- 1Department of Animal Science, Universidade do Estado de Santa Catarina, Chapecó, Brazil
- 2Institute of Animal Science, Beef Cattle Research Center, Sertãozinho, Brazil
- 3Yessinergy do Brasil Agroindustrial LTDA, Campinas, Brazil
- 4Department of Animal Sciences, Washington State University, Pullman, WA, United States
Introduction: The study aimed to assess yeast-based additives' effects, as monensin alternatives, on rumen fermentation parameters, greenhouse gas emissions, and ruminal kinetics of ruminant diets using an in vitro system. Three experiments were conducted, each individually evaluating escalating levels of three yeast-based additives.
Methods: Three experiments were designed: Experiment 1 evaluated prebiotic blend 1—yeast culture [Saccharomyces cerevisiae (Scer)], beta-glucans, fructooligosaccharides, galactooligosaccharides, and mannanoligosaccharides; Experiment 2 investigated prebiotic blend 2—beta-glucan fractions and mannanoligosaccharides from Scer; Experiment 3 examined yeast cells—hydrolyzed, inactivated, and spray-dried yeast (Scer) cells. Uniform experimental design and procedures were employed across the three experiments. Each experiment had six treatments: monensin (Rumensin®, 25 mg/kg DM) as positive control, and yeast additive levels (0, 533, 1,067, 1,600, and 2,133 mg/kg on DM basis) added to ruminant diets (60% corn silage and 40% concentrate). An in vitro gas production (GP) system with 50 AnkomRF bottles assessed total GP (at 24 and 48 hours), kinetics, fermentation profiles, methane (CH4), and carbon dioxide (CO2) concentrations. Comparison with monensin utilized Dunnett’s test (5%). Yeast additive levels were analyzed for linear and quadratic responses.
Results: In Experiment 1, the 1,600 mg/kg yeast additive had lower concentrations of propionate, isobutyrate, valerate, and branched-chain volatile fatty acids (BCVFAs), and a higher acetate concentration and acetate-to-propionate ratio than monensin. In Experiment 2, the 1,600 mg/kg yeast additive led to lower total VFA and isovalerate concentrations than monensin. Additionally, compared to the 1,067 mg/kg yeast additive, monensin showed lower isovalerate concentration and higher NH3-N concentration. In Experiment 3, the 533 mg/kg yeast additive resulted in lower valerate and BCVFA concentrations, and higher CH4 and CO2 concentrations than monensin. Monensin had lower total VFA, butyrate, and acetate-to-propionate ratio, and higher propionate concentration compared to the 2,133 mg/kg yeast additive.
Discussion: Collectively, these findings suggest yeast-based additives could be monensin alternatives, enhancing animal nutrient utilization efficiency and contributing to improved livestock sustainability.
Introduction
Nutrition plays an essential role in the productivity and sustainability of beef and dairy production systems (Montes et al., 2013; Honan et al., 2021). The utilization of feed additives is one of the most widely used nutritional strategies in ruminant nutrition to enhance productivity (Azzaz et al., 2015). These additives modify the ruminal fermentation process to improve feed fermentation, increase host energy supply, reduce enteric methane (CH4) production, and, consequently, enhance animal feed utilization (Jia et al., 2018; Vyas et al., 2018). In this regard, the ionophore monensin has been used for decades in ruminant nutrition and its effects to improve animal feed efficiency is well documented in the literature (Tedeschi et al., 2003; Azzaz et al., 2015). Monensin improves rumen fermentation efficiency by increasing propionate production at the expense of acetate, resulting in a decrease of substrates [i.e., hydrogen (H2) and carbon dioxide (CO2)] for CH4 production (Rook, 2005; Benedeti et al., 2018). Furthermore, the use of monensin also inhibits the growth of lactic acid-producing bacteria, thus decreasing the acidosis risk (Ipharraguerre and Clark, 2003). However, there is a concern that the use of antimicrobials in animal feed might leave residues in animal products, which in turn leads to some bacterial strains becoming resistant to said antimicrobials (Vikram et al., 2017). Thus, due to government regulations and public concern, since January 2006, the European Union has suspended the use of antibiotic ionophores in animal feed. Therefore, there is an ongoing search for alternative additives with similar efficacy to ionophores, such as yeast-based products.
Yeast-based prebiotics are used with the aim of increasing the rumen’s beneficial bacterial population (Chaucheyras-Durand et al., 2012). In addition, these additives can potentially improve rumen fermentation and animal performance by improving feed degradation and nutrient use by microorganisms without threatening human health (Jia et al., 2018). Furthermore, yeasts do not play an antibiotic role, but provide favorable conditions for the better development of specific bacterial groups. Moreover, yeasts act as oxygen sinks, which favor strictly anaerobic bacteria, such as cellulolytic bacteria (McGinn et al., 2004). In addition, yeast cell walls are composed of polysaccharides, which can interact with bacteria, binding to them and preventing the attachment of harmful microorganisms to the animal gastrointestinal tract (Kogan and Kocher, 2007; Herrera, 2012). Therefore, these natural additives have the potential to be a more secure replacement for monensin when used to improve ruminal fermentation. Indeed, research has demonstrated that yeast-based additives can enhance feed digestibility and nutrient utilization in beef cattle (Batista et al., 2022).
Natural bioactive molecules, such as oligosaccharides and beta-glucans, have also demonstrated the potential to be used in animal nutrition due to their antioxidant, immunostimulant, antibacterial, and metabolic regulatory activities (Krüger and van der Werf, 2018; Li et al., 2018). Thus, these molecules could be used as monensin alternatives to improve ruminal fermentation patterns. Indeed, researchers observed that beta-glucans can improve fiber digestibility and increase volatile fatty acid (VFA) production by modulating rumen fermentation (Grove et al., 2006). However, the additives used in combination with these molecules, with and without yeast cultures, have not been evaluated and compared with monensin; therefore, the ideal dosages of these additives are not well established in ruminant nutrition. Thus, this study aimed to evaluate the effects of yeast-based additives, as alternatives to monensin, on rumen fermentation parameters and ruminal kinetics of ruminant diets using in vitro systems. Our hypothesis was that yeast-based additives would enhance nutrient utilization and could potentially serve as a suitable alternative to monensin, acting as beneficial modulators of ruminal fermentation.
Materials and methods
Experimental designs and chemical analysis
We conducted three experiments, aiming that each would serve as an evaluation of three yeast-based additives. The experiments were as follows: experiment 1, prebiotic blend 1—yeast culture [Saccharomyces cerevisiae (Scer)], beta-glucans, fructooligosaccharides, galactooligosaccharides, and mannanoligosaccharides (Golf®; Yessinergy LTDA, Campinas, SP, Brazil). This blend consisted of a mixture of a minimum of 210 g/kg of glucomannans, 150 g/kg of beta-glucans, 120 g/kg of fructooligosaccharides, 72 g/kg of galactooligosaccharides, and 60 g/kg of mannanoligosaccharides. Experiment 2, prebiotic blend 2—beta-glucan fractions and mannanoligosaccharides from Scer (GlucanMos®; Yessinergy LTDA). This blend consisted of a mixture of a minimum of 440 g/kg of glucomannans, 240 g/kg of beta-glucans, and 140 g/kg of mannanoligosaccharides. Experiment 3, yeast cells—hydrolyzed, inactivated, and spray-dried yeast (Scer) cells (BioHydro®; Yessinergy LTDA). The experimental design and procedures were identical across the three experiments. Thus, six treatments were evaluated within each experiment, with a monensin-based additive being used as the positive control [Rumensin®, 25 mg/kg dry matter (DM)] and 0, 533, 1,067, 1,600, and 2,133 mg/kg (DM basis) of yeast additive being included in the finishing beef diets. The experimental diets consisted of a composition of 60% corn silage and 40% concentrate (DM basis), formulated to fulfill the recommended nutrient requirements for beef cattle (Valadares Filho et al., 2016). The ingredients and chemical composition of the basal diet are presented in Table 1.
For all experiments, a 25-bottle automated in vitro gas production (GP) system (ANKOM Technology, Macedon, NY, USA) was utilized. The system was equipped with wireless pressure sensors, which were connected to a computer to evaluate the ruminal fermentation pattern of the tested ingredients. The treatments were evaluated through three 48-hour fermentation incubations to assess the in vitro GP profiles and ruminal fermentation parameters. Within each incubation, the treatments were individually incubated in 250-mL bottles, which were randomly arranged in the incubator. Therefore, each fermentation batch consisted of three replicates for each treatment, along with three blanks (consisting only of rumen/mineral/buffer solution), resulting in a total of 63 observations.
All ingredients utilized in these studies were finely ground using a 1-mm screen (Wiley mill; Thomson Scientific Inc.) to ensure consistency in all incubations and analyses. Subsequently, the samples underwent analysis for dry matter (method G-003/1), ash (method M-001/1), crude protein (method N-001/1), and ether extraction (EE; method G-005/1), following the procedures outlined by Detmann et al. (2012). The organic matter (OM) content was calculated as the difference between the DM and ash contents. For the neutral detergent fiber (NDF) analysis, the samples were treated with thermostable alpha-amylase, omitting sodium sulfite, in accordance with the method described by Van Soest et al. (1991), and adapted for use with the Ankom200 Fiber Analyzer (ANKOM Technology).
Ruminal fluid collection and buffer solution preparation
The rumen fluid was collected from three Nellore steers with rumen cannulas (with an average body weight of 640 kg) for this study. The steers were maintained on a total mixed diet comprising 60% corn silage and 40% concentrate, which consisted of ground corn grain, citrus pulp pellet, soybean meal, and a mineral mixture. The rumen fluid samples were collected 2 hours after feeding, with each animal contributing 2,000 mL. The collected rumen fluid was promptly filtered through four layers of cheesecloth, transferred into prewarmed (39°C) thermal bottles, and immediately transported to the laboratory (Yáñez-Ruiz et al., 2016).
