- 1Department of Behavioural Biology, University of Münster, Münster, Germany
- 2Deutsche Forschungsgemeinschaft (DFG) Research Training Group EvoPAD, University of Münster, Münster, Germany
- 3Department of Behavioural Biology, Osnabrück University, Osnabrück, Germany
- 4Center of Cellular Nanoanalytics, Osnabrück University, Osnabrück, Germany
- 5Experimental Endocrinology, Department of Biological Sciences and Pathobiology, University of Veterinary Medicine, Vienna, Austria
To ensure good welfare of animals in human hands, it is essential to modify housing conditions according to the animals’ needs. Traditionally, the effects of such modifications are studied by means of group-level comparisons, thereby widely neglecting consistent inter-individual differences (i.e., so-called ‘animal personalities’). However, as animals with distinct personality types might differ in their environmental needs and hence react differently to the same environment, such systematic inter-individual differences might have important welfare consequences. This becomes particularly apparent under laboratory conditions, where animals are typically housed under highly standardized and barren environments. Against this background, we here aim to investigate personality-dependent welfare consequences in response to different housing conditions in laboratory mice. Female C57BL/6J mice were characterized for their personality type in exploration behavior and the most and the least explorative individuals were set up in either simple or in highly complex housing conditions that included constantly changing environmental enrichment items. We monitored individual welfare by studying behavioral, physiological, and immunological outcome measures. Besides personality-dependent differences in immune parameters and overall improved welfare under complex housing conditions, we indeed found hints that individual mice were differently affected in their welfare depending on the specific combination of personality type and housing condition. Specifically, highly explorative mice appeared to be more adversely affected by simple housing, but also profited more from complex housing compared to low explorative mice. These findings indicate that welfare promoting adjustments do not necessarily benefit all individuals equally and therefore, call for a shift of perspectives in the evaluation of animal welfare.
1 Introduction
Growing ethical awareness increasingly emphasizes the importance of ensuring good welfare for animals in human hands. For animals in captivity, the housing conditions represent one of the main targets for improving animal welfare. Whereas restrictive housing conditions often impair animal welfare, enriched housing conditions have several positive effects on the welfare of captive animals, like improved immunocompetence, anti-cancer and anti-obesity effects, improved cognitive abilities, increased neurogenesis, delayed disease progression and a reduced hypothalamic–pituitary–adrenal (HPA) activity, a good indicator for reduced stress responses (e.g., Kempermann et al., 1997; Nithianantharajah and Hannan, 2006; Meijer et al., 2007; Cao et al., 2010, 2011; Zheng et al., 2020). Furthermore, animals in enriched housing environments show less anxiety- and depression-like behaviors and less abnormal behaviors, like stereotypies (e.g., Gross et al., 2011; Jha et al., 2011; Fureix et al., 2016; Bahi, 2017; Hobbiesiefken et al., 2021). Different enrichment items, like running wheels, structural elements, tunnels or shelter, or the usage of different materials, achieve these positive effects as they overall motivate physical activity and increase sensory, motor and cognitive stimulation (Slater and Cao, 2015).
So far, these studies have concentrated on group-level comparisons. However, in 2008 Donald Broom already pointed out, that welfare is about the individual. And indeed, related fields, such as behavioral ecology puts the individual more at the center and extensively investigates repeatable individual behavioral differences (i.e., differences that are consistent across time and/or context) (Réale et al., 2007). Under the term animal personality, such consistent inter-individual differences have been reported in many taxa, ranging from invertebrates to mammals (Gosling, 2001; Bell et al., 2009). Furthermore, they have been found to arise despite identical genetic and environmental background (Freund et al., 2013; Bierbach et al., 2017). Importantly, personality differences have also been shown to have ecological consequences, e.g., individuals with different personality types are differently adapted to their environment (e.g., Sih et al., 2004; Réale et al., 2007; Laskowski et al., 2022) and some personality types are assumed to be better suited than others to thrive under specific environmental conditions (Eccard et al., 2022).
As difficulties in adapting to the environment are often associated with poor welfare (Broom, 2008), the question arises, whether personality differences might bear a consequence for animal welfare, too. More precisely, the query is as to whether the effects of enriched housing on animal welfare described above, affect all individuals in the same way or whether individuals with different personality types are affected differently. In line with this assumption, few studies already suggest that animal personality might influence the welfare of animals in captivity (Huntingford and Adams, 2005; Tetley and O’Hara, 2012; Carere and Maestripieri, 2013; Richter and Hintze, 2019). For example, though not focusing on the interplay between personality and housing, personality differences in rats have been linked to differences in stress levels (Franks et al., 2014). Likewise, consistent individual differences in stress coping strategies in mice and rats have been discussed to have consequences for health and disease (de Boer et al., 2017). However, empirical studies which systematically investigate the welfare consequences of personality differences in different housing environments are still missing.
Against this background, we here transfer the concept of animal personality from behavioral ecology to animal welfare science and argue that the personality of animals might be relevant to ensure good welfare of laboratory animals. More specifically, by using laboratory mice, we aimed to investigate whether the combination of personality type and housing condition results in differences in individual welfare. As in laboratory practice, housing conditions are typically designed in such a way that they constrain exploration behavior, we concentrated on consistent individual differences in exploration and contrasted two housing environments, a simple and a more complex environment. Previous studies have already shown that exploration behavior is one of the most repeatable behavioral traits in animals (Bell et al., 2009), making it a good candidate to explore personality-dependent responses to simple or complex environments. Based on the assumption that personality-dependent differences in environmental needs exist, we hypothesized that highly explorative mice show stronger welfare impairments in simple conditions compared to low explorative mice. Contrastingly, we expected the personality types to show more similar welfare responses in complex conditions as such conditions, because of their diversity, can presumably satisfy the environmental needs of multiple personality types. In addition to interaction effects between personality and housing on animal welfare, we also expected to find housing-independent differences between the personality types and overall improved welfare in more complex compared to simple housing conditions. Welfare was assessed by behavioral (e.g., Mason, 1991; Yalcin et al., 2007; Gaskill et al., 2013; Brown et al., 2017), physiological (e.g., Baumans, 2010; Hau et al., 2016; Bailoo et al., 2018a) and immunological (e.g., Staley et al., 2018; Ambrée et al., 2019) indicators.
2 Methods
2.1 Animals and housing conditions
The study included 72 female C57BL/6J mice, ordered from Charles River Laboratories (Research Models and Services, Germany GmbH, Sulzfeld, Germany) at about 4 weeks of age (PND (postnatal day) 28; please note that Charles River Laboratories delivers mice with an age range from PND 28 – 34, however, for simplicity the age of all mice was considered as PND 28).
Upon arrival, animals were housed in groups of four individuals per cage (= initial housing conditions). Allocation to cages was balanced with respect to catchability out of the delivery boxes (i.e., mouse 1 in cage 1, mouse 2 in cage 2, etc.). To enable individual identification within a cage, mice received partial ear punches. No analgesics were applied, as this routine procedure induces only slight and short-lived pain (Taitt and Kendall, 2019). During this initial housing phase, mice were housed in transparent cages (Makrolon cages type III, 38 × 23 × 15 cm³) containing wood shavings as bedding material (Allspan German Horse Super, Allspan German Horse Vertrieb GmbH & Co. KG, Wismar, Germany) and a paper tissue, a wooden stick (2 × 2 × 10 cm³; ZOONLAB GmbH, Castrop-Rauxel, Germany), a semi-transparent red plastic house and tunnel (Mouse House, Tecniplast Deutschland GmbH, Hohenpeißenberg, Germany; Mouse tunnel, ZOONLAB GmbH) as enrichment. At the age of 16 weeks (PND 112/113 (batch 2/batch 1), see section “Experimental design” for more details), animals were re-grouped into pairs and subjected to two different housing conditions according to the experimental design (“simple” and “complex” housing conditions, see section “Experimental design” for more details). These conditions looked as follows: ‘Simple’ housing conditions (Figure 1) were as initial housing but provided different enrichment items as nesting and gnawing material, which were a cotton nestlet (5 x 5 x 0.5 cm³, ZOONLAB GmbH) and a wooden square (3 x 3 x 3 cm³). As purely barren cages cause yet stronger welfare impairments, we believe that the animals’ welfare responses in housing conditions providing this basic enrichment do not interfere with our study question. ‘Complex’ housing conditions comprised ‘simple’ conditions as home cage, and additionally offered permanent access to a highly-structured ‘playground’ [Figure 1; 50 × 32 × 52 cm3, adapted from (Bračić et al., 2022)], that included additional levels, food sources, nesting and bedding resources and structural elements of different materials (see Supplementary Table S2). Thereby, the home cage was positioned within the playground, where the exact position varied between two batches (see section “Experimental design” and Supplementary Figure S1 for more details). An opening (9 x 4 cm2) was inserted in the lid of the home cage, through which mice could enter the playground. In order to prevent habituation and to continuously offer opportunities to explore, 6 different playgrounds (Figures 1A-F) that varied in exact design and combination of items, were constructed. Over the course of the experiment, complex housed mice experienced each playground, always one playground per week. Each pair had a different playground in the first week, while the sequence of presented playgrounds was the same for all pairs in complex housing. Simple and complex housing conditions differed in space availability, however mere additional space of enriched housing has little influence on welfare (Whittaker et al., 2012; Bailoo et al., 2018b).
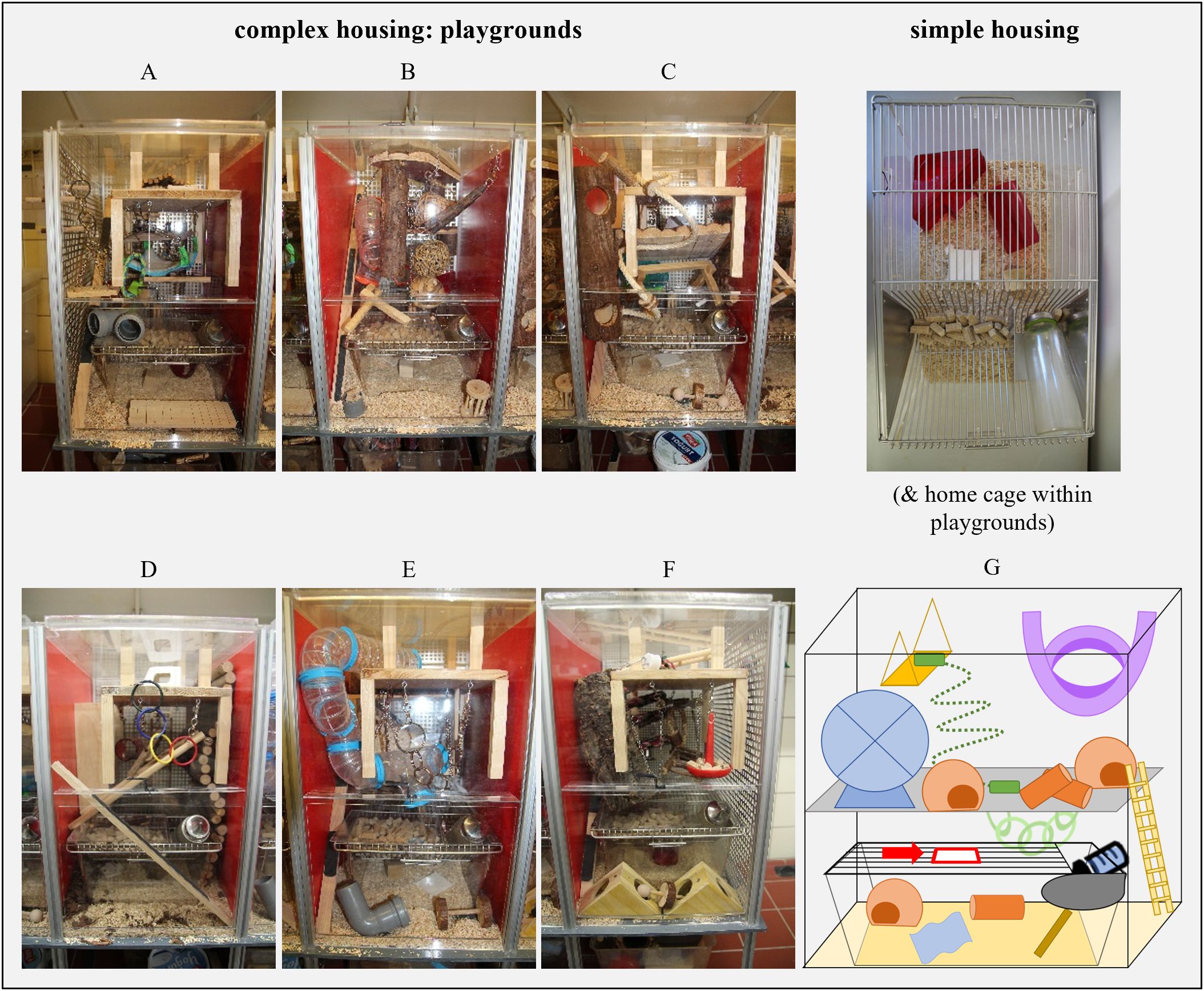
Figure 1. Housing conditions. (A–F) Six different playgrounds that were used in complex housing conditions. Each playground contained multiple enrichment items and one home cage. Identical home cages without access to a playground were used in simple housing conditions. (G) Schematic sketch of a playground arrangement, including the position of the home cage and the access from the home cage to the playground (red arrow).
Cages were changed weekly and enrichment items were changed biweekly. Playground enrichment was changed weekly. Changeover to the next playground was conducted 3 days after home cage changes, so that mice perceived the playground as an add-on opportunity to explore in addition to their home cages. Food (Altromin 1324, Altromin Spezialfutter GmbH & Co KG, Lage, Germany) and water was provided ad libitum. The housing room was maintained at a reversed 12h dark/light cycle (dark phase starting at 9 am), including a 15 minutes twilight phase, an average temperature of about 22°C and average humidity of about 58%.
2.2 Experimental design
The experiment took place in two batches each including 36 mice, i.e., all procedures were performed in the same way with a 17 weeks long time interval in between (for differences between the batches please see the specific sections of “Specific methods” and Supplementary Table S1).
The experiment consisted of two main phases (Figure 2): a ‘personality characterization’ phase, in which animals were characterized for their personality types by means of their exploration behavior and a ‘housing’ phase, in which animals were subjected to different housing environments and were assessed in terms of their individual welfare and characteristics of their immune system.
