- 1Marine Microbial Pathogenesis and Vaccinology Lab, Department of Ocean Sciences, Memorial University of Newfoundland, St. John’s, NL, Canada
- 2Vacci-vet Inc., Quebec, QC, Canada
- 3Department of Fisheries, Forestry and Agriculture, Government of Newfoundland and Labrador, Halifax, NL, Canada
- 4Cooke Aquaculture Inc., Halifax, NB, Canada
- 5Division of Biomedical Sciences, Faculty of Medicine, Memorial University, St. John’s, NL, Canada
- 6Dr. Joe Brown Aquatic Research Building, Memorial University of Newfoundland, St. John’s, NL, Canada
Lumpfish (Cyclopterus lumpus) have become the predominant cleaner fish species used in North American salmon aquaculture. Vibrio anguillarum is a frequent pathogen of lumpfish in Atlantic Canada, and current vaccines against local isolates conferred low to moderate efficacy. This study evaluated the safety and efficiency of a V. anguillarum autogenous vaccine under controlled and field conditions. Two safety trials were conducted following the Canadian Food Inspection Agency (CFIA) regulations before field trial testing. The first safety trial was a common garden assay, and 250 PIT-tsgged lumpfish were used for five treatments per tank (PBS-negative control, Lab vaccine-positive control, autogenous intraperitoneal (IP) vaccine, autogenous dip vaccine, autogenous dip-IP boosted vaccine) in triplicates. Weight, gross pathology, and IgM titers were evaluated to determine the vaccine’s safety. After 10 weeks post-vaccination (wpv), lumpfish were bath-challenged with a lethal dose (1.24 x 106 CFU/mL) of V. anguillarum serotype O2. Survival rate, IgM titers, memory immune response, and cross-immune protection were evaluated. The second safety trial was conducted using a double vaccine dose in two groups, PBS and autogenous IP. The gross pathology score indicated a normal immune response without tissue damage, and no mortality nor fish health issues were observed in the immunized animals. The IP route of administration conferred the highest protection against the V. anguillarum challenge. The autogenous vaccine conferred long-term immunity but did not confer cross-protection against V. anguillarum serotype O1. To evaluate the efficiency of the autogenous vaccine under field conditions, 58,976 naïve lumpfish (8-10 g) were IP immunized with either the autogenous vaccine or the commercial Forte micro IV vaccine. After 7 wpv, the lumpfish were equally distributed into eight sea cages and co-cultivated with Atlantic salmon smolts (60,000 per cage). Lumpfish IgM titers, tissue bacterial loads, mortality, and sea lice count on salmon were monitored. The autogenous vaccine and Forte vaccine confer acceptable protection to the lumpfish in sea cages. No significant difference was observed between lumpfish vaccinated with autogenous and commercial vaccines under field conditions.
1 Introduction
Sea lice (e.g., Lepeophtheirus salmonis, Caligus elongatus, etc.) infestation is an animal health challenge that limits the production of Atlantic salmon (Salmo salar) (Torrissen et al., 2011; Torrissen et al., 2013). Sea lice cause immunosuppression and contribute to stress, osmoregulatory imbalance, skin lesions, and an increased risk of secondary infection (Bowers et al., 2000; Fjelldal et al., 2022). These effects have significant economic implications due to treatment costs and production losses (Grimnes and Jakobsen, 1996; Brooker et al., 2018). Various strategies for controlling sea lice in salmon farming have been implemented in response to economic pressures, environmental concerns, and welfare considerations. Since 2010, one of the most accepted alternatives for integrated pest management strategies in the North Atlantic is the use of cleaner fish as a biological control (Brooker et al., 2018). In the North Atlantic, salmon farmers utilize several cleaner fish species, including lumpfish (Cyclopterus lumpus) and wrasse (Ballan wrasse (Labrus bergylta); Corkwing wrasse (Symphodus melops); Goldsinny wrasse (Ctenolabrus rupestris) (Bolton-Warberg, 2018; Gentry et al., 2020). However, lumpfish is a commonly utilized species because it performs well in cold environments (Nytrø et al., 2014; Brooker et al., 2018). Using cleaner fish is feasible as it is less stressful to farm fish (Treasurer, 2002; Treasurer, 2012) and reduces the use of chemotherapeutants (Pike and Wadsworth, 1999; Liu and Vanhauwaer Bjelland, 2014). This practice also aligns with the standards of integrated multi-trophic sustainable aquaculture (Martinez-Espiñeira et al., 2016).
Lumpfish is a native marine teleost of the North Atlantic Ocean (Pampoulie et al., 2014; Vasconcelos et al., 2014). Recently, lumpfish has emerged as a potential solution for removing sea lice from Atlantic salmon, acting as a cleaner fish in cold-water environments. In initial experiments conducted in small sea pens, the outcomes were highly encouraging, demonstrating a significant reduction of 93-97% in sea lice infestation (specifically adult female lice) in pens where lumpfish were present (Imsland et al., 2014a; Imsland et al., 2014b; Imsland et al., 2015). The great demand for cleaner fish caused an increase in the number of lumpfish hatcheries in Atlantic Canada (Maya, 2019). However, mortality of lumpfish has been observed after post-transfer to salmon cages due to bacterial diseases (Rønneseth et al., 2017) (Marcos-López et al., 2013; Vasquez et al., 2020a). Vibrio anguillarum is a Gram-negative aquatic pathogen and the causative agent for vibriosis (Yu et al., 2012; Gao et al., 2018), a fatal disease affecting freshwater and marine finfish aquaculture (Toranzo et al., 2005; Kim et al., 2012; Ma et al., 2017). V. anguillarum is one of the most frequent bacterial pathogens affecting lumpfish (Marcos-López et al., 2013; Rønneseth et al., 2017; Vasquez et al., 2020a), its occurrences in lumpfish have been reported in Canada, Norway, and Scotland (Marcos-López et al., 2013; Gulla et al., 2016; Powell et al., 2018; Chakraborty et al., 2019; Vasquez et al., 2020a). As the mortality incidence of lumpfish is often linked to bacterial diseases (Reynolds et al., 2022a), vaccine development that protects lumpfish against bacterial infections, including vibriosis, has been recognized as a high priority by the cleaner fish aquaculture industry (Brooker et al., 2018; Powell et al., 2018).
Currently, at least three commercial vaccines are available for lumpfish in Canada (https://active.inspection.gc.ca/netapp/veterinarybio-bioveterinaire/vetbioe.aspx). However, proof of their efficacy is not supported. Recently, an evaluation of the effectiveness of commercial polyvalent vaccines available in Canada (e.g., Alpha ject Micro IV, Pharmaq; Vibriogene 2, Elanco; Forte Micro 4, Novartis) for lumpfish against V. anguillarum was conducted with negative to modest results (Cao et al., 2018; Chakraborty et al., 2019; Vasquez et al., 2020a). Designing and evaluating different vaccine preparations for C. lumpus were also conducted. As a result of this research, it was determined that commercial vaccines trigger low immune protection in vaccinated lumpfish (2-54%). However, in contrast, a V. anguillarum experimental vaccine preparation conferred up to 77-80% protection under controlled conditions (Cao et al., 2018; Vasquez et al., 2020a). These vaccine trials have been repeated using a more significant number of fish, with similar results (Dang et al., 2021). The success of these vaccines under controlled conditions has taken the attention of the NL aquaculture industry, which urged their evaluation under more relevant field conditions. Two safety trials under controlled conditions must be evaluated before proceeding to the field trial. This study aims to evaluate an autogenous vaccine’s safety and protective efficiency in lumpfish against V. anguillarum under controlled and field conditions.
2 Materials and methods
2.1 Bacterial culture
V. anguillarum J360, serotype O2, a fully characterized virulent strain isolated from lumpfish in Newfoundland (Vasquez et al., 2020a) was utilized for this study. V. anguillarum J360 was routinely cultured at 15˚C in 3 mL of trypticase soy broth (TSB; Difco, Franklin Lakes, New Jersey, USA) supplemented with 2% NaCl using a 16 mm diameter glass tube. The bacterial cultures were cultivated in a MaxQ shaker (Thermo Scientific, USA) at 180 rpm for 48 h. An inoculum from fresh V. anguillarum liquid culture was streaked and mechanically diluted onto a trypticase soy agar (TSA) 2% NaCl plate using a 1 μL inoculating loop. TSA working seed plates were incubated at 15˚C for 48 h, preserved at 4˚C for routine use, and renewed every 4 weeks. The growth of the bacteria was monitored spectrophotometrically (Thermo Spectronic Genesys 10 U.V., Rochester, New York, USA) and plated onto TSA 2% NaCl.
2.2 Preparation of the laboratory vaccine
A laboratory vaccine bacterin base was prepared according to established protocols (Cao et al., 2018; Dang et al., 2021) and used as an internal positive control. Briefly, a single colony of V. anguillarum J360 was inoculated in a 16 mm glass tube containing 4 mL of TSB 2% NaCl and incubated at 28 ˚C with aeration (180 rpm) for 24 h. An aliquot of 4 mL of a mild-logarithmic V. anguillarum culture (O.D. 600 nm ~0.7; ~108 CFU/mL) was added to 400 mL of TSB 2% NaCl in a 1 L flat bottom flask and incubated at 28˚C with aeration (180 rpm) until O.D.600nm ~1.5 (~1010 CFU/mL). The bacterial culture was inactivated according to established protocols (Dang et al., 2021) with modifications to the formalin percentage. Briefly, 0.4% formalin (Fisher BioReagents, New Jersey, USA) was used to inactivate V. anguillarum overnight at 4˚C. Inactivation was evaluated by plating 100 µL of the formalin-killed whole-cell (FKC) into 3 mL of TSB 2% NaCl and TSA 2% NaCl plates, respectively to ensure that the inactivation was effective. The plates and the liquid cultures were incubated at 28°C from 48 to 72 h to check the sterility of the final laboratory vaccine. The liquid cultures were contrast to TSB (negative control) by O.D. 600 nm. The final number of inactivated cells was quantified using flow cytometry (BD FASCS Aria™/Diva version 7.0 software, San Jose, USA) and the Invitrogen Live/Dead BacLight™ bacterial viability and counting kit (Thermo Fisher, Oregon, USA). The laboratory vaccine preparation was adjusted to 2.0 x 107 cells/mL and mixed with 10% (v/v) of Carbigen adjuvant (MVP adjuvant™ Omaha, NE, USA; 45 mL of laboratory vaccine, 5 mL of Carbigen) and mixed for 30 min. According to the manufacturer’s instructions, the final pH was adjusted to 7 with 10 N NaOH.
A certified V. anguillarum J360 injectable and bath autogenous vaccine formulations were produced by Vacci-Vet (Saint-Hyacinthe, QC, Canada). V. anguillarum was grown in TSA 2% NaCl at 28 °C until the desired concentration, inactivated, and adjuvanted with aluminum oxide.
2.3 Fish holding
Lumpfish was raised and maintained at the Dr. Joe Brown Aquatic Research Building (JBARB; Ocean Sciences Centre, St. John’s, NL, Canada) under optimal conditions. Briefly, the lumpfish were kept in 500 L tanks using a flow-through system (75 L min-1) of UV-treated seawater, 95–100% air saturation, and ambient photoperiod (12 h light:12 h dark). Proper biomass density (5–30 kg per cubic meter) was maintained throughout all the experimental periods. The fish were fed twice daily (10 am and 2 pm) at 0.5% body weight with a commercial diet (Europa 15-Skretting).
All fish-related procedures in this study were reviewed and approved by the Memorial University of Newfoundland’s (MUN) (https://www.mun.ca/research/about/acs/acc/) Institutional Animal Care Committee and the Biosafety Committee based on the procedures of the Canadian Council on Animal Care (https://ccac.ca/) under protocol #18-01-JS, #18-03-JS, and biohazard license L-01.
2.4 Fish immunization trials under controlled conditions
Two immunization trials were conducted to obtain federal Canadian Food Inspection Agency (CFIA) approval for field trials. First, a common garden experiment design was utilized to evaluate the safety and efficiency of vaccine preparations (Figure 1A). Briefly, 750 naïve lumpfish juveniles (20 – 50 g) were Passive Integrated Transponders (PIT)-Tagged and divided into equal groups of 250 fish/per tank into three 500 L tanks. Each fish group/tank consisted of five treatments (2 controls and 3 vaccines). In each tank, 50 lumpfish were intraperitoneally (IP) injected with 100 µL of PBS or the laboratory vaccine and used as a negative and positive control, respectively. The experimental autogenous vaccine was administered by IP injection to 50 lumpfish and by dipping to 100 lumpfish, 50 of which were IP boosted 4 weeks afterwards. The dipping immunization with the experimental autogenous vaccine was utilized at a ratio of 1:10 (vaccine: seawater) according to the manufacturer’s instructions. The dipping preparation was used only once to ensure consistency between immunized groups. The safety of the vaccine was determined by daily monitoring the fish swimming behavior and checking for mortalities. In addition, a second safety trial under controlled conditions was conducted to satisfy the CFIA requirements for field trial approval. Naïve lumpfish (15-25 g) non-PIT tagged were divided into two groups of 50 fish each in independent 500 L tanks (Figure 1B). The vaccinated group received a double dose (200 μL) of the injectable autogenous vaccine (Vacci-Vet). Similarly, a double dose of sterile PBS (Cytiva, HyClone™, South Logan, UT, USA) was injected and used as a negative control. Mortality was monitored twice a day for 3 (weeks post-vaccination) wpv. Evaluation of the vaccine efficacy is described in detail in section 2.8, these fish were challenged with V. anguillarum J360 and mortalities monitored for 60 d.