The buffer mineral solutions for all experiments were prepared following the method outlined by Menke and Steingass (1988). The buffer solution was maintained at a constant temperature of 39°C in a water bath and continuously purged with nitrogen gas (N2) for 30 minutes. Resazurin was employed as a color indicator to monitor the pH and oxidation–reduction potential, ensuring proper saturation of the buffer with N2. The rumen fluid was mixed with the buffer solution in a volumetric ratio of 1: 2 (rumen fluid: buffer) under anaerobic conditions, accomplished by flushing with N2 within a water bath maintained at 39°C.
In vitro gas production
For the kinetics of gas production, VFA, and ammonia-nitrogen (NH3-N) measurements, the bottle valves of the ANKOMRF gas production system were adjusted to allow venting. Each 250-mL bottle was filled with 0.5 g of the respective diet, and deionized water was added to hydrate the samples and prevent particle dispersion. Subsequently, the bottles were inoculated with 75 mL of rumen/buffer solution, while ensuring continuous flushing of the headspace with N2. Once inoculated, the bottles were sealed and placed in an air-ventilated shaker incubator (EI-450T; ENGCO, Piracicaba, SP, Brazil) operating at a controlled temperature of 39°C and at an agitation speed of 83 rpm. A data acquisition software (gas pressure monitor; ANKOM Technology) was configured to record the cumulative pressure every 5 minutes, with data recorded at 60-minute intervals for a duration of 48 hours. The valves were programmed to automatically release gas when the pressure reached 3.4 kPa, as described by Tagliapietra et al. (2011).
The cumulative gas pressures at 24 hours and 48 hours were converted into milliliters (mL) using the conversion method described by Tagliapietra et al. (2011), as shown below:
where Pc represents the cumulative pressure change (kPa) in the headspace of the bottle; Vo denotes the volume of the bottle’s headspace (545 mL); and Po represents the atmospheric pressure recorded by the equipment at the commencement of the measurement.
The final GP volumes in the bottles were adjusted to account for the contribution of the inoculum by subtracting the final GP value obtained from the blank bottles. To obtain the total GP over time, the cumulative pressure values were adjusted using the dual-pool model proposed by Schofield et al. (1994):
where Vt represents the gas volume produced up to the specific time (mL); V1 and V2 denote the maximum gas volumes achieved from the complete digestion of each pool (mL); K1 and K2 represent the specific rates of digestion for each pool (h–1); and L signifies the lag time (h). The metabolizable energy (ME) was calculated following the method described by Menke and Steingass (1988), with the lipid content disregarded (Grings et al., 2005). The ME calculation is as follows:
where GP200 (mL/200 mg of DM incubated) represents the gas production measured at 48 hours. The pH of the solution was measured using an Accumet™ AP61 pH meter (Fisher Scientific, Atlanta, GA) at the initiation and conclusion of each 48-hour incubation period.
The in vitro OM digestibility (IVOMD) was determined using the method described by Menke and Steingass (1988), which is expressed as follows:
where GP200 represents the net gas production (mL/200 mg DM) measured at 24 hours and 48 hours. At the end of the fermentation batch for each experiment, the bottles were removed from the incubator and were cooled in an ice bath for 15 minutes, to stop the fermentation process. The pH of each bottle was measured (portable pH meter, model TEC-7; Tecnal, Ourinhos, SP, Brazil) and the content of the bottle was filtered through two layers of gauze. The solid content (residue) was weighed and dried at 60°C in an oven with mechanical air circulation and then weighed to determine the NDF. Subsamples of 10 mL from each bottle’s filtered content were added in Falcon tubes (15 mL) containing 0.2 mL of a 50% (v/v; volume to volume) sulfuric acid (H2SO4) solution, and centrifugated (4°C, 15 minutes, 1,000 g; centrifuge Avanti JXN-26, Beckman Coulter, Nyon, Switzerland). The supernatant was transferred to Eppendorf Tubes® (2 mL), which were centrifugated (4°C, 30 minutes, 20,000 g; Sorvall ST 8R, Thermo Fisher Scientific, Germany) for the determination of NH3-N and VFA concentrations.
Enteric carbon dioxide and methane production
For enteric CO2 and CH4 data, the AnkomRF system bottle valves were set to be closed. All other procedures and designs were the same as those used in the total GP essay. After the inoculation process, the bottles were sealed and placed in an air-ventilated shaker incubator set at a temperature of 39°C. On completion of each fermentation batch, which lasted for 48 hours, the production of CO2 and CH4 from the headspace was quantified using a gas chromatograph (Nexis GC-2030; Shimadzu) equipped with a GS-CarbonPLOT column (Agilent Technologies, Santa Clara, CA, USA). The carrier gas used was helium (999.9 mL/L). The enteric CH4 and CO2 production from the bottles was adjusted for the contribution of the inoculum by subtracting the final gas production obtained from the blank bottles. The pH of the solution was measured at the beginning and end of each 48-hour incubation using an Accumet AP61 pH meter.
Statistical analysis
In this study, we carried out two statistical comparisons: in the first, we conducted a quantitative analysis (regression) of yeast additive levels (0, 533, 1,067, 1,600, and 2,133 mg/kg) to assess the potential linear and quadratic behaviors resulting from increasing these additives; and, in the second, we conducted a qualitative analysis, comparing each additive level against monensin (positive control) to examine the effects of yeast additives relative to the ionophore.
All collected results underwent tests for residual normality and homogeneity of variance (Davis and Stephens, 1989). Data from all experiments were collected and analyzed using a randomized block design, employing mixed models that accounted for additives as fixed factors and incubation as a random factor. The average values obtained from the bottles within each incubation served as the experimental units. The non-linear (NLIN) procedure of SAS 9.4 software was utilized to estimate the fermentation rate and gas pool size. Subsequently, the parameters of the non-linear functions were compared through linear regression analysis, with differences considered statistically significant at a level of p ≤ 0.05 and trending when 0.05< p ≤ 0.10. All other variables were analyzed using the PROC MIXED function in SAS (SAS Institute, Cary, 2013). Analysis of variance was conducted, and in cases of significance, the Dunnett’s test (at 5% significance level) was employed to compare the yeast additives with the positive control (monensin). The levels of the yeast additives were examined for linear and quadratic responses using the following model:
where Yijk represents the response variable obtained from the ith level of the yeast additive in the diet of the jth incubation and the kth experimental unit. The indices i, j, and k denote the levels of inclusion of the yeast additive, the random factor of incubation, and the random factor of bottle, respectively. The regression parameters of the model are denoted as B0, B1, and B2. The Xi represents the effect of the ith level of the fixed quantitative factor (inclusion of the yeast additive), Pj represents the effect of the level of the random factor incubation, Ak represents the effect of the level of the random factor bottle, and eijkl represents the residual error, assumed to follow a normal distribution (0, s2).
Results
Experiment 1: prebiotic blend 1
The effects of additive inclusion and monensin on ruminal fermentation variables in GP systems are presented in Table 2 and Figure 1. In comparing the yeast additive with monensin, we found that there was no difference in the production levels of total GP (at 24 hours and 48 hours), ME, IVOMD, CH4, and CO2, and in the final pH. In addition, the concentrations of total VFAs and butyrate did not differ among treatments (p > 0.10). The 1,600 mg/kg (DM basis) of yeast additive included had lower concentrations of propionate (p = 0.02), isobutyrate (p = 0.05), valerate (p = 0.01), and BCVFAs (p = 0.05) and a higher acetate concentration (p = 0.05), acetate-to-propionate ratio (p = 0.02) than monensin. Monensin also had a higher acetate (p = 0.02) and lower isovalerate concentration (p = 0.02) than the treatment without the additives. The 1,067 mg/kg of yeast included also tended to have a lower NH3-N concentration than monensin (p = 0.07). Monensin diets had a lower lag time and degradation rate of the second pool than all levels of the yeast additive (p< 0.05).
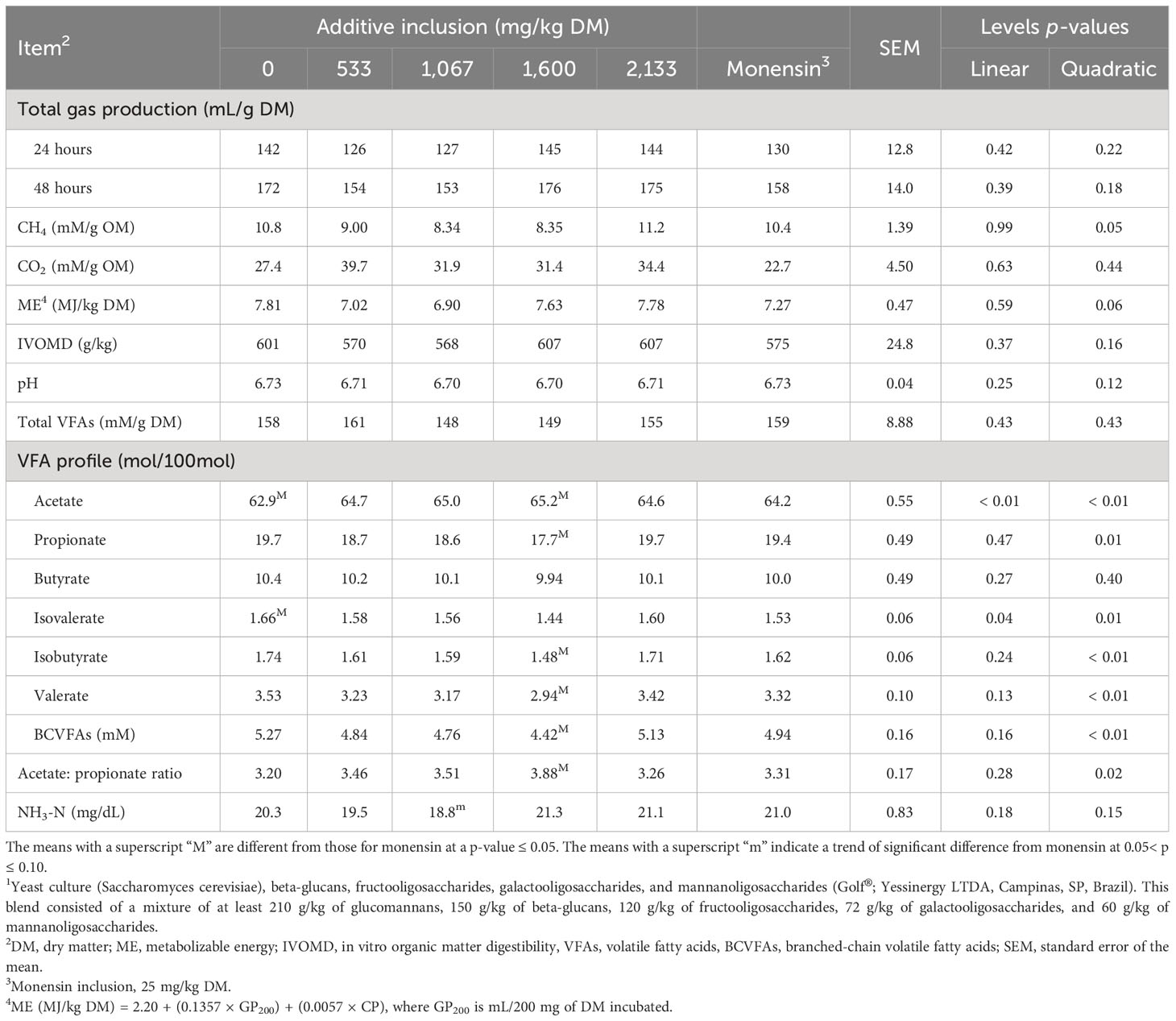
Table 2 Effects of yeast additive (prebiotic blend 11) inclusion levels and monensin on ruminal fermentation variables in the gas production systems (experiment 1).
