- 1Genus-PIC, Schleswig, Germany
- 2Topigs Norsvin, Helvoirt, Netherlands
- 3Danish Agriculture & Food Council, Aarhus, Denmark
- 4Hendrix Genetics, Boxmeer, Netherlands
- 5European Forum of Farm Animal Breeders, Brussels, Belgium
- 6Genus-PIC, Sant Cugat del Valles, Spain
Introduction: Litter size in pigs has increased steadily since 1990. Because of unfavorable genetic correlations with piglet mortality, breeding goals should include survival traits next to litter size. Unbalanced breeding programs that neglect this requirement have produced increased mortality levels, attracting negative public attention. Balanced breeding does not have this disadvantage, but the general public is largely unaware of this.
Methods: We present long-term time trends as realized in commercial breeding. The data includes (i) phenotypes of litter size, piglet birth weight, and piglet mortality, as used in routine breeding value estimation; and (ii) the genomic Best Linear Unbiased Prediction (gBLUP) estimated breeding values thus obtained. Piglet mortality (2001–2022) and birth weight (2009–2022) phenotypes were related to litter size by recording year. Estimated breeding values (EBVs) for the mortality traits were regressed on those for litter size by birth year (2012–2022).
Results: Average litter size is very weakly correlated to the mortality (R2 ≤ 0.06) and birth weight (0.07 ≤ R2 ≤ 0.26) traits, and those correlations are unfavorable (antagonistic) within each year. However, all traits analyzed here show favorable simultaneous phenotypic and genetic trends over time: the antagonisms are neutralized by balanced breeding. Above the annual mean litter size level, farrowing and lactation mortality rates increased with increasing litter size in every year (unfavorable), but the annual intercepts and the slopes decreased from 2001 to 2022 (favorable). Average litter birth weight decreased with litter size in every year (unfavorable), but the annual intercepts increased and the slopes decreased from 2009 to 2022 (favorable). The within-litter birth weight variation coefficient increased with litter size in every year (unfavorable), but the annual intercepts decreased from 2009 to 2022 (favorable). The proportion of low birth weights (i.e.,< 0.9 kg) for a given litter size is decreasing over time, and the critical birth weight level (below which lactation mortality increases strongly) is clearly population dependent and changes over time too.
Discussion: The increases in litter size and piglet survival rates due to balanced breeding policies lead to reduced total numbers of dead piglets (i.e., per country, per year) coinciding with a certain pig production volume (i.e., with a certain total number of weaned piglets).
1 Introduction
Just like any other industry, the livestock sector must improve its production efficiency to remain competitive and sustainable. The number of weaned piglets produced per sow per year is a very relevant factor in achieving this. Considerable genetic variation in litter size, piglet survival, and birth weight exists. Breeding companies make use of this genetic variation to assist farmers in realizing higher production levels (number of weaned piglets per sow per year) and improved efficiency within a production system. Recent years have seen increasing public concern about the quantity of animal losses before weaning. This article describes the opportunities that arise from existing genetic variation, and the change in relevant traits over time. Commercial pig breeding plays an important role in finding genetic solutions to the survival of piglets in modern production systems, while also improving efficiencies in other areas of pork production.
Selection for litter size in pigs became a successful breeding strategy once Best Linear Unbiased Prediction (BLUP) was implemented for breeding value estimation around 1990 (Knap et al., 1993). Litter size has steadily increased since then. This has led to significantly more cost- and resource-efficient global production systems: the sow herd in most European countries has decreased by 25% since 2000, whereas the number of pigs slaughtered increased by a similar proportion. This has made a significant contribution to reducing the overall production cost and environmental footprint of a kilogram of pig meat.
The genetic correlation of litter size with piglet survival is unfavorable (e.g., Bidanel, 2011, Table 10.4), which leads to an increase in the piglet mortality rate if selection for increased litter size is not accompanied by selection for increased survival. By using existing genetic variation, mortality rates are actually being reduced, as we will show below. However, despite initiatives such as Code-EFABAR, which was set up in 2005 by the European Forum of Farm Animal Breeders to “contribute to increased transparency in the food production chain, building understanding and the dialogue that is part of this process” (https://www.effab.info/modern-animal-breeding/responsible-breeding), key stakeholders and the general public are largely unaware of the efforts by breeding companies to improve this important aspect of animal welfare. The scientific literature [and, derived from that, popular media, animal welfare pressure groups, non-governmental organizations (NGOs), public opinion, and governmental policy] appears largely ignorant of modern developments in commercial pig breeding and assumes that mortality rates must necessarily continue to increase with increasing litter size. As is so often the case in discussions about livestock production, many of these views are based on information that is largely out of date and/or derived from very limited data, and is therefore not representative of the current state of production (see Appendix 1 for a notable example by the EFSA AHAW Panel et al., 2022). This is essentially due to insufficient transparency and communication from the pig breeding sector—a side effect of intense commercial competition among the players in this sector, with marketing to pig producers logically focusing on profitability. This clearly must change, and this article is intended to contribute to this change. Therefore, to place the issue into proper perspective and to increase transparency, we present here the genetic and phenotypic time trends of litter size, piglet mortality, and piglet birth weight, as they have been realized in commercial pig breeding programs that together supplied breeding stock to more than half of the 2022 Western pig production sector.
This field has been described and reviewed in a great deal of useful biological and genetic detail by Gaskins and Kelley (1995), Herpin and Le Dividich (1995), Lay et al. (2002), Rutherford et al. (2011), Edwards and Baxter (2015), Yuan et al. (2015), Pandolfi et al. (2017), Heuß et al. (2019), Villanueva-García et al. (2021), and Knol et al. (2022), among many others. For the sake of brevity, we refer to these sources for background information, and do not attempt to summarize them here.
Piglet mortality is a relevant issue for two different reasons. The conventional point of view is the one of the pig producer: the farmer whose foremost interest is in his own livelihood, and, therefore, profitability. The relevant element here is the mortality rate: the proportion of piglets born (dead or alive) in a litter that do not survive up to a certain point in time when sold. Essentially, this is an element of production efficiency and in commercial pig breeding this has always been the logical parameter when quantifying the weighting of the trait relative to other traits in the breeding goal (such as litter size), because the pig producer is the breeder’s customer, whose interests must be catered for.
A different point of view is the one communicated by animal welfare pressure groups, illustrated by provocative quotations (translated by us from Danish and Dutch) such as “25,000 piglets are destroyed every day” (Via Ritzau, 2010), “piglet mortality hits a new record: 29,514 die every day” (Arp, 2022), or “6 million pigs die on the farm every year. A large portion of these pigs (4.6 million) die in the first 3 or 4 weeks of life“ (Varkens in Nood, 2016, p. 6). The figure used here is the total nationwide number (count) of piglets that die per year.
The main difference between the two points of view is that an increase in mortality rate is economically acceptable if the net number of surviving pigs per litter nevertheless increases (because litter size increases at a faster rate than mortality rate does), whereas an animal welfare perspective focuses on the total number of dying piglets, not the surviving ones. However, the two points of view are not exclusive; there is much overlap between them and we will show how both can be (and continue to be) catered for by commercial pig breeding.
1.1 A history of litter size and piglet mortality
Data on wild boar and feral pigs from 49 sources (published from 1951 to 2011) summarized in Appendix 3 of Rutherford et al. (2011), and from Jezierski (1977); Barrett (1978); Náhlik and Sándor (2003), and Chinn et al. (2021), show average values for litter size that range from 3.1 to 7.6 (average 4.9), average values for farrowing mortality rate that range from 6% to 40% (average 16%), and average values for postnatal mortality rate that range from 5% to 90% (average 42%).
The Spanish Ibérico breed was not artificially selected for reproductive traits until 2010, when part of the population was transferred from the classical extensive outdoor system to intensive production systems (see e.g., Muñoz et al., 2017). Data on extensively kept Ibérico pigs from 19 sources (published from 1983 to 2015) summarized in Table 2 of Nieto et al. (2019), and from González et al. (2007); Ibáñez-Escriche et al. (2014); Charneca et al. (2015); Muñoz et al. (2017), and Varona et al. (2020), show average values for litter size that range from 6.4 to 9.8 (average 8.3) and average values for postnatal mortality rate that range from 3% to 28% (average 15.1%). After three generations of selection in one of the intensively kept subpopulations in which one of our breeding companies performs breeding value estimation, the average total number born was 9.3 and the lactation mortality rate was 11.8%, illustrating that balanced selection can move both these traits into the desired direction in the Ibérico pig as well.
During the 1980s, several European countries and the USA imported pigs of the Chinese Meishan breed, having noticed that these pigs combine large litter size with low piglet mortality rates. Data from Bidanel et al. (1989); Bidanel et al. (1994) on the Meishan population in France reports the average total number born at 15.1, the farrowing mortality rate at 6.8%, and the postnatal mortality rate at 10.6%.
For Large White pigs not effectively selected for reproductive traits, Fahmy and Bernard (1971) reported an average litter size (total number born) of 8.9, a farrowing mortality rate of 7.2%, and a lactation mortality rate of 16.4% in a research station in Canada in the 1950s and 1960s. They mention that these values “are comparable with other reports in the literature, taking into consideration that mortality is mostly affected by many uncontrollable environmental and managerial factors [ … ] Few reports give preweaning mortality estimates of less than 20% [ … ] while mortality percentages exceeding 25% were reported.”. In comparison, the data in Denmark in 1992, just before effective selection for litter size was initiated there, showed an average of 11.5 total born, a farrowing mortality rate of 6.2%, and a lactation mortality rate of 12.5% at the commercial level—more favorable than the much earlier Canadian research station levels above. Subsequently, 12 years of selection for improved average total number born in Denmark resulted in 2005 levels of 14.9 average total born, a farrowing mortality rate of 11.4%, and a lactation mortality rate of 12.8% (Rutherford et al., 2011, their Table 3 and Figure 2). By then the selection criterion had been changed to the number of surviving piglets at day 5, and Christensen et al. (2018, their Figures C1 to C3) reported 2016 levels of 18.0 average total born, a farrowing mortality rate of 9.4%, and a lactation mortality rate of 13.5%. However, Henryon et al. (2022) and Zaalberg et al. (2022) noticed that the revised selection criterion was still not sufficiently balanced, and would benefit from a two-trait approach (total number born and survival rate) rather than a single trait one; this was implemented in 2022 (https://tinyurl.com/2p8s6f7s).
The abovementioned average levels of litter size and lactation mortality have been summarized in Figure 1, where we also included some results of our phenotypic analysis described below. This reveals two interesting things. First, although wild boar and feral pigs can exhibit more of their natural behavior patterns than domestic pigs, their reproductive performance represents an unfavorable outlier when compared with any form of domestic pig breeding, in terms of both litter size and piglet survival. Second, the housing and nutrition aspects of domestication, either in their own right or in combination with balanced breeding policies, have been successful in increasing litter size while reducing lactation mortality rate (Figure 1A) in a surprisingly smooth pattern. A very similar pattern appears in Figure 1B for the main welfare-relevant element, i.e., the number of lactation mortalities per piglet weaned—bearing in mind that a certain number of weaned piglets per year is the main production target of any modern pig production system.
