- 1Department of Animal Science, University of California, Davis, Davis, CA, United States
- 2Agriculture and Agri-Food Canada, Lacombe Research and Development Centre, Lacombe, AB, Canada
- 3Department of Animal Sciences, Stellenbosch University, Cape Town, South Africa
Recent government bans on industrial trans fatty acids (TFA) in developed countries has left naturally occurring TFA from ruminant products (e.g., dairy, beef, and lamb) as the sole source of TFA in the food supply. In contrast to industrial TFA, which have undisputed adverse health effects, ruminant TFA such as trans vaccenic acid (TVA; trans11-18:1), rumenic acid (RA; cis9, trans11-18:2) and trans palmitoleic acid (TPA; trans9-16:1) have been associated with reduced risk for some diseases such as type 2 diabetes. The present review summarizes the findings from observational, animal and human studies investigating the effects of ruminant TFA on metabolic parameters related to type 2 diabetes, and provides an update on the current knowledge of their biosynthesis, intake and factors affecting their concentrations in ruminant derived foods. Overall, observational studies and a small number of animal studies suggest that ruminant TFA may be protective against type 2 diabetes, whereas the same benefits have not been observed in other animal studies or in human clinical trials. Additional clinical and mechanistic studies are needed to better understand the isomer-specific effects of ruminant TFA. Until then, production practices resulting in increased levels of this group of fatty acids in ruminant milk and meat should be carefully reconsidered.
Introduction
Trans fatty acids (TFA) are unsaturated fatty acids that contain at least one double bond in the trans configuration (i.e. the two hydrogen atoms are on opposite sides of the carbon to carbon double bond), resulting in a straighter shape more similar to saturated fatty acids. Consequently, TFA are less fluid and have a higher melting point than unsaturated fatty acids with cis double bonds, which are the major monounsaturated fatty acids (MUFA) and polyunsaturated fatty acids (PUFA) in plants and animals (Bhardwaj et al., 2011).
TFA in foods mainly come from two sources, including partially hydrogenated vegetable oils (i.e. industrial TFA) and ruminant-derived foods such as dairy and beef (i.e. ruminant TFA). Industrial TFA are found in partially hydrogenated vegetable oils generated using hydrogen in the presence of a catalyst (Bhardwaj et al., 2011), while ruminant TFA are made by rumen bacteria using a process called biohydrogenation (Lichtenstein, 2000; Dhaka et al., 2011).
Until recently, industrial TFA were extensively used by the food industry as a replacement for saturated fats. However, in recent years, many countries have banned industrial TFA from the food supply due to their detrimental effects on cardiovascular health (Lichtenstein, 2000; Mozaffarian et al., 2006). Recent government bans on industrial TFA in developed countries including the U.S, Canada and the E.U has left ruminant-derived fats (e.g. dairy, beef, lamb and goat) as the sole source of TFA in the food supply. As such, there is heightened interest in the composition, content, and health effects of ruminant TFA particularly conjugated linoleic acid (CLA) and trans 18:1 isomers (Brouwer et al., 2010; Prada et al., 2022). The aim of this review is to provide an update of the current state of knowledge on the biosynthesis, concentration range and factors affecting the concentration of TFA in ruminant fats, current intake of ruminant TFA, and to evaluate evidence on their health effects with an emphasis on type 2 diabetes.
Biosynthesis of ruminant TFA
Ruminant TFA are formed via biohydrogenation of unsaturated fatty acids in the rumen. The process of biohydrogenation is performed by rumen bacteria, during which dietary unsaturated fatty acids are converted to saturated fatty acids (Lichtenstein, 2000; Dhaka et al., 2011; Dugan et al., 2018). Dietary unsaturated fatty acids are toxic to rumen microbes, hence they biohydrogenate them to saturated fatty acids which are neutral or less toxic (Jenkins et al., 2008). The biohydrogenation process includes several isomerization and hydrogenation steps which result in the formation of many intermediates including conjugated and non-conjugated trienoic, dienoic and monoenoic trans fatty acids (Jenkins et al., 2008; Vahmani et al., 2015). A small portion of these intermediates passes the rumen and subsequently find their way into tissues and milk after post-ruminal absorption (Lichtenstein, 2000; Dugan et al., 2018).
The predominant fatty acids in ruminant diets include 18:2n-6 (linoleic acid; LNA) and 18:3n-3 (alpha-linolenic acid; ALA) and thus are considered the main substrates for ruminal biohydrogenation. It is estimated that on average, about 80% and 92% of dietary LNA and ALA are biohydrogenated in the rumen (Conte et al., 2017). Several different biohydrogenation pathways have been proposed for LNA and ALA. Several factors including forage-to-concentrate ratio and ruminal passage rate/residence time can determine the pathway and extent of the biohydrogenation of LNA and ALA (Chilliard et al., 2014; Dugan et al., 2018; Dewanckele et al., 2020).
The main pathways for the biohydrogenation of LNA and ALA have been described by Harfoot and Hazlewood (1997). Pathways for both LNA and ALA are characterized by initial isomerization of the cis double bond at carbon 12 to a trans double bond at carbon 11 resulting in the production of cis9, trans11-18:2, an isomer of CLA (also known as rumenic acid; RA), and cis9, trans11, cis15-18:3, respectively (Figure 1). This is followed by rounds of hydrogenation and isomerization leading to a trans 18:1 isomer (e.g., trans11-18:1) and eventually complete hydrogenation to 18:0 (stearic acid) as the end product (Dewanckele et al., 2020).