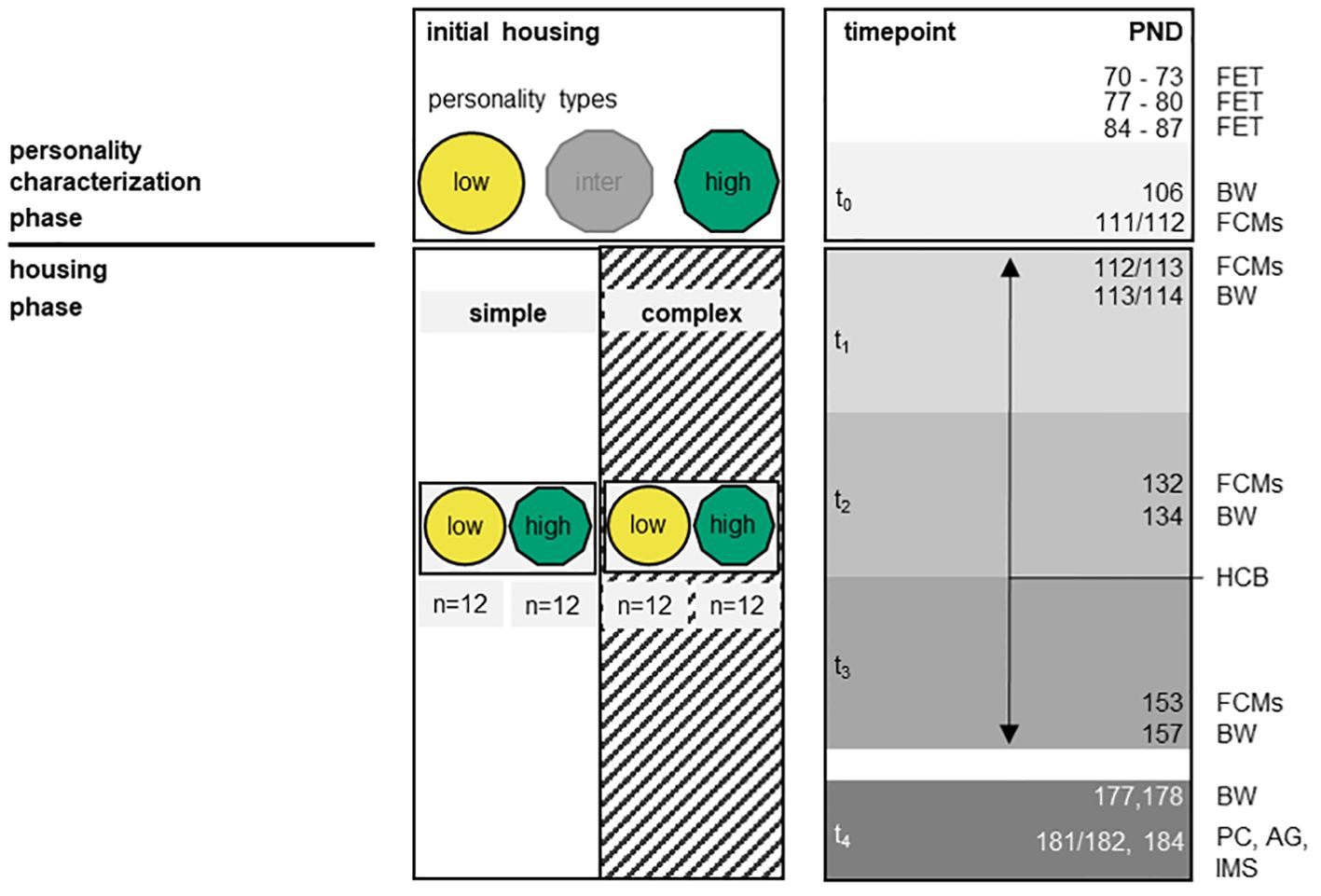
Figure 2. Experimental design. The left panel marks the two phases of the experiment, the personality characterization phase and the housing phase. The middle panel indicates the different exploration personality types and the different housing conditions that were applied in each phase. In the personality characterization phase, mice with unknown exploration personality were characterized into low, intermediate or highly explorative individuals (initial housing). In the housing phase, one low and one highly explorative mouse were then paired in a cage and subjected to either simple or complex housing. The sample size refers to the number of individuals per exploration personality type and housing condition. The right panel illustrates experimental timepoints (t0 – t4), the animals’ age in postnatal days (PND) and the performed tests: The free explorations test (FET) was used to characterize mice for their exploration personality type. At different timepoints, we measured body weight (BW), collected samples for the analysis of fecal corticosterone metabolites (FCMs), observed the home cage behavior (HCB), measured plasma corticosterone (PC) and adrenal gland weight (AG) and analyzed parameters of the immune system (IMS) to evaluate mice welfare and their immune system.
Briefly, upon arrival, all mice were habituated to the initial housing conditions for 6 weeks (PND 28 – 69). During this time, they were accustomed to tunnel handling, which was applied throughout the experiment (Hurst and West, 2010; Gouveia and Hurst, 2013, 2017).
The ‘personality characterization’ started at 10 weeks of age (PND 70) and aimed at identifying consistently distinct personality types in exploration behavior. Therefore, each mouse was repeatedly tested in the Free exploration test (FET) and subsequently assigned to one out of three possible personality types based on the average latency to explore (i.e., voluntarily enter) the FET-arena (see section “Free exploration test” for details): i) low explorers that showed longest latencies to explore the arena (i.e., lowest exploration behavior), ii) intermediate explorers with intermediate latencies and iii) high explorers that showed shortest latencies to explore the arena (i.e., strongest exploration behavior). To contrast the most extreme personality types, only high and low explorers (24 individuals each) participated in this experiment. Intermediate explorative mice were subjected to another study.
The ‘housing’ phase started when mice were 16 weeks old (PND 112/113 (batch 2/batch 1)). Mice were grouped in pairs of always one highly and one low explorative mouse and assigned to either simple or complex housing conditions (see section “Pairing of personality types and allocation to housing conditions” for details). All mice remained in these constellations until the end of the experiment. According to a 2 x 2 factorial design, this resulted in the following four groups, each comprising 12 animals: highly explorative mice in simple housing, highly explorative mice in complex housing, low explorative mice in simple housing and low explorative mice in complex housing (n = 12/group).
During this ‘housing’ phase, the welfare of the animals was monitored at different timepoints (‘t0’ - ‘t4’, please note that with respect to t0 the measurements represent baseline values and were thus taken shortly before animals were subjected to the different housing conditions, see section “Housing phase” for details) and characteristics of the immune system (IMS) were assessed at ‘t4’ to quantify potential personality-dependent reactions to the two different housing environments. Thereby, the welfare assessment was based on behavioral observations (HCB), body weight measurements (BW), the determination of fecal corticosterone metabolite (FCM) and plasma corticosterone (PC) concentrations, on the weight of adrenal glands (AG), and splenic immune cell composition (see the specific sections of “Housing phase”). In order to sample blood for PC measures, to dissect adrenal glands and to analyze splenocytes, animals were sacrificed at the end of the experiment.
Please note, that in between the welfare assessment of ‘t3’ and ‘t4’ (from PND 158 - 178) the mice’s exploration personality type was re-characterized in identical manner as before, i.e., 3 trials of FET as part of another study question (targeting cross-contextual stability of exploration personality). Those data are not included here. Mice were kept in their experimental pair- and housing-constellations during that time and all animals were treated in the same way.
2.3 Specific methods
2.3.1 Personality characterization phase
To identify distinct personality types in exploration behavior, each mouse was tested 3 x in the Free exploration test with 1 week pause in between. Cage enrichment was changed the week before each trial. The four individuals (a, b, c, d) that lived in the same cage were tested on 4 consecutive days, one mouse per day. Hence, mice were tested at PND 70 - 73 (trial 1), at PND 77 - 80 (trial 2) and at PND 84 - 87 (trial 3). All animals were tested between 9:30 am and 1:30 pm (beginning of the dark phase). The sequence in which animals were tested was randomized across cages and the same for the 4 consecutive test days of a trial (i.e., trial 1 day 1: cage 8 mouse a, cage 2 mouse a, …, cage 5 mouse a; trial 1 day 2: cage 8 mouse b, cage 2 mouse b, …, cage 5 mouse b). As time of day can influence behavior (Bodden et al., 2019), this testing sequence of mice was kept identical for the 3 trials. After behavioral testing, mice were kept in the same housing and group conditions as before for additional 3 weeks until they were re-grouped. During this time their FET behavior was analyzed and the pairing of the personality types and the allocation to the housing conditions was organized.
2.3.1.1 Free exploration test
The apparatus of the free exploration test was located in a separate testing room and was comprised of a white coated plywood arena (60 x 60 cm2) with 35 cm high walls. In one of the walls, an opening (15 x 11 cm2) was inserted. The light intensity at the center of the arena bottom was set to 35 (± 7) lux. Mice were individually transported to the testing room in their home cages, which were covered with a black blanket during transport. For the time of testing (approx. 20 minutes), cage mates that were not tested were transferred to a waiting cage, which was identical to the home cage. The home cage was equipped with a sliding door, that connected the home cage to the arena via a transparent square plexiglas tunnel (15 x 9 x 10 cm3). After 1 minute of acclimation time, the sliding door was opened and individuals could freely explore the arena for 15 minutes. The experimenter quietly left the room immediately after the test started. The animals’ behavior was recorded with a camera (The Imaging Source, DMK 22AUC03) and the video tracking software ANY-maze (version 7.14b, release: 2022, Stoelting Co., Wood Dale, IL, United States). Between tests the apparatus was cleaned with 70% ethanol. ‘Latency to explore the arena’, ‘time spent in the arena’, ‘number of entries’ and ‘distance travelled in the arena’ were recorded as proxy for exploration behavior.
2.3.1.2 Characterization of distinct personality types
Statistical repeatability analyses revealed that mice consistently differed in their exploration behavior in all four FET parameters, meaning that the subjects in fact exhibited distinct animal personality types in exploration behavior (see Supplementary Table S6). However, the FET parameter ‘number of entries’, ‘distance travelled in the arena’ ‘time spent in the arena’ might not only code for exploration, but could potentially be biased by the animals’ activity or anxiety-like behavior (Bourin and Hascoët, 2003; Almeida-Souza et al., 2015). Therefore, allocation to different exploration personality types was founded on ‘latency to explore the arena’. Based on their average latency across 3 trials, mice were assigned to three personality types via tertile split per batch (see Supplementary Tables S4, S5): shortest latencies equal high explorers, intermediate latencies equal intermediate explorers and longest latencies equal low explorers.
2.3.1.3 Pairing of personality types and allocation to housing conditions
As the social environment can influence animal behavior and animal welfare (Crawley et al., 1975; Pike et al., 2008; Chen et al., 2009; Guayasamin et al., 2017), we aimed at keeping the social environment as similar as possible for all mice. Therefore, each mouse was paired with a social partner that i) contrasted the mouse in its exploration personality and ii) was unfamiliar prior to the re-grouping (i.e., social partners had not lived within the same cage during previous phases). Consequently, mice were grouped in pairs of always one highly and one low explorative mouse. Even though high and low explorative mice – by definition - considerably differed in their exploration behavior, both personality types still included between-individual within-group (personality type) variation (i.e., individuals of the same personality type varied in their exact exploration value, see Supplementary Table S4). We aimed at balancing this variation among pairs and across housing conditions. When selecting partner animals for a pair, the most explorative mouse out of highly explorative animals was therefore paired with the most explorative mouse out of low explorative animals and so forth, so that the difference in exploration behavior between partners was as similar as possible for all pairs. Exceptions from this rule were made only, if mice-to-be-paired had previously lived within the same home cage. All 48 mice were given additional ear punches at this point to allow for individual identification in the new pairs.
Pairs were pseudo-randomly assigned to either simple or complex housing conditions, meaning that pairs that were on average more (or less) explorative compared to other pairs were balanced across housing conditions (see Supplementary Table S4).
2.3.2 Housing phase
Welfare consequences as a result of possible personality-dependent responses to the different housing conditions may arise as a function of time (Reimert et al., 2023). Therefore, the welfare of the animals was assessed at different timepoints during the experiment (Figure 2). More specifically, baseline values (BW, FCMs) were taken for both personality types shortly before animals were subjected to different housing regimes (‘t0’), i.e., after being habituated to the initial housing environment for about 12 weeks (PND 28 – 111/112 (batch 2/batch 1)). After re-grouping the animals, the early personality-dependent response (up to 2 weeks of housing) in behavior, body weight and stress hormone concentrations to the new housing conditions, incl. the new cage partners, was determined (‘t1’: HCB, BW, FCMs). Following these initial measurements, ‘t2’ and ‘t3’ measures in HCB, BW and FCMs were taken to depict the personality-dependent response after experiencing the housing conditions for either about 3 or about 6 weeks, respectively. Lastly, ‘t4’measures of BW, PC and AG were taken to assess the animals’ long-term response after living for up to 10 weeks in their respective housing conditions. At this timepoint, animals were sacrificed in order to sample blood for PC measures without causing pain and to extract adrenal glands and the spleen for an analysis of the animals’ immune system (IMS).
2.3.2.1 Home cage observations
To investigate the effects of personality-environment-interactions on spontaneous home cage behavior, live observations were performed in the housing room under red light conditions. Behavioral observations covered times from 9:30 am to 5:30 pm, i.e., the animals’ active phase (Bodden et al., 2019). Several welfare-related behaviors were recorded (Table 1): stereotypic (Mason, 1991), nesting (Gaskill et al., 2013), resting (Brown et al., 2017), self-grooming (Yalcin et al., 2007), social grooming, social sniff (File and Seth, 2003; Hisaoka-Nakashima et al., 2019) and agonistic behavior (Van Loo et al., 2002). Applying focal sampling and continuous recording (Bateson and Martin, 2021), individual mice were repeatedly observed for 5 - minute sessions at each timepoint (‘t1‘ : PND 112/113 – 122 (batch 2/batch 1); ‘t2‘ : PND 125 – 136; ‘t3‘ : PND 139 – 151/153 (batch 1/batch 2)). Spread over a period of 2 weeks per timepoint, individuals were observed on 8 (± 2) days, with 2 (± 1) sessions per day. This led to a total observation time of 60 – 85 minutes per mouse at each timepoint. Total observation times slightly differed between batches (see Supplementary Table S3). Behavioral durations and/or frequencies per minute were corrected within each session for the time the animal was not visible and were averaged across the sessions of a timepoint. The observer was blind to the personality type of the animals.
2.3.2.2 Body weight
To monitor effects of personality-environment interactions on the development of body weight, mice were weighed at 5 timepoints along the experiment: ‘t0’ (PND 106), ‘t1’ (PND 113/114 (batch 2/ batch 1)), ‘t2’ (PND 134), ‘t3’ (PND 157) and ‘t4’ (PND 177/178, each day half of the animals). Individual mice were transferred into a white plastic bowl (8 x 8 x 5 cm3) which was placed on a digital scale (capacity: 2100 g, resolution: 0.01 g).
2.3.2.3 Fecal corticosterone metabolites
To study the effects of personality-environment interactions on pituitary-adrenocortical activity, the animals FCMs were monitored non-invasively at 4 timepoints across the experiment: ‘t0’ was taken on PND 111/112 (batch 2/batch 1), which was the last day before animals were subjected to the different housing conditions. ‘t1’ was measured the very next day at PND 112/113 (batch 2/batch 1), which was the first day of the housing experience. This ‘t1’ FCM measurement, captures the immediate response after being transferred to the new housing, minus the first 30 minutes, which were considered to mainly mirror handling stress. ‘t2’ (PND 132) and ‘t3’ (PND 153) depict the stress response after 3 or 6 weeks of experiencing the housing condition, respectively.
2.3.2.3.1 Feces collection
During the dark phase, FCM concentrations represent responses to an event, which occurred around 4 – 6 h earlier (Touma et al., 2003). At all timepoints fecal samples were collected from 5 – 8 pm (± 30 minutes), capturing the animals’ corticosterone levels of around 11 am - 4 pm (± 30 minutes). To assure individual-level feces collection, females had to be separated from their social partners for the time of feces collection. As separation from social partners is suggested to be a major stressor for female mice (Martin and Brown, 2010), two mice out of one home cage were kept partly separated within one ‘sample cage’ for fecal sampling. Sample cages were comprised of a new Makrolon type III cage with a small amount of fresh bedding, which was divided into two halves with a transparent plexiglass, including a 19 x 9 cm2 metal mesh (0.8 cm mesh size) in the middle. This allowed visual, auditory, olfactory and slight body contact between the two cage partners. Each side contained a new semi-transparent red tunnel, a wooden stick and a paper towel as enrichment. Food and water were offered ad libitum.
Mice were transferred into sample cages in a randomized order and stored in a separate rack in the housing room. Exactly after 3 h, mice were transferred back to their home cages. All fecal boli were collected the next morning with gloves and stored in individually distinct 1,5 ml Eppendorf tubes (Eppendorf SE, Hamburg, Germany) at -20°C.