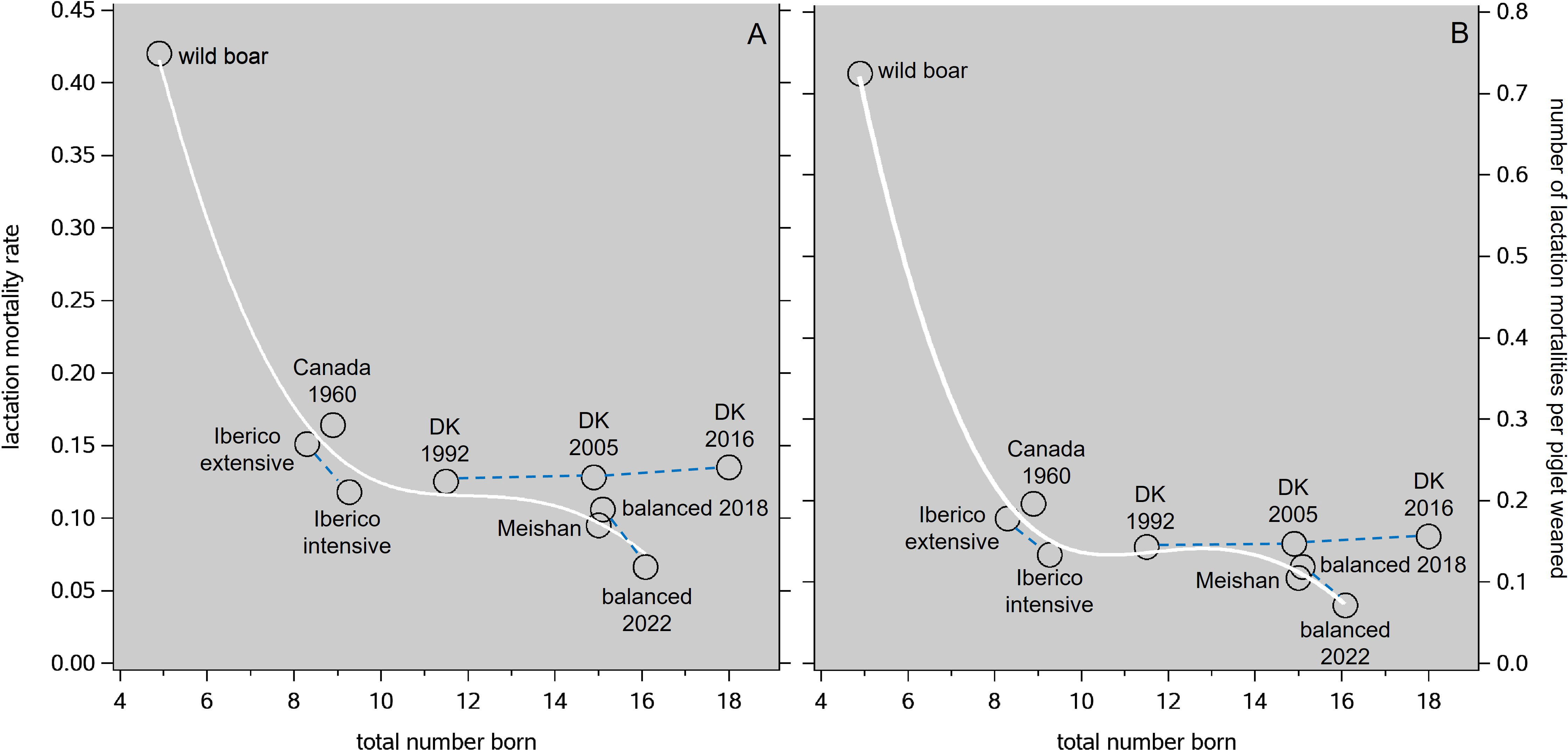
Figure 1 Average levels of the lactation mortality rate (A) and the number of lactation mortalities per piglet weaned (B) in relation to total number born, as reported in the literature on wild boar; the Spanish Ibérico breed kept in traditional extensive conditions; an Ibérico subpopulation after three generations of balanced selection, the Chinese Meishan breed in France, the Canadian Large White around 1960; commercial sows in Denmark (DK) in 1992, 2005, and 2016; and some of our other maternal lines in 2018 and 2022 (labeled as "balanced"; these are results from the analysis in the present study, see Figure 5C; 2018 because total number born was then at the same level as for Denmark in 2005). The white trendlines are spline interpolations through all the datapoints except the ones for Denmark in 2005 and 2016. The blue dashed lines connect instances of the same genetic population; note that all other datapoints are disconnected.
1.2 Genetics is part of the solution: modern multitrait breeding goals
As mentioned in the Introduction, selection for litter size in pigs became a successful breeding strategy once BLUP was implemented for breeding value estimation around 1990. It was found that traits with a low heritability (such as reproduction traits) can be genetically improved when (i) the correct statistical methodology is applied to large data volumes and (ii) the resulting estimated breeding values (EBVs) are actually selected on, as opposed to phenotypic selection. From that moment on, the critical element was not the accuracy of the EBVs but the weighting of the various trait EBVs in the breeding goal. Litter size at farrowing has a higher heritability than piglet mortality (e.g., Bidanel, 2011, his Table 10.3) and it is much easier to record, thus its data volume is always larger. Therefore, the breeding goals of those years focused on litter size, with spectacular results in terms of its genetic improvement (e.g., Knap and Rauw, 2009, their Figure 12.1.b).
A decade later it had become clear that piglet mortality rates had been increasing together with litter size, due to unfavorable genetic correlations. (Baxter and Edwards, 2018, pp. 75–76) describe the evolutionary process as follows:
"a certain amount of piglet mortality is a predisposed and inevitable event [ … ] this is a form of natural selection implemented by the sow whereby only the fittest will survive. This results from a form of parental optimism whereby the sow overproduces offspring at birth [ … ] the evolutionary strategy involves unequal provision of resources (i.e., milk) by the mother to her litter, resulting in intense sibling rivalry and early mortality of weaker individuals. [ … ] the capacity for parental optimism, individual embryo mortality and ‘siblicide’ are dependent on absolute litter size. Attempting to reduce piglet mortality under domestic conditions has had limited success and a major contributor to this is likely to be these hard-wired evolutionary strategies. Thus, exploiting biology (i.e., selecting for high litter sizes) means battling against it (i.e., [against] the associated mortality)".
In summary, this is a hard-wired genetically antagonistic system, which requires focused artificial selection to be neutralized. (Baxter and Edwards, 2018, p. 85) continue: “The link between litter size and mortality can be influenced by a more balanced selection policy, incorporating survival traits as well as litter size traits in the breeding index and assigning appropriate weightings to each”. This was written almost two decades after such “balanced selection policies” had in fact been implemented in commercial pig breeding; our Figure 2 shows that such a policy (implemented in the late 1990s in four maternal lines) successfully neutralized the antagonism of litter size versus farrowing and lactation piglet survival: since 2002, these three traits show simultaneous genetic improvement—partly due to successful selection against congenital defects, such as scrotal and umbilical hernia, which represent additional causes of lactation mortality.
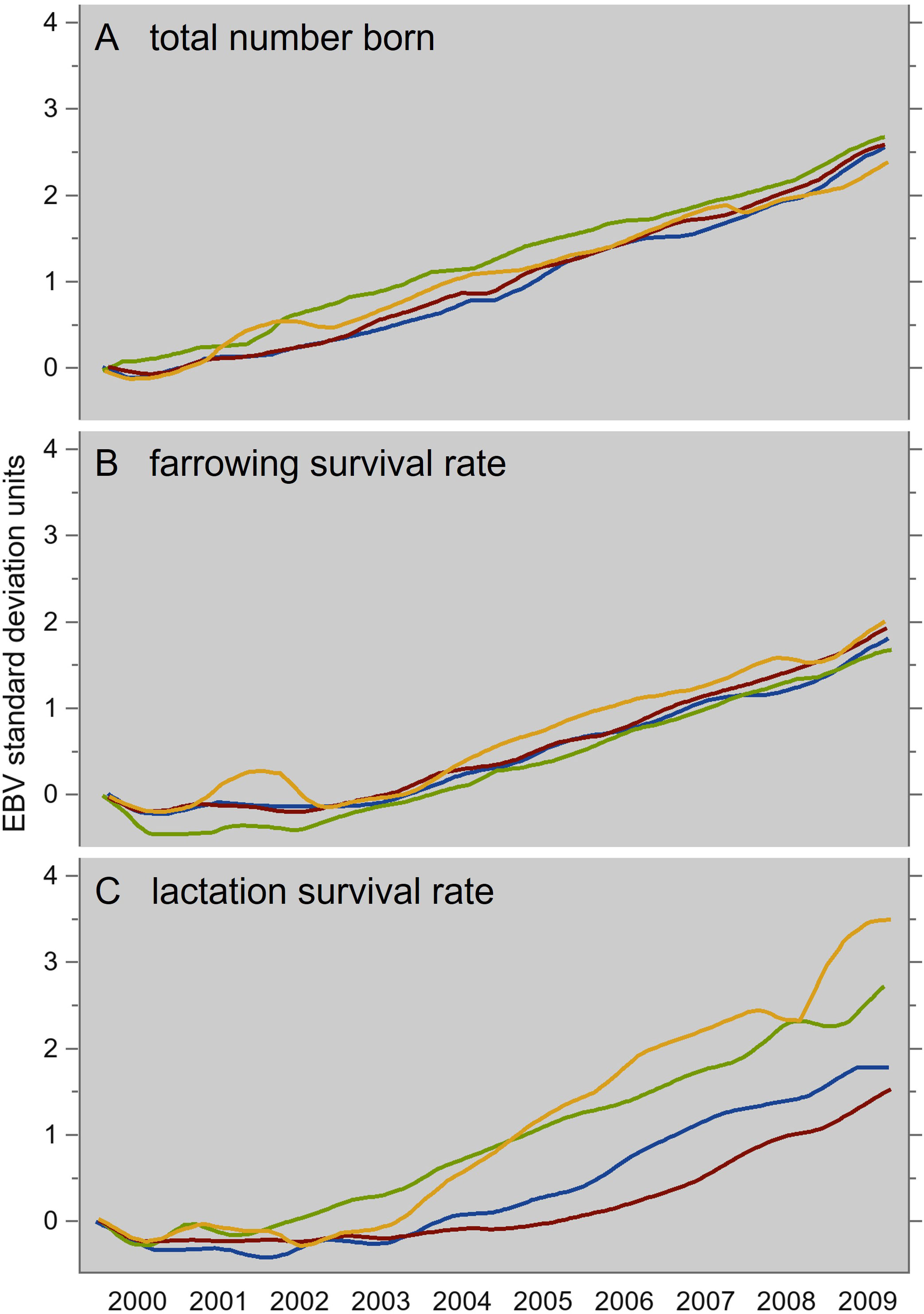
Figure 2 Simultaneous genetic time trends of litter size (A), and of farrowing (B) and lactation (C) piglet survival, in four maternal PIC lines from 2000 to 2009. Spline interpolations of routinely estimated trait breeding values, plotted against the date of birth of the animal. Modified from Figure 9 in Knap (2012).
At that same time, the breeding organization Topigs had concluded that “emphasis in selection for reproduction traits can be changed in the direction of survival, without decreasing total economic gain. This will give a better-balanced genetic improvement, a lower trend for total number born and positive trends for survival traits. [ … ] Knowledge of the genetic relations makes it possible to build an index which will allow a more balanced genetic progress, with an increase in piglet survival instead of a decrease” (Knol, 2001, pp. 96–97), and a few years later “selection on piglet survival” was implemented at Topigs (https://tinyurl.com/bdhupbxp). Also, breeding organization Danavl wrote: “The breeding system has been successful over the past dozen years in improving litter size of the maternal breeds. The criterion has been total number born. The success has almost become a problem! [ … ] it was decided to change the reproductive breeding goal from total number born to piglets surviving to day 5 [ … ] it focuses on viable piglets [ … ] from mid-June 2004” (Krabbe Olesen, 2004; our translation from Danish).
Since then, similar selection policies have been implemented by many pig breeding organizations. For example, Bidanel et al. (2020) described the developments in France as follows: “The increase in farrowing and lactation mortality correlated with the strong increase in litter size led to the replacement, in the breeding goals of Landrace and Large White, of total number born by a combination of number born alive and the number of functional teats (2002). Subsequently, these criteria were completed with litter size at weaning (2010–2014) and with the within-litter average and standard deviation of birth weight (2014)” (our translation from French). Table 1 summarizes the focus on litter size versus piglet survival traits in the breeding goals of other organizations. In addition, the breeding organizations (CCSI, 2021, p. 26), BHZP (https://tinyurl.com/bxmuk3c3), and Topigs Norsvin (https://tinyurl.com/5f2m4svc) report simultaneous genetic improvement for similar combinations of traits as in our Figure 2.