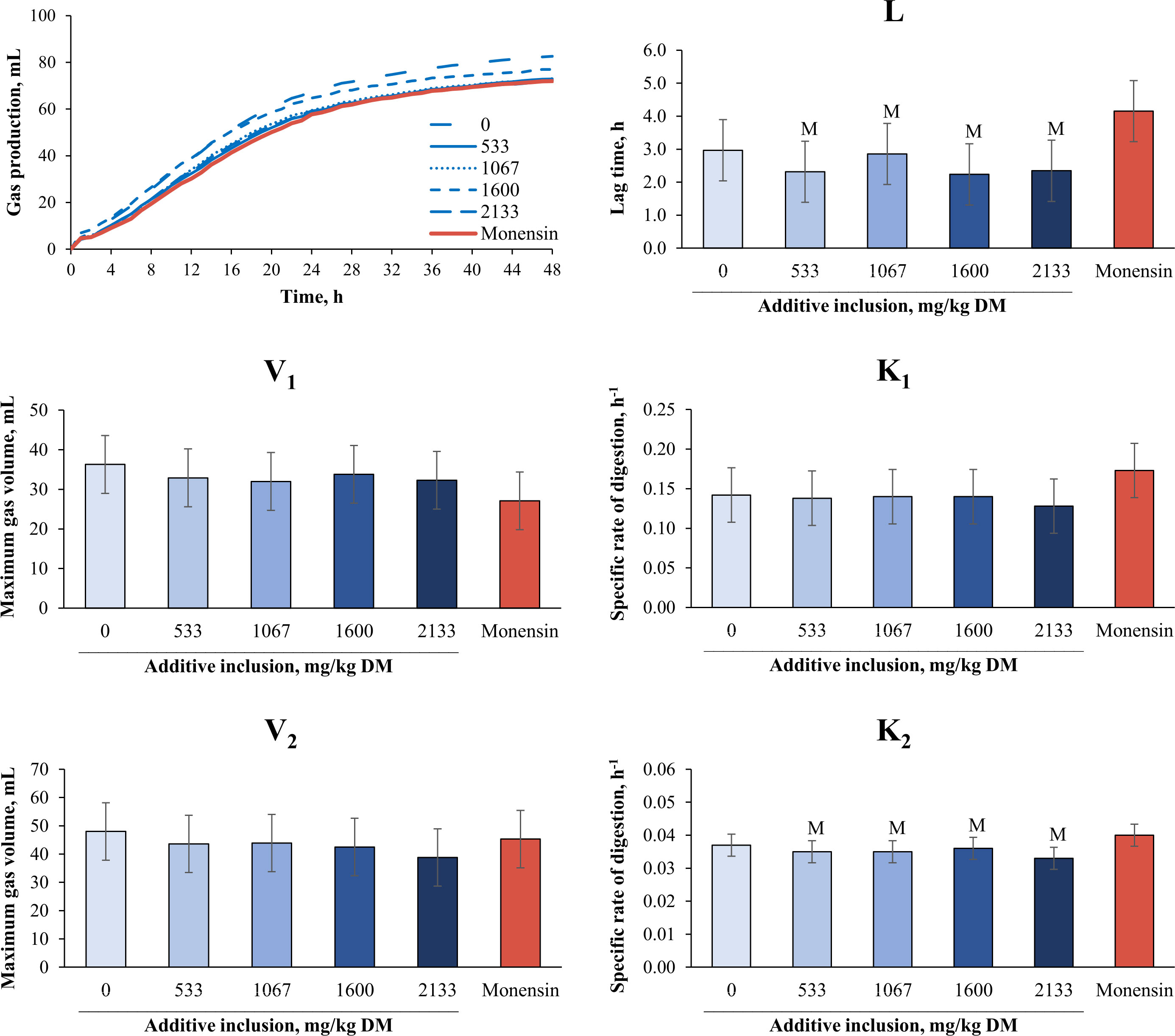
Figure 1 Effects of yeast additive (prebiotic blend 11) inclusion and monensin on in vitro kinetic variables2 of gas production in experiment 1. The means with a superscript “M” were different from those for monensin at a p-value ≤ 0.05. The means with a superscript “m” indicate a trend of significant difference from monensin at 0.05< p ≤ 0.10. The symbol “Ƚ” indicates a linear effect and the symbol “ɋ” indicates a quadratic effect for the levels of additive inclusion (p ≤ 0.05). 1Yeast culture (Saccharomyces cerevisiae), beta-glucans, fructooligosaccharides, galactooligosaccharides, and mannanoligosaccharides (Golf®; Yessinergy LTDA, Campinas, SP, Brazil). This blend consisted of a mixture of at least 210 g/kg of glucomannans, 150 g/kg of beta-glucans, 120 g/kg of fructooligosaccharides, 72 g/kg of galactooligosaccharides, and 60 g/kg of mannanoligosaccharides. 2V1 and V2 = maximum gas volume of each pool (mL); K1 and K2 = specific rate of digestion of each pool (h–1); and L = lag time (h).
Regarding levels regression, ME (trend, p = 0.06), CH4 production (p = 0.05), acetate (p< 0.01), propionate (p = 0.01), isovalerate (p = 0.01), isobutyrate (p< 0.01), valerate (p< 0.01), and BCVFA (p< 0.01) concentrations, and the acetate-to-propionate ratio (p = 0.02) responded quadratically. The 1,600 mg/kg (DM basis) of yeast additive included had the lowest concentrations of propionate, isovalerate, isobutyrate, valerate, and BCVFAs and the highest acetate concentration and acetate-to-propionate ratio. In addition, the 1,067 mg/kg (DM basis) of yeast additive included had the lowest CH4 concentration and ME. The remaining variables were not affected by additive inclusion (p > 0.05).
Experiment 2: prebiotic blend 2
The effects of additive inclusion and monensin on ruminal fermentation variables in GP systems are presented in Table 3 and Figure 2. In comparing the yeast additive with monensin, we found that the latter had lower total VFA (trend, p = 0.08), isovalerate (p = 0.03), and maximum gas volume of the first pool (trend, p = 0.08) concentrations than the 1,600 mg/kg (DM basis) of the yeast additive included. In addition, monensin had a lower isovalerate concentration (p = 0.04) and higher NH3-N (p< 0.01) and maximum gas volume of the second pool (trend, p = 0.08) concentrations than the 1,067 mg/kg (DM basis) of yeast additive included. The 2,133 mg/kg (DM basis) of yeast additive included had a lower NH3-N concentration (trend, p = 0.06) and lag time (p = 0.03), and higher concentrations of butyrate (p = 0.06), isovalerate (p = 0.08), and maximum gas volume of the first pool (p = 0.04) than monensin. Monensin had higher degradation rates in the first and second pools than all levels of the yeast additive (p< 0.05). Finally, the treatment without additives had higher concentrations of butyrate (p = 0.01), isovalerate (p< 0.01), isobutyrate (p = 0.04), valerate (p = 0.04), and BCVFAs (p = 0.05) and a lower acetate concentration (p< 0.01) than monensin. There was no difference in the remaining variables among the treatments (p > 0.10).
