico). These farms grow the Mediterranean mussel (M. galloprovincialis) and the giant kelp (Macrocystis sp.) on longlines and were established after 2004.
3.2.3 Northwest Atlantic
The farms are situated in the Gulf of St. Lawrence (Canada) and along the New England coast (USA). These are used to cultivate the blue mussel (M. edulis) and the sugar kelp (S. latissima) on LLs and were established after 2005.
3.2.4 Northeast Atlantic
The farms are dispersed from the North Sea to Algarve, Portugal. The main species cultured are the blue mussel (Mytilus sp.), the Pacific oyster (M. gigas) and the sugar kelp (S. latissima) on LLs. The oldest farm was established in the Pertuis Breton (France) in 1991 while most of the others were established after 2006.
3.2.5 Mediterranean and Black Seas
Exposed farms in this region grow the Mediterranean mussel (M. galloprovincialis) on LLs. Some also grow or condition oysters. There are no tunicate and kelp farms in the region. The farms in France and Italy were established in the mid-1980s, those in Spain and Bulgaria in the late 1990s and 2000s and those in Crimea (Ukraine), Krasnodar Krai (Russia), Turkey and Morocco after 2015. Four AZAs (total area: 4,200 ha) were created in the 1980s for mussel culture in the exposed waters off the Occitanie coast (France). In the early 1990s there were over 2,000 ha leased producing 8,000 t of mussels annually using LLs (Danioux et al., 2000). Due to heavy spat predation by fishes, many of the leases were abandoned or used to condition oysters grown in coastal lagoons (Cepralmar, 2017). HRALMs were not available for Turkey. The only extractive species cultured in this country is the Mediterranean mussel (FAO, 2023a). Mussel production increased from virtually zero to 4,500 t between 2015 and 2021. The farms are all situated in the sheltered waters of the Aegean and Marmara seas (Balci Akova, 2015; Yildirim, 2021) except for two new LL farms established in 2022 off the exposed Black Sea coast (Gucukluoglu, 2022). HRALMs are not available for the Krasnodar Krai (Russia). Other sources indicate that there are at least 14 exposed mussel (M. galloprovincialis) and oyster (M. gigas) farms along the Russian Black Sea coast, most of them established after 2019.
3.2.6 Temperate South America
Except for one site used for giant kelp culture (Macrocystis sp.), the exposed sites in Chile and Peru are used to grow the purple scallop (A. purpuratus) on LLs. In Chile, all sites are along the northern coast. The oldest farms were established in the 1980s. In Argentina, two sites were established after 2009 and are used to grow blue mussels (Mytilus sp.) on LLs.
3.2.7 Temperate South Africa
All shellfish farms are located in sheltered sites and there are no kelp and tunicate farms in this region.
3.2.8 Temperate Australasia
The main species cultured are the blue mussel (Mytilus sp.) in Australia and the green-lipped mussel (P. canaliculus) in New Zealand. All farms were established after 2000 and use LLs. In New Zealand, more than 60% of the 176 km2 of exposed area consist of very large leases (400 to 3,800 ha each) that are partly or not yet developed. Another 25% are only used for bivalve spat catching during summer on a rotational basis (TDC, 2019).
3.3 Longline design and farm layout
Detailed information on LL design and farm layout was available for only 28 of the exposed sites inventoried and in several of these cases information on farm layout is lacking. Tables 7 and 8 present a summary for these sites listed by region and by the main species cultured, respectively. The sites cover a wide range of locations (7 of the 8 regions), year of establishment (1980–2023) and size (8–7,500 ha). Roughly 75% are situated less than 5 km from the coast and in water depths of less than 30 m and all are situated less than 11 km from the coast in water depths of less than 70 m. Twenty-four sites are classified as moderately exposed, 4 as fully exposed and none as very exposed. The fully exposed sites are # 7 (Basque Country, Spain), # 9 (southern Brittany, France), # 11 (New Hampshire, USA) and # 26 (Bay of Plenty, NZ). Twenty-one sites mainly grow mussels, 4 grow kelp, two grow scallops and one grows oysters. The information on LL design and farm layout is summarized below by cultured species.
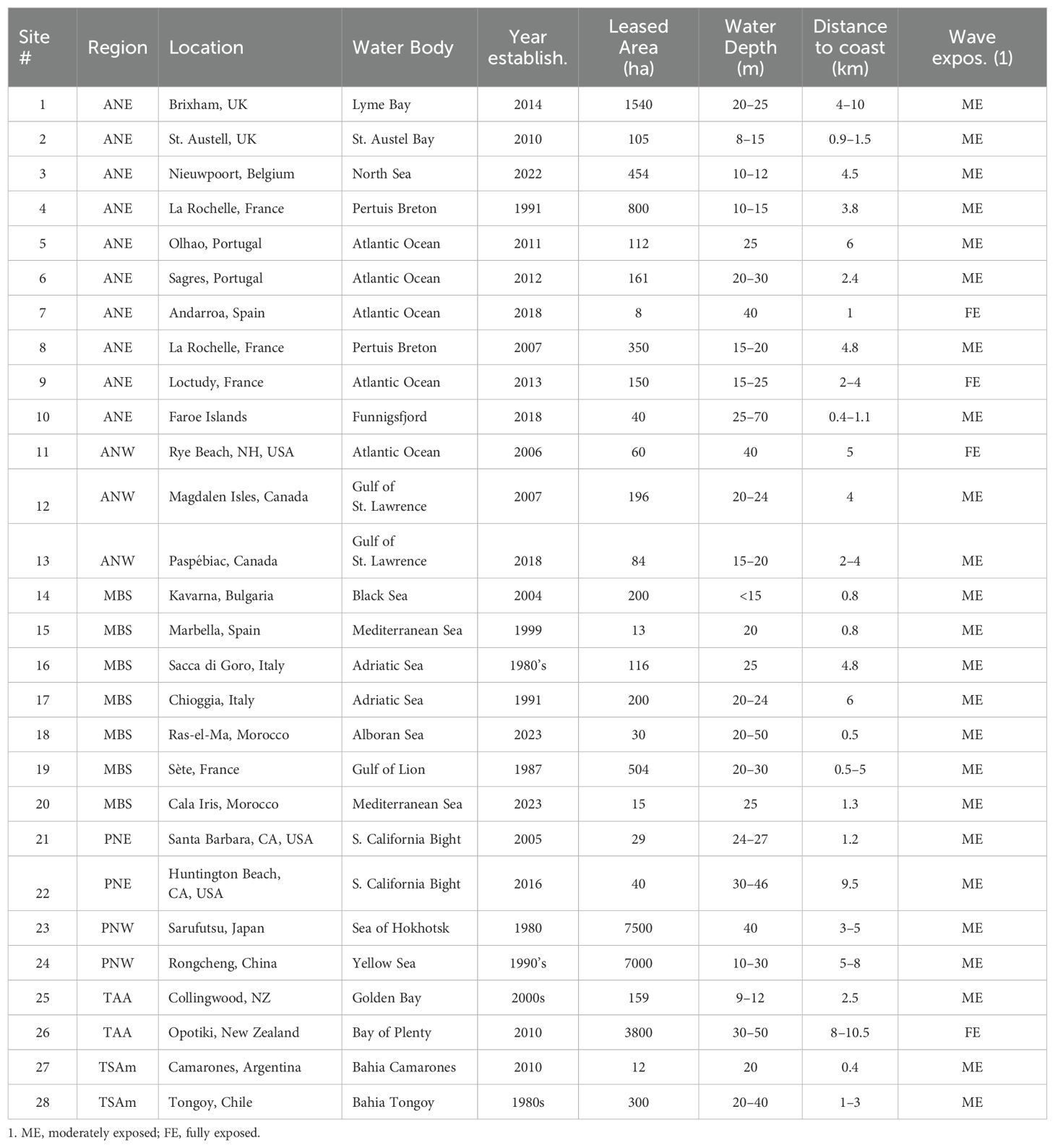
Table 7. Situation of the 28 exposed sites for which details on longline design and farm layout are available.