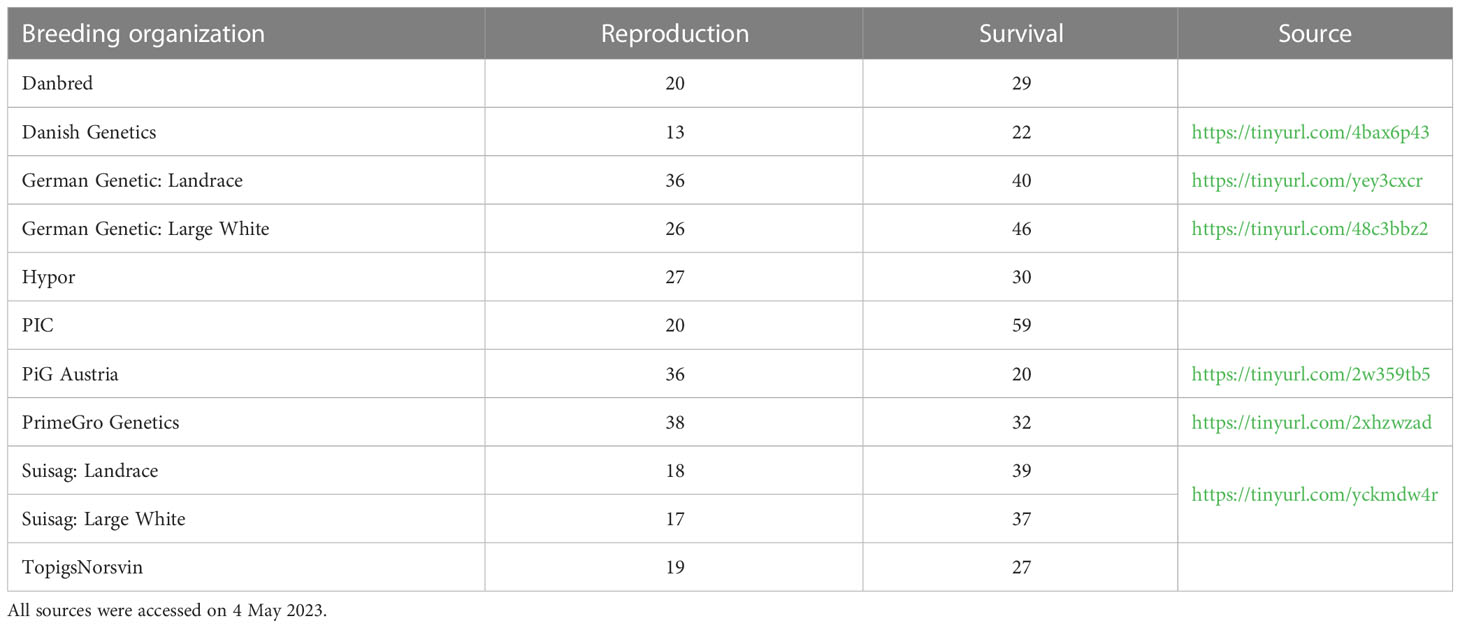
Table 1 Proportions (%) of pig breeding goals aimed at reproduction traits (litter size and weaning–mating interval) and at piglet survival traits (including teat number).
2 Materials and methods
In this study we use phenotypic data on our traits of primary interest, i.e., litter size and piglet mortality (n = 192,083 litters), and on the related “indicator” traits piglet birth weight (n = 116,009 litters) and teat number (n = 138,264 sows), as they are routinely recorded in the maternal lines of our breeding programs, mostly at the pure-line nucleus level where most of the genetic improvement is created. These data form the basis of estimated breeding values (EBVs) for these traits, which we routinely calculate in genomic BLUP (gBLUP) analyses (e.g., Mrode and Pocrnic, 2023); as a central part of the breeding programs, these EBVs are combined into the multitrait selection indexes that we use to select our breeding stock.
To quantify the univariate genetic time trend for a trait, we regress and plot the EBVs for that trait against the date of birth of the relevant animals (as was previously done in Figure 2). To quantify bivariate genetic time trends, we regress the EBV for piglet mortality (farrowing, lactation, or total mortality) on the EBV for litter size, within year of birth, and we plot the successive annual regression lines.
To quantify bivariate phenotypic time trends we relate, within year of birth, (i) the raw phenotypes of piglet mortality (farrowing, lactation, or total mortality) and of birth weight and its within-litter variation to the raw phenotypes of litter size, and (ii) the raw phenotypes of lactation mortality to the raw phenotypes of birth weight. We also plot the successive annual trendlines.
To quantify the relevant time trends in teat number we calculate, for each litter in the data, the difference between teat number (TTN) and litter size (number born alive, NBA); we plot the frequency distribution of this (TTN—NBA) difference by year. Routine teat number recording takes place mainly in pureline breeding farms where replacement rates are high so that most litters are born in early parities, and this leads to lower litter sizes than in commercial farms with crossbred sows and lower replacement rates. To correct the biased (TTN - NBA) difference, we restrict this analysis to second-parity litters only (in our data, average second-parity NBA is very close to the weighted average NBA across parities one to eight) and we increase NBA by 8% to mimic heterosis (Bidanel, 2011, his Table 10.2); we plot the adjusted difference against time.
Because our respective companies are in competition with each other on the worldwide pig breeding market, much of these data are commercially sensitive. Therefore, we refer to the data sources behind our results as “company A”, “company B”, etc. For the sake of brevity, we present data for each case from one representative source only.
3 Results
3.1 Selection for piglet survival works: realized genetic time trends
We present here the estimated genetic time trends of litter size (total number born, TNB), the number stillborn (NSB), the lactation mortality rate (LAMR), and piglet birth weight (BWT) in the maternal lines of one breeding company from 2012 to 2022.
According to the linear regressions in Figure 3, in this 9-year period the genetic potential for TNB increased by 2.8 piglets per litter. Simultaneously, the genetic potential for the number stillborn piglets was reduced by 0.53 piglets per litter, the genetic potential for the lactation mortality rate was reduced by 7.1%, and the genetic potential for piglet birth weight increased by 0.27 kg. It follows that the genetic antagonisms of TNB versus piglet survival and birth weight can be neutralized by undertaking balanced selection, as was shown in Figure 2 for an earlier period. This does not mean that the unfavorable genetic correlations among these traits are removed, but that dedicated artificial selection can work around them. This is illustrated in Figure 4, where the EBV correlations of TNB with the lactation mortality rate and number stillborn remain unfavorable over time but experience a two-trait genetic improvement in each case. Figure 8A of Neeteson-Van Nieuwenhoven et al. (2013) shows similar patterns for an earlier period.
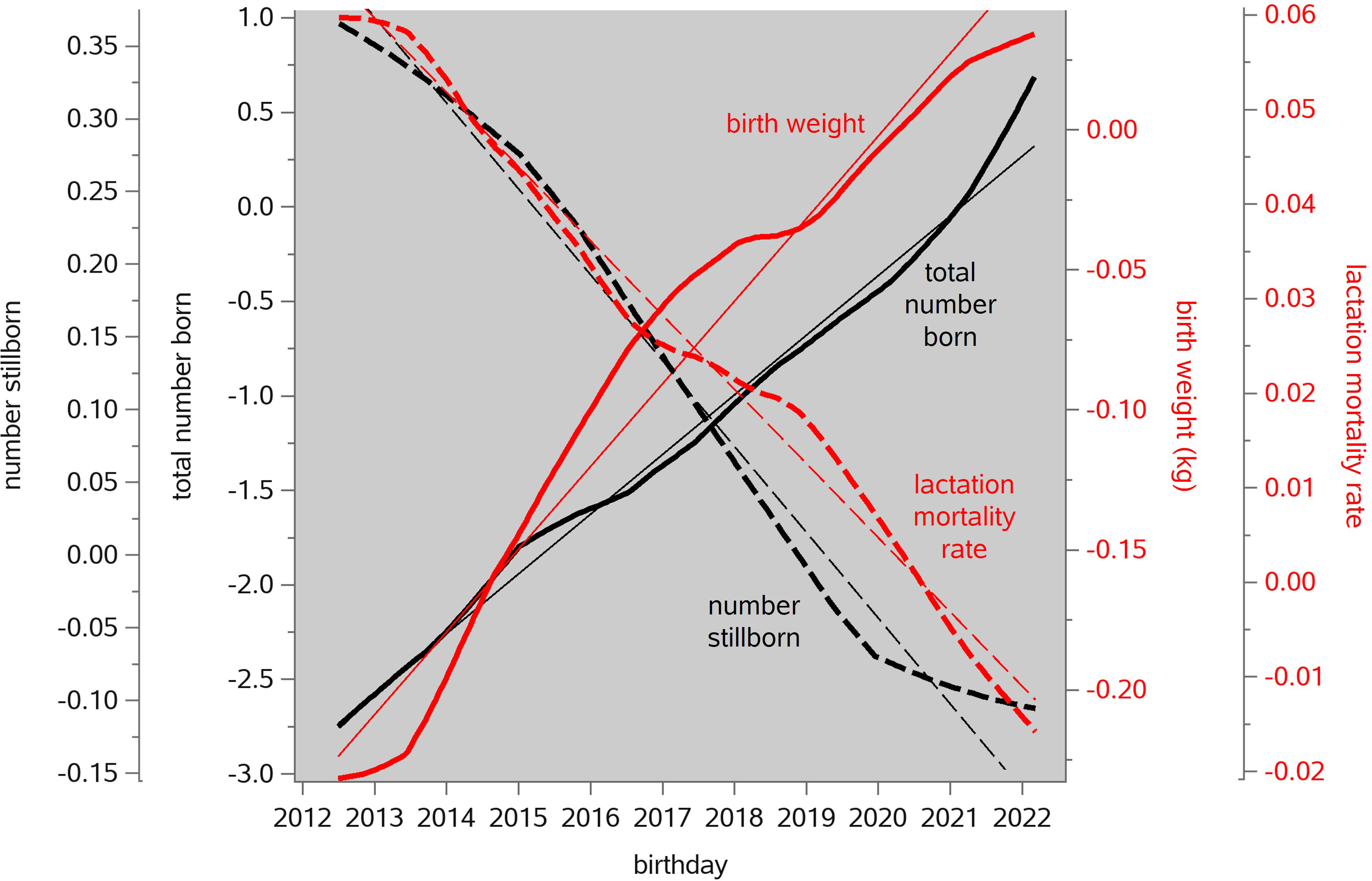
Figure 3 Genetic time trends of litter size (total number born, TNB), the number stillborn (NSB), the lactation mortality rate (LAMR), and piglet birth weight (BWT) in the maternal lines of one breeding company from 2012 to 2022. The curvy trendlines are Locally Estimated Scatterplot Smoothing (LOESS) interpolations through routinely estimated trait breeding values, plotted against the date of birth of the animal. The straight trendlines are linear regressions, with regression coefficients of +0.32 piglets per litter per year for TNB, –0.059 piglets per litter per year for NSB, –0.0078 (i.e., –0.78%) per year for LAMR, and +0.030 kg per year for BWT.
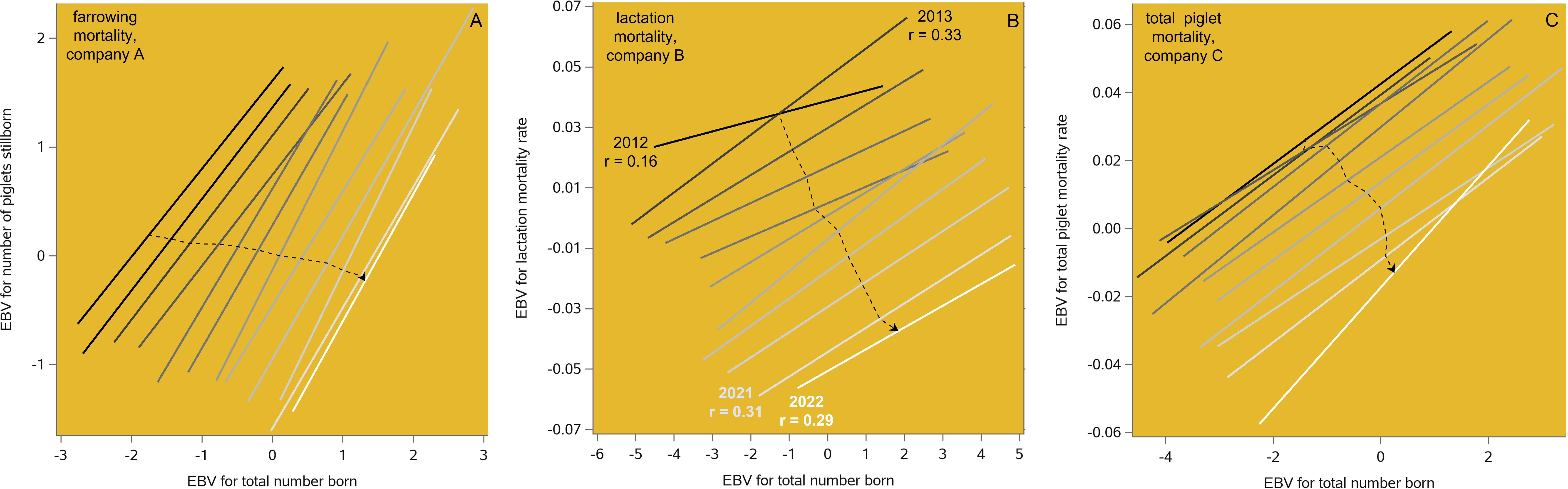
Figure 4 Linear regressions of routinely estimated breeding values (EBVs) for piglet mortality rate ((A) farrowing mortality, (B) lactation mortality, (C) total mortality) on the EBVs for total number born (TNB) in the maternal lines of three breeding companies, by year of birth from 2012 to 2022. The dashed lines connect the annual means and are therefore equivalent to genetic time trends such as shown in Figure 3.
