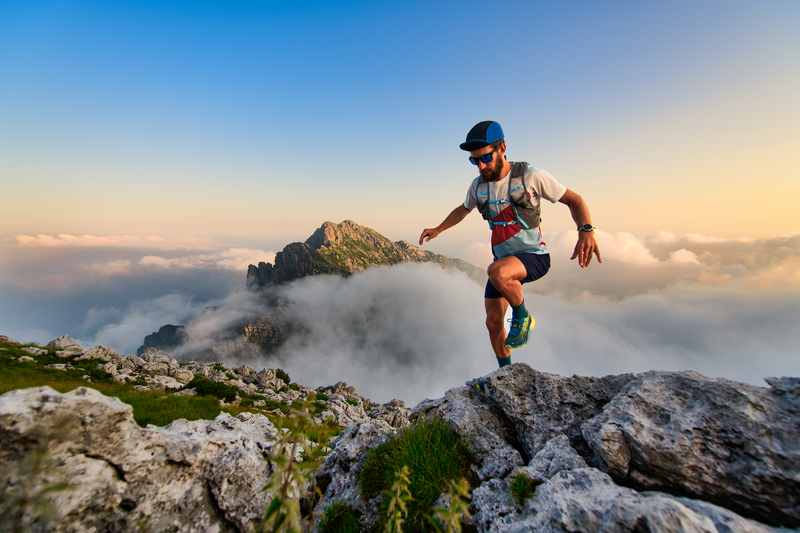
94% of researchers rate our articles as excellent or good
Learn more about the work of our research integrity team to safeguard the quality of each article we publish.
Find out more
ORIGINAL RESEARCH article
Front. Anim. Sci. , 19 November 2024
Sec. Animal Nutrition
Volume 5 - 2024 | https://doi.org/10.3389/fanim.2024.1459552
In recent years, the use of corn DDGS has alleviated the problem of protein feed shortages to a certain extent; however, its high mycotoxin content seriously restricts its application in feedstuffs. In this study, the conditions of solid-state fermented corn DDGS were optimized to investigate changes in the nutrient composition of solid-state fermented corn DDGS and their effects on the growth performance and histological section of yellow-feathered broilers. The optimal strain ratio for solid-state fermentation was determined by orthogonal testing as 1:1:2 for B. desmois JA3:B. subtilis ANSB01G: and B. subtilis ANSB060, and the response value was the score of the degradation rate of Deoxynivalenol (DON), zearalenone (ZEN), and aflatoxin B1 (AFB1) weighted by 4:4:2. Optimal conditions for the solid-state fermentation of maize DDGS were determined by B-Behnken response surface design: the inoculum amount was 1%, the feed to liquid ratio was 1:1.25, the fermentation temperature was 37°C, and the fermentation time was 29.5 h. The optimal conditions for solid-state fermentation of maize DDGS were as follows: the inoculum amount was 1%, the material-liquid ratio was 1:1.25, the fermentation temperature was 37°C, the fermentation time was 29.5h, and the degradation rates of DON, ZEN, and AFB1 in the fermented maize DDGS were 81.6%, 55.18%, and 41.14%, respectively. Moreover, solid-state fermentation significantly improved the nutrient content, in which the amounts of amino acids, crude ash, and crude fat were significantly increased (P < 0.05) and the content of crude fiber was significantly decreased (P < 0.05). The in vitro digestibility of dry matter, crude protein and crude fiber increased significantly (P < 0.05); in addition, compared with the control group, the addition of fermented maize DDGS significantly increased the daily calorific intake of broiler chickens, the daily weight gain (P < 0.05); heart, liver and intestinal tissues underwent no obvious pathological changes.
In recent years, the increasing shortage of raw feed ingredients such as corn and soybean meal, combined with rising prices, has made the issue of protein feed scarcity increasingly prominent (Guodong et al., 2018). The cost of compound feed, primarily composed of corn and soybean meal, has increased sharply, significantly affecting breeding enterprises and further raising their economic costs (Skinner et al., 2012). The primary strategy to address these problems is to develop new feed resources and alternative raw materials, with increasing attention being focused on corn DDGS. This is a byproduct formed by drying the remaining fermentation residues after the fermentation of corn seeds with selected yeast to produce ethanol at low temperatures (Chatzifragkou et al., 2015; El-Sheikh and Salama, 2020). This process preserves and concentrates nearly all the nutrients in corn, excluding starch, while featuring high levels of protein and fat; it is rich in amino acids, vitamins, and minerals; demonstrates comprehensive nutritional value and high yield (Corassa et al., 2019; Iram et al., 2020). However, as the nutrient content of maize DDGS becomes concentrated, the mycotoxin levels in maize DDGS increase significantly, exacerbating contamination concerns and complicating its utilization. In this context, removing mycotoxins becomes a crucial consideration for the effective utilization of corn DDGS and for enhancing its overall quality (Fahrenholz, 2008; Qingguo et al., 2019; Wickramasuriya et al., 2020; Shuo et al., 2020).
Fermented feed represents a nutritionally rich biological feedstock, characterized by increased palatability and viable bacterial concentration. Through microbial fermentation, it decomposes large molecules such as polysaccharides, proteins, and fats, producing organic acids, soluble peptides, and other small molecules under artificially controlled conditions (Adebayo-Tayo and Needum, 2011; Bo et al., 2018; Yang et al., 2021). Fermentation enhances feed quality, feed digestibility, and utilization while extending feed storage life by reducing anti-nutritional factors and degrading toxins. Additionally, it promotes growth, prevents diarrhea, and strengthens immunity by regulating the microecological balance. Research has shown that complex interrelationships influence the fermentation process involving mixed bacterial cultures (Smid and Lacroix, 2013; Zhang et al., 2021a). Zhai et al. (2020) investigated the effects of sources and levels of liquor distillers’ grains with solubles on the growth performance, carcass characteristics, and serum parameters of Cherry Valley ducks. They found that the dosage of corn DDGS negatively impacted the growth performance and health of broilers. Fermented DDGS increased final body weight (BW), average daily feed intake (ADFI), and thigh muscle yield while decreasing the feed conversion ratio (F:G) in ducks. Roberson et al. (2005) demonstrated that incorporating 10% corn DDGS into the diet significantly improved egg yolk color in laying hens, while also significantly increasing the linoleic acid content in the egg yolk. Corn DDGS contain higher metabolizable energy and crude protein content (Li et al., 2013), making them a viable alternative for inclusion in pig diets to reduce feed costs. Several microorganisms, including Bacillus subtilis (Gao et al., 2011) and Devosia sp (Wang et al., 2019), have been shown to effectively degrade toxins. Deoxynivalenol (DON) can be safely and efficiently degraded by Devosia, which produces two enzymes, DepA and DepB. DepA functions as an oxidase while DepB acts as a reductase. Through oxidation and reduction reactions, the epimerization of the 3-hydroxyl group in deoxynivalenol (DON) is accomplished, resulting in the formation of the stereoisomer 3-epi-DON, which is currently recognized as a relatively safe conversion product of deoxynivalenol (He et al., 2015). The characteristics of endophytic spores of Bacillus subtilis contribute to their functional stability in both feed production conditions and the internal environments of livestock. Additionally, these spores play a significant role in promoting intestinal health and enhancing the growth of livestock and poultry. In this study, Delvos bacteria and Bacillus subtilis were utilized as fermentation strains, with corn DDGS serving as the fermentation substrate. The aim was to optimize the technological conditions for the solid-state fermentation of corn DDGS, reduce the mycotoxin levels, enhance the nutritional value of the fermented corn DDGS feed, improve the overall quality of corn DDGS, and facilitate its application in animal nutrition.
Ingredients: corn DDGS (Mengzhou Houyuan Biotechnology Co., Ltd.).
The strains Devos JA3 (Bacterium A), B. subtilis ANSB01G (Bacterium B), and B. subtilis ANSB060 (Bacterium C) were provided by HeNan Trillion-Zhongyuan Bio-Tech. Co., Ltd.
Medium: Luria-Bertani (LB) liquid medium.
Instruments and equipment included a UV-visible spectrophotometer (model T6, Beijing Puxi General Instrument Co., Ltd. China), vortex mixer (model Voetex TM-1, Wiggens Technology Co., Ltd. China), electrothermal constant-temperature blast drying oven (model DH6-924385-III, Shanghai Xinmiao Medical Treatment Apparatus Manufacturing Co.,Ltd. China), one in ten thousand electronic balance (model FA2004, Shanghai SUNNY HENGPING Scientific Instrument Co., Ltd. China), biochemical incubator (model DNP-9272BS-III, Shanghai Xinmiao Medical Treatment Apparatus Manufacturing Co.,Ltd. China), pressure steam sterilizer (model LS-75HD, Jiangyin Binjiang Medical Equipment Co., Ltd. China), Kay-type nitrogen analyzer (model NKY-6120, Beijing Puxi General Instrument Co., Ltd. China), muffle furnace (model XL-2000, Chongqing Hengke Instrument Co., Ltd. China), oxygen bomb calorimeter(model XRY-1A,Zhejiang Juneng Instrument Equipment Co., Ltd. China), and enzyme label instrument (model Spark 10M, TECAN. CH).