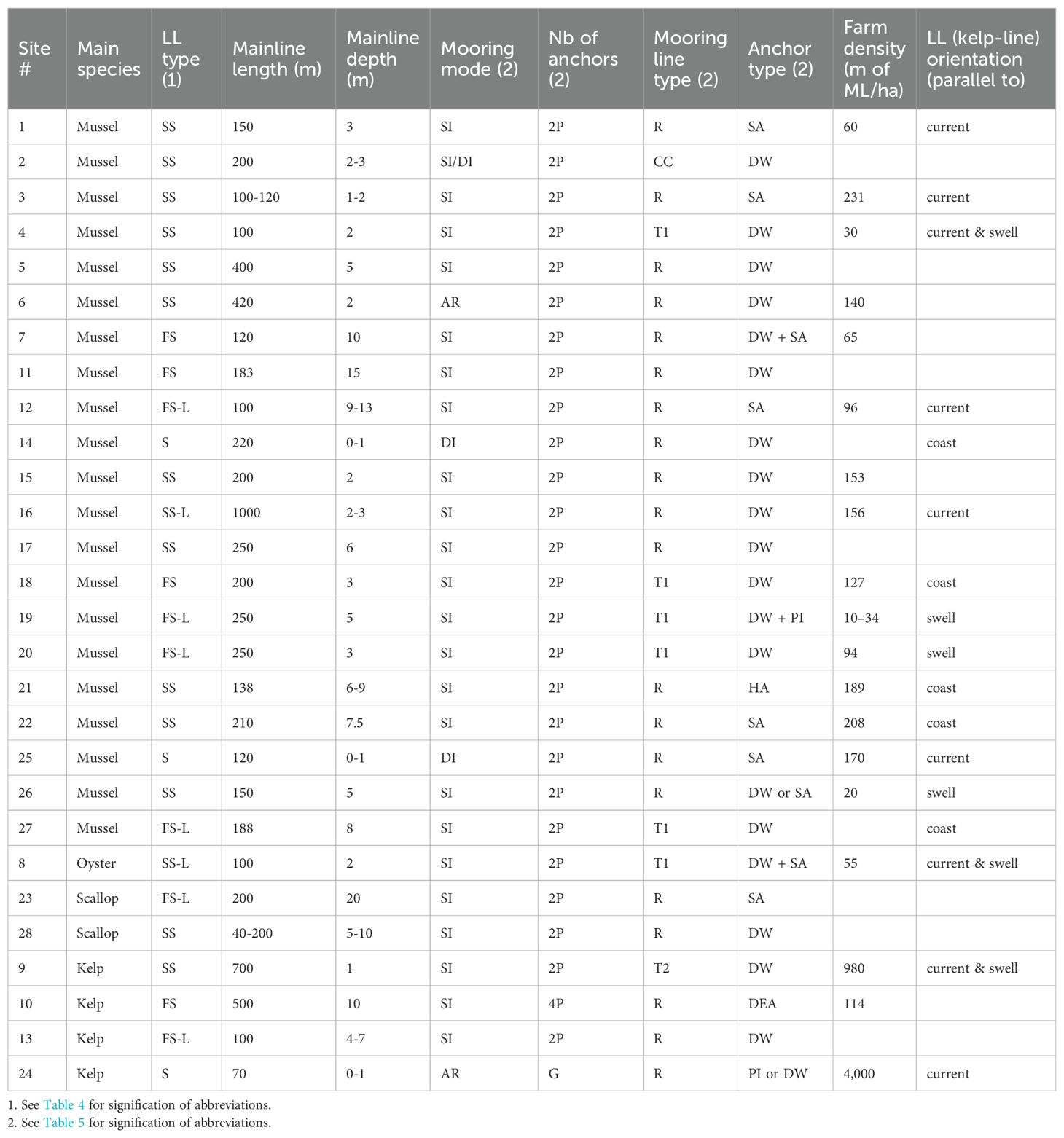
Table 8. Longline design and farm layout of the 28 exposed sites of Table 7.
3.3.1 Mussel and oyster farms
Most mussel and oyster farms use individually anchored semi-submerged or fully submerged LLs. ML length varies between 100 and 1,000 m and its depth varies between 1 and 15 m, the deepest in the fully exposed sites. The 1,000 m semi-submerged LLs in Site # 16 are fitted with legs every 75 m along the ML. In most sites the simplest mooring and anchoring system is used: 2-point, single rope and deadweight anchors. Farm density (m of ML/ha) decreases with increasing lease area mainly because large leases are subdivided into blocks of LLs separated by wide navigational channels and buffer zones. In most of the moderately exposed sites, LLs are oriented parallel to the currents or the coast (and presumably to the currents). In four moderately exposed sites and two fully exposed sites (# 9 and # 26), they are oriented parallel to swell propagation.
3.3.2 Scallop farms
The two scallop sites are situated in northern Hokkaido (Sea of Okhostk) for spat catching and intermediate culture of the Japanese scallop (M. yessoensis) and northern Chile for all phases of culture of the purple scallop (A. purpuratus). There are several published descriptions of the scallop LLs used in Japan but most of these focus on sheltered areas (Taguchi, 1977; Ventilla, 1982; Beal, 1999; Kosaka, 2016). There are no standard LL design and farm layout; they depend on the culture phase (spat collection, intermediate culture or final grow-out), available lease area and degree of exposure to waves, currents and drifting sea ice. Longlines may be anchored individually or in arrays of several LLs as large as 16 ha. Along the exposed coast of the Sea of Okhotsk the Sarufutsu cooperative (Site # 23) uses fully-submerged LLs for the intermediate culture phase. The ML is maintained 20 m below the sea surface by a combination of surface floats and legs spaced at 25–50 m intervals (Lucien-Brun and Lachaux, 1983). Tongoy Bay (Site # 28) is the main scallop culture site in Chile. The LLs used there are semi-submerged with the ML maintained between 5 and 10 m below the sea surface depending on the water depth.
3.3.3 Kelp farms
The four kelp farms in Table 8 show that there is currently no standard design to grow this species group in exposed sites. Arrays are used in the moderately exposed area offshore Sanggou Bay, China (Site # 24). Each array is composed of 4 or 5, 70–100 m long surface LLs individually anchored 4 m apart with kelp-lines attached horizontally between two adjacent LLs at 1–2 m intervals. The use of surface LLs in this moderately exposed site is likely possible because of wave attenuation by the very high farm density (Liu and Zhang, 2022). In the moderately exposed Quebec farm (Site # 13), individually anchored fully submerged LLs with legs are used. The ML is maintained 7 m below the sea surface in winter because of drift ice and is raised to a depth of 4 m, below the surface freshet, in spring. The kelp-line is attached parallel to the ML and 1 m below it. In the fully exposed farm in southern Brittany (Site # 9), semi-submerged LLs 700 m long with legs attached at 100 m intervals are used. The density of this farm is also very high due to the small distance between LLs (10 m). In the Faroe Islands moderately exposed farm (Site # 10), individually anchored fully-submerged LLs without legs are used and the 500 m long ML is maintained 10 m below the surface. The kelp-lines are 10 m long vertical ropes attached at 1–2 m intervals to the ML and fitted with a small buoy at their free end that maintains the kelp floating above the ML in the surface water layer.
3.3.4 Tunicate farms
No detailed information was found for individual tunicate farms. Generally, tunicates (H. roretzi and Styela clava) are grown on LLs similar to those used for mussels. The tunicates attach to ropes and form vertically hanging droppers similar in shape and size to mussel droppers (Lambert et al., 2016).
3.4 Structural damage and loss of cultured biomass
3.4.1 Structural damage
LLs in sheltered sites are vulnerable to extreme storms. For example, an extreme post-tropical storm (Fiona) severely damaged LL farms in sheltered sites in the Gulf of St. Lawrence, Canada in 2022 (CBC, 2022). In the main sheltered mussel farming area in New Zealand (Marlborough Sounds), between 500 and 1,500 buoys are lost every year due to rough weather (MPI, 2021). In moderately exposed sites, large extra-tropical storms caused severe damage to semi-submerged LLs along the northwestern Adriatic coast, Italy, in 2010 and 2017 (Vianello, 2013; Mistri and Munari, 2019), in the Pertuis Breton, France, in 2008 (Site # 8; Mille and Blachier, 2009), in Tongoy Bay, Chile, in 2015 (Site # 28; Bakit et al., 2022), in Tasman Bay, New Zealand, in 2021 (Gee, 2021) and to surface kelp LLs in Rongcheng County (Site # 24), China (Liu et al., 2019). Eyrolles et al. (2018) report structural damage to semi-submerged LLS in a Brittany fully exposed farm (site # 9) due to waves and vessels. In November 2023 an extreme storm destroyed most of the semi-submerged farms along the moderately exposed Crimean and Russian Black Sea coast (PROyugAgro, 2024). However, I found no report of structural damage in the case of fully submerged LLs in commercial farms except for the Occitanie AZA (Site # 19), France where deadweight anchors slipped during a 1990 winter storm (Bompais, 1991). In most areas the response to hydrodynamic damage was to increase the pressure resistance of the buoys, the strength of buoy attachments, the size of the ropes and the holding capacity of the anchors with various combinations: screw anchors, multiple in-line deadweights, deadweight plus drag embedment anchors or piles (Ensor, 2011; Bompais, 1991; Blachier, 2011; Mille and Le Moine, 2011; Lee et al., 2015).