In conclusion, the genetic antagonisms of litter size versus farrowing and lactation piglet survival can be successfully neutralized by balanced selection policies, which have actually been realized in commercial pig breeding since 2002; see Figures 2–4. The logical question that follows is to what extent this has been expressed phenotypically.
3.2 Selection for piglet survival works: realized phenotypic time trends
Knol et al. (2022) gave an exhaustive overview of the phenotypic relationships among the traits discussed here, and of their genetic and physiological backgrounds. However, they do not show how these traits have developed over time due to the selection policies and genetic improvement described in the previous sections. The focus of our current phenotypic analysis is on how trait levels, and the relationships among them, change over time as a result of a “balanced selection policy, incorporating survival traits as well as litter size traits in the breeding index and assigning appropriate weightings to each” (Baxter and Edwards, 2018) that was implemented in the late 1990s.
Figure 5A shows that the phenotypic relationship between the farrowing mortality rate and total number born (TNB) is very messy; accordingly, the set of annual trendlines in Figure 5B achieves only a very low R2 of 0.06. Nevertheless, the trends are logically curvilinear; the initial decrease in mortality rate is partly due to a roughly stable number of stillbirths per litter that gets divided by an increasing TNB while moving from left to right in the graph. Curiously, the optimum (i.e., minimum) farrowing mortality rate seems to occur around each year’s mean TNB value. TNB increased from a mean level of 11.6 in 2000 to 15.7 in 2021, up to a TNB of about 12 piglets (which becomes less frequent over time). The farrowing mortality pattern did not change much over time: the absolute level decreases somewhat (which is favorable), but the slope stays the same. By contrast, at the higher TNB levels (which become more frequent over time) the unfavorable positive slope decreased steadily (i.e., became less unfavorable) over time, so that the farrowing mortality rate at any particular TNB level decreased too, and more so at the higher TNB levels. The lactation mortality rate (Figure 5C) shows a similarly desirable phenotypic time pattern, with a similarly low R2. In summary, the antagonism of both types of mortality versus TNB is gradually neutralized over time. The insets in these graphs show these same time patterns across other (disconnected) pig populations.
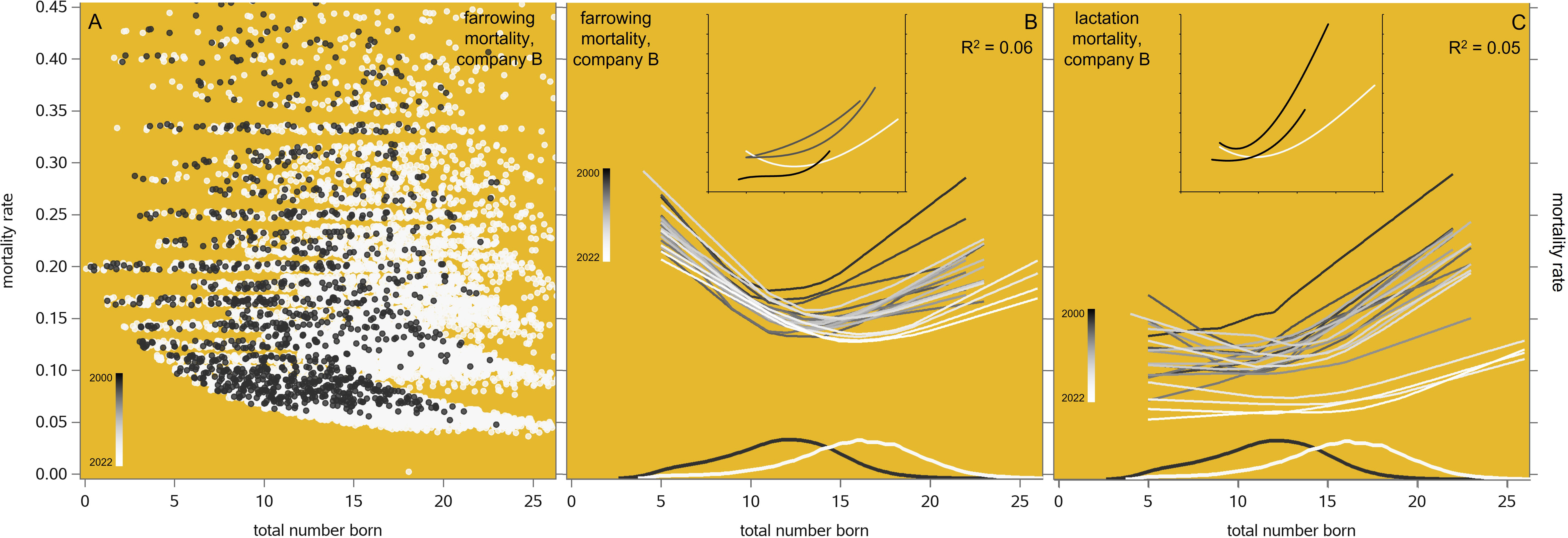
Figure 5 Farrowing (A, B) and lactation (C) mortality rate in relation to litter size (total number born, TNB) in the maternal lines of one breeding company, by recording year from 2000 to 2022. The datapoints in A have been jittered to make them all visible. The annual trendlines in B and C are Locally Estimated Scatterplot Smoothing (LOESS) interpolation plots. The density plots at the bottom of each graph show the frequency distributions of TNB in 2001 and 2021. The insets have axes with the same scale as the main graphs; their trendlines are cubic polynomials, showing comparable trends in other maternal lines (data recorded in France in the 1970s: Legault et al., 1985; in Australia in 1999: Hermesch, 2000; in Denmark in 2002–2003: Nielsen and Henriksen, 2004; and in Spain in 1999–2006: Ibáñez-Escriche et al., 2009).
4 Discussion
4.1 Representativeness of our data for commercial production conditions
Routine recording of preweaning survival rate, individual birth weight, and teat number is very uncommon in commercial farms—this is why much of the fragmentary literature on these traits draws from experimental settings or small-scale field projects. Therefore, our analyses rely on data recorded at the nucleus level of our breeding programs; this involves purebred sows producing mostly purebred litters, with fast generation turnover so that most litters are born at a lower parity and with a better animal health status than on most commercial farms. It follows that in our data litter size at farrowing is lower than in commercial conditions due to the parity effect and the absence of heterosis; in addition, better healthcare increases preweaning survival rate, but the absence of heterosis reduces it. The logical question is then to what extent genotype–environment interaction will influence the relevance of our results to commercial conditions. The literature holds very little meaningful evidence on this topic; a relevant example is Roehe et al. (2010), who divergently selected AI boars of two genetic lines according to their estimated breeding values for preweaning survival, based on data recorded in intensive high-health indoor nucleus conditions, and used their semen to produce sows (from one line) and their litters (from the other line) in outdoor conditions in the Scottish Highlands. They concluded that “the realized selection response [ … ] suggests that genotype-environmental interactions were negligible between indoor and outdoor conditions”. Notice that these indoor conditions involved all the purebred low-parity high-health elements mentioned at the start of this paragraph, and the outdoor conditions featured winter temperatures between –1 and +5°C (https://www.metoffice.gov.uk/research/climate/maps-and-data/uk-and-regional-series) and predation by foxes and buzzards. Based on that, we are confident that our nucleus data on preweaning mortality are sufficiently representative for a wide range of commercial conditions.
An important issue here is that this article is not about the phenotypic levels of any trait (or relationships between traits) as they occur at any point in time, but about the change over time in such levels and relationships. The driving factor here is genetic change due to artificial selection, and this affects the genetic potential of future animals for traits such as litter size and piglet survival. With heritabilities around 0.1, this genetic potential typically determines only 10% of the visible variation in these traits at any point in time, and the genetic lag between the nucleus and commercial levels is typically 4 to 5 years. As a consequence, much of what we show here is what is in the genetic pipeline; it will take time and careful data recording to actually notice the results at the commercial level. See also section 4.5.
4.2 Priorities: mortality rates versus numbers of dead piglets
When litter size increases over time, the production of a particular number of weaned piglets requires progressively fewer litters, especially when piglet mortality rates decrease at the same time. As we mentioned in connection to Figure 1B, a particular number of annually weaned piglets is one of the main production targets of any modern pig production system; this is then a useful basis for assessing two sustainability issues of such a system: animal welfare as it is impacted by piglet mortality and the environmental footprint of production. These issues can be usefully quantified by analyzing the number of litters and associated number of dead piglets for every, say, 1,000 weaned piglets.
Figure 6 presents these data, based on the results of our phenotypic analysis in the previous sections. From 2001 to 2021, the number of litters required to produce 1,000 weaned piglets in this population decreased from 126 to 80 (because litter size at weaning increased, phenotypically, from 8.0 to 12.6). The associated absolute numbers of dead piglets decreased from 268 to 172 farrowing mortalities (stillbirths), from 183 to 76 lactation mortalities, and hence from 451 to 248 total mortalities. Hence the total number of dead piglets in such a system almost halved in this 20-year period, and the percentage of these that were lactation mortalities (a much more important animal welfare issue than farrowing mortality) decreased from 41% to 31%.
The US state of Iowa produced a similar number (22 million) of slaughter pigs in 1960 as Denmark did in 2001 (Danske, 2002; Putman et al., 2018, their Figure 2), which must have required about 23.4 million weaned piglets in each case. With 6.9 piglets weaned per litter (according to the data for Canada around 1960 used in Figure 1), this amounts to 3.39 million litters; using those same Canadian mortality levels (which are conservative, given the difference between a research station and commercial production conditions at that time) this must have led to 3.39 million × 8.9 × 0.072 = 2.17 million farrowing mortalities and 3.33 million × 8.26 × 0.164 = 4.59 million lactation mortalities that year, i.e., 18,500 dead piglets per day. In other words, at the 2001 Danish annual production volume of 22 million slaughter pigs, a little less than 20,000 dead piglets per day has been the accepted mortality level in the Western world for at least 60 years—fully in line with Baxter and Edwards’s (2018) notion that “a certain amount of piglet mortality is a predisposed and inevitable event”. Figure 1B also shows that a hypothetical annual hunting harvest of 22 million wild boar (i.e., about six times the current European total) would typically involve 48,000 lactation mortalities (plus 22,000 farrowing mortalities not shown in Figure 1, optimistically assuming 10% post-weaning mortality) per day, which would be unlikely to attract public attention.
As an alternative approach, based on our genetic analysis of these same maternal lines, we considered the following (see Appendix 2 for derivations):
1. The EBV for number born alive (NBA) follows from total number born (TNB) and from number stillborn (NSB) as:
2. The EBV for litter size at weaning (ebvLSW) follows from NBA and from the lactation mortality rate (LAMR) as:
where mLAMR and mNBA denote the population means of LAMR and NBA, respectively, across the reporting period.
3. The number of litters required to produce 1,000 weaned piglets (L1000) is 1,000/LSW. The annual change in L1000 (dL1000) follows from the annual change in LSW (dLSW) as:
where mLSW is the population mean of LSW across the reporting period.
4. The associated number of farrowing mortalities (FAM) in those litters is L1000 × NSB. The annual change in FAM (dFAM) follows from dL1000 and from the annual change in NSB (dNSB) as:
where mL1000 is the average value of L1000 across the reporting period.
5. The associated number of lactation mortalities (LAM) in those litters is L1000 × NBA × LAMR. The annual change in LAM (dLAM) follows from dL1000, from dNBA and from the annual change in LAMR (dLAMR) as:
From the linear regression coefficients in Figure 3 (i.e., the realized annual genetic trends), dLSW = +0.418 piglets per year, dNBA = +0.374 piglets per year, dNSB = –0.059 piglets per year, and dLAMR = –0.00785 proportional units (i.e., –0.785%) per year. The population means across the reporting period are mLSW = 11.4 piglets, mNBA = 12.6 piglets, mNSB = 2.19 piglets, and mLAMR = 0.0995 (proportion). Consequently, dL1000 = –3.22 litters per year, dFAM = –12.21 piglets per year, and dLAM = –9.47 piglets per year. Across the 20-year period 2001–2021, the realized genetic trends would then reduce the number of litters required to produce 1,000 weaned piglets by 64 litters, the associated number of farrowing mortalities (stillbirths) by 244 piglets, the number of lactation mortalities by 189 piglets, and, therefore, the total number of dead piglets by 433 piglets. By contrast, the realized phenotypic trends in Figure 6 represent reductions of 46 litters and 96 + 107 dead piglets. Hence the phenotypic expression over time of these characteristics was 72%, 39%, 57%, and 47% of the genetic potential; therefore, there is also a strong need for non-genetic strategies to reduce piglet mortality—see section 4.5. Animal management (including nutrition) must keep up with genetic improvement to ensure that genetic potential is actually expressed as phenotypic performance; the two approaches have to work together.