Devos and B. subtilis on slant medium were inoculated into LB liquid medium and incubated at 37°C, 180 rpm for 12 h.
The mycotoxin levels in feed were evaluated by DON level detection kit, zearalenone (ZEN) detection kit and aflatoxin B1 (AFB1) detection kit (Shanghai Enzyme-linked Biotechnology Co., Ltd., Shanghai, China) and high performance liquid chromatography (HPLC), but the main method was HPLC method. Enzyme-linked immunosorbent assay was used to identify whether the results obtained by HPLC method were accurate; Dry matter (DM) was identified for samples. For this purpose samples were dried in an air-forced oven at 65°C for 48 h to determine the primary moisture content. Coarse ash (Ash) was determined by incineration at 550°C in mufle furnace for 12 h. Crude protein (CP) was determined by using Kjeldahl method (Thiex, 2009). Ether extract (EE) content was obtained through the Soxhlet extraction method using anhydrous diethyl ether (Bligh and Dyer, 1959). Crude fiber (CF) content was determined by using 12.5% H2SO4, and 12.5% NaOH solutions (Dong and Rasco, 1987). Gross energy (GE) content was determined using an automatic oxygen-nitrogen calorimeter.
Detection conditions of HPLC method for DON. Chromatographic column, C18 column (4.6mm*250mm, 5μm), Mobile phase, chromatographic acetonitrile+ deionized water (10 + 90, v/v). Flow rate, 0.8 mL/min. Injection volume, 20μL. Detection wavelength, UV detector, wavelength 218 nm; Detection conditions of HPLC method for ZEN. Chromatographic column, C18 column (4.6mm * 250mm, 5μm). Mobile phase, acetonitrile + water + methanol (46 + 46 + 8, v/v). Flow rate, 1.0 mL/min. Injection volume, 20 μL. Detection wavelength, fluorescence detector. excitation wavelength 274 nm. Emission wavelength 440 nm; Detection conditions of HPLC method for AFB1. Chromatographic column, C18 column (4.6mm * 250mm, 5μm). Mobile phase, methanol + water (45+55, v/v). Flow rate, 0.8 mL/min. Injection volume, 20 μL. Detection wavelength, fluorescence detector. Excitation wavelength 360 nm. Emission wavelength 440 nm.
The experimental design of the inoculation ratio is shown in Table 1.
Optimization of strain ratio at 1% inoculum was performed, and a three-bacteria co-fermentation test was conducted. The initial material-to-water ratio was 1:1. A total of 1,000 g of corn DDGS was placed in a fermentation tank under the condition of neutral pH, with constant temperature fermentation at 37°C for 36 h. The degradation rates of DON, ZEN, and AFB1 in fermented corn DDGS were measured at a 4:4:2 ratio.
According to the inoculation ratio determined in the previous section, a single-factor experiment was designed and mixed fermentation with the three bacteria was conducted. Based on the degradation rates of DON, ZEN, and AFB1, the degradation rate of fermented corn DDGS was weighted be 4:4:2. The effects of inoculation amount, fermentation temperature, ratio of feed to water, and fermentation time on the DDGS of fermented maize were studied. The initial fermentation conditions included: inoculum quantity, 1%; fermentation temperature, 37°C; liquid-solid ratio, 1:1; and fermentation time, 36 h. Factors and levels of the Box-Behnken response surface test are listed in Table 2.
According to the principles of the Box-Behnken central combination design, response surface analysis tests were designed for 29 experimental sites with four factors and three levels: including liquid-solid ratio, inoculation amount, fermentation time, and fermentation temperature. SAS 9.2 software was used to determine the factor level of the response surface analysis. Theoretical optimal conditions were obtained from the test results, and further tests were conducted to verify the effectiveness of the model.
The changes of moisture, crude fiber, crude fat, crude ash, crude protein and energy content of corn DDGS before and after fermentation were determined and the detection method was referred to the part of 1.4.
SDS-special kit (Hunan Zhongben Intelligent Technology Development Co., Ltd.), a special digestive enzyme reagent for chicken digestion, was used for in vitro digestion test. The specific steps are as follows,
Gastric digestion stage: Approximately 4 g of sifted samples and 4 mL of simulated gastric solution (Pepsin was dissolved in 250 mL hydrochloric acid solution with pH 2.0, and the simulated gastric solution with pepsin activity of 1550 U/mL was prepared) were added to a 60 mL gastric buffer solution (NaCl 2.59 g, KCl 0.25 g and NaH2PO4 6 g were put into beaker and added water to 500 mL, then adjusted pH to 2 with 6 mol/L hydrochloric acid solution) containing 2 mL of 0.5 mg/mL chloramphenicol ethanol solution. The mixture was adjusted to pH 3 with 0.2 mol/mL hydrochloric acid solution and incubated at 38°C for 4 h.
Small intestinal fluid enzyme hydrolysis stage: At the end of the gastric digestion stage, 40 mL of small intestinal buffer solution (NaH2PO4 5.84 g, NaHPO4 7.99 g, penicillin 1.6 million U and C6H7O2K 1 g were put into a beaker and diluted to 500 ml with water. The pH was adjusted to 7.15 with 6 mol/L sodium hydroxide solution) was added to the solution, and the pH of the digestive solution was adjusted to 6.6 with 1 mol/L NaOH solution. Then, 4 mL of small intestinal simulated solution and 1.6 million U of penicillin sodium salt were added to the mixture and incubated at 38°C for 4 h.
Filtrable stage: After digestion, 20 mL 20% sulfosalicylic acid solution was added to the digestion residue, placed at 25°C for 30 min. The residue was vacuumized, and filtered to determine the dry matter, crude protein and crude fiber content.
A total of 600 one-day-old Linnan yellow-feathered broiler chickens (fast-growing breed), comprising 300 males and 300 females, weighing an average of 37.70 ± 0.27 g were divided randomly into seven groups, consisting of 6 replicates of 10 chickens each, which were then denoted as control group (corn-soybean meal basal diet)、experimental groups 1, 2, and 3 (basal diet with 5%, 10%, and 15% corn DDGS, respectively),experimental groups 4, 5, and 6 (basal diet with 5%, 10%, and 15% fermented corn DDGS, respectively). Their composition and nutritional levels are listed in Table 3. The feeding program included two feeds during the chicken-raising (1–21 d) and breeding (22–42 d) stages. The experiment was conducted in an experimental chicken house in Liuweiyuan in Zhongmou County, Zhengzhou City, Henan Province. The cage-rearing method was adopted in this experiment. Each 10 chickens were raised in a cage of 150 × 80 × 60 cm. The broiler house was kept ventilated, hygienic, and illuminated for 24 h. Broilers were equally distributed in areas with the same temperature, humidity, and light intensity to eliminate environmental bias caused by different cage positions.
On days 1, 21, and 42, fasted weighing was performed on broilers after ceasing eating for 12 h in advance. Body weight (BW) and feed weight was recorded. Average daily gain (ADG), daily feed intake (DFI), and feed conversion (FC) were determined from these data.
On the 42nd day, one sample was randomly selected from each replicate of seven experimental groups, for a total of 42 samples. The chickens were killed by cervical dislocation method. After bloodletting through the neck, Blood samples were collected from 42 test chickens, and 10 mL carotid blood was taken from each of them and placed in a coarse coagulation tube, which was placed at room temperature for 30 min and then centrifuged at 3,000 r/min at 4°C for 10 min to prepare serum. The serum was transferred to a 2 mL centrifuge tube and stored in a −80°C freezer for later use. The total superoxide dismutase (T-SOD), glutathione peroxidase (GSH-Px), malondialdehyde (MDA), and total antioxidant capability (T-AOC) levels were determined using a kit produced by the Nanjing Jiancheng Institute of Biological Engineering.
On the 42nd day, one sample was randomly selected from each replicate of seven experimental groups, for a total of 42 samples. The chickens were killed by cervical dislocation method. After bloodletting through the neck. Liver, heart and jejunum tissues of 42 chickens were collected, and the same parts were cut into small pieces and placed in a tube, frozen in liquid nitrogen, and stored in a freezer at −80°C. Paraffin-embedded liver, heart and jejunum tissues were sliced (5 μm) and stained with hematoxylin and eosin (H&E).