2.3.2.3.2 Analysis of fecal corticosterone metabolites
After wet weight was determined (scale: Kern 510-23, Kern & Sohn GmbH, Ballingen, Germany; weighing capacity: 300 g, resolution: 0.001 g) samples were dried in an oven (Model 500, Memmert GmbH + Co. KG, Schwabach, Germany) for 2 h at 80°C. Subsequently, samples were weighed again and stored in 2 ml safe-lock Eppendorf tubes. Feces were pulverized with a ball mill (Mixer mill MM 400, RETSCH GmbH, Haan, Germany) by using two stainless steel balls (diameter: 5 mm, RETSCH GmbH, Haan, Germany) for 2 minutes with 30 beads per seconds. If feces were not completely pulverized, pulverization was repeated for maximum one more time. Samples bigger than 300 mg were divided in half before pulverization and joint together afterwards. 50 mg of feces powder was diluted in 1 ml of 80% methanol, vortexed (Multi-vortex, V-32, Kisker Biotech GmbH & Co. KG, Steinfurt, Germany) for 30 minutes and centrifuged (Universal 320 R, Andreas Hettich GmbH & Co.KG, Tuttlingen, Germany) for 10 minutes at 2500 g speed. Subsequently, 500 µl of the supernatant was stored at -20°C in a 1.5 ml safe-lock Eppendorf tube. FCM concentrations were analyzed by using a 5α-pregnane-3β,11β,21-triol-20-one enzyme immunoassay previously validated for mice (Touma et al., 2003, 2004).
2.3.2.4 Plasma corticosterone concentrations
2.3.2.4.1 Blood sampling
To avoid pain inflicted by veins puncture, blood was collected from dead animals. Mice were sacrificed at PND 181/182 (batch 2/batch 1) or PND 184 (half the pairs at each day, balanced across housing conditions) in between 7:45 and 8:45 am (± 15 minutes). To reduce stress, the entire process until final death was performed together with the social partner. Pairs were transported from their home cages to a separate room in a red plastic transport box (22 x 22 x 16 cm3), that filtered out light at wave lengths visible for mice (Busch et al., 2014). Both mice were transferred into a narcotic chamber (23 x 13 x 11 cm3, covered with red plastic) that was flooded with 5% isoflurane in oxygen (flow rate 3.5 L/minute). When animals were anaesthetized to stage III, they were decapitated. Immediately after, blood was collected from the carotid artery into a 1 ml EDTA colleting tube (EDTA 1000 A PP, KABE-Labortechnik GmbH, Nümbrecht-Elsenroth, Germany). This process, from opening the home cage until the complete blood sample was collected took no longer than 3 minutes. Tubes were centrifuged at 2000 x g for 10 minutes at 4°C. Plasma was collected in a 1.5 ml Eppendorf tube and stored at –20°C.
2.3.2.4.2 Corticosterone analysis
Plasma corticosterone levels were assessed using a commercially available EIA kit (DRG Diagnostics) according to the manufacturer’s instructions. All standards and samples were analyzed in duplicates.
2.3.2.5 Adrenal glands
Left- and right-side adrenal glands were dissected, freed from fat and stored separately in 1.5 ml Eppendorf tubes. One h after dissection, they were weighed using a digital scale (scale: Mettler AE 200 S, Mettler Instrumente GmbH, Giessen, Germany; resolution: 0.0001 g).
2.3.2.6 Immune cells and cytokines of the innate and adaptive immune system
The spleen was dissected and stored in PBS (pH 7.4) on ice. After spleen collection was completed, splenocytes were mechanically dissociated by pressing them through a cell strainer (100 µm mesh size) into a petri dish and pipetting cells up and down. Cells were filtered again through a 100 µm strainer and washed in FACS buffer (PBS with 1% FBS and 1 mM EDTA).
To investigate cells of the myeloid lineage, in a 24-well plate 4 wells per animal containing 3 x 106 cells/300 µl each were cultured in DMEM containing 10% FBS, 1% Pen/Strep and 1% β-mercaptoethanol (Gibco) overnight. Two of the four wells were stimulated with 100 ng/ml lipopolysaccharide (LPS from E. coli serotype O127:B8, Sigma-Aldrich). The other two wells of each animal were not stimulated. Fluorescent staining was performed for one stimulated and one unstimulated sample of each animal with the following antibodies: (i) FITC-conjugated anti-mouse CD11b (clone M1/70), PerCP/Cy5.5-conjugated anti-mouse I-A/I-E (clone M5/114.15.2), PE/Cy7-conjugated anti-mouse CD11c (clone N418), PE-conjugated anti-mouse IL-12/IL-23p40 (clone C15.6) and PE/Dazzle594-conjugated anti-mouse TNF (clone MP6-XT22) and the other two sample with (ii) FITC anti CD11b, PerCP/Cy5.5-conjugated anti-mouse Ly6G (clone 1A8), PE/Cy7-conjugated anti-mouse Ly6C (clone HK1.4), PE-conjugated anti-mouse IL-6 (clone MP5-20F3) and PE/Dazzle594-conjugated anti-mouse IL-10 (clone JES5-16E3) (all from Biolegend).
For the investigation of T lymphocytes, 4 wells per animal containing 3 x 106 cells/300 µl each were cultured in IMDM containing 10% FBS, 1% Pen/Strep and 1% β-mercaptoethanol (Gibco) overnight. Two of the four samples were incubated in CD3/CD28 coated plates and stimulated with PMA (10 µg/ml) and ionomycin (500 ng/ml). The other two samples were incubated in uncoated plates where they remained unstimulated. Fluorescent staining was performed as above with the following antibodies: (iii) FITC-conjugated anti-mouse CD3e (clone 17A2), PerCP/Cy5.5-conjugated anti-mouse CD4 (clone RM4-5), PE/Cy7-conjugated anti-mouse CD25 (clone PC61), PE anti IL-6 and PE/Dazzle594 anti IL-10 or (iv) FITC anti CD3e, PerCP/Cy5.5 anti CD4, PE/Cy7-conjugated anti-mouse CD8a (clone 53-6.7), PE-conjugated anti-mouse IFN-g (clone XMG1.2) and PE/Dazzle594-conjugated anti-mouse IL-17A (clone TC11-18H10.1).
To prepare cells for intracellular stainings, all samples were treated with Brefeldin-A and Monensin (Biolegend) to avoid the release of cytokines for a maximum period of 12 h. Intracellular stainings were performed using the IC fixation and permeabilization buffers (eBioscience) according to the manufacturer’s instructions. Samples were acquired using an Attune NxT flow cytometer (ThermoFisher) with two lasers (488 and 568 nm). Fc files were exported and gated in FlowJo (BD, version 10.8.1). Gating comprised a live gate to exclude debris and dead cells as well as side scatter (SSC) area and height correlations to exclude doublets from single cells. The gating strategies used for analysis are shown in the respective Figures.
2.4 Statistical analysis
All statistical analyses were conducted using the software R (Version 4.2.2, 2022-10-31; R Core Team, 2021) and R Studio (Version 2022.12.0 + 353; Posit team, 2022). Graphical representation of data was created using the packages “ggplot2” and “ggpattern” (Wickham, 2016; FC and Davis, 2022).
To determine an appropriate sample size for the study, we performed an a priori sample size calculation with an estimated medium effect size (power = 80%, alpha-error = 5%, Cohen’s f ≈ 0.3), which yielded a total sample size of 72 individuals.
2.4.1 Personality characterization phase
To test for repeatability of exploration behavior in the FET, linear mixed-effect models, including ‘trial’ and ‘home cage ID’ as fixed effects and ‘mouse ID’ as random effect were fitted using the package “lme4” (Bates et al., 2015). Subsequently, we applied the package “rptR” (Stoffel et al., 2017) to calculate adjusted repeatabilities (R) and the statistical significance of repeatabilities being different from zero was tested by likelihood-ratio test and parametric bootstrapping (n = 1000, confidence level = 95%).
2.4.2 Housing phase
Behavioral, physiological and immunological data were analyzed using linear models, linear mixed-effects models or generalized linear mixed-effects models with binomial(“logit”) link function using the packages “lme4”, “lmerTest” and “car” (Bates et al., 2015; Kuznetsova et al., 2017; Fox and Weisberg, 2019). The package “emmeans” (Lenth, 2023) was applied posthoc for pair-wise comparisons (method to correct for multiple comparisons = “holm”).
When linear (mixed-effect) models were fitted (including models for the personality characterization, see above), model residuals were visually checked for constant error variance and normal distribution. If uncertain, we additionally consulted “check_heteroscedasity” and the Shapiro–Wilk test provided by the package “performance” (Shapiro and Wilk, 1965; Lüdecke et al., 2021). If residuals did not meet model assumptions, data were transformed so that residuals fitted model assumptions best. Residuals of binomial generalized linear mixed-effects models were checked using the package “DHARMa” (Hartig, 2022). Some parameters could not be transformed (adrenal gland weight and batch 2 number of entries to FET arena) or transformation did not yield normally distributed residuals (resting frequency, frequency of agonistic behavior). As simulation studies suggest linear-mixed models to be relatively robust against violations of distributional assumptions (Schielzeth et al., 2020; Knief and Forstmeier, 2021), we nevertheless report results of these models. Wherever multiple measurements per individual were included in the analysis, ‘mouse ID’ was fitted as random effect to allow for individual intercepts.
To test for physiological baseline differences between personality types at ‘t0’, fecal corticosterone metabolite (FCM) concentrations and body weights (BW) were analyzed with ‘personality’ (two level: high explorers, low explorers) as fixed effect:
Possible personality-dependent responses of FCM concentrations and BW to the different housing conditions over time were analyzed by including ‘personality’, ‘housing’ (two level: simple, complex) and timepoint (FCM: three level: t1, t2, t3; BW: four level: t1, t2, t3, t4) as fixed-effect interaction and baseline levels of ‘t0’ as additional fixed effect.
Plasma corticosterone levels and weight of adrenal glands (both collected at the final timepoint ‘t4’) were analyzed by including ‘personality’ and ‘housing’ as interaction term. Weight of adrenal glands represents the summed weight of left- and right-side adrenal gland corrected for ‘final’ (‘t4’) body weight.
We either analyzed duration and frequency of behaviors separately (resting, grooming) or we analyzed frequency only (social sniff, agonistic behavior). Some rarely occurring behaviors (stereotypic, nesting and social grooming behavior) were transformed into binomial responses: yes = animal showed behavior at that timepoint; no = animal did not show behavior at that timepoint). All behaviors were analyzed by including ‘personality’ and ‘housing’ as fixed-effect interaction and ‘timepoint’ (three level: t1, t2, t3) as additional fixed effect:
However, due to limited data quality stereotypic and nesting behavior could not be fitted including ‘timepoint’ and were therefore analyzed separate per timepoint:
Immune parameters were analyzed by including ‘personality’ and ‘housing’ as fixed effect interaction. For four variables, one outlier deviating more than two standard deviations from the mean was excluded from the analysis. As procession of spleen cells was conducted over multiple sessions, ‘session’ (four level: batch 1 day 1, batch 1 day 2, batch 2 day 1, batch 2 day 2) was included as random effect:
In the following, we report effects (based on common practice in the literature) to be significant when p < 0.05 or as “trends” when 0.05 < p < 0.1. However, we are aware of a rising critical debate about sharp thresholds of p values (Wasserstein and Lazar, 2016). We therefore decided to additionally report partial eta squared (η2p) or partial R2 as a measure of the magnitude of the reported effects (Lakens, 2013) using the packages “effectsize”, “partR2” and “rsq” (Ben-Shachar et al., 2020; Stoffel et al., 2021; Zhang, 2023).
2.5 Ethical note
All procedures complied with the regulations covering animal experimentation within Germany (Animal Welfare Act) and the EU (European Communities Council DIRECTIVE 2010/63/EU) and were approved by the local (Gesundheits-und Veterinäramt Münster, Nordrhein-Westfalen) and federal authorities (Landesamt für Natur, Umwelt und Verbraucherschutz Nordrhein-Westfalen “LANUV NRW,” reference number 81-02.04.2021.A127).
3 Results
The aim of this experiment was to investigate whether mice show personality-dependent welfare differences in response to the environment. Following this objective, we begin describing if and how behavior, physiology and immunology were influenced by the interplay of both, personality type and housing condition together. Additionally, we also investigated whether personality alone, irrespective of the housing conditions, caused welfare and immune system differences between highly explorative and low explorative individuals and whether and how mice that were housed under simple and complex conditions vary with respect to their welfare and their immune system.
3.1 Indications for personality-dependent responses to different housing environments in behavior, physiology and immunology
We first investigated whether mice of two distinct personality types differed in their reactions to different housing conditions. By means of behavioral home cage observations, we found a trend for an interaction effect between personality and housing with respect to stereotypic behavior. Shortly after the animals were subjected to the different housing environments this trend was not yet present (t1: df = 1, χ2 = 0.239, p = 0.625, R2 < -0.01), but became evident after mice had lived in their respective housing condition for a longer time (i.e., t2 and t3; Figure 3A). Here, the proportion of animals that showed stereotypic behavior tended to differ between the personality types, depending on the housing condition they lived in (as a trend: df = 1, χ2 = 2.866, p = 0.090, R2 = 0.03; please note that the proportion of animals and thus test statistics were identical for t2 and t3, however, identity of stereotypic animals was not entirely identical in t2 and t3). With 6 out of 12 highly explorative (50%) and 7 out of 12 low explorative individuals (58%) at both timepoints, a comparable number of mice of both personality types showed stereotypies in simple housing conditions. Contrastingly, in complex housing conditions, the personality types clearly differed in their reaction: not a single highly explorative mouse and 3 low explorative mice (25%) showed stereotypic behavior.