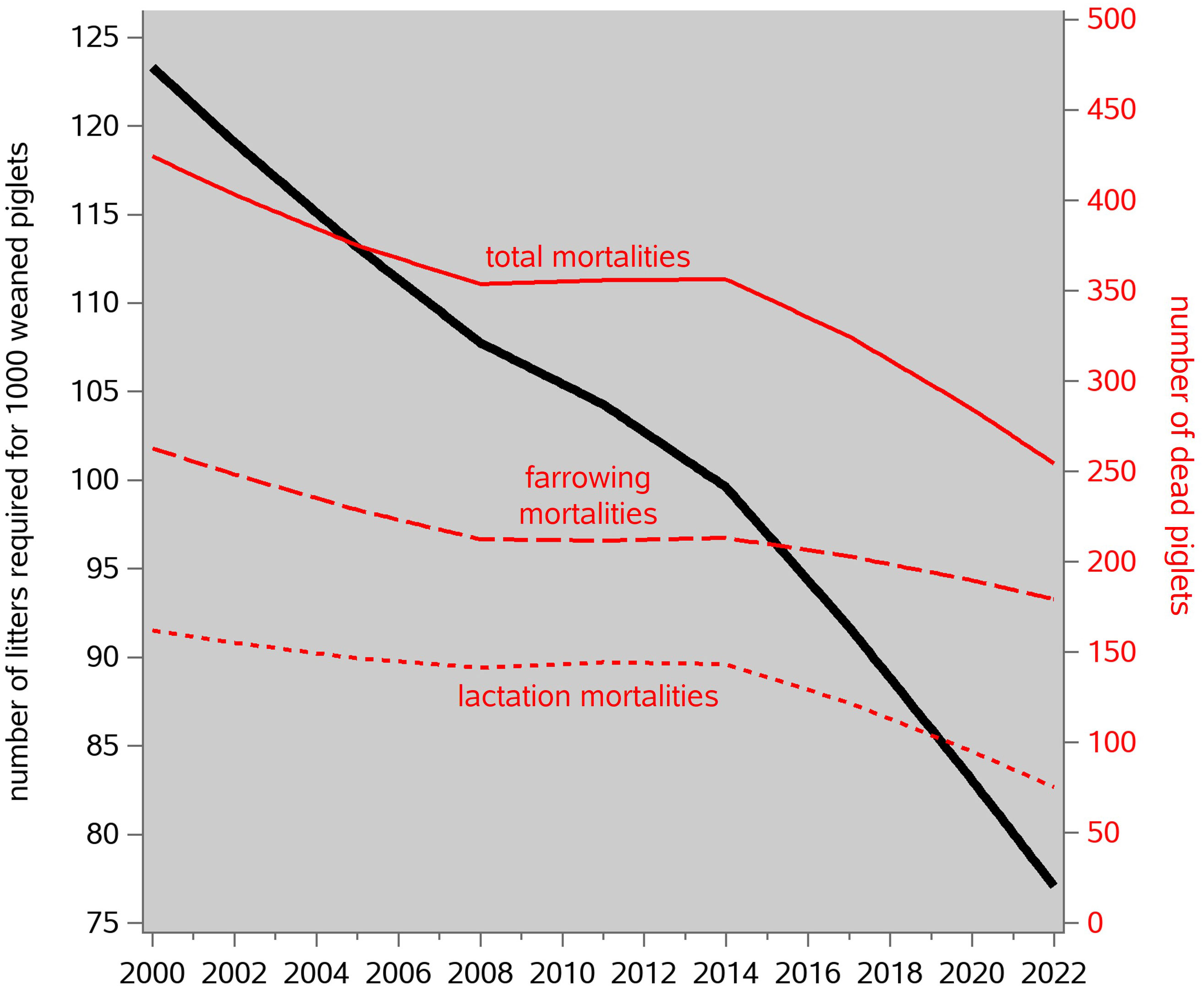
Figure 6 Time trends of the number of litters required to produce 1,000 weaned piglets, and the associated numbers of dead piglets, based on the patterns of total number born, and farrowing and lactation mortality rate, shown in Figure 5.
Notice that “fewer litters” require fewer sows; as a direct result of that, it has been possible to reduce the sow herd in most European countries by 25% since 2000, whereas the number of pigs slaughtered increased by a similar proportion. This has made a significant contribution to reducing the overall environmental footprint per kilogram of pig meat; it would be interesting to quantify this in more detail, but that is beyond the scope of this article.
4.3 Indicator trait: birth weight
As mentioned above, Bidanel et al. (2020) listed piglet birth weight and its within-litter variation as selection criteria that were implemented to manage piglet mortality in France. This strategy was recommended by Roehe (1999) and Roehe and Kalm (2000), who noticed that birth weight is favorably correlated to, and has a higher heritability than, preweaning survival. They also noticed that it is a continuous trait rather than a binary one, which makes breeding value estimation much more robust. By contrast, at that same time (Knol, 2001, pp. 86 and 96) concluded the following: “it is unlikely that direct selection for birth weight [ … ] will increase survival. [ … ] Selection for litter size [ … ] will decrease birth weight (rG = –0.49) and increase pre-weaning mortality (rG = 0.45), giving rise to the idea that selection for increased birth weight will increase pre-weaning survival, while the direct correlation between the two is only 0.11. [ … ] Selection on birth weight as an indirect way to improve piglet survival is not a good strategy”. Vanden Hole et al. (2018) studied locomotor development in low-weight versus normal-weight piglets (this is relevant to piglet survival because slow-moving piglets are more at risk of being crushed by the sow when she lies down) and found a “difference in neuromotor skills at birth. This implies a difference in neuromotor development in utero, indicating that low birth weight piglets might be considered a fictitious younger version of normal piglets”. Similarly (Edwards et al. (2019), pp. 212–213) wrote the following on intrauterine growth retardation (IUGR) due to intrauterine crowding: “piglets which show IUGR characteristics [ … ] also show other differences which indicate reduced maturity at birth” in terms of brain, muscle, heart and kidney physiology. Leenhouwers et al. (2002) concluded that “observed differences in fetal development in relation to [the EBV for piglet survival] suggest a higher degree of physiological maturity in litters with high EBVs. [ … ] The results imply that selection for improved piglet survival will lead to slightly smaller piglets that nevertheless have an improved ability to cope with hazards during birth or within the first days of life”; Knol et al. (2022) gave a more detailed review of these issues. In summary, the role of birth weight in piglet mortality is still under debate and the more relevant trait may be the newborn piglet’s stage of fetal maturity—although weight may still be a convenient proxy to measure this.
Figure 7A shows the well-known phenotypic pattern of linearly decreasing average birth weight with increasing TNB. The unfavorable slope becomes gradually less negative over time, partly neutralizing the antagonism of birth weight with TNB. According to the inset in this graph, the literature does not show that same time pattern across other (disconnected) pig populations. The within-litter standard deviation of birth weight (Figure 7B) decreases over time, with more volatility than the increase of the average in 7A: the unfavorable slope remains but the intercept goes steadily down. The within-litter coefficient of variation (standard deviation as a proportion of the average; Figure 7C) follows that same pattern but in a much more stable way. The inset in this graph shows that same phenotypic time pattern across other (disconnected) pig populations. It follows that the antagonism of birth weight uniformity with TNB is also gradually neutralized over time. Again, the R2 is very low for all these patterns.
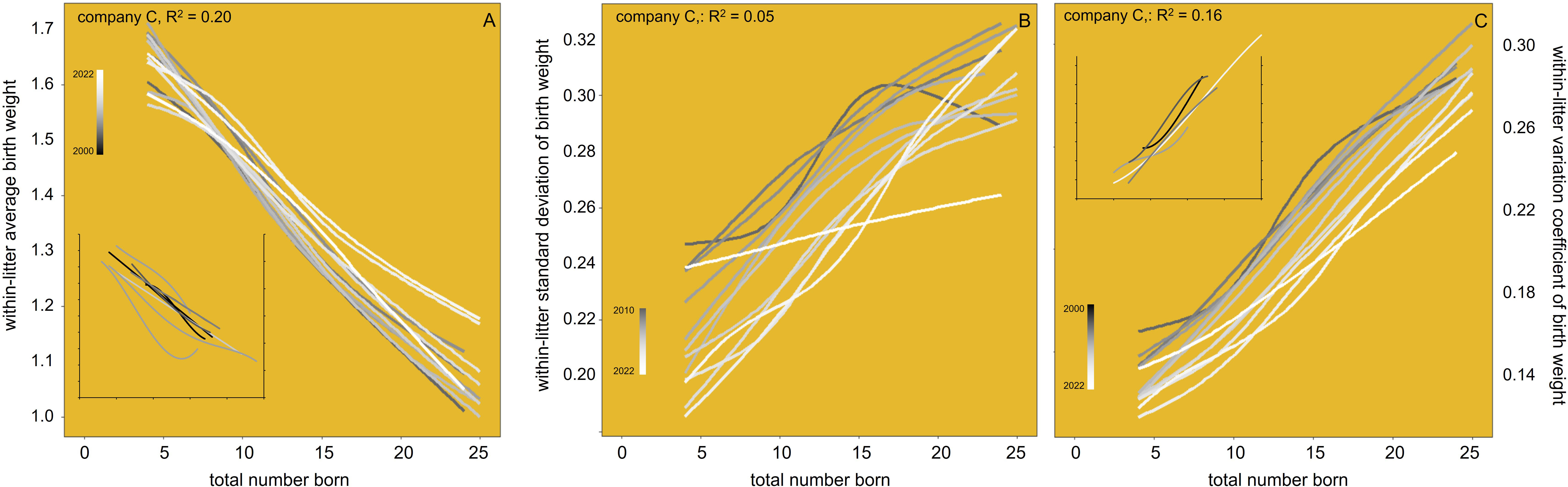
Figure 7 Within-litter average (A), standard deviation (B), and variation coefficient (C) of birth weight in relation to litter size (total number born, TNB) in the maternal lines of one breeding company, by recording year from 2010 to 2022. The annual trendlines are Locally Estimated Scatterplot Smoothing (LOESS) interpolation plots. The insets have axes with the same scale as the main graphs; their trendlines are cubic polynomials, showing comparable trends in other maternal lines (data recorded in Germany in 1988–1994: Roehe and Kalm, 1997; in the UK in the early 1990s: Kerr and Cameron, 1995; in the USA in 1993–2006: Smit, 2007; in France in 1998–2000: Quiniou et al., 2002; in France in 2000–2004: Quesnel et al., 2008; in the Czech Republic in the early 2000s: Čechová, 2006; in the USA in 2008: Bergstrom, 2011; in the USA in 2009–2013: Krahn, 2015; in Sweden in 2011–2012: Wahlgren, 2018; and in the UK in 2015–2016: Edwards et al., 2019).
The within-litter variation of birth weight quantifies the proportion of very small piglets, which are particularly at risk (Knol (2001), p.96) argued that “[lack of] variation in birth weight is important for survival and it shows genetic variation. An indirect strategy to improve piglet survival could be to select for decreased variation in birth weight”. This may be why both the average birth weight and its standard deviation were included in the French breeding goal, as mentioned at the start of this section. Quesnel et al. (2008) present the frequency distribution of piglet birth weight for various categories of litter size as recorded in France in the early 2000s; Figure 8A shows that with increasing litter size, the frequency of low birth weights increased at the expense of the frequency of high birth weights. Edwards et al. (2019) present a more detailed (and perhaps therefore very different) pattern from data recorded in the Netherlands 10 years later; we show these data in Figure 8B, rescaled the same way as in Figure 8A.
