- 1Livestock Issues Research Unit, Agricultural Research Service, United States Department of Agriculture, Lubbock, TX, United States
- 2Department of Animal and Food Sciences, Texas Tech University, Lubbock, TX, United States
- 3Department of Veterinary Sciences, Texas Tech University, Lubbock, TX, United States
- 4Division of Animal Sciences, University of Missouri, Columbia, MO, United States
This study was designed to determine whether exposure to low-dose endotoxin (lipopolysaccharide; LPS) during gestation can enhance immunity to a subsequent LPS challenge in piglets after weaning. Pregnant sows (parity: 2.6 ± 1.4) were assigned to prenatal immune stimulation (PIS; n = 7; administered 2.5 µg/kg BW LPS, i.m.) or saline treatment groups (CON; n = 7) administered at day 78 ± 1.8 of gestation. From the two prenatal treatment groups, barrows (n = 17 PIS, 17 CON) were identified at weaning (21 ± 1.3 day of age) to subsequently receive a post-weaning LPS challenge. On day −1, the pigs were fitted with indwelling jugular catheters and subcutaneous temperature loggers. On day 0, the pigs were challenged i.v. with LPS (10 µg/kg BW), and blood samples were collected at −2, 0, 1, 2, 4, 6, 8, 12, and 24 h relative to LPS challenge. There was a treatment × time interaction for subcutaneous temperature (P < 0.01), where the temperature increased more quickly at 1 and 2 h post-challenge in PIS compared to CON pigs. There was a tendency (P = 0.08) for less change in white blood cells, relative to baseline values, in PIS compared to CON pigs. There was a treatment × time interaction (P = 0.01) for lymphocyte concentrations where the concentrations were reduced in PIS compared to CON pigs at 8 h post-challenge. There was also a treatment × time interaction (P = 0.01) for the change in eosinophil concentrations, where there was less change in eosinophil concentrations from 1 to 12 h in PIS compared to CON pigs. There was a tendency (P ≤ 0.06) for a treatment × time interaction for serum interleukin-6 (IL-6) and IL-8. Granulocyte-macrophage colony-stimulating factor tended to be greater, and tumor necrosis factor-α tended to be reduced in PIS compared to CON pigs (P ≤ 0.08). These data suggest that exposure to endotoxin in utero may influence the postnatal innate immune response to endotoxin. More research is necessary to further understand the mechanism behind the differences observed and the potential long-term influence of prenatal immune stimulation on pig offspring.
1 Introduction
One area of swine production where large improvements can be made is swine health. Changes in genetic selection and the focus on rapid growth and lean muscle accretion have increased the susceptibility of pigs to diseases (Prunier et al., 2010; Flori et al., 2011). The increased disease susceptibility is accentuated during severe outbreaks of PRRS, PEDV, and ASF that have had devastating impacts on swine production around the world. Outbreaks of PRRS alone result in over $664 million in losses to the US swine industry annually. Additionally, there has been increased consumer and legislative pressure to reduce the use of antimicrobials in livestock, thus increasing the need for antibiotic alternatives. While some of these products, such as probiotics, have shown potential, they typically need to be fed prior to a health challenge, which is difficult to predict outside of the typical stressful production stages (e.g., weaning). Therefore, alternative strategies are needed to help maintain and improve swine health.
One alternative method to improve pig health may be through altering immune responsiveness prior to birth. The immune system of pigs at birth is immature due to the lack of exposure to pathogens and no transfer of immunity across the placenta (Salmon et al., 2009). Thus, neonatal pigs are dependent upon passive transfer of immunity from colostrum shortly after birth. Vaccination of the pregnant sow is used such that immunoglobulins in the colostrum offer some protection against common swine pathogens. However, some vaccines have poor lactogenic immunity and thus do not provide sufficient protection to piglets (Matias et al., 2017; Langel et al., 2020).
Previous work in our laboratory studied the effect of low-dose lipopolysaccharide (LPS) during gestation on the growth and immune response to a subsequent LPS challenge after weaning in cattle. Data from these studies indicate that a single low-dose LPS administration in the last third of gestation increased the 205-day adjusted weaning weights in steer and heifer calves (Burdick Sanchez et al., 2017). Additionally, when heifer calves were administered LPS post-weaning, they had an extended vaginal temperature and interleukin-6 (IL-6) response compared to control heifers (Carroll et al., 2017). However, when LPS was administered to calves once in each third of gestation, no differences in vaginal temperature response were observed, and the IL-6 response was less in calves exposed to LPS in utero compared to control calves (Carroll et al., 2021). These studies indicate that immune responsiveness in cattle may be altered using low-dose LPS administration during gestation, thus suggesting that a similar method can be used in swine. Therefore, this study was designed to determine whether the administration of low-dose LPS during the last third of gestation in sows would alter the acute phase response of piglets to a subsequent LPS challenge after weaning. The hypothesis was that piglets exposed to LPS during gestion would have an altered immune response to a second LPS exposure following weaning.
2 Materials and methods
2.1 Experimental design
All experimental procedures were in compliance with the Guide for the Care and Use of Agricultural Animals in Research and Teaching and approved by the Institutional Animal Care and Use Committee at Texas Tech University (IACUC Protocol # 2022-116) and the Livestock Issues Research Unit (IACUC Protocol # 2022_06).
2.1.1 Sow LPS challenge
Pregnant Camborough sows (parity 2.6 ± 1.4) were assigned to one of two treatments: (1) prenatal immune stimulation (PIS; n = 7), administered 2.5 µg/kg BW LPS from E. coli O111:B4 (Sigma Aldrich, St. Louis, MO, USA) intramuscularly in the shoulder, or (2) control (CON; n = 7), administered a similar volume of saline intramuscularly in the shoulder. Treatments were administered at day 78 ± 1.8 of gestation. Relative to the administration of LPS at 0 h, rectal temperature was measured manually at −0.5, 0, 1, 2, 3, 4, 5, 6, 7, 8, 16, 24, 32, 40, and 48 h using a handheld thermometer. Following the administration of treatments, sow gestation was allowed to proceed normally according to farm standard operating procedures. At weaning (21 ± 1.3 days of age), 17 CON and 17 PIS barrows, balanced by sow and body weight, were selected and transported appropriately 10 km to the USDA-ARS Livestock Issues Research Unit Swine Facility for immune challenge and sampling.
2.1.2 Piglet challenge
Upon arrival, the piglets were placed in individual stainless steel pens (0.6 × 1.2 m) in an environmentally controlled room. Pigs had ad libitum access to water through nipple waterers, and a standard nursery ration was formulated to meet or exceed NRC requirements. After a 7-day acclimatization period, the pigs were fitted with jugular vein catheters (Carroll et al., 1999) for serial blood sample collection and subcutaneous temperature loggers (Burdick Sanchez et al., 2023) that were programmed to measure subcutaneous body temperature continuously at 5-min intervals. Following these procedures, the pigs were returned to their pen and allowed to rest overnight. On the next day (day 0), the pigs were administered 10 µg/kg BW LPS intravenously at 0 h. Whole blood samples (4 mL) were collected into evacuated tubes containing EDTA at −2, 0, 2, 4, 6, 8, 12, and 24 h relative to LPS administration at 0 h for the measurement of complete blood counts using ProCyte DX Hematology Analyzer (IDEXX Laboratories, Inc., Westbrook, ME, USA). Two additional 4-mL samples were collected in evacuated tubes containing no additive or containing Li heparin for isolation of serum and plasma, respectively, at -2, 0, 1, 2, 4, 6, 8, 12, and 24 h relative to LPS administration at 0 h. For plasma isolation, the samples were centrifuged immediately at 1,500 × g for 20 min at 4°C, and plasma was aliquoted and stored at −80°C until analysis. For serum isolation, the samples were allowed to clot at room temperature for 30 min prior to centrifugation at 1,500 × g for 20 min at 4°C. Serum was aliquoted and stored at −80°C until analysis.
2.2 Serum analysis
All serum analyses were performed in duplicate. Serum cortisol concentrations were determined using an enzyme immunoassay according to the manufacturer’s instructions (RayBioTech, Peachtree Corners, GA, USA). Concentrations were determined based on a standard curve of known cortisol concentrations. The intra- and inter-assay coefficients of variation were 10.3% and 12.5%, respectively. Serum glucose concentrations were determined by modification of the enzymatic Autokit Glucose (Wako Diagnostics, Richmond, VA, USA) to fit a 96-well format as previously described (Burdick Sanchez et al., 2016). The intra- and inter-assay coefficients of variation were 9.1% and 8.0%, respectively. Serum LPS binding protein (LBP) concentrations were determined on samples collected at 0, 2, 4, 6, and 8 h using ELISA according to the manufacturer’s instructions (Hycult Biotech Inc., Wayne, PA, USA). The concentrations were determined based on a standard curve of known LBP concentrations. The intra- and inter-assay coefficients of variation were 6.6% and 2.0%, respectively. The serum concentrations of IL-1β, IL-4, IL-6, IL-8, IL-10, IL-12p40p70, GM-CSF, IFN-γ, TFG-β1, and TNF-α were measured by RayBiotech using Quantibody Multiplex ELISA (Porcine Cytokine Array Q1, RayBiotech, Peachtree Corners, GA, USA). Serum cytokine concentrations were measured on serum samples collected, with the exception of the 12-h sample.
2.3 Statistical analysis
Prior to analysis, all temperature data were averaged into 1-h intervals. All data were analyzed as repeated measures over time using the MIXED procedure of SAS (SAS Inst. Inc., Cary, NC, USA; v. 9.4). Treatment, time, and the treatment × time interaction were included as fixed effects, with pig within treatment included as the experimental unit. The covariance structure used was based on having the lowest AIC fit statistic value. When the main effects were significant, the means were separated using the PDIFF option in SAS, with P ≤ 0.05 considered significant and 0.05 < P ≤ 0.10 considered a tendency. All data are presented as the least squares means ± standard error of the mean.
3 Results
3.1 Sow rectal temperature
To monitor the acute inflammatory response in sows administered LPS, rectal temperature was monitored in all sows relative to the challenge. Following the administration of LPS in sows at 0 h, there was a treatment × time interaction for rectal temperature (P < 0.01; Figure 1). Specifically, the rectal temperature was increased in sows in the PIS treatment group compared to the CON treatment group (administered saline) beginning at 2 h post-challenge, and the rectal temperature remained elevated through 16 h post-challenge before returning to baseline values. The sow rectal temperature increased approximately 1.5°C above baseline values and did not increase above 39.5°C during the challenge.