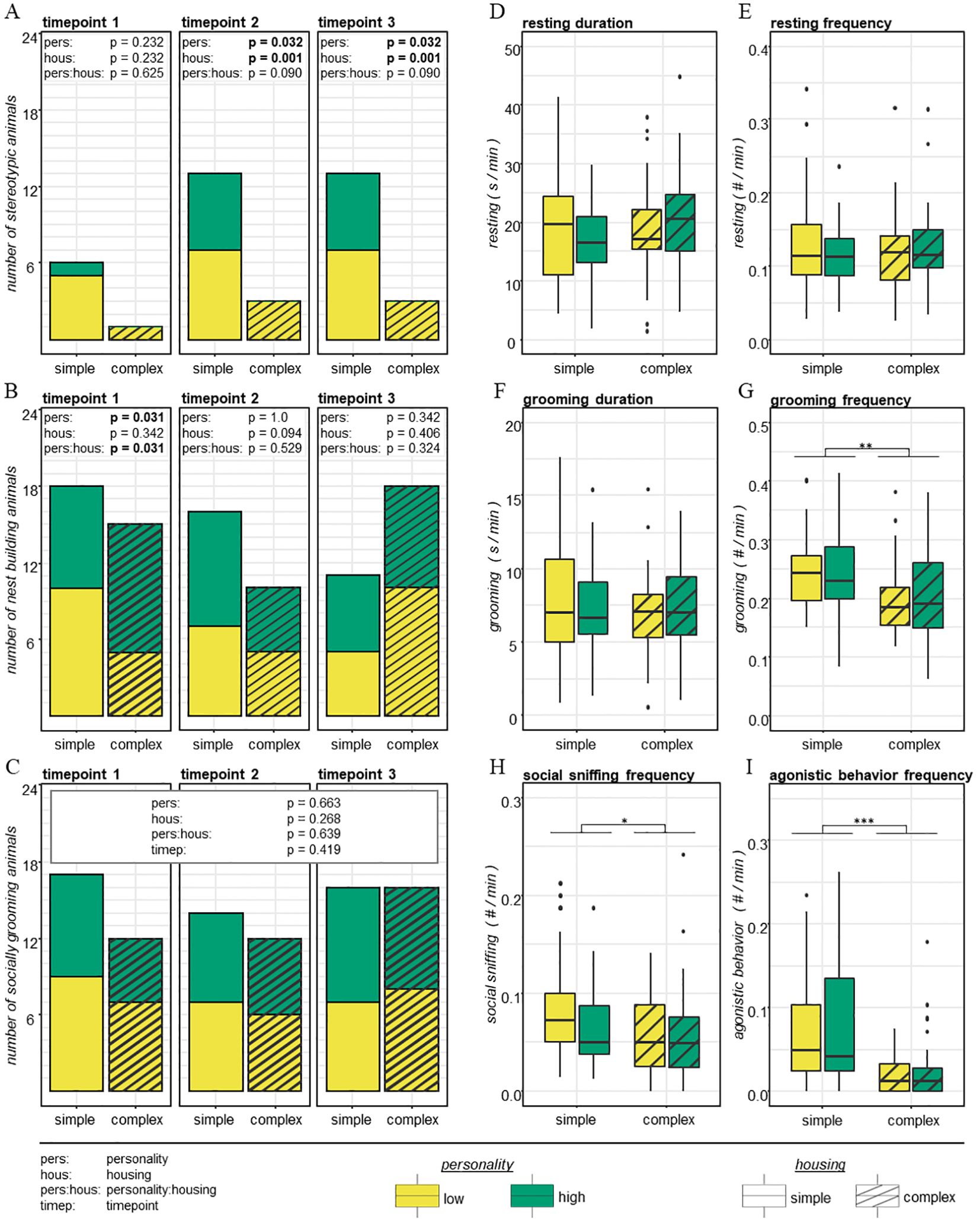
Figure 3. Behavioral welfare indicators. (A–C) Number of low and high explorers in simple and complex housing conditions at timepoints 1, 2 and 3 that showed stereotypic behavior (A), nest building behavior (B) and social grooming behavior (statistical analysis of social grooming was not separated per timepoint, C). (D–I) Mean values of resting duration (D), resting frequency (E), grooming duration (F), grooming frequency (G), social sniffing frequency (H), agonistic behavior frequency (G), that low and high explorers in simple and complex housing showed averaged across timepoints 1, 2 and 3. The upper and lower whiskers extend to the largest value no further than 1.5 x IQR (inter-quartile range). Data beyond are plotted individually (D–I). Sample size = 12 per group (low explorers in simple housing, high explorers in simple housing, low explorers in complex housing, high explorers in complex housing). Main results from statistical analysis are indicated above each plot either as text (A-C) or indicated by *** = p < 0.001, ** = p < 0.01, * = p < 0.05 (D–I).
We also found a significant interaction effect between personality and housing on nesting behavior. However, the proportion of animals showing nesting behavior was exclusively affected at t1 (df = 1, χ2 = 4.650, p = 0.031, R2 = 0.10; Figure 3B), but not at later timepoints (t2: df = 1, χ2 = 0.397, p = 0.529, R2 < 0.01; t3: df = 1, χ2 = 0.975, p = 0.324, R2 =0.02). Whereas in simple housing conditions, 10 low explorers and 8 high explorers were observed to show nesting behavior at t1, in complex housing conditions 5 low explorers and 10 high explorers were noted to show nesting behavior. Besides this, we could not find any further significant personality-by-housing interaction effects on other solitary (resting, grooming) or social (social grooming, social sniff, agonistic behavior) behaviors (Figures 3C–I, see Supplementary Table S7).
In addition to behavior, we investigated whether mice of the two personality types differed in their physiological responses to the different housing environments. Regarding corticosterone levels, there was no evidence that personality and housing together shaped fecal corticosterone metabolite concentrations at any timepoint (F1,43 = 1.322, p = 0.257, η2 = 0.03; Figure 4A). However, we found a trend for an interaction effect between personality and housing on plasma corticosterone concentrations (F1,44 = 2.876, p = 0.097, η2 = 0.06; Figure 4C). At t4, the two personality types showed a trend towards housing-dependent expression patterns of plasma corticosterone: Whereas in simple housing conditions, highly explorative mice showed higher levels than low explorative mice, in complex housing conditions we observed the opposite: here, low explorative individuals expressed higher levels than highly explorative individuals. Descriptively, the difference in plasma corticosterone levels between low explorers that had lived in simple and low explorers that had lived in complex housing conditions was marginal. Yet, high explorers that had lived in simple housing conditions expressed considerably higher plasma corticosterone levels compared to high explorers that had lived in complex housing conditions. Lastly, variation in body weight and the weight of adrenal glands was not affected by an interaction effect between personality and housing (Figures 4B, D, see Supplementary Table S9).
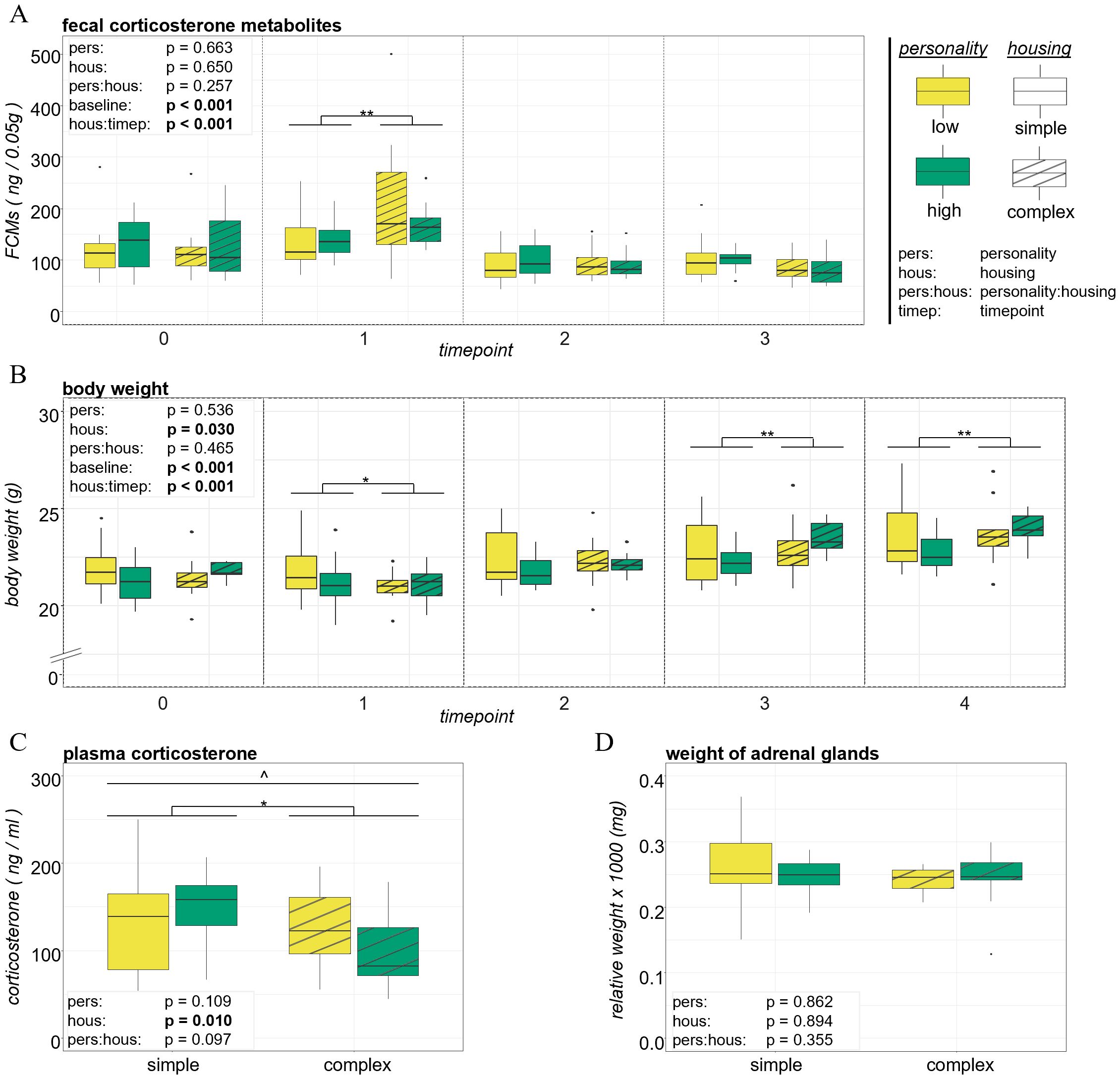
Figure 4. Physiological welfare indicators. Mean values of low and high explorers in simple and complex housing of fecal corticosterone metabolite concentrations at timepoints 0, 1, 2 and 3 (A), of body weight at timepoints 0, 1, 2, 3 and 4 (B), of plasma corticosterone concentrations (C) and the relative weight of adrenal glands (D) at timepoint 4. The upper and lower whiskers extend to the largest value no further than 1.5 x IQR (inter-quartile range). Data beyond are plotted individually. Sample size = 12 per group (low explorer in simple housing, high explorer in simple housing, low explorer in complex housing, high explorer in complex housing), except N = 11 for fecal corticosterone metabolite concentrations at timepoint 1 for high explorers in complex housing. Main results from statistical analysis are indicated above each plot as text and by ** = p < 0.01, * = p < 0.05, ^ = 0.05 < p < 0.1.
Regarding characteristics of the immune system, we found that the total number of spleen cells did not differ between the four groups of mice (low explorers in simple or complex and high explorers in simple or complex housing conditions (see Supplementary Table S11)). Therefore, all results will be presented as percentages of all splenocytes or specific cell population as indicated. The flow cytometric analysis of innate and adaptive immune cells revealed an interaction effect regarding IL-6 producing CD11b+ myeloid cells after ex vivo LPS stimulation (F1,41 = 6.915, p = 0.012, η2 = 0.14; Figure 5D). In simple housing conditions, low explorative mice showed increased percentages of IL-6 producing CD11b+ myeloid cells compared to highly explorative mice. In complex housing conditions, highly explorative mice presented higher percentages of these cells. A similar effect was indicated as a trend in non-stimulated IL-6 producing myeloid cells (F1,41 = 3.214, p = 0.080, η2 = 0.07) and in IL-6 producing CD4+ T cells (F1,41 = 2.982, p = 0.092, η2 = 0.07 and see Supplementary Table S11). Statistical trends for personality-by-housing interactions were also observed for IL-12 producing myeloid cells after LPS stimulation (F1,41 = 2.867, p = 0.099, η2 = 0.07, Figure 5F) and unstimulated TNF producing myeloid cells (F1,41 = 3.030, p = 0.089, η2 = 0.07). There were no further interaction effects regarding any other immune cell type investigated, i.e., myeloid cells, CD11c+ MHC-II+ dendritic cells, CD4 and CD8 T cells and respective cytokine producing subsets of these cell types (see Supplementary Table S11 or Figure 5E for IL-10 producing myeloid cells after LPS stimulation).
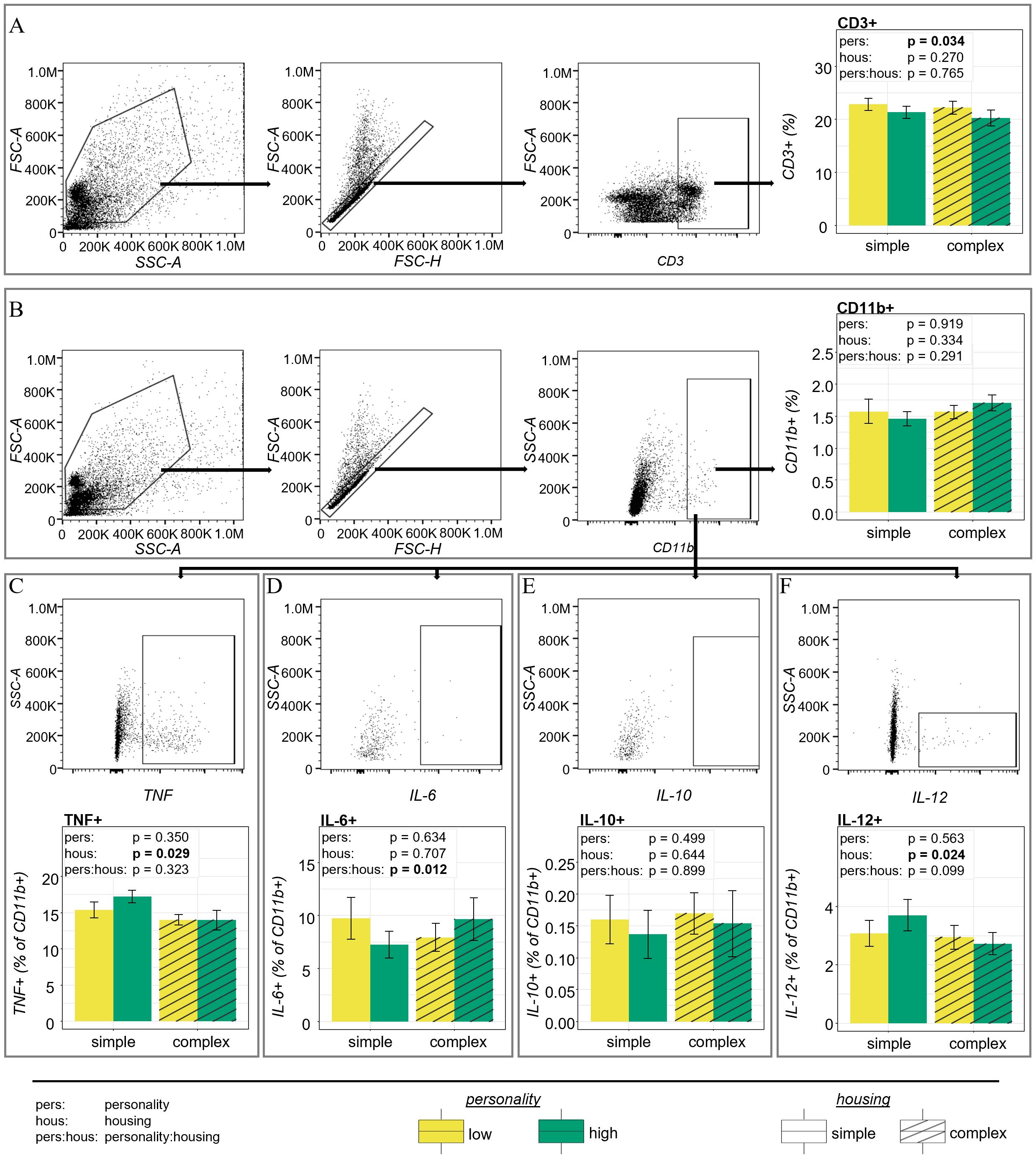
Figure 5. Immune parameters. (A) Gating strategy for T cells included gating life cells by SSC-A vs. FSC-A and single cells by FSC-H vs. FSC-A, followed by SSC-H vs. SSC-A (not shown) and expression of CD3. Low explorative mice showed increased percentages of T cells compared to high explorative mice. (B) Gating strategy for myeloid cells included gating life cells by SSC-A vs. FSC-A and single cells by FSC-H vs. FSC-A, followed by SSC-H vs. SSC-A (not shown) and expression of CD11b. Percentages of CD11b+ myeloid cells revealed no differences between personality types or housing environments. (C-F) Gating of cytokine producing myeloid cells (as percentage of CD11b+ cells). (C) Mice from simple housing conditions showed elevated percentages of TNF producing CD11b+ myeloid cells. (D) Percentages of IL-6 producing myeloid cells were dependent on an interaction of personality type and housing environment. Low explorative mice showed more IL-6 producing CD11b+ cells than high explorative mice in simple housing conditions while it was the other way around in complex housing conditions. (E) Percentages of IL-10 producing myeloid cells did not differ. (F) Mice from simple housing conditions showed elevated percentages of IL-12 producing CD11b+ myeloid cells. Depicted data are based on cells after PMA/Iono stimulation (A) or LPS stimulation (B–F). Main results from statistical analysis are indicated above each plot as text.