At the end of the trial, one sample was randomly selected from each replicate of seven experimental groups, for a total of 42 samples. The middle part of the jejunum was cut out about 1 cm, washed with normal saline, and placed in 4% formaldehyde fixing solution.The paraformaldehyde-fixed jejunal tissue specimens were cut into longitudinal sections, encased with petrolatum, and sliced (5 μm), and then observed morphologically with hematoxylin and eosin staining. The marked segments were initially viewed under low magnification, and the suitable locations were picked for picture capturing under high magnification, all done using a light microscope. With the aid of the picture analysis program Image ProPlus 6.0, three readings were done on each slide for villi height and crypt depth.
The experimental data were initially collated using Excel 2021, and a single-factor analysis of variance was performed using SAS 9.2 software. The results are represented as means ± standard deviation, and P < 0.05 was used as the criterion for statistically significant differences.
The effects of various inoculation ratios on the fermentation of DDGS from maize are presented in Table 4.
Based on the degradation rates of DON, ZEN, and AFB1 following fermentation, the highest mycotoxin degradation rate in fermented corn DDGS was observed in experimental group 6, with a weighted result of 48.944, achieved with an inoculation ratio of Devosia bacterium JA3, B. subtilis ANSB01G, and B. subtilis ANSB060 of 1:1:2.
As illustrated in the Figure 1A, the degradation rate of mycotoxins generally showed a trend of increasing and then decreasing with rising inoculation amounts, reaching a maximum at an inoculation level of 1% before declining. Overall, utilizing a higher inoculation level and encouraging microbial growth will accelerate the degradation process, peaking to achieve the desired effect (Hu et al., 2008). This is due to the capacity of numerous microorganisms to rapidly produce substantial amounts of hydrolases, which facilitate the fermentation of the substrate matrix (Yang et al., 2021; Liu et al., 2023). However, excessive inoculation can result in overgrowth of microbial colonies, leading to increased viscosity of the culture medium and insufficient dissolved oxygen, thus negatively impacting the fermentation process. It can also cause a rapid rise in fermentation temperature, which is challenging to control (Juanjuan et al., 2011). Conversely, insufficient inoculation alters fermentation time and increases the risk of bacterial contamination. Through a comprehensive evaluation of the experimental results, it can be concluded that the optimal inoculation ratio is 1%.
Figure 1. The effects of inoculation amount (A), solid-liquid ratio (B), fermentation temperature (C) and fermentation time (D) on corn DDGS fermentation.
As illustrated in the Figure 1B, as the liquid-to-solid ratio increases, the degradation rate of DDGS mycotoxins in fermented corn first increases and then decreases, reaching a peak value of 80.168%at a liquid-to-solid ratio of 1.1. Water in the fermentation substrate dissolves various nutrients, facilitating their direct utilization by microorganisms; however, both excessive and insufficient water levels can hinder the growth and reproduction of microorganisms (Wang et al., 2011). Extremely low moisture content inhibits the growth and reproduction of microorganisms, while excessive moisture content reduces the porosity of the matrix, air volume, and permeability, consequently affecting the growth and reproduction of bacteria (Wang et al., 2010).
As illustrated in the Figure 1C, the degradation rate of mycotoxins in fermented corn DDGS initially increased and then subsequently decreased with the rise in fermentation temperature, peaking at a value of 56.594% at 37°C, before gradually declining. Hence, 37°C was chosen as the optimal fermentation temperature. Each type of microorganism has an optimal growth temperature, and variations in temperature can significantly influence their growth and metabolism, thereby altering their growth rates (Gonzalez and Aranda, 2023). Furthermore, excessively high or low temperatures hinder microbial growth and metabolism, adversely affecting the fermentation process. A one-way analysis of variance (ANOVA) confirmed the significant impact of fermentation temperature on mycotoxin levels in fermented corn DDGS.
As illustrated in the Figure 1D, the mycotoxin degradation rate of fermented corn DDGS exhibited an increasing trend within 12–36 h, peaking at a maximum value of 46.656% at 36 h before gradually declining. If the fermentation time is insufficient, the number of active microorganisms on the fermentation substrate is relatively small, resulting in low enzyme activity, which adversely affects the breakdown of macromolecular substances. Conversely, excessive fermentation time generates significant bioheat from energy consumption during fermentation, which accelerates bacterial aging and negatively impacts enzyme activity in high-temperature conditions (Sun et al., 2013).
A single-factor test indicated that the inoculation amount, liquid-to-solid ratio, fermentation temperature, and fermentation time significantly influenced the degradation of mycotoxin levels in corn DDGS. The test design factors and levels of response surface analysis based on the findings from the single-factor test are outlined in Table 5. The dynamic parametric equation for the optimized response value was analyzed utilizing SAS version 9.2 software.
Y=−582.43+77.24A+99.18B+26.99C+2.59D−60.74AB+5.37AC+0.04AD+3.89BC−0.22BD−0.015CD−99.91A2−69.60B2−0.50C2−0.028D2
SAS version 9.2 software was utilized for the analysis. The design and findings of the response surface test are summarized in Table 6. Optimal technological conditions were established, followed by a validation test to confirm the accuracy of the model predictions.
SAS 9.2 software was used to analyze the regression model, and the response surface and contour plots of DDGS weighted scores of fermented maize were obtained; these were influenced by four factors: inoculation amount, liquid-solid ratio, fermentation temperature, and fermentation time.
According to Figure 2, the three-dimensional analysis and the corresponding contour diagrams of each response surface illustrate the interaction between two independent variables maintained at specific levels. As shown in Figure 2B, the interaction between A (inoculation amount) and C (temperature) is significant (P = 0.0289 < 0.05), indicating that there is no simple linear relationship between these variables and mycotoxin content following the fermentation of DDGS from corn. Within a certain range of A, increasing both A and B leads to an initial increase in the degradation rate of mycotoxins, which subsequently decreases after reaching a maximum. Figures 2A, D also demonstrate that the interactions AB (P = 0.1901) and BC (P = 0.0993) contribute to increased degradation rates of mycotoxins in the DDGS of fermented maize, although the significance of these interactions is not substantial. Table 7 presents the results of the response surface variance analysis.
Figure 2. Response surface and contour plots of the interaction between inoculated amount and liquid-solid ratio (A), inoculation amount and fermentation temperature (B), inoculation amount and fermentation time (C), liquid-solid ratio and fermentation temperature (D), liquid-solid ratio and fermentation time (E), fermentation temperature and fermentation time (F).
As shown in the Table, P < 0.01 of the model indicated that the quadratic power of the model was extremely significant, whereas the missing fitting item is not significant, indicating the absence of abnormal data in the model. The effects of each factor on the degradation rate of mycotoxins followed the order: liquid–solid ratio > inoculum quantity > fermentation time > fermentation temperature. Through variance analysis of the regression equation, it can be concluded that primary item B was extremely significant (P < 0.01); A, C, and D were significant (P < 0.05); secondary item C2 was extremely significant (P < 0.01); A2, B2 and D2 were significant (P < 0.05); interaction item AC was significant (P < 0.05); and AB, AD, BC, BD, and CD were not significant (P > 0.05), indicating the efficiency of the regression equation in describing the real relationship between each factor and the response value. Table 8 shows variance analysis of the quadratic regression equation.
The complex correlation coefficient of the regression equation was 0.8567, the correction correlation coefficient was 0.7133, the coefficient of variation was 6.62%, and the signal-to-noise ratio was 8.368, indicating a good degree of fit of the regression equation.
After deriving the quadratic model and response surface, the maximum point on the surface is determined by calculating the extreme value of the quadratic nonlinear model equation. The optimization results obtained from solving the maximum value of the regression equation are presented in Table 9.
When A=1.02, B=1.25, C=36.98, D=29.46, that is, when the inoculation amount was 1.02%, the ratio of liquid to solid was 1.25, the fermentation temperature was 36.98°C, and the fermentation time was 29.46 h, the degradation rates of DON, ZEN, and AFB1 in DDGS of fermented corn were 4:4:2. The predicted weighted score was 62.927%.
Based on the optimal fermentation parameters and actual conditions, the resulting optimal fermentation conditions were as follows: inoculation quantity 1%, liquid-to-material ratio 1.25, fermentation temperature 37°C, and fermentation time 29.5 h. The degradation rate of mycotoxins in the DDGS of fermented corn was 62.976%, while the theoretical result predicted by the model was 62.927%. The good fit between the theoretical and experimental values confirmed the validity of the model and demonstrated its high efficiency in accurately reflecting actual fermentation conditions.
Composition analysis serves as the foundation for feed identification. Accurately determining feed composition is critical for enhancing the feed utilization rate and effectively managing feed quality. The value and quality of fermented corn DDGS can be assessed by analyzing its conventional components. Table 10 illustrates the changes in the conventional components of DDGS due to fermentation in corn.