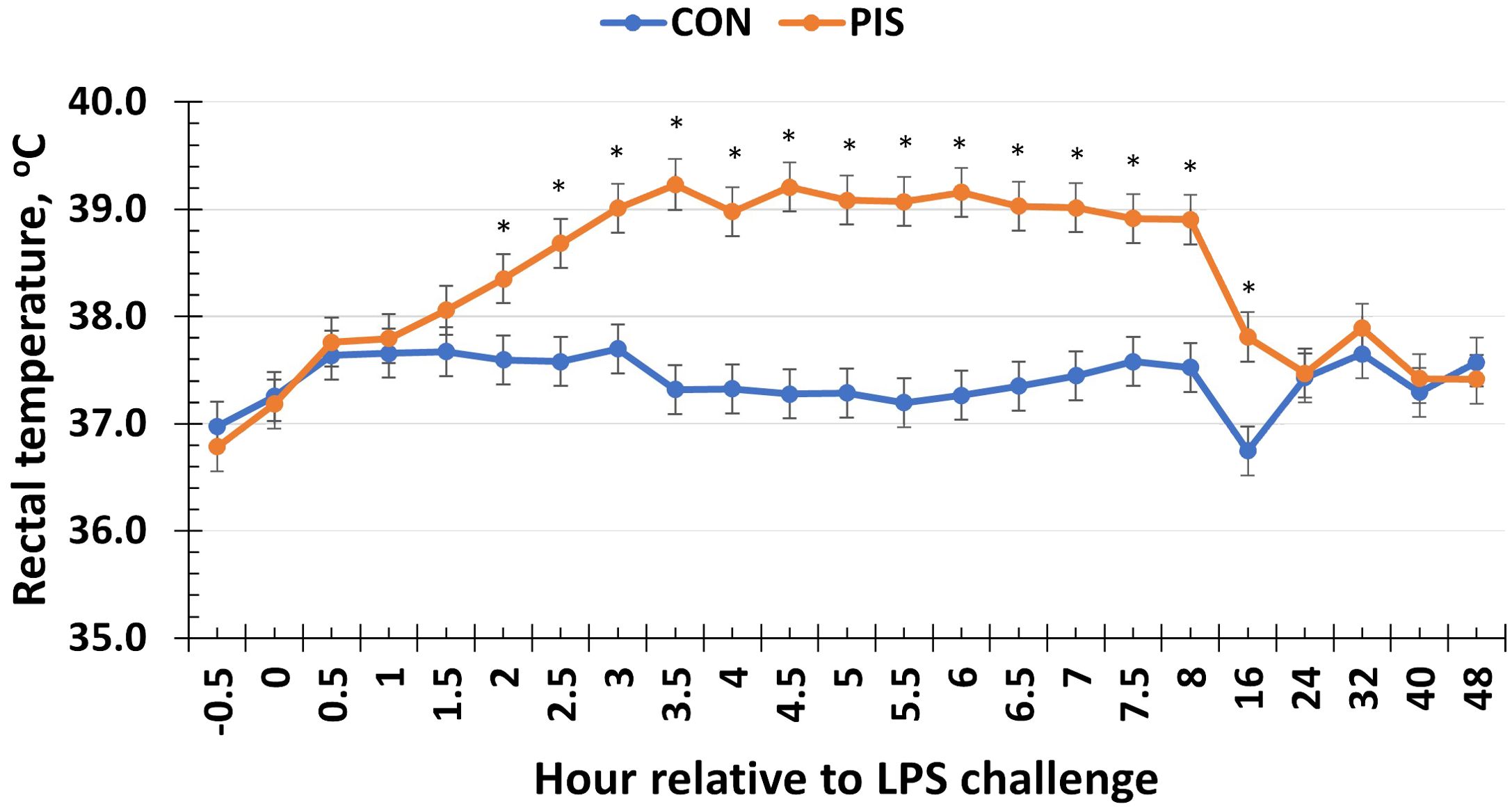
Figure 1. Rectal temperature of sows in response to a low-dose lipopolysaccharide (LPS; 2.5 µg/kg body weight, i.m.) administration (PIS) or saline (CON) at 78 ± 1.8 days of gestation. There was a treatment × time interaction (P < 0.01) for sow rectal temperature, where PIS sows had greater rectal temperature from 2 to 16 h relative to LPS administration at 0 h. Data are presented as LSM ± SEM. The asterisk means that the treatments differ (P ≤ 0.02).
3.2 Piglet subcutaneous body temperature
There was a treatment × time interaction (P < 0.01; Figure 2) for piglet subcutaneous body temperature in response to LPS administration in pigs following weaning. Specifically, the temperature increased more quickly in PIS piglets compared to CON piglets as evidenced by the greater temperature observed in PIS piglets at 1 and 2 h post-challenge. However, there were no differences (P ≥ 0.30) between treatment groups for the remainder of the challenge, and both treatment groups returned to baseline values by 12 h post-challenge.
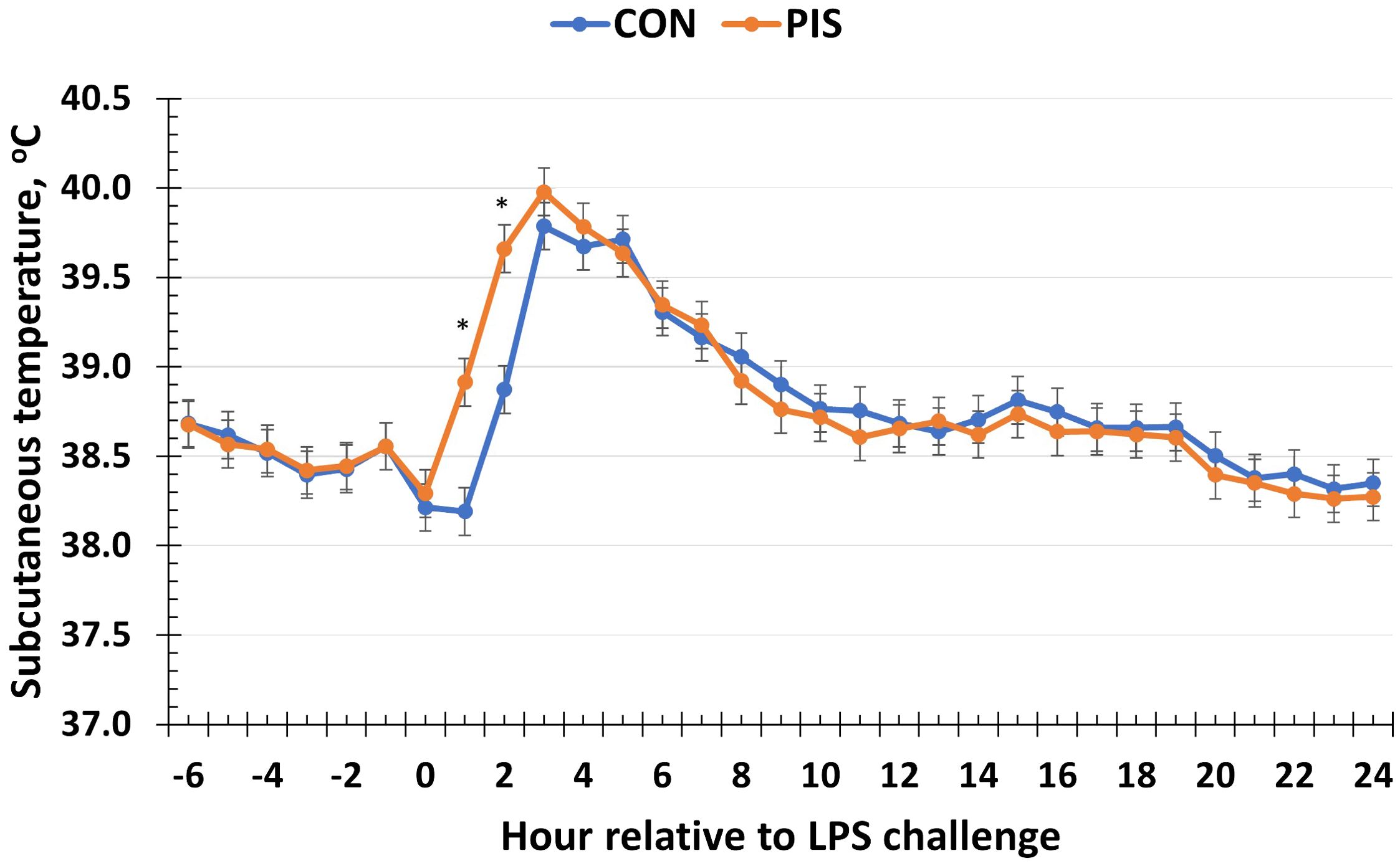
Figure 2. Subcutaneous temperature of piglets in response to a lipopolysaccharide challenge post-weaning. Piglets were selected at weaning from sows previously exposed to low-dose LPS at 78 ± 1.8 days of gestation (PIS) or saline (CON; n = 17 per treatment) and were subsequently challenged with LPS (10 µg/kg body weight i.v.). There was a treatment × time interaction (P < 0.01) for subcutaneous temperature, where temperature was greater in PIS compared to CON piglets at 1 and 2 h post-challenge. Data are presented as LSM ± SEM. The asterisk means that the treatments differ (P ≤ 0.01).
3.3 Hematology analysis
Hematology data are summarized in Table 1. There were no treatment differences nor treatment × time interactions for red blood cells, hemoglobin, hematocrit, platelets, neutrophils, monocytes, or basophils (P ≥ 0.14), although all variables changed over time (P < 0.01). There was also no treatment nor treatment × time interaction for total white blood cells (P ≥ 0.22); however, there was a tendency (P = 0.08) for pre-challenge concentrations of white blood cells at −2 h to be less in PIS than CON pigs. Therefore, the change in white blood cell concentrations relative to pre-challenge (−2 and 0 h) values was analyzed. There was a tendency (P = 0.08; Figure 3) for a treatment effect for the change in total white blood cells such that there was less change in PIS piglets compared to CON piglets (−0.26 versus −2.57 ± 0.91 K/µL). There was a treatment × time interaction for lymphocytes (P = 0.01; Figure 4), where PIS piglets had decreased lymphocyte concentrations at 8 h post-challenge compared to CON piglets. Similar to total white blood cells, there was a pre-challenge treatment difference (P = 0.04) in eosinophil concentrations, and therefore the change in eosinophil concentrations relative to pre-challenge values was analyzed. There was a treatment × time interaction (P < 0.01; Figure 5) for the change in eosinophil concentrations, where there was less change relative to the pre-challenge values in PIS piglets compared to CON piglets from 1 to 12 h post-LPS challenge.
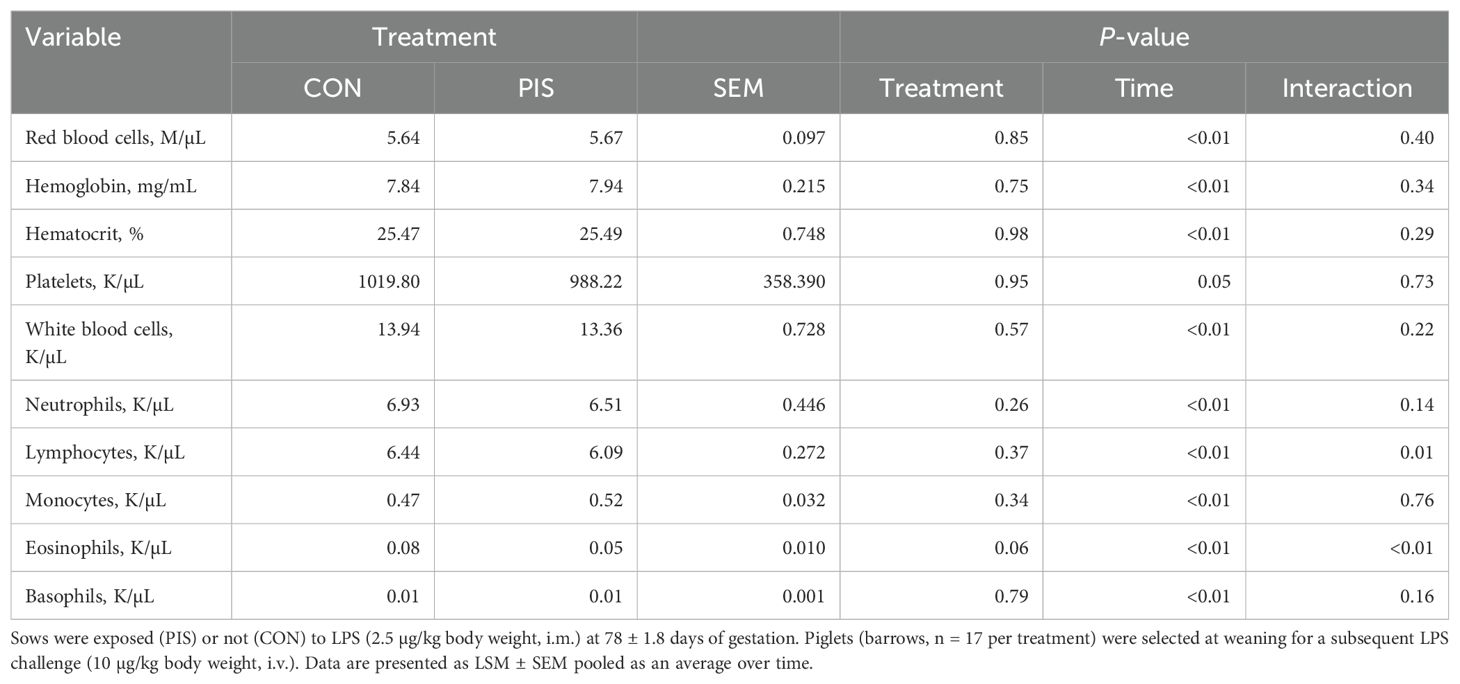
Table 1. Summary of blood hematology variables in piglets exposed or not to low-dose lipopolysaccharide (LPS) during gestation on the subsequent response to LPS post-weaning.