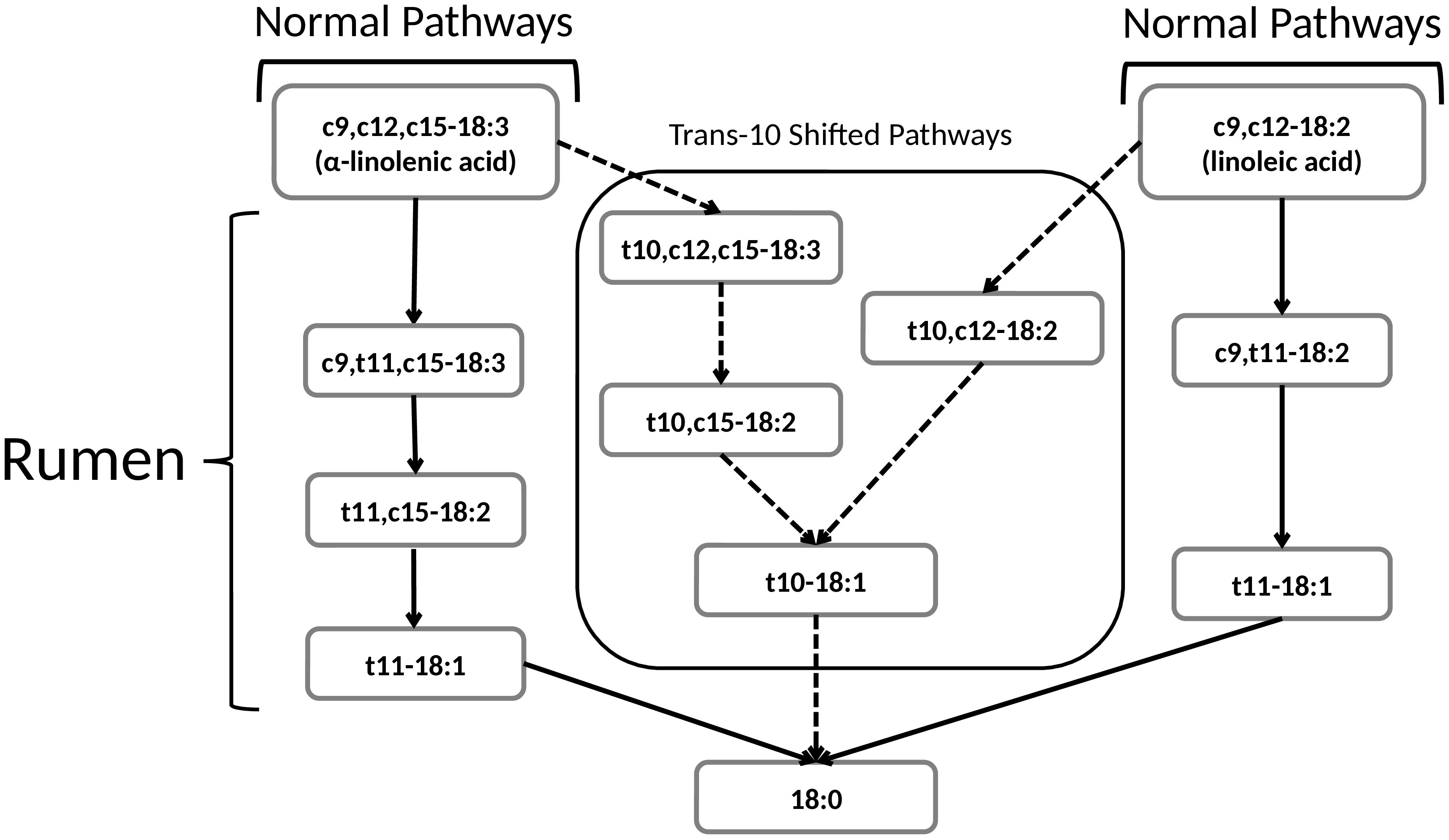
Figure 1 Normal and trans-10 shifted biohydrogenation pathways of linoleic acid (cis9,cis12-18:2) and alpha-linolenic acid (cis9,cis12,cis15-18:3). Arrow with solid lines show the main pathways in ruminants fed forage-based diets, and arrows with dashed lines show pathways in ruminants fed grain-based diets (Adopted from Alves et al., 2021).
It is noteworthy that the abovementioned pathways were elucidated when greater forage-to-grain ratios (forage-based diets) were fed. When feeding grain-based diets (e.g., feedlot diets), isomerization of the cis 9 double bonds for LA shifts towards a trans double bond at carbon 10, with the same happening for ALA shifts, resulting in the production of trans10, cis12-18:2 and trans10, cis12, cis15-18:3, respectively (Alves and Bessa, 2014). These have been referred to as the “trans-10 shifted” biohydrogenation pathways (Figure 1) which result in the accumulation of trans10-18:1 as the main TFA in ruminant products such as beef from feedlot (grain-finished) cattle (Alves and Bessa, 2014; Alves et al., 2021). Whereas trans11-18:1 (trans vaccenic acid; TVA) is typically the main TFA found in milk and meat from ruminants fed forage-based diets (e.g., grass-fed beef) (Jaakamo et al., 2019). Findings from animal model studies suggest that trans10-18:1 may harm health in a similar fashion to industrial TFA (Alves et al., 2021). On the other hand, TVA can be converted to RA (cis9, trans 11-CLA), both of which have been associated with several health benefits such as prevention of cancer, cardiovascular disease, and inflammation as well as improved immune function (Derakhshande-Rishehri et al., 2014; Kim et al., 2016). Consequently, TVA and RA are sometimes referred to as “good” TFA (Diane et al., 2016; Vahmani et al., 2020).
In addition to the main biohydrogenation pathways mentioned above, there are numerous minor pathways active in the rumen resulting in a plethora of biohydrogenation intermediates including conjugated and non-conjugated trienoic (18:3), dienoic (18:2) and monoenoic (18:1 and 16:1) TFA isomers (Vahmani et al., 2020). In fact, milk and meat fats from ruminants (e.g. cattle, sheep and goats) have the most complex fatty acid composition (> 100 different fatty acids) among all edible fats, in part due to the presence of numerous biohydrogenation intermediates. However, the human health effects of many of these intermediates are for the most part unknown.
Types and concentrations of TFA in ruminant fats
The biohydrogenation process results in about 50 different types of TFA including trans-16:1 (trans6- to trans12-16:1), trans-18:1 (trans4- to trans16-18:1), conjugated 18:2 known as CLA (≥ 12 different CLA isomers with cis/trans or trans/cis configurations), non-conjugated non-methylene interrupted 18:2 known as atypical dienes (≥ 10 different isomers with cis/trans or trans/cis configurations) and conjugated 18:3 (≥ 3 different isomers with cis/trans/trans or cis/trans/cis configurations). Trans-18:1s are the predominant TFA (70-80% of total TFA), followed by CLA (10-25% of total TFA), atypical dienes (5-15% of total TFA), trans-16:1 (5-10% of total TFA) and conjugated linolenic acid (CLnA, <5% of total TFA) (Table 1). Among individual TFA isomers, TVA, RA and trans9-16:1 (trans palmitoleic acid; TPA) have been the most studied isomers in terms of health effects and bioactivity, due to their high prevalence in ruminant foods and commercial availability (i.e. pure fatty acid isomers).
Current intake of ruminant TFA
There have been numerous studies done on the health effects of industrial TFA during the past 4 decades. These studies have consistently found that industrial TFA have been associated with an increased risk of cardiovascular disease, mainly by lowering HDL and raising LDL levels (Stender et al., 2008; Brouwer et al., 2010; Radtke et al., 2017; Oteng et al., 2019). In the mid-1990s, many developed countries including the EU made recommendations to limit the intake of industrial TFA to 1% of the daily energy intake (Wanders et al., 2017). According to a study in 2010, the average daily TFA intake for adults was around 2.5 to 3.49% of daily energy intake in the U.S. (Micha et al., 2014), which was much higher than the 1% recommended limit. About 10 years later in 2015, the U.S. Food and Drug Administration (FDA) ruled that industrial TFA are not safe in food and set a June 2018 deadline for their removal from the food system (USDA, 2020). The official ban on industrial TFA has left ruminant-derived fats as the sole dietary source of TFA in the U.S., as well as in Canada and most European countries.
The estimated dietary intake of ruminant TFA varies between 0.8% to 1.7% of total energy intake depending on the country, with the average intake of ruminant TFA in the U.S. estimated to be about 1.2% of energy intake (Gebauer et al., 2011). In a more recent study, however, ruminant TFA intake in Europe and the U.S. was estimated to be around 0.5% of energy intake (Brouwer et al., 2013). Given recent bans on industrial TFA, there is need for new studies to determine the current intake of TFA from ruminant derived foods.