Solid-state fermentation enhances the nutritional content of corn DDGS and improves its nutritional value. As shown in the table, the contents of EE, Ash, and moisture in corn before and after DDGS fermentation were significantly different (P < 0.05), with increases of 5.44%, 8.27%, and 473.67%, respectively. In contrast, the contents of CF and GE decreased by 37.37% and 6.51%, respectively (P < 0.05). Although the CP content improved, no significant difference was observed when compared to the values before fermentation. Jazi et al. (2017) demonstrated an increase in CP content during their study on fermented cottonseed meal, which aligns with the findings of the present study. This increase is attributed to the elevated protein content resulting from enzymes produced by microorganisms during fermentation, along with their growth and reproduction, which promote the synthesis of bacterial proteins. Additionally, during fermentation, the reduction in dry matter can contribute to a corresponding increase in CP content. This study also observed a significant increase in the ash content of corn DDGS during fermentation, aligning with the findings reported by Gungor and Erener (2020). This change may be due to a decrease in the contents of nitrogen-free extract, crude fiber, neutral detergent fiber, acid detergent fiber, and lignin. Alad et al. (2013) conducted solid-state fermentation on a mixture of cassava root and palm kernel cake, finding a significant reduction in CF content. Consistent with the findings of this study, the CF content in fermented corn DDGS also decreased, and a moderate amount of CF may promote intestinal peristalsis, thereby enhancing digestion and absorption in animals. This reduction in CF content may be attributed to the cellulase produced by bacteria, which decomposes cellulose, consequently lowering the CF content (Liu et al., 2020). Ma Wenqiang et al. (2008) investigated the nutrient content of soybean meal before and after fermentation using B. subtilis, Saccharomyces cerevisiae, and lactic acid bacteria, and found that the EE content increased by 18.18% after fermentation, aligning with the findings of this experiment. Consistent with the results of this study, the DM content of fermented corn DDGS was significantly lower than that of unfermented corn DDGS, likely due to the substantial addition of water during the fermentation process or the strong affinity of the newly formed molecular complexes for water molecules (Feiming et al., 2018). Furthermore, the reduction in GE content may be attributed to the loss of nutrients and energy during bacterial growth and reproduction.
During the fermentation of corn DDGS, macromolecular proteins are gradually transformed into small peptides and free amino acids, resulting in alterations to the amino acid content, as presented in Table 11.
Table 11. Changes in amino acid contents of corn before and after DDGS fermentation (dry matter content).
The demand for proteins by animals parallels the demand for amino acids. In animals, proteins are digested, absorbed, and utilized as free amino acids and small peptides (Ullrich et al., 2019). Essential amino acids can only be sourced from feed and cannot be synthesized de novo. As shown in the table, levels of aspartic acid, threonine, serine, valine, glutamic acid, alanine, leucine, tyrosine, phenylalanine, and proline significantly increased during fermentation (P < 0.05). Conversely, lysine content decreased from 0.84% before fermentation to 0.64% after fermentation. The total free amino acid content increased significantly from 27.18% to 29.44% (P < 0.05) and the total amino acid content increased significantly after fermentation (P < 0.05). Numerous studies have demonstrated that the levels of most free amino acids in fermentation substrates are significantly enhanced following the microbial fermentation of feed ingredients. Gebing et al. (2011) utilized mixed fermentation of cottonseed protein and found that the free amino acid content in the fermented product was significantly higher compared to single-strain fermentation, with both methods leading to substantial increases in amino acid levels. In a study on the solid-state fermentation of rapeseed meal, Changyou et al. (2015) reported significant increases in the contents of total amino acids and essential amino acids, including aspartic acid, serine, phenylalanine, threonine, and tyrosine, following fermentation. Consistent with the findings of this experiment, both the partial amino acid content and total amino acid content of fermented corn DDGS were significantly enhanced. This increase can be attributed to the gradual conversion of macromolecular proteins into small peptides and free amino acids during the fermentation process of corn DDGS. However, a significant decrease in lysine content was observed following fermentation, which may be linked to factors such as the strain type, inoculation amount, and fermentation conditions (Wang et al., 2017a).
The main reason for the application of corn DDGS is that the mycotoxin content in corn DDGS was significantly higher. Consequently, the changes in mycotoxin levels in both unfermented and fermented corn DDGS predominantly involve the toxins DON, ZEN, and AFB1. The test results are presented in Table 12.
Analysis of Table 12 indicates that the detoxification effect of strain fermentation on corn DDGS was significant (P < 0.05), with degradation rates of DON, ZEN, and AFB1 recorded at 81.69%, 55.18%, and 41.14%, respectively. Yuanhythi et al. (2022) examined corn DDGS, and other by-products, identifying DON, ZEN, and AFB1 as the primary contaminants. The detection rates of AFB1 in corn DDGS were 94.91% and 90.83% for 2019 and 2020, respectively, while the detection rates of ZEN and DON remained consistently at 100.00% for both years. The primary mechanism through which fermentation reduces mycotoxin content is competition for nutrients between mixed strains and molds, along with the production of antibacterial substances that directly inhibit mold growth and mycotoxin formation. Nakayama et al. demonstrated that certain strains of Bacillus subtilis can produce iturin, an antibacterial compound, which consequently inhibits mycotoxin production. Moreover, mixed bacteria generate metabolites that bind to mycotoxins during growth, thereby reducing their harmful effects. Zhang et al. (2021b) conducted a study on five strains of lactic acid bacteria and found that one of these strains exhibited a high capacity to degrade AFB1 during solid-state fermentation of wheat bran substrate.
Changes in the in vitro digestibility of dry matter, crude protein, and crude fiber before and after DDGS fermentation are shown in Table 13.
Table 13. Changes in in vitro digestibility of dry matter, protein and crude fiber before and after DDGS fermentation of corn.
The term “digestibility” refers to the proportion of nutrients in feed that can be digested and absorbed by animals. Measuring digestibility—such as for dry matter (DM), crude protein (CP), and crude fiber (CF)—is a crucial indicator for assessing feed quality. A higher digestibility value indicates a greater extent of nutrient utilization by the animal, thus enhancing the nutritional value of the feed. In vitro digestibility of DM, CP, and CF in fermented corn DDGS was significantly greater than in corn DDGS (P < 0.05), with increases of 42.18%, 15.12%, and 28.33%, respectively. This is likely attributable to B. subtilis and Devosia’s ability to synthesize proteases, cellulases, and other enzymes, which soften the tissue of corn DDGS during fermentation, increase the content of soluble substances, decompose macromolecular components, and enhance the digestion rate of corn DDGS. The effects of liquid fermentation on the in vitro digestibility of feed ingredients were investigated by Zhao et al. (Na et al., 2011). The in vitro digestibility of DM and CP in corn and corn-soybean meal diets at different ratios after fermentation was higher than that of the control group. This improvement may be due to enhanced protein quality of the raw materials post-fermentation, which increased the availability of digestive enzymes and, consequently, the in vitro digestibility of CP. The findings from Zhang Chunji’s study (Chunji, 2023) corroborated these results, indicating that the in vitro digestibility of DM, CP, and CF in fermented feed was significantly elevated.
The effects of fermented corn DDGS on growth performance of broilers are shown in Table 14. Growth performance serves as the most direct indicator of broiler production and is crucial for enhancing economic returns (Upadhayay et al., 2014). Recently, fermentation has attracted considerable attention from researchers as a method for improving the nutritional quality of feed and increasing the yield of livestock and poultry products.
Table 14. Effects of adding different levels of fermented corn DDGS on growth performance of broilers.