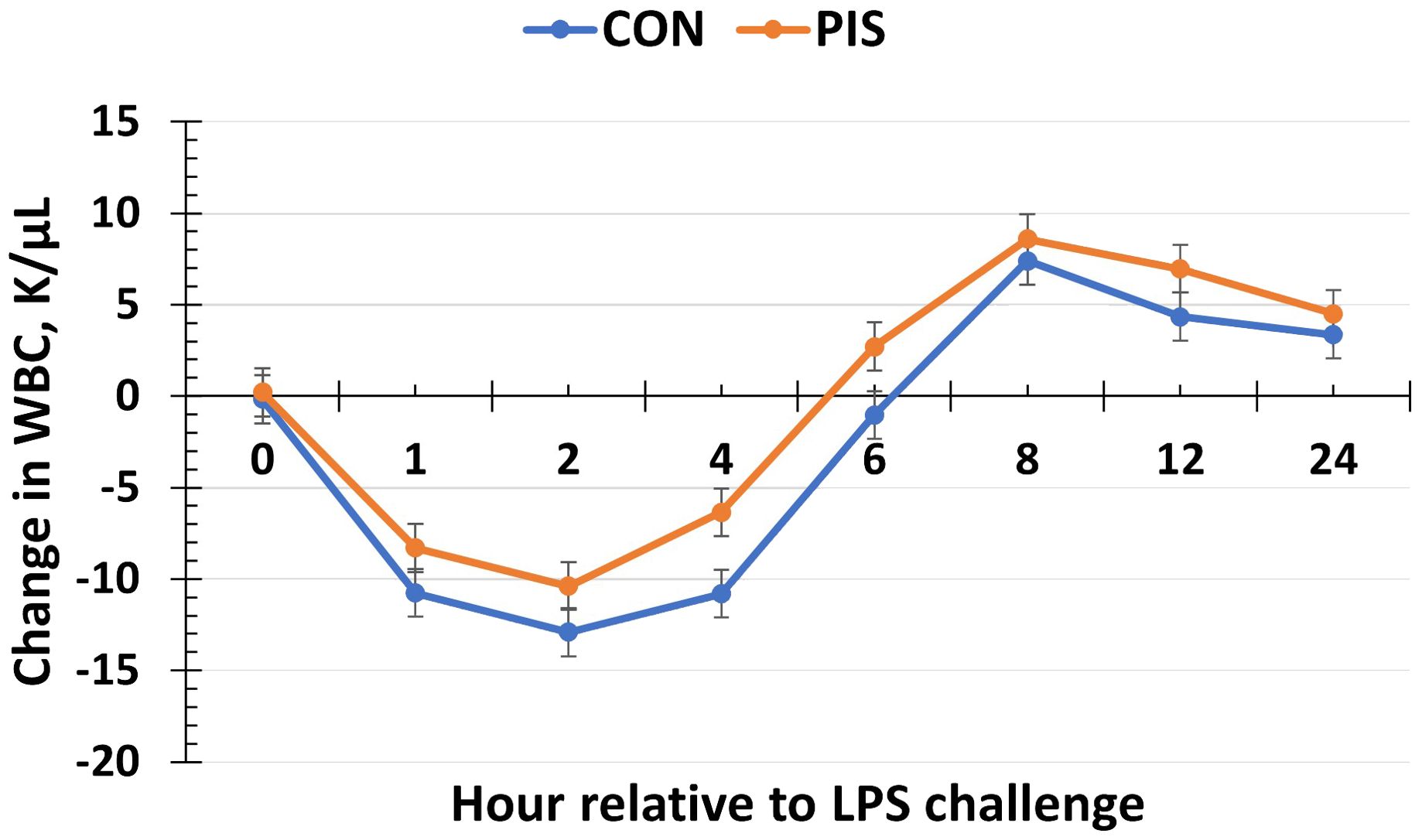
Figure 3. Change in white blood cells (WBC) in piglets in response to a lipopolysaccharide challenge post-weaning. Piglets were selected at weaning from sows previously exposed to low-dose LPS at 78 ± 1.8 days of gestation (PIS) or saline (CON; n = 17 per treatment) and were subsequently challenged with LPS (10 µg/kg body weight i.v.). There was a tendency (P = 0.08) for a treatment effect for the change in white blood cells relative to baseline (−2 and 0 h) values, where there was less change in PIS piglets compared to CON piglets. Data are presented as LSM ± SEM.
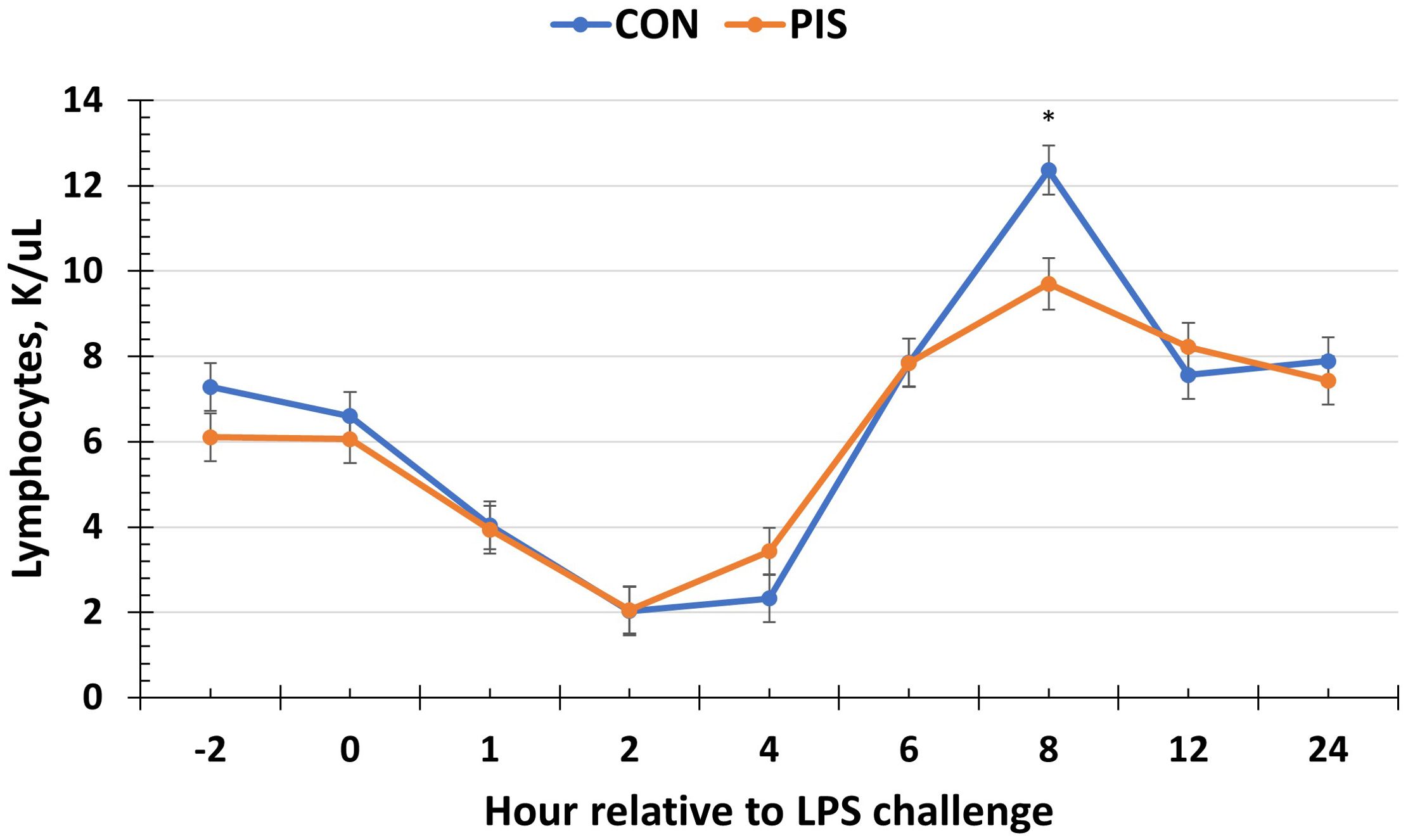
Figure 4. Lymphocyte concentration in piglets in response to a lipopolysaccharide challenge post-weaning. Piglets were selected at weaning from sows previously exposed to low-dose LPS at 78 ± 1.8 days of gestation (PIS) or saline (CON; n = 17 per treatment) and were subsequently challenged with LPS (10 µg/kg body weight i.v.). There was a treatment × time interaction (P = 0.01) for lymphocytes, where PIS piglets had less lymphocytes at 8 h post-challenge compared to CON piglets. Data are presented as LSM ± SEM. The asterisk means that the treatments differ (P < 0.01).