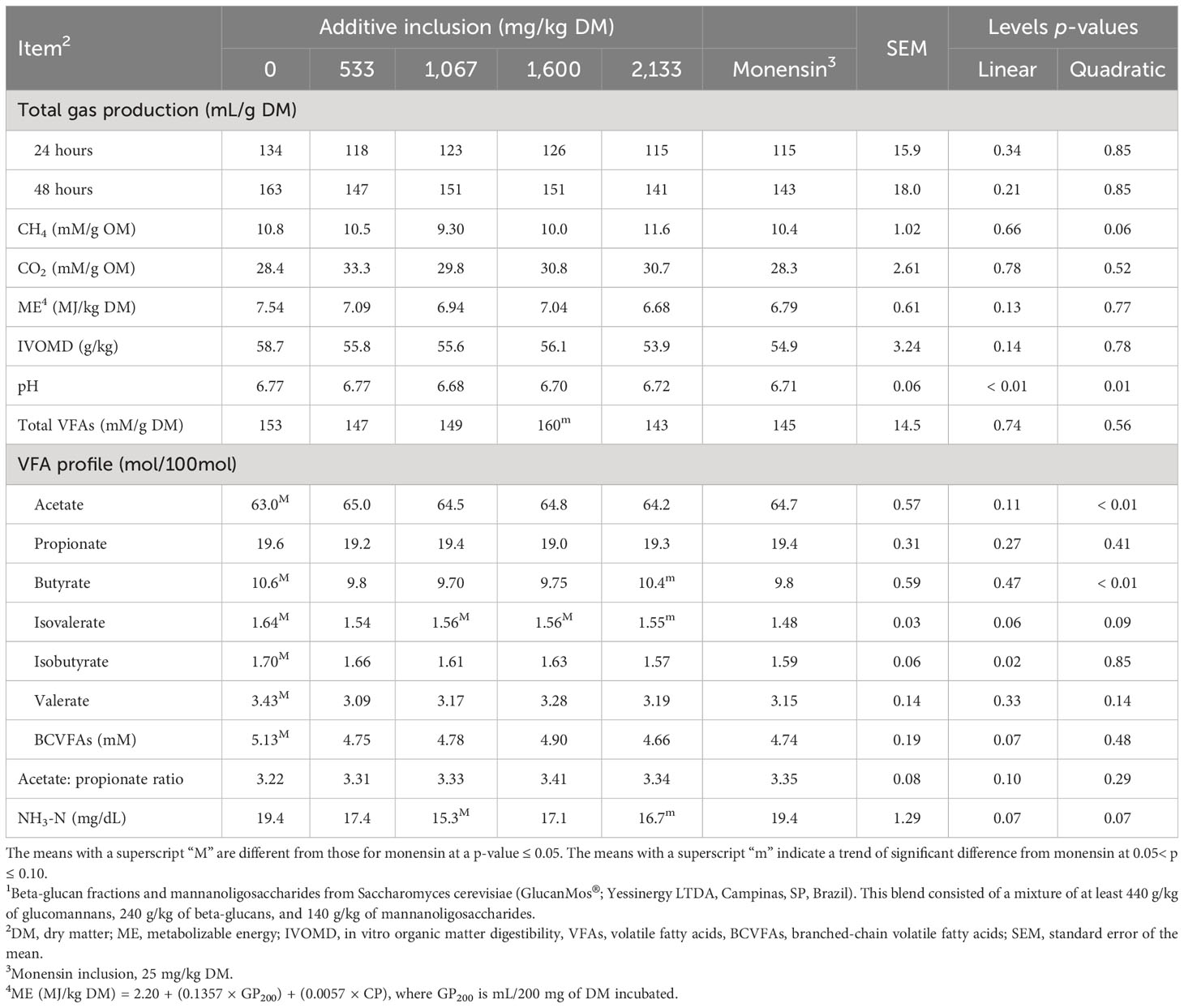
Table 3 Effects of yeast additive (prebiotic blend 21) inclusion and monensin on ruminal fermentation variables in the gas production systems (experiment 2).
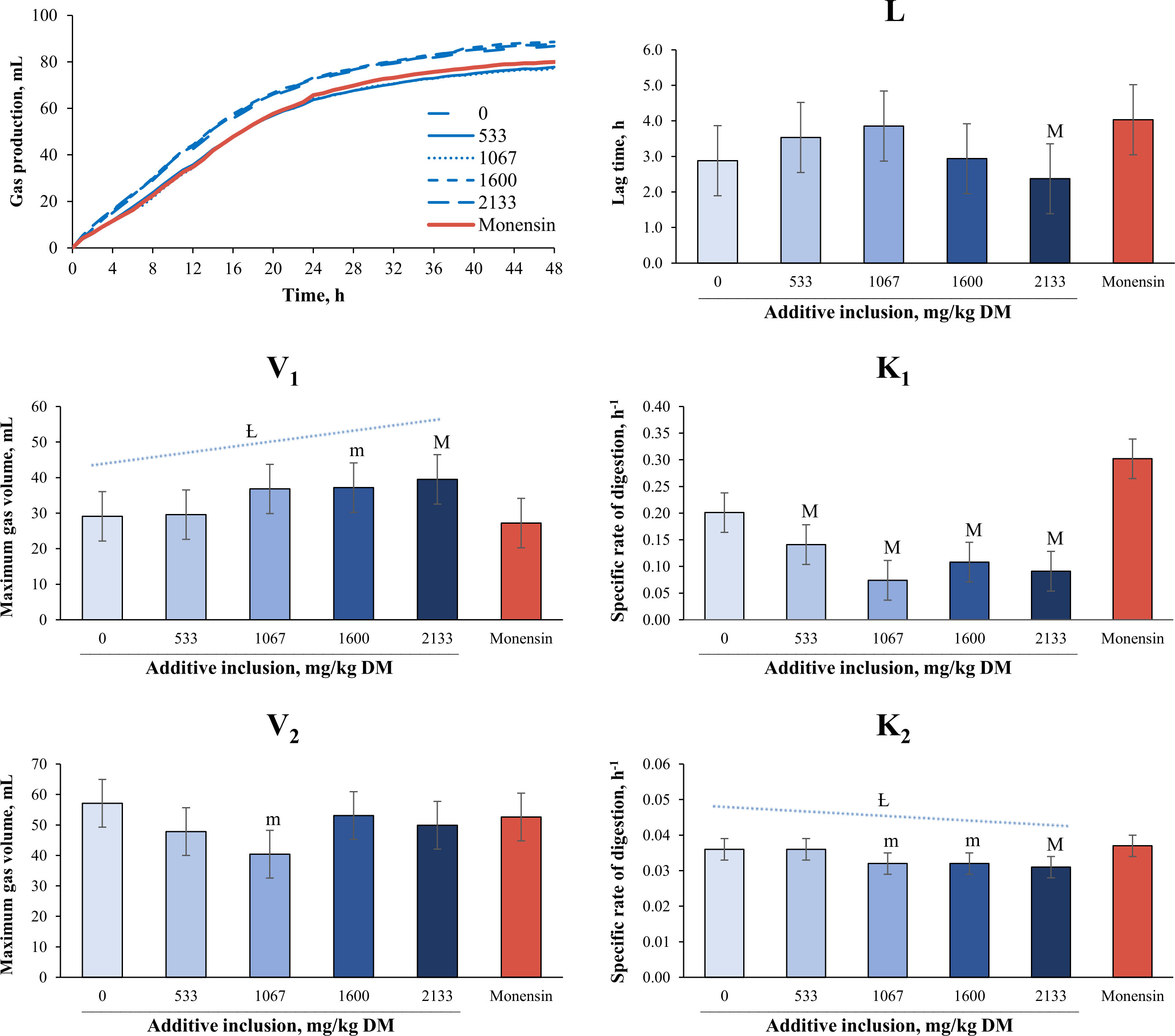
Figure 2 Effects of yeast additive (prebiotic blend 21) inclusion and monensin on in vitro kinetic variables2 of gas production in experiment 2. The means with a superscript “M” are different from those for monensin at a p-value ≤ 0.05. The means with a superscript “m” indicate a trend of significant difference from monensin at 0.05< p ≤ 0.10. The symbol “Ƚ” indicates linear effect and the symbol “ɋ” indicates a quadratic effect for the levels of additive inclusion (p ≤ 0.05). 1Beta-glucan fractions and mannanoligosaccharides from Saccharomyces cerevisiae (GlucanMos®; Yessinergy LTDA, Campinas, SP, Brazil). This blend consisted of a mixture of at least 440 g/kg of glucomannans, 240 g/kg of beta-glucans, and 140 g/kg of mannanoligosaccharides. 2V1 and V2 = maximum gas volume of each pool (mL); K1 and K2 = specific rate of digestion of each pool (h–1); and L = lag time (h).
For levels of regression, the final pH (p = 0.01), CH4 (trend, p = 0.06), acetate (p< 0.01), butyrate (p< 0.01) isovalerate (trend, p = 0.09), and NH3-N (trend, p = 0.07) concentrations responded quadratically. The 1,067 mg/kg (DM basis) of yeast additive included had the lowest final pH, CH4, butyrate, and NH3-N concentrations. In addition, the 533 mg/kg (DM basis) of yeast additive included had the lowest isovalerate concentration and highest acetate concentration. The maximum gas volume of the first pool linearly increased (p = 0.04), and the degradation rate of the second pool linearly decreased (p = 0.02) with the inclusion of the yeast additives. The remaining variables were not affected by additive inclusion (p > 0.05).
Experiment 3: prebiotic blend 3
The effects of additive inclusion and monensin on ruminal fermentation variables in GP systems are presented in Table 4 and Figure 3. In comparing the yeast additive with monensin, we found that there was no difference in the total GP (at 24 hours and 48 hours), ME, IVOMD, and NH3-N concentrations among the treatments (p > 0.10). The 533 mg/kg (DM basis) of yeast additive included had lower concentrations of valerate (p = 0.02) and BCVFAs (p< 0.05), and higher CH4 (p = 0.03) and CO2 (p< 0.01) concentrations than monensin. The 1,067 mg/kg (DM basis) of yeast additive included had a lower propionate concentration (trend, p = 0.09) and a higher CO2 (p< 0.01) concentration and acetate-to-propionate ratio (trend, p = 0.07) than monensin. In addition, monensin tended to have lower CO2 production than the 1,600 mg/kg (DM basis) of yeast additive included (p = 0.09). Monensin also had lower total VFA (trend, p = 0.10), butyrate (trend, p = 0.08), and isovalerate (trend, p = 0.07) concentrations, in addition to a higher acetate-to-propionate ratio (p = 0.01), and propionate concentration (p< 0.01) than the 2,133 mg/kg (DM basis) of yeast additive included. Monensin also had a higher acetate concentration (p< 0.01) and acetate-to-propionate ratio (p = 0.01), and lower butyrate (p = 0.01), isovalerate (p< 0.01), isobutyrate (p< 0.01), BCVFA (p = 0.04), and valerate (p = 0.04) concentrations than the treatment without additives. Finally, all the yeast additive treatments had a higher maximum gas volume in the first pool and lower lag time, degradation rate, and maximum gas volume in the second pool than monensin (p< 0.05).