During the three growth stages (1–21d, 22–42d, and 1–42d), the ADG, ADFI, and FCR of broilers fed a diet containing fermented DDGS exhibited both linear and quadratic changes compared to the control group. As the fermented corn DDGS addition increased, the ADG and ADFI of broilers correspondingly increased, while the FCR showed an inverse relationship. No significant changes in BW were observed at 21 or 42 days of age (P > 0.05). Chah et al. found that fermented soybeans improved nitrogen and dry matter utilization in broiler diets, thereby enhancing ADG and FCR. Furthermore, Ashayerizadeh et al. (2018) demonstrated that substituting soybean meal with fermented oilseed meal significantly enhanced the ADG of broilers. These results align with those of the current study. One reason for these findings is that fermented feed is readily digestible and absorbable by animals. The removal of anti-nutritional factors from feed ingredients can enhance the nutritional quality, palatability, and digestibility of the feed, thereby improving the growth performance of poultry (Zhou et al., 2023). In broilers fed corn DDGS, ADG and FCR values exhibited linear and quadratic changes at 21–42 d and 1–42 d, while body weight at 21 d and 42 d also showed similar linear and quadratic trends compared to the control group. No significant changes in ADFI, ADG, or FCR were observed on 1–21 d, nor in ADFI values on 1–42 d and 21–42 d (P > 0.05). Compared to the control group, the body weight of broilers at 21 and 42 days of age significantly decreased with increasing levels of corn DDGS in the diet (P < 0.05). The ADG values for broilers fed diets containing 10% and 15% corn DDGS were significantly reduced during the 21–42 day and 1–42 day periods (P < 0.05), while the FCR values significantly increased (P < 0.05). Trenholm et al. (1994) demonstrated that when the concentration of DON is between 0–14 mg/kg, each 1 mg/kg increase in DON leads to a 6% reduction in feed intake of pigs; if the concentration reaches 10 mg/kg or more, this results in an anti-feeding phenomenon. The ADG, ADFI, and FCR of AA broilers were significantly decreased when 400 μg/kg of AFB1 was added to the feed. In this experiment, compared to the control group, the BW of broilers fed corn DDGS was significantly lower, and the FCR increased, indicating that the presence of mycotoxins can impair the growth performance of the broilers. However, the growth performance of broilers receiving a fermented corn DDGS diet improved, suggesting that solid-state fermentation effectively reduces mycotoxin levels in corn DDGS and mitigates the negative effects of these mycotoxins on broiler production performance.
The effects of fermented corn DDGS on serum antioxidant properties of broilers are shown in Table 15.
SOD and GSH-Px are crucial antioxidant enzymes in the body that can be induced by increased levels of free radical compounds. Their concentration and activity significantly influence the level of reactive oxygen radicals in the body (Al-Jassabi, 2004; Wills, 1965). The MDA levels indirectly reflect the extent of cellular damage and indicate the degree of lipid peroxidation. T-AOC serves as a comprehensive indicator of the body’s antioxidant system functionality, reflecting both the antioxidant enzyme system and free radical metabolism (Tang et al., 2019). Diets supplemented with corn DDGS and fermented corn DDGS led to elevated serum levels of T-AOC, MDA, and GSH-Px in broilers (P < 0.05), while there was no significant effect on T-SOD levels (P > 0.05). In the present study, both linear and quadratic decreases in T-AOC and linear and quadratic increases in MDA levels were observed in broiler serum with the increased addition of corn DDGS. When corn DDGS was supplemented at 15%, the serum T-AOC levels in broilers were significantly lower than those in the control group, which may be attributed to the oxidative stress damage caused by mycotoxins present in the feed. Furthermore, the presence of multiple mycotoxins exacerbates oxidative stress in the animals (Wang et al., 2017b). The levels of GSH-Px in the serum of broiler chickens exhibited both linear and quadratic increases (P < 0.001) with the addition of fermented corn and corn DDGS, which were significantly higher than those in the control group. The T-SOD content in the serum of broilers was not significantly affected (P > 0.05) by the supplementation of corn DDGS or fermented corn DDGS. These findings suggest that the addition of fermented corn DDGS to the diets of broilers can prevent lipid peroxidation (Xiaoshuai et al., 2017). This effect may be attributed to the presence of bacteria and functional components such as polysaccharides, phenols, and flavonoids, which could serve as effective mechanisms to alleviate the burden on both enzymatic and non-enzymatic antioxidant systems and reduce cellular antioxidant consumption (Tao et al., 2020).
The effects of fermented corn DDGS on morphological changes in the liver tissue are shown in Figure 3.
Figure 3. Liver tissue pathology (hematoxylin−eosin staining; magnification, x200) (black arrows indicate the position of inflammatory cell) in the following extract groups: (control) control group; (1) experimental group 1; (2) experimental group 2; (3) experimental group 3; (4) experimental group 4; (5) experimental group 5; (6) experimental group 6.
The liver is the largest and most essential metabolic and detoxification organ in the body, making it vulnerable to various pathogenic factors (El-Bahrawy et al., 2016). The figure demonstrates that the hepatic tissue structure in the control group appeared normal, exhibiting an orderly distribution of hepatic cells with clear contours. No significant degeneration or infiltration of inflammatory cells was observed in these tissues. In Experimental Group 1, the structure of the liver tissue was slightly abnormal, with minor inflammatory cell infiltration noted in the portal area, indicated by the black arrow, while liver cell structure remained intact without significant degeneration. In Experimental Group 2, moderate abnormalities were noted in the liver tissue, characterized by a slightly loose arrangement of hepatic cells, dilated hepatic sinuses, and a small amount of inflammatory cell infiltration in the portal area. The number of inflammatory cells increased compared to Experimental Group 1, as depicted by the black arrow. In Experimental Group 3, severe abnormalities were evident in the liver tissues, marked by a significant influx of inflammatory cells into the portal area. The degree of inflammatory infiltration was the most pronounced, as indicated by the black arrows. In Experimental Groups 4, 5, and 6, the liver tissues exhibited a predominantly normal structure, with clear outlines of liver cells and no evident degeneration. This degree was similar to that of the control group, with no significant differences noted. Dolensek et al. (2021) discovered that both DON and ZEN could induce liver lesions in mice. Furthermore, co-infection with DON and ZEN increased the rate of apoptosis in liver and kidney cells of mice, resulting in tissue damage in both organs. Deng Hui et al. (2022) demonstrated that substituting soybean meal with fermented expanded rapeseed meal did not produce significant pathological damage to the hepatocytes of broilers. Furthermore, the inclusion of fermented expanded rapeseed meal was not associated with the degree of liver cell damage. In a similar study, Feng Jian et al. (2016) replaced fish meal with fermented soybean meal while examining the liver histology of juvenile large yellow croakers. They noted that the liver tissues remained relatively normal in both the 15% and 30% fermented soybean meal groups, which aligns with the findings of this study. These results suggest that fermented corn DDGS may possess the potential to mitigate mycotoxin effects and prevent mycotoxin-induced alterations in tissue morphology.
The effects of fermented corn DDGS on cardiac tissue morphology are shown in Figure 4.
Figure 4. Heart tissue pathology (hematoxylin−eosin staining; magnification, x200) in the following extract groups: (control) control group; (1) experimental group 1 (black arrows indicate the position of heterophil granulocyte); (2) experimental group 2 (black arrows indicate the position of lymphocyte); (3) experimental group 3 (black arrows indicate the position of heterophil granulocyte and red arrows indicate the position of lymphocyte); (4) experimental group 4; (5) experimental group 5; (6) experimental group 6.
The effects of fermented corn DDGS on cardiac tissue morphology are illustrated in Figure 4. Research indicates that broiler chickens are more sensitive to mycotoxins, which can significantly impact their cardiac health. Aflatoxins are known to impair mitochondrial function in cardiomyocytes, activate the production of ROS, induce apoptosis, and lead to considerable damage and toxicity in cardiomyocytes (Wang et al., 2017).The myocardial tissue structure of the control group appeared normal, with myocardial fibers arranged tightly and orderly, clearly contoured nuclei, no increased spacing, and no infiltration of inflammatory cells in the tissue. In Experimental Group 1, the myocardial tissue structure exhibited slight abnormalities, characterized by a looser arrangement of myocardial fibers, localized enlargement of spaces, and a small infiltration of inflammatory cells, predominantly consisting of heterophil granulocytes, as indicated by the black arrow. In Experimental Group 2, the myocardial tissue structure displayed moderate abnormalities, with a significant number of myocardial fibers arranged unevenly, enlarged spaces, and notable infiltration of inflammatory cells, primarily comprising lymphocytes. As indicated by the black arrow, the degree of damage was greater when compared to Experimental Group 1. In Experimental Group 3, the myocardial tissue structure exhibited pronounced abnormalities, characterized by loosely arranged myocardial fibers, increased gaps, and significant infiltration of inflammatory cells. The inflammatory cells included heterophils (as indicated by black arrows) and lymphocytes (as indicated by red arrows). In Experimental Groups 4, 5, and 6, the myocardial tissue structure appeared normal, with closely arranged and orderly myocardial fibers, well-defined nuclear contours, and no increase in gaps. The degree of damage was comparable to that observed in the control group, although the differences were not statistically significant.
The effects of fermented corn DDGS on morphological changes in the intestinal tissue of broilers are illustrated in Figure 5.
Figure 5. intestinal tissue pathology (hematoxylin−eosin staining; magnification, x200) in the following extract groups: (control) control group (black arrows indicate the position of mucosal epithelial cells arranged closely and orderly); (1) experimental group 1 (black arrows indicate the position of mucosal epithelial cells erosion off); (2) experimental group 2 (black arrows indicate the position of mucosal epithelial cells erosion off); (3) experimental group 3 (black arrows indicate the position of Mucosal epithelial cells erosion off); (4) experimental group 4; (5) experimental group 5; (6) experimental group 6.