In the case of experimental LLs, their performance in exposed sites depended on their type. Long-tubes and surface longlines failed completely and were deemed unsuitable for exposed sites (Mueller-Fuega and Bompais, 1989; Buck and Buchholz, 2004; Daly, 2007; Minnhagen et al., 2019). Semi-submerged and fully submerged LLs without legs performed well except when the submerged buoys did not resist the hydrostatic pressure and imploded and when buoyancy adjustments could not be made in time before they collapsed to the sea bottom. Some were destroyed by a hurricane, fishing vessels or an unknown cause (Pajot, 1989; Paul and Grosenbaugh, 2000; Langan (2002); Langan and Horton, 2003; Buck, 2007; Lindell, 2015; Minnhagen et al., 2019; Bonardelli et al., 2019; Lona et al., 2020). Fully submerged LLs with legs had no major problems (Karayücel et al., 2015; Bourque and Myrand, 2014; Id Halla et al., 2017) except for a poorly designed configuration in Sweden (Bonardelli et al., 2019). Metal connections (shackles, thimbles, swivels, rings) are often the weak point of the design and they should be avoided (Buck, 2007; Bonardelli et al., 2019; Bak et al., 2020).
Although large tsunamis have a return period of several decades, some of them have been responsible for severe or catastrophic damage to longlines recently in Japan, Chile and New Zealand. Farms in sheltered bays along the Pacific coast of northern Honshu, Japan, were severely damaged by a tsunami in 2010 (Kato et al., 2010) and completely destroyed by the Great Eastern Japan (Tohuku) tsunami of March 2011 (Suppasri et al., 2018). In Tongoy Bay, Chile (Site # 28), scallop farms were severely damaged by the 2011 Japanese tsunami and again in 2015 (Bakit et al., 2022). Farms in some sheltered sites in New Zealand were damaged by tsunamis in 2004 and 2010 (Ensor, 2011). After the large tsunamis of 1960, 2010 and 2011 on the Pacific coast of Japan, the level of damage to LLs was much higher in sheltered areas than in open ocean sites and was not related to sea surface elevation but rather to current velocity; LLs were destroyed when it exceeded 1.0-1.2 m/s (Kato et al., 2010; Suppasri et al., 2018). On the open coast this velocity is rarely attained during a tsunami in areas where the water is deeper than 65 m and consequently open deep waters are a refuge from tsunamis for surface structures (Lynett et al., 2014).
3.4.2 Loss of cultured biomass caused by hydrodynamic forcing
Several mechanical interactions may cause significant losses of the cultured biomass on LLs, mainly in the case of mussels and kelps that grow attached to ropes without containment. The attachment strength of individual S. latissima blades to ropes depends on the origin of the seedlings; those coming from exposed sites are strong enough to withstand high drag forces and the sheltering effect between kelp blades on a LL further reduces the probability of being dislodged (Buck and Buchholz, 2005; Chen et al., 2023). This species is cultivated at high densities (hundreds of plants per m of kelp-line) and the losses due to hydrodynamic forces are masked by the natural self-thinning process that considerably reduces plant density during the grow-out cycle (Kerrison et al., 2017). In the case of S. japonica in Sanggou Bay, China (Site # 24), which is cultivated at low densities (20 plants/m), Zhang et al. (2011) report that 16% of the kelp plants were dislodged mostly during winter and 4% of the blades were broken mostly at the end of the grow-out cycle while Liu et al. (2019; 2022b) report a high level of seedling fall-off (up to 50%) and blade breakage (up to 70%) in the outermost exposed areas off Sanggou Bay because the cultivars used were not developed for high energy environments. In late Spring or mid-Summer depending on the latitude, heavy fouling of the kelp blade starts, kelp tissue deteriorates and breakage increases rapidly; biomass loss can reach 50% by late summer and almost 100% in late fall (Gendron and Tamigneaux, 2008; Fieler et al., 2021; Skjermo et al., 2020). This is why in most areas kelp is harvested in late spring before heavy fouling starts.
In the case of mussel droppers, the important variable appears to be the bulk force with which the mussel matrix attaches to the rope rather than the attachment strength of individual mussels (Gagnon, 2019). This force decreases (or does not increase enough) as the mussels grow and fully grown droppers are prone to sloughing (fall-off) if there are snap loads in the dropper line. Wu et al. (2021 and 2024) report severe mussel sloughing near harvest time on surface LLs in Shengsi County, China, due to passing tropical storms. In some farming areas, sloughing is mitigated by inserting short vertical pegs through the dropper line at roughly 20 cm intervals (Çelik et al., 2015). Friction between adjacent droppers because their linear weight varies along the ML may cause erosion of the mussel matrix (Bompais, 1991). The use of continuous droppers where the dropper forms loops under the ML reduces the likelihood of this happening. When the distance between the LLs is small (< 10 m) and the pretension is not the same in all MLs, friction between the MLs erodes the kelp (Flavin et al., 2013) and mussel matrix (Bompais, 1991). In large waves perpendicular or oblique to the ML, kelp blades attached directly to the ML (Zhu, 2020) and mussel droppers (Lien and Fredheim, 2001) may roll-over the ML. This may reduce the attachment strength of the kelp and mussel matrix.
When contained in pearl nets and lantern nets, oysters and particularly scallops are very sensitive to the acceleration and inclination of their enclosures by currents and waves (Oshino, 2010; Natsuike et al., 2022; Goseberg et al., 2017; Campbell and Gray, 2023). Scallop mortality may increase by 25% and growth decrease by 20% in enclosures coupled with a surface buoy compared to those coupled with a buoy submerged 6.5 m below the sea surface (Freites et al., 1999). Similarly, survival may increase by 34% and growth by 50% when the scallops are artificially attached to the lantern net compared to when they are free to move inside (Ventilla, 1982). In the case of the ear-hanging method, where the scallops are not enclosed but rather tied by a hole drilled through their shell to dropper lines, it is mostly limited to sheltered sites (Ventilla, 1982).
4 Discussion and conclusion
The two main constraints to the expansion of mariculture in wave exposed environments is the distance between the farm and the servicing port and the intensity of the hydrodynamic forces acting on the aquaculture structures and servicing vessel. The first constraint is mainly economic (operational costs increase with distance to port and remote operations and monitoring are expensive) while the second one has economic (capital costs), technological (structure design), logistical (operational window), biological (survival, retention, growth and quality of the cultured biomass) and human health & safety aspects. The ICES Working Group on Open Ocean Aquaculture (ICES, 2023) has recently developed hydrodynamic exposure indices to standardize the characterization of existing and future aquaculture sites based on metocean data relative to current and wave energy. These indices will be published soon.
Considering that several thousands of aquaculture sites had to be classified as sheltered or exposed for the systematic inventory carried-out in this paper, simple wave fetch criteria easy to apply on HRALMs were used to preselect exposed sites. Wave climatological data, when available, were then used to finalize the selection. Climatological data based on in situ measurements (i.e. wave buoys) in or close to commercial farms are very scarce. The final selection was mostly based on maps (Supplementary Table S4) produced from wind-wave models applied at intermediate (0.02–0.2°) to high (50–500 m) spatial resolution (Guillou et al., 2020). These maps do not cover completely the study area and each source maps only one or two of the four variables retained for the selection. For several sites they provided contradictory results as to whether they are sheltered or exposed. More weight was given to high resolution maps in the determination of the exposure level of such sites. These sites are also likely to experience intense currents (> 1.0 m/s) and winds (> 25 m/s) during large storms. This approach is less complex than that used by Lader et al. (2017) to classify the 1,070 salmon farms registered in Norway with respect to their exposure to wind seas (swells excluded). Their 3-step methodology includes for each site, 1) a detailed fetch analysis, 2) construction of time series of wave height and period estimates based on wind and fetch data, and 3) extremal analysis. Their results show that only 1.1% of the salmon farms have a continuous 40-km-fetch window wider than 45° and none of the sites has a 50-year-return-period wave height larger than 4 m. This is consistent with the fact that with the approach I used, all the 230 bivalve and kelp farms in Norway were classified as sheltered.