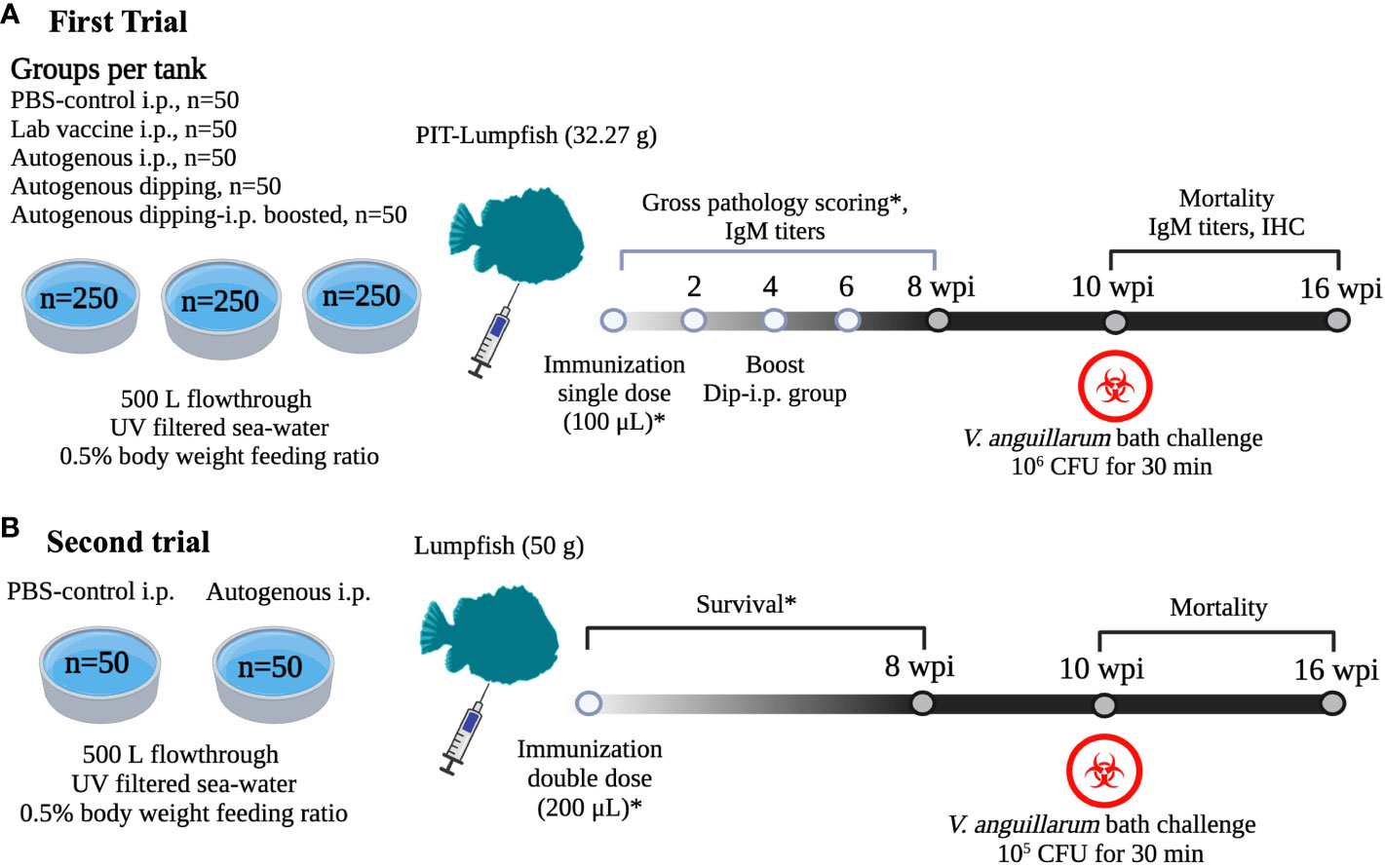
Figure 1 Safety trials under controlled conditions in lumpfish. (A) Common garden experimental design to test safety and efficacy of V. anguillarum O1 autogenous vaccine (Vacci-Vet). All the immunizations were conducted at JBARB, and infections at the AQ3 certified biocontainment unit at CDRF. A total of 750 lumpfish were equally distributed into three tanks, and each tank consisted of five groups (PBS-control IP, Lab vaccine IP, autogenous IP, autogenous dipping, and autogenous dipping-IP boost) of 50 Passive Integrated Transponder (PIT)-tagged fish. The dipping-i.p. vaccine group was i.p. boosted at 4 wpv. At 9 wpv, the lumpfish was moved to the AQ3 unit and bath challenged at 10 wpv with 10 LD50 of V. anguillarum J360 (106 CFU/mL). Serum samples were taken from 6 fish at 2, 4, 6, 8 wpv, 1, and 4 wpc.Gross pathology was evaluated at 4 and 8 wpv, immunohistochemistry was evaluated at 4 wpv and 1 wpc. Fish mortality was monitored for 60 days after the V. anguillarum challenge. (B) Safety and efficacy trial using a double dose of the V. anguillarum O2 injectable autogenous vaccine (Vacci-Vet). A total of 100 lumpfish were equally distributed into two tanks. Each tank was immunized with PBS-control IP and autogenous IP respectively. Control and vaccinated groups were challenged by bath with V. anguillarum J360 (105 CFU/mL) at 10 wpv. Fish mortalities were monitored for 60 days after the V. anguillarum challenge. The image was created using biorender (https://app.biorender.com/).
2.5 Weight measurement
The weight of 30 lumpfish was measured from all the treatments at 0, 4, and 8 wpv. The weight was measured using the sensitive weighing scale (Oharus, Parsippany, NJ, USA). The mean weight parameters were used to compare the weight difference between the control and vaccinated groups.
2.6 Lumpfish gross pathology determination
A total of 5 fish per treatment were sampled for the pathological assay at 4 and 8 wpv. The fish was netted and immediately euthanized with an overdose of MS222 (400 mg/L). The gross pathology was determined using a Spielberg scoring system (Figures 2A, B) developed in this study for lumpfish vaccines according to the following protocol (Midtlyng et al., 1996). Briefly, 60 individual lumpfish were injected with an oil-based vaccine (Alpha Ject Micro IV), and 60 were injected with PBS. Gross pathology was evaluated and scored at 4, 6, and 8 wpv, based on previous descriptions (Midtlyng et al., 1996; Erkinharju et al., 2017). Five trained independent individuals did the scoring of vaccinated lumpfish. The oil-based vaccinated fish had the highest level of adhesion and remnant, and it was compared with the PBS group. The level of adhesion ranged from 0-5, where 0 means no level of adhesion, and 5 is the greatest possible degree of adhesion. The level of vaccine remnant ranged from 0-5, where 0 means no level of vaccine remnant, and 5 is the highest level of vaccine remnant.
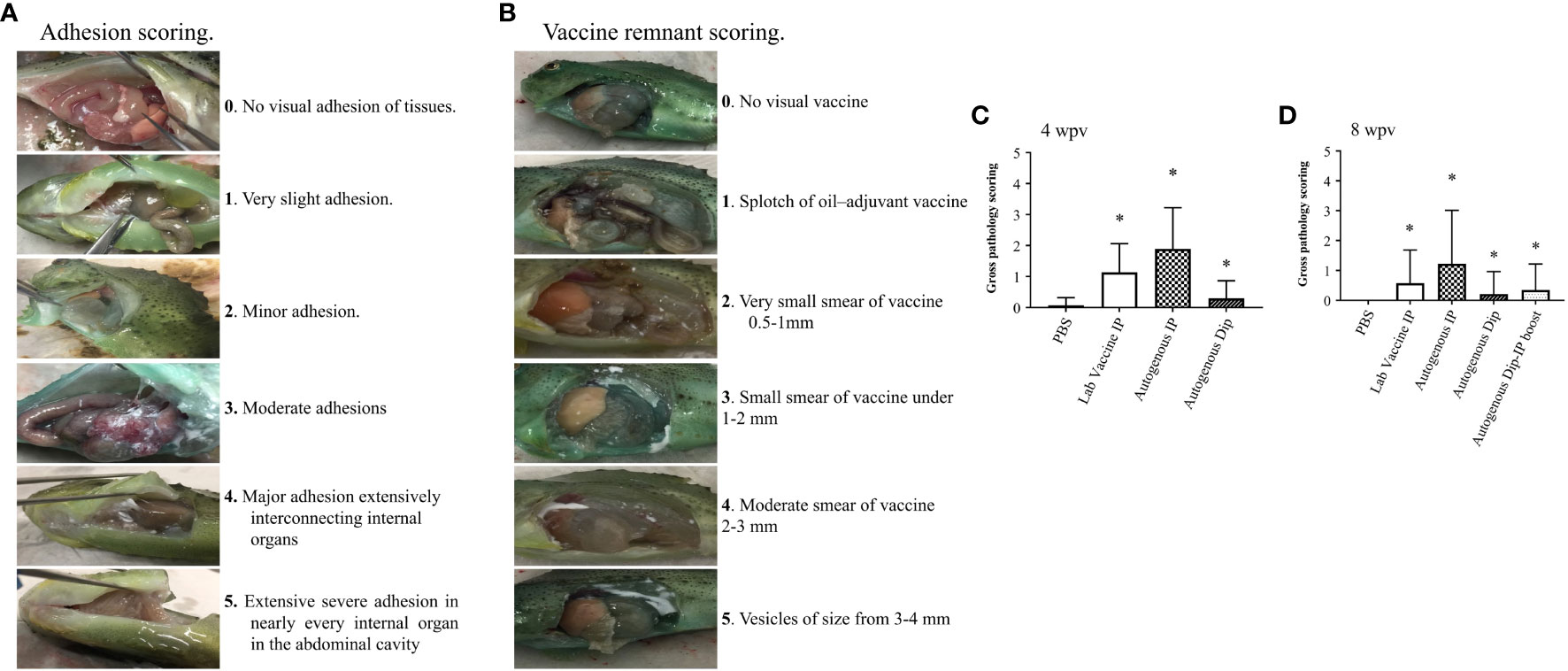
Figure 2 Gross pathology scoring of lumpfish. (A) Scoring for adhesion ranging from 0 (none) to 5 (severe); (B) Scoring for vaccine remnant ranging from 0 (none) to 5 (severe); (C) Gross pathology scoring of lumpfish at 4 wpv in 5 fish per treatment conducted by 5 independent evaluators. (D) Gross pathology scoring of lumpfish 8 wpv in 5 fish per treatment conducted by 5 independent evaluators. The star symbol indicates significant differences with respect to the PBS control group (*p ≤ 0.001).
2.7 Immunohistochemistry
Tissue samples (spleen and head kidney) from the first trial were collected from PBS and autogenous IP group at 4 wpv and 1 week post-challenge (wpc). The tissues were fixed in 10% formalin at room temperature for 3 d; after this period, formalin was replaced with PBS, and the tissues were preserved at 4 ˚C until analysis. Paraffin blocks of lumpfish tissues were sectioned onto positively charged slides, and slides were processed for immunohistochemistry and/or stained with standard Geimsa. Immunohistochemistry for CD45 was performed as we described previously (Gendron et al., 2020; Gendron et al., 2022) and visualized using red alkaline phosphatase substrate using mouse monoclonal antibody DLT22, which was raised against sea bass (Dicentrarchus labrax) CD45 (Marozzi et al., 2012; Nuñez Ortiz et al., 2014). Immunohistochemistry for IgM was performed and visualized using red alkaline phosphatase substrate using a custom anti-lumpfish IgM antibody produced in chicken in collaboration with Somru BioScience (Charlottetown, PEI, Canada), as previously described (Gendron et al., 2020). All IHC sections were not counterstained to emphasize the IHC signals better.
2.8 V. anguillarum challenge assay
As mentioned at the end of section 2.4, vaccinated fish from trials 1 and 2 were challenged with V. anguillarum as follows. The vaccinated lumpfish were transferred to the certified AQ3 biocontainment unit at the Cold-Ocean and Deep-Sea Research Facility (CDRF) at 9 wpv and acclimated for 1 week before infection challenge. The fish were challenged with V. anguillarum J360 using established protocols (Cao et al., 2018; Dang et al., 2021). Briefly, the infection challenge was done by transferring fish into 50 L tanks supplemented with oxygen and inoculating the tank with the V. anguillarum dose. Trials 1 and 2 were challenged with V. anguillarum 1.24 x 106 CFU/mL and 2.0 x 105 CFU/mL, respectively for 30 min, and then the animals were transferred to 500 L tanks with optimal conditions. Mortalities were monitored daily for 60 d. The relative percentage survival (RPS) was calculated according to the formula: (Chakraborty et al., 2019). Blood, liver, spleen, and head kidney were collected from the first trial at 11 and 14 wpv (1 and 4 wpc) to determine the V. anguillarum loads in each tissue. Blood was collected into a 1.5 mL micro-centrifuge tube (Fisherbrand, Fisher Scientific, USA) coated with 100 µg heparin (Sigma-Aldrich, St. Louis, MO, USA) and serially diluted in PBS (1:10), plated onto TSA 2% NaCl, and incubated at 15˚C for 3 d to determine V. anguillarum CFU per mL of blood. Likewise, the spleen, liver, and head kidney were aseptically removed and placed in sterilized sample bags (Nasco Whirl-Pak, Fort Atkinson, WI, USA), weighed, and PBS was added to attain a final volume of 1 mL. The tissues were homogenized, and the suspensions were serially diluted in PBS (1:10). The dilutions were plated onto TSA 2% NaCl and incubated at 15˚C for 3 d to determine the CFU of V. anguillarum per gram tissue.