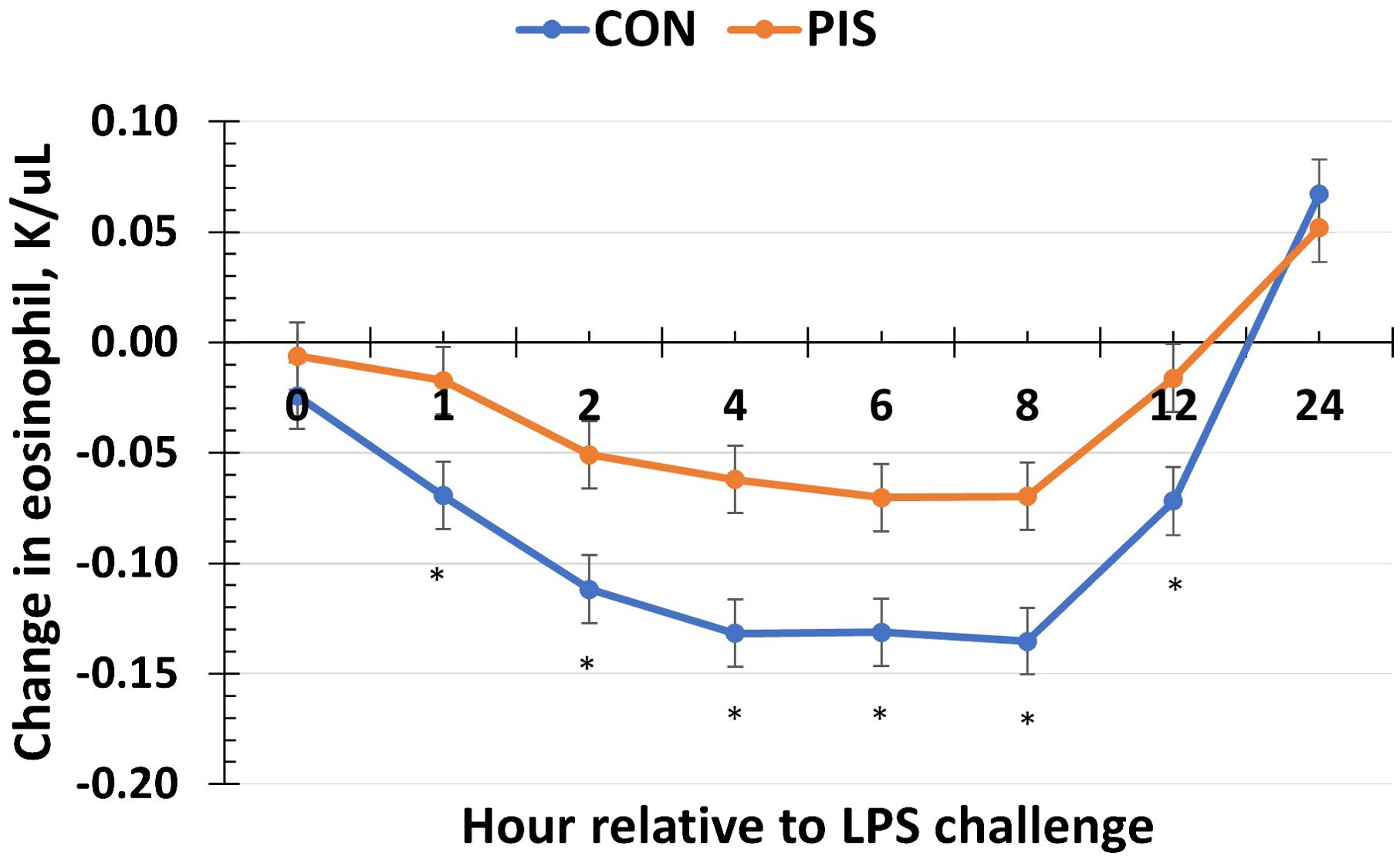
Figure 5. Change in eosinophils in piglets in response to a lipopolysaccharide challenge post-weaning. Piglets were selected at weaning from sows previously exposed to low-dose LPS at 78 ± 1.8 days of gestation (PIS) or saline (CON; n = 17 per treatment) and were subsequently challenged with LPS (10 µg/kg body weight i.v.). There was a treatment × time interaction (P <0.01) for the change in eosinophils relative to baseline (−2 and 0 h) values, where there was less change in PIS piglets compared to CON piglets from 1 to 12 h post-challenge. Data are presented as LSM ± SEM. The asterisk means that the treatments differ (P ≤ 0.02).
3.4 Serum analysis
3.4.1 Cytokines
All cytokines except IL-10 changed over time (P ≤ 0.05; Table 2). There was no treatment nor treatment × time interaction for IL-1β, IL-4, IL-10, IL-12p40p70, IFN-γ, or TGF-β1 (P ≥ 0.13). There was a treatment effect (P = 0.05; Figure 6) for serum concentrations of IL-8, where the concentrations were decreased in PIS compared to CON piglets. There also was a tendency (P = 0.06) for a treatment × time interaction, where the concentrations of IL-8 were reduced in PIS compared to CON piglets from 1 to 4 h post-LPS. Similarly, there was a tendency (P = 0.08; Figure 7) for a treatment × time interaction for concentrations of IL-6, where IL-6 concentrations were reduced in PIS compared to CON pigs from 1 to 4 h post-challenge. There also was a tendency (P = 0.06; Figure 8) for a treatment effect for TNF-α concentrations where PIS piglets tended to have reduced concentrations compared to CON piglets. For serum GM-CSF concentrations, there was a tendency (P = 0.08; Figure 9) for a treatment effect where PIS piglets had greater concentrations compared to CON piglets. Additionally, there was a tendency (P = 0.10) for a treatment × time interaction for serum GM-CSF, where PIS piglets had greater concentrations of GM-CSF than CON piglets from −2 to 0 h relative to LPS administration.
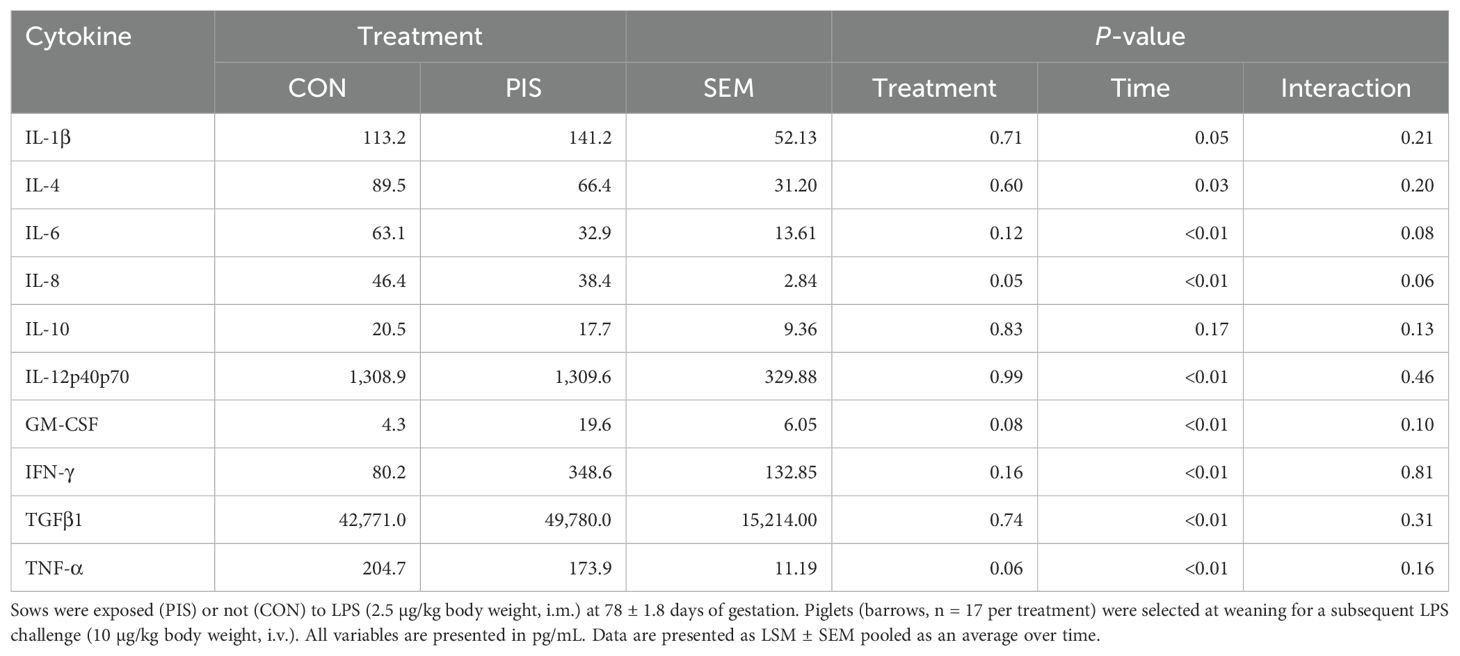
Table 2. Summary of serum cytokines variables in piglets exposed or not to low-dose lipopolysacharide (LPS) during gestation on the subsequent response to LPS post-weaning.
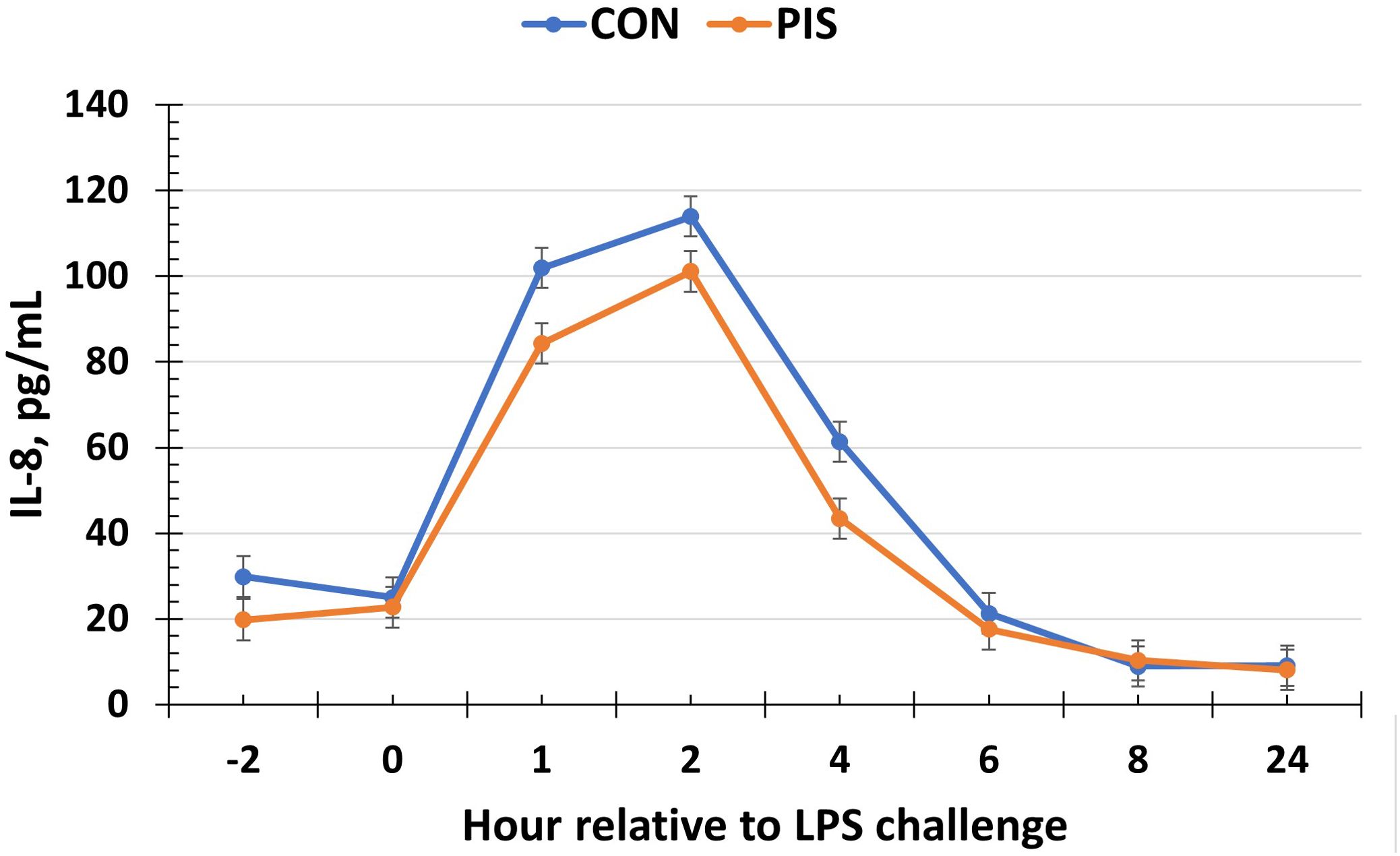
Figure 6. Serum interleukin-8 (IL-8) concentration in piglets in response to a lipopolysaccharide challenge post-weaning. Piglets were selected at weaning from sows previously exposed to low-dose LPS at 78 ± 1.8 days of gestation (PIS) or saline (CON; n = 17 per treatment) and were subsequently challenged with LPS (10 µg/kg body weight i.v.). There was a treatment effect (P = 0.05) for serum IL-8 concentrations where concentrations were reduced in PIS piglets compared to CON piglets. There was also a tendency (P = 0.06) for a treatment × time interaction for serum IL-8 concentrations, where IL-8 concentrations were reduced in PIS piglets from 1 to 4 h post-challenge compared to CON piglets. Data are presented as LSM ± SEM.