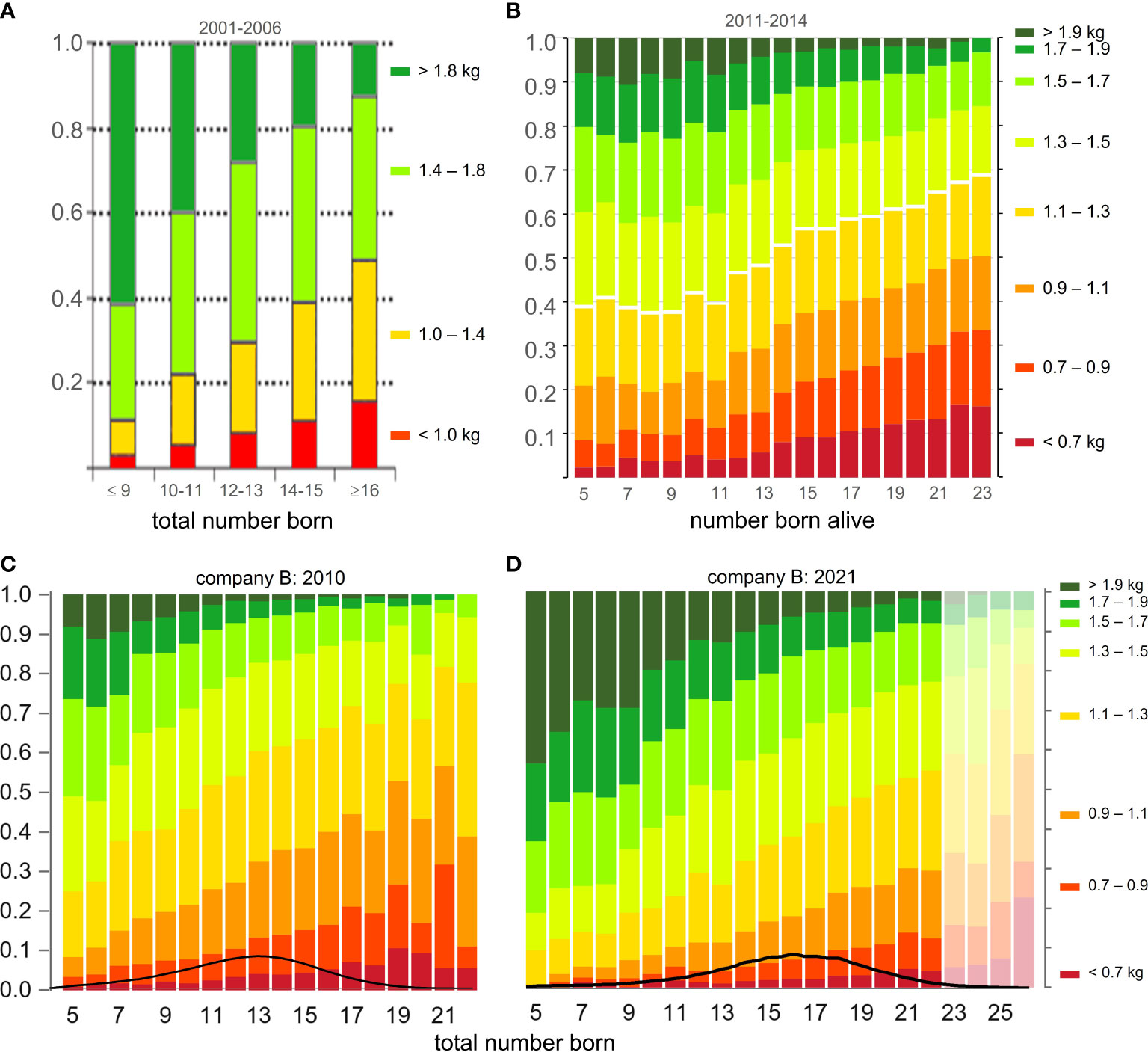
Figure 8 Distributions of piglet birth weight in relation to litter size. (A) modified from Figure 5 in Quesnel et al. (2008) and (B) modified from Figure 8 in Edwards et al. (2019) and information provided by Xandra Benthem de Grave (personal communication, 2022). (C, D) represent the maternal lines of one breeding company, as recorded in 2010 and in 2021. The bars for TNB > 22 piglets in D were dimmed to facilitate the comparison with C. The density plots at the bottom of (C, D) show the frequency distributions of TNB in each year.
In the farms where the maternal lines of one of our breeding companies are kept, the phenotypic distribution of piglet birth weight in relation to litter size in 2010 (Figure 8C) followed a somewhat more favorable pattern than what is shown in Figure 8B for data recorded a few years later on another pig population in another farm. After a further 11 years (Figure 8D), this distribution had become considerably more favorable again. For example, the proportion of piglets born in a litter of 16 with a birth weight lower than 1.1 kg (the red and orange zones) decreased from 40% in 2010 to 18% in 2021. As was shown in Figure 7, this is due to increases over time in average birth weight and its uniformity.
The lactation mortality rate in our maternal lines relates phenotypically to piglet birth weight (once more with a very low R2; see Figure 9), following patterns very similar to the 12 sources summarized by Gatford et al. (2017) and Feldpausch et al. (2019). From their own data (n = 4,068; recorded in the USA in 2008 and in Spain in 2014), Feldpausch et al. (2019) estimated a threshold birth weight level (below which the unfavorable mortality slope is considerably stronger than above it) at 1.11 kg; they also note that “additional research is warranted across different geographies, farms, and genetic lines to validate” this result. Applying their piecewise linear regression analysis to our data presented in Figure 9 produced annual estimates of this threshold birth weight level that increase from 0.76 kg in 2009 (n = 18,203) to 0.94 kg in 2016 (n = 50,766), and then decrease again to 0.85 kg in 2022 (n = 32,974), suggesting that Feldpausch et al. were correct to surmise that this issue is population dependent. In our data, the mortality slope versus birth weight becomes more negative (i.e., more unfavorable) over time (p< 0.0001) for birth weights below these very low threshold levels (which of course become less frequent over time; see the density plots at the bottom of Figure 9 and see also Figure 8), but not above them (p = 0.4). In summary, as far as birth weight has a causative effect on lactation mortality, this effect is decidedly non-linear, with most of its impact below a certain threshold value. This threshold value seems to vary between pig populations and also over time with genetic change; the proportion of piglets that fall below it (and are therefore at risk) also decreases over time with genetic change.
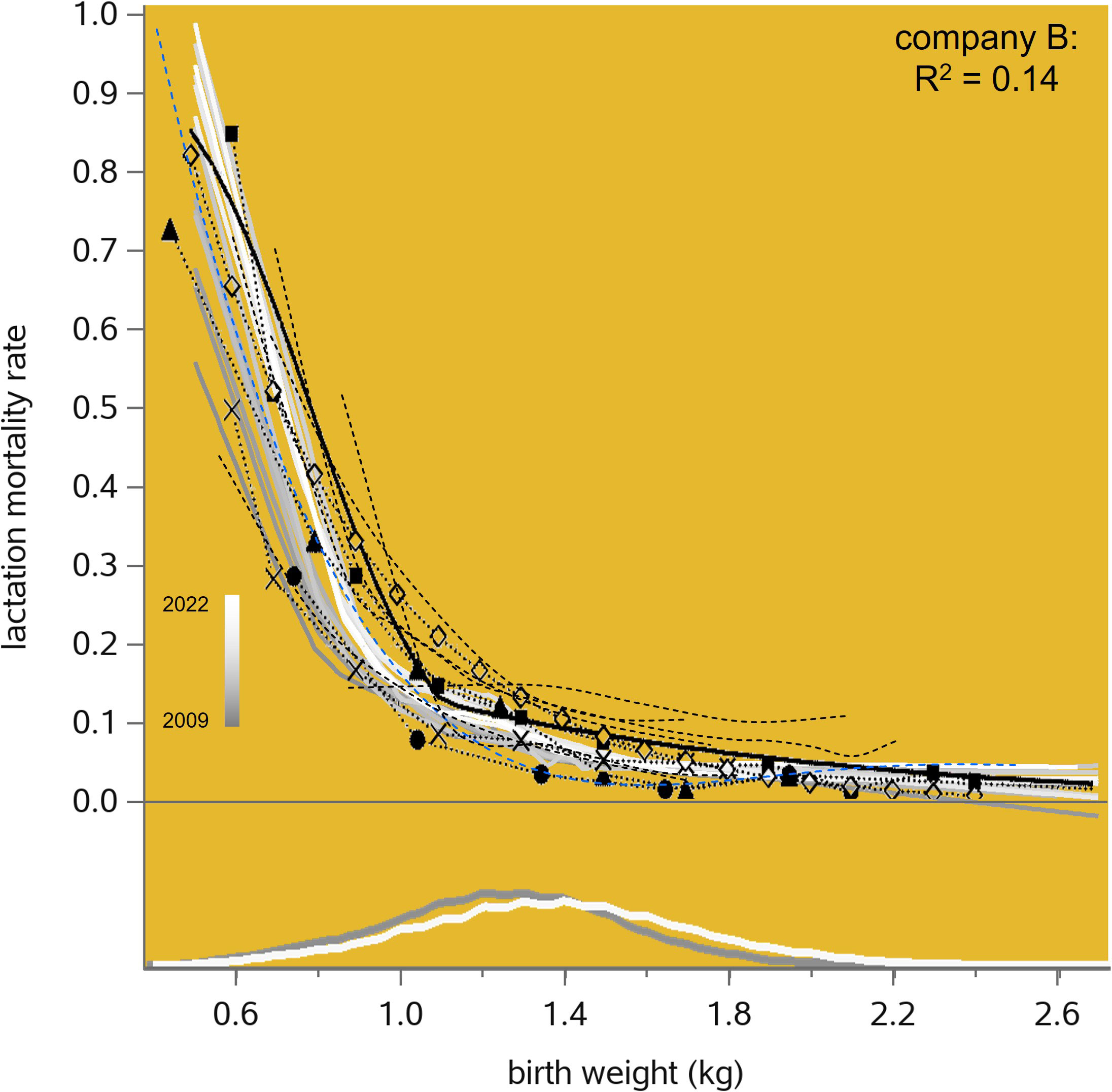
Figure 9 Lactation mortality rate in relation to birth weight in the maternal lines of one breeding company, by recording year from 2009 to 2022. The annual trendlines are Locally Estimated Scatterplot Smoothing (LOESS) interpolation plots. The density plots at the bottom of the graph show the frequency distributions of birth weight in 2009 and 2021. Comparable data presented in Figure 1 by (Gatford et al. (2017); the black dashed lines with symbols were copied from that graph) and Figure 9 by (Feldpausch et al. (2019); ditto) is superimposed.
4.4 Indicator trait: teat number
Lundeheim et al. (2013) wrote that “since the sow lacks a mammary cistern, milk is not available to piglets unless they are suckling during the actual few seconds (10–20) of milk flow [ … ]. This indicates the need of one functional teat for each piglet, and also an increase in number of teats parallel to the increased litter size, obtained by selection”. Crossfostering is a common practice in pig production: (i) piglets from large litters are transferred to smaller litters and (ii) within-litter piglet weight can be uniformized at the same time. Both these actions can improve piglet survival, particularly when they are applied together and for piglets with low birth weight (e.g., Neal and Irvin, 1991; Deen and Bilkei, 2004; Camargo et al., 2013; Vande Pol et al., 2021b). The transfer has been shown to increase damaging preweaning behavior and to reduce preweaning growth performance (but may have the opposite effect on the postweaning equivalents) when high proportions of piglets are transferred frequently, and later than 2 days after farrowing (e.g., Price et al., 1994; Straw et al., 1998a; Giroux et al., 2000; Robert and Martineau, 2001; Wattanaphansak et al., 2002; King et al., 2020; Zhang et al., 2021). Therefore, the conventional recommendation is to crossfoster between 12 and 24 h after farrowing to safeguard colostrum intake and to be ahead of teat fidelity establishment (e.g., Cutler et al., 2006; Alexopoulos et al., 2018); a practical guide by one of our breeding companies is available at https://tinyurl.com/3cej4pbd. Much of the evidence comes either from trials with very low numbers of records (e.g., Price et al., 1994: 24 litters; Robert and Martineau, 2001: 14 litters; Bierhals et al., 2011: 30 litters; Heim et al., 2012: 39 litters; Ramsay et al., 2018: 28 litters) or from analyses of field data with confounded effects of litter size and/or birth weight (e.g., Straw et al., 1998b; Camargo et al., 2013; Calderón Díaz et al., 2018). Crossfostering is the main way to ensure that each suckling piglet has a teat available to supply it with milk; obviously it is more effective in large farms where many sows that have recently farrowed can adopt very young piglets. As Lundeheim noted above (and as we show in this article), the number born alive (NBA) has increased over time due to selection for total number born and farrowing survival. Teat number (TTN) has also always been an important balanced breeding goal trait (e.g., Marois and Larochelle, 2008; Van Son et al., 2015; Knol et al., 2016). The objective is to keep both traits (NBA and TTN), and their rates of genetic improvement, in functional balance with each other. At the farm level, the critical parameter is the average difference between TTN and NBA. In line with this, Oliviero (2022) defined hyperprolific sows as “the ones that give birth to more piglets than their functional teats”.