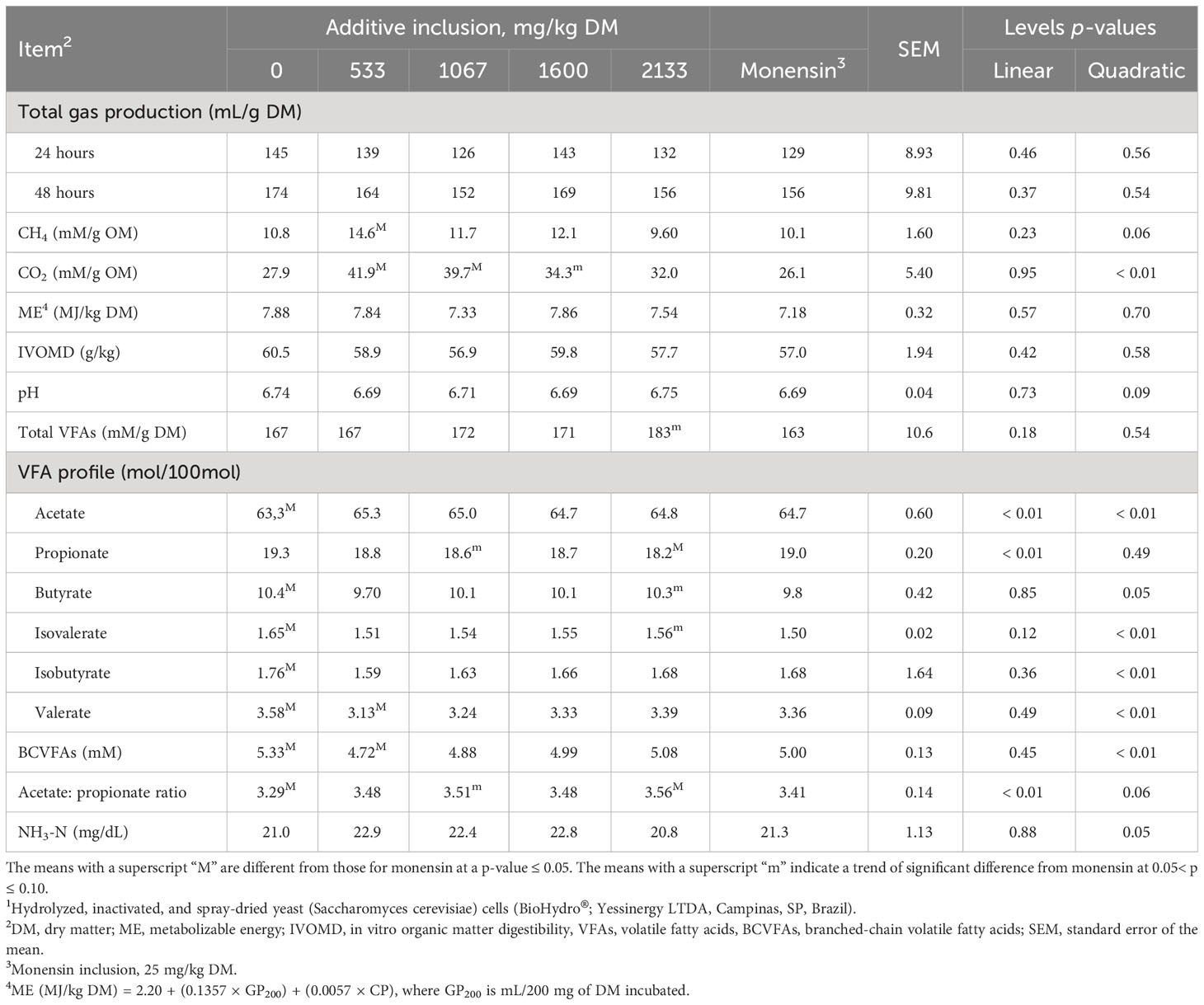
Table 4 Effects of yeast additive (yeast cells1) inclusion and monensin on ruminal fermentation variables in the gas production systems (experiment 3).
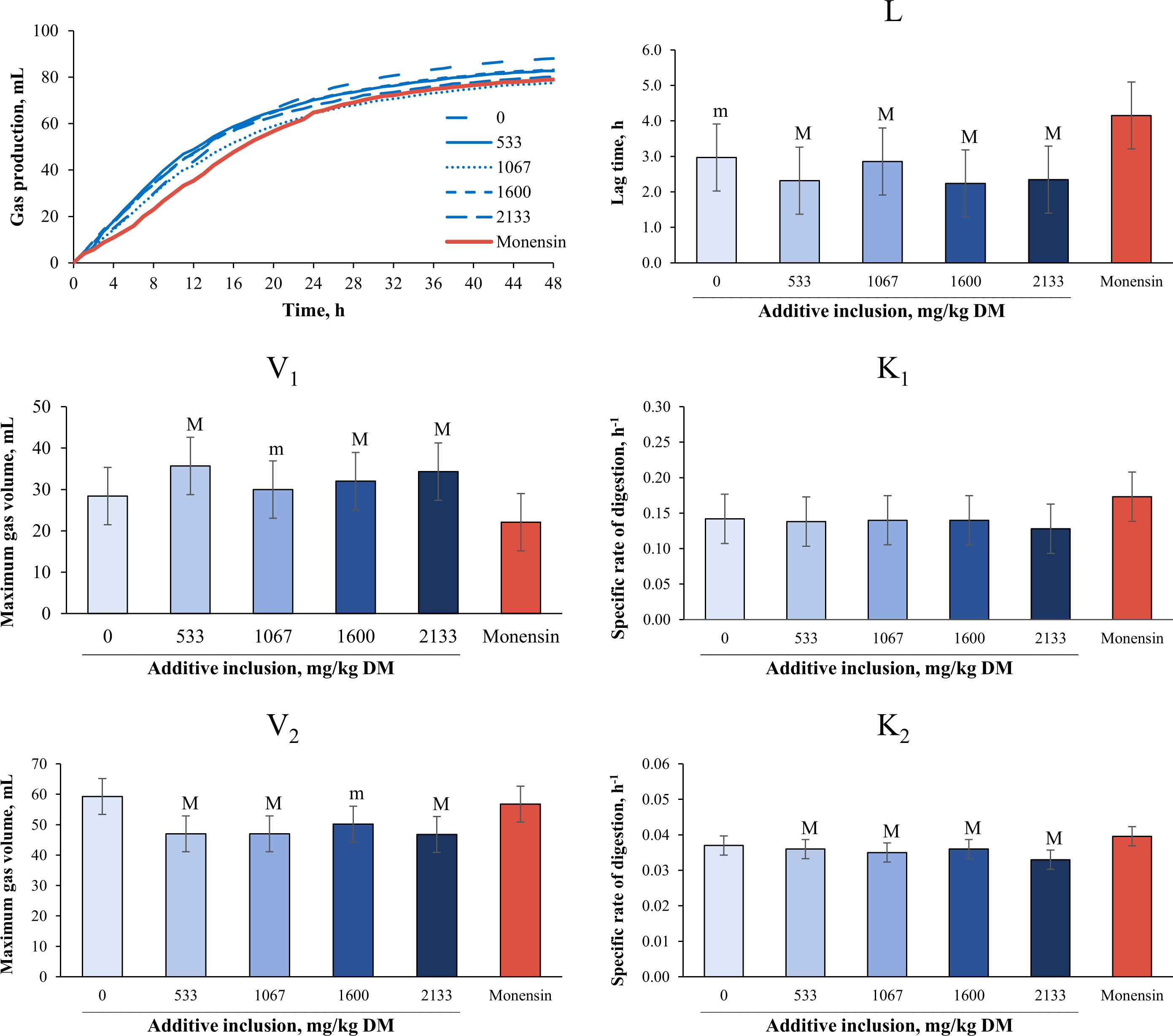
Figure 3 Effects of yeast additive (yeast cells1) inclusion and monensin on in vitro kinetic variables2 of gas production in experiment 3. The means with a superscript “M” are different from those for monensin at a p-value ≤ 0.05. The means with superscript “m” indicate a trend of significant difference from monensin at 0.05< p ≤ 0.10. The symbol “Ƚ” indicates linear effect and the symbol “ɋ” indicates a quadratic effect for the levels of additive inclusion (p ≤ 0.05). 1Hydrolyzed, inactivated, and spray-dried yeast (Saccharomyces cerevisiae) cells (BioHydro®; Yessinergy LTDA, Campinas, SP, Brazil). 2V1 and V2 = maximum gas volume of each pool (mL); K1 and K2 = specific rate of digestion of each pool (h–1); and L = lag time (h).
Regarding additive levels, the inclusion of the yeast additive linearly decreased the propionate concentration (p< 0.01) and linearly increased the acetate-to-propionate ratio (p< 0.01). In addition, the CH4 (trend, p = 0.06), CO2 (p< 0.01), acetate (p< 0.01), butyrate (p = 0.05), isovalerate (p< 0.01), isobutyrate (p< 0.01), valerate (p< 0.01), BCVFA (p< 0.01), and NH3-N (p = 0.05) concentrations responded quadratically. The 533 mg/kg (DM basis) of yeast additive included had the lowest butyrate, isovalerate, isobutyrate, valerate, and BCVFA concentrations, and the highest CH4, CO2, and acetate concentrations. The remaining variables were not affected by additive inclusion (p > 0.05).