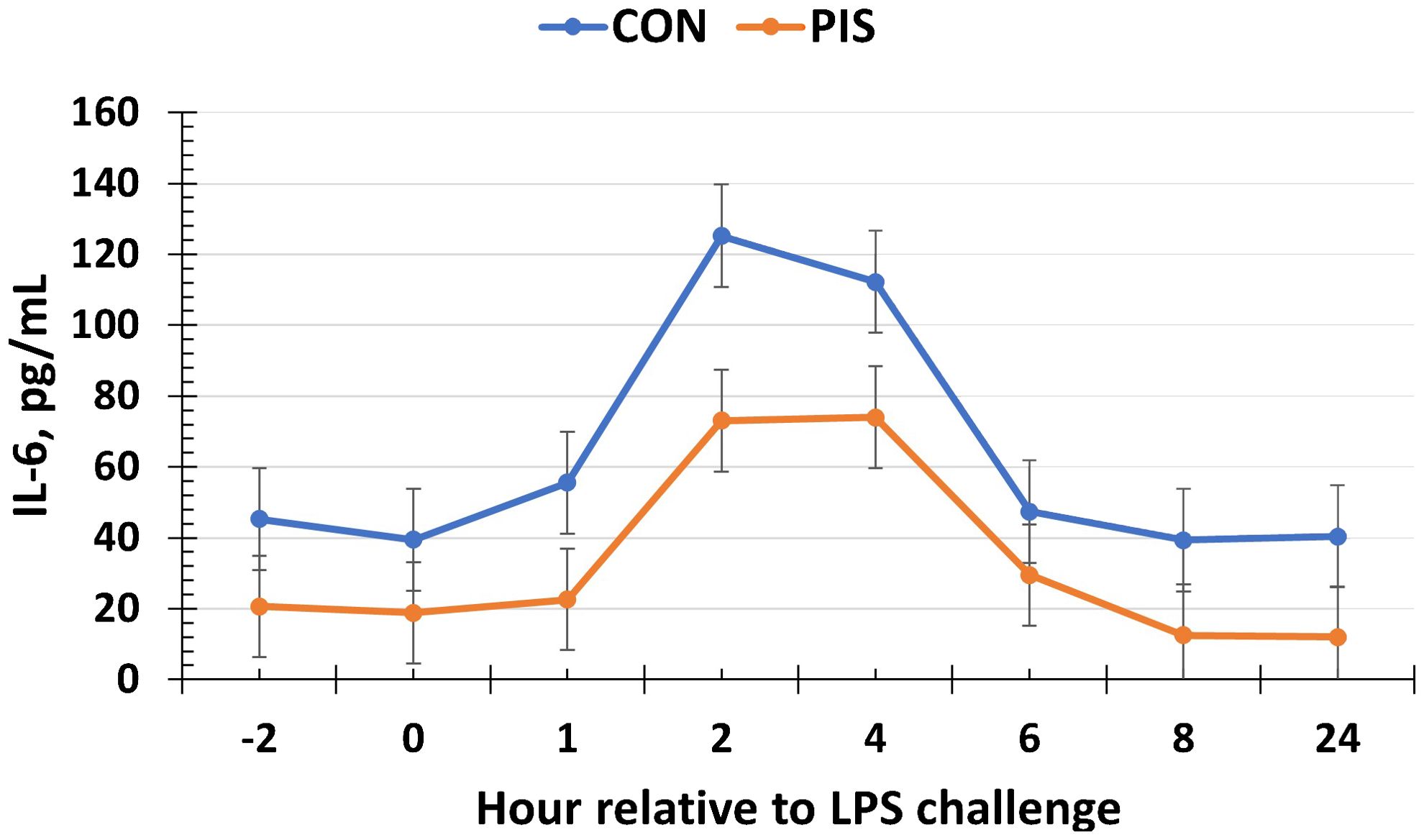
Figure 7. Serum interleukin-6 (IL-6) concentration in piglets in response to a lipopolysaccharide challenge post-weaning. Piglets were selected at weaning from sows previously exposed to low-dose LPS at 78 ± 1.8 days of gestation (PIS) or saline (CON; n = 17 per treatment) and were subsequently challenged with LPS (10 µg/kg body weight i.v.). There was a tendency (P = 0.08) for a treatment × time interaction for serum IL-6 concentrations, where IL-6 concentrations were reduced in PIS piglets from 1 to 4 h post-challenge compared to CON piglets. Data are presented as LSM ± SEM.
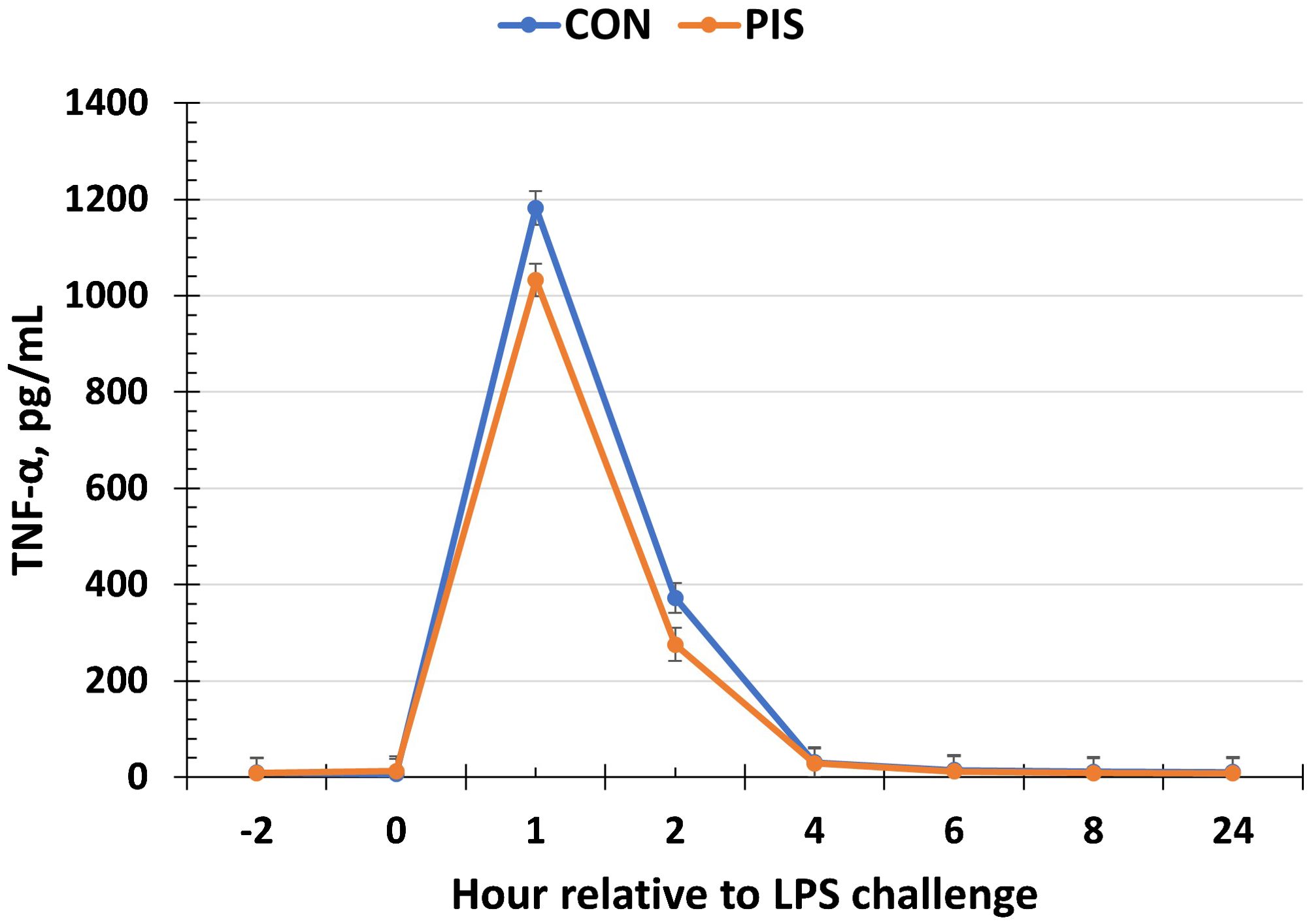
Figure 8. Serum tumor necrosis factor-alpha (TNF-α) concentration in piglets in response to a lipopolysaccharide challenge post-weaning. Piglets were selected at weaning from sows previously exposed to low-dose LPS at 78 ± 1.8 days of gestation (PIS) or saline (CON; n = 17 per treatment) and were subsequently challenged with LPS (10 µg/kg body weight i.v.). There was a tendency (P = 0.06) for a treatment effect for serum TNF-α concentrations, where TNF-α concentrations tended to be reduced in PIS compared to CON piglets. Data are presented as LSM ± SEM.
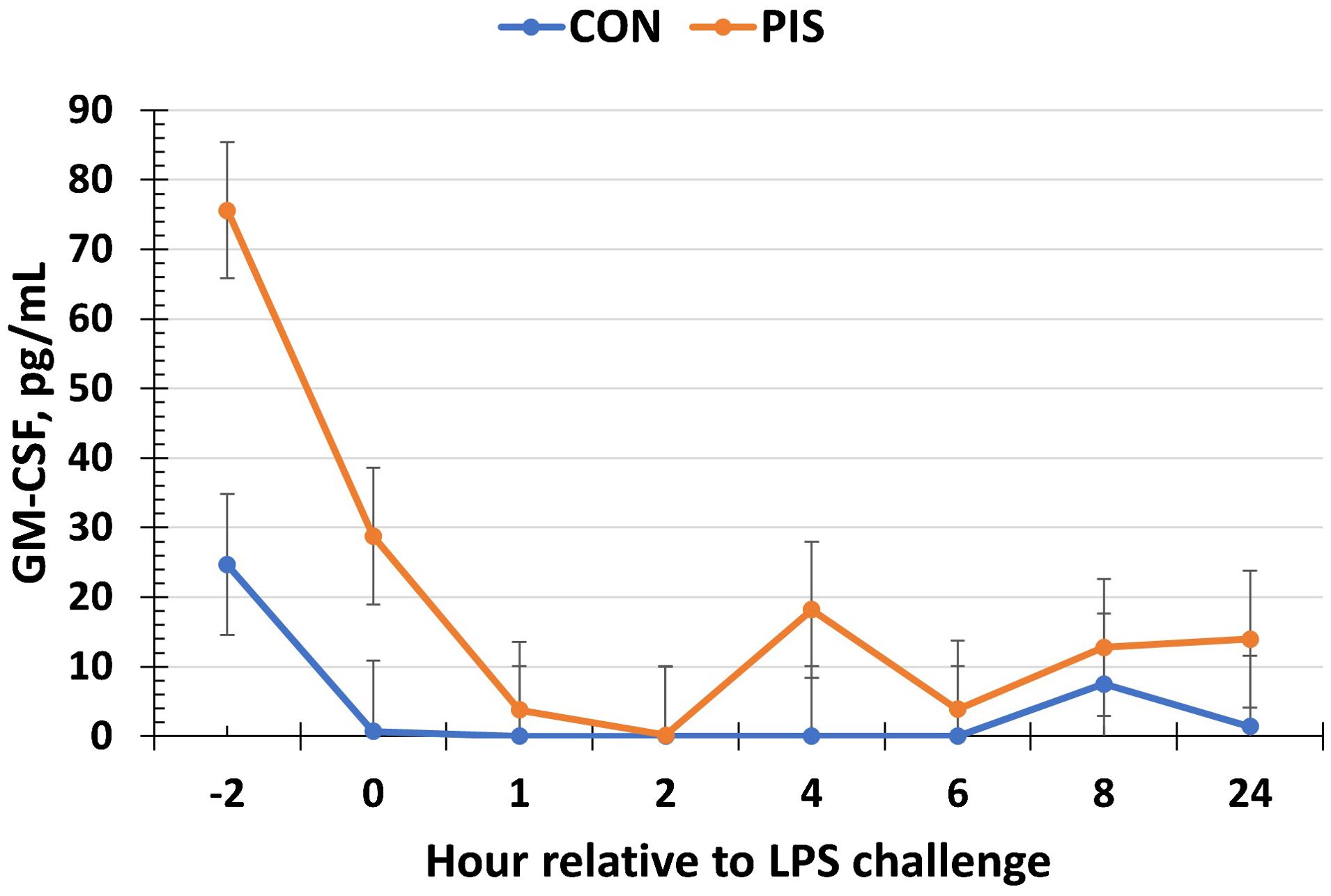
Figure 9. Serum granulocyte macrophage-colony stimulating factor (GM-CSF) concentration in piglets in response to a lipopolysaccharide challenge post-weaning. Piglets were selected at weaning from sows previously exposed to low-dose LPS at 78 ± 1.8 days of gestation (PIS) or saline (CON; n = 17 per treatment) and were subsequently challenged with LPS (10 µg/kg body weight i.v.). There was a tendency (P = 0.08) for a treatment effect for serum GM-CSF, where PIS piglets had greater concentrations of GM-CSF relative to CON piglets. Additionally, there was a tendency (P = 0.10) for a treatment × time interaction for serum GM-CSF, where PIS piglets had greater concentrations from −2 to 0 h relative to CON piglets. Data are presented as LSM ± SEM.