This critical parameter can be usefully described in terms of its phenotypic time trend in the maternal lines studied here, as in Figure 10A where its frequency distribution is shown by birth year of the sow, from 2012 to 2021. We see here that in these pure lines the number of teats increases more slowly over time than the number born alive does, but the frequency distributions are skewed to the right, so that in each year there were many more litters (especially smaller ones at the early parities) with a surplus of teats than with a shortage. Hence there is ample opportunity for crossfostering. After adjustment for parity number and heterosis to convert to the more relevant commercial levels, the time trend of this surplus is shown in Figure 10B: because the number born alive has increased faster than teat number, the surplus has gradually decreased in all three populations, until it was redirected between 2019 and 2021. It is important to note that the impact of all this on piglet mortality is very limited: Figure 10C shows that the correlation between teat number and lactation mortality in systems with sufficient opportunity for crossfostering is essentially zero.
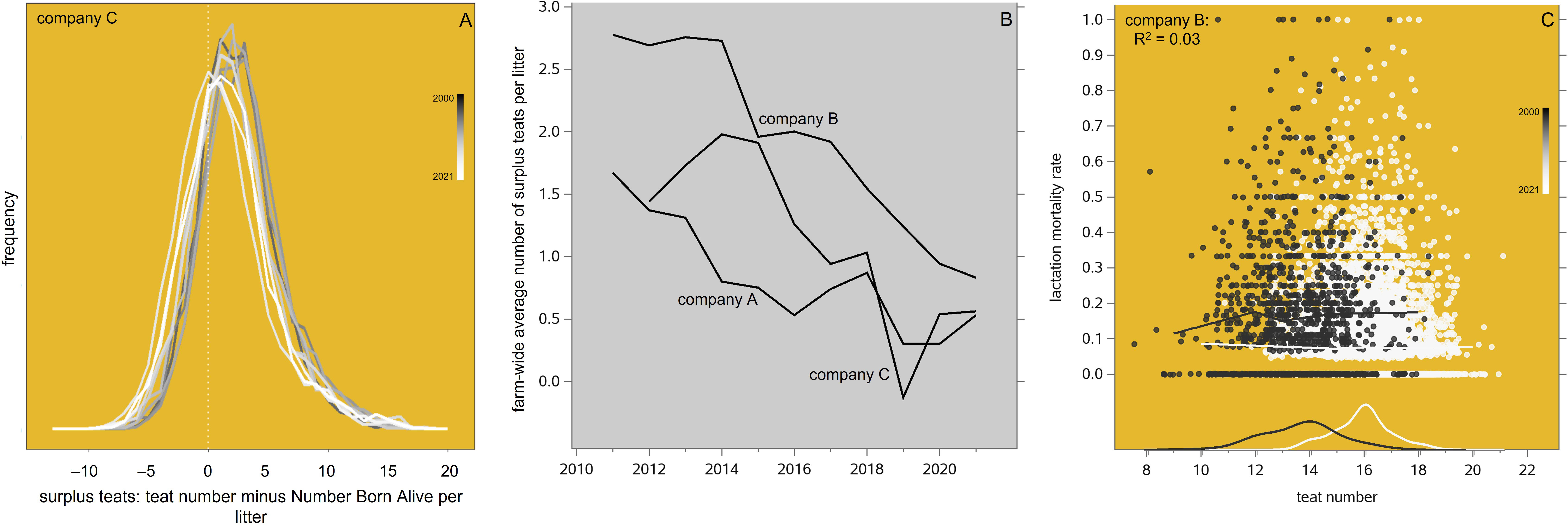
Figure 10 (A) Frequency distributions of the phenotypic difference between teat number and number born alive (TTN – NBA) in the pureline maternal lines of one breeding company, by recording year from 2012 to 2021. (B) Time trends of the phenotypic surplus teat number per litter in the maternal lines of three breeding companies, after adjustment to bring the pureline nucleus levels down to crossbred commercial levels. (C) Lactation mortality rate in relation to teat number in the maternal lines of one breeding company, in the recording years 2001 and 2021; the datapoints have been jittered to make them all visible. The trendlines are Locally Estimated Scatterplot Smoothing (LOESS) interpolation plots. The density plots at the bottom of the graph show the frequency distributions of teat number in those years.
4.5 Non-genetic strategies to reduce piglet mortality
There is a very extensive literature on animal management and healthcare and nutrition for sows and their piglets. Helpful reviews include those by Pluske et al. (1995), Le Dividich et al. (2003), Rutherford et al. (2011), Kirkden et al. (2013), Edwards and Baxter (2015), Sanjoaquin Romero (2019), Baxter et al. (2020), Farmer and Edwards (2021), Threadgold et al. (2021), Tucker et al. (2021), and Vande Pol et al. (2021a). The most relevant issues mentioned in these reviews are commonly included in technical services manuals issued by pig breeding companies; see for example https://tinyurl.com/2p8umm84, https://tinyurl.com/2t3pnbc4, https://tinyurl.com/3cn5j4jv, and https://tinyurl.com/msa8bd7y. Of course, these strategies must go hand in hand with genetic improvement.
4.6 Future genetic strategies: constraints and opportunities
An increase in litter size, piglet survival, and piglet birth weight will naturally increase the fetal mass to be accommodated in the sow’s uterus; a critical factor is therefore uterine capacity and, consequently, the mature size of the sow. We can extrapolate from the results of a divergent selection experiment for litter size in mouse lines (Rauw et al., 1999; Holt et al., 2005). Around the 100th generation, the low and high selection lines had reached 11.5 and 22.7 total number born (TNB) at mature maternal weights of 31.2 and 43.0 g, respectively. Hence with logarithmic regression, a 1% increase in TNB caused a 0.47% increase in mature weight; applying this value to pigs with a TNB at 16 suggests that mature sow weight will increase from 275 kg by 0.47 × (1/16) × 275 = 8 kg per additional piglet born per litter—this is an underestimate because it does not take increasing birth weight (and therefore fetal size) into account. This represents a constraint for pig producers because from time to time it will require a redesign of farrowing facilities and feeding standards.
Preweaning mortality is influenced by the genetic effects of three actors: the piglet, its dam, and the sow that is nursing it. The genetic effect of the piglet derives from its dam and its sire; the dam and the nursing sow are often the same individual. This structure makes it difficult to separate these genetic effects statistically and to quantify their respective contributions to mortality. For example, Knol et al. (2002) estimated variance components for preweaning mortality in two breeds: a dam line with 2,820 mostly crossbred litters with 18% crossfostering, and a sire line with 2,874 purebred litters with 6% crossfostering. Analysis with a model that included all three of the abovementioned effects produced unrealistic variance components for the dam line, and for the sire line produced heritability estimates of 0.01 (piglet), 0.24 (dam), and 0.15 (nursing sow). Genetic correlations in the sire line were –0.6 (piglet × dam), +0.4 (piglet × nursing sow), and +0.9 (dam × nursing sow): a highly unrealistic pattern but only the +0.9 estimate was significantly different from zero. A model with only the piglet and nursing sow effects produced heritabilities between 0.01 and 0.04, and genetic correlations at +0.7 (for the dam line) and –0.6 (for the sire line). It follows that (i) all three actors influence mortality, (ii) dam and nursing sow influence mortality more so than the piglet (and its sire), and (iii) this is a very demanding statistical exercise with (iv) separation of the dam and nursing sow effects being the most challenging part. The latter issue would require much more intensive crossfostering than the 6% in Knol’s data; the ideal data structure for this purpose would be complete randomization of all newborn piglets across all relevant sows in the herd. Different sows would then receive different numbers of piglets (mostly different from their initial litter size), and this would lead to randomization of the postdistribution litter size across the sows’ genetic potential for litter size. An interesting question is then if and how the currently perceived genetic antagonism between litter size and preweaning mortality would change. Based on the annual correlations between EBVs in Figure 4B (+0.3), the number of records (i.e., fully crossfostered litters with traceable piglets from pedigreed parents) required to produce such genetic correlation estimates with 95% significance and 95% statistical power can be estimated at 39,000 (shiny.cnsgenomics.com/gctaPower, which implements theory by Visscher et al., 2014). Given proper genetic parameter estimates from such a massive exercise, routine breeding value estimation may not require complete randomization; a (decidedly non-random) crossfostering intensity of double the commercial level of around 10% may be sufficient to resolve the confounding. This can only produce meaningful results if crossfostering is carried out only once and very soon after farrowing so that piglet welfare will be minimally impacted (e.g., Bierhals et al., 2011; Heim et al., 2012).
Figure 9 shows that lactation mortality relates to birth weight in a clear diminishing-returns pattern: above a birth weight of 1.5 kg the relationship is basically absent, and such birth weights are likely to contribute to the intrauterine fetal competition mentioned at the end of section 1.2 and to dystocia (e.g., Nam and Sukon, 2022). Kapell et al. (2011) analyzed farrowing mortality and birth weight data in five pig lines and concluded that the variation in genetic parameter estimates among them “indicates that the strategy of selection for an optimal birth weight with lowest variation within litter should be considered per line individually, to maximize overall genetic improvement in piglet survival and growth”. Hence the most promising short-term strategy may be to increase average birth weight to somewhat less than 1.5 kg and then keep it at that intermediate optimum level while reducing within-litter variation; this could involve a quadratic index approach (e.g., Céron-Rojas and Crossa, 2018, pp. 38–40) or explicit selection for lower within-litter variation (e.g., Sell-Kubiak et al., 2015; Blasco et al., 2017).
An increase in litter size, piglet survival, and piglet birth weight will also increase the number of piglets to be supplied with milk and their total biomass; critical factors are then teat number and resource allocation for the mammary glands. As mentioned above, teat number can easily be genetically improved. The functioning of the mammary glands has a useful proxy in litter weaning weight if it is properly adjusted for litter size at farrowing, and this trait can also be genetically improved quite easily (e.g., Figure 4B in Knap, 2014). An important issue here is that with increased udder development and milk production, the lactating sow will have to increase her protein intake, which involves an increase in the protein content of lactating sow feed, or of the sow’s lactation feed intake capacity, or both.
In Figure 1A the “balanced-2018” datapoint coincides with the Meishan datapoint, and the “balanced-2022” datapoint is more favorable yet, in both its dimensions. This recalls the speculation of (Knol, 2001, p. 96) that “it is possible to change current commercial lines to ones which will resemble the Meishan breed [ … ] it would take some 15 generations to change a Large White into a Meishan" in terms of litter size, birth weight, and birth weight variation. It seems that balanced breeding in European maternal pig lines has indeed achieved a similar pattern in terms of litter size and lactation mortality over approximately 22 years, which is 15 pig generations at the nucleus breeding level. Knol continued to speculate that “other information from Meishan studies might also be relevant for direct use”, and such studies have focused on issues such as amino acid, glucose, and lipid metabolism and their connections to neonate hypoglycemia and the resulting hypothermia, and to fetal maturation patterns (Fainberg et al., 2012; Yao et al., 2017; Voillet et al., 2018; Lefort et al., 2020; Villanueva-García et al., 2021). From a different perspective, Vonnahme et al. (2002) and Miles and Vallet (2021) studied placental development. Those studies involve transcriptomics, proteomics, and metabolomics, and these technologies are now gradually becoming available for routine animal breeding; as a result, we may indeed see more technology-driven improvements in piglet survival rates. Placental development is functionally related to intrauterine crowding, and studies of the genetic aspects of intrauterine growth retardation (Matheson et al., 2018) may lead to biomarkers that can feature in the metabolomics approach.
The (EFSA AHAW Panel et al., 2022, p. 154) mentioned that piglet “mortality increases by 24% [a range of 3% to 59%] for non-crated sows compared with crated ones. The uncertainty is mainly caused by lacking representativeness of the selected countries for the whole of Europe”. More specifically, Olsson et al. (2018) compared loose-housed and temporarily confined farrowing sows in the same farm, and found that “on average, 0.4 more pigs per litter survived until weaning if the sow was temporarily confined compared with being loose” (p = 0.03). It follows that the currently imminent European policy shift toward free farrowing systems (as recommended by the EFSA AHAW Panel et al., 2022, p. 275) will likely require the pig breeding sector to place additional focus on piglet mortality in the breeding goals to counter the adverse effects of the novel conditions and unaccustomed farm staff.
5 Conclusions and recommendations
Selection for piglet survival works: since the early 2000s, balanced pig breeding policies have been successful in increasing litter size while simultaneously increasing piglet survival rate. This is an ongoing process, and we expect it to improve over time with the introduction of novel technology and the continued leverage of increasing selection intensity. Genetics is clearly part of the solution.
Indicator traits help, but do not form the full solution: the role of piglet birth weight as a causative factor of piglet mortality is still unclear and fetal maturity may be a more relevant trait. Still, balanced breeding policies have increased birth weight simultaneously with increasing litter size.