3.2 Exploration personality influences behavior and the immune system, but not physiology
In a next step, we investigated whether highly and low explorative mice differed in their welfare and characteristics of their immune system, irrespective of the housing environments they lived in.
Home cage observations revealed that the two personality types significantly differed with respect to stereotypic and nesting behavior. The proportion of individuals that showed stereotypic behavior was not yet affected by personality at t1 (df = 1, χ2 = 1.430, p = 0.232, R2 < 0.01). However, at later timepoints (i.e., at t2 and t3), the proportion of stereotypic and non-stereotypic animals significantly differed between the two personality types (df = 1, χ2 = 4.589, p = 0.032, R2 < 0.01; Figure 3A). The likelihood to develop stereotypic behavior was significantly higher among low explorative mice: 10 out of 24 low explorers (42%), but only 6 out 24 high explorers (25%) showed stereotypic behavior. Personality differences in nesting behavior were only present shortly after the introduction to the housing conditions, with significantly more high explorers showing nesting behavior (t1: df = 1, χ2 = 4.641, p = 0.031, R2 < 0.01; Figure 3B). At later timepoints, there were no personality differences in nesting behavior anymore (t2: df = 1, χ2 = 0.000, p = 1.000, R2 < 0.01; t3: df = 1, χ2 = 0.902, p = 0.342, R2 < 0.01). Similarly, high and low explorers did not show differences in the other solitary (resting, grooming) or social (social grooming, social sniff, agonistic) behaviors, that were assessed (Figures 3C–I, see Supplementary Table S7).
To account for any pre-existing (i.e., prior to the introduction of housing conditions) personality differences in physiological parameters, baseline FCMs and baseline body weight were assessed shortly before high and low explorers were subjected to the two different housing environments at t0. Neither FCMs nor body weight were found to differ between highly and low explorative individuals (FCM: df = 1, F = 0.645, p = 0.426, η2 = 0.01; BW: df = 1, F = 0.121, p = 0.729, η2 < 0.01). At later timepoints, we could not detect an influence of personality type alone on FCMs, plasma corticosterone, body weight or the weight of adrenal glands (Figure 4, see Supplementary Table S9).
We further aimed to elucidate whether highly and low explorative personalities types were associated with distinct immune phenotypes. Regarding myeloid and dendritic cells, no personality effects were observed (see Supplementary Table S11). Percentages of CD3+ T cells among isolated splenocytes, however, were increased in low explorative mice compared to highly explorative mice, both, after PMA/Iono stimulation (F1,41 = 4.796, p = 0.034, η2 = 0.10; Figure 5A) and without stimulation (F1,41 = 4.922, p = 0.032, η2 = 0.11). Neither the proportions of CD4 or CD8 T cells among all T cells nor those of cytokine producing subtypes differed between personality types except a trend for increased percentages of unstimulated TNF producing dendritic cells among the CD11c+ MHC-II+ dendritic cell population in highly explorative mice (F1,41 = 3.465, p = 0.070, η2 = 0.08 and see Supplementary Table S11).
3.3 Housing environments influence behavior, physiology and the immune system
Lastly, we focused on personality-independent effects of housing and investigated whether mice that were housed in simple and complex housing conditions differed in behavior, physiology and characteristics of their immune system.
Several behavioral parameters were found to be influenced by the housing conditions, irrespective of the personality type. Stereotypic behavior was already observed shortly after the animals were subjected to their respective housing environments. But while there was no statistically significant effect of housing on stereotypic behavior at t1 (df = 1, χ2 = 1.430, p = 0.232, R2 < 0.01), we did find that the proportion of animals that developed stereotypic behavior was significantly higher in simple than in complex housing conditions at t2 and t3 (df = 1, χ2 = 10.357, p = 0.001, R2 < 0.01; Figure 3A). Whereas in simple conditions 13 out of 24 animals (54%) developed stereotypies, in complex housing conditions only 3 out of 24 animals (13%) showed stereotypic behavior. Additionally, there was a trend for an influence of housing on nesting behavior at t2, with more simple housed mice that were observed to show nesting behavior than complex housed mice (df = 1, χ2 = 2.805, p = 0.094, R2 < 0.01; Figure 3B). At t1 and t3, nesting behavior was not affected by housing (t1: df = 1, χ2 = 0.902, p = 0.342, R2 < 0.01; t3: df = 1, χ2 = 0.689, p = 0.406, R2 < 0.01). While there was also no housing effect on resting behavior, social grooming and solitary grooming duration (Figures 3C-F, see Supplementary Table S7), we found a significant housing effect on the frequency of solitary grooming (F1,138 = 10.827, p = 0.001, η2 = 0.07; Figure 3G). Even though, mice in simple and complex housing conditions displayed similar average grooming durations, simple housed mice started grooming bouts significantly more often. Furthermore, we found that simple housed mice showed social sniffing (F1,44 = 5.902, p = 0.019, η2 = 0.12; Figure 3H) and agonistic behavior (F1,44 = 29.793, p < 0.001, η2 = 0.4; Figure 3I) significantly more often than complex housed mice. Although not the main question of the study, pair-wise comparisons (see Supplementary Table S8) revealed that agonistic behavior across both housing conditions significantly increased from t1 to t2 (df = 94, t.ratio = -2.588, p = 0.022), and remained at a similar frequency thereafter (t2 to t3: df = 94, t.ratio = -0.333, p = 0.740).
Of the assessed physiological parameters, personality-independent differences between simple and complex housing conditions were observed in fecal corticosterone metabolite and plasma corticosterone concentrations, as well as in body weight, but not in weight of adrenal glands.
We found a significant interaction effect of housing and timepoint on fecal corticosterone metabolite concentrations (F2,87 = 8.867, p < 0.001, η2 = 0.17; Figure 4A). Pair-wise comparisons (see Supplementary Table S10) revealed that complex housed mice showed significantly higher FCM levels compared to simple housed mice immediately after being subjected to the respective housing condition (t1: df = 121, t.ratio = 3.171, p = 0.002, padjusted = 0.010). In contrast, at t2, no housing differences could be detected (df = 120, t.ratio = -0.144, p = 0.885, padjusted = 0.885). At the last timepoint t3, the direction of housing differences switched and simple housed mice did show higher FCMs levels than complex housed mice. However, this result was non-significant after correction for multiple testing (df = 120, t.ratio = -2.128, p = 0.035, padjusted = 0.141). FCMs in both housing conditions significantly decreased from the first reaction to the new housing situation until at t2 (t1 to t2; simple: df = 87, t.ratio = 4.636, padjusted < 0.001; complex: df = 88, t.ratio = 8.252, padjusted < 0.001), but did not change thereafter (t2 to t3; simple: df = 87, t.ratio = -0.890, padjusted = 0.752; complex: df = 87, t.ratio = 1.317, padjusted = 0.573). Overall, our analyses revealed a significant effect of baseline FCM levels (that were measured the last day before animals entered the new housing conditions at t0) on FCM levels at later timepoints (t1-3: F1,44 = 27.535, p < 0.001, η2 = 0.38), suggesting a relative individual stability of FCM levels. Thus, animals with initially (i.e., at t0, before they entered the new housing conditions) higher FCM levels compared to conspecifics, expressed higher levels at later timepoints (t1, t2, t3). At t4, corticosterone concentrations measured in blood plasma corroborate the housing-induced differences in FCMs: simple housed mice expressed significantly higher plasma corticosterone levels compared to complex housed mice (F1,44 = 7.204, p = 0.010, η2 = 0.14; Figure 4C).
Similar to FCMs, body weight was significantly affected by a housing-by-timepoint interaction (F3,132 = 12.037, p < 0.001, η2 = 0.21; Figure 4B). Again, pair-wise comparisons (see Supplementary Table S10) revealed housing differences at t1, shortly after animals were subjected to the new housing situation, with simple housed mice being significantly heavier than complex housed mice (df = 136.6, t.ratio = -2.297, padjusted = 0.046). While no housing differences could be detected at t2 (df = 136.6, t.ratio = 1.046, padjusted = 0.297), complex housed mice were significantly heavier than simple housed mice at t3 (df = 136.6, t.ratio = 3.854, padjusted = 0.001) and t4 (df = 136.6, t.ratio = 3.654, padjusted = 0.002). Moreover, the animals’ body weight significantly increased throughout the experiment in both housing conditions (see Supplementary Table S10). And additionally, body weight was significantly affected by the baseline body weight that was measured at t0 (t1-4: F1,43 = 221.765, p < 0.001, η2 = 0.84), meaning that mice that were heavier (compared to conspecifics) before being subjected to the respective housing conditions, were heavier at later timepoints. The weight of adrenal glands was not affected by the housing conditions applied in this study (F1,44 = 0.018, p = 0.894, η2 < 0.01; Figure 4D).
The housing environment did not affect percentages of CD11b+ myeloid cells (F1,41 = 0.955, p = 0.334, η2 = 0.02 Figure 5B). However, after LPS stimulation percentages of IL-12 producing cells among CD11b+ cells were higher in mice from simple housing compared to mice from complex housing (F1,41 = 5.516, p = 0.024, η2 = 0.13; Figure 5F). A similar effect was observed for TNF producing cells that showed higher percentages in mice from simple housing compared to those from complex housing (F1,41 = 5.137, p = 0.029, η2 = 0.12; Figure 5C). In contrast, mice from complex housing presented higher percentages of unstimulated Ly6Ghi Ly6Cint neutrophilic granulocytes (F1,41 = 6.356, p = 0.016, η2 = 0.13; see Supplementary Table S11) while there was a similar statistical trend for stimulated neutrophils (F1,41 = 3.622, p = 0.064, η2 = 0.08). In addition, Ly6Chi Ly6Glo inflammatory monocytes after LPS stimulation (F1,41 = 3.137, p = 0.084, η2 = 0.07) as well as the CD8+ subset of T cells after PMA/Iono stimulation (F1,41 = 3.482, p = 0.069, η2 = 0.08) tended to be increased in mice from simple housing conditions (see Supplementary Table S11). There were no further differences between mice from the two housing conditions regarding any other immune cell type investigated, i.e., CD11c+ MHC-II+ dendritic cells, CD4 and CD8 T cells and other cytokine producing subsets of the cell types investigated (see Supplementary Table S11).
So far unreported results (such as main effects of timepoint on behavior and physiology or additional immune parameters) are summarized in the Supplementary Material (see Supplementary Tables S7-S11).
4 Discussion
The aim of this study was to investigate possible personality-dependent consequences in mouse welfare and the immune system in response to different housing environments. Therefore, we analyzed several behavioral, physiological and immunological parameters of highly and low explorative mice that had lived in either simple or complex housing conditions for a total time of 10 weeks. We indeed found personality-dependent reactions of highly explorative and low explorative mice to the two environments. We further found housing-independent personality differences and general housing differences in the assessed welfare measures.
4.1 The combination matters - personality-dependent consequences of different housing conditions on the animals’ welfare
Interestingly, we indeed observed interactions between the animals’ personality and the housing conditions they lived in that critically affected the individuals’ welfare in the respective housing environment. Differences occurred in behavioral, physiological and immunological markers, but the pattern of the observed effects was highly dependent on the specific outcome measure. Briefly, in simple housing conditions, a similar number of mice of both personality types showed stereotypic behavior, but highly explorative mice showed higher levels of plasma corticosterone and lower numbers of IL-6 producing myeloid cells compared with low explorative mice. In complex housing conditions, low explorative mice showed stereotypic behavior, while highly explorative mice did not. Moreover, we here found an opposite pattern, with highly explorative mice showing lower levels of plasma corticosterone and higher numbers of IL-6 producing myeloid cells compared with low explorative mice. Also nesting behavior was influenced. However, as alterations in nesting behavior occur only when welfare is severely impaired (Gaskill et al., 2013) and the effect found here was only visible at t1 (i.e., within the first 2 weeks of housing), it is unlikely that our results on nesting behavior allow any conclusions about the animals’ welfare. Other behavioral, physiological or immunological parameters were not affected by the interplay of personality type and housing condition.
4.1.1 Welfare-promoting effects of complex conditions are more powerful for high explorers than for low explorers
With respect to stereotypic behavior, personality seems to play a negligible role in simple housing conditions. Contrastingly, in complex housing conditions, personality critically influenced whether or not animals developed stereotypic behavior. Stereotypic behavior is among the most powerful behavioral indicators for impaired welfare (Mason and Rushen, 2006; Campos-Luna et al., 2019). Enriched environments have been repeatedly reported to increase welfare and consequently prevent the development of stereotypic behavior (Gross et al., 2011; Jones et al., 2011). With 25% of low explorers vs. 0% of high explorers that showed stereotypic behavior in complex housing conditions, our results suggest that the general positive influence of enriched environments was much more effective in highly explorative mice than it was in low explorative mice.
Why did low explorers still develop stereotypic behavior in the improved complex housing conditions? Coping style theory – a concept that is related to animal personality (Carere et al., 2010; Finkemeier et al., 2018) might provide a possible explanation. It describes consistent sets of behavioral and physiological responses to stress or challenging situations (Natarajan et al., 2009; de Boer et al., 2017). Based on coping style-related variation in the sensitivity of the dopaminergic system, which is involved in the initiation of stereotypic behavior (Koolhaas et al., 1999; Ijichi et al., 2013), consistent individual differences in the predisposition for stereotypic behavior were hypothesized (Ijichi et al., 2013). Such a personality-dependent predisposition in low explorative mice may explain the here observed variation in stereotypic behavior in complex housing conditions.
An alternative explanation may be individual differences in habituation patterns and sensitivity towards novel environments, which is often accompanied by variation in corticosterone levels, a good indicator for HPA-activity (Koolhaas et al., 1999; van der Goot et al., 2020). Complex housing conditions included constant changes of the environment, to which low explorers – in contrast to high explorers - may not have been able to habituate. This is also mirrored by higher plasma corticosterone concentrations of low compared with high explorers, which indicate increased stress levels in low explorative mice in complex housing conditions (Sapolsky et al., 2000; Hau et al., 2016). Besides stress, physical activity can also lead to elevated corticosterone concentrations (Coleman et al., 1998; Girard and Garland, 2002). However, activity levels expectedly should be highest in highly explorative individuals that explore the bigger complex environments. As we found the opposite, stress associated with the different housing conditions is more likely to explain the observed differences in corticosterone levels.
4.1.2 High explorers are more responsive to the housing conditions than low explorers
Contrasting the animals’ response in complex conditions, highly explorative individuals displayed higher plasma corticosterone levels than low explorative individuals, when animals lived in simple conditions. Hence, in line with previous studies, the general negative effect of poor housing conditions translated into increased corticosterone levels (Sztainberg et al., 2010), but only in highly explorative individuals. Descriptively our results suggest that merely highly explorative mice showed housing-dependent differences in plasma corticosterone concentrations. By comparison, low explorative mice barely differed between the simple and complex housing conditions, which reflects our results on stereotypic behavior. Similarly, a study by Kazlauckas and colleagues (Kazlauckas et al., 2011) showed that highly explorative, but not low explorative mice reacted with increased corticosterone concentrations to a stress paradigm, indicating a higher stress sensitivity in high explorers. In line with our initial hypothesis, one may thus conclude that highly explorative individuals experienced simple housing conditions more adversely compared with individuals that are generally less explorative.