Factors affecting concentrations of TFA in ruminant-derived foods
The TFA composition of ruminant derived foods is largely influenced by dietary, management and animal factors. Among these factors, diet composition is the main determinant of biohydrogenation pathways, and consequently of the content and composition of TFA in ruminant fats. Adding sources of PUFA to the diet (e.g. plant oil and oilseeds) significantly increases the contents of TFA in ruminant milk and meat including RA, TVA and TPA (Scollan et al., 2017; Guillocheau et al., 2020; Guo et al., 2023). The source of PUFA has the largest impact, with LNA-rich oils, yielding the greatest RA, TVA and TPA contents (Bessa et al., 2015; Kliem and Shingfield, 2016). Feeding ALA-rich sources also enhances CLnA content in ruminant fats (Kliem and Shingfield, 2016; Chikwanha et al., 2018). Seemingly effective novel alternative oil sources for increasing TFA, such as insect oils, warrant further investigation (Hervás et al., 2022). Furthermore, the amount of PUFA increases TFA content, reaching a peak when feeding between 50 and 80 g/kg DM intake (Scollan et al., 2017; Chikwanha et al., 2018). Besides PUFA, feeding forage-based diets, as opposed to grain-based diets, effectively increases TFA with trans-11 double bonds (TVA and RA) in ruminant fats (Chikwanha et al., 2018; Cabiddu et al., 2022). However, the effectiveness of forage-based diets at increasing TFA is determined by several factors relating to the source of forage including species diversity, cultivars, phenological stage, maturity, conservation method, particle length, presence of bioactive compounds and seasonality (Frutos et al., 2020; Cabiddu et al., 2022). Noteworthy, feeding a combination of forages and PUFA for an extend period substantially enhances presence of TFA in ruminant fats (Kliem and Shingfield, 2016; Vahmani et al., 2020; Alves et al., 2021), particularly when forage and PUFA sources are feed separately (Vahmani et al., 2017).
High-grain diets supplemented with LNA substantially increases the content of trans10-18:1 and trans10,cis12-CLA (i.e., trans10-shift) in ruminant meat and milk (Mapiye et al., 2015; Kliem and Shingfield, 2016). The t10-shift is exacerbated by feeding small grains (i.e. barley and wheat versus corn), pelleting, and increasing feeding duration (Mapiye et al., 2012; Mutsvangwa et al., 2012). A multitude of strategies to avoid the trans10-shift such as the addition of forages, non-starch fibers, strong buffers, antibiotics, antioxidants, yeast, chitosan, and agro-industrial by-products to high-grain diets, have been attempted with varying success (Alves et al., 2021; Amin and Mao, 2021; Hervás et al., 2022). Additionally, PUFA protective treatments and adsorbents inhibit the trans10-shift to a limited extent (Guo et al., 2023).
Notably, contents of TFA are more effectively increased in milk versus meat and in small versus large ruminants (Chilliard et al., 2007; Dugan et al., 2011; Chikwanha et al., 2018). The TFA contents in milk are somewhat influenced by animal individuality, breed, stage of lactation and parity (Samková et al., 2012). In meat animals, breed, sex, slaughter age and weight, anatomical location of fat depot and muscle type have marginal effects on TFA contents (Mapiye et al., 2015).
Metabolism of ruminant TFA in the human body
Most published data on TFA metabolism comes from studies on trans 18:1, which are the predominant fatty acid type in both ruminant and industrial TFA. Trans 18:1 isomers are intestinally absorbed to the same extent as cis 18:1 isomers and the double-bond position has little or no effect on their absorption (Baer et al., 2003). After absorption, trans 18:1 isomers can be incorporated into cell membrane phospholipids, or stored in the triacylglycerols of adipose tissues. Trans 18:1 isomers can be metabolized by oxidation, elongation, and desaturation processes, which result in isomer-specific metabolites with different biochemical properties (Vahmani et al., 2020). For TVA, two main metabolic fates have been characterized which include chain shortening to TPA and delta-9 desaturation to RA (Figure 2). It has been estimated that ~19% of TVA, the main t-18:1 isomer in milk and meat from forage-fed ruminants consumed by humans, can be converted to RA by tissue-level Δ-9 desaturation which is catalyzed by the stearoyl-CoA desaturase 1 (SCD1) enzyme (Turpeinen et al., 2002; Miller et al., 2003).
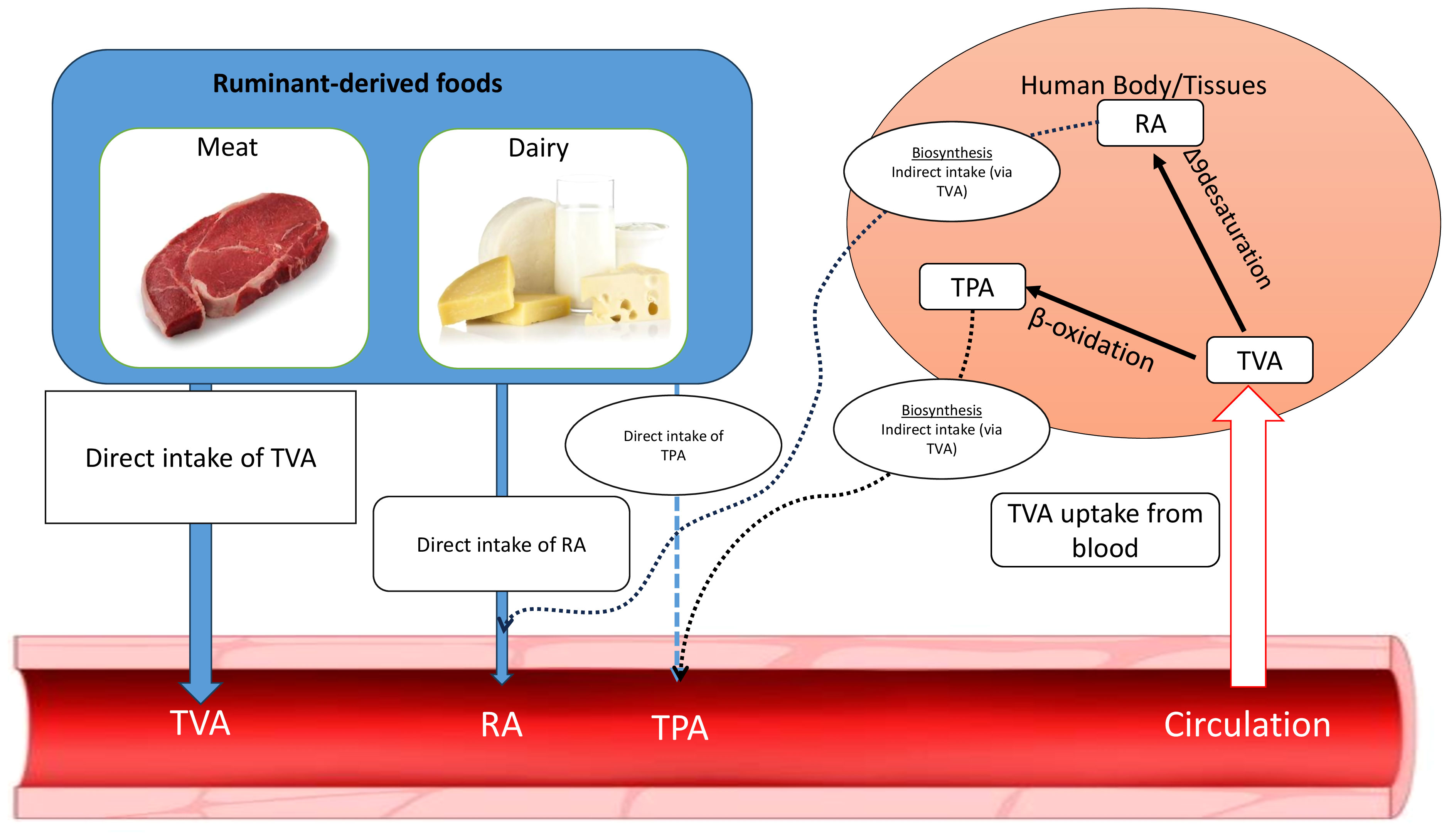
Figure 2 Origin of ruminant trans fatty acids in human blood. TPA, trans-palmitoleic acid (trans9-16:1); TVA, trans vaccenic acid (trans11-18:1); RA, rumenic acid (cis9,trans11-18:2); Arrows with thick solid lines describes the major origins, arrow with narrow lines indicates minor origins, arrows with dashed lines shows the very minor origins (Turpeinen et al., 2002; Miller et al., 2003; Jaudszus et al., 2014).