Discussion
Experiment 1: prebiotic blend 1
Monensin is a common additive used in ruminant nutrition, known for its documented efficacy in enhancing rumen fermentation and feed efficiency (Tedeschi et al., 2003; Azzaz et al., 2015). However, there is a concern about the potential effects of antibiotic ionophores on the resistance of certain pathogenic bacteria (Vikram et al., 2017). On the other hand, the yeast additives might be applied to improve feed degradability, consequently leading to greater nutrient absorption (Broadway et al., 2015). Moreover, the additives used in this study are based on a mixture of prebiotics intended to increase the populations of beneficial bacteria that carry out feed degradation without causing risks to animal health (Monnerat et al., 2013). In addition, prebiotics can also reduce the levels of pathogens, such as Escherichia coli, through competitive exclusion, as both have an affinity for the same activation sites (Cross et al., 2002; Krehbiel et al., 2003). Furthermore, the composition of yeast walls promotes rumen health through the stimulation of lactic acid-utilizing bacteria growth, which creates an environment with improved pH conditions and immunomodulatory effects (Garcia et al., 2017). Therefore, we expected this prebiotic blend to have similar effects to monensin on feed degradability and the rumen fermentation patterns. Indeed, the total GP and VFA, ME, and IVOMD concentrations did not differ among treatments in the first trial. However, our results indicate that the prebiotic (at 1,600 mg/kg DM) promoted VFA profile changes (compared with monensin), with an increase in acetate concentration in exchange for propionate, valerate, and BCVFAs. The different modes of action of the additives could explain these alterations. On the one hand, monensin acts by selecting microorganisms that lead rumen fermentation to succinate and propionate (Schären et al., 2017). On the other hand, prebiotic yeast provides a ruminal environment with better conditions for anaerobic bacteria by reducing levels of O2 (Vohra et al., 2016). Anaerobic bacteria are responsible for fiber degradation, leading to higher levels of acetate production (Gharechahi and Salekdeh, 2018). Furthermore, the yeast additive used in this study is a blend rich in amino acids, which some rumen bacteria might use, leading to BCVFA production (Reynolds and Kristensen, 2008). It has also been reported that BCVFAs are related to the increase of fibrolytic bacteria (Ruminococcus albus, Ruminococcus flavefaciens, Fibrobacter succinogenes, and Butyrivibrio fibrisolvens), enzyme activity (carboxymethyl cellulose, cellobiase, and xylanase), and NDF degradation (Wang et al., 2020). These findings are in line with the higher acetate-to-propionate ratio observed in our study. Furthermore, these results suggest that it would be interesting to test these yeast additives in high-forage diets (especially at 1,600 mg/kg DM) and evaluate ruminal NDF fermentation in future studies.
The VFA profile changes are closely correlated with enteric greenhouse gas production (Ku-Vera et al., 2020). The propionate formation pathways in the rumen result in a modification of the overall electron balance, serving as a hydrogen sink and subsequently reducing the availability of hydrogen (Pereira et al., 2022). Furthermore, CO2 is not produced in any phase of the propionate production pathways (Benedeti et al., 2018). However, the pathways to acetate formation release two CO2 molecules and eight hydrogens from each hexose (Benedeti et al., 2018). These are substrates for CH4 production (Pereira et al., 2022). Controversially, the quadratic effects suggested that the yeast additive at levels of 1,067 and 1,600 mg/kg (DM basis) had the highest acetate concentration and the lowest CH4 production. Greenhouse enteric gas emissions are undesirable from both environmental and productive perspectives. The lost carbons in CH4 and CO2 forms could be used for carbohydrate, lipid, or amino acid building (Tiago et al., 2016; Li et al., 2019). As stated by Elghandour et al. (2014), the presence of yeast additives may induce a competition for co-metabolizing H2 between acetogenic and methanogenic microorganisms. Previous studies also observed CH4 reduction when the yeast additives were evaluated in rumen fermentation (Polyorach et al., 2014; Pedraza-Hernández et al., 2019). Thus, the CH4 reduction in our study could be an indication of better nutrient utilization.
We hypothesized that diets with yeast additives included could have similar rumen kinetics to those with monensin included. Interestingly, we observed that the prebiotic reduced the required time to start fermentation. Others have also observed a reduction in the DM lag time when yeast based on S. cerevisiae were tested in both in vivo and in vitro trials (Williams et al., 1991; Pedraza-Hernández et al., 2019). According to the aforementioned authors, the utilization of S. cerevisiae-based additives has the potential to enhance the initial rate of fiber degradation in the rumen. Thus, this effect can be attributed to the presence of macromolecules within the yeast that stimulate the growth and activity of fibrolytic microorganisms. However, contradicting our hypothesis, monensin promoted a higher degradation rate of the second pool, which might be related to the fermentation of slower degraded components, such as fiber. Nevertheless, this higher degradation rate of the second pool for monensin should be the reason for the similar total GP observed for all treatments at the end of the fermentation baths.
Likewise, in the BCVFA results, part of ruminal NH3-N comes from protein degradation (Refat et al., 2015). Furthermore, some rumen fibrolytic microorganisms can build their amino acids from NH3-N and carbon skeletons (Reynolds and Kristensen, 2008). Hence, we speculate that the reduced NH3-N levels observed in the yeast additive group, at a dose of 1,067 mg/kg (DM basis), indicate that there is a greater conversion of NH3-N into microbial protein when compared with the other doses. Moreover, lower concentrations of NH3-N and higher acetate concentrations (as observed here for yeast) are also indicators of increased fibrolytic bacterial growth. However, we did not evaluate this parameter in our study. Nevertheless, despite the differences in the VFA profile and NH3-N concentration between the monensin and prebiotic blends, these additives promoted similar OM digestibility, ME values, total GP, and concentrations of VFAs. Moreover, the yeast additive also showed the potential to decrease CH4 enteric production and increase fiber fermentation, which is highly desirable from an environmental perspective.
Experiment 2: prebiotic blend 2
The findings of this second trial also confirmed our hypothesis that yeast and monensin have the same total GP, ME, and IVOMD. Moreover, in this experiment we observed that the yeast additive at 1,600 mg/kg (DM basis) had a higher total VFA concentration than monensin. To understand these results, we point out that this additive originates from S. cerevisiae strains, which have characteristics of controlling pH and removing O2, which maximizes anaerobic bacterial growth (Bach et al., 2007; Broadway et al., 2015). In regard to the VFA profile, only the concentrations for butyrate (at 2,133 mg/kg DM) and isobutyrate (at 1,067, 1,600, and 2,133 mg/kg DM) were higher for the yeast additive than monensin. Butyrate (at 2,133 mg/kg DM) and isobutyrate (at 1,067, 1,600, and 2,133 mg/kg DM) concentrations were higher for the yeast additive than monensin. However, butyrate, isobutyrate, isovalerate, and BCVFA concentrations were reduced, whereas the acetate concentration and acetate-to-propionate ratio increased when the yeast additive was added to the diet. These results are in line with those from experiment 1 and thus suggest that the yeast additives might lead to greater fibrolytic bacteria proliferation and reduced decarboxylation of branched-chain amino acids (isoleucine, leucine, and valine), which in turn indicates that the addition of the additive at intermediate doses led to a lower degradation of these amino acids (Refat et al., 2015). Furthermore, it is important to emphasize that the tested additive provided an additional source of BCVFAs. Therefore, rumen bacteria did not need to degrade branched-chain amino acids for the formation of BCVFAs. As a result, a larger portion of these compounds could potentially be absorbed in the small intestine, leading to an increase in protein synthesis.
Regarding greenhouse gas production, we observed a trend of quadratic effect for CH4, with the minimum values reaching 1,067 and 1,600 mg/kg (DM basis). The ruminal CH4 production can be reduced in different ways: (1) by decreasing H2 availability (dos Santos et al., 2023); (2) by increasing H2-consuming pathways, such as the production of compounds that act as H2 sinks; or (3) by reducing the population of methanogenic Archaea. Although we did not observe propionate changes, our results suggest that the decrease in CH4 concentration was due to the better efficiency of microbial mass, which decreased the available H2 for CH4 production (Mombach et al., 2021).
Changes in fermentation pattern affected the rumen kinetics of yeast-additive diets compared with monensin. Thus, it seems that this prebiotic promoted a faster fermentation initiation that increased the total GP of the first pool (at 1,600 and 2,133 mg/kg DM), even with a lower degradation rate. Moreover, the first pool total fermentation increased with increasing levels of additive inclusion. On the other hand, monensin seems to induce a higher degradation rate of the second pool, which might have been the reason for its second pool maximum gas volume being higher than that of the treatment with 1,067 mg/kg (DM basis) of yeast additive. An interesting advantage of conducting trials that evaluate gas production (kinetics and total volume) together with fermentation parameters (such as VFA, IVOMD, and greenhouse gases) is that it is possible to follow the feed carbon destination (Nelson et al., 2009). This chemical element might be used to form essential nutrients (carbohydrates, lipids, and protein) to provide microbial energy reserves or structures (Tiago et al., 2016; Li et al., 2019). In the worst scenario, it can be released as a greenhouse gas (CO2 and CH4), which is undesirable from both environmental and production perspectives (McAllister et al., 2011). Therefore, the higher total VFA concentration and maximum gas production of the first pool (faster lag time), the reduction of NH3-N and CH4 for the yeast additives, and also the non-differences in total GP (at 24 hours and 48 hours) and fast lag time might suggest that rumen microorganisms more efficiently used carbon from these diets for their growth.
Experiment 3: prebiotic blend 3
The results of experiment 3 are indicative of the same effects as the previous trial, where the yeast additive promoted similar total GP, ME, and OM, and also higher total VFA (at 2,133 mg/kg DM) concentrations than monensin. Moreover, here we also observed changes in the VFA profile, with a higher concentration of butyrate at the expense of propionate and BCVFAs for yeast treatments, which also increased the acetate-to-propionate ratio. We also observed similar results for ruminal kinetics, finding that there was a lower lag time, degradation rate, maximum gas volume of the second pool, and higher maximum gas volume produced in the first pool for the yeast additive diets than for monensin. Thus, we could see the same fermentation pattern changes for all tested additives. However, we observed contradicting results for CH4 and CO2 production in this trial, where the yeast additives produced a greater amount of these gases than monensin. Curiously, these effects were more prominent for the lower levels, especially at 533 mg/kg (DM basis) of additive inclusion. However, these differences diminished with increasing levels of inclusion until the highest level (2,133 mg/kg DM), where greenhouse gas emissions were equivalent to those observed with monensin supplementation.