4.1.3 The immune response of high and low explorers in different housing conditions
Regarding characteristics of the immune systems, interleukin-6 (IL-6)-producing myeloid cells was the only immune parameter that revealed a significant personality-by-housing interaction effect. The cytokine IL-6 is well known for its high responsiveness to different kinds of acute and chronic stressors (Rohleder et al., 2012). This interaction may thus reflect an effect similar to the corticosterone effect discussed above. Usually, there is a positive association between stress and IL-6 serum levels (Niraula et al., 2019). In the present study, however, IL-6 producing myeloid cells were reduced in highly explorative mice compared to low explorative mice in simple housing. In complex housing conditions, they were increased in highly explorative mice, opposite to corticosterone levels. A potential reason for this finding might be that corticosterone leads to a mobilization of IL-6 producing myeloid cells from the spleen into the blood as it has been shown in mice exposed to repeated social defeat (Wohleb et al., 2014; Niraula et al., 2018). In total, only minor differences in immune cell composition were found. This is in line with only minor differences in activation of the HPA axis, which may likely be a mediator of changes in immune cell composition (Niraula et al., 2018).
4.1.4 Is it a match? Compatibility between personality type and housing condition
Overall, highly explorative mice seemed to benefit more from complex housing conditions and seemed to be more adversely affected by simple housing conditions than low explorative mice. A possible explanation may be the lack of exploration opportunities in simple housing conditions (Würbel, 2001). The possibility to express the full behavioral repertoire is a prerequisite for good welfare. Whether or not animals can do so (e.g., explore their environment) is dependent on the environmental conditions they live in (Baumans, 2005). In the present study, complex conditions provided and simple conditions limited exploration opportunities. However, similar to the match-mismatch hypothesis from psychological theory (Schmidt, 2011; Hoogland and Ploeger, 2022) individual welfare can be affected when environmental conditions do not match the individuals’ behavioral and neuroendocrine phenotype (Sachser et al., 2011; Schmidt, 2011; Sachser et al., 2013). Thus, complex housing conditions may match and simple housing conditions may mismatch the specific needs of highly explorative individuals. On the other hand, individuals that are generally less explorative are potentially also less affected by the availability of exploration opportunities. Likewise, the match-mismatch hypothesis may also explain why low explorers developed stereotypic behavior in the improved complex housing conditions: the housing conditions the animals habituated to were simple and constant (initial housing conditions), while the housing conditions the animals faced later in live were complex and constantly changing (complex housing conditions). Low explorative individuals may cope worse with this mismatch than individuals with highly explorative tendencies, that hence, quickly familiarize with the novel environment.
4.2 Effects of personality differences on animal welfare and the immune system
In line with previous studies, we could identify consistent individual differences in exploration behavior in mice (Freund et al., 2013; Krebs et al., 2019; Verjat et al., 2020) and found that these differences in exploration personality affected the animals’ behavior and their immune system, but not the assessed physiological parameters. Specifically, as already discussed above, more low explorers than high explorers displayed stereotypic behavior and fewer low explorers than high explorers showed nesting behavior. Moreover, low explorative mice expressed higher percentages of T cells compared with highly explorative mice.
4.2.1 High and low explorers do not show housing-independent welfare differences in behavioral and physiological indicators
Personality-dependent differences in nesting behavior were, again, only found shortly after animals encountered the new housing conditions, that included unfamiliar nesting materials and may therefore rather represent explorative tendencies in interacting with novel items than differences in the animals’ welfare (Verjat et al., 2020).
Moreover, we did not find differences between low and highly explorative mice in any physiological parameter. With respect to the weight of adrenal glands, this is in line with a previous study (Kazlauckas et al., 2011). However, contrary to our study, Kazlauckas and colleagues (Kazlauckas et al., 2011) showed that low explorative mice expressed higher levels of plasma corticosterone. The inconsistent results between the studies may be explained by a different choice of exploration parameters that do not necessarily measure the same aspect of an animals’ exploration personality (Krebs et al., 2019). Whereas we measured exploration personality by the ‘latency of voluntary entries to an unfamiliar arena’, Kazlauckas and colleagues (Kazlauckas et al., 2011) focused on the ‘time spent close to a novel object in an open field’ in a forced situation. The latter is likely to capture also anxiety-like tendencies in mice (Crawley, 1985; Griebel et al., 1993; Heredia et al., 2014).
Based on behavioral and physiological indicators, we could not detect welfare differences between high and low explorers that were independent of the housing condition. This stands in contrast to our expectations, as previous studies have reported links between personality- or behavioral differences and welfare-related variation in behavior and physiology. Highly explorative mice were e.g., shown to be less anxious (Kazlauckas et al., 2005), but more pessimistic (Verjat et al., 2020) than low explorative mice. Likewise, a meta-study revealed weak, but significant associations between personality and intrinsic state (e.g., hormones or metabolism) (Niemelä and Dingemanse, 2018). Moreover, existing individual differences in mice’s vulnerability to stress (Ambrée et al., 2018) were associated with different behavioral patterns (Krishnan et al., 2007), and different coping strategies have been linked with predispositions for specific diseases (e.g., cardiovascular diseases) (Koolhaas et al., 1999). However, as the specific pattern seems to be highly dependent on the personality trait and the parameter under investigation, this may explain why we did not find clear personality-associated differences in animal welfare in our study.
4.2.2 Personality-associated differences in the immune system might be adaptive
Covariations between personality, behavior, physiology and immunology may often be an adaptive result of evolutionary processes (Koolhaas et al., 1999; Réale et al., 2007; Koolhaas, 2008) and previous research revealed differences in immune parameters between different personality types (Koolhaas, 2008). However, it is not clear whether the here observed difference in T cell proportions is a prerequisite of becoming highly or low explorative or a consequence of the personality type. Depending on the evolutionary hypothesis, highly explorative individuals should either invest more in their immune defenses (risk-of-parasitism hypothesis (Barber and Dingemanse, 2010)) or shift investment from immune defenses to current reproduction (pace-of-life hypothesis (Jacques-Hamilton et al., 2017)). Based on our findings on immune cell composition we cannot support or reject either of the two. Since we found reduced proportions of T cells in highly explorative mice, while the total numbers of splenocytes did not differ, there must be other cell populations with increased proportions in these animals. This rather supports the idea that the personality type is associated with a shift in immune function, which makes the animals more resistant to some pathogens and more susceptible to others as it has been shown before (Koolhaas, 2008). Whether the observed changes in immune cell composition would markedly influence the effectiveness of the immune defense, would have to be tested in future experiments.
4.3 Complex housing environments improve animal welfare
Lastly, we contrasted simple with complex housing conditions for their effects on animal welfare and the immune system. Mice housed under simple conditions showed more often stereotypic and nesting behavior and displayed increased agonistic behavior, but also increased social sniffing and grooming frequencies (but not durations) compared with mice housed under complex conditions. Shortly after being subjected to the new housing conditions, complex housed mice showed elevated corticosterone concentrations and reduced body weight, but at the end of the experiment, simple housed mice displayed elevated corticosterone concentrations, lower bodyweight and showed lower proportions of neutrophils while they had increased percentages of both, TNF- and IL-12-producing myeloid cells after LPS stimulation.
Regarding stereotypic and agonistic behaviors, as well as physiological and immunological parameters at the end of the experiment, our results are in line with the current state of knowledge: among various other positive effects that enriched environments have for the welfare of animals in captivity, reduced stereotypic and agonistic behavior, reduced corticosterone levels and increased bodyweight have been repeatedly reported (e.g., Sztainberg et al., 2010; Gross et al., 2011; Nip et al., 2019; Hobbiesiefken et al., 2021). Also, changes in immune responses and immune cell composition, especially adaptive immune cells comprising T cells and T cell subsets, but also natural killer cells are often affected by housing conditions (Marashi et al., 2003; Gurfein et al., 2014; Meng et al., 2019; Xiao et al., 2021; de Sousa Fernandes et al., 2022). Increased percentages of neutrophils have e.g., been reported in the blood of enriched housed mice (Brod et al., 2017). Hence, our results support previous research and clearly indicate improved welfare under complex housing conditions.
Pointing in another direction, mice in simple housing conditions showed increased frequencies of solitary grooming and social sniffing behavior, which are often interpreted as indicators for positive welfare (Freund et al., 2015; Kästner et al., 2019; Cunha et al., 2020). However, as increased frequencies of solitary grooming can also indicate stress-evoked grooming (Kalueff and Tuohimaa, 2004; Gould et al., 2009) and social sniffing behavior was shown to increase in smaller cages as an artefact of space-induced social interaction rates (Vestal and Hellack, 1977; Nip et al., 2019; but see: Ferhat et al., 2015), these results do not compellingly contradict our overall finding of improved welfare under complex conditions. Furthermore, shortly after being subjected to the new housing conditions, we observed a temporary increase in FCM concentrations accompanied by a reduction in body weight in complex housed mice. Differences between initial and experimental housing were more pronounced in complex conditions: whereas in simple conditions two enrichment items in the otherwise identical cage were exchanged, complex conditions additionally presented an entire three-dimensionally structured compartment with many unfamiliar items and materials. Familiarization with the bigger playgrounds requires more physical activity, which increases corticosterone levels in mice (Coleman et al., 1998; Girard and Garland, 2002) and is also reflected by a lower bodyweight at the same time. Though, also novelty-induced stress leads to short-term increase of corticosterone levels and exploratory locomotion in mice (Kurumaji et al., 2011). However, corticosterone concentrations and body weight normalized shortly after and even pointed towards lower FCM levels and heavier mice in complex housing conditions at t3 (i.e., from week 6 onwards), which was further supported by lower plasma corticosterone levels at the end of the experiment, indicating improved welfare in complex conditions in the long term.
4.4 Conclusion
To the best of our knowledge, this is the first study that empirically investigated (and found) personality-dependent welfare consequences in response to the housing environment in laboratory mice. We showed that refined housing conditions do not necessarily improve the welfare of all individuals equally. This has implications for the evaluation of animal welfare, where until now personality differences of individual animals are largely neglected (Richter and Hintze, 2019). Depending on the personality, individuals may vary in physiological (e.g., hormones, metabolism) or immunological aspects, which can affect individual susceptibility to stress or diseases (Koolhaas, 2008; Carere et al., 2010; de Boer et al., 2017; Niemelä and Dingemanse, 2018). Moreover, individuals differ in their responses to the environment they live in (Herrelko et al., 2012; Asher et al., 2016; Joshi and Pillay, 2016). Consequently, a “one-size-fits-all” tactic may not only be difficult to achieve, but also be an unsuitable approach. In contrast, scientists need to consider individual needs (Broom, 2008) in order to provide housing environments that fit the personality of all individuals of a group or population.
As an outlook, follow-up studies might investigate welfare consequences of personality-environment interactions over a longer period of time, as many animals (e.g., breeding animals) live much longer in their facilities than the 10 weeks we have covered. Furthermore, welfare-relevant personality-environment interactions are certainly not limited to exploration personality and/or differently complex housing environments but can include various environmental factors (e.g., animal density or social context (Whittaker et al., 2012; Kappel et al., 2017)) and related personality traits (e.g., aggression or sociability). Whether or not a personality-environment combination critically influences individual welfare is likely to depend on the specific ecology of the species in question. Therefore, it remains to be investigated whether our results for female C57BL6/J mice can also be transferred to other groups of animals (i.e., the other sex, other strains and species). Hence, more studies are needed that address personality differences and their consequences for individual animal welfare.
Data availability statement
The original contributions presented in the study are publicly available. This data can be found here: https://doi.org/10.5281/zenodo.12704686.
Ethics statement
The animal study was approved by Landesamt für Natur, Umwelt und Verbraucherschutz Nordrhein-Westfalen “LANUV NRW,” reference number 81-02.04.2021.A127. The study was conducted in accordance with the local legislation and institutional requirements.
Author contributions
MGUS: Conceptualization, Data curation, Formal analysis, Investigation, Methodology, Project administration, Visualization, Writing – original draft, Writing – review & editing. OA: Data curation, Formal analysis, Investigation, Methodology, Resources, Supervision, Visualization, Writing – original draft, Writing – review & editing. CD: Data curation, Investigation, Writing – review & editing. RP: Investigation, Writing – review & editing. SK: Conceptualization, Funding acquisition, Investigation, Methodology, Resources, Supervision, Writing – review & editing. SHR: Conceptualization, Funding acquisition, Investigation, Methodology, Resources, Supervision, Writing – review & editing.
Funding
The author(s) declare financial support was received for the research, authorship, and/or publication of this article. This project was funded by the German Research Foundation (DFG) to SHR–RTG2220–project number 281125614.
Acknowledgments
The authors thank Clarissa Lindemann, Edith Ossendorf and Rebecca Ross for excellent animal care and technical assistance, Nadine Sudhof and Eileen Flakowski for the analysis of plasma corticosterone and immune system measures, and Edith Klobetz-Rassam for FCM analysis.
Conflict of interest
The authors declare that the research was conducted in the absence of any commercial or financial relationships that could be construed as a potential conflict of interest.
Publisher’s note
All claims expressed in this article are solely those of the authors and do not necessarily represent those of their affiliated organizations, or those of the publisher, the editors and the reviewers. Any product that may be evaluated in this article, or claim that may be made by its manufacturer, is not guaranteed or endorsed by the publisher.
Supplementary material
The Supplementary Material for this article can be found online at: https://www.frontiersin.org/articles/10.3389/fanim.2024.1423814/full#supplementary-material
References
Almeida-Souza T. H., Goes T. C., Teixeira-Silva F. (2015). Pharmacological validation of the free-exploratory paradigm in male Wistar rats: A proposed test of trait anxiety. Pharmacol. Biochem. Behav. 135, 114–120. doi: 10.1016/j.pbb.2015.03.024
Ambrée O., Ruland C., Scheu S., Arolt V., Alferink J. (2018). Alterations of the innate immune system in susceptibility and resilience after social defeat stress. Front. Behav. Neurosci. 12. doi: 10.3389/fnbeh.2018.00141
Ambrée O., Ruland C., Zwanzger P., Klotz L., Baune B. T., Arolt V., et al. (2019). Social defeat modulates T helper cell percentages in stress susceptible and resilient mice. Int. J. Mol. Sci. 20, 1–14. doi: 10.3390/ijms20143512
Asher L., Friel M., Griffin K., Collins L. M. (2016). Mood and personality interact to determine cognitive biases in pigs. Biol. Lett. 12, 15–18. doi: 10.1098/rsbl.2016.0402
Bahi A. (2017). Environmental enrichment reduces chronic psychosocial stress-induced anxiety and ethanol-related behaviors in mice. Prog. Neuropsychopharmacol. Biol. Psychiatry 77, 65–74. doi: 10.1016/j.pnpbp.2017.04.001
Bailoo J. D., Murphy E., Boada-Saña M., Varholick J. A., Hintze S., Baussière C., et al. (2018a). Effects of cage enrichment on behavior, welfare and outcome variability in female mice. Front. Behav. Neurosci. 12. doi: 10.3389/fnbeh.2018.00232
Bailoo J. D., Murphy E., Varholick J. A., Novak J., Palme R., Würbel H. (2018b). Evaluation of the effects of space allowance on measures of animal welfare in laboratory mice. Sci. Rep. 8, 1–11. doi: 10.1038/s41598-017-18493-6
Barber I., Dingemanse N. J. (2010). Parasitism and the evolutionary ecology of animal personality. Philos. Trans. R. Soc. B: Biol. Sci. 365, 4077–4088. doi: 10.1098/rstb.2010.0182
Bates D., Mächler M., Bolker B. M., Walker S. C. (2015). Fitting linear mixed-effects models using lme4. J. Stat. Softw. 67 (1), 1–48. doi: 10.18637/jss.v067.i01
Bateson M., Martin P. (2021). Measuring behaviour: An introductory guide. 4th edition (Cambridge, UK: Cambridge University Press). doi: 10.1017/9781108776462
Baumans V. (2005). Environmental enrichment for laboratory rodents and rabbits: Requirements of rodents, rabbits, and research. ILAR J. 46, 162–170. doi: 10.1093/ilar.46.2.162
Baumans V. (2010). “The laboratory mouse,” in The UFAW handbook on The Care and Management of Laboratory and Other Research Animals, Eighth Edition. Eds. Hubrecht R., Kirkwood, J. (Wiley-Blackwell, United Kingdom).