In addition to Δ-9 desaturation, chain shortening of TVA by β-oxidation (peroxisomal β-oxidation of TVA) can lead to elevated levels of TPA in the plasma or tissues after consuming foods containing TVA, particularly grass-fed beef and dairy products. The conversion rate of TVA to TPA has been estimated to be 10% in cultured rat hepatocytes incubated with TVA (Jaudszus et al., 2014), however, the whole body (in vivo) conversion rate of TVA to TPA is not known. Given the very low concentration of TPA in the food supply including ruminant-derived foods (<0.05% of total fatty acids), the major origin of circulating TPA in humans is assumed to be from TVA intake from consumption of ruminant derived foods (Jaudszus et al., 2014).
Health effects of ruminant TFA
The effects of ruminant- versus industrial- TFA on human health have been controversial and a subject of debate for many years. The recent removal of industrial TFA from the food supply in developed countries has renewed interest in understanding the human health effects of ruminant TFA. Several epidemiological studies have shown that, in contrast to industrial TFA, ruminant TFA do not appear to increase cardiovascular disease (CVD) risk and mortality (Ascherio et al., 1994; Pietinen et al., 1997; Jakobsen et al., 2008; Bendsen et al., 2011; Sacks et al., 2017). This has been attributed to the different isomeric profile of trans18:1 between ruminant and industrial TFA, as well as the low concentrations of total TFA in ruminant fats (2-6% of total fatty acids) compared to partially hydrogenated vegetable oils (60-65% of total fatty acids) (Stender et al., 2008). Conversely, based on recent human clinical trials, both industrial- and ruminant- TFA adversely affect cholesterol homeostasis (i.e. increased blood LDL-cholesterol and reduced HDL cholesterol) when consumed at comparable levels (Motard-Bélanger et al., 2008; Brouwer et al., 2013; Gebauer et al., 2015; Stender, 2015; Verneque et al., 2022). However, there is limited information available on the effect of ruminant TFA on other cardiovascular disease risk markers and the development of other chronic diseases such as type 2 diabetes. In the following section, we summarize published data from observational studies, clinical trials and animal studies on the effects of ruminant TFA on metabolic parameters related to type 2 diabetes.
Observational studies
Since 2010, several prospective epidemiological studies have consistently shown that increased blood levels of TPA, the chain shortening product of TVA (the most abundant TFA in ruminant fats) was associated with lower risk and incidence of type 2 diabetes (Mozaffarian et al., 2010). Similarly, two meta-analyses reported circulating TPA was inversely associated with type 2 diabetes (Imamura et al., 2018). In a more recent epidemiological study, circulating TVA but not TPA was inversely associated with diabetes risk (Prada et al., 2022). This discrepancy could be in part due to the limitations in the analytical methods used to determine TFA isomeric profile in human blood samples (Guillocheau et al., 2020). Although available data from observational studies point towards potential antidiabetic properties of ruminant TFA, a cause-and-effect relationship has not yet been proven in humans.
Clinical trials
There is very limited clinical data on the human health effects of ruminant TFA. Gebauer et al. (2015) compared the effects of isocaloric diets containing different TFA isomers in a randomized, crossover feeding trial in 106 healthy subjects who were each provided the diets for 24 days. Diets were designed to have stearic acid replaced with the following TFA isomers (percentage of energy): ~3% pure TVA, ~3% mixed isomers of industrial TFA from partially hydrogenated vegetable oil, or 1% pure RA. In this study, there was no difference among treatments in terms of metabolic parameters related to type 2 diabetes including blood glucose, insulin, or insulin resistance index (HOMA-IR). Another study using a hyperinsulinemic-euglycemic clamp in abdominally obese male subjects reported that supplementation with pure RA (~1% of energy intake for 12 weeks) reduced insulin sensitivity compared with an olive oil placebo (Risérus et al., 2004). To our knowledge, there has been no clinical study investigating the health effect of TPA supplementation in humans, likely due to the lack of pure TPA (Guillocheau et al., 2020). In fact, the above two studies are the only two human clinical studies examining health effects of pure ruminant TFA isomers. However, there are four published clinical studies in which ruminant TFA-enriched dairy fats were fed. In these studies, TVA+RA-enriched butter (1–1.5% of energy intake from TVA+RA) did not alter blood glucose and insulin, HOMA-IR or glucose tolerance in healthy subjects compared with standard butters (Tholstrup et al., 2006; Tricon et al., 2006; Brown et al., 2011; Penedo et al., 2013; Werner et al., 2013). Although observational studies showed that higher circulating levels of ruminant TFA may be protective against type 2 diabetes, available data from clinical trials do not support a beneficial effect of ruminant TFA on glucose homeostasis in humans. It is noteworthy, however, almost all of these clinical trials were done in healthy subjects and not in people with prediabetes or type 2 diabetes.
Animal studies
Most available data regarding the promising health effects of ruminant TFA including their postulated antidiabetic properties come from animal model studies. Feeding a diet enriched with 1% pure TVA (~4.5% of energy intake) to obese insulin resistant JCR-LA:cp rats resulted in significant reductions in fasting and postprandial insulin levels and an increase in insulin sensitivity (lower HOMA-IR) (Jacome-Sosa et al., 2014). The authors attributed these insulin-sensitizing effects of TVA to activation of peroxisome proliferator-activated receptor-γ. In fact, both TVA and its delta-9 desaturation product, RA, have been shown to act as ligands for PPARγ and PPARα, which are transcription factors for several genes involved in lipid and glucose metabolism (Moya-Camarena et al., 1999; Wang et al., 2012). Feeding beef fat enriched with TVA and RA to obese/insulin-resistant JCR : LA-cp rats reduced fasting insulin and HOMA-IR, and lowered insulin secretion following a meal tolerance test, which were accompanied by higher protein expression of PPARγ and PPARα in the liver (Diane et al., 2016). Similarly, feeding Wister rats a high-fat diet containing TVA+RA-enriched butter reduced fasting serum insulin and increased hepatic PPARγ protein expression compared to rats fed a control high-fat diet containing standard butter (De Almeida et al., 2014). The apparent insulin-sensitizing effects of TVA and RA in the above rodent studies were attributed to their potential to bind and activate PPARγ-regulated pathways in the liver and adipose tissues. Moreover, it has been suggested that TVA can also restore glucose homeostasis by promoting insulin secretion from pancreatic islets. In diabetic Sprague–Dawley rats (induced by high-fat diet/streptozotocin), 8 weeks supplementation with pure TVA (1.2% of diet mass) reduced both fed and fasting blood glucose, and increased β-cell area (Wang et al., 2016). In addition, in this study, hyperglycemic clamp showed that TVA increased glucose turnover in diabetic rats, accompanied by an elevated plasma C-peptide concentration, suggesting improved insulin secretion. Moreover, isolated islets from TVA fed diabetic rats had higher glucose-stimulated insulin secretion (GSIS) than the control diabetic rats (Wang et al., 2016). Thus, the authors concluded that TVA may improve glucose homeostasis in diabetic rats in part by promoting insulin secretion (Wang et al., 2016). Consistent with these findings, Wang et al. recently reported that feeding pure TVA to diabetic rats could promote insulin secretion through stimulating G-protein coupled receptor 40 (GPR40) expression and signaling in islets (Wang et al., 2019).