The number of exposed bivalve sites in the study area (excluding China, Russia and North Korea) represents 2% of the total number of farms (sheltered + exposed) that Clawson et al. (2022) estimated for the same area. In the case of kelp sites, the total number of farms (sheltered + exposed) in the study area is unknown. The area of exposed kelp farming I estimated for temperate China (200 km2) corresponds to 43% of the total area of kelp farming in the same provinces (Jin et al., 2023). There are some caveats regarding the interpretation of the results of this inventory. Since the selected sites cover a very wide range of sizes (8 to 7000 ha), the total leased area gives a better idea of the contribution of each country to global production than the total number of sites. However, the relative importance of each country in terms of leased area can also be misleading as the proportion of the leased area occupied by LLs decreases with increasing lease area and the development of very large leases is staged over several years. Currently, non-fed off-bottom mariculture in exposed sites is concentrated in the PNW Region, mainly in Japan and China. In the former country LLs have been used for more than fifty years to grow scallops while in China, exposed sites are used to grow kelp in very high density LL fields since the 1990s. Outside the PNW, exposed farms are currently very scarce in countries or country subdivisions benefiting from extensive areas of sheltered sites like Ireland, Scotland, Tasmania, Canada, Alaska, Maine and southern Chile. Exposed farms exist since the 1980s in France, Italy and northern Chile. Several exposed farms have been established after 2010 in all regions except Temperate South Africa.
Predicting how mariculture in exposed sites will evolve in the future is out of the scope of the present paper. It will depend on several factors including technological and biological (genetic) developments, economical feasibility, market demand, government policy and climate change. In the case of the latter factor it should be possible to model how much suitable areas will be gained or lost for each cultured species using future sea state conditions based on IPCC scenarios. For instance, will mussel culture still be possible in the MBS Region in 2050 (Cubillo et al., 2021). Given that things are currently changing quite rapidly due to climate change and the strong momentum for offshore expansion, this inventory should be considered a snapshot of the early 2020s that can eventually be used later as a benchmark to measure what has actually been gained or lost. Climate change will have a significant effect on the extent of the temperate regions and exposed areas as defined in this paper. More specifically, the IPCC (2022) predicts for the second half of 21th century a pole-ward migration of the 5° and 20° SST isotherms used in this paper to delimit the study area and it is likely that some of the sites not included in this inventory will exceed the wave height and power density thresholds used to identify exposed sites.
With a few exceptions, the exposed farms in the present inventory are located in environments that are less energetic than where offshore wind and wave farms are or will be established in the next decade (4C Offshore, 2023). For example, the average annual wave power density in the North Sea wind farms varies between 4 kW/m off Belgium and 33 kW/m off Norway (Beels et al., 2007; Rusu and Rusu, 2021).
The best sources of information on LL design and farm layout are leasing/permitting documents produced by governmental authorities and applicants, but these documents are very scarce on the Internet. Peer-reviewed articles and company websites rarely provide complete information on specific farms, likely to protect sensitive commercial information. For most of those for which the information is available, semi-submerged or fully submerged LL designs were adopted. These were first developed in Japan in the 1970s and adapted commercially in the 1980s or early 1990s in France (Bompais, 1991; Mille and Blachier, 2009), eastern Canada (Bonardelli, 1996) and Italy (Danioux et al., 2000) using a trial and error approach. Fully submerged LLs were successfully experimented in exposed sites for up to five years (Paul and Grosenbaugh, 2000; Langan and Horton, 2003; Karayücel et al., 2015; Bourque and Myrand, 2014; Id Halla et al., 2017; Mizuta and Wikfors, 2019). Although it is vulnerable to extreme storms like any marine structure, this technology has proven its suitability for farming in fully exposed environments for more than 30 years. The question remains whether it is suitable for very exposed environments where there are plans to co-locate them with marine renewable energy farms. In the past 10 years, several R&D programs have terminated or been initiated to determine the feasibility of bivalve and kelp farming in wind and wave farms and other very exposed sites (Table 9). Currently used designs, new designs based on the basic longline and other technologies have or will be tested. In Part 2 of this article (Gagnon, 2024), I review the loading and motion of LLs in currents and waves and during husbandry operations and I compare the advantages and disadvantages of the various LL types.
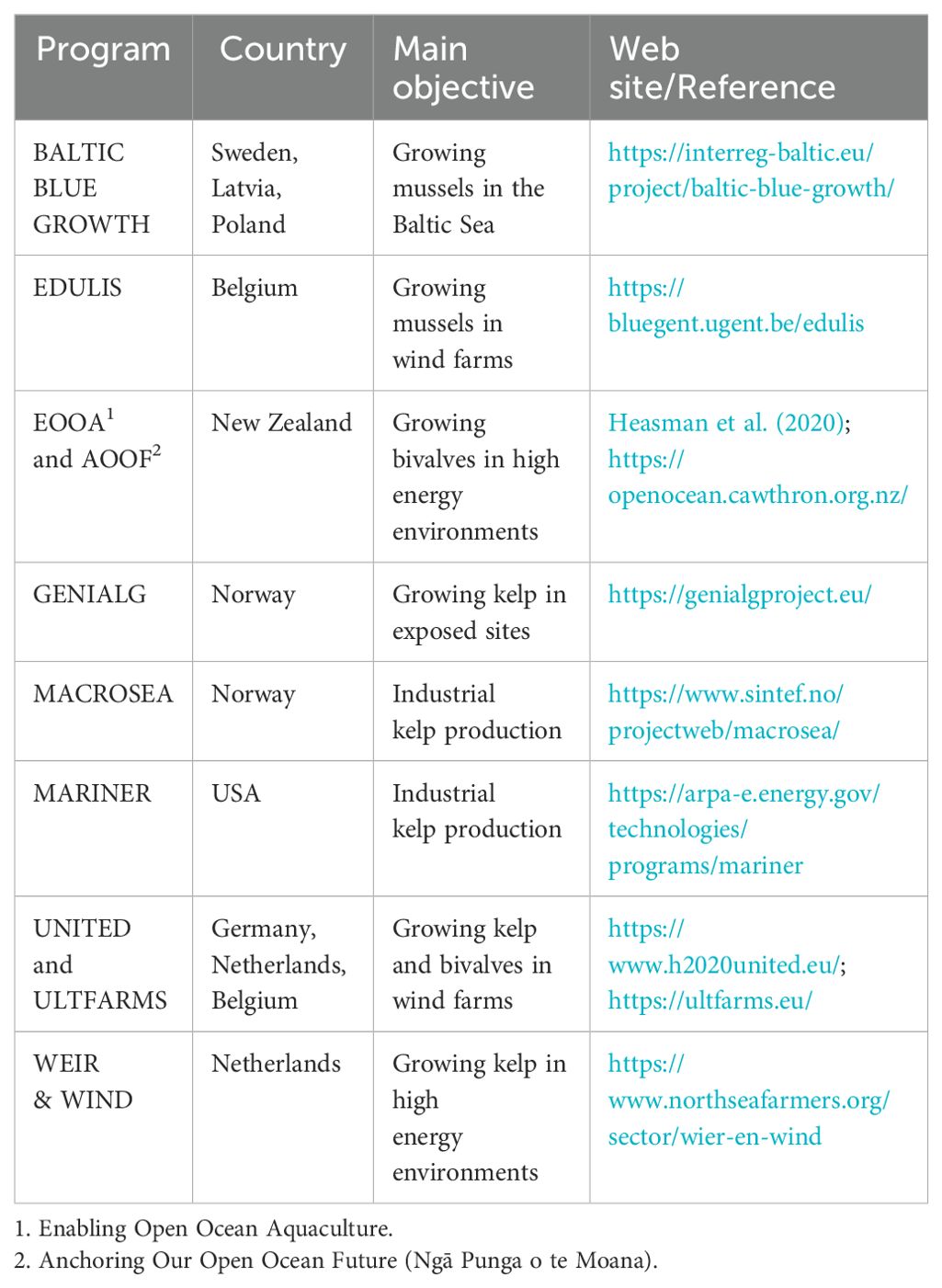
Table 9. List of R&D programs focusing on kelp and bivalve farming in high energy environments (terminated or initiated between 2014 and 2024).
Data availability statement
The raw data supporting the conclusions of this article will be made available by the authors, without undue reservation.
Author contributions
MG: Conceptualization, Formal analysis, Methodology, Writing – original draft, Writing – review & editing.
Funding
The author(s) declare that no financial support was received for the research, authorship, and/or publication of this article.
Acknowledgments
The authors wish to thank the reviewers for their helpful comments and suggestions.
Conflict of interest
Author MG was employed by the company Biorex Inc.
Publisher’s note
All claims expressed in this article are solely those of the authors and do not necessarily represent those of their affiliated organizations, or those of the publisher, the editors and the reviewers. Any product that may be evaluated in this article, or claim that may be made by its manufacturer, is not guaranteed or endorsed by the publisher.