3.4.2 Cortisol, glucose, and lipopolysaccharide binding protein
The serum concentrations of cortisol and glucose increased (P < 0.01) in response to the LPS challenge in piglets post-weaning but were not affected by treatment or a treatment × time interaction (P ≥ 0.45; data not shown). Specifically, the serum cortisol concentrations increased within 1 h post-LPS, peaked at 2 h post-LPS, and decreased until baseline values were reached at 8 h post-LPS. The serum glucose concentrations peaked at 1 h post-LPS before returning to baseline values (time: P < 0.01), but there was no treatment (P = 0.98) nor treatment × time interaction (P = 0.45; data not shown). Similarly, there was no effect of treatment nor treatment × time interaction (P ≥ 0.21) for serum LBP concentrations, although there was a tendency (P = 0.10) for a change over time (Figure 10).
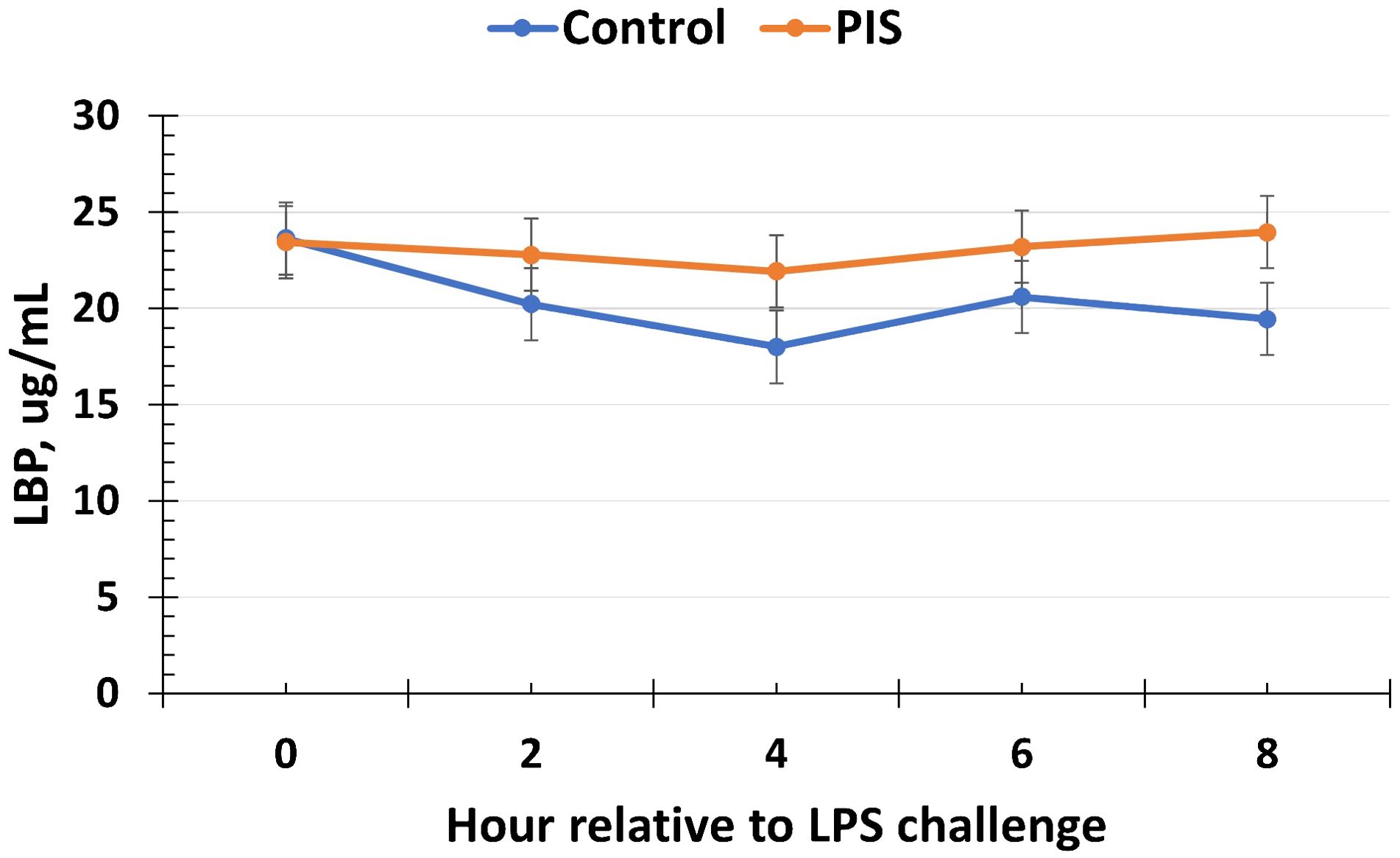
Figure 10. Serum lipopolysaccharide binding protein (LBP) concentration in piglets in response to a lipopolysaccharide challenge post-weaning. Piglets were selected at weaning from sows previously exposed to low-dose LPS at 78 ± 1.8 days of gestation (PIS) or saline (CON; n = 17 per treatment) and were subsequently challenged with LPS (10 µg/kg body weight i.v.). The concentrations of LBP were not affected by treatment or by a treatment × time interaction (P ≥ 0.21). However, there was a tendency (P = 0.10) for the concentrations of LBP to change over time. Data are presented as LSM ± SEM.
4 Discussion
There is limited information on the effect of an LPS challenge in sows during gestation and the impact on the subsequent immune response of offspring. Indeed many of the studies where LPS was administered to sows occurred very close to expected parturition dates (You et al., 2019; Zou et al., 2020), or piglets were retrieved by caesarean section within days following LPS administration (Cilieborg et al., 2011; Muk et al., 2020). Thus, the current study is one of the first studies to demonstrate the impact of low-dose LPS administration on the inflammatory response in both pregnant sows early in the last third of gestation and the subsequent effects of LPS administration on the inflammatory response in piglets. The administration of LPS closer to parturition as in the study by You et al. (2019) suggests that this induced more of a stress response rather than an immune response in the sow and piglets, as the dose administered to the sows was much larger than the dose used on sows in the current study (10 versus 2.5 µg/kg body weight). The purpose of the current study was not to induce stress but to induce mild and acute immune stimulation using a low dose of LPS to better prepare the offspring for subsequent perinatal life.
The sow LPS dose used in the current study was chosen based on a previous work in cattle, where pregnant cows were administered a subcutaneous LPS dose of 0.1 µg/kg BW compared to the dose of 0.5 µg/kg that was subsequently administered to weaned calves (Burdick Sanchez et al., 2017; Carroll et al., 2017). In this aforementioned study, the vaginal temperature of cows increased by 0.5°C, on average, for approximately 7 h. In the current study, the 2.5-µg/kg BW dose of LPS was chosen for the sows based on a preliminary study which compared the doses of 0, 2.5, and 5 µg/kg BW LPS in non-pregnant sows. The selected dose of LPS resulted in an acute temperature and sickness behavior response in the sows which returned to baseline values within 24 and 7.5 h, respectively (unpublished data). The increase in rectal temperature in the sows administered LPS verifies acute immune stimulation, with temperature values staying below the fever threshold throughout the challenge period and returning to baseline values within 16 to 24 h.
The timing of immune challenges during gestation is important. Immunocompetence is established in pigs at approximately 70 days of gestation (Veru et al., 2014; Matias et al., 2017). The average gestational date for LPS administration in sows in the current study was gestational day 78, which places it within the period where piglets should be responsive to immune challenges in utero. Based on a rodent model, LPS does not appear to cross the placental barrier (Parant and Chedid, 1964; Sinkora and Butler, 2009), and thus it cannot have a direct effect on the fetus. Yet, it is unclear what disruptions acute inflammation or infection has on placental barriers (Sinkora and Butler, 2009) and how changes in other variables in the sow, such as body temperature, cortisol, leukocytes, and cytokines (e.g., secondary effects), may influence the fetus. For example, LPS administration in late gestation in mice led to an observation of an increase in concentrations of pro-inflammatory cytokines in the placenta and uterus (Liu et al., 2018), providing an avenue for further research in the pig model. Interestingly, work in humans and rodents has found that LPS administration may result in preeclampsia due to placental inflammation, which may affect the inflammatory response of the offspring (Guillemette et al., 2015; Gatford et al., 2020; Michalczyk et al., 2020). However, these species have a more invasive placentation, and it is unclear what direct effects LPS has in the gestating sow. More information on the inflammatory response in the sow and fetus in response to LPS is needed to further characterize any direct or indirect (e.g., secondary) effects of prenatal LPS on the fetus.
Piglets exposed to LPS during gestation responded more quickly with an increase in body temperature compared to CON piglets. However, there was no difference in the temperature response following the peak in body temperature at 2 h after LPS administration. Similarly, gilts exposed to LPS during gestation at day 111 had greater rectal temperature 1 h following a post-weaning LPS challenge than piglets from sows administered saline (You et al., 2019). It is possible that the greater temperature response in PIS pigs is due to quicker recognition of LPS. However, there were no treatment differences in LBP, a major acute phase protein involved in the recognition of LPS (Meng et al., 2021). Yet, it is possible that there were differences in other cellular components involved in the recognition of LPS (e.g., CD14). Piglets whose dams were exposed to cortisol during gestation and were subsequently challenged with LPS at 8 weeks of age produced a greater temperature response to LPS than piglets from control dams (de Groot et al., 2007). This is supportive of potential secondary effects of LPS administration on the offspring in utero, as LPS is known to cause an increase in cortisol concentrations, which may have an effect on the offspring, as will be discussed in greater detail later.