The above studies should be weighed against other studies that have not found ruminant TFA to improve glucose homeostasis in animal models of insulin resistance and type 2 diabetes. In obese/insulin resistant JCR-LA:cp rats, feeding a diet enriched with pure TVA (1.5% of diet as TVA) did not alter fasting levels of insulin or glucose, nor insulin and glucose responses to a meal tolerance test (Wang et al., 2008). Another study using Wistar rats showed that 8 weeks of feeding diets enriched (4% of energy intake) with either TVA, mixed industrial TFA mixed isomers or oleic acid to did not alter insulin and glucose responses to an intraperitoneal glucose tolerance test (Tardy et al., 2008). Similarly, dietary supplementation with pure TPA (0.7% of energy intake) did not modify glucose homeostasis in high fat diet-induced obese (DIO) mice as measured by glucose/insulin tolerance tests and insulin-mediated Akt activation (Chávaro-Ortiz et al., 2022). Another recent study using DIO mice showed that feeding a high-fat diet containing beef fat naturally enriched with TVA and RA (beef fat from flaxseed-fed cattle) for 19 weeks worsened glucose tolerance and liver steatosis compared to mice fed a control high fat diet (Xu et al., 2022). The authors partly attributed the adverse effects on glucose tolerance and liver health to other TFA present in TVA+RA enriched beef fat. It is noteworthy that no study to date has tested the effects of pure TVA or RA in DIO mouse model which is one of the most clinically translatable animal models to test the efficacy of natural compounds and/or drugs against prediabetes and type 2 diabetes.
The discrepancy in findings may be attributed to methodological differences among studies, such as animal model, diet composition, study duration, as well as specific techniques used to assess glucose homeostasis and parameters related to type 2 diabetes. It is noteworthy that no study to date has tested the effects of pure TVA or RA in DIO mouse model which is one of the most clinically translatable animal models to test the efficacy of natural compounds and/or drugs against prediabetes and type 2 diabetes.
Conclusion
Taken together, although TVA and its metabolites (TPA and RA) have been touted as having antidiabetic properties based on data from observational studies and a small number of animal studies, while the same effects have not been observed in other animal studies or in human clinical trials. Additional clinical and mechanistic studies are needed to better understand the isomer-specific effects of ruminant TFA. Nevertheless, based on the current knowledge regarding potential adverse effects of ruminant TFA on blood lipoprotein profiles, practices resulting in increased levels of this group of fatty acids in ruminant milk and meat should be carefully reconsidered.
Author contributions
PV: Conceptualization, Writing – original draft, Writing – review & editing. YX: Writing – original draft. MD: Writing – review & editing. CM: Writing – original draft, Writing – review & editing.
Funding
The author(s) declare that no financial support was received for the research, authorship, and/or publication of this article.
Conflict of interest
The authors declare that the research was conducted in the absence of any commercial or financial relationships that could be construed as a potential conflict of interest.
The author(s) declared that they were an editorial board member of Frontiers, at the time of submission. This had no impact on the peer review process and the final decision.
Publisher’s note
All claims expressed in this article are solely those of the authors and do not necessarily represent those of their affiliated organizations, or those of the publisher, the editors and the reviewers. Any product that may be evaluated in this article, or claim that may be made by its manufacturer, is not guaranteed or endorsed by the publisher.
References
Alves S. P., Bessa R. J. B. (2014). The trans-10,cis-15 18:2: A missing intermediate of trans-10 shifted rumen biohydrogenation pathway? Lipids 49, 527–541. doi: 10.1007/s11745-014-3897-4
Alves S. P., Vahmani P., Mapiye C., McAllister T. A., Bessa R. J. B., Dugan M. E. R. (2021). Trans-10 18:1 in ruminant meats: A review. Lipids 56, 539–562. doi: 10.1002/lipd.12324
Amin A. B., Mao S. (2021). Influence of yeast on rumen fermentation, growth performance and quality of products in ruminants: A review. Anim. Nutr. 7, 31–41. doi: 10.1016/j.aninu.2020.10.005
Ascherio A., Hennekens C. H., Buring J. E., Master C., Stampfer M. J., Willett W. C. (1994). Trans-fatty acids intake and risk of myocardial infarction. Circulation 89, 94–101. doi: 10.1161/01.CIR.89.1.94
Baer D. J., Judd J. T., Kris-Etherton P. M., Zhao G., Emken E. A. (2003). Stearic acid absorption and its metabolizable energy value are minimally lower than those of other fatty acids in healthy men fed mixed diets. J. Nutr. 133, 4129–4134. doi: 10.1093/jn/133.12.4129
Bendsen N. T., Christensen R., Bartels E. M., Astrup A. (2011). Consumption of industrial and ruminant trans fatty acids and risk of coronary heart disease: A systematic review and meta-analysis of cohort studies. Eur. J. Clin. Nutr. 65, 773–783. doi: 10.1038/ejcn.2011.34
Bessa R. J. B., Alves S. P., Santos-Silva J. (2015). Constraints and potentials for the nutritional modulation of the fatty acid composition of ruminant meat. Eur. J. Lipid Sci. Technol. 117, 1325–1344. doi: 10.1002/ejlt.201400468
Bhardwaj S., Passi S. J., Misra A. (2011). Overview of trans fatty acids: Biochemistry and health effects. Diabetes Metab. Syndr. Clin. Res. Rev. 5, 161–164. doi: 10.1016/j.dsx.2012.03.002
Brouwer I. A., Wanders A. J., Katan M. B. (2010). Effect of animal and industrial Trans fatty acids on HDL and LDL cholesterol levels in humans - A quantitative review. PloS One 5. doi: 10.1371/journal.pone.0009434
Brouwer I. A., Wanders A. J., Katan M. B. (2013). Trans fatty acids and cardiovascular health: Research completed? Eur. J. Clin. Nutr. 67, 541–547. doi: 10.1038/ejcn.2013.43
Brown A. W., Trenkle A. H., Beitz D. C. (2011). Diets high in conjugated linoleic acid from pasture-fed cattle did not alter markers of health in young women. Nutr. Res. 31, 33–41. doi: 10.1016/j.nutres.2010.12.003
Cabiddu A., Peratoner G., Valenti B., Monteils V., Martin B., Coppa M. (2022). A quantitative review of on-farm feeding practices to enhance the quality of grassland-based ruminant dairy and meat products. Animal 16, 100375. doi: 10.1016/j.animal.2021.100375
Chávaro-Ortiz L. I., Tapia B. D., Navarrete-Fuentes M., Gutiérrez-Aguilar R., Frigolet M. E. (2022). Trans-palmitoleic acid prevents weight gain, but does not modify glucose homeostasis in a rodent model of diet-induced obesity. Clin. Nutr. Open Sci. 44, 42–48. doi: 10.1016/j.nutos.2022.06.003
Chikwanha O. C., Vahmani P., Muchenje V., Dugan M. E. R., Mapiye C. (2018). Nutritional enhancement of sheep meat fatty acid profile for human health and wellbeing. Food Res. Int. 104, 25–38. doi: 10.1016/j.foodres.2017.05.005