2.9 Memory immune response and cross-immune protection
The surviving fish from the first trial were re-challenged at 19 wpv (9 wpc) with V. anguillarum J360, serotype O2 (106 CFU/mL) or V. anguillarum J382, serotype O1 (106 CFU/mL). Two tanks with survivors (n=71) were bath infected with V. anguillarum J360 or J382, and the third tank was used as a negative non-infected control. The second challenge to determine whether there is a memory immune response and whether the vaccine can protect against serotype O1. Mortalities were monitored daily for 60 d. The RPS was calculated as described previously.
2.10 Indirect enzyme-linked immunosorbent assay
The blood samples were collected from lumpfish in the first trial at 2, 4, 6, 8, 11, and 14 wpv using 1 mL syringes (BD, New Jersey, USA) with the 26, 23, and 21G needles (BD, New Jersey, USA), respectively. Blood samples of 500 µL were collected into a 1.5 mL microcentrifuge tube from 6 fish and placed on ice. The blood samples were centrifuged at 10,000 rpm (9,600 x g) for 5 min. The serum was collected, the precipitated blood cells were discarded, and the serum was stored at -80˚C until processing. The serum was deactivated at 56˚C for 30 min and treated for lipid removal with 100 µL chloroform (Sigma-Aldrich, St. Louis, MO, USA) for 10 min at room temperature (Vasquez et al., 2020b). Serum samples were centrifuged at 10,000 rpm at room temperature for 5 min. After centrifugation, the supernatant was collected into a fresh 1.5 mL microcentrifuge tube and stored at -80˚C until analysis.
Formalin-killed V. anguillarum J360 was used as an antigen to coat 96-well ELISA plates (Ultra-High Binding Polystyrene Microtiter, ThermoFisher Scientific, Waltham, MA, USA). The optimal antigen concentration was determined by standard procedures (Hnasko, 2015) at 106 cells/well. The plate was incubated overnight at 4˚C and washed thrice with 100 μL of PBS-T (PBS 0.1% Tween 20). Then, the well plates were blocked with 150 µL of Chondrex blocking buffer (Chondrex Inc., Woodinville, WA, USA), incubated at 37 ˚C for 1 h, and washed thrice with PBS-T. The serum was serially diluted 1:2 with PBS-T (60 µL of PBS-T:60 µL of serum) starting at 1:2 until 1:164 dilution. The first column was used as a non-serum blank control. Serial dilutions (1:2) were done by transferring 60 µL from the first row to the second row till it reached the last row. The well plates were incubated at 37˚C for 1 h. The plate was washed 5 times with PBS-T, after which 100 µL of the chicken IgY anti-lumpfish IgM (1:10,000 dilution in PBS-T) was added and incubated at 37˚C for 1 h. The well-plates were washed 5 times, after which 100 µL of streptavidin-HRP (Southern Biotech, Southern Biotech, Birmingham, AL, USA; 1:10,000 in PBS-T) was added and then incubated at 37 ˚C for 1 h. The well-plates were washed 5 times with PBS-T, after which 50 µL of 3,3’,5,5’ tetramethylbenzidine (TMB; Invitrogen™, ThermoFisher, Waltham, MA, USA) was added and incubated at room temperature for 15 min. The reaction was stopped with 2 M H2SO4 and read at 450 nm using a molecular device SpectraMax M5e microplate reader (SpectraMax M5e, San Jose, CA, USA).
2.11 Fish immunization trial under field conditions
A total of 58,976 lumpfish weighing 8-10 g were used for the field trial (Figure 3). A total of 29,488 lumpfish were IP injected with 100 µL of V. anguillarum O1 Vacci-Vet autogenous vaccine, while 29,488 lumpfish were IP vaccinated with the commercial vaccine Forte micro IV (Aeromonas salmonicida, V. anguillarum serotypes I & II, V. ordalii and V. salmonicida serotypes I & II; Novatis, Montreal, QC, Canada). Seven weeks after vaccination, lumpfish were evenly distributed into eight sea cages (7,372 per sea cage), each of them containing 60,000 Atlantic salmon. Serum samples were taken from 6 Vacci-vet vaccinated lumpfish at 0 and 5, 14 and 26 wpv, while serum samples were only taken from 6 Forte vaccinated lumpfish at 14 and 26 wpv. Tissue samples (spleen and head kidney) were taken to determine potential bacteria load. Lumpfish mortalities were monitored weekly for 20 weeks (7 to 27 wpv) from all the sea cages. Also, the average sea lice (juveniles, movable, and adult females) count was recorded from 20 salmon per sea cage for 20 weeks.
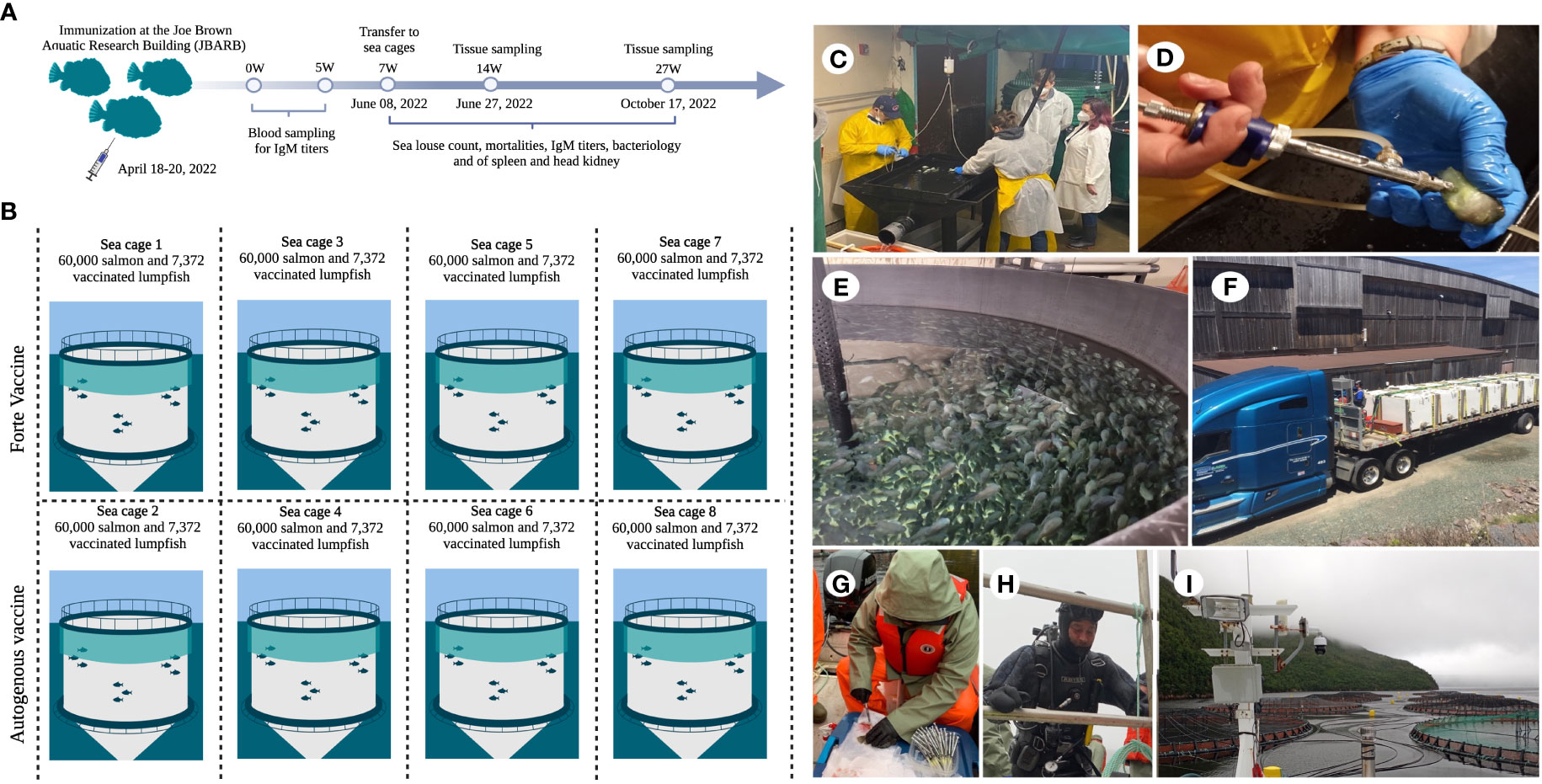
Figure 3 Field safety trial. (A) Immunization of 29, 488 lumpfish with autogenous vaccine at JBARB and 29,488 lumpfish immunized with Forte vaccine was done at another facility. Lumpfish were transfer to sea cages at 7 wpv, blood samples were taken from 6 fish at 0, 5, 14 and 26 wpv from the autogenous vaccine group and at 14 and 26 wpv from the Forte vaccine group. Tissue samples (head kidney and spleen) were taken for bacteriology at 14 and 26 wpv from at least 3 fish per treatment, sea louse count and mortality count were taken weekly for 20 2weeks (7 – 27 wpv); (B) Vaccinated lumpfish were evenly distributed into 8 sea cages with 60,000 salmon smolt each; (C) Vaccination of 29,488 lumpfish at JBARB; (D) Vaccinating the lumpfish with autogenous vaccine using injection gun; (E) Lumpfish in holding tanks after vaccination; (F) Truck transferring fish from JBARB to sea cage site; (G) Blood sampling for iELISA on the cage site; (H) Diver for lumpfish capture in sea cages. (I) Sea cages.
2.12 Phenotypic and molecular characterization of bacteria strains
Bacteria strains isolated from vaccinated lumpfish tissues in the field were subjected to phenotypic characterization using the API 20E and API 20NE according to the manufacturer’s instructions. The isolated strains were also subjected to molecular identification by amplifying and sequencing the 16S gene using the universal 16S rRNA bacterial primers 27F (5’-AGAGTTTGATCCTGGCTCAG-3’) and 1392R (5’-GGTTACCTTGTTACGACTT-3’). Briefly, bacterial DNA was extracted from purified bacterial cultures using Wizard® Genomic DNA Purification kit (Promega, Wisconsin, USA) according to the manufacturer’s instructions. The concentration, purity, and integrity were evaluated by gel electrophoresis (1% agarose) and Genova-Nano Spectrophotometer (Jenway, UK). A 50 µL reaction mixture contains 2.5 µL each of forward and reverse primers, 200 ng of DNA template, 25 µL of Q5 high-fidelity 2X master mix, and nuclease-free water. The amplification was performed on BioRad MJ Mini thermo cycler (Model: PTC-1148, Singapore). The thermocycling conditions were 98˚C for 30 sec, followed by 30 cycles of 98˚C for 10 sec, 45˚C for 30 sec, and 72˚C for 30 sec, followed by 72˚C for 2 min and 4˚C hold. The PCR product integrity was evaluated by gel electrophoresis (1% agarose) and visualized. The PCR products were sequenced at Genome Quebec using Sanger sequencing. The sequences were trimmed and aligned using Clustal Omega, and identification was done on the National Centre for Biotechnology Information (NCBI).
2.13 Statistical analysis
Data were displayed as the mean ± standard deviation. A one‐way ANOVA was used to ascertain significance. Differences were considered significant at p ≤ 0.05 or p ≤ 0.001. All statistical analyses were achieved using GraphPad Prism 9.0 (GraphPad Software). The Kaplan–Meier estimator was used to obtain survival fractions after the challenges, and the log-rank test was used to determine the differences between treatments.
3 Results
3.1 Autogenous V. anguillarum vaccine safety test
To test the safety of the V. anguillarum autogenous vaccine, naive lumpfish were vaccinated via IP and immersion using a standard commercial dose (100 μL/dose for the IP; 1:10 dilution for 30 min for bath). During the 21 d observation period required by the CFIA, overseen by the provincial veterinarian, all the fish survived, and no adverse clinical signs after vaccination were observed. None of the vaccine treatments evaluated influenced the growth of the fish (Figure S1). The second safety trial was conducted by IP using a double dose of a commercial vaccine dose (200 μL/dose). Similar to the first trial, no adverse effects were observed with respect the PBS control. The fish maintained regular feeding behavior, and no mortality was observed.
3.2 Autogenous V. anguillarum vaccine effect in lumpfish gross pathology
Gross pathology was evaluated in five fish per treatment at 4 and 8 wpv. We did not observe vaccine remnants in all the treatment groups. The adhesion level was significantly higher (p ≤ 0.001) in the vaccinated groups at 4 and 8 wpv compared to the control group (Figures 2C, D).