Supplementary material
The Supplementary Material for this article can be found online at: https://www.frontiersin.org/articles/10.3389/faquc.2024.1411749/full#supplementary-material
References
4C Offshore (2023). Global offshore windfarms map. Available online at: https://map.4coffshore.com/offshorewind/ (Accessed March 15, 2024).
Andrews J., Akroyd J., Hough A., Kimura P. (2013). MSC assessment report for Japanese scallop hanging and seabed enhanced fisheries. Version 5: Public Certification Report (Moody Marine Ltd). 284 p.
Aquavostok (2023). Available online at: https://aquavostok.ru/#project (Accessed December 12, 2023).
Arinaga R. A., Cheung K. F. (2012). Atlas of global wave energy from 10 years of reanalysis and hindcast data. Renew. Energy 39, 49–64. doi: 10.1016/j.renene.2011.06.039
Avdelas L., Avdic-Mravlje E., Marques A. C. B., Cano S., Capelle J. J., Carvalho N., et al. (2021). The decline of mussel aquaculture in the European Union: causes, economic impacts and opportunities. Rev. Aquacult. 13, 91–118. doi: 10.1111/raq.12465
Bak U. G., Gregersen Ó., Infante J. (2020). Technical challenges for offshore cultivation of kelp species: lessons learned and future directions. Bot. Mar. 63, 341–353. doi: 10.1515/bot-2019-0005
Bakit J., Alvarez G., Diaz P. A., Uribe E., Sfeir R., Villasante S., et al. (2022). Disentangling environmental, economic, and technological factors driving scallop (Argopecten purpuratus) aquaculture in Chile. Fishes 7, 380. doi: 10.3390/fishes7060380
Balci Akova S. (2015). Aquaculture and its distribution in Turkey. J. Aquacult. Eng. Fish. Res. 1, 160–190. doi: 10.3153/JAEFR15018
Beal B. F. (1999) in The culture of Japanese scallops (Patinopecten yessoensis): report of the Maine delegation to Aomori Prefecture, Japan, May 14-21, 1999. (Orono, ME, USA: The Maine Aquaculture Innovation Center). Publ. 99–01.
Beels C., Henriques J. C. C., De Rouck J., Pontes M. T., De Backer G., Verhaeghe H. (2007). “Wave energy resource in the North Sea. n,” in Proceedings of the European Wave and Tidal Energy Conference (EWTEC), Porto, Portugal, 11–13 September 2007. doi: 10.13140/2.1.4640.6403
Blachier P. (2011). Éléments de reflexion pour le choix d’un ancrage adapté aux filières ostréicoles: compte rendu des essais réalisés en mer en 2009 (Elements of reflection for choosing an anchorage adapted to oyster longlines: report of the tests carried out at sea in 2009) (Château d'Oléron, France: Centre régional d’Expérimentation et d’Application aquacole (CREAA). 22 p.
Bompais X. (1991). Mussel growing longlines: a practical guide (Les filières pour l’élevage des moules: guide pratique) (Institut français de recherche pour l’exploitation de la mer (Brest, France: IFREMER). 247 p.
Bonardelli J. C. (1996). “Longline shellfish culture in exposed and drift-ice environments,” in Proc. Open Ocean Aquacult. Int. Conf, Portland, Maine, 1996. 235–252. (University of New Hampshire/University of Maine Sea Grant College Program), 1996 - 642 pages.
Bonardelli J. C. (2013). Technical and practical requirements for Baltic mussel culture. Rep. Aquabest Project 4. Project 4, 215 p. ISBN 978-952-303-049-7
Bonardelli J. C., Kokaine L., Ozolina Z., Aigars J., Purina I., Persson P., et al. (2019). Technical evaluation of submerged mussel farms in the Baltic Sea (Report of the Baltic Blue Growth project, WP3, GoA3.4.). 74 p.
Bourque F., Myrand B. (2014). Potentiel de production mytilicole en milieu ouvert aux Îles-de-la-Madeleine (Open ocean mussel production potential in the Magdalen Islands), Merinov R&D Rep. 14–09, 33 p.
Buck B. H. (2007). Experimental trials on the feasibility of offshore seed production of the mussel Mytilus edulis in the German Bight: installation, technical requirements and environmental conditions. Helgoland Mar. Res. 61, 87–101. doi: 10.1007/s10152-006-0056-1
Buck B. H., Buchholz C. M. (2004). The offshore-ring: a new system design for the open ocean aquaculture of macroalgae. J. Appl. Phycol. 16, 355–368. doi: 10.1023/B:JAPH.0000047947.96231.ea
Buck B. H., Buchholz C. M. (2005). Response of offshore cultivated Laminaria saccharina to hydrodynamic forcing in the North Sea. Aquaculture 250, 674–691. doi: 10.1016/j.aquaculture.2005.04.062
Buck B. H., Langan R. (2017). Aquaculture perspective of multi-use sites in the open ocean: the untapped potential for marine resources in the Anthropocene (Cham: Springer).
Buck B. H., Nevejan N., Wille M., Chambers M., Chopin T. (2017). “Offshore and multi-use aquaculture with extractive species: seaweeds and bivalves,” in Aquaculture perspective of multi-use sites in the open ocean: the untapped potential for marine resources in the Anthropocene. Eds. Buck B. H., Langan R. (Springer), 23–69. doi: 10.1007/978-3-319-51159-7_2
Buck B. H., Troell M. F., Krause G., Angel D. L., Grote B., Chopin T. (2018). State of the art and challenges for offshore integrated multi-trophic aquaculture (IMTA). Front. Mar. Sci. 5. doi: 10.3389/fmars.2018.00165
Campbell B., Gray M. W. (2023). Evaluating the influence of cage motion on the growth and shell characteristics of oysters (Crassostrea virginica) among several gear types. Aquaculture 579, 740223. doi: 10.1016/j.aquaculture.2023.740223
CBC (2022). Fiona's widespread devastation needs flexible response from government, P.E.I. farmers say (CBC News).
Çelik M. Y., Karayücel S., Karayücel I., Eyüboğlu B., Öztürk R. (2015). Settlement and growth of the mussels (Mytilus galloprovincialis, Lamarck 1819) on different collectors suspended from an offshore submerged longline system in the black Sea. Aquacult. Res. 47, 3765–3776. doi: 10.1111/are.12827
Cepralmar (2017). La conchyliculture en Occitanie 2016-2017 (Shellfish culture in Occitanie 2016-2017) (Sète, France: Centre d’étude pour la promotion des activités lagunaires et maritimes, Fact Sheet).
Chen M., Yim S. C., Cox D. T., Yang Z., Huesemann M. H., Mumford T. F., et al. (2023). Modeling and analysis of a novel offshore binary species free-floating longline macroalgal farming system. J. Offshore Mech. Arct. Eng. 145, 021301. doi: 10.1115/1.4055803
Cheney D., Langan R., Heasman K., Friedman B., Davis J. (2010). Shellfish culture in the open ocean: lessons learned for offshore expansion. Mar. Technol. Soc J. 44, 55–67. doi: 10.4031/MTSJ.44.3.6
Clawson G., Kuempel C. D., Frazier M., Blasco G., Cottrell R. S., Froehlich H. E., et al. (2022). Mapping the distribution of global mariculture production. Aquaculture 553, 738066. doi: 10.1016/j.aquaculture.2022.738066
Cubillo A. M., Ferreira J. G., Lencart-Silva J., Taylor N. G. H., Kennerley A., Guilder J., et al. (2021). Direct effects of climate change on productivity of European aquaculture. Aquacult. Int. 29, 1561–1590. doi: 10.1007/s10499-021-00694-6
Daly T. (2007). Moving mussels offshore. Available online at: https://slideplayer.com/slide/6151158/ (Accessed March 13, 2024). Presentation Sept 24, 2007.
Danioux C., Bompais X., Paquotte P., Loste C. (2000). “Offshore mollusc production in the Mediterranean Basin,” in Mediterranean offshore aquaculture. Eds. Muir J., Barsurco B. (Montpellier, France: CIHEAM), 115–140.
Dong J., Shi J., Zhao J. C., Zhang C., Xu H. (2020). Wave energy assessment in the Bohai Sea and the Yellow Sea based on a 40-year hindcast. Water 12, 2087. doi: 10.3390/w12082087
Ensor B. (2011). Tsunami mauls mussel farms (Marlborough Express). Available online at: https://www.stuff.co.nz/marlborough-express/news/picton/4773798/Tsunami-mauls-mussel-farms (Accessed March 14, 2024).