The intestine serves as the primary site for digestion and absorption in animals, making intestinal development crucial for their health and growth. From the figure, it can be observed that the jejunal tissue structure in the control group was normal, with elongated villi and closely arranged, orderly mucosal epithelial cells, exhibiting no noticeable degeneration or shedding, as indicated by the black arrow. In Experimental Group 1, the jejunum tissue exhibited slight damage, with some mucosal epithelial cells eroded and detached, as indicated by the black arrows. The jejunum tissue in Experimental Group 2 displayed moderate damage, characterized by erosion and exfoliation of some mucosal epithelial cells. The incidence of mucosal epithelial cell erosions and shedding significantly increased compared to that observed in Experimental Group 1, as indicated by the black arrow. Experimental Group 3 displayed severe jejunal tissue damage, characterized by extensive erosion and detachment of epithelial cells, with the lamina propria also being detached, as indicated by the black arrows. The jejunal tissues of Experimental Groups 4, 5, and 6 were comparable to those of the control group, showing no significant lesions or injuries. DON induces intestinal damage by inhibiting protein synthesis in intestinal epithelial cells. In this study, the increase in the corn DDGS content in the feed corresponded with elevated levels of mycotoxins, leading to intestinal lesions in broilers. However, no significant differences were observed between Experimental Groups 4, 5, and 6 and the control group, suggesting that fermentation effectively reduced the mycotoxin levels present in maize DDGS.
The results of VH, CD, and VH/CD in the intestinal tract of broilers fed fermented corn DDGS are shown in Table 16.
The jejunum serves as the primary site for nutrient digestion and absorption in broiler chickens, and the development of jejunal villi is a critical indicator of the jejunum’s digestive and absorptive functions (Soumeh et al., 2019). The addition of corn DDGS to the diet resulted in both linear and quadratic decreases in jejunal villus height (VH) and the ratio of villus height to crypt depth (CD) in broilers. The VH/CD ratio reflects the functional status of the jejunum; an increase in this ratio enhances mucosal integrity, improves digestion and absorption, and promotes growth and development. Conversely, a decrease in this ratio signals mucosal damage and a reduction in digestive and absorptive capabilities. In contrast, the incorporation of fermented maize DDGS into the diet led to linear and quadratic increases in the height of jejunal villi in broilers. While the addition of both maize DDGS and fermented maize DDGS to the diet increased the crypt depth, there was no significant effect on the depth of jejunal crypts in broilers fed the fermented maize DDGS. CD serves as an indicator of the rate of cell generation in the intestines. Shallow crypts suggest an increased maturation of intestinal epithelial cells and enhanced absorptive capacity. The addition of fermented feeds significantly decreased the CD in both the duodenum and jejunum, while an increased VH/CD ratio was reported by Liu et al. (2021). Shi Hongtao et al. (2019) investigated the effects of fermented astragalus on the intestinal tissue morphology of broilers and discovered that the inclusion of the fermented astragalus group significantly enhanced VH, markedly reduced CD, and substantially increased the VH/CD ratio compared to the control group and the addition of astragalus. This indicates that fermented astragalus can effectively improve the intestinal tissue morphology of broilers. Consistent with these findings, the present study demonstrated that feeding fermented corn DDGS improved both VH and VH/CD values in the jejunum of broilers. This suggests that the addition of fermented corn DDGS is beneficial for increasing VH/CD values, enhancing VH, promoting nutrient absorption, and improving the intestinal morphology of broilers. These improvements may be attributed to metabolites produced during fermentation, including organic acids, small-molecule peptides, and amino acids, which provide essential nutrients that facilitate the proliferation of intestinal epithelial cells, thus enhancing the nutritional status and morphology of the intestinal tract.
In the present study, the inoculation ratio of the mixed fermentation strains Devosia JA3, B. subtilis ANSB01G, and B. subtilis ANSB060 was evaluated through an orthogonal experiment. The conditions for solid-state fermentation of corn distillers dried grains with solubles (DDGS) were optimized using a single-factor test and a response surface design test. After microbial fermentation, the levels of DON, ZEN, and AFB1 in corn DDGS were significantly reduced. The fermentation process enhanced the nutritional characteristics of corn DDGS. However, incorporating corn DDGS into feed resulted in decreased growth performance in broilers and caused varying degrees of damage to the liver, heart, and intestine, as well as reduced antioxidant capacity and intestinal performance. In contrast, adding fermented corn DDGS improved the growth performance of broilers, repaired damage to the heart, liver, and intestine, enhanced antioxidant capacity, and improved intestinal function.
The datasets presented in this study can be found in online repositories. The names of the repository/repositories and accession number(s) can be found in the article/supplementary material.
The studies involving humans were approved by Scientific Ethics Committee of Henan University of Technology. The studies were conducted in accordance with the local legislation and institutional requirements. The participants provided their written informed consent to participate in this study. The animal studies were approved by Scientific Ethics Committee of Henan University of Technology. The studies were conducted in accordance with the local legislation and institutional requirements. Written informed consent was obtained from the owners for the participation of their animals in this study.
YP: Conceptualization, Data curation, Formal analysis, Investigation, Methodology, Software, Writing – original draft, Writing – review & editing. MW: Conceptualization, Data curation, Formal analysis, Methodology, Validation, Writing – original draft. ZZ: Data curation, Investigation, Software, Validation, Writing – review & editing. LW: Conceptualization, Methodology, Software, Validation, Writing – review & editing. XZ: Formal analysis, Methodology, Project administration, Supervision, Writing – review & editing. KC: Formal analysis, Methodology, Supervision, Writing – review & editing. YZ: Formal analysis, Funding acquisition, Methodology, Project administration, Resources, Supervision, Visualization, Writing – review & editing.
The author(s) declare financial support was received for the research, authorship, and/or publication of this article. This study was supported by Henan Science and Technology Research Project (222102110446), and High-level Talents Research Start-up Fund of Henan University of Technology (31401528).
This study was supported by Henan Science and Technology Research Project (222102110446), and High-level Talents Research Start-up Fund of Henan University of Technology (31401528).
The authors declare that the research was conducted in the absence of any commercial or financial relationships that could be construed as a potential conflict of interest.
All claims expressed in this article are solely those of the authors and do not necessarily represent those of their affiliated organizations, or those of the publisher, the editors and the reviewers. Any product that may be evaluated in this article, or claim that may be made by its manufacturer, is not guaranteed or endorsed by the publisher.
Adebayo-Tayo B. C., Needum G. E. (2011). Microbiological, physicochemical and sensory evaluation of "ori-ese" produced from fortified sorghum. Afr. J. Food Agric. Nutr. Dev. 11, 4785–4799. doi: 10.4314/ajfand.v11i3.66630
Aladi N. O., Isinguzo C., Okorondu S., Okoli I. C., Okeudo N. J. (2013). Improvements on the physicochemical characteristics of cassava root pulp and palm kernel mixtures under solid state fungal fermentation. Int. J. Agric. Technol. 9 (5), 1111–1124.
Al-Jassabi S. (2004). Effect of methylglyoxal on antioxidant enzymes of the liver and spleen of the mice. J. Biol. Sci. 4, 605–608. doi: 10.3923/jbs.2004.605.608
Ashayerizadeh A., Dastar B., Shams Shargh M., Sadeghi Mahoonak A. R., Zerehdaran S. (2018). Effects of feeding fermented rapeseed meal on growth performance, gastrointestinal microflora population, blood metabolites, meat quality, and lipid metabolism in broiler chickens - sciencedirect. Livest. Sci. 216, 183–190. doi: 10.1016/j.livsci.2018.08.012
Bligh E. G., Dyer W. J. (1959). A rapid method of total fat extraction and purification. Can. J. Biochem. Physiol. 37, 911–9177. doi: 10.1139/o59-099
Bo H., Bin Z., Peng C., Yuanhua Q., Xueliang F. (2018). Production and application of microbial fermentation feed. Farm Products Processing. 21, 68–70. doi: 10.16693/j.cnki.1671-9646(X).2018.11.018
Changyou S., He J., Yu J., Yu B., Zhiqing H., Xiangbing M., et al. (2015). Solid state fermentation of rapeseed cake with aspergillus Niger for degrading glucosinolates and upgrading nutritional value. J. Anim. Sci. Biotechnol. 6, 7. doi: 10.1186/s40104-015-0015-2
Chatzifragkou A., Kosik O., Prabhakumari P. C., Lovegrove A., Frazier R. A., Shewry P. R., et al. (2015). Biorefinery strategies for upgrading distillers' dried grains with solubles (ddgs). Process Biochem. 50, 2194–2207. doi: 10.1016/j.procbio.2015.09.005
Chunji Z. (2023). Effects of different fermentation time on nutrient components, mycotoxin content andenergy digestibility in vitro of complete feed. China Feed 02), 33–36. doi: 10.15906/j.cnki.cn11-2975/s.20230209
Corassa A., Stuani J. L., Ton A. P. S., Kiefer C., Gonalves D. B. C. (2019). Nutritional value of distillers dried grains with solubles from corn and sorghum and xylanase in diets for pigs. Rev. Bras. Zootecnia. 48, e20190012. doi: 10.1590/rbz4820190012
Dolensek T., Svara T., Knific T., Gombac M., Luzar B., Jakovac-Strajn B. (2021). The influence of Fusarium mycotoxins on the liver of gilts and their suckling piglets. Animals. 11, 2534. doi: 10.3390/ani11092534
Dong F. M., Rasco B. A. (1987). The neutral detergent fiber, acid detergent fiber, crude fiber, and lignin contents of distillers’ dried grains with solubles. J. Food Sci. 52, 403–405. doi: 10.1111/j.1365-2621.1987.tb06624.x
El-Bahrawy A., Zaid A., Sunden Y., Sakurai M., Ito H., Ito T., et al. (2016). Pathogenesis of renal lesions in chickens after experimental infection with 9a5b newcastle disease virus mutant isolate. Vet. Pathol. 54, 94–98. doi: 10.1177/0300985816655852
El-Sheikh S., Salama A. (2020). Utilization of dry corn grains distillation by-product with soluble (ddgs) as an alternative non-conventional feed stuff in laying hen diets. Egyptian J. Nutr. Feeds. 23, 473–483. doi: 10.21608/EJNF.2020.148140
Fahrenholz A. C. (2008). The effects of ddgs inclusion on pellet quality and pelleting performance. Kansas State Univ. Available at: http://hdl.handle.net/2097/1077.