3.3 IHC analysis in vaccinated lumpfish
Decreased expression of CD45 was observed in the spleen of both PBS and autogenous IP group at 4 wpv (Figures 4A, B). Interestingly, in spleens of 1 wpc from both the PBS autogenous vaccine IP group (Figures 4C, D), CD45 (arrows) was expressed in vascular linings. Increased expression of IgM in the parenchyma can be observed in the spleen between the PBS group 4 wpv (Figure 4E) and the autogenous IP group 4 wpv (Figure 4F). In the spleen of the PBS group at 1 wpc and the autogenous IP group at 1 wpc (Figures 4G, H), increased staining indicative of IgM can be observed in a more diffuse manner in the autogenous IP spleen parenchyma (Figure 4H). In the head kidney of the PBS group at 4 wpv and 1 wpc (Figures 4M, O), an increase in CD45 expression in the glomeruli was observed. The intense CD45 staining and damage of renal tubules and glomeruli were observed (Figure 4O). Intense CD45 staining can also be observed in the glomeruli and renal tubules in the head kidney of the autogenous IP group at 1wpc (Figure 4P). Increased IgM expression was also observed in the head kidney parenchyma in the PBS group (Figure 4S) at 1 wpc compared to PBS group at 4 wpv (Figure 4Q). Fish receiving autogenous IP vaccine had near-normal levels of CD45 and IgM immunoreactivity in head kidney (Figures 4N, R, T). Geimsa stains of adjacent sections of the tissues (Figures 4I–L, U–X) indicated increased eosinophilia in the spleen of PBS at 1wpc (Figure 4K), glomerular damage in the head kidney of PBS and autogenous IP group at 1 wpc (Figures 4W, X), and the Giemsa stain (Gendron et al., 2022) in the cells of the renal tubules with deep purple histological changes consistent with increased chromatin in the head kidney in both the PBS and Autogenous IP at 1 wpc (Figures 4W, X).
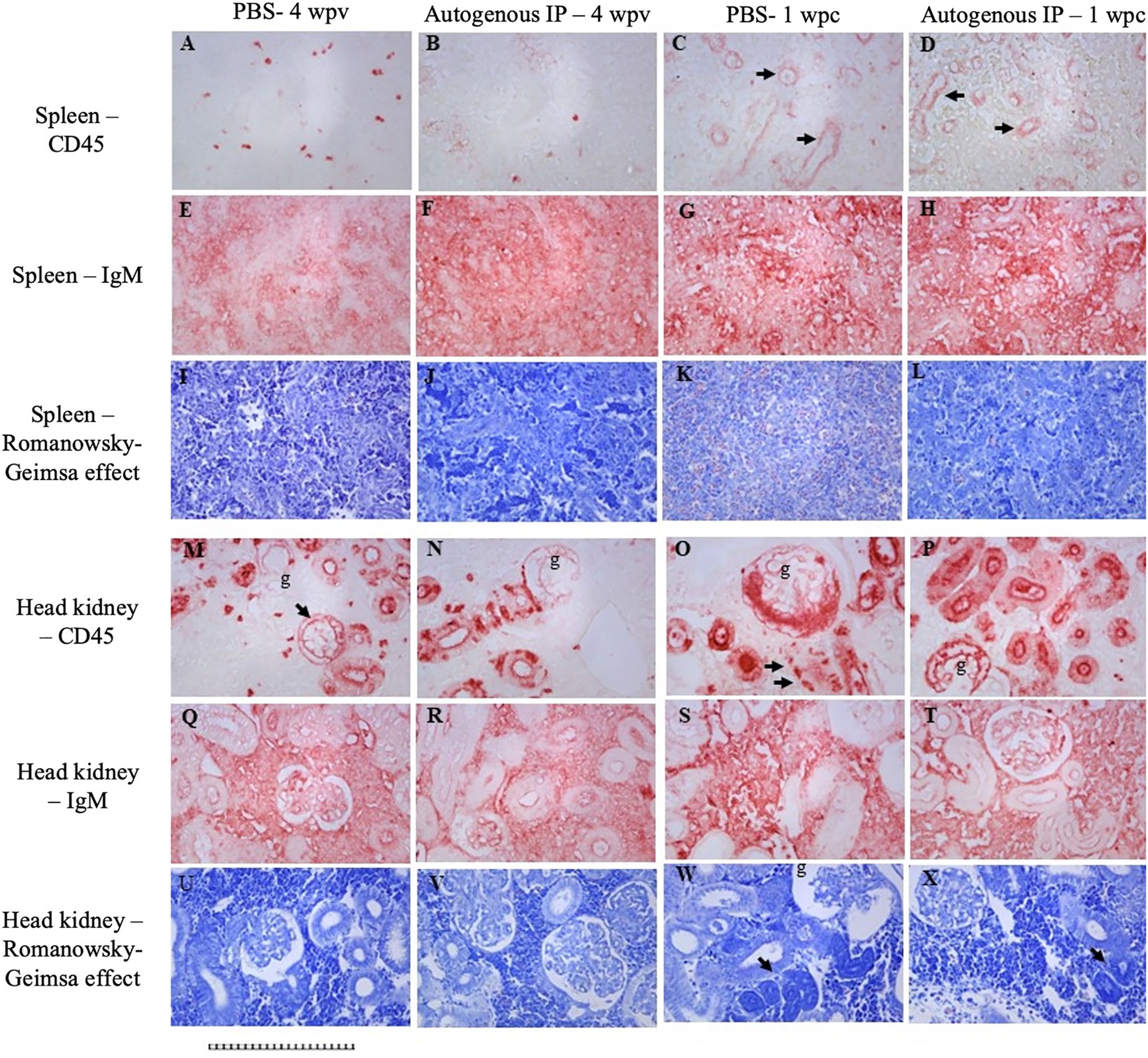
Figure 4 Immunohistochemistry for CD45 (A–D; M–P) and IgM (E–H; Q–T). Spleen from PBS group at 4 wpv (A, E, I). Head kidney from PBS group at 4 wpv (M, Q, U). Spleen from autogenous vaccine IP group at 4 wpv (B, F, J). Head kidney from autogenous vaccine IP group at 4 wpv (N, R, V). Spleen from PBS group at 1 wpc (C, G, K). Head kidney from PBS group at 1 wpc (O, S, W). Spleen from autogenous vaccine IP group at 1 wpc (D, H, L). Head kidney from autogenous vaccine IP group at 1 wpc (P, T, X). The arrows in Figures (C, D, M, O) shows the expression of CD45 in vascular linings. The arrows in Figures (W, X) shows increased chromatin. All panels have been magnifid 200X, scale bar : 1 square = 10 microns.
3.4 Autogenous V. anguillarum vaccine efficacy
To evaluate the autogenous vaccine efficiency test under controlled conditions, the fish were bath-challenged at 10 wpv with V. aguillarum J360 (1.24 x 106 CFU/mL), and the RPS was calculated at 60 dpc (Figure 5B). The RPS for the laboratory-vaccine IP, autogenous IP, autogenous dip, and autogenous dip and IP boosted were 76.12%, 72.12%, 0%, and 68.75%, respectively. The RPS of the laboratory vaccine and autogenous IP was greater than 70%, which makes these preparations the most effective. The moribund fish exhibit clinical signs, including skin discoloration, exophthalmia, lesions on the skin, and peritonitis (Figure 5D). V. anguillarum tissue colonization was evaluated at 1 wpc and 4 wpc (Figures 5E, F). At 1 wpc, the PBS-control and autogenous dipping group observed a high CFU count. We observed significant differences in CFU count between the IP vaccinated groups (laboratory-vaccine IP, autogenous IP, and autogenous dip and IP boost) and the PBS-control in the liver, head kidney, and blood (Figure 5E). On the contrary, no significant differences were observed between the PBS-control and the autogenous dip groups (Figure 5E). No significant differences were observed in the spleen (Figure 5E). CFU counts were significantly reduced at 4 wpc, and no significant differences between the groups were at 4 wpc (Figure 5F).
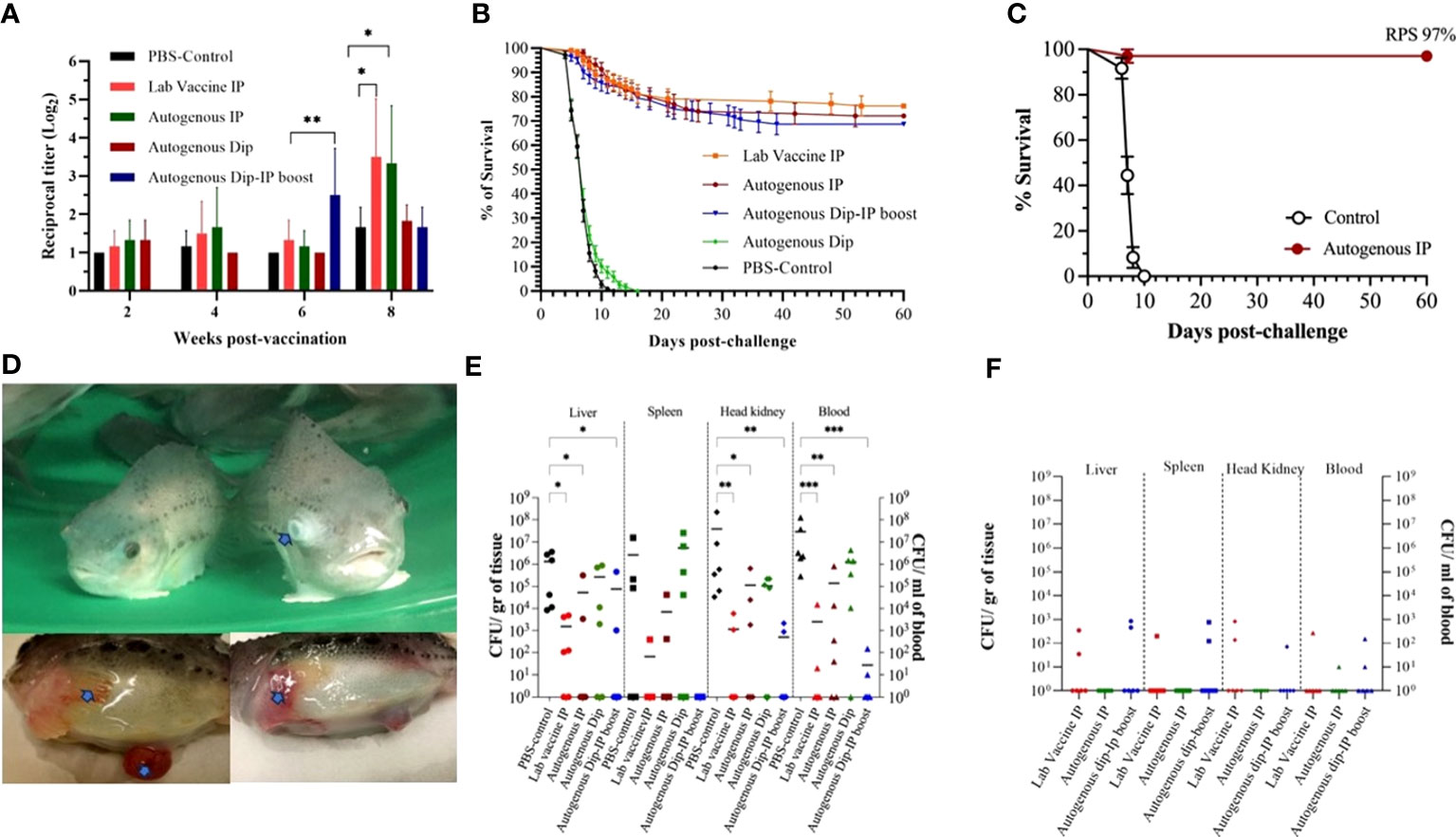
Figure 5 Autogenous V. anguillarum O2 vaccine immunity and efficacy. (A) Lumpfish IgM titers for Vibrio anguillarum after vaccination under controlled conditions. Serum samples were collected at 2, 4, 6, and 8 wpv from six fish per treatment. Specific IgM titers were evaluated by iELISA. Statistical differences between the control (PBS) and the vaccinated groups were analyzed (*p≤ 0.05, **p≤ 0.005); (B) Cumulative survival rate of the first safety trial of dipping and i.p. immunized lumpfish after bath challenge with V. anguillarum (1.24 × 106 CFU/mL). The Relative Percentage Survival (RPS) for the lab vaccine i.p. autogenous i.p., and autogenous Dip-i.p. boosted was 76.12%, 72.12%, and 68.75%, respectively; (C) Cumulative survival rate of the second safety trial of i.p. immunized lumpfish after bath challenge with V. anguillarum (2.0 × 105 CFU/mL). The RPS for the autogenous i.p. was 97%; (D) Clinical signs after V. anguillarum challenge. Clinical signs, the clinical signs (arrows) are exophthalmia, peritonitis, hemorrhage of the fin and skin; (E) Tissue bacteria colonization at 1-week post-challenge. Statistical differences between the control (PBS) and the vaccinated group were analyzed ((*p≤ 0.05, **p≤ 0.005, ***p ≤ 0.0001); (F) Tissue bacteria colonization at 4-week post-challenge.