Bell A. M., Hankison S. J., Laskowski K. L. (2009). The repeatability of behaviour: a meta-analysis. Anim. Behav. 77, 771–783. doi: 10.1016/j.anbehav.2008.12.022
Ben-Shachar M., Lüdecke D., Makowski D. (2020). effectsize: estimation of effect size indices and standardized parameters. J. Open Source Softw. 5, 2815. doi: 10.21105/joss.02815
Bierbach D., Laskowski K. L., Wolf M. (2017). Behavioural individuality in clonal fish arises despite near-identical rearing conditions. Nat. Commun. 8, 15361. doi: 10.1038/ncomms15361
Bodden C., von Kortzfleisch V. T., Karwinkel F., Kaiser S., Sachser N., Richter S. H. (2019). Heterogenising study samples across testing time improves reproducibility of behavioural data. Sci. Rep. 9, 1–9. doi: 10.1038/s41598-019-44705-2
Bourin M., Hascoët M. (2003). The mouse light/dark box test. Eur. J. Pharmacol. 463, 55–65. doi: 10.1016/S0014-2999(03)01274-3
Bračić M., Bohn L., Siewert V., Von Kortzfleisch V. T., Schielzeth H., Kaiser S., et al. (2022). Once an optimist, always an optimist? Studying cognitive judgment bias in mice. Behav. Ecol. 33, 775–788. doi: 10.1093/beheco/arac040
Brod S., Gobbetti T., Gittens B., Ono M., Perretti M., D’Acquisto F. (2017). The impact of environmental enrichment on the murine inflammatory immune response. JCI Insight 2, 1–14. doi: 10.1172/jci.insight.90723
Broom D. M. (2008). Welfare assessment and relevant ethical decisions: Key concepts. In. Annu. Rev. Biomed. Sci. 10, 79–90. doi: 10.5016/1806-8774.2008.v10pT79
Brown L. A., Hasan S., Foster R. G., Peirson S. N. (2017). COMPASS: Continuous open mouse phenotyping of activity and sleep status. Wellcome Open Res. 1, 1–17. doi: 10.12688/wellcomeopenres.9892.1
Busch M., Chourbaji S., Dammann P., Gerold S., Haemisch A., Jirkof P., et al. (2014). Fachinformation: Tiergerechte Haltung von Labormäusen Ausschuss für Tiergerechte Labortierhaltung. GV-SOLAS Soc. Lab. Anim. Sci. Fachinform. Available at: https://www.gv-solas.de/dokumente/
Campos-Luna I., Miller A., Beard A., Leach M. (2019). Validation of mouse welfare indicators: a Delphi consultation survey. Sci. Rep. 9, 1–11. doi: 10.1038/s41598-019-45810-y
Cao L., Choi E. Y., Liu X., Martin A., Wang C., Xu X., et al. (2011). White to brown fat phenotypic switch induced by genetic and environmental activation of a hypothalamic-adipocyte axis. Cell Metab. 14, 324–338. doi: 10.1016/j.cmet.2011.06.020
Cao L., Liu X., Lin E. J. D., Wang C., Choi E. Y., Riban V., et al. (2010). Environmental and genetic activation of a brain-adipocyte BDNF/leptin axis causes cancer remission and inhibition. Cell 142, 52–64. doi: 10.1016/j.cell.2010.05.029
Carere C., Caramaschi D., Fawcett T. W. (2010). Covariation between personalities and individual differences in coping with stress: Converging evidence and hypotheses. Curr. Zool. 56, 728–740. doi: 10.1093/czoolo/56.6.728
Carere C., Maestripieri D. (2013). “Introduction: animal personalities: who cares and why?,” in Animal Personalities: Behavior, Physiology, and Evolution. Eds. Carere C., Maestripieri D. (University of Chicago Press, Chicago).
Chen Q., Panksepp J. B., Lahvis G. P. (2009). Empathy is moderated by genetic background in mice. PloS One 4, e4387. doi: 10.1371/journal.pone.0004387
Coleman M. A., Garland T., Marler C. A., Newton S. S., Swallow J. G., Carter P. A. (1998). Glucocorticoid response to forced exercise in laboratory house mice (Mus domesticus). Physiol. Behav. 63, 279–285. doi: 10.1016/S0031-9384(97)00441-1
Crawley J. N. (1985). Exploratory behavior models of anxiety in mice. Neurosci. Biobehav. Rev. 9, 37–44. doi: 10.1016/0149-7634(85)90030-2
Crawley J. N., Schleidt W. M., Contrera J. F. (1975). Does social environment decrease propensity to fight in male mice? Behav. Biol. 15, 73–83. doi: 10.1016/S0091-6773(75)92105-7
Crusio W. E., Sluyter F., Gerlai R. T. (2013). Ethogram of the mouse. Behav. Genet. Mouse I, 17–22. doi: 10.1017/CBO9781139541022
Cunha A. M., Pereira-Mendes J., Almeida A., Guimarães M. R., Leite-Almeida H. (2020). Chronic pain impact on rodents’ behavioral repertoire. Neurosci. Biobehav. Rev. 119, 101–127. doi: 10.1016/j.neubiorev.2020.09.022
de Boer S. F., Buwalda B., Koolhaas J. M. (2017). Untangling the neurobiology of coping styles in rodents: Towards neural mechanisms underlying individual differences in disease susceptibility. Neurosci. Biobehav. Rev. 74, 401–422. doi: 10.1016/j.neubiorev.2016.07.008
de Sousa Fernandes M. S., Santos G. C. J., Filgueira T. O., Gomes D. A., Barbosa E. A. S., dos Santos T. M., et al. (2022). Cytokines and immune cells profile in different tissues of rodents induced by environmental enrichment: systematic review. Int. J. Mol. Sci. 23, 1–16. doi: 10.3390/ijms231911986
Eccard J. A., Herde A., Schuster A. C., Liesenjohann T., Knopp T., Heckel G., et al. (2022). Fitness, risk taking, and spatial behavior covary with boldness in experimental vole populations. Ecol. Evol. 12, 1–15. doi: 10.1002/ece3.8521
FC M., Davis T. (2022). _ggpattern: ‘ggplot2’ Pattern Geoms_. R package version 1.0.1. Available online at: https://CRAN.R-project.org/package=ggpattern.
Ferhat A. T., Le Sourd A. M., De Chaumont F., Olivo-Marin J. C., Bourgeron T., Ey E. (2015). Social communication in mice - Are there optimal cage conditions? PloS One 10, 1–19. doi: 10.1371/journal.pone.0121802
File S. E., Seth P. (2003). A review of 25 years of the social interaction test. Eur. J. Pharmacol. 463, 35–53. doi: 10.1016/S0014-2999(03)01273-1
Finkemeier M. A., Langbein J., Puppe B. (2018). Personality research in mammalian farm animals: Concepts, measures, and relationship to welfare. Front. Vet. Sci. 5. doi: 10.3389/fvets.2018.00131
Fox J., Weisberg S. (2019). An R Companion to Applied Regression. 3rd ed. (Thousand Oaks, CA: Sage PublicationsSage CA).
Franks B., Higgins E. T., Champagne F. A. (2014). A theoretically based model of rat personality with implications for welfare. PloS One 9 (4), e95135. doi: 10.1371/journal.pone.0095135
Freund J., Brandmaier A. M., Lewejohann L., Kirste I., Kritzler M., Krüger A., et al. (2013). Emergence of individuality in genetically identical mice. Science 340, 756–759. doi: 10.1126/science.1235294
Freund J., Brandmaier A. M., Lewejohann L., Kirste I., Kritzler M., Krüger A., et al. (2015). Association between exploratory activity and social individuality in genetically identical mice living in the same enriched environment. Neuroscience 309, 140–152. doi: 10.1016/j.neuroscience.2015.05.027
Fureix C., Walker M., Harper L., Reynolds K., Saldivia-Woo A., Mason G. (2016). Stereotypic behaviour in standard non-enriched cages is an alternative to depression-like responses in C57BL/6 mice. Behav. Brain Res. 305, 186–190. doi: 10.1016/j.bbr.2016.02.005
Gaskill B. N., Karas A. Z., Garner J. P., Pritchett-Corning K. R. (2013). Nest building as an indicator of health and welfare in laboratory mice. J. Visual. Experiments : JoVE 82, 51012. doi: 10.3791/51012
Girard I., Garland T. (2002). Plasma corticosterone response to acute and chronic voluntary exercise in female house mice. J. Appl. Physiol. 92, 1553–1561. doi: 10.1152/japplphysiol.00465.2001
Gosling S. D. (2001). From mice to men: What can we learn about personality from animal research? psychol. Bull. 127, 45–86. doi: 10.1037/0033-2909.127.1.45
Gould T. D., Dao D. T., Kovacsics C. E. (2009). Mood and anxiety related phenotypes in mice. Neuromethods 42, 1–20. doi: 10.1007/978-1-60761-303-9
Gouveia K., Hurst J. L. (2013). Reducing mouse anxiety during handling: effect of experience with handling tunnels. PloS One 8 (6): e66401. doi: 10.1371/journal.pone.0066401
Gouveia K., Hurst J. L. (2017). Optimising reliability of mouse performance in behavioural testing: The major role of non-aversive handling. Sci. Rep. 7, 1–12. doi: 10.1038/srep44999
Griebel G., Belzung C., Misslin R., Vogel E. (1993). The free-exploratory paradigm: an effective method for measuring neophobic behaviour in mice and testing potential neophobia-reducing drugs. Behav. Pharmacol. 4, 637–644. doi: 10.1097/00008877-199312000-00009
Gross A. N., Engel A. K. J., Richter S. H., Garner J. P., Würbel H. (2011). Cage-induced stereotypies in female ICR CD-1 mice do not correlate with recurrent perseveration. Behav. Brain Res. 216, 613–620. doi: 10.1016/j.bbr.2010.09.003
Guayasamin O. L., Couzin I. D., Miller N. Y. (2017). Behavioural plasticity across social contexts is regulated by the directionality of inter-individual differences. Behav. Process. 141 (2), 196–204. doi: 10.1016/j.beproc.2016.10.004
Gurfein B. T., Davidenko O., Premenko-Lanier M., Milush J. M., Acree M., Dallman M. F., et al. (2014). Environmental enrichment alters splenic immune cell composition and enhances secondary influenza vaccine responses in Mice. Mol. Med. 20, 179–190. doi: 10.2119/molmed.2013.00158
Hartig F. (2022). _DHARMa: Residual Diagnostics for Hierarchical (Multi-Level/Mixed) Regression Models_. R package version 0.4.6. Available online at: https://CRAN.R-project.org/package=DHARMa.
Hau M., Casagrande S., Ouyang J. Q., Baugh A. T. (2016). Glucocorticoid-mediated phenotypes in vertebrates: multilevel variation and evolution. Adv. Study Behav. 48 (1), 41–115. doi: 10.1016/bs.asb.2016.01.002
Heredia L., Torrente M., Colomina M. T., Domingo J. L. (2014). Assessing anxiety in C57BL/6J mice: A pharmacological characterization of the open-field and light/dark tests. J. Pharmacol. Toxicol. Methods 69, 108–114. doi: 10.1016/j.vascn.2013.12.005
Herrelko E. S., Vick S. J., Buchanan-Smith H. M. (2012). Cognitive research in zoo-housed chimpanzees: influence of personality and impact on welfare. Am. J. Primatol. 74, 828–840. doi: 10.1002/ajp.22036
Hisaoka-Nakashima K., Tomimura Y., Yoshii T., Ohata K., Takada N., Zhang F. F., et al. (2019). High-mobility group box 1-mediated microglial activation induces anxiodepressive-like behaviors in mice with neuropathic pain. Prog. Neuropsychopharmacol. Biol. Psychiatry 92, 347–362. doi: 10.1016/j.pnpbp.2019.02.005
Hobbiesiefken U., Mieske P., Lewejohann L., Diederich K. (2021). Evaluation of different types of enrichment - their usage and effect on home cage behavior in female mice. PloS One 16, 1–22. doi: 10.1371/journal.pone.0261876
Hoogland M., Ploeger A. (2022). Two different mismatches: integrating the developmental and the evolutionary-mismatch hypothesis. Perspect. psychol. Sci. 17, 1737–1745. doi: 10.1177/17456916221078318
Huntingford F., Adams C. (2005). Behavioural syndromes in farmed fish: Implications for production and welfare. Behaviour 142, 1207–1221. doi: 10.1163/156853905774539382
Hurst J. L., West R. S. (2010). Taming anxiety in laboratory mice. Nat. Methods 7, 825–826. doi: 10.1038/nmeth.1500
Ijichi C. L., Collins L. M., Elwood R. W. (2013). Evidence for the role of personality in stereotypy predisposition. Anim. Behav. 85, 1145–1151. doi: 10.1016/j.anbehav.2013.03.033
Jacques-Hamilton R., Hall M. L., Buttemer W. A., Matson K. D., Gonçalves da Silva A., Mulder R. A., et al. (2017). Personality and innate immune defenses in a wild bird: Evidence for the pace-of-life hypothesis. Hormones Behav. 88, 31–40. doi: 10.1016/j.yhbeh.2016.09.005
Jha S., Dong B., Sakata K. (2011). Enriched environment treatment reverses depression-like behavior and restores reduced hippocampal neurogenesis and protein levels of brain-derived neurotrophic factor in mice lacking its expression through promoter IV. Trans. Psychiatry 1, 1–11. doi: 10.1038/tp.2011.33
Jones M. A., Mason G., Pillay N. (2011). Early environmental enrichment protects captive-born striped mice against the later development of stereotypic behaviour. Appl. Anim. Behav. Sci. 135, 138–145. doi: 10.1016/j.applanim.2011.08.015
Joshi S., Pillay N. (2016). Personality predicts the responses to environmental enrichment at the group but not within-groups in stereotypic African striped mice, Rhabdomys dilectus. Appl. Anim. Behav. Sci. 182, 44–52. doi: 10.1016/j.applanim.2016.06.006
Kalueff A. V., Tuohimaa P. (2004). Grooming analysis algorithm for neurobehavioural stress research. Brain Res. Protoc. 13, 151–158. doi: 10.1016/j.brainresprot.2004.04.002
Kappel S., Hawkins P., Mendl M. T. (2017). To group or not to group? Good practice for housing male laboratory mice. Animals 7, 1–25. doi: 10.3390/ani7120088
Kästner N., Richter S. H., Urbanik S., Kunert J., Waider J., Lesch K. P., et al. (2019). Brain serotonin deficiency affects female aggression. Sci. Rep. 9, 1–9. doi: 10.1038/s41598-018-37613-4
Kazlauckas V., Kalinine E., Leke R., Oses J. P., Nunes F., Espinosa J., et al. (2011). Distinctive effects of unpredictable subchronic stress on memory, serum corticosterone and hippocampal BDNF levels in high and low exploratory mice. Behav. Brain Res. 218, 80–86. doi: 10.1016/j.bbr.2010.11.030
Kazlauckas V., Schuh J., Dall’Igna O. P., Pereira G. S., Bonan C. D., Lara D. R. (2005). Behavioral and cognitive profile of mice with high and low exploratory phenotypes. Behav. Brain Res. 162, 272–278. doi: 10.1016/j.bbr.2005.03.021
Kempermann G., Kuhn H. G., Gage F. H. (1997). More hippocampal neurons in adult mice living in an enriched environment. Nature 386, 493–495. doi: 10.1038/386493a0
Knief U., Forstmeier W. (2021). Violating the normality assumption may be the lesser of two evils. Behav. Res. Methods 53, 2576–2590. doi: 10.3758/s13428-021-01587-5
Koolhaas J. M. (2008). Coping style and immunity in animals: Making sense of individual variation. Brain Behavior Immun. 22, 662–667. doi: 10.1016/j.bbi.2007.11.006
Koolhaas J. M., Korte S. M., De Boer S. F., van der Vegt B. J., Van Reenen C. G., Hopster H., et al. (1999). Coping styles in animals: Current status in behavior and stress- physiology. Neurosci. Biobehav. Rev. 23, 925–935. doi: 10.1016/S0149-7634(99)00026-3
Krebs R., Linnenbrink M., Guenther A. (2019). Validating standardised personality tests under semi-natural conditions in wild house mice (Mus musculus domesticus). Ethology 125, 761–773. doi: 10.1111/eth.12930
Krishnan V., Han M. H., Graham D. L., Berton O., Renthal W., Russo S. J., et al. (2007). Molecular adaptations underlying susceptibility and resistance to social defeat in brain reward regions. Cell 131, 391–404. doi: 10.1016/j.cell.2007.09.018
Kurumaji A., Umino M., Nishikawa T. (2011). Effects of novelty stress on hippocampal gene expression, corticosterone and motor activity in mice. Neurosci. Res. 71, 161–167. doi: 10.1016/j.neures.2011.06.006
Kuznetsova A., Brockhoff P. B., Christensen R. H. B. (2017). lmerTest package: tests in linear mixed effects models. J. Stat. Softw. 82, 1–26. doi: 10.18637/jss.v082.i13
Lakens D. (2013). Calculating and reporting effect sizes to facilitate cumulative science: A practical primer for t-tests and ANOVAs. Front. Psychol. 4. doi: 10.3389/fpsyg.2013.00863
Laskowski K. L., Chang C. C., Sheehy K., Aguiñaga J. (2022). Consistent individual behavioral variation: what do we know and where are we going? Annu. Rev. Ecol. Evol. System. 53, 161–182. doi: 10.1146/annurev-ecolsys-102220-011451
Lenth R. (2023). _emmeans: Estimated Marginal Means, aka Least-Squares Means_. R package version 1.8.7. Available online at: https://CRAN.R-project.org/package=emmeans.