Chilliard Y., Glasser F., Ferlay A., Bernard L., Rouel J., Doreau M. (2007). Diet, rumen biohydrogenation and nutritional quality of cow and goat milk fat. Eur. J. Lipid Sci. Technol. 109, 828–855. doi: 10.1002/ejlt.200700080
Chilliard Y., Toral P. G., Shingfield K. J., Rouel J., Leroux C., Bernard L. (2014). Effects of diet and physiological factors on milk fat synthesis, milk fat composition and lipolysis in the goat: A short review. Small Rumin. Res. 122, 31–37. doi: 10.1016/j.smallrumres.2014.07.014
Conte G., Serra A., Mele M. (2017). “Dairy cow breeding and feeding on the milk fatty acid pattern,” in Nutrients in dairy and their implications for health and disease (London, United Kingdom: Academic Press, Elsevier). doi: 10.1016/B978-0-12-809762-5.00002-4
De Almeida M. M., Luquetti S. C. P. D., Sabarense C. M., Corrêa J. O. D. A., Dos Reis L. G., Da Conceição E. P. S., et al. (2014). Butter naturally enriched in cis-9, trans-11 CLA prevents hyperinsulinemia and increases both serum HDL cholesterol and triacylglycerol levels in rats. Lipids Health Dis. 13. doi: 10.1186/1476-511X-13-200
Derakhshande-Rishehri S. M., Mansourian M., Kelishadi R., Heidari-Beni M. (2014). Association of foods enriched in conjugated linoleic acid (CLA) and CLA supplements with lipid profile in human studies: A systematic review and meta-analysis. Public Health Nutr. 18, 2041–2054. doi: 10.1017/S1368980014002262
Dewanckele L., Toral P. G., Vlaeminck B., Fievez V. (2020). Invited review: Role of rumen biohydrogenation intermediates and rumen microbes in diet-induced milk fat depression: An update. J. Dairy Sci. 103, 7655–7681. doi: 10.3168/jds.2019-17662
Dhaka V., Gulia N., Ahlawat K. S., Khatkar B. S. (2011). Trans fats-sources, health risks and alternative approach - A review. J. Food Sci. Technol. 48, 534–541. doi: 10.1007/s13197-010-0225-8
Diane A., Borthwick F., Mapiye C., Vahmani P., David R. C., Vine D. F., et al. (2016). Beef fat enriched with polyunsaturated fatty acid biohydrogenation products improves insulin sensitivity without altering dyslipidemia in insulin resistant JCR:LA-cp rats. Lipids 51, 821–831. doi: 10.1007/s11745-016-4148-7
Dugan M., Aldai N., Aalhus J., Rolland D., Kramer J. (2011). Review: Trans-forming beef to provide healthier fatty acid profiles. Can. J. Anim. Sci. 91, 545–556. doi: 10.4141/cjas2011-044
Dugan M. E. R., Mapiye C., Vahmani P. (2018). “Polyunsaturated fatty acid biosynthesis and metabolism in agriculturally important species,” in. Polyunsaturated Fatty Acid Metab., 61–86. doi: 10.1016/B978-0-12-811230-4.00004-1
Frutos P., Hervás G., Natalello A., Luciano G., Fondevila M., Priolo A., et al. (2020). Ability of tannins to modulate ruminal lipid metabolism and milk and meat fatty acid profiles. Anim. Feed Sci. Technol. 269, 114623. doi: 10.1016/j.anifeedsci.2020.114623
Gebauer S. K., Chardigny J. M., Jakobsen M. U., Lamarche B., Lock A. L., Proctor S. D., et al. (2011). Effects of ruminant trans fatty acids on cardiovascular disease and cancer: A comprehensive review of epidemiological, clinical, and mechanistic studies. Adv. Nutr. 2, 332–354. doi: 10.3945/an.111.000521
Gebauer S. K., Destaillats F., Dionisi F., Krauss R. M., Baer D. J. (2015). Vaccenic acid and trans fatty acid isomers from partially hydrogenated oil both adversely affect LDL cholesterol: A double-blind, randomized controlled trial. Am. J. Clin. Nutr. 102, 1339–1346. doi: 10.3945/ajcn.115.116129
Guillocheau E., Legrand P., Rioux V. (2020). Trans-palmitoleic acid (trans-9-C16:1, or trans-C16:1 n-7): Nutritional impacts, metabolism, origin, compositional data, analytical methods and chemical synthesis. A review. Biochimie 169, 144–160. doi: 10.1016/j.biochi.2019.12.004
Guo Q., Li T., Qu Y., Liang M., Ha Y., Zhang Y., et al. (2023). New research development on trans fatty acids in food: Biological effects, analytical methods, formation mechanism, and mitigating measures. Prog. Lipid Res. 89, 101199. doi: 10.1016/j.plipres.2022.101199
Harfoot C., Hazlewood G. P. (1997). “Lipid metabolism in the rumen,” in The rumen microbial ecosystem. Eds. Hobson P. N., Stewart C. S.. (Amsterdam, The Netherlands: Elsevier Science Publishers). 382–426. doi: 10.1007/978-94-009-1453-7_9
Hervás G., Boussalia Y., Labbouz Y., Della Badia A., Toral P. G., Frutos P. (2022). Insect oils and chitosan in sheep feeding: Effects on in vitro ruminal biohydrogenation and fermentation. Anim. Feed Sci. Technol. 285, 115222. doi: 10.1016/j.anifeedsci.2022.115222
Imamura F., Fretts A., Marklund M., Ardisson Korat A. V., Yang W. S., Lankinen M., et al. (2018). Fatty acid biomarkers of dairy fat consumption and incidence of type 2 diabetes: A pooled analysis of prospective cohort studies. PloS Med. 15. doi: 10.1371/journal.pmed.1002670
Jaakamo M. J., Luukkonen T. J., Kairenius P. K., Bayat A. R., Ahvenjärvi S. A., Tupasela T. M., et al. (2019). The effect of dietary forage to concentrate ratio and forage type on milk fatty acid composition and milk fat globule size of lactating cows. J. Dairy Sci. 102, 8825–8838. doi: 10.3168/jds.2018-15833
Jacome-Sosa M. M., Borthwick F., Mangat R., Uwiera R., Reaney M. J., Shen J., et al. (2014). Diets enriched in trans-11 vaccenic acid alleviate ectopic lipid accumulation in a rat model of NAFLD and metabolic syndrome. J. Nutr. Biochem. 25, 692–701. doi: 10.1016/j.jnutbio.2014.02.011
Jakobsen M. U., Overvad K., Dyerberg J., Heitmann B. L. (2008). Intake of ruminant trans fatty acids and risk of coronary heart disease. Int. J. Epidemiol. 37, 173–182. doi: 10.1093/ije/dym243
Jaudszus A., Kramer R., Pfeuffer M., Roth A., Jahreis G., Kuhnt K. (2014). Trans Palmitoleic acid arises endogenously from dietary vaccenic acid. Am. J. Clin. Nutr. 99, 431–435. doi: 10.3945/ajcn.113.076117
Jenkins T. C., Wallace R. J., Moate P. J., Mosley E. E. (2008). BOARD-INVITED REVIEW: Recent advances in biohydrogenation of unsaturated fatty acids within the rumen microbial ecosystem 1. J. Anim. Sci. 86, 397–412. doi: 10.2527/jas.2007-0588
Kim J. H., Kim Y., Kim Y. J., Park Y. (2016). Conjugated linoleic acid: potential health benefits as a functional food ingredient. Annu. Rev. Food Sci. Technol. 7, 221–265. doi: 10.1146/annurev-food-041715-033028
Kliem K. E., Shingfield K. J. (2016). Manipulation of milk fatty acid composition in lactating cows: Opportunities and challenges. Eur. J. Lipid Sci. Technol. 118, 1661–1683. doi: 10.1002/ejlt.201400543
Klopatek S. C., Xu Y., Yang X., Oltjen J. W., Vahmani P. (2022). Effects of multiple grass- and grain-fed production systems on beef fatty acid contents and their consumer health implications. ACS Food Sci. Technol. 2, 712–721. doi: 10.1021/acsfoodscitech.2c00021
Lichtenstein A. H. (2000). “Dietary trans fatty acid,” in J. Cardiopulm. Rehabil. (Amsterdam, The Netherlands: B.V.) 20, 143–146. Available at: http://www.embase.com/search/results?subaction=viewrecord&from=export&id=L30368506.