The RPS for the second safety vaccine trial conducted using double the IP vaccine dose was 97%. Also, these immunized animals were challenged with a lower dose (105 CFU/mL), which influenced their survival (Figure 5C).
3.5 Memory immune response and cross-protection
Lumpfish survivors that were re-challenged with V. anguillarum J360 (serotype O2) displayed better survival for autogenous IP, lab vaccine IP, and autogenous dip-IP boost with a RPS of 95%, 89.3%, and 78.6%, respectively (Figure 6A). In contrast, the survivors re-challenged with V. anguillarum J382 serotype O1 (Figure 6B) showed no survival after 7 dpc.
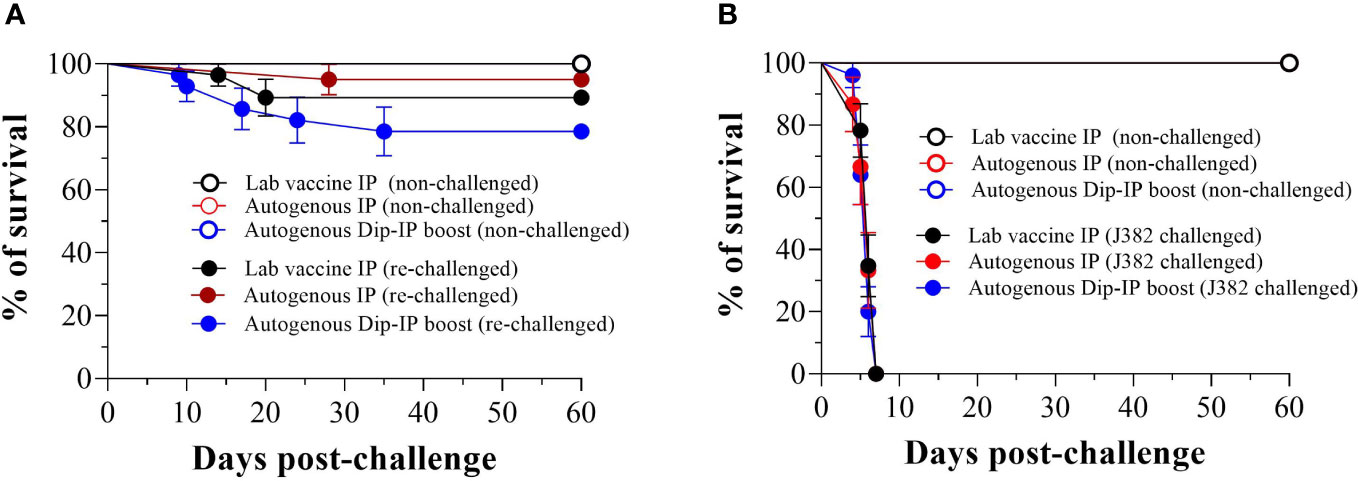
Figure 6 Memory immune response after a second infection with V. anguillrum O2 and cross-protection against V. anguillarum 01. (A) Survival of immunized lumpfish re-challenged by bath with V. anguillarum O2 J360 (106 CFU/mL) at 60 days post-challenge. The calculated RPS for lab vaccine IP, autogenous IP, and autogenous Dip-IP boost were 89.3%, 95%, and 78.6%, respectively; (B) Survival of immunized lumpfish re-challenged by bath with V. anguillarum O1 J382 (106 CFU/mL) at 60 days post-challenge.
3.6 Humoral immune response of immunized lumpfish under controlled conditions
The specific serum V. anguillarum IgM was determined by indirect ELISA post-vaccination and up to 4 wpc (Figures 5A, S2). The antibody titers of the autogenous dipping-IP boost immunized group were significantly higher than the control group at 6 wpv. The antibody titer of the laboratory vaccine IP and autogenous IP group peaked at 8 wpv and was significantly higher than the control group (Figure 5A). There was no significant difference between the vaccinated and the control groups after the V. anguillarum challenge (Figure S2). The specific-antibody response produced by the different vaccine groups relates to the RPS of each vaccine.
3.7 Vaccine efficacy test under field conditions
The fish were transferred to sea cages at 7 wpv; serum samples were taken at 0 (before vaccination), 5, 14, and 26 wpv (Figure 7A). The highest peak of antibody titer for both vaccines (Vacci-vet and Forte micro IV) was observed at 14 wpv, and there was no significant difference between the Vacci-vet and the Forte micro IV. Lumpfish mortalities were monitored for 20 weeks (Figure 7B). The percentage of cumulative mortalities of lumpfish vaccinated with Vacci-vet was 12.0%, whereas lumpfish vaccinated with Forte micro IV was 5.4%. No significant difference was observed between lumpfish mortalities of the Vacci-vet and Forte groups. Three stages of sea lice (L. salmonis) were recorded for the sea lice count from 20 salmon fish per sea cage for 20 weeks (Figure 7C). They are L. salmonis juveniles, movable, and adult females. The sea lice stage with higher prevalence was the movable stage in both the Vacci-vet and Forte micro IV sea cages. The average L. salmonis adult female stage was recorded lowest for both Vacci-vet and Forte micro IV sea cages. No significant difference was discovered between the sea lice count of the Vacci-vet and Forte micro IV cages across the different stages of the sea lice.
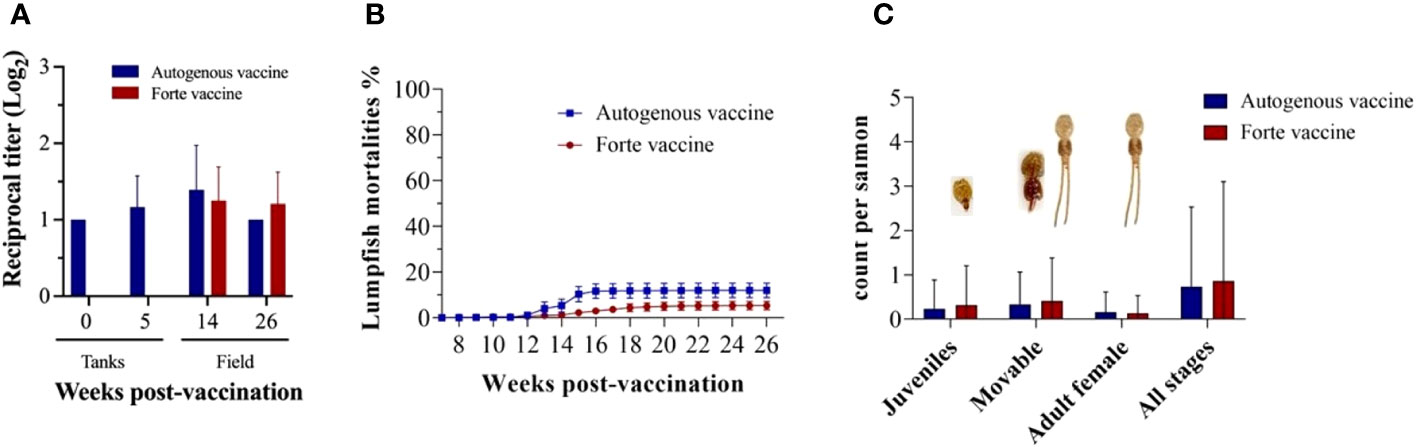
Figure 7 Vaccine evaluation for immunity and efficacy. (A) Lumpfish IgM titers for Vibrio anguillarum after vaccination under field conditions. Serum samples were collected at 0, 5, 14, and 26 wpv from six fish per treatment. Specific IgM titers were evaluated by iELISA. Statistical differences between the autogenous and forte groups were analyzed (p> 0.05); (B) Lumpfish mortality was observed and recorded weekly for 20 weeks. The cumulative percentage mortality for autogenous and forte vaccine group were 12.0% and 5.4% respectively; (C) Average Sea louse count was observed and recorded weekly for 20 weeks. Three stages of Lepeophtheirus salmonis (Juveniles, Movable and adult females) were recovered during the sampling period from both vaccinated groups. Statistical differences between the autogenous and forte groups were analysed (p> 0.05).
Tissue colonization was evaluated at 14 and 26 wpv to determine the bacteria load (Figure 8). Three bacterial strains (J385, J386, and J387) were isolated from the head kidney and spleen at 14 wpv (Figures 8A, B), and four bacterial strains (J385, J388, J389, and J390) were isolated from the head kidney and spleen at 26 wpv (Figures 8C, D). High bacteria load was observed in fish vaccinated with the Forte micro IV compared to the Vacci-vet vaccine at both time points. The spleen significantly differed at 26 wpv with the J390 bacterial strain (Figure 8D). The strains isolated were subjected to phenotypic and molecular tests for identification. For API 20E (Table S1, Figure S3), J387 and J390 were identified as Photobacterium damselae with a percentage identity of 95.2 and 96%. Other strains had no percentage identity. For AP1 20NE (Table S2, Figure S4), J385, J386, J387, J388, J389, and J390 were identified as Shewanella putrefaciens (46.9%), Vibrio vulnificus (99.6%), P. damselae (95.2%), Pasteurella sp (42.5%), Vibrio alginolyticus (60.4%), and P. damselae (97.4%) respectively. The molecular characterization (Table S3) identified J385, J386, J387, J388, J389, and J390 as Shewanella sp (100%), Vibrio sp (99.65%), Photobacterium sp. (99.63%), Aliivibrio sp. (99.88%), V. anguillarum (99.87%), and Photobacterium sp. (99.16%), respectively.
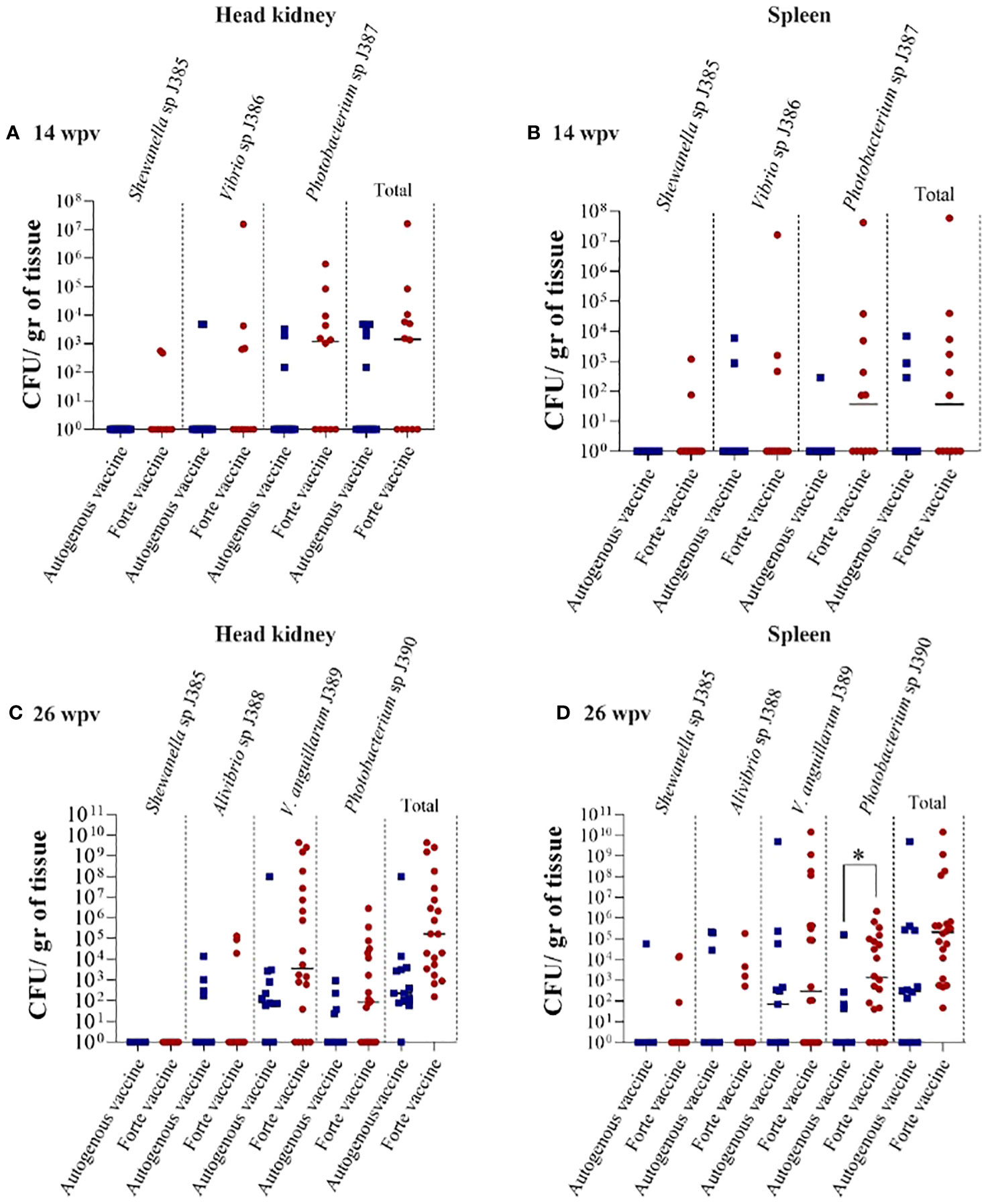
Figure 8 Lumpfish tissue bacterial colonization under field trial. (A) Head kidney bacterial colonization at 14 wpv. Three strains were also recovered: J385 (Shewanella sp.), J386 (Vibrio sp.), and J387 (Photobacterium sp.); (B) Spleen bacterial colonization at 14 wpv. Three strains were also recovered; J385 (Shewanella sp.), J386 (Vibrio sp.), and J387 (Photobacterium sp.); (C) Head kidney bacterial colonization at 26 wpv. Four strains were recovered: J385 (Shewanella sp.), J388 (Pasteurella sp), J389 (V. anguillarum), and J390 (Photobacterium sp); (D) Spleen bacterial colonization at 26 wpv. Four strains were also recovered; J385 (Shewanella sp.), J388 (Pasteurella sp), J389 (V. anguillarum), and J390 (Photobacterium sp). Statistical differences between the autogenous and forte groups were analyzed (*p ≤ 0.05).