Eyrolles P., Lesueur M., Le Bris H., Laidin C., Brunner, Hughes A. L., et al. (2018). Définir un cadre pour le développement de l’AMTI sur l’Arc Atlantique: Lot n°6 - Rapport d’action N°2: exemples d’implantations de systèmes AMTI sur l’Arc Atlantique.: Délivrable 6-2 (Beg Meil, France: Agrocampus Ouest). 30 p.
FAO (2013). Applying spatial planning for promoting future aquaculture growth (COFI). Available online at: www.fao.org/cofi/31155–084a1eedfc71131950f896ba1bfb4c302.pdf (Accessed March 14, 2024).
FAO (2022). The State of world fisheries and aquaculture 2022 (Rome: FAO). doi: 10.4060/cc0461en (Accessed March 14, 2024).
FAO (2023a). FishStatJ - Software for fishery and aquaculture statistical time series. Available online at: https://www.fao.org/fishery/en/statistics/software/fishstatj (Accessed October 25, 2023).
FAO (2023b). National aquaculture overviews. Available online at: https://www.fao.org/fishery/en/naso/search (Accessed October 23, 2023).
Fernand F., Israel A., Skjermo J., Wichard T., Timmermans K. R., Golberg A. (2017). Offshore macroalgae biomass for bioenergy production: Environmental aspects, technological achievements and challenges. Renew. Sustain. Energy Rev. 75, 35–45. doi: 10.1016/j.rser.2016.10.04
Fieler R., Greenacre M., Matsson S., Neves L., Forbord S., Hancke K. (2021). Erosion dynamics of cultivated kelp, Saccharina latissima, and implications for environmental management and carbon sequestration. Front. Mar. Sci. 8. doi: 10.3389/fmars.2021.632725
Flavin K., Flavin N., Flahive B. (2013). Kelp farming manual: a guide to the processes, techniques, and equipment for farming kelp in New England waters. Ocean Approved.
Freites L., Cote J., Himmelman J. H., Lodeiros C. J. (1999). Effects of wave action on the growth and survival of the scallops Euvola ziczac and Lyropecten nodosus in suspended culture. J. Exp. Mar. Biol. Ecol. 239, 47–59. doi: 10.1016/S0022-0981(99)00029-5but
Froehlich H. E., Smith A., Gentry R. R., Halpern B. S. (2017). Offshore aquaculture: I know it when I see it. Front. Mar. Sci. 4. doi: 10.3389/fmars.2017.00154
Fujita R., Brittingham P., Cao L., Froehlich H., Thompson M., Voorhees T. (2023). Toward an environmentally responsible offshore aquaculture industry in the United States: ecological risks, remedies, and knowledge gaps. Mar. Policy 147, 105351. doi: 10.1016/j.marpol.2022.105351
Gagnon M. (2019). Self-organization and mechanical properties of mussel culture suspensions: a critical review. Aquacult. Eng. 87, 102024. doi: 10.1016/j.aquaeng.2019.102024
Gaitan-Espitia J. D., Quintero-Galvis J. F., Mesas A., D'Elia G. (2016). Mitogenomics of southern hemisphere blue mussels (Bivalvia: Pteriomorphia): insights into the evolutionary characteristics of the 1598 Mytilus edulis complex. Sci. Rep. 6, 26853. doi: 10.1038/srep26853
Galparsoro I., Murillas A., Pinarbasi K., Sequeira A., Stelzenmuller V., Borja A., et al. (2020). Global stakeholder vision for ecosystem-based marine aquaculture expansion from coastal to offshore areas. Rev. Aquacult. 12, 2061–2079. doi: 10.1111/raq.12422
Gagnon M. (2024). Status of off-bottom mariculture in wave exposed environments. Part 2. Comparative loading and motion of longline designs currently used in exposed commercial farms. Front. Aquac. 3, 1422173. doi: 10.3389/faquc.2024.1422173
Gee S. (2021). Mussel farmers millions of dollars out of pocket after storms. New Zealand Country, 30 July 2021.
Gendron L., Tamigneaux L. (2008). Expériences de culture de l’algue brune Saccharina longicruris en 2007: essais en bassin et en mer au large de Paspébiac et de Grande-Rivière (Québec). Can. Tech. Rep. Fish. Aquat. Sci. 2820, 48.
Goseberg N., Chambers M. D., Heasman K., Fredriksson D., Fredheim A., Schlurmann T. (2017). “Technological approaches to longline- and cage based aquaculture in open ocean environments,” in Aquaculture perspective of multi-use sites in the open ocean: the untapped potential for marine resources in the Anthropocene. Eds. Buck B., Langan R. (Springer), 71–95.
Gucukluoglu G. (2022). Mussel farming started in Sinop for the first time in Black Sea (Inlas Haber Ajans). Haberianlik.
Guillou N., Lavidas G., Chapalain G. (2020). Wave energy resource assessment for exploitation-a review. J. Mar. Sci. Eng. 8, 705. doi: 10.3390/jmse8090705
Harvey M., Barrett L. T., Morris R. L., Swearer S. E., Dempster T. (2024). Ocean sprawl: the global footprint of shellfish and algae aquaculture and its implications for production, environmental impact, and biosecurity. Aquaculture 586, 740747. doi: 10.1016/j.aquaculture.2024.740747
He H. L., Xu Y. (2016). Wind-wave hindcast in the Yellow Sea and the Bohai Sea from the year 1988 to 2002. Acta Oceanol. Sin. 35, 46–53. doi: 10.1007/s13131-015-0786-5
Heasman K. G., Scott N., Ericson J. A., Taylor D. I., Buck B. H. (2020). Extending New Zealand’s marine shellfish aquaculture into exposed environments—Adapting to modern anthropogenic challenges. Front. Mar. Sci. 7. doi: 10.3389/fmars.2020.565686
Hilbish T. J., Mullinax A., Dolven S. I., Meyer A., Koehn R. K., Rawson P. D. (2000). Origin of the antitropical distribution pattern in marine mussels (Mytilus spp.): routes and timing of transequatorial migration. Mar. Biol. 136, 69–77. doi: 10.1007/s002270050010
Howarth L. M., Reid G. K., Lewis-McCrea. L. (2022). Supporting the development of offshore aquaculture in Nova Scotia (Dartmouth, Nova Scotia, Canada: Centre for Marine Applied Research (CMAR). 44 p.
Hu Z.-M., Shan T.-F., Zhang J., Zhang Q.-S., Critchley A. T., Choi H.-G., et al. (2021). Kelp aquaculture in China: a retrospective and future prospects. Rev. Aquacult. 13, 1324–1351. doi: 10.1111/raq.12585
ICES (2012). Report of the Working Group on Marine Shellfish Culture (WGMASC) Vol. 15 (Copenhagen: ICES CM2012/SSGHE). 117 p.
ICES (2023). Working group on open ocean aquaculture (WGOAA): outputs from 2022 meeting. Sci. Rep. 5, 17.
Id Halla M., Nhhala H., Kassila J., Chattou E. M. A., Orbi A., Moukrim A. (2017). Comparative production of two mussel species (Perna perna and Mytilus galloprovincialis) reared on an offshore submerged longline system in Agadir, Morocco. Int. J. Sci. Eng. Res. 8, 1203–1213.
IPCC (2022). “Chapter 3. Oceans and coastal ecosystems and their services,” in Climate change 2022: impacts, adaptation and vulnerability (Sixth assessment report).
Jiang B., Wei Y., Jiang X., Wang H., Wang X., Ding J., et al. (2016). “Assessment of wave energy resource of the Bohai Sea, Yellow Sea and East China Sea based on 10-year numerical hindcast data,” in Proceedings of the OCEANS 2016, Shanghai, China, 10–13 April 2016.
Jin R., Ye Z., Chen S., Gu J., He J., Huang L., et al. (2023). Accurate mapping of seaweed farms with high-resolution imagery in China. Geocarto. Int. 38, 2203114. doi: 10.1080/10106049.2023.2203114
Kapetsky J. M., Aguilar-Manjarrez J. (2007). Geographic information systems, remote sensing and mapping for the development and management of marine aquaculture Vol. 458 (Rome, Italy: FAO Fish. Tech. Paper). 125 p.
Karayücel S., Çelik M. Y., Karayücel I., Öztürk R., Eyüboğlu B. (2015). Effects of stocking density on survival, growth and biochemical composition of cultured mussels (Mytilus galloprovincialis, Lamarck 1819) from an offshore submerged longline system. Aquac. Res. 2013, 1–15. doi: 10.1111/are.12291
Kato H., Tanji Y., Fujima K., Sighihara Y. (2010). Study on measures against drifting of cultivation rafts by tsunami: report on the results of 2010 Chile Earthquake Tsunami. Jap. Inst. Fish. Infrastuct. Communities, Article Collection 21, 111–120.