Mapiye C., Aldai N., Turner T. D., Aalhus J. L., Rolland D. C., Kramer J. K. G., et al. (2012). The labile lipid fraction of meat: From perceived disease and waste to health and opportunity. Meat Sci. 92, 210–220. doi: 10.1016/j.meatsci.2012.03.016
Mapiye C., Vahmani P., Mlambo V., Muchenje V., Dzama K., Hoffman L. C., et al. (2015). The trans-octadecenoic fatty acid profile of beef: Implications for global food and nutrition security. Food Res. Int. 76, 992–1000. doi: 10.1016/j.foodres.2015.05.001
Micha R., Khatibzadeh S., Shi P., Fahimi S., Lim S., Andrews K. G., et al. (2014). Global, regional, and national consumption levels of dietary fats and oils in 1990 and 2010: A systematic analysis including 266 country-specific nutrition surveys. BMJ 348. doi: 10.1136/bmj.g2272
Miller A., McGrath E., Stanton C., Devery R. (2003). Vaccenic acid (t11-18:1) is converted to c9,t11-CLA in MCF-7 and SW480 cancer cells. Lipids 38, 623–632. doi: 10.1007/s11745-003-1107-8
Motard-Bélanger A., Charest A., Grenier G., Paquin P., Chouinard Y., Lemieux S., et al. (2008). Study of the effect of trans fatty acids from ruminants on blood lipids and other risk factors for cardiovascular disease. Am. J. Clin. Nutr. 87, 593–599. doi: 10.1093/ajcn/87.3.593
Moya-Camarena S. Y., Vanden Heuvel J. P., Blanchard S. G., Leesnitzer L. A., Belury M. A. (1999). Conjugated linoleic acid is a potent naturally occurring ligand and activator of PPARα. J. Lipid Res. 40, 1426–1433. doi: 10.1016/s0022-2275(20)33384-8
Mozaffarian D., Cao H., King I. B., Lemaitre R. N., Song X., Siscovick D. S., et al. (2010). Trans-palmitoleic acid, metabolic risk factors, and new-onset diabetes in U.S. Adults. Ann. Intern. Med. 153, 790–799. doi: 10.1059/0003-4819-153-12-201012210-00005
Mozaffarian D., Katan M. B., Ascherio A., Stampfer M. J., Willett W. C. (2006). Trans fatty acids and cardiovascular disease. Obstet. Gynecol. Surv. 61, 525–526. doi: 10.1097/01.ogx.0000228706.09374.e7
Mutsvangwa T., Hobin M. R., Gozho G. N. (2012). Effects of method of barley grain processing and source of supplemental dietary fat on duodenal nutrient flows, milk fatty acid profiles, and microbial protein synthesis in dairy cows. J. Dairy Sci. 95, 5961–5977. doi: 10.3168/jds.2012-5491
Oteng A. B., Loregger A., van Weeghel M., Zelcer N., Kersten S. (2019). Industrial trans fatty acids stimulate SREBP2-mediated cholesterogenesis and promote non-alcoholic fatty liver disease. Mol. Nutr. Food Res. 63. doi: 10.1002/mnfr.201900385
Penedo L. A., Nunes J. C., Gama M.A.Ô.S., Leite P. E. C., Quirico-Santos T. F., Torres A. G. (2013). Intake of butter naturally enriched with cis9,trans11 conjugated linoleic acid reduces systemic inflammatory mediators in healthy young adults. J. Nutr. Biochem. 24, 2144–2151. doi: 10.1016/j.jnutbio.2013.08.006
Pietinen P., Ascherio A., Korhonen P., Hartman A. M., Willett W. C., Albanes D., et al. (1997). Intake of fatty acids and risk of coronary heart disease in a cohort of Finnish men. The Alpha-Tocopherol, Beta-Carotene Cancer Prevention Study. Am. J. Epidemiol. 145, 876–887. doi: 10.1093/oxfordjournals.aje.a009047
Prada M., Wittenbecher C., Eichelmann F., Wernitz A., Kuxhaus O., Kroger J., et al. (2022). Plasma industrial and ruminant trans fatty acids and incident type 2 diabetes in the EPIC-potsdam cohort. Diabetes Care 45, 845–853. doi: 10.2337/dc21-1897
Radtke T., Schmid A., Trepp A., Dähler F., Coslovsky M., Eser P., et al. (2017). Short-term effects of trans fatty acids from ruminant and industrial sources on surrogate markers of cardiovascular risk in healthy men and women: A randomized, controlled, double-blind trial. Eur. J. Prev. Cardiol. 24, 534–543. doi: 10.1177/2047487316680691
Risérus U., Vessby B., Ärnlöv J., Basu S. (2004). Effects of cis-9, trans-11 conjugated linoleic acid supplementation on insulin sensitivity, lipid peroxidation, and proinflammatory markers in obese men. Am. J. Clin. Nutr. 80, 279–283. doi: 10.1093/ajcn/80.2.279
Rosemond R. (2021). Impacts of Feeding Monensin to High Producing Dairy Cattle fed a Contemporary California Diet. UC Davis. ProQuest ID: Rosemond_ucdavis_0029M_20367.