4 Discussion
Vibriosis, caused by V. anguillarum, is a common disease affecting lumpfish aquaculture, and its also responsible for significant mortality in various fish species (Haastein and Holt, 1972; Frans et al., 2011; Brooker et al., 2018; Vasquez et al., 2020a; Erkinharju et al., 2021; Kverme et al., 2022). Although several commercial vaccines against V. anguillarum are available, not all trigger adequate immune protection in lumpfish (Chakraborty et al., 2019). An experimental V. anguillarum autogenous vaccine was recently successfully evaluated in lumpfish (Dang et al., 2021). Therefore, we aimed to assess this vaccine under field conditions. In this study, we conducted safety assays under controlled conditions and a field trial in lumpfish.
The CFIA required experiments under controlled conditions to assess the autogenous vaccine’s safety before proceeding with the field trial Canadian Food Inspection Agency (CFIA) (2023). The authorities requested us to evaluate mortality, behavior, and adverse reactions in the vaccinated fish using a single and double dose to ensure the safety and welfare of fish populations and minimize risks associated with vaccine use in aquaculture. Interestingly, the regulatory agency did not request the vaccine’s efficacy or immunogenicity. Perhaps this requirement speeds up the approval of emergency vaccines, like autogenous vaccines, but it could increase the risk of diseases by approving non-effective vaccines. Commercial vaccines are widely available for major aquatic diseases in finfish. Alongside these autogenous vaccines are also utilized in aquaculture, which are custom developed using locally sourced pathogen strains for specific locations (Kennedy et al., 2016). Aquaculture vaccine development primarily focuses on preventing disease symptoms that hinder growth or cause mortality rather than halting infection and transmission. Vaccines that offer protection against disease symptoms while permitting pathogen infection and transmission can promote the development of heightened virulence. Various studies support this concept (Gandon et al., 2001; Gandon and Day, 2008; Kennedy et al., 2016) and could lead to reduced vaccine effectiveness and the emergence of more severe diseases. Perhaps request vaccine efficacy for finfish could improve fish health and decrease disease transmission in aquaculture settings.
The first trial aimed to assess the safety and efficiency of the autogenous vaccine under controlled conditions. Various parameters were evaluated, including weight, gross pathology, bacterial load, IgM titers, and RPS. The vaccinated fish showed normal weight, no remnant vaccine, and slight adhesion, which could be attributed to the aluminum-based adjuvant in the autogenous vaccine (Figures 2C, D, S1). In contrast, it has been observed that fish vaccinated with the autogenous vaccine containing a paraffin-liquid adjuvant exhibited an exacerbated adhesion and decreased growth due to inflammation (Torres-Corral et al., 2021). It is believed that oil-based adjuvants may reduce growth in vaccinated fish due to severe inflammation, leading to decreased feed intake (Rønsholdt and McLean, 1999). Here, we observe that aluminum or nanoemulsion (Carbigen, Phibro) adjuvanted vaccines did not cause adverse effects on the lumpfish. Histological and IHC analyses supported a conclusion that the autogenous vaccine conferred some level of microscopically detectable immune protection as demonstrated by tissue morphological differences and differential expression of immune markers CD45 and IgM in the control group compared to the vaccinated group. The tissue structural abnormalities we observed in head kidney samples treated with PBS post bacterial challenge (Figure 4O) compared to fish treated with the vaccine (Figure 4P) could be associated with cellular infiltration. While the decrease in cellular CD45 expression we observed in the head kidneys of the autogenous IP group (Figure 4B) compared to the PBS group 4 wpv (Figure 4A) support immune protection, the expression of similar levels of CD45 in the vascular linings of spleens of both post challenge groups (Figures 4C, 4D) suggests that the immune protection might be more pronounced in head kidney. Moreover, the expression of CD45 in splenic vasculature is intriguing and deserves exploration in future studies. The increase of IgM expression in the head kidney parenchyma observed in fish treated with PBS 1 week post bacterial challenge (Figure 4S) compared to the vaccinated fish (Figure 4T) further supports some level of microscopically detectable immune protection. The increased chromatin condensation in the head kidney of both groups post bacterial challenge (Figures 4W, 4X) indicates that complete protection was not conferred by the vaccine. Overall, histological signs of bacterial infection were observed to be more pronounced in fish treated with PBS compared to fish treated with the autogenous vaccine.
We evaluated the effectiveness of the vaccine preparations and their route of administration by infection challenge with V. anguillarum J360. Also, we assessed the immune sterility of the vaccine by sampling the tissues to determine the bacterial load. The bacterial load present in the tissues suggests a direct parameter that reveals the number of pathogens present in control and vaccinated fish post-challenge and can be used to determine the effective eradication or reduction of a pathogen in organisms protected by a vaccine (Liu et al., 2014; Tang et al., 2018; Xing et al., 2019) Under the controlled condition, high levels of V. anguillarum were observed in the control and dipping immunized fish, which correlates with the mortality data (Figures 5B, E, F). The RPS was also used to measure vaccine efficacy at 60 days after the challenge. Several factors, including the route of administration, vaccine dose, water temperature, and adjuvant, can influence the effectiveness of a vaccine (Munang’andu and Evensen, 2019; Xing et al., 2019). This study compared the RPS values between IP, dip, dip-IP boosted immunized lumpfish of a V. anguillarum bath infection. Dipping or bath vaccination is easier and less stressful but ineffective (Chakraborty et al., 2019; Dang et al., 2021) (Figure 5B). During dip vaccination, antigens could be absorbed through the gills, gut, and skin, triggering a mucosal immune response that may lead to protection. The limited antigen uptake during dip vaccination may explain the lack of adaptive responses observed with repeated vaccinations compared to the IP route (Bøgwald and Dalmo, 2019; Dang et al., 2021). Antigen-specific antibodies play a crucial role in the humoral immune response following vaccination, providing vaccinated fish with protective immunity (Munang’andu and Evensen, 2019). These antibodies facilitate phagocytosis and enhance the killing activity of phagocytes while also stimulating antigen-specific B cells (de Ståhl et al., 2003; Zhang et al., 2021). Fish vaccinated via IP exhibited significantly higher V. anguillarum-specific antibody titers than the control and dip-vaccinated lumpfish in their serum. This indicates the vaccine’s ability to generate a protective response (Mohamad et al., 2022), similar to the discoveries of Zhang et al (Tang et al., 2018), who reported higher antibody titers in vaccinated fish compared to the control group.
The memory immune response in vaccinated fish enables rapid recognition and response to a pathogen upon re-exposure (Murphy et al., 2017; Stosik et al., 2021). Memory B and T cells preserve information from vaccination and can identify specific pathogen components (Murphy et al., 2017). Memory B cells swiftly generate antibodies that neutralize the pathogen, while memory T cells enhance the immune response by enlisting and activating other immune cells. This expedited reaction results in quicker elimination of the pathogen, decreasing the severity and duration of the infection (Marshall et al., 2018). In this study, we found that lumpfish that survived a primary exposure to V. anguillarum generated a memory immune response, denoted by a second V. anguillarum lethal infection with a RPS of 95% for the autogenous vaccine (Figure 6A). Similarly (Tattiyapong et al., 2020), reported an RPS of 100% after a second challenge of the Tilapia Lake virus in Tilapia.
In this study, we also established that there is no cross-protection. The V. anguillarum autogenous vaccine (serotype O2) was not designed to protect against V. anguillarum serotype O1 pathogen. Cross-protection between V. anguillarum serotype O2 and O1 is limited due to their distinct LPS antigenic differences (Machimbirike et al., 2023). Serotypes have unique surfaces of antigens recognized by the immune system, and vaccines targeting one serotype may not effectively neutralize the other if there are significant antigenic disparities (Servín-Blanco et al., 2016). Vaccines designed for serotype O2 are unlikely to confer cross-protection against serotype O1 due to the immune response’s inability to recognize and neutralize the antigens of serotype O1 (Toranzo et al., 1997).
The success of using lumpfish for biological control of sea lice depends on the ability of lumpfish to regularly consume a steady population of sea lice through grazing (Boissonnot et al., 2022). Bacterial infections such as V. anguillarum pose the primary obstacle to the efficacy of lumpfish delousing and their survival rates in sea cages (Marcos-López et al., 2013; Brooker et al., 2018; Powell et al., 2018). Therefore, creating preventive vaccines for lumpfish, specifically targeting bacterial infections like vibriosis, is a top priority in the cleaner aquaculture sector (Brooker et al., 2018; Powell et al., 2018). For the field trial, we demonstrated the effect of vaccinated lumpfish on sea lice infestation in the salmon farm. We compared the autogenous vaccinated lumpfish with Forte Micro IV lumpfish, even though this experiment’s control should be unvaccinated. There was no significant difference in the sea lice count between the vaccinated groups. The average adult female sea lice count for autogenous vaccine and Forte micro IV were 0.16 (0.8%) and 0.13 (0.7%), respectively, which is significantly lower compared to the findings of Imsland et al (Imsland et al., 2014a; Imsland et al., 2014b; Imsland et al., 2015), who reported 3-7% of sea lice infection (adult female) in sea pens containing unvaccinated lumpfish. Sea lice exhibit a life cycle influenced by the temperature, with faster development observed in warmer waters (Hamre et al., 2019). We observed high sea lice counts between October and November, the peak of the summer season. During the initial months at sea, lumpfish generally experienced good or slightly reduced welfare. However, beyond that period, a significant proportion of the population exhibited clear reductions in welfare, primarily due to severe skin and eye damage (Figure S5). This finding is consistent with the study conducted by Gutierrez et al (Gutierrez Rabadan et al., 2021), which identified poor eye condition and body damage as the most common welfare issues in lumpfish housed in sea cages. These damages pose health concerns for lumpfish, as the skin serves as the primary defense against pathogens (Ángeles Esteban, 2012), and maintaining good eye health is crucial for their feeding behavior, considering that lumpfish are visual feeders (Jonassen et al., 2017; Powell et al., 2018). Our study observed a lumpfish cumulative mortality rate of 12.0% for Vacci-vet and 5.4% for Forte-micro IV over 5 months. The effectiveness of the lumpfish vaccination on the reduction of mortality is supported by the comparison of our results with those of Boissonnot et al. (2023). They documented a cumulative mortality rate of naive lumpfish ranging from 59.6% to 96.8% over a period of 51-55 weeks in a field trial, suggesting that vaccinated lumpfish have significantly reduced mortality.
Infections are frequently identified as the primary factor contributing to the decline in welfare and survival of lumpfish in sea cages (Brooker et al., 2018; Mortensen et al., 2021; Reynolds et al., 2022b; Sommerset et al., 2022; Boissonnot et al., 2023). Vaccination efficiently prevents a vast range of bacterial diseases (Ma et al., 2019). Even though vaccinated fish can still exhibit bacterial colonization, like in this study, their bacterial load is often lower than non-vaccinated fish (Silvaraj et al., 2020).
5 Conclusion
This study reported the evaluation of V. anguillarum autogenous vaccine under controlled and field conditions. Under controlled conditions, no mortality after vaccination was observed. The gross pathology score indicated a normal immune response without tissue damage. The IP route of administration conferred the highest efficacy against the V. anguillarum O2 challenge. However, bath immunization did not confer immune protection. The autogenous vaccine conferred long-term immunity against serotype O2 but does not confer protection against serotype O1. Under the field condition, the V. anguillarum autogenous vaccine and commercial Forte micro IV vaccine adequately protect the lumpfish in sea cages. No significant difference was observed between lumpfish vaccinated with autogenous and commercial vaccines under field conditions. Lumpfish were co-infected by several novels or undescribed marine bacterial pathogens (e.g., Shewanella sp, Photobacterium sp, Vibrio sp), which can be further used for virulence and vaccinology studies.
Data availability statement
The original contributions presented in the study are included in the article/Supplementary Material. Further inquiries can be directed to the corresponding author.
Ethics statement
The animal experiments under controlled conditions where approved by Dr. Jennifer Keyte, jkeyte@mun.ca. The filed assay was authorized by CFIA, Cooke, and NL provicial authorities. Written informed consent was obtained from the individual(s) for the publication of any identifiable images or data included in this article.