Kerrison P. D., Stanley M. S., Black K. D., Hughes A. D. (2017). Assessing the suitability of twelve polymer substrates for the cultivation of macroalgae Laminaria digitata and Saccharina latissima (Laminariales). Algal Res. 22, 127–134. doi: 10.1016/j.algal.2016.10.001
Komatsu T., Sasa S., Montani S., Nishimur O., Sakamaki T., Yoshimura C., et al. (2016). Coast hit by a huge tsunami on 11 March 2011. Satoumi approach for realizing sustainable coastal use in rias-type bay: a case of Shizugawa Bay in Sanriku (St Petersburg, Russia: MECS 11 – Sea Coasts XXVI).
Kosaka Y. (2016). “Scallop fisheries and aquaculture in Japan,” in Scallops: biology, ecology and aquaculture. Eds. Shumway S., Parsons G. J. (Elsevier, Amsterdam, Netherlands), 891–936.
Lader P., Kristiansen D., Alver M., Bjelland H. V., Myrhaug D. (2017). “Classification of aquaculture locations in Norway with respect to wind wave exposure,” in Proceedings of the ASME 2017 36th International Conference on Ocean, Offshore and Arctic Engineering: Ocean Space Utilization, Trondheim, Norway, June 25–30, 2017, Vol. 6. doi: 10.1115/OMAE2017-61659
Lambert G., Karney R. C., Rhee W. Y., Carman M. R. (2016). Wild and cultured edible tunicates: A review. Manage. Biol. Invasions 7, 59–66. doi: 10.3391/mbi.2016.7.1.08
Langan R. (2002). Demonstration of technology and methods for open ocean aquaculture of molluscan shellfish in New England. CINEMar/OOA Aquaculture Annual Progress Report for the period 1/01/01 through 12/31/01. U. New Hampshire, Durham, NU, USA.
Langan R., Chambers M., DeCew J. (2010). AZTI Tecnalia Project: technical feasibility study on submerged longline culture technologies for the open ocean of Bay of Biscay. Report on Activity T4: engineering analysis and operational design of a prototype submerged longline system for mussel culture (Durham, NU, USA: University of New Hampshire). 46 p.
Langan R., Horton C. F. (2003). Design, operation and economics of submerged longline mussel culture in the open ocean. Bull. Aquaculture Assoc. Canada 103, 11–20.
Lee J., Hong S., Jang Y. C., Lee M. J., Kang D., Shim W. J. (2015). Finding solutions for the styrofoam buoy debris problem through participatory workshops. Mar. Policy 51, 182–189. doi: 10.1016/j.marpol.2014.08.008
Lien E., Fredheim A. (2001). “Development of longtube mussel systems for cultivation of mussels,” in Proceedings of the Open Ocean Aquaculture IV Symposium, St. Andrews, NB, Canada, 17–20 June 2001. (Ocean Springs, MS: Mississippi-Alabama Sea Grant Consortium). MASGP-01-006.
Lindell S. (2015). Developing improved management practices for mussel farming in Southern New England: final report. Sea Grant 2010-38500-21074 (Woods Hole, MA, USA: Woods Hole Oceanographic Institution). Subaward # Z540401.
Liu Y., Liang Z. R., Yu W. W., Chang L. R., Lu L. F., Xiao L. Y., et al. (2022b). Effects of cultivation area of different offshore distances on the agronomic traits and breeding rope aging of Saccharina japonica. Progr. Fish. Sci. 43, 156–164. doi: 10.19663/j.issn2095-9869.20210317002
Liu F. L., Liang Z. R., Zhang P. Y., Wang W. J., Sun X. T., Wang F. J., et al. (2019). Preliminary discussion on the development of Saccharina japonica offshore aquaculture in China. Progr. Fish. Sci. 40, 161–166. doi: 10.19663/j.issn2095-9869.20180726002
Liu X., Liu Y., Jiang Z., Cao L. (2024). Spatiotemporal variation of China’s mariculture potential under climate change. Rev. Fish Biol. Fish. 34, 315–335. doi: 10.1007/s11160-023-09814-2
Liu X., Wang Z., Yang X., Liu Y., Liu B., Zhang J., et al. (2022a). Mapping China’s offshore mariculture based on dense time-series optical and radar data. Int. J. Digit. Earth 15, 1326–1349. doi: 10.1080/17538947.2022.2108923
Liu X., Zhang X. (2022). Impacts of high-density suspended aquaculture on water currents: observation and modeling. J. Mar. Sci. Eng. 10, 1151. doi: 10.3390/jmse10081151
Lona E., Endresen P. C., Skjermo J., Tsarau A., Stefanakos C., Broch O. J. (2020). AKVALAB project summary: evaluation of seaweed cultivation technology for weather exposed locations Vol. 2020 (Trondheim, Norway: SINTEF Rep), 00593, ISBN: 978-82-14-06514-5.
Lovatelli A., Manjarrez J., Soto D. (2013). Expanding mariculture farther offshore. Technical, environmental, spatial and governance challenges (Rome: FAO Technical Workshop).
Lucien-Brun H., Lachaux A. (1983). Évolution de la pectiniculture au Japon (Evolution of scallop culture in Japan). La Pêche Maritime Juillet 1983, 388–396.
Lynett P. J., Borrero J., Son S., Wilson R., Miller K. (2014). Assessment of the tsunami-induced current hazard. Geophys. Res. Lett. 41, 2048–2055. doi: 10.1002/2013GL058680
Macias J. C., Avila Zaragozá P., Karakassis I., Sanchez-Jerez P., Massa F., Fezzardi D., et al. (2019). Allocated zones for aquaculture: a guide for the establishment of coastal zones dedicated to aquaculture in the Mediterranean and the Black Sea (Rome: General Fisheries Commission for the Mediterranean. Studies and Reviews). No 97 FAO90 p.
Mascorda Cabre L., Hosegood P., Attrill M. J., Bridger D., Sheehan E. V. (2021). Offshore longline mussel farms: a review of oceanographic and ecological interactions to inform future research needs, policy and management Rev. Aquacult. 13, 1864–1887. doi: 10.1111/raq.12549
Massa F., Onofri L., Fezzardi D. (2017). “Aquaculture in the Mediterranean and the Black Sea: a blue growth perspective,” in Hanbook on the Economics and Management of Sustainable Oceans. Eds. Bunes P. A. L. D., Svennson L. E., Markadya A. (Edward Elgar Publishing, Cheltenham), 93–122.
Mille D., Blachier P. (2009). Mutation conchylicoles. État des lieux et perspectives de development des productions en eau profonde a l’automne 2008 (Changing shellfish aquaculture practice: status and development perspectives of deep water production, Fall 2008). Aglia Rep. Febr.
Mille D., Le Moine O. (2011). Adaptabilité des activités conchylicoles aux notifications de leur environnement: Scénarii et solutions. Available online at: http://archimer.ifremer.fr/doc/00061/17257/ (Accessed March 18, 2024).
Minnhagen S., Moltke Lyngsgaard M., Wallach T., Staufenberger T., Emilsson M., Bailey J., et al. (2019). Results from Baltic Blue Growth Project’s mussel farms and way forward for mussel farming in the Baltic Sea (EU Interreg-project). Report from Baltic Blue Growth.
Mistri M., Munari C. (2019). Effects of a storm surge on mussel off-shore farming in the Po Delta, northern Adriatic Sea. J. Aquac. Mar. Biol. 8, 151–152. doi: 10.15406/jamb.2019.08.00255
Mizuta D. D., Wikfors G. H. (2019). Depth selection and in situ validation for offshore mussel aquaculture in Northeast United States Federal Waters. J. Mar. Sci. Eng. 7, 293. doi: 10.3390/jmse7090293
Morris J. A. Jr, MacKay J. K., Jossart J. A., Wickliffe L. C., Randall A. L., Bath G. E., et al. (2021). An aquaculture opportunity area atlas for the southern California Bight Vol. 29 (NOAA Technical Memorandum NOS NCCOS). 485 p. doi: 10.25923/tmx9-ex26
MPI (2021). New design aims to avoid runaway floats in mussel farming (Ministry of Primary Industries: Media release), 26–02-2021.
Mueller-Fuega A., Bompais. X. (1989). Mytiliculture en mer ouverte: bilan des travaux (Region Bretagne: IFREMER DIT/SOM 89.69). 32 p.