Total white blood cell concentrations tended to differ, and eosinophil concentrations differed prior to the LPS challenge, suggesting an effect of prenatal LPS exposure on circulating basal white blood cells. It is typical for LPS to induce a decrease in circulating white blood cells within 30 to 120 min following administration (Burdick Sanchez et al., 2018; Liu et al., 2019), as leukocytes leave the circulation in search of the infection. Considering the baseline treatment differences, the decrease in total white blood cells and eosinophils was less in pigs exposed to prenatal LPS than those exposed to saline, suggesting that the pigs from sows administered with LPS during gestation were less responsive or more tolerant to the challenge than CON pigs. The peak in lymphocytes in CON pigs at 8 h post-challenge is unusual and may not be of biological significance as the concentrations returned to baseline at 12 and 24 h.
Cytokines released by leukocytes and endothelial cells help drive the direction of the inflammatory response to LPS, which is also shaped by increasing concentrations of cortisol. Overall, the pro-inflammatory response appeared to be reduced in PIS pigs compared to CON pigs. The tendencies for decreased TNF-α and IL-6 in PIS pigs are intriguing, as these two cytokines are well known to play a role in the induction of fever to LPS (Dantzer, 2001). It is likely that other factors outside of these cytokines are responsible for the quicker temperature response in PIS pigs. Work in rats observed increased concentrations of pro-inflammatory cytokines in the amniotic fluid after pregnant rats were administered 100 µg/kg BW of LPS on day 18 of pregnancy as well as changes in pro-inflammatory gene expression in the fetal pup brain (Gayle et al., 2004). Furthermore, rodents exposed to high concentrations of LPS during gestion were observed to have reduced cytokine concentrations after birth (Lasala and Zhou, 2007; Beloosesky et al., 2010). However, rodents have a more invasive placentation than pigs (hemochorial versus epitheliochorial, respectively), and therefore this response may not be observed in pigs. In support, the administration of LPS to pregnant ewes, who have similar placentation to pigs, increased maternal IL-6 concentrations but did not elicit an increase in IL-6 concentrations in fetal lambs (Grigsby et al., 2003). Similarly, McClure et al. (2005) reported increases in maternal concentrations of TNF-α and IL-6 concentrations following systemic LPS administration, but with no concurrent increases in fetal concentrations of these cytokines in fetal sheep. These studies demonstrate the protection of the fetus from the maternal response to LPS in species with a less-invasive placenta.
Previous exposure to LPS has been observed to alter the cytokine response to a second exposure. Exposure of pig CD14+ spleen cells to a low dose of LPS and subsequent re-exposure resulted in a reduction in TNF-α and IL-8 concentrations, suggestive of tolerance (Cagiola et al., 2006). Additionally, pre-treatment of human monocytes with LPS from Salmonella enteritidis decreased TNF-α production to a second exposure, although IL-6 concentrations were decreased and IL-8 and GM-CSF concentrations were unaffected (Peck et al., 2004). Lastly, repeated LPS administration to lambs 5 days apart resulted in an attenuated TNF-α and IL-6 response suggestive of endotoxin tolerance (McClure et al., 2005). Thus, it is possible that the LPS challenge experienced by the pigs in utero resulted in a tolerogenic response to a second LPS challenge after birth.
In contrast to the other pro-inflammatory cytokines, GM-CSF was threefold greater in PIS pigs than CON prior to the administration of LPS. The concentrations of GM-CSF decreased during the pre-challenge period and remained low for the duration of the study. Studies suggest that increases in GM-CSF lead to increased or enhanced pro-inflammatory cytokine production by stimulated macrophages (Brissette et al., 1995; Kreutz et al., 1999); however, this was not observed in the current study. Therefore, the role of elevated GM-CSF prior to the LPS challenge is an area that requires additional study.
Interestingly, no difference in serum cortisol concentration was observed between PIS and CON piglets throughout the challenge period. Walker et al. (2006) also reported no differences in corticosterone production in male rats exposed to LPS during gestation during subsequent LPS administration as an adult. In contrast, administration of LPS to sows on day 112 of pregnancy resulted in a greater cortisol response to a subsequent LPS challenge in male offspring (You et al., 2019). Additionally, female sheep exposed to LPS once during late gestation (day 135 of the 145-day gestation length) had a greater cortisol response than control lambs, while lambs exposed to a lower dose of LPS over three consecutive days (days 135–137) had a reduced cortisol response (Fisher et al., 2010). However, this response was sex-specific, such that it was observed in female lambs but not in male lambs. Therefore, the timing of LPS during gestation appears to influence the subsequent cortisol response in the offspring. Furthermore, work in sheep found that pregnant ewes produced a heightened cortisol response to LPS administration compared with non-pregnant ewes (Kabaroff et al., 2006), suggesting a greater hypothalamic–pituitary–adrenal (HPA) axis activation during that stage of pregnancy. Grigsby et al. (2003) also reported that cortisol increased in both the ewe and the fetal lamb 2 h after intravenous LPS administration to the ewe and remained elevated through 24 h post-LPS administration. Thus, it is possible that some of the differences observed in the piglets were due to changes in prenatal cortisol concentrations or other factors resulting from LPS administration during gestation.
As discussed, one possible explanation for the differences observed in the immune response of PIS piglets is through secondary effects, such as changes in other stress, immune, or metabolic variables in the sow that affected the offspring. For example, changes in nutrient availability (e.g., glucose) in the sow during exposure to LPS may affect the fetuses, as it is well known that the immune system requires a significant amount of energy to respond to a threat (Battaglia and Meschia, 1978; Serriere et al., 2011; Kvidera et al., 2017). Thus, it is possible that reductions in glucose availability or changes in other nutrients due to fever and inflammation may have altered how PIS pigs responded to LPS in the postnatal period. In support of this are the changes observed in postnatal immune responsiveness of offspring when exposed to nutritional changes in utero (Tuchscherer et al., 2012). While changes in cortisol and glucose were not measured in the sows following LPS administration, interestingly, there were no treatment differences in the piglet glucose response to the LPS challenge. Future studies should aim to measure stress and immune variables in the sow in response to low-dose LPS administration.
It is unclear if any protective effects may be transferred to piglets from the sow through the colostrum and whether the response observed is protection via passive transfer of immunity. In addition to containing immunoglobulins, colostrum also contains leukocytes that help provide immune protection to the piglets during the neonatal period. In fact, there is an increase in recruitment of leukocytes to the mammary gland in sows near the 80th day of pregnancy (Matias et al., 2017). Therefore, if antibodies and leukocytes specific for the LPS administered to the sow were transferred to the piglet after birth, this may partially explain the reduced immune response observed. The measurement of E. coli-specific antibodies in the serum of piglets from sows immunized with E. coli supports the role of colostral antibody transfer and protection (Poonsuk and Zimmerman, 2018). While colostrum was not collected and the serum antibodies against the LPS used in this study were not analyzed, this is an avenue for further study in subsequent experiments.
5 Conclusions
In summary, prenatal exposure to LPS altered the postnatal innate immune response to LPS in weaned pigs. This resulted in a quicker increase in subcutaneous body temperature accompanied by reduced change in leukocyte populations and concentrations of pro-inflammatory cytokines. However, there were no changes observed in cortisol, glucose, or LBP concentrations. These data suggest that exposure to endotoxin in utero may influence the postnatal innate immune response to a subsequent endotoxin challenge. Furthermore, this study suggests that it is possible to influence the innate immune response of pigs in utero, which may serve as a method to positively influence pig postnatal immune responsiveness. However, more research must be completed to elucidate the mechanisms driving the differences observed in the current study. Additionally, studies must also investigate how the differences in immune responses observed may influence and impact long-term health outcomes and productivity in pig offspring.
Data availability statement
The raw data supporting the conclusions of this article will be made available by the authors, without undue reservation.
Ethics statement
The animal study was approved by Texas Tech University Institutional Animal Care and Use Committee; USDA-ARS Livestock Issues Research Unit Institutional Animal Care and Use Committee. The study was conducted in accordance with the local legislation and institutional requirements.
Author contributions
NB: Conceptualization, Formal Analysis, Investigation, Writing – original draft, Writing – review & editing. TM: Investigation, Writing – review & editing. PB: Investigation, Writing – review & editing. BB: Investigation, Writing – review & editing. ED: Investigation, Writing – review & editing. TD: Investigation, Writing – review & editing. SB: Investigation, Writing – review & editing. JL: Conceptualization, Investigation, Writing – review & editing. AP: Conceptualization, Investigation, Writing – review & editing. JC: Conceptualization, Investigation, Writing – review & editing.
Funding
The author(s) declare that no financial support was received for the research, authorship, and/or publication of this article.
Acknowledgments
The data presented is an expansion of data previously presented in abstract form (Burdick Sanchez NC, Mitchell T, Broadway PR, Bowen BM, Davis EM, Dobbins T, Barker SN, Legako JF, Petry AL, and Carroll. J. Anim Sci. (2023) 101(Suppl. 3):26). The authors would like to thank J.R. Carroll (USDA-ARS) for their excellent technical support.
Conflict of interest
The authors declare that the research was conducted in the absence of any commercial or financial relationships that could be construed as a potential conflict of interest.
Publisher’s note
All claims expressed in this article are solely those of the authors and do not necessarily represent those of their affiliated organizations, or those of the publisher, the editors and the reviewers. Any product that may be evaluated in this article, or claim that may be made by its manufacturer, is not guaranteed or endorsed by the publisher.
Author disclaimer
Mention of trade names or commercial products in this article is solely for the purpose of providing specific information and does not imply recommendation or endorsement by the US Department of Agriculture. USDA is an equal opportunity provider and employer.