Feiming L., Xia L., Renzhi H., Junwen A., Xinpei Y., Xi H., et al. (2018). Effects of fermented mulberry leaf feed on growth,slaughtering performance and meat quality of ningxiang crossbred pig. Acta Sericologica Sinica. 44 (6), 0929–0935. doi: 10.13441/j.cnki.cykx.2018.06.015
Gao X., Ma Q., Zhao L., Lei Y., Shan Y., Ji C. (2011). Isolation of Bacillus subtilis: screening for aflatoxins B 1, M 1, and G 1 detoxification. Eur. Food Res. Technol. 232, 957–962. doi: 10.1007/s00217-011-1463-3
Gebing Z., Jun L., Huiying F., Jing S., Meng Z., Gejian Z. (2011). Optimization of mixed fermentation on cottonseed meal and research on the synergisticeffect of b.subtilis h1 and a.niger p1. China Biotechnol. 31, 62–68. doi: 10.13523/j.cb.20110910
Gonzalez J., Aranda B. (2023). Microbial growth under limiting conditions-future perspectives. Microoeganisms 11, 1641. doi: 10.3390/microorganisms11071641
Gungor E., Erener G. (2020). Effect of dietary raw and fermented sour cherry kernel (prunus cerasus l.) On growth performance, carcass traits, and meat quality in broiler chickens. Poult. Sci. 99 (1), 301–309. doi: 10.3382/ps/pez490
Guodong H., Xiaolan L., Yan W., Chunxia Y., Chengying J., Weigong J., et al. (2018). Study on solid fermentation of corn yellow meal feed by mixed bacteria. Feed Industry. 39, 44–48. doi: 10.13302/j.cnki.fi.2018.16.010
He J. W., Bondy G. S., Zhou T., Caldwell D., Boland G. J., Scott P. M. (2015). Toxicology of 3-epi-deoxynivalenol, a deoxynivalenol-transformation product by Devosia mutans 17-2-E-8. Food Chem. Toxicol. 84, 250–259. doi: 10.1016/j.fct.2015.09.003
Hongtao S., Hongxing Q., Mingxia F., Xu Z. (2019). Effect of fermented astragalus on intestinal tissue morphology of broilers. Heilongjiang Anim. Sci. Veterinary. 16, 139–142. doi: 10.13881/j.cnki.hljxmsy.2018.09.0329
Hu J., Lu W., Wang C., Zhu R., Qiao J. (2008). Characteristics of solid-state fermented feed and its effects on performance and nutrient digestibility in growing-finishing pigs. Asian Australas. J. Anim. Sci. 21, 1635–1641. doi: 10.5713/ajas.2008.80032
Hui D., Shulin W., Dong Q., Bin L. (2022). Effects of the extruded rapeseed meal fermented in advance on meat quality and flavor, liverpathology of broilers. China Feed. 11), 133–137. doi: 10.15906/j.cnki.cn11-2975/s.20221124
Iram A., Cekmecelioglu D., Demirci A. (2020). Distillers' dried grains with solubles (ddgs) and its potential as fermentation feedstock. Appl. Microbiol. Biot. 104, 6115–6128. doi: 10.1007/s00253-020-10682-0
Jazi V., Boldaji F., Dastar B., Hashemi S. R., Ashayerizadeh A. (2017). Effects of fermented cottonseed meal on the growth performance, gastrointestinal microflora population and small intestinal morphology in broiler chickens. Br. Poult. Sci. 58, 402–408. doi: 10.1080/00071668.2017.1315051
Jian F., Ping W., Jiaojiao H., Yudong L., Hui D., Rong D. (2016). Effects of replacement of fish meal by fermented soybean meal on growthperformance,body composition,serum biochemical indices and liver tissue morphology ofjuvenile large yellow croaker(larimichthys crocea). Chin. J. Anim. Nutr. 28, 3493–3502. doi: 10.3969/j.issn.1006-267x.2016.11.016
Juanjuan W., Shunxi W., Wenqing L. (2011). Effects of antibiotics-free solid-state fermented feed on immune response and antioxidant status of piglet. China Feed. 16, 25–27. doi: 10.15906/j.cnki.cn11-2975/s.2011.16.010
Li F., Liu Y., Yin R. Q., Yang X. J., Yao J. H., Sun F. F., et al. (2013). Nitrogen-corrected true metabolizable energy and amino acid digestibility of chinese corn distillers dried grains with solubles in adult cecectomized roosters. Asian Australas. J. Anim. Sci. 26, 838–844. doi: 10.5713/ajas.2012.12594
Liu S., Du M., Tu Y., You W., Chen W., Liu G., et al. (2023). Fermented mixed feed alters growth performance,carcass traits,meat quality and muscle fatty acid and amino acid profiles in finishing pigs. Anim. Nutr. 12, 87–95. doi: 10.1016/j.aninu.2022.09.003
Liu Y., Feng J., Wang Y., Lv J., Li J., Guo L., et al. (2021). Fermented corn-soybean meal mixed feed modulates intestinal morphology, barrier functions and cecal microbiota in laying hens. Animals 11 (11), 3059. doi: 10.3390/ani11113059
Liu H., Huang X., Xiao Q., Yu Y., Deng L., Wang F. (2020). Production of cellulase by microbulbifer hydrolyticus through co-fermentation of glucose and xylose from lignocellulose. BioResources 15, 8689–8695. doi: 10.15376/biores.15.4.8689-8695
Na Z., Shaozhang L., Xuehai Y., Jintao W., Shaowen H. (2011). Effect of liquid fermented feedstuff on digestibility coefficient in vitro. Feed Industry. 32 (11), 25–26. doi: 1001-991X(2011)11-0025-02
Qingguo J., Riyu W., Yaodong G., Lisheng F., Yipeng X., Jing C., et al. (2019). Effects of dietary supplemental distillers dried grains with solubles on growth performance,rumen fermentation and nutrient digestibility of dairy heifers. China Feed. 6, 22–25. doi: 10.15906/j.cnki.cn11-2975/s.20190605
Roberson K. D., Kalbfleisch J. L., Pan W., Charbeneau R. A. (2005). Effect of corn distiller`s dried grains with solubles at various levels on performance of laying hens and egg yolk color. Int. J. Poultry Science. 4, 44–51. doi: 10.3923/ijps.2005.44.51
Shuo Z., Qingxiang M., Hao W. U., Zhenming Z. (2020). Research progress on application of microbial fermented feed in ruminants production. Chin. J. Anim. Science. 01, 25–29. doi: 10.19556/j.0258-7033.20190227-05
Skinner S., Weersink A., Delange C. F. (2012). Impact of dried distillers grains with solubles (ddgs) on ration and fertilizer costs of swine farmers. Can. J. Agric. Economics/revue Can. Dagroeconomie. 60, 335–356. doi: 10.1111/j.1744-7976.2011.01237.x
Smid E. J., Lacroix C. (2013). Microbe–microbe interactions in mixed culture food fermentations. Curr. Opin. Biotechnol. 24, 148–154. doi: 10.1016/j.copbio.2012.11.007
Soumeh E. A., Mohebodini H., Toghyani M., Shabani A., Ashayerizadeh A., Jazi V. (2019). Synergistic effects of fermented soybean meal and mannan-oligosaccharide on growth performance, digestive functions, and hepatic gene expression in broiler chickens. Poultry science. 98, 6797–6807. doi: 10.3382/ps/pez409
Sun H., Tang J. W., Fang C. L., Yao X. H., Wu Y. F., Wang X., et al. (2013). Molecular analysis of intestinal bacterial microbiota of broiler chickens fed diets containing fermented cottonseed meal. Poultry Science. 92, 392–401. doi: 10.3382/ps.2012-02533
Tang Q., Su Y. W., Xian C. J. (2019). Determining oxidative damage by lipid peroxidation assay in rat serum. Bio-Protocol. 9, 3263. doi: 10.21769/BioProtoc.3263
Tao H., Si B., Xu W., Tu Y., Diao Q. (2020). Effect of broussonetia papyrifera l. Silage on blood biochemical parameters, growth performance, meat amino acids and fatty acids compositions in beef cattle. Anim. Biosci. 5, 732–741. doi: 10.5713/AJAS.19.0150