Therefore, based on our findings, we can draw the conclusion that yeast-based additives might be used as ruminal fermentation modulators to improve nutrient usage. In summary, our results have indicated promising treatment options for each additive examined. Regarding prebiotic blend 1, we highlight the inclusion of 1,600 mg/kg (DM basis). For prebiotic blend 2, 1,600 g of additive included for each kg of DM was the most promising treatment. Concerning yeast cells, the better treatment was the inclusion of 2,133 mg/kg (DM basis). However, in spite of the results found in this study, there is still a gap in the body of knowledge regarding the use of yeast-based products as feed additives to improve ruminal fermentation efficiency in the diets of ruminants. Therefore, future studies evaluating these additives on rumen fermentation parameters and animal performance are needed. Based on the results presented, we concluded that yeast-based additives possess the potential to modulate ruminal fermentation. This modulation is characterized by an increase in the total concentration of VFAs and a reduction in the concentrations of NH3-N and BCVFAs, and in CH4 production. Thus, these additives have the potential to be used as alternatives to monensin to improve nutrient utilization from feeds. In addition, the greenhouse gas results observed in this study might also indicate that yeast-based products could potentially be used to improve livestock sustainability. However, we did not observe any effects of additive inclusion on the total gas production, ME, and IVOMD in this study. Therefore, further studies are warranted to validate the findings obtained in this study and assess the effects of these additives on in vivo ruminal fermentation parameters, animal performance, and economic feasibility.
Data availability statement
The raw data supporting the conclusions of this article will be made available by the authors, without undue reservation.
Ethics statement
The animal study was approved by Animal Use Ethics Committee of the Instituto de Zootecnia under protocol n° 249-19. The study was conducted in accordance with the local legislation and institutional requirements.
Author contributions
ARC: methodology, investigation, data curation, writing (original draft), and visualization. EM: methodology, investigation, data curation, writing (review and editing), and visualization. FR: methodology, investigation, writing (review and editing), and visualization. KL: methodology, investigation, writing (review and editing), and visualization. ACC: methodology, investigation, writing (review and editing), and visualization. BA: methodology, investigation, writing (review and editing), and visualization. JS: conceptualization, resources, writing (review and editing), visualization, and funding acquisition. VS: conceptualization, resources, writing (review and editing), visualization, and funding acquisition. MM: methodology, validation, formal analysis, data curation, writing (review and editing), and visualization. EP: conceptualization, methodology, validation, formal analysis, resources, data curation, writing (original draft), visualization, supervision, project administration, and funding acquisition. PB: conceptualization, methodology, validation, formal analysis, data curation, writing (original draft), visualization, supervision, and funding acquisition. RB: conceptualization, validation, resources, writing (review and editing), visualization, supervision, project administration, and funding acquisition. All authors contributed to the article and approved the submitted version
Funding
The authors gratefully acknowledge the funding from the company Yessinergy LTDA (Campinas, SP, Brazil), and the São Paulo Research Foundation (FAPESP) for funding the project (grant number 2018/19743-7; grant number 2017/50339-5). They also thank the members of the Laboratory of Nutrition and Ruminal Fermentation of the Beef Cattle Research Center of Instituto de Zootecnia for their assistance with sample collection and laboratory analyses. The authors thank the Fundação de Amparo à Pesquisa e Inovação do Estado de Santa Catarina (FAPESC, grant numbers TO2021TR937 and TO2023TR535). The authors are also thankful to Programa de Bolsas de Iniciação Científica of Universidade do Estado de Santa Catarina (PROBIC/UDESC), which provided ARC with the scholarship.
Conflict of interest
Authors JS and VS were employed by the company Yessinergy LTDA.
The remaining authors declare that the research was conducted in the absence of any commercial or financial relationships that could be construed as a potential conflict of interest.
The authors declare that this study received funding from Yessinergy LTDA. The funder had the following involvement in the study: resources, writing (review and editing), visualization, and funding acquisition.
Publisher’s note
All claims expressed in this article are solely those of the authors and do not necessarily represent those of their affiliated organizations, or those of the publisher, the editors and the reviewers. Any product that may be evaluated in this article, or claim that may be made by its manufacturer, is not guaranteed or endorsed by the publisher.
References
Azzaz H. H., Murad H. A., Morsy T. A. (2015). Utility of ionophores for ruminant animals: a review. Asian J. Anim. Sci. 9, 254–265. doi: 10.3923/ajas.2015.254.265
Bach A., Iglesias C., Devant M. (2007). Daily rumen pH pattern of loose-housed dairy cattle as affected by feeding pattern and live yeast supplementation. Anim. Feed Sci. Technol. 136, 146–153. doi: 10.1016/j.anifeedsci.2006.09.011
Batista L. H. C., Cidrini I. A., Prados L. F., Cruz A. A. C., Torrecilhas J. A., Siqueira G. R., et al. (2022). A meta-analysis of yeast products for beef cattle under stress conditions: Performance, health and physiological parameters. Anim. Feed Sci. Technol. 283, 115182. doi: 10.1016/j.anifeedsci.2021.115182
Benedeti P. D. B., Fonseca M. A., Shenkoru T., Marcondes M. I., Paula E. M., da L. G., et al. (2018). Does partial replacement of corn with glycerin in beef cattle diets affect in vitro ruminal fermentation, gas production kinetic, and enteric greenhouse gas emissions? PloS One 13, e0199577. doi: 10.1371/journal.pone.0199577
Broadway P., Carroll J., Sanchez N. (2015). Live yeast and yeast cell wall supplements enhance immune function and performance in food-producing livestock: a review. Microorganisms 3, 417–427. doi: 10.3390/microorganisms3030417
Chaucheyras-Durand F., Chevaux E., Martin C., Forano E. (2012). “Use of yeast probiotics in ruminants: effects and mechanisms of action on rumen pH, fibre degradation, and microbiota according to the diet,” in Probiotic in Animals ((Rijeka, Croatia: InTech). doi: 10.5772/50192
Cross F. R., Archambault V., Miller M., Klovstad M. (2002). Testing a mathematical model of the yeast cell cycle. Mol. Biol. Cell 13, 52–70. doi: 10.1091/mbc.01-05-0265
Davis C. S., Stephens M. A. (1989). Algorithm AS 248: empirical distribution function goodness-of-fit tests. Appl. Stat. 38, 535. doi: 10.2307/2347751
Detmann E., Souza M. S., Valadares Filho S. C., Queiroz A., Berchielli T., Saliba E. O., et al. (2012). Métodos Para Análise de Alimentos (Visconde Do Rio Branco, Mg. Suprema: Suprema Gráfica).
dos Santos M. V., Goes R. H. T. B., Takiya C. S., Cabral L. da S., Mombach M. A., Oliveira R. T., et al. (2023). Effect of increasing doses of chitosan to grazing beef steers on the relative population and transcript abundance of Archaea and cellulolytic and amylolytic bacteria. Anim. Biotechnol. 34, 246–252. doi: 10.1080/10495398.2021.1954936
Elghandour M. M. Y., Chagoyán J. C. V., Salem A. Z. M., Kholif A. E., Castañeda J. S. M., Camacho L. M., et al. (2014). Effects of Saccharomyces cerevisiae at direct addition or pre-inCubation on in vitro gas production kinetics and degradability of four fibrous feeds. Ital. J. Anim. Sci. 13, 3075. doi: 10.4081/ijas.2014.3075
Garcia M., Bradford B. J., Nagaraja T. G. (2017). Invited review: ruminal microbes, microbial products, and systemic inflammation. Professional Anim. Scientist. 33 (6), 635–650. doi: 10.15232/pas.2017-01663
Gharechahi J., Salekdeh G. H. (2018). A metagenomic analysis of the camel rumen’s microbiome identifies the major microbes responsible for lignocellulose degradation and fermentation. Biotechnol. Biofuels 11, 216. doi: 10.1186/s13068-018-1214-9
Grings E. E., Blümmel M., Südekum K.-H. (2005). Methodological considerations in using gas production techniques for estimating ruminal microbial efficiencies for silage-based diets. Anim. Feed Sci. Technol. 123–124, 527–545. doi: 10.1016/j.anifeedsci.2005.04.041
Grove A. V., Kaiser C. R., Iversen N., Hafla A., Robinson B. L., Bowman J. G. P. (2006). Digestibility of barley beta-glucan in beef cattle. Proc. Western Section Am. Soc. Anim. Sci. 57, 367–369.
Honan M., Feng X., Tricarico J. M., Kebreab E. (2021). Feed additives as a strategic approach to reduce enteric methane production in cattle: modes of action, effectiveness and safety. Anim. Prod. Sci. 62, 1303–1317. doi: 10.1071/AN20295
Ipharraguerre I. R., Clark J. H. (2003). Usefulness of ionophores for lactating dairy cows: a review. Anim. Feed Sci. Technol. 106, 39–57. doi: 10.1016/S0377-8401(03)00065-8
Jia P., Cui K., Ma T., Wan F., Wang W., Yang D., et al. (2018). Influence of dietary supplementation with Bacillus licheniformis and Saccharomyces cerevisiae as alternatives to monensin on growth performance, antioxidant, immunity, ruminal fermentation and microbial diversity of fattening lambs. Sci. Rep. 8, 16712. doi: 10.1038/s41598-018-35081-4
Kogan G., Kocher A. (2007). Role of yeast cell wall polysaccharides in pig nutrition and health protection. Livest Sci. 109, 161–165. doi: 10.1016/j.livsci.2007.01.134
Krehbiel C. R., Rust S. R., Zhang G., Gilliland S. E. (2003). Bacterial direct-fed microbials in ruminant diets: Performance response and mode of action12. J. Anim. Sci. 81, E120–E132. doi: 10.2527/2003.8114_suppl_2E120x
Krüger D., van der Werf M. (2018). Benefits of Application of Yeast β-Glucans in Ruminants (Hamburg, Germany: Ohly Application Note).