Author contributions
OO: Data curation, Investigation, Writing – original draft, Writing – review & editing, Formal analysis, Methodology, Software, Validation, Visualization. TC: Data curation, Investigation, Methodology, Writing – review & editing, Supervision. IV: Data curation, Investigation, Methodology, Supervision, Writing – review & editing. JC-O: Investigation, Methodology, Supervision, Writing – review & editing. HG: Investigation, Methodology, Supervision, Writing – review & editing, Data curation, Formal analysis, Visualization. AH: Investigation, Methodology, Writing – review & editing. VM: Investigation, Methodology, Writing – review & editing, Data curation, Supervision, Validation, Visualization. YH-R: Methodology, Writing – review & editing, Resources. AntK: Resources, Writing – review & editing. AndK: Resources, Writing – review & editing, Methodology. NO’B: Methodology, Resources, Writing – review & editing, Supervision. SG: Methodology, Resources, Writing – review & editing, Investigation. AS: Investigation, Methodology, Resources, Writing – review & editing, Supervision. RG: Investigation, Methodology, Resources, Writing – review & editing. RK: Investigation, Methodology, Writing – review & editing, Data curation, Formal analysis. DT: Methodology, Writing – review & editing, Resources. JM: Methodology, Writing – review & editing, Investigation. JP: Methodology, Resources, Writing – review & editing. DB: Methodology, Resources, Writing – review & editing, Conceptualization, Funding acquisition, Investigation, Project administration, Supervision. JS: Conceptualization, Funding acquisition, Investigation, Methodology, Project administration, Resources, Supervision, Writing – review & editing, Data curation, Formal analysis, Writing – original draft.
Funding
The author(s) declare financial support was received for the research, authorship, and/or publication of this article. This work was funded through Canadian Center for Fisheries and Innovation (CCFI), Canada First -Ocean Frontier Institute (sub-module J3), NSERC-Discovery grant (RGPIN-2018-05942), and Atlantic Fisheries Fund, Canada.
Acknowledgments
We thank all the staff at the Dr. Joe Brown Aquatic Research Building and at the Cold-Ocean and Deep-Sea Research Facility, Zhiyu Chen and Stephen Hill for their expert technical assistance.
Conflict of interest
Authors YH-R, AntK and AndK were employed by the company Vacci-vet Inc. Authors SG and AS were employed by the company Cooke Aquaculture Inc.
The remaining authors declare that the research was conducted in the absence of any commercial or financial relationships that could be construed as a potential conflict of interest.
The author(s) JS declared that they were an editorial board member of Frontiers, at the time of submission. This had no impact on the peer review process and the final decision.
Publisher’s note
All claims expressed in this article are solely those of the authors and do not necessarily represent those of their affiliated organizations, or those of the publisher, the editors and the reviewers. Any product that may be evaluated in this article, or claim that may be made by its manufacturer, is not guaranteed or endorsed by the publisher.
Supplementary material
The Supplementary Material for this article can be found online at: https://www.frontiersin.org/articles/10.3389/faquc.2023.1306503/full#supplementary-material
References
Ángeles Esteban M. (2012). An overview of the immunological defenses in fish skin. ISRN Immunol. 2012, 1–29. doi: 10.5402/2012/853470
Canadian Food Inspection Agency (CFIA). (2023). VB-GL-3.2: Regulation of biotechnology-derived veterinary biologics. Available at: https://inspection.Canada.ca/animal-health/veterinary-biologics/guidelines-forms/3-2/eng/1328244737525/1328244875804.
Bøgwald J., Dalmo R. A. (2019). Review on immersion vaccines for fish: an update 2019. Microorganisms 7 (12), 627. doi: 10.3390/microorganisms7120627
Boissonnot L., Karlsen C., Reynolds P., Austad M., Stensby-Skjærvik S., Remen M., et al. (2023). Welfare and survival of lumpfish (Cyclopterus lumpus) in Norwegian commercial Atlantic salmon (Salmo salar) production. Aquaculture 572, 739496. doi: 10.1016/j.aquaculture.2023.739496
Boissonnot L., Kharlova I., Iversen N. S., Staven F. R., Austad M. (2022). Characteristics of lumpfish (Cyclopterus lumpus) with high cleaning efficacy in commercial Atlantic salmon (Salmo salar) production. Aquaculture 560, 738544. doi: 10.1016/j.aquaculture.2022.738544
Bolton-Warberg M. (2018). An overview of cleaner fish use in Ireland. J. Fish Dis. 41 (6), 935–939. doi: 10.1111/jfd.12731
Bowers J. M., Mustafa A., Speare D. J., Conboy G. A., Brimacombe M., Sims D. E., et al. (2000). The physiological response of Atlantic salmon, Salmo salar L., to a single experimental challenge with sea lice, Lepeophtheirus salmonis. J. Fish Dis. 23 (3), 165–172. doi: 10.1046/j.1365-2761.2000.00225.x
Brooker A. J., Papadopoulou A., Gutierrez C., Rey S., Davie A., Migaud H. (2018). Sustainable production and use of cleaner fish for the biological control of sea lice: recent advances and current challenges. Vet Rec. Br. Vet Assoc. 183 (12), 383. doi: 10.1136/vr.104966
Cao T. T., Chakraborty S., Hossein A., Gnanagobal H., Dang M., Vasquez I., et al. (2018). “Vibrio anguillarum Vaccine Candidates for Lumpfish (Cyclopterus lumpus),” in In 8th International Symposium on Aquatic Animal Health Charlottetown, Prince Edward Island, Canada.
Chakraborty S., Cao T., Hossain A., Gnanagobal H., Vasquez I., Boyce D., et al. (2019). Vibrogen-2 vaccine trial in lumpfish (Cyclopterus lumpus) against Vibrio anguillarum. J. Fish Dis. 42 (7), 1057–1064. doi: 10.1111/jfd.13010
Dang M., Cao T., Vasquez I., Hossain A., Gnanagobal H., Kumar S., et al. (2021). Oral Immunization of Larvae and Juvenile of Lumpfish (Cyclopterus lumpus) against Vibrio anguillarum Does Not Influence Systemic Immunity. Vaccines (Basel) 9 (8), 819. doi: 10.3390/vaccines9080819
de Ståhl T. D., Dahlström J., Carroll M. C., Heyman B. (2003). A role for complement in feedback enhancement of antibody responses by igG3. J. Exp. Med. 197 (9), 1183–1190. doi: 10.1084/jem.20022232
Erkinharju T., Dalmo R. A., Hansen M., Seternes T. (2021). Cleaner fish in aquaculture: review on diseases and vaccination. Rev. Aquacult Wiley-Blackwell 13, 189–237. doi: 10.1111/raq.12470
Erkinharju T., Lundberg M. R., Isdal E., Hordvik I., Dalmo R. A., Seternes T. (2017). Studies on the antibody response and side effects after intramuscular and intraperitoneal injection of Atlantic lumpfish (Cyclopterus lumpus L.) with different oil-based vaccines. J. Fish Dis. 40 (12), 1805–1813. doi: 10.1111/jfd.12649
Fjelldal P. G., Fraser T. W. K., Hansen T. J., Karlsen Ø, Bui S. (2022). Effects of laboratory salmon louse infection on mortality, growth, and sexual maturation in Atlantic salmon. Pernet F, editor. ICES J. Mar. Sci. 79 (5), 1530–1538. doi: 10.1093/icesjms/fsac078
Frans I., Michiels C. W., Bossier P., Willems K. A., Lievens B., Rediers H. (2011). Vibrio anguillarum as a fish pathogen: virulence factors, diagnosis and prevention. J. Fish Dis. 34 (9), 643–661. doi: 10.1111/j.1365-2761.2011.01279.x
Gandon S., Day T. (2008). Evidences of parasite evolution after vaccination. Vaccine 26 (SUPPL. 3), C4–C7. doi: 10.1016/j.vaccine.2008.02.007
Gandon S., MacKinnon M., Nee S., Read A. F. (2001). Imperfect vaccines and the evolution of pathogen virulence. Nature 414, 751–756. doi: 10.1038/414751a
Gao X., Pi D., Chen N., Li X., Liu X., Yang H., et al. (2018). Survival, virulent characteristics, and transcriptomic analyses of the pathogenic Vibrio anguillarum under starvation stress. Front. Cell Infect. Microbiol. 8. doi: 10.3389/fcimb.2018.00389/full
Gendron R. L., Hyde T., Paradis H., Cao T., Machimbirike V. I., Segovia C., et al. (2022). CD45 in ocular tissues during larval and juvenile stages and early stages of V. anguillarum infection in young lumpfish (Cyclopterus lumpus). Fish Shellfish Immunol. 128, 523–535. doi: 10.1016/j.fsi.2022.08.023
Gendron R. L., Paradis H., Ahmad R., Kao K., Boyce D., Good W. V., et al. (2020). CD10+ Cells and igM in pathogen response in lumpfish (Cyclopterus lumpus) eye tissues. Front. Immunol. 11. doi: 10.3389/fimmu.2020.576897/full
Gentry K., Bui S., Oppedal F., Dempster T. (2020). Sea lice prevention strategies affect cleaner fish delousing efficacy in commercial Atlantic salmon sea cages. Aquac Environ. Interact. [Internet] 12, 67–80. doi: 10.3354/aei00348
Grimnes A., Jakobsen P. J. (1996). The physiological effects of salmon lice infection on post-smolt of Atlantic salmon. J. Fish Biol. 48, 1179–1194. doi: 10.1111/j.1095-8649.1996.tb01813.x
Gulla S., Duodu S., Nilsen A., Fossen I., Colquhoun D. J. (2016). Aeromonas salmonicida infection levels in pre- and post-stocked cleaner fish assessed by culture and an amended qPCR assay. J. Fish Dis. 39 (7), 867–877. doi: 10.1111/jfd.12420
Gutierrez Rabadan C., Spreadbury C., Consuegra S., Garcia de Leaniz C. (2021). Development, validation and testing of an Operational Welfare Score Index for farmed lumpfish Cyclopterus lumpus L. Aquaculture 531, 735777. doi: 10.1016/j.aquaculture.2020.735777
Haastein T., Holt G. (1972). The occurrence of vibrio disease in wild Norwegian fish. J. Fish Biol. 4, 33–37. doi: 10.1111/j.1095-8649.1972.tb05650.x
Hamre L., Bui S., Oppedal F., Skern-Mauritzen R., Dalvin S. (2019). Development of the salmon louse Lepeophtheirus salmonis parasitic stages in temperatures ranging from 3 to 24°C. Aquac Environ. Interact. 11, 429–443. doi: 10.3354/aei00320
Hnasko R. (2015). ELISA: Methods and Protocols, Methods in Molecular Biology Vol. 1318 (Springer New York Heidelberg Dordrecht London: Springer Science+Business Media New York). Available at: http://www.springer.com/series/7651. doi: 10.1007/978-1-4939-2742-5_6
Imsland A. K., Reynolds P., Eliassen G., Hangstad T. A., Foss A., Vikingstad E., et al. (2014a). The use of lumpfish (Cyclopterus lumpus L.) to control sea lice (Lepeophtheirus salmonis Krøyer) infestations in intensively farmed Atlantic salmon (Salmo salar L.). Aquaculture 424–425, 18–23. doi: 10.1016/j.aquaculture.2013.12.033
Imsland A. K., Reynolds P., Eliassen G., Hangstad T. A., Nytrø A. V., Foss A., et al. (2014b). Assessment of growth and sea lice infection levels in Atlantic salmon stocked in small-scale cages with lumpfish. Aquaculture 433, 137–142. doi: 10.1016/j.aquaculture.2014.06.008
Imsland A. K., Reynolds P., Eliassen G., Hangstad T. A., Nytrø A. V., Foss A., et al. (2015). Feeding preferences of lumpfish (Cyclopterus lumpus L.) maintained in open net-pens with Atlantic salmon (Salmo salar L.). Aquaculture 436, 47–51. doi: 10.1016/j.aquaculture.2014.10.048
Jonassen T., Hamadi M., Remø S. C., Waagbø R. (2017). An epidemiological study of cataracts in wild and farmed lumpfish (Cyclopterus lumpus L.) and the relation to nutrition. J. Fish Dis. 40 (12), 1903–1914. doi: 10.1111/jfd.12664
Kennedy D. A., Kurath G., Brito I. L., Purcell M. K., Read A. F., Winton J. R., et al. (2016). Potential drivers of virulence evolution in aquaculture. Evol. Appl. 9 (2), 344–354. doi: 10.1111/eva.12342
Kim E. Y., Kim Y. R., Kim D. G., Kong I. S. (2012). A susceptible protein by proteomic analysis from Vibrio anguillarum under various environmental conditions. Bioprocess Biosyst. Eng. 35 (1–2), 273–282. doi: 10.1007/s00449-011-0636-6
Kverme K. O., Kallekleiv M., Larsen K., Rønneseth A., Wergeland H. I., Samuelsen O. B., et al. (2022). Antibacterial treatment of lumpfish (Cyclopterus lumpus) experimentally challenged with Vibrio anguillarum, atypical Aeromonas salmonicida and Pasteurella atlantica. J. Fish Dis. 45 (1), 153–163. doi: 10.1111/jfd.13545
Liu Y., Vanhauwaer Bjelland H. (2014). Estimating costs of sea lice control strategy in Norway. Prev. Vet. Med. 117 (3–4), 469–477. doi: 10.1016/j.prevetmed.2014.08.018
Liu X., Wu H., Chang X., Tang Y., Liu Q., Zhang Y. (2014). Notable mucosal immune responses induced in the intestine of zebrafish (Danio rerio) bath-vaccinated with a live attenuated Vibrio anguillarum vaccine. Fish Shellfish Immunol. 40 (1), 99–108. doi: 10.1016/j.fsi.2014.06.030
Ma J., Bruce T. J., Jones E. M., Cain K. D. (2019). A review of fish vaccine development strategies: conventional methods and modern biotechnological approaches. Microorganisms 7 (11), 569. doi: 10.3390/microorganisms7110569
Ma Y., Wang Q., Xu W., Liu X., Gao X., Zhang Y. (2017). Stationary phase-dependent accumulation of ectoine is an efficient adaptation strategy in Vibrio anguillarum against cold stress. Microbiol. Res. 205, 8–18. doi: 10.1016/j.micres.2017.08.005
Machimbirike V. I., Vasquez I., Cao T., Chukwu-Osazuwa J., Onireti O., Segovia C., et al. (2023). Comparative genomic analysis of virulent Vibrio (Listonella) anguillarum serotypes revealed genetic diversity and genomic signatures in the O-antigen biosynthesis gene cluster. Microorgan [Internet] 11 (3), 792. doi: 10.3390/microorganisms11030792
Marcos-López M., Donald K., Stagg H., McCarthy U. (2013). Clinical Vibrio anguillarum infection in lumpsucker Cyclopterus lumpus in Scotland. Vet. Rec 173 (13), 319. doi: 10.1136/vr.101763
Marozzi C., Bertoni F., Randelli E., Buonocore F., Timperio A. M., Scapigliati G. (2012). A monoclonal antibody for the CD45 receptor in the teleost fish Dicentrarchus labrax. Dev. Comp. Immunol. 37 (3–4), 342–353. doi: 10.1016/j.dci.2012.03.015
Marshall J. S., Warrington R., Watson W., Kim H. L. (2018). An introduction to immunology and immunopathology. Allergy Asthma Clin. Immunol. 14 (S2), 49. doi: 10.1186/s13223-018-0278-1
Martinez-Espiñeira R., Chopin T., Robinson S., Noce A., Knowler D., Yip W. (2016). A contingent valuation of the biomitigation benefits of integrated multi-trophic aquaculture in Canada. Aquacult Econ Manage. 20 (1), 1–23. doi: 10.1080/13657305.2016.1124935
Maya L. (2019). Cleaner-fish technology now ready for commercialization. Aquacult North America 10 (5), 20–22.