Lüdecke D., Ben-Shachar M., Patil I., Waggoner P., Makowski D. (2021). performance: an R package for assessment, comparison and testing of statistical models. J. Open Source Softw. 6, 3139. doi: 10.21105/joss.03139
Marashi V., Barnekow A., Ossendorf E., Sachser N. (2003). Effects of different forms of environmental enrichment on behavioral, endocrinological, and immunological parameters in male mice. Hormones Behav. 43, 281–292. doi: 10.1016/S0018-506X(03)00002-3
Martin A. L., Brown R. E. (2010). The lonely mouse: Verification of a separation-induced model of depression in female mice. Behav. Brain Res. 207, 196–207. doi: 10.1016/j.bbr.2009.10.006
Mason G. J. (1991). Stereotypies: a critical review. Anim. Behav. 41, 1015–1037. doi: 10.1016/S0003-3472(05)80640-2
Mason G., Rushen J. (2006). Stereotypic animal behaviour: Fundamentals and applications to welfare, 2nd ed (Oxfordshire, UK: CAB International). doi: 10.1079/9780851990040.0000
Meijer M. K., Sommer R., Spruijt B. M., Van Zutphen L. F. M., Baumans V. (2007). Influence of environmental enrichment and handling on the acute stress response in individually housed mice. Lab. Anim. 41, 161–173. doi: 10.1258/002367707780378168
Meng Z., Liu T., Song Y., Wang Q., Xu D., Jiang J., et al. (2019). Exposure to an enriched environment promotes the terminal maturation and proliferation of natural killer cells in mice. Brain Behavior Immun. 77, 150–160. doi: 10.1016/j.bbi.2018.12.017
Natarajan D., De Vries H., Saaltink D. J., De Boer S. F., Koolhaas J. M. (2009). Delineation of violence from functional aggression in mice: An ethological approach. Behav. Genet. 39, 73–90. doi: 10.1007/s10519-008-9230-3
Niemelä P. T., Dingemanse N. J. (2018). Meta-analysis reveals weak associations between intrinsic state and personality. Proc. R. Soc. B: Biol. Sci. 285 (1873), 20172823. doi: 10.1098/rspb.2017.2823
Nip E., Adcock A., Nazal B., MacLellan A., Niel L., Choleris E., et al. (2019). Why are enriched mice nice? Investigating how environmental enrichment reduces agonism in female C57BL/6, DBA/2, and BALB/c mice. Appl. Anim. Behav. Sci. 217, 73–82. doi: 10.1016/j.applanim.2019.05.002
Niraula A., Wang Y., Godbout J. P., Sheridan J. F. (2018). Corticosterone production during repeated social defeat causes monocyte mobilization from the bone marrow, glucocorticoid resistance, and neurovascular adhesion molecule expression. J. Neurosci. 38, 2328–2340. doi: 10.1523/JNEUROSCI.2568-17.2018
Niraula A., Witcher K. G., Sheridan J. F., Godbout J. P. (2019). Interleukin-6 induced by social stress promotes a unique transcriptional signature in the monocytes that facilitate anxiety. Biol. Psychiatry 85, 679–689. doi: 10.1016/j.biopsych.2018.09.030
Nithianantharajah J., Hannan A. J. (2006). Enriched environments, experience-dependent plasticity and disorders of the nervous system. Nat. Rev. Neurosci. 7, 697–709. doi: 10.1038/nrn1970
Pike T. W., Samanta M., Lindström J., Royle N. J. (2008). Behavioural phenotype affects social interactions in an animal network. Proc. R. Soc. B: Biol. Sci. 275, 2515–2520. doi: 10.1098/rspb.2008.0744
Posit team (2022). RStudio: Integrated Development Environment for R (Boston, MA: Posit Software, PBC). Available at: http://www.posit.co/.
R Core Team (2021). R: A language and environment for statistical computing (Vienna, Austria: R Foundation for Statistical Computing). Available at: https://www.R-project.org/.
Réale D., Reader S. M., Sol D., McDougall P. T., Dingemanse N. J. (2007). Integrating animal temperament within ecology and evolution. Biol. Rev. 82, 291–318. doi: 10.1111/j.1469-185X.2007.00010.x
Reimert I., Webb L. E., van Marwijk M. A., Bolhuis J. E. (2023). Review: Towards an integrated concept of animal welfare. Animal 17, 100838. doi: 10.1016/j.animal.2023.100838
Richter S. H., Hintze S. (2019). From the individual to the population – and back again? Emphasising the role of the individual in animal welfare science. Appl. Anim. Behav. Sci. 212, 1–8. doi: 10.1016/j.applanim.2018.12.012
Rohleder N., Aringer M., Boentert M. (2012). Role of interleukin-6 in stress, sleep, and fatigue. Ann. New York Acad. Sci. 1261, 88–96. doi: 10.1111/j.1749-6632.2012.06634.x
Sachser N., Hennessy M. B., Kaiser S. (2011). Adaptive modulation of behavioural profiles by social stress during early phases of life and adolescence. Neurosci. Biobehav. Rev. 35, 1518–1533. doi: 10.1016/j.neubiorev.2010.09.002
Sachser N., Kaiser S., Hennessy M. B. (2013). Behavioural profiles are shaped by social experience: When, how and why. Philos. Trans. R. Soc. B: Biol. Sci. 368 (1618), 20120344. doi: 10.1098/rstb.2012.0344
Sapolsky R. M., Romero L. M., Munck A. U. (2000). How do glucocorticoids influence stress responses? Integrating permissive, suppressive, stimulatory, and preparative actions. Endocr. Rev. 21, 55–89. doi: 10.1210/er.21.1.55
Schielzeth H., Dingemanse N. J., Nakagawa S., Westneat D. F., Allegue H., Teplitsky C., et al. (2020). Robustness of linear mixed-effects models to violations of distributional assumptions. Methods Ecol. Evol. 11, 1141–1152. doi: 10.1111/2041-210X.13434
Schmidt M. V. (2011). Animal models for depression and the mismatch hypothesis of disease. Psychoneuroendocrinology 36, 330–338. doi: 10.1016/j.psyneuen.2010.07.001
Shapiro S. S., Wilk M. B. (1965). An analysis of variance test for normality (Complete samples). Biometrika 52, 591. doi: 10.2307/2333709
Sih A., Bell A., Johnson J. C. (2004). Behavioral syndromes: An ecological and evolutionary overview. Trends Ecol. Evol. 19, 372–378. doi: 10.1016/j.tree.2004.04.009
Slater A. M., Cao L. (2015). A protocol for housing mice in an enriched environment. J. Visual. Experiments 2015, 1–8. doi: 10.3791/52874
Staley M., Conners M. G., Hall K., Miller L. J. (2018). Linking stress and immunity: Immunoglobulin A as a non-invasive physiological biomarker in animal welfare studies. Hormones Behav. 102, 55–68. doi: 10.1016/j.yhbeh.2018.04.011
Stoffel M. A., Nakagawa S., Schielzeth H. (2017). rptR: repeatability estimation and variance decomposition by generalized linear mixed-effects models. Methods Ecol. Evol. 8, 1639–1644. doi: 10.1111/2041-210X.12797. British Ecological Society.
Stoffel M. A., Nakagawa S., Schielzeth H. (2021). partR2: Partitioning R2 in generalized linear mixed models. PeerJ 9, 1–17. doi: 10.7717/peerj.11414
Sztainberg Y., Kuperman Y., Tsoory M., Lebow M., Chen A. (2010). The anxiolytic effect of environmental enrichment is mediated via amygdalar CRF receptor type 1. Mol. Psychiatry 15, 905–917. doi: 10.1038/mp.2009.151
Taitt K. T., Kendall L. V. (2019). Physiologic stress of ear punch identification compared with restraint only in mice. J. Am. Assoc. Lab. Anim. Sci. 58, 438–442. doi: 10.30802/AALAS-JAALAS-18-000120
Tetley C. L., O’Hara S. J. (2012). Ratings of animal personality as a tool for improving the breeding, management and welfare of zoo mammals. Anim. Welf. 21, 463–476. doi: 10.7120/09627286.21.4.463
Touma C., Palme R., Sachser N. (2004). Analyzing corticosterone metabolites in fecal samples of mice: A noninvasive technique to monitor stress hormones. Hormones Behav. 45, 10–22. doi: 10.1016/j.yhbeh.2003.07.002
Touma C., Sachser N., Möstl E., Palme R. (2003). Effects of sex and time of day on metabolism and excretion of corticosterone in urine and feces of mice. Gen. Comp. Endocrinol. 130, 267–278. doi: 10.1016/S0016-6480(02)00620-2
van der Goot M. H., Boleij H., van den Broek J., Salomons A. R., Arndt S. S., van Lith H. A. (2020). An individual based, multidimensional approach to identify emotional reactivity profiles in inbred mice. J. Neurosci. Methods 343, 108810. doi: 10.1016/j.jneumeth.2020.108810
Van Loo P. L. P., Kruitwagen C. L. J. J., Koolhaas J. M., Van De Weerd H. A., Van Zutphen L. F. M., Baumans V. (2002). Influence of cage enrichment on aggressive behaviour and physiological parameters in male mice. Appl. Anim. Behav. Sci. 76, 65–81. doi: 10.1016/S0168-1591(01)00200-3
Verjat A., Devienne P., Rödel H. G., Féron C. (2020). More exploratory house mice judge an ambiguous situation more negatively. Anim. Cogn. 24, 53–64. doi: 10.1007/s10071-020-01414-y
Vestal B. M., Hellack J. J. (1977). Effects of available space on social interactions in male white-footed mice (Peromyscus leucopus). Behav. Biol. 19, 289–299. doi: 10.1016/S0091-6773(77)91598-X
Wasserstein R. L., Lazar N. A. (2016). The ASA’s statement on p-values: context, process, and purpose. Am. Statistic. 70, 129–133. doi: 10.1080/00031305.2016.1154108
Whittaker A. L., Howarth G. S., Hickman D. L. (2012). Effects of space allocation and housing density on measures of wellbeing in laboratory mice: A review. Lab. Anim. 46, 3–13. doi: 10.1258/la.2011.011049
Wickham H. (2016). ggplot2: Elegant Graphics for Data Analysis. 2nd ed. (Switzerland: Springer Nature of the Springer International Publishing AG). doi: 10.1007/978-3-319-24277-4
Wohleb E. S., McKim D. B., Shea D. T., Powell N. D., Tarr A. J., Sheridan J. F., et al. (2014). Re-establishment of anxiety in stress-sensitized mice is caused by monocyte trafficking from the spleen to the brain. Biol. Psychiatry 75, 970–981. doi: 10.1016/j.biopsych.2013.11.029
Würbel H. (2001). Ideal homes? Housing effects on rodent brain and behaviour. Trends Neurosci. 24, 207–211. doi: 10.1016/S0166-2236(00)01718-5
Xiao R., Ali S., Caligiuri M. A., Cao L. (2021). Enhancing effects of environmental enrichment on the functions of natural killer cells in mice. Front. Immunol. 12. doi: 10.3389/fimmu.2021.695859
Yalcin I., Aksu F., Bodard S., Chalon S., Belzung C. (2007). Antidepressant-like effect of tramadol in the unpredictable chronic mild stress procedure: Possible involvement of the noradrenergic system. Behav. Pharmacol. 18, 623–631. doi: 10.1097/FBP.0b013e3282eff109
Zhang D. (2023). _rsq: R-Squared and Related Measures_. R package version 2.6. Available online at: https://CRAN.R-project.org/package=rsq.
Keywords: animal personality, animal welfare, immune system, housing, environmental enrichment, laboratory mice
Citation: Sroka MGU, Ambree O, Dohmen C, Palme R, Kaiser S and Richter SH (2024) Personality matters – The interplay between consistent individual differences and mouse welfare in female C57BL6/J mice. Front. Anim. Sci. 5:1423814. doi: 10.3389/fanim.2024.1423814
Received: 26 April 2024; Accepted: 25 July 2024;
Published: 20 August 2024.
Edited by:
E. Tobias Krause, Friedrich-Loeffler-Institute, GermanyReviewed by:
Debra Hickman, Purdue University, United StatesJeremy Davidson Bailoo, Texas Tech University Health Sciences Center, United States
Copyright © 2024 Sroka, Ambree, Dohmen, Palme, Kaiser and Richter. This is an open-access article distributed under the terms of the Creative Commons Attribution License (CC BY). The use, distribution or reproduction in other forums is permitted, provided the original author(s) and the copyright owner(s) are credited and that the original publication in this journal is cited, in accordance with accepted academic practice. No use, distribution or reproduction is permitted which does not comply with these terms.
*Correspondence: Marlene G. U. Sroka, bWFybGVuZS5zcm9rYUB1bmktbXVlbnN0ZXIuZGU=