Ku-Vera J. C., Jiménez-Ocampo R., Valencia-Salazar S. S., Montoya-Flores M. D., Molina-Botero I. C., Arango J., et al. (2020). Role of secondary plant metabolites on enteric methane mitigation in ruminants. Front. Vet. Sci. 7. doi: 10.3389/fvets.2020.00584
Li Z., Bai H., Zheng L., Jiang H., Cui H., Cao Y., et al. (2018). Bioactive polysaccharides and oligosaccharides as possible feed additives to manipulate rumen fermentation in Rusitec fermenters. Int. J. Biol. Macromol. 109, 1088–1094. doi: 10.1016/j.ijbiomac.2017.11.098
Li R., Teng Z., Lang C., Zhou H., Zhong W., Ban Z., et al. (2019). Effect of different forage-to-concentrate ratios on ruminal bacterial structure and real-time methane production in sheep. PloS One 14, e0214777. doi: 10.1371/journal.pone.0214777
McAllister T. A., Beauchemin K. A., McGinn S. M., Hao X., Robinson P. H. (2011). Greenhouse gases in animal agriculture—Finding a balance between food production and emissions. Anim. Feed Sci. Technol. 166–167, 1–6. doi: 10.1016/j.anifeedsci.2011.04.057
McGinn S. M., Beauchemin K. A., Coates T., Colombatto D. (2004). Methane emissions from beef cattle: Effects of monensin, sunflower oil, enzymes, yeast, and fumaric acid1. J. Anim. Sci. 82, 3346–3356. doi: 10.2527/2004.82113346x
Menke H. H., Steingass H. (1988). Estimation of the energetic feed value obtained from chemical analysis and in vitro gas production using rumen fluid. Anim. Res. Development 28, 7–55.
Mombach M. A., da Silva Cabral L., Lima L. R., Ferreira D. C., Pedreira B. C. E., Pereira D. H. (2021). Association of ionophores, yeast, and bacterial probiotics alters the abundance of ruminal microbial species of pasture intensively finished beef cattle. Trop. Anim. Health Prod. 53, 172. doi: 10.1007/s11250-021-02617-2
Monnerat J. P. I., dos S., Paulino P. V. R., Detmann E., Valadares Filho S. C., Valadares R. D. F., et al. (2013). Effects of Saccharomyces cerevisiae and monensin on digestion, ruminal parameters, and balance of nitrogenous compounds of beef cattle fed diets with different starch concentrations. Trop. Anim. Health Prod. 45, 1251–1257. doi: 10.1007/s11250-013-0356-9
Montes F., Meinen R., Dell C., Rotz A., Hristov A. N., Oh J., et al. (2013). SPECIAL TOPICS — Mitigation of methane and nitrous oxide emissions from animal operations: II. A review of manure management mitigation options1. J. Anim. Sci. 91, 5070–5094. doi: 10.2527/jas.2013-6584
Nelson D. L., Cox M. M., Lehninger A. L. (2009). Lehninger principles of biochemistry (New York: WH Freeman).
Pedraza-Hernández J., Elghandour M. M. M. Y., Khusro A., Camacho-Diaz L. M., Vallejo L. H., Barbabosa-Pliego A., et al. (2019). Mitigation of ruminal biogases production from goats using Moringa oleifera extract and live yeast culture for a cleaner agriculture environment. J. Clean Prod. 234, 779–786. doi: 10.1016/j.jclepro.2019.06.126
Pereira A. M., de Lurdes Nunes Enes Dapkevicius M., Borba A. E. S. (2022). Alternative pathways for hydrogen sink originated from the ruminal fermentation of carbohydrates: Which microorganisms are involved in lowering methane emission? Anim. Microbiome 4, 5. doi: 10.1186/s42523-021-00153-w
Polyorach S., Wanapat M., Cherdthong A. (2014). Influence of yeast fermented cassava chip protein (YEFECAP) and roughage to concentrate ratio on ruminal fermentation and microorganisms using in vitro gas production technique. Asian-Australas J. Anim. Sci. 27, 36–45. doi: 10.5713/ajas.2013.13298
Refat B., Anele U., He Z. X., Bassiony S. M., Abdel-Rahman G. A., Yang W. Z. (2015). Effect of sainfoin hay and pomegranate peel extracts on in vitro fermentation and protein degradation using the RUSITEC technique. Can. J. Anim. Sci. 95, 417–423. doi: 10.4141/cjas-2014-142
Reynolds C. K., Kristensen N. B. (2008). Nitrogen recycling through the gut and the nitrogen economy of ruminants: An asynchronous symbiosis1. J. Anim. Sci. 86, E293–E305. doi: 10.2527/jas.2007-0475
Rook G. A. W. (2005). Microbes, immunoregulation, and the gut. Gut 54, 317–320. doi: 10.1136/gut.2004.053785
SAS Institute, Cary, (2013). SAS, Institute. Base SAS 9.4 procedures guide: statistical procedures. (Cary, NC, USA: SAS Institute Inc,) 2013.
Schären M., Drong C., Kiri K., Riede S., Gardener M., Meyer U., et al. (2017). Differential effects of monensin and a blend of essential oils on rumen microbiota composition of transition dairy cows. J. Dairy Sci. 100, 2765–2783. doi: 10.3168/jds.2016-11994
Schofield P., Pitt R. E., Pell A. N. (1994). Kinetics of fiber digestion from in vitro gas production. J. Anim. Sci. 72, 2980–2991. doi: 10.2527/1994.72112980x
Tagliapietra F., Cattani M., Hansen H. H., Hindrichsen I. K., Bailoni L., Schiavon S. (2011). Metabolizable energy content of feeds based on 24 or 48h in situ NDF digestibility and on in vitro 24h gas production methods. Anim. Feed Sci. Technol. 170, 182–191. doi: 10.1016/j.anifeedsci.2011.09.008
Tedeschi L. O., Fox D. G., Tylutki T. P. (2003). Potential environmental benefits of ionophores in ruminant diets. J. Environ. Qual. 32, 1591–1602. doi: 10.2134/jeq2003.1591
Tiago N. P. V., Erico da S.L., Wallacy B.R.d. S., Andr eacute ia S. C., aacute rio C., aacute ssio J. T., et al. (2016). Ruminal microorganism consideration and protein used in the metabolism of the ruminants: A review. Afr. J. Microbiol. Res. 10, 456–464. doi: 10.5897/AJMR2016.7627
Valadares Filho S. de C., Silva L. F. C. E., Gionbelli M. P., Rotta P. P., Marcondes M. I., Chizzotti M. L., et al. (2016). BR-CORTE: Nutrient requirements of Zebu and crossbred cattle (BR-CORTE) 2023. Nutrient requirements of Zebu and crossbred cattle, 3rd revised edition. ( Viçosa, MG, Brazil: Suprema Grafica Ltda) 466. doi: 10.26626/978-85-8179-194-4.2023.B001
Van Soest P. J., Robertson J. B., Lewis B. A. (1991). Methods for dietary fiber, neutral detergent fiber, and nonstarch polysaccharides in relation to animal nutrition. J. Dairy Sci. 74, 3583–3597. doi: 10.3168/jds.S0022-0302(91)78551-2
Vikram A., Rovira P., Agga G. E., Arthur T. M., Bosilevac J. M., Wheeler T. L., et al. (2017). Impact of “Raised without antibiotics” Beef cattle production practices on occurrences of antimicrobial resistance. Appl. Environ. Microbiol. 83. doi: 10.1128/AEM.01682-17
Vohra A., Syal P., Madan A. (2016). Probiotic yeasts in livestock sector. Anim. Feed Sci. Technol. 219, 31–47. doi: 10.1016/j.anifeedsci.2016.05.019
Vyas D., Alemu A. W., McGinn S. M., Duval S. M., Kindermann M., Beauchemin K. A. (2018). The combined effects of supplementing monensin and 3-nitrooxypropanol on methane emissions, growth rate, and feed conversion efficiency in beef cattle fed high-forage and high-grain diets1. J. Anim. Sci. 96, 2923–2938. doi: 10.1093/jas/sky174
Wang C., Liu C., Zhang G. W., Du H. S., Wu Z. Z., Liu Q., et al. (2020). Effects of rumen-protected folic acid and betaine supplementation on growth performance, nutrient digestion, rumen fermentation and blood metabolites in Angus bulls. Br. J. Nutr. 123, 1109–1116. doi: 10.1017/S0007114520000331
Williams P. E., Tait C. A. G., Innes G. M., Newbold C. J. (1991). Effects of the inclusion of yeast culture (Saccharomyces cerevisiae plus growth medium) in the diet of dairy cows on milk yield and forage degradation and fermentation patterns in the rumen of steers. J. Anim. Sci. 69, 3016–3026. doi: 10.2527/1991.6973016x
Yáñez-Ruiz D. R., Bannink A., Dijkstra J., Kebreab E., Morgavi D. P., O’Kiely P., et al. (2016). Design, implementation and interpretation of in vitro batch culture experiments to assess enteric methane mitigation in ruminants—a review. Anim. Feed Sci. Technol. 216, 1–18. doi: 10.1016/j.anifeedsci.2016.03.016
Keywords: greenhouse gases, methane, monensin, prebiotics, ruminant nutrition
Citation: Cagliari AR, Magnani E, Rigon F, Loregian KE, Casagrande AC, Amâncio BR, Bueno da Silva J, Lisboa Santos V, Marcondes MI, Paula EM, Del Bianco Benedeti P and Branco RH (2023) Evaluation of yeast-based additives, as an alternative to ionophores, on rumen fermentation of ruminant diets using an in vitro gas production system. Front. Anim. Sci. 4:1233273. doi: 10.3389/fanim.2023.1233273
Received: 01 June 2023; Accepted: 14 August 2023;
Published: 07 September 2023.
Edited by:
Rodolpho Martin do Prado, Laval University, CanadaReviewed by:
Hossam H. Azzaz, National Research Center, EgyptDaniel Rico, Centre de Recherche en Sciences Animales de Deschambault, Canada
Copyright © 2023 Cagliari, Magnani, Rigon, Loregian, Casagrande, Amâncio, Bueno da Silva, Lisboa Santos, Marcondes, Paula, Del Bianco Benedeti and Branco. This is an open-access article distributed under the terms of the Creative Commons Attribution License (CC BY). The use, distribution or reproduction in other forums is permitted, provided the original author(s) and the copyright owner(s) are credited and that the original publication in this journal is cited, in accordance with accepted academic practice. No use, distribution or reproduction is permitted which does not comply with these terms.
*Correspondence: Pedro Del Bianco Benedeti, cGVkcm8uYmVuZWRldGlAdWRlc2MuYnI=