References
Battaglia F. C., Meschia G. (1978). Principal substrates of fetal metabolism. Physiol. Rev. 58, 499–527. doi: 10.1152/physrev.1978.58.2.499
Beloosesky R., Maravi N., Weiner Z., Khatib N., Awad N., Boles J., et al. (2010). Maternal lipopolysaccharide-induced inflammation during pregnancy programs impaired offspring innate immune responses. Am. J. Obstet Gynecol 203, 185 e181–184. doi: 10.1016/j.ajog.2010.04.033
Brissette W. H., Baker D. A., Stam E. J., Umland J. P., Griffiths R. J. (1995). GM-CSF rapidly primes mice for enhanced cytokine production in response to LPS and TNF. Cytokine 7, 291–295. doi: 10.1006/cyto.1995.0035
Burdick Sanchez N. C., Carroll J. A., Arthingon J. D., Lancaster P. A. (2017). Exposure to lipopolysaccharide in utero alters the postnatal metabolic response in heifers. J. Anim. Sci. 95, 5176–5183. doi: 10.2527/jas2016.0885
Burdick Sanchez N. C., Carroll J. A., Broadway P. R., Bass B. E., Frank J. W. (2018). Modulation of the acute phase response following a lipopolysaccharide challenge in pigs supplemented with an all-natural Saccharomyces cerevisiae fermentation product. Livest Sci. 208, 1–4. doi: 10.1016/j.livsci.2017.11.022
Burdick Sanchez N. C., Carroll J. A., Broadway P. R., Hughes H. D., Roberts S. L., Richeson J. T., et al. (2016). Cattle temperament influences metabolism: metabolic response to glucose tolerance and insulin sensitivity tests in beef steers. Domest Anim. Endocrinol. 56, 85–95. doi: 10.1016/j.domaniend.2016.02.009
Burdick Sanchez N. C., Dailey J. W., Broadway P. R., Davis E. M., Bowen B. M., Petry A. L., et al. (2023). A viable less-invasive alternative for continuous temperature measurement in weaned pigs. Livest Sci. 267, 105126. doi: 10.1016/j.livsci.2022.105126
Cagiola M., Giulio S., Miriam M., Katia F., Paola P., Macri A., et al. (2006). In vitro down regulation of proinflammatory cytokines induced by LPS tolerance in pig CD14+ cells. Vet. Immunol. Immunopathol. 112, 316–320. doi: 10.1016/j.vetimm.2006.04.002
Carroll J. A., Burdick Sanchez N. C., Arthingon J. D., Nelson C. D., Benjamin A. L., Korkmaz F. T., et al. (2017). In utero exposure to LPS alters the postnatal acute-phase response in beef heifers. Innate Immun. 23, 97–108. doi: 10.1177/1753425916678472
Carroll J. A., Burdick Sanchez N. C., Broadway P. R., Silva G. M., Ranches J., Warren J., et al. (2021). Prenatal immune stimulation alters the postnatal acute phase and metabolic responses to an endotoxin challenge in weaned beef heifers. Transl. Anim. Sci. 5, txab097. doi: 10.1093/tas/txab097
Carroll J. A., Daniel J. A., Keisler D. H., Matteri R. L. (1999). Non-surgical catheterization of the jugular vein in young pigs. Lab. Anim. 33, 129–134. doi: 10.1258/002367799780578345
Cilieborg M. S., Schmidt M., Skovgaard K., Boye M., Weber N. R., Heegaard P. M., et al. (2011). Fetal lipopolysaccharide exposure modulates diet-dependent gut maturation and sensitivity to necrotising enterocolitis in pre-term pigs. Br. J. Nutr. 106, 852–861. doi: 10.1017/S000711451100047X
Dantzer R. (2001). Cytokine-induced sickness behavior: where do we stand? Brain Behav. Immun. 15, 7–24. doi: 10.1006/brbi.2000.0613
de Groot J., Kranendonk G., Fillerup M., Hopster H., Boersma W., Hodgson D., et al. (2007). Response to LPS in female offspring from sows treated with cortisol during pregnancy. Physiol. Behav. 90, 612–618. doi: 10.1016/j.physbeh.2006.11.013
Fisher R. E., Karrow N. A., Quinton M., Finegan E. J., Miller S. P., Atkinson J. L., et al. (2010). Endotoxin exposure during late pregnancy alters ovine offspring febrile and hypothalamic-pituitary-adrenal axis responsiveness later in life. Stress 13, 334–342. doi: 10.3109/10253891003663762
Flori L., Gao Y., Laloe D., Lemonnier G., Leplat J. J., Teillaud A., et al. (2011). Immunity traits in pigs: substantial genetic variation and limited covariation. PloS One 6, e22717. doi: 10.1371/journal.pone.0022717
Gatford K. L., Andraweera P. H., Roberts C. T., Care A. S. (2020). Animal models of preeclampsia: causes, consequences, and interventions. Hypertension 75, 1363–1381. doi: 10.1161/HYPERTENSIONAHA.119.14598
Gayle D. A., Beloosesky R., Desai M., Fataneh A., Nunez S. E., Ross M. G. (2004). Maternal LPS induces cytokines in the amniotic fluid and corticotropin releasing hormone in the fetal brain. Am. J. Physiol. Regul. Integr. Comp. Physiol. 286, R1024–R1029. doi: 10.1152/ajpregu.00664.2003
Grigsby P. L., Hirst J. J., Scheerlinck J. P., Phillips D. J., Jenkin G. (2003). Fetal responses to maternal and intra-amniotic lipopolysaccharide administration in sheep. Biol. Reprod. 68, 1695–1702. doi: 10.1095/biolreprod.102.009688
Guillemette L., Lacroix M., Allard C., Patenaude J., Battista M. C., Doyon M., et al. (2015). Preeclampsia is associated with an increased pro-inflammatory profile in newborns. J. Reprod. Immunol. 112, 111–114. doi: 10.1016/j.jri.2015.09.003
Kabaroff L., Boermans H., Karrow N. A. (2006). Changes in ovine maternal temperature, and serum cortisol and interleukin-6 concentrations after challenge with Escherichia coli lipopolysaccharide during pregnancy and early lactation. J. Anim. Sci. 84, 2083–2088. doi: 10.2527/jas.2005-625
Kreutz M., Hennemann B., Ackermann U., Grage-Griebenow E., Krause S. W., Andreesen R. (1999). Granulocyte-macrophage colony-stimulating factor modulates lipopolysaccharide (LPS)-binding and LPS-response of human macrophages: inverse regulation of tumour necrosis factor-alpha and interleukin-10. Immunology 98, 491–496. doi: 10.1046/j.1365-2567.1999.00904.x
Kvidera S. K., Horst E. A., Mayorga E. J., Sanz-Fernandez M. V., Abuajamieh M., Baumgard L. H. (2017). Estimating glucose requirements of an activated immune system in growing pigs. J. Anim. Sci. 95, 5020–5029. doi: 10.2527/jas2017.1830
Langel S. N., Wang Q., Vlasova A. N., Saif L. J. (2020). Host factors affecting generation of immunity against porcine epidemic diarrhea virus in pregnant and lactating swine and passive protection of neonates. Pathogens 9, 130. doi: 10.3390/pathogens9020130
Lasala N., Zhou H. (2007). Effects of maternal exposure to LPS on the inflammatory response in the offspring. J. Neuroimmunol 189, 95–101. doi: 10.1016/j.jneuroim.2007.07.010
Liu Y., Choe J., Lee J. J., Kim J., Campbell J. M., Polo J., et al. (2018). Spray-dried plasma attenuates inflammation and lethargic behaviors of pregnant mice caused by lipopolysaccharide. PloS One 13, e0203427. doi: 10.1371/journal.pone.0203427
Liu H., Feye K. M., Nguyen Y. T., Rakhshandeh A., Loving C. L., Dekkers J. C. M., et al. (2019). Acute systemic inflammatory response to lipopolysaccharide stimulation in pigs divergently selected for residual feed intake. BMC Genomics 20, 728. doi: 10.1186/s12864-019-6127-x
Matias J., Berzosa M., Pastor Y., Irache J. M., Gamazo C. (2017). Maternal Vaccination. Immunization of Sows during Pregnancy against ETEC Infections. Vaccines 5, 48. doi: 10.3390/vaccines5040048
McClure L., O’Connor A. E., Hayward S., Jenkin G., Walker D. W., Phillips D. J. (2005). Effects of age and pregnancy on the circulatory activin response of sheep to acute inflammatory challenge by lipopolysaccharide. J. Endocrinol. 185, 139–149. doi: 10.1677/joe.1.06051
Meng L., Song Z., Liu A., Dahmen U., Yang X., Fang H. (2021). Effects of lipopolysaccharide-binding protein (LBP) single nucleotide polymorphism (SNP) in infections, inflammatory diseases, metabolic disorders and cancers. Front. Immunol. 12. doi: 10.3389/fimmu.2021.681810
Michalczyk M., Celewicz A., Celewicz M., Wozniakowska-Gondek P., Rzepka R. (2020). The role of inflammation in the pathogenesis of preeclampsia. Mediators Inflammation 2020, 3864941. doi: 10.1155/2020/3864941
Muk T., Jiang P. P., Stensballe A., Skovgaard K., Sangild P. T., Nguyen D. N. (2020). Prenatal endotoxin exposure induces fetal and neonatal renal inflammation via innate and th1 immune activation in preterm pigs. Front. Immunol. 11. doi: 10.3389/fimmu.2020.565484
Parant M., Chedid L. (1964). Protective effect of chlorpromazine against endotoxin-induced abortion. P Soc. Exp. Biol. Med. 116, 906–909. doi: 10.3181/00379727-116-29404
Peck O. M., Williams D. L., Breuel K. F., Kalbfleisch J. H., Fan H., Tempel G. E., et al. (2004). Differential regulation of cytokine and chemokine production in lipopolysaccharide-induced tolerance and priming. Cytokine 26, 202–208. doi: 10.1016/j.cyto.2004.02.011
Poonsuk K., Zimmerman J. (2018). Historical and contemporary aspects of maternal immunity in swine. Anim. Health Res. reviews/Conference Res. Workers Anim. Dis. 19, 31–45. doi: 10.1017/S1466252317000123
Prunier A., Heinonen M., Quesnel H. (2010). High physiological demands in intensively raised pigs: impact on health and welfare. Animal 4, 886–898. doi: 10.1017/S175173111000008X
Salmon H., Berri M., Gerdts V., Meurens F. (2009). Humoral and cellular factors of maternal immunity in swine. Dev. Comp. Immunol. 33, 384–393. doi: 10.1016/j.dci.2008.07.007
Serriere S., Barantin L., Seguin F., Tranquart F., Nadal-Desbarats L. (2011). Impact of prenatal stress on 1H NMR-based metabolic profiling of rat amniotic fluid. MAGMA 24, 267–275. doi: 10.1007/s10334-011-0260-0
Sinkora M., Butler J. E. (2009). The ontogeny of the porcine immune system. Dev. Comp. Immunol. 33, 273–283. doi: 10.1016/j.dci.2008.07.011
Tuchscherer M., Otten W., Kanitz E., Grabner M., Tuchscherer A., Bellmann O., et al. (2012). Effects of inadequate maternal dietary protein:carbohydrate ratios during pregnancy on offspring immunity in pigs. BMC Vet. Res. 8, 232. doi: 10.1186/1746-6148-8-232
Veru F., Laplante D. P., Luheshi G., King S. (2014). Prenatal maternal stress exposure and immune function in the offspring. Stress 17, 133–148. doi: 10.3109/10253890.2013.876404
Walker F. R., Hodyl N. A., Krivanek K. M., Hodgson D. M. (2006). Early life host-bacteria relations and development: long-term individual differences in neuroimmune function following neonatal endotoxin challenge. Physiol. Behav. 87, 126–134. doi: 10.1016/j.physbeh.2005.09.008
You L., Lee A. V., Oh S. Y., Fisher-Heffernan R. E., Edwards M., De Lange K., et al. (2019). Effect of lipopolysaccharide-induced immune stimulation and maternal fish oil and microalgae supplementation during late pregnancy on nursery pig hypothalamic-pituitary-adrenal function. J. Anim. Sci. 97, 2940–2951. doi: 10.1093/jas/skz166
Keywords: acute phase response, cytokine, gestation, innate immunity, lipopolysaccharide, pig, prenatal immune stimulation
Citation: Burdick Sanchez NC, Mitchell T, Broadway PR, Bowen BM, Davis EM, Dobbins T, Barker SN, Legako JF, Petry AL and Carroll JA (2024) Evaluation of the use of prenatal immune stimulation to alter postnatal immune function in weaned pigs. Front. Anim. Sci. 5:1418557. doi: 10.3389/fanim.2024.1418557
Received: 16 April 2024; Accepted: 13 August 2024;
Published: 05 September 2024.
Edited by:
Ignacio R. Ipharraguerre, University of Kiel, GermanyReviewed by:
Lautaro Rostoll Cangiano, University of Wisconsin-Madison, United StatesJavier Polo, APC Europe S.L., Spain
Copyright At least a portion of this work is authored by Nicole C. Burdick Sanchez, Paul R. Broadway, and Jeffery A. Carroll on behalf of the U.S. Government and as regards Dr. Burdick Sanchez, Dr. Broadway, Dr. Carroll, and the U.S. Government, is not subject to copyright protection in the United States. Foreign and other copyrights may apply. This is an open-access article distributed under the terms of the Creative Commons Attribution License (CC BY). The use, distribution or reproduction in other forums is permitted, provided the original author(s) and the copyright owner(s) are credited and that the original publication in this journal is cited, in accordance with accepted academic practice. No use, distribution or reproduction is permitted which does not comply with these terms.
*Correspondence: Nicole C. Burdick Sanchez, bmljb2xlLnNhbmNoZXpAdXNkYS5nb3Y=