Sacks F. M., Lichtenstein A. H., Wu J. H. Y., Appel L. J., Creager M. A., Kris-Etherton P. M., et al. (2017). Dietary fats and cardiovascular disease: A presidential advisory from the American Heart Association. Circulation 136, e1–e23. doi: 10.1161/CIR.0000000000000510
Samková E., Špička J., Pešek M., Pelikánová T., Hanuš O. (2012). Animal factors affecting fatty acid composition of cow milk fat: A review. S. Afr. J. Anim. Sci. 42, 83–100. doi: 10.4314/sajas.v42i2.1
Scollan N. D., Price E. M., Morgan S. A., Huws S. A., Shingfield K. J. (2017). Can we improve the nutritional quality of meat? Proc. Nutr. Soc 76, 603–618. doi: 10.1017/S0029665117001112
Stender S. (2015). In equal amounts, the major ruminant trans fatty acid is as bad for LDL cholesterol as industrially produced trans fatty acids, but the latter are easier to remove from foods. Am. J. Clin. Nutr. 102, 1301–1302. doi: 10.3945/ajcn.115.123646
Stender S., Astrup A., Dyerberg J. (2008). Ruminant and industrially produced trans fatty acids: Health aspects. Food Nutr. Res. 52. doi: 10.3402/fnr.v52i0.1651
Tardy A. L., Giraudet C., Rousset P., Rigaudière J. P., Laillet B., Chalancon S., et al. (2008). Effects of trans MUFA from dairy and industrial sources on muscle mitochondrial function and insulin sensitivity. J. Lipid Res. 49, 1445–1455. doi: 10.1194/jlr.M700561-JLR200
Tholstrup T., Raff M., Basu S., Nonboe P., Sejrsen K., Straarup E. M. (2006). Effects of butter high in ruminant trans and monounsaturated fatty acids on lipoproteins, incorporation of fatty acids into lipid classes, plasma C-reactive protein, oxidative stress, hemostatic variables, and insulin in healthy young men. Am. J. Clin. Nutr. 83, 237–243. doi: 10.1093/ajcn/83.2.237
Tricon S., Burdge G. C., Jones E. L., Russell J. J., El-Khazen S., Moretti E., et al. (2006). Effects of dairy products naturally enriched with cis-9,trans-11 conjugated linoleic acid on the blood lipid profile in healthy middle-aged men. Am. J. Clin. Nutr. 83, 744–753. doi: 10.1093/ajcn/83.4.744
Turpeinen A. M., Mutanen M., Aro A., Salminen I., Basu S., Palmquist D. L., et al. (2002). Bioconversion of vaccenic acid to conjugated linoleic acid in humans. Am. J. Clin. Nutr. 76, 504–510. doi: 10.1093/ajcn/76.3.504
USDA (2020). Dietary guidelines for american–2025. (Washington, D.C., U.S.: U.S. Department of Health and Human Services). doi: 10.1177/21650799211026980
Vahmani P., Mapiye C., Prieto N., C.Rolland D., A.McAllister T., L.Aalhus J., et al. (2015). The scope for manipulating the polyunsaturated fatty acid content of beef: a review. J. Anim. Sci. Biotechnol. 6. doi: 10.1186/s40104-015-0026-z
Vahmani P., Ponnampalam E. N., Kraft J., Mapiye C., Bermingham E. N., Watkins P. J., et al. (2020). Bioactivity and health effects of ruminant meat lipids. Invited Review. Meat Sci. 165, 108114. doi: 10.1016/j.meatsci.2020.108114
Vahmani P., Rolland D. C., Mapiye C., Dunne P. G., Aalhus J. L., Juarez M., et al. (2017). Increasing desirable polyunsaturated fatty acid concentrations in fresh beef intramuscular fat. CAB Rev. Perspect. Agric. Vet. Sci. Nutr. Nat. Resour. 12. doi: 10.1079/PAVSNNR201712020
Verneque B. J. F., MaChado A. M., de Abreu Silva L., Lopes A. C. S., Duarte C. K. (2022). Ruminant and industrial trans-fatty acids consumption and cardiometabolic risk markers: A systematic review. Crit. Rev. Food Sci. Nutr. 62, 2050–2060. doi: 10.1080/10408398.2020.1836471
Wanders J. A., Zock L. P., Brouwer A. I. (2017). Trans fat intake and its dietary sources in general populations worldwide: A systematic review. Nutrients 9. doi: 10.3390/nu9080840
Wang X., England A., Sinclair C., Merkosky F., Chan C. B. (2019). Trans-11 vaccenic acid improves glucose homeostasis in a model of type 2 diabetes by promoting insulin secretion via GPR40. J. Funct. Foods 60. doi: 10.1016/j.jff.2019.06.012
Wang X., Gupta J., Kerslake M., Rayat G., Proctor S. D., Chan C. B. (2016). Trans-11 vaccenic acid improves insulin secretion in models of type 2 diabetes in vivo and in vitro. Mol. Nutr. Food Res. 60, 846–857. doi: 10.1002/mnfr.201500783
Wang Y., Jacome-Sosa M. M., Ruth M. R., Lu Y., Shen J., Reaney M. J., et al. (2012). The intestinal bioavailability of vaccenic acid and activation of peroxisome proliferator-activated receptor-α and -γ in a rodent model of dyslipidemia and the metabolic syndrome. Mol. Nutr. Food Res. 56, 1234–1246. doi: 10.1002/mnfr.201100517
Wang Y., Lu J., Ruth M. R., Goruk S. D., Reaney M. J., Glimm D. R., et al. (2008). Trans-11 vaccenic acid dietary supplementation induces hypolipidemic effects in JCR:LA-cp rats. J. Nutr. 138, 2117–2122. doi: 10.3945/jn.108.091009
Werner L. B., Hellgren L. I., Raff M., Jensen S. K., Petersen R. A., Drachmann T., et al. (2013). Effects of butter from mountain-pasture grazing cows on risk markers of the metabolic syndrome compared with conventional Danish butter: A randomized controlled study. Lipids Health Dis. 12. doi: 10.1186/1476-511X-12-99
Keywords: conjugated linoleic acid, trans vaccenic acid, trans palmitoleic acid, type 2 diabetes, ruminant fats
Citation: Xu Y, Dugan MER, Mapiye C and Vahmani P (2023) Health effects of ruminant trans fatty acids with emphasis on type 2 diabetes. Front. Anim. Sci. 4:1278966. doi: 10.3389/fanim.2023.1278966
Received: 17 August 2023; Accepted: 20 October 2023;
Published: 06 November 2023.
Edited by:
Jeff Wood, University of Bristol, United KingdomReviewed by:
Valerie Berthelot, AgroParisTech Institut des Sciences et Industries du Vivant et de L’environnement, FranceHongGu Lee, Konkuk University, Republic of Korea
Copyright © 2023 Xu, Dugan, Mapiye and Vahmani. This is an open-access article distributed under the terms of the Creative Commons Attribution License (CC BY). The use, distribution or reproduction in other forums is permitted, provided the original author(s) and the copyright owner(s) are credited and that the original publication in this journal is cited, in accordance with accepted academic practice. No use, distribution or reproduction is permitted which does not comply with these terms.
*Correspondence: Payam Vahmani, cHZhaG1hbmlAdWNkYXZpcy5lZHU=