Thiex N. (2009). Evaluation of analytical methods for the determination of moisture, crude protein, crude fat, and crude fiber in distillers dried grains with solubles. J. AOAC Int. 92, 61–73. doi: 10.1093/jaoac/92.1.61
Trenholm H. L., Thompson B. K., Foster B. C., Charmley L. L., Albassam M. A. (1994). Effects of feeding diets containing fusarium (naturally) contaminated wheat or pure deoxynivalenol (don) in growing pigs. Can. J. Anim. Sci. 74, 361–369. doi: 10.4141/cjas94-049
Ullrich C., Langeheine M., Brehm R., Taube V., Galera M. R., Rohn K., et al. (2019). Influence of different methionine sources on performance and slaughter characteristics of broilers. Animals: an Open Access J. MDPI. 9, 984. doi: 10.3390/ani9110984
Upadhayay U. P. P. D. D., Vishwa P. C. V.. (2014). Growth promoters and novel feed additives improving poultry production and health, bioactive principles and beneficial applications: the trends and advances-a review. Int. J. Pharmacol. 10 (3), 129-159. doi: 10.3923/ijp.2014.129.159
Wang Y., Deng Q., Song D., Wang W., Li A. (2017). Effects of fermented cottonseed meal on growth performance, serum biochemical parameters, immune functions, antioxidative abilities, and cecal microflora in broilers. Food Agric. Immunol. 28, 725–738. doi: 10.1080/09540105.2017.1311308
Wang J. P., Liu N., Song M. Y., Qin C. L., Ma C. S. (2011). Effect of enzymolytic soybean meal on growth performance, nutrient digestibility and immune function of growing broilers. Anim. Feed Sci. Technology. 169, 224–229. doi: 10.1016/j.anifeedsci.2011.06.012
Wang G., Wang Y., Ji F., Xu L., Yu M., Shi J., et al. (2019). Biodegradation of deoxynivalenol and its derivatives by Devosia insulae A16. Food Chem. 276, 436–442. doi: 10.1016/j.foodchem.2018.10.011
Wang T. Y., Wu Y. H., Jiang C. Y., Liu Y. (2010). Solid state fermented potato pulp can be used as poultry feed. Br. Poult. Sci. 51, 229–234. doi: 10.1080/00071661003781864
Wang W. J., Xu Z. L., Cheng Y., Xu X. H. (2017a). Effects of aflatoxin B1 on mitochondrial respiration, ROS generation and apoptosis in broiler cardiomyocytes. Anim. Sci. J. 10, 1561–1568. doi: 10.1111/asj.12796
Wang W. J., Xu Z. L., Yu C., Xu X. H. (2017b). Effects of aflatoxin b1 on mitochondrial respiration, ros generation and apoptosis in broiler cardiomyocytes. Anim. Sci. J. 10, 1561–1568. doi: 10.1111/asj.12796
Wenqiang M., Jie F., Xin L. (2008). Nutritional characteristics of microbe fermented soybean mea. J. Chin. Cereals Oils Assoc. 23, 121–124. doi: 10.19556/j.0258-7033.20190227-05
Wickramasuriya S., Macelline S. P., Kim E., Cho H. M., Shin T. K., Yi Y. J., et al. (2020). Physiological impact on layer chickens fed corn distiller's dried grains with solubles naturally contaminated with deoxynivalenol. ASIAN-AUSTRALASIAN J. OF Anim. Sci. 33, 313–322. doi: 10.5713/ajas.19.0199
Wills E. D. (1965). Mechanisms of lipid peroxide formation in tissues role of metals and haematin proteins in the catalysis of the oxidation of unsaturated fatty acids. Biochim. Biophys. Acta (BBA) - Lipids Lipid Metab. 98, 238–251. doi: 10.1016/0005-2760(65)90118-9
Xiaoshuai C., Haiming Y., Jun M., Zhiyue W., Hui Z., Zhengfeng Y. (2017). Effects of compound probiotics on growth performance, slaughter performance, immune organ index and serum biochemical parameters of squabs. Chin. J. Of Anim. Nutr. 29, 2384–2390. doi: 10.3969/j.issn.1006-267x.2017.07.021
Yang L., Zeng X., Qiao S. (2021). Advances in research on solid-state fermented feed and its utilization: the pioneer of private customization for intestinal microorganisms - sciencedirect. Anim. Nutr. 7, 905–916. doi: 10.1016/j.aninu.2021.06.002
Yuanpei L., Jianchuan Z., Wenge Z., Lihong Z., Cheng J. (2022). A survey report on the mycotoxin contamination of compound feed and lts raw materialsof China in 2019-2020. Feed Industry. 43, 59–64. doi: 10.13302/j.cnki.fi.2022.20.010
Zhai S. S., Tian L., Zhang X. F., Wang H., Li M. M., Li X. C., et al. (2020). Effects of sources and levels of liquor distiller's grains with solubles on the growth performance, carcass characteristics, and serum parameters of cherry valley ducks. Poultry Science. 99, 6258–6266. doi: 10.1016/j.psj.2020.07.025
Zhang Y., Ishikawa M., Koshio S., Yokoyama S., Dossou S., Wang W., et al. (2021a). Optimization of soybean meal fermentation for aqua-feed with bacillus subtilis natto using the response surface methodology. Fermentation. 7, 306. doi: 10.3390/fermentation7040306
Zhang Y., Wang P., Kong Q., Cotty P. J. (2021b). Biotransformation of aflatoxin b1 by lactobacillus helviticus fam22155 in wheat bran by solid-state fermentation. Food Chem. 341, 128180.1–128180.9. doi: 10.1016/j.foodchem.2020.128180
Zhou X., Zhang H., Li S., Jiang Y., Deng J., Yang C., et al. (2023). Effects of different levels of citri sarcodactylis fructus by-products fermented feed on growth performance, serum biochemical, and intestinal health of cyan-shank partridge birds. Sci. Rep. 13 (1), 20130. doi: 10.1038/s41598-023-47303-5
Keywords: response surface method, mixed bacterial solid fermentation, corn DDGS, fermentation quality, growth, antioxidant status
Citation: Peng Y, Wang M, Zheng Z, Wang L, Zhang X, Cheng K and Zhang Y (2024) Optimization of solid-state fermented corn distillers dried grains with solubles: effects on growth performance and tissue morphology in broiler chickens. Front. Anim. Sci. 5:1459552. doi: 10.3389/fanim.2024.1459552
Received: 05 July 2024; Accepted: 08 October 2024;
Published: 19 November 2024.
Edited by:
Anusorn Cherdthong, Khon Kaen University, ThailandReviewed by:
Mayra A. D. Saleh, University of the Azores, PortugalCopyright © 2024 Peng, Wang, Zheng, Wang, Zhang, Cheng and Zhang. This is an open-access article distributed under the terms of the Creative Commons Attribution License (CC BY). The use, distribution or reproduction in other forums is permitted, provided the original author(s) and the copyright owner(s) are credited and that the original publication in this journal is cited, in accordance with accepted academic practice. No use, distribution or reproduction is permitted which does not comply with these terms.
*Correspondence: Yong Zhang, eW9uZ3poYW5nMjA4QGhhdXQuZWR1LmNu
Disclaimer: All claims expressed in this article are solely those of the authors and do not necessarily represent those of their affiliated organizations, or those of the publisher, the editors and the reviewers. Any product that may be evaluated in this article or claim that may be made by its manufacturer is not guaranteed or endorsed by the publisher.
Research integrity at Frontiers
Learn more about the work of our research integrity team to safeguard the quality of each article we publish.