Midtlyng P. J., Reitan L. J., Speilberg L. (1996). Experimental studies on the efficacy and side-effects of intraperitoneal vaccination of Atlantic salmon (Salmo salar L.) against furunculosis. Fish Shellfish Immunol. 6, 335–350. doi: 10.1006/fsim.1996.0034
Mohamad A., Mursidi F. A., Zamri-Saad M., Amal M. N. A., Annas S., Monir M. S., et al. (2022). Laboratory and field assessments of oral vibrio vaccine indicate the potential for protection against vibriosis in cultured marine fishes. Animals 12 (2), 133. doi: 10.3390/ani12020133
Mortensen S., Halvorsen K. T., Skiftesvik A. B., Durif C., Stien L. H., Jansson E., et al. (2021). Towards a sustainable fishery and use of cleaner fish in salmonid aquaculture - challenges and opportunities. Nordic Council Ministers.
Munang’andu H. M., Evensen Ø. (2019). Correlates of protective immunity for fish vaccines. Fish Shellfish Immunol. 85, 132–140. doi: 10.1016/j.fsi.2018.03.060
Nuñez Ortiz N., Gerdol M., Stocchi V., Marozzi C., Randelli E., Bernini C., et al. (2014). T cell transcripts and T cell activities in the gills of the teleost fish sea bass (Dicentrarchus labrax). Dev. Comp. Immunol. 47 (2), 309–318. doi: 10.1016/j.dci.2014.07.015
Nytrø A. V., Vikingstad E., Foss A., Hangstad T. A., Reynolds P., Eliassen G., et al. (2014). The effect of temperature and fish size on growth of juvenile lumpfish (Cyclopterus lumpus L.). Aquaculture 434, 296–302. doi: 10.1016/j.aquaculture.2014.07.028
Pampoulie C., Skirnisdottir S., Olafsdottir G., Helyar S. J., Thorsteinsson V., Jónsson SÞ, et al. (2014). Genetic structure of the lumpfish Cyclopterus lumpus across the North Atlantic. ICES J. Mar. Sci. 71 (9), 2390–2397. doi: 10.1093/icesjms/fsu071
Pike A. W., Wadsworth S. L. (1999). Sealice on salmonids: their biology and control. Adv. Parasitol. 44, 233–337. doi: 10.1016/S0065-308X(08)60233-X
Powell A., Treasurer J. W., Pooley C. L., Keay A. J., Lloyd R., Imsland A. K., et al. (2018). Use of lumpfish for sea-lice control in salmon farming: challenges and opportunities. Rev. Aquac 10 (3), 683–702. doi: 10.1111/raq.12194
Reynolds P., Imsland A. K. D., Boissonnot L. (2022). Causes of mortality and loss of lumpfish Cyclopterus lumpus. Fishes 7 (6), 328. doi: 10.3390/fishes7060328
Rønneseth A., Haugland G. T., Colquhoun D. J., Brudal E., Wergeland H. I. (2017). Protection and antibody reactivity following vaccination of lumpfish (Cyclopterus lumpus L.) against atypical Aeromonas salmonicida. Fish Shellfish Immunol. 64, 383–391. doi: 10.1016/j.fsi.2017.03.040
Rønsholdt B., McLean E. (1999). The effect of vaccination and vaccine components upon short-term growth and feed conversion efficiency in rainbow trout. Aquaculture 174 (3–4), 213–221. doi: 10.1016/S0044-8486(99)00016-2
Servín-Blanco R., Zamora-Alvarado R., Gevorkian G., Manoutcharian K. (2016). Antigenic variability: Obstacles on the road to vaccines against traditionally difficult targets. Hum. Vaccin Immunother. 12 (10), 2640–2648. doi: 10.1080/21645515.2016.1191718
Silvaraj S., Md Yasin IS A., Karim M. M., Saad M. Z. (2020). Elucidating the efficacy of vaccination against vibriosis in lates calcarifer using two recombinant protein vaccines containing the outer membrane protein k (r-ompk) of Vibrio alginolyticus and the dna chaperone j (r-dnaj) of Vibrio harveyi. Vaccines (Basel) 8 (4), 660. doi: 10.3390/vaccines8040660
Sommerset I., Walde C. S., Bang Jensen B., Wiik-Nielsen J., Bornø G., Henrique Silva de Oliveira V., et al. (2022) The Norwegian Veterinary Institute’s annual review of fish health in Norway Editors Suggested citations. Available at: www.vetinst.no.
Stosik M., Tokarz-Deptuła B., Deptuła W. (2021). Immunological memory in teleost fish. Fish Shellfish Immunol. 115, 95–103. doi: 10.1016/j.fsi.2021.05.022
Tang X., Liu F., Sheng X., Xing J., Zhan W. (2018). Recombinant NADP-dependent isocitrate dehydrogenase of Edwardsiella tarda induces both Th1 and Th2 type immune responses and evokes protective efficacy against edwardsiellosis. Vaccine 36 (17), 2337–2345. doi: 10.1016/j.vaccine.2018.03.022
Tattiyapong P., Dechavichitlead W., Waltzek T. B., Surachetpong W. (2020). Tilapia develop protective immunity including a humoral response following exposure to tilapia lake virus. Fish Shellfish Immunol. 106, 666–674. doi: 10.1016/j.fsi.2020.08.031
Toranzo A. E., Magariños B., Romalde J. L. (2005). A review of the main bacterial fish diseases in mariculture systems. Aquaculture 246 (1–4), 37–61. doi: 10.1016/j.aquaculture.2005.01.002
Toranzo A. E., Santos Y., Barja J. L. (1997). Immunization with bacterial antigens: Vibrio infections. Dev. Biol. Stand 90, 93–105.
Torres-Corral Y., Girons A., González-Barreiro O., Seoane R., Riaza A., Santos Y. (2021). Effect of Bivalent Vaccines against Vibrio anguillarum and Aeromonas salmonicida Subspecie achromogenes on Health and Survival of Turbot. Vaccines (Basel) 9 (8), 906. doi: 10.3390/vaccines9080906
Torrissen O., Jones S., Asche F., Guttormsen A., Skilbrei O. T., Nilsen F., et al. (2013). Salmon lice - impact on wild salmonids and salmon aquaculture. J. Fish Dis. 36 (3), 171–194. doi: 10.1111/jfd.12061
Torrissen O., Olsen R. E., Toresen R., Hemre G. I., Tacon A. G. J., Asche F., et al. (2011). Atlantic salmon (Salmo salar): the “Super-chicken” of the sea? Rev. Fish Sci. 19 (3), 257–278. doi: 10.1080/10641262.2011.597890
Treasurer J. W. (2002). A review of potential pathogens of sea lice and the application of cleaner fish in biological control. Pest Manag Sci. 58 (6), 546–558. doi: 10.1002/ps.509
Treasurer J. W. (2012). Diseases of north European wrasse (Labridae) and possible interactions with cohabited farmed salmon, Salmo salar L. J. Fish Dis. 35 (8), 555–562. doi: 10.1111/j.1365-2761.2012.01389.x
Vasconcelos P., Monteiro C. C., Santos M. N., Gaspar M. B. (2014). First record of the lumpfish (Cyclopterus lumpus Linnaeus, 1758) off the Algarve coast (southern Portugal): southward extension of the species distributional range. J. Appl. Ichthyol 20, 159–160. doi: 10.1046/j.1439-0426.2003.00531.x
Vasquez I., Cao T., Chakraborty S., Gnanagobal H., O’Brien N., Monk J., et al. (2020a). Comparative Genomics Analysis of Vibrio anguillarum Isolated from Lumpfish (Cyclopterus lumpus) in Newfoundland Reveal Novel Chromosomal Organizations. Microorganisms 8 (11), 1666. doi: 10.3390/microorganisms8111666
Vasquez I., Cao T., Hossain A., Valderrama K., Gnanagobal H., Dang M., et al. (2020b). Aeromonas salmonicida infection kinetics and protective immune response to vaccination in sablefish (Anoplopoma fimbria). Fish Shellfish Immunol. 104, 557–566. doi: 10.1016/j.fsi.2020.06.005
Xing J., Xu H., Tang X., Sheng X., Zhan W. (2019). A DNA vaccine encoding the VAA gene of Vibrio anguillarum induces a protective immune response in flounder. Front. Immunol. 10 (MAR). doi: 10.3389/fimmu.2019.00499/full
Yu L., Hu Y., Sun B., Sun L. (2012). C312M: an attenuated Vibrio anguillarum strain that induces immunoprotection as an oral and immersion vaccine. Dis. Aquat Organ 102 (1), 33–42. doi: 10.3354/dao02544
Keywords: sea lice, lumpfish, Vibrio anguillarum, vibriosis, Atlantic salmon, autogenous vaccine, field vaccine trial
Citation: Onireti OB, Cao T, Vasquez I, Chukwu-Osazuwa J, Gnanagobal H, Hossain A, Machimbirike VI, Hernandez-Reyes Y, Khoury A, Khoury A, O’Brien N, George S, Swanson A, Gendron RL, Kwabiah R, Tucker D, Monk J, Porter J, Boyce D and Santander J (2023) Evaluation of the protective efficiency of an autogenous Vibrio anguillarum vaccine in lumpfish (Cyclopterus lumpus) under controlled and field conditions in Atlantic Canada. Front. Aquac. 2:1306503. doi: 10.3389/faquc.2023.1306503
Received: 03 October 2023; Accepted: 17 November 2023;
Published: 22 December 2023.
Edited by:
Prabhugouda Siriyappagouder, Nord University, NorwayReviewed by:
Adnan H. Gora, Central Marine Fisheries Research Institute (ICAR), IndiaDeepti Manjari Patel, Nord University, Norway
M. Camino Ordás, Rey Juan Carlos University, Spain
Copyright © 2023 Onireti, Cao, Vasquez, Chukwu-Osazuwa, Gnanagobal, Hossain, Machimbirike, Hernandez-Reyes, Khoury, Khoury, O’Brien, George, Swanson, Gendron, Kwabiah, Tucker, Monk, Porter, Boyce and Santander. This is an open-access article distributed under the terms of the Creative Commons Attribution License (CC BY). The use, distribution or reproduction in other forums is permitted, provided the original author(s) and the copyright owner(s) are credited and that the original publication in this journal is cited, in accordance with accepted academic practice. No use, distribution or reproduction is permitted which does not comply with these terms.
*Correspondence: Javier Santander, anNhbnRhbmRlckBtdW4uY2E=