Natsuike M., Nishida Y., Kanamori M., Sato M., Honke K. (2022). Field observations of the physical environment and behavior of culture cages during juvenile Yesso scallop (Mizuhopecten yessoensis) culture in Funka Bay, Hokkaido, Japan. Sci. Rep. Hokkaido Fish. Res. Inst. 102, 23–30.
Norway NS9415 Standard (2009). Floating aquaculture facilities: site survey, design, execution and use. Available online at: https://online.standard.no/nb/ns-9415-2021 (Accessed November 14, 2023).
Ögmundarson Ó., Holmyard J., Þórðarson G., Sigurðsson F., Gunnlaugsdóttir H. (2011). Offshore aquaculture farming—Report from the initial feasibility study and market requirements for the innovations from the project. MATIS Rep. 29-11, 26.
Oshino A. (2010). Macroscopic relationship between scallop (Patinopecten yessoensis) on-growing and buoyancy caused by wave action in Miyagi coast. Myagi Pref. Rep. Fish. Sci. 10, 45–62.
Oyinlola M. A., Reygondeau G., Wabnitz C. C. C., Troell M., Cheung W. W. L. (2018). Global estimation of areas with suitable environmental conditions for mariculture species. PLoS One 13, e0191086. doi: 10.1371/journal.pone.0191086
Pajot R. (1989). Étude de l’exploitation d’une filière mytilicole sub-flottante (Study on the operation of a semi-submerged mussel longline). IFREMER, Dir. Eng. Technol., 60.
Paul W., Grosenbaugh M. (2000). Submerged coastal offshore mussel aquaculture system (SCOMAS): a multidisciplinary approach (MIT Sea Grant Coll. Prog., Tech. Rep. MIT-T-00-001 C2). 11 p.
PROyugAgro (2024). In Kuban, a reduction in the production of mussels and oysters is expected in 2024. Kuban RBC, April 18, 2024.
Rusu E., Rusu L. (2021). An evaluation of the wave energy resources in the proximity of the wind farms operating in the North Sea. Energy Rep. 7, 19–27. doi: 10.1016/j.egyr.2021.05.058
Saether S., Diehl N., Monteiro C., Niedzwiedz S., Burgunter-Delamare B., Bischof K., et al. (2024). The sugar kelp Saccharina latissima. II: Recent advances in farming and applications. J. Appl. Phycol. 36, 1953–1985. doi: 10.1007/s10811-024-03213-1
Sanchez-Jerez P., Karakassis I., Massa F., Fezzardi D., Aguilar-Manjarrez J., Soto D., et al. (2016). Aquaculture’s struggle for space: the need for coastal spatial planning and the potential benefits of allocated zones for aquaculture (AZAs) to avoid conflict and promote sustainability. Aquacult. Env. Interact. 8, 41–54. doi: 10.3354/aei00161
Skjermo J., Broch O. J., Endresen P. C., Forbord S., Lona E. (2020). Analysis of growth of cultivated macroalgae at an exposed and a sheltered site in Møre og Romsdal - Tareal 2 Vol. 2020 (Trondheim, Norway: SINTEF Rep), 01053, ISBN: 978-82-14-06496-4.
Steneck R. S., Graham M. H., Bourque B. J., Corbett D., Erlandson J. M., Estes J. A., et al. (2002). Kelp forest ecosystems: biodiversity, stability, resilience and future. Environ. Conser. 29, 436–459. doi: 10.1017/S0376892902000322
Sumaila U. R., Pierruci A., Oyinlola M. A., Cannas R., Froese R., Glaser S., et al. (2022). Aquaculture over-optimism? Front. Mar. Sci. 9. doi: 10.3389/fmars.2022.984354
Suppasri A., Fukui K., Yamashita K., Leelawat N., Hiroyuki O., Imamura F. (2018). Developing fragility functions for aquaculture rafts and eelgrass in the case of the 2011 Great East Japan tsunami. Nat. Hazards Earth Syst. Sci. 18, 145–155. doi: 10.5194/nhess-18-145-2018
Taguchi T. (1977). A manual of scallop culture methodology and management. DFO Fish. Mar. Serv. Transl. Ser. 4198, 146.
TDC (2019). Resource management plan. Chapter 22. Aquaculture. Available online at: https://www.tasman.govt.nz/my-council/key-documents/tasman-resource-management-plan/volume-1-text/part-3-coastal-marine-area/ (Accessed March 15, 2024).
Tullberg R. M., Nguyen H. P., Wang C. M. (2022). Review of the status and developments in seaweed farming infrastructure. J. Mar. Sci. Eng. 10, 1447. doi: 10.3390/jmse10101447
Ventilla R. F. (1982). The scallop industry in Japan. Adv. Mar. Biol. 20, 309–382. doi: 10.1016/S0065-2881(08)60142-X
Vianello R. (2013). (Université de Bretagne occidentale). Available online at: https://theses.hal.science/tel-01674161 (Accessed 14-03-2024).
von Brand E., Abarca A., Merino G. E., Stotz W. (2016). “Scallop fishery and aquaculture in Chile: a history of developments and decline,” in Scallops: biology, aquaculture and fisheries. Eds. Shumway S. E., Parsons G. J. (Develop. Aquacult. Fish. Sci., Elsevier), 1047–1072.
Wang J., Yang X., Wang Z., Ge D., Kang J. (2022). Monitoring marine aquaculture and implications for marine spatial planning: an example from Shandong Province, China. Remote Sens. 14, 732. doi: 10.3390/rs14030732
Watanabe S., Sakami T. (2021). “Problems and challenges of aquaculture in Japan,” in Proceedings of the International Workshop on the Promotion of Sustainable Aquaculture, Aquatic Animal Health, and Resource Enhancement in Southeast Asia, Aquaculture Department, Southeast Asian Fisheries Development Center, Iloilo City, Philippines, 25–27 June 2019. 64–69.
World Bank (2013). Fish to 2030: prospects for fisheries and aquaculture (World Bank, Washington, DC, USA: Rep. 83177-GLB). 80 p.
Wu Z., Huang L., Wang F., Zhang S., Zhang S. (2021). Impacts of tropical cyclones on mussel culture area and yield in Shengsi. J. Agric. Sci. Technol. (Beijing). 23, 171–183. doi: 10.13304/j.nykjdb.2019.1044
Wu Z., Xiong M., Shi Y., Song Y., Dai Y., Zhang S. (2024). Characteristics and distribution model of wind and wave in Shengsi mussel culture area under the impacts of tropical cyclones. J. Water Climate Change 15, 518. doi: 10.2166/wcc.2024.448
Yildirim S. (2021). “Mussel farming,” in Basic issues in bivalve farming. Eds. Serdar S., Yildirim S., Coban D. (Iksad Publ. House, Ankara, Turkey), 123–148.
Zhang J., Fang J., Wang W., Du M., Gao Y., Zhang M. (2011). Growth and loss of mariculture kelp Saccharina japonica in Sungo Bay, China. J. Appl. Phycol. 24, 1209–1216. doi: 10.1007/s10811-011-9762-4
Zheng Y. H., Jin R. J., Zhang X. J., Wang Q. X., Wu J. P. (2019). The considerable environmental benefits of seaweed aquaculture in China. Stochastic Envir. Res. Risk Assess. 33, 1203–1221. doi: 10.1007/s00477-019-01685-z
Zhu L. (2020). (University of Maine). Available online at: https://digitalcommons.library.umaine.edu/etd/3222 (Accessed November 5, 2023).
Keywords: aquaculture, offshore, open ocean, exposed, temperate, extractive species, global, status
Citation: Gagnon M (2024) Status of off-bottom mariculture in wave-exposed environments. Part 1. Global inventory of extractive species commercial farms in temperate waters. Front. Aquac. 3:1411749. doi: 10.3389/faquc.2024.1411749
Received: 03 April 2024; Accepted: 06 August 2024;
Published: 03 September 2024.
Edited by:
Kevin Gerald Heasman, Cawthron Institute, New ZealandReviewed by:
Vjekoslav Tičina, Institute of Oceanography and Fisheries (IZOR), CroatiaHarry Gorfine, Victorian Fisheries Authority, Australia
Copyright © 2024 Gagnon. This is an open-access article distributed under the terms of the Creative Commons Attribution License (CC BY). The use, distribution or reproduction in other forums is permitted, provided the original author(s) and the copyright owner(s) are credited and that the original publication in this journal is cited, in accordance with accepted academic practice. No use, distribution or reproduction is permitted which does not comply with these terms.
*Correspondence: Marc Gagnon, bWdhZ25vbkBiaW9yZXguY29t