The within-litter variation in piglet birth weight is a more likely causative factor of piglet mortality. This variation is most appropriately quantified in terms of the variation coefficient (i.e., the standard deviation divided by the mean). Balanced breeding policies are reducing the variation of birth weight while simultaneously increasing litter size.
Earlier published threshold levels of piglet birth weight (such as 1.1 kg, below which mortality rates were exceptionally high in that population) are clearly population specific, and, therefore, not generally valid. Because birth weight and its uniformity are increasing as a result of balanced breeding, these threshold levels are also less and less relevant.
Increases in litter size and piglet survival rates due to balanced breeding policies lead to reduced total numbers of dead piglets (e.g., per country, per year) coinciding with a certain pig production volume (i.e., with a certain total number of weaned piglets). These increases also lead to a reduction in the size of the sow herd and in the related overhead costs, which improves the environmental footprint of a kg of pig meat.
Owing to insufficient transparency and communication from the pig breeding sector, the scientific literature, public opinion, and policy makers are not up to date with modern pig breeding strategies. This has to change, and it is our sincere hope that this article will contribute to that.
Data availability statement
The data analyzed in this study is subject to the following licenses/restrictions: the datasets were extracted from the routine commercial databases of four mutually competing breeding companies. They are not publicly accessible. Requests to access these datasets should be directed at cGlldGVyLmtuYXBAZ2VudXNwbGMuY29t.
Ethics statement
Ethics review and approval were not required for the animal study in accordance with local legislation and institutional requirements. Written informed consent from the owners for the participation of their animals in this study was not required in accordance with national legislation and institutional requirements.
Author contributions
Conceptualization: CL and PK. First analyses and first draft of the manuscript: PK. Subsequent analyses and additions to the manuscript: EK, ACS, AH, DvdS, LZ, and AG. All authors contributed to the article and approved the submitted version.
Acknowledgments
The authors would like to thank Xandra Benthem de Grave, Agustín Blasco, Regina Imhäuser, and Jan Jourquin.
Conflict of interest
Authors PK and CL are employed by the company Genus-PIC.
The remaining authors declare that the research was conducted in the absence of any commercial or financial relationships that could be construed as a potential conflict of interest.
Publisher’s note
All claims expressed in this article are solely those of the authors and do not necessarily represent those of their affiliated organizations, or those of the publisher, the editors and the reviewers. Any product that may be evaluated in this article, or claim that may be made by its manufacturer, is not guaranteed or endorsed by the publisher.
Supplementary material
The Supplementary Material for this article can be found online at: https://www.frontiersin.org/articles/10.3389/fanim.2023.1218175/full#supplementary-material
References
Alexopoulos J. G., Lines D. S., Hallett S., Plush K. J. (2018). A review of success factors for piglet fostering in lactation. Animals 8, 38. doi: 10.3390/ani8030038
Arp A. (2022). Dødelighed hos pattegrise slår ny rekord: 29.514 dør om dagen (Copenhagen: Altinget). Available at: https://www.altinget.dk/artikel/doedelighed-hos-pattegrise-slaar-ny-rekord-29514-doer-om-dagen.
Barrett R. H. (1978). The feral hog on the dye creek ranch, California. Hilgardia 46 (9), 283–355. doi: 10.3733/hilg.v46n09p283
Baxter E. M., Edwards S. A. (2018). “Piglet mortality and morbidity: inevitable or unacceptable?,” in Advances in pig welfare. Ed. Špinka M. (Cambridge, UK: Woodhead Publishing), 73–100. doi: 10.1016/B978-0-08-101012-9.00003-4
Baxter E. M., Schmitt O., Pedersen L. J. (2020). “Managing the litter from hyperprolific sows,” in The suckling and weaned piglet. Ed. Farmer C. (Netherlands: Wageningen Academic Publishers), 71–106. Available at: doi.10.3920/978-90-8686-894-0_3.
Bergstrom J. R. (2011). Effects of birth weight, finishing feeder design, and dietary astaxanthin and ractopamine HCl on the growth, carcass, and pork quality characteristics of pigs; and meta-analyses to improve the prediction of pork fat quality (USA: Kansas State University). Available at: https://krex.k-state.edu/dspace/handle/2097/9218.
Bidanel J. P. (2011). “Biology and genetics of reproduction,” in The genetics of the pig. Eds. Rothschild M. F., Ruvinsky A. (Wallingford, UK: CABI), 218–241.
Bidanel J. P., Caritez J. C., Legault C. (1989). Estimation of crossbreeding parameters between Large white and meishan porcine breeds. 1: reproductive performance. Genet. Selection Evol. 21, 507–526. doi: 10.1051/gse:19890410
Bidanel J. P., Gruand J., Legault C. (1994). “An overview of twenty years of selection for litter size in pigs using "hyperprolific" schemes,” in 5th world congress on genetics applied to livestock production (Guelph, Canada). Available at: http://www.wcgalp.org/system/files/proceedings/1994/overview-twenty-years-selection-litter-size-pigs-using-hyperprolific-schemes.pdf.
Bidanel J. P., Silalahi P., Tribout T., Canario L., Ducos A., Garreau H., et al. (2020). Cinquante années d’amélioration génétique du porc en France: bilan et perspectives. INRAE Productions Animales 33 (1), 1–16. doi: 10.20870/productions-animales.2020.33.1.3092
Bierhals T., Gonçalves Mellagi A. P., Heim G., Bernardi M. L., Wentz I., Bortolozzo F. P. (2011). Desempenho de leitegadas após a uniformização cruzada de leitões entre fêmeas de ordem de parto 1 e 5. Acta Scientiae Veterinariae 39 (1), 942. Available at: https://tinyurl.com/2yaaus9c.
Blasco A., Martínez−Álvaro M., García M. L., Ibáñez−Escriche N., Argente M. J. (2017). Selection for environmental variance of litter size in rabbits. Genet. Selection Evol. 49, 48. doi: 10.1186/s12711-017-0323-4
Calderón Díaz J. A., García Manzanilla E., Diana A., Boyle L. A. (2018). Cross-fostering implications for pig mortality, welfare and performance. Front. Veterinary Sci. 5. doi: 10.3389/fvets.2018.00123
Camargo E. G., Rego J. C. C., Dias L. T., Teixeira R. A. (2013). Effects of cross-fostering on performance of piglets. Rev. Bras. Saúde e Produção Anim. 14, 142–148. doi: 10.1590/S1519-99402013000100015
CCSI (2021). Annual report 2020 (Ottawa: Canadian Centre for Swine Improvement). Available at: https://www.ccsi.ca/meetings/annual/2020AnnualReport.pdf.
Čechová M. (2006). Analysis of some factors influencing the birth weight of piglets. Slovak J. Anim. Sci. 39 (3), 139–144. Available at: http://www.cvzv.sk/slju/06_3/Cechova.pdf.
Céron-Rojas J. J., Crossa J. (2018). Linear selection indices in modern plant breeding (Cham, Switzerland: Springer International Publishing). doi: 10.1007/978-3-319-91223-3_2
Charneca R., Vila-Viçosa M. J., Infante P., Nunes J., Le Dividich J. (2015). Colostrum production of alentejano and Large-white × landrace sows: consumption, passive immunity and mortality of piglets. Spanish J. Agric. Res. 13 (4), e0611. doi: 10.5424/sjar/2015134-7537
Chinn S. M., Kilgo J. C., Vukovich M. A., Beasley J. C. (2021). Influence of intrinsic and extrinsic attributes on neonate survival in an invasive large mammal. Sci. Rep. 11, 11033. doi: 10.1038/s41598-021-90495-x
Christensen O. F., Kongsted H., Lund M. S. (2018). Evaluering af avl for LG5 (Denmark: Aarhus University). Available at: https://pure.au.dk/portal/files/128087355/LG5_evaluering010618.pdf.
Cutler R. S., Fahy V. A., Cronin G. M., Spicer E. M. (2006). “Preweaning mortality,” in Diseases of swine, 9th edition. Eds. Straw B. E., Zimmerman J. J., D’Allaire S., Taylor D. J. (Ames IO, USA: Blackwell), 993–1009.
Danske S. (2002). Statistik 2001 (Copenhagen: Danske Slagterier). Available at: https://lf.dk/-/media/lf/tal-og-analyser/aarsstatistikker/statistik-svin/2001-/aarsstat2001.pdf.
Deen M. G. H., Bilkei G. (2004). Cross fostering of low-birthweight piglets. Livestock Production Sci. 90, 79–284. doi: 10.1016/j.livprodsci.2004.02.012
Edwards S., Baxter E. (2015). “Piglet mortality: causes and prevention,” in The gestating and lactating sow. Ed. Farmer C. (Netherlands: Wageningen Academic Publishers), 253–278. doi: 10.3920/978-90-8686-803-2
Edwards S., Matheson S., Baxter E. (2019). “Genetic influences on intra-uterine growth retardation of piglets and management interventions for low birth weight piglets,” in Nutrition of hyperprolific sows. Ed. Yagüe A. P. (Saint Charles MI, USA: Libro Novus), 141–166. Available at: https://www.novusint.com/pages/nutritionofhyperprolificsows.
EFSA AHAW Panel, Nielsen S. S., Alvarez J., Bicout D. J., Calistri P., Canali E., et al. (2022). Scientific opinion on the welfare of pigs on farm. EFSA J. 20 (8), 7421. doi: 10.2903/j.efsa.2022.7421
Fahmy M. H., Bernard C. (1971). Causes of mortality in Yorkshire pigs from birth to 20 weeks of age. Can. J. Anim. Sci. 51, 351–359. doi: 10.4141/cjas71-048
Fainberg H. P., Bodley K., Bacardit J., Li D., Wessely F., Mongan N. P., et al. (2012). Reduced neonatal mortality in meishan piglets: a role for hepatic fatty acids? PloS One 7 (11), e49101. doi: 10.1371/journal.pone.0049101
Farmer C., Edwards S. A. (2021). Improving the performance of neonatal piglets. Animal 100350. doi: 10.1016/j.animal.2021.100350
Feldpausch J. A., Jourquin J., Bergstrom J. R., Bargen J. L., Bokenkroger C. D., Davis D. L., et al. (2019). Birth weight threshold for identifying piglets at risk for preweaning mortality. Trans. Anim. Sci. 3, 633–640. doi: 10.1093/tas/txz076
Gaskins H. R., Kelley K. W. (1995). “Immunology and neonatal mortality,” in The neonatal pig: development and survival. Ed. Varley M. A. (Wallingford, UK: CABI), 39–56.
Gatford K. L., Roberts C. T., Kind K. L., Hynd P. I. (2017). Off to the right start: how pregnancy and early life can determine future animal health and production. Anim. Production Sci. 58 (3), 459–475. doi: 10.1071/AN17014
Giroux S., Robert S., Martineau G. P. (2000). The effects of cross-fostering on growth rate and post-weaning behavior of segregated early-weaned piglets. Can. J. Anim. Sci. 80 (4), 533–538. doi: 10.4141/A99-122
González F., Vargas J. D., Robledo J., Prieto L., Andrada J. A., Aparicio M. A. (2007). “Influence of environmental conditions in Iberian pig rearing systems,” in Proceedings of 6th international symposium on the Mediterranean pig. Eds. Nanni Costa L., Zambonelli P., Russo V., 153–160 (Italy: Bologna, DIPROVAL). Available at: https://amsacta.unibo.it/2513/3/Proceedings_6th_Symp:Mediterranean:Pig_3v.pdf.
Heim G., Mellagi A. P. G., Bierhals T., De Souza L. P., De Fries H. C. C., Piuco P., et al. (2012). Effects of cross-fostering within 24h after birth on pre-weaning behaviour, growth performance and survival rate of biological and adopted piglets. Livestock Sci. 150, 121–127. doi: 10.1016/j.livsci.2012.08.011
Henryon M., Ostersen T., Guo X., Su G., Sørensen A. C. (2022). Breeding for component traits of litter size at day 5 increases piglet survival while maintaining litter size at day 5. 12th world congress on genetics applied to livestock production (the Netherlands: Rotterdam). Available at: https://www.wageningenacademic.com/pb-assets/wagen/WCGALP2022/68_016.pdf.
Hermesch S. (2000). A first analysis of piglet mortality identifying important factors (Armidale, Australia: AGBU Pig Genetics Workshop). Available at: http://agbu.une.edu.au/pig_genetics/pdf/2000/Paper7_Afirstanalysis_Hermesch_2000.pdf.
Herpin P., Le Dividich J. (1995). “Thermoregulation and the environment,” in The neonatal pig: development and survival. Ed. Varley M. A. (Wallingford, UK: CABI), 57–98.
Heuß E. M., Pröll-Cornelissen M. J., Neuhoff C., Tholen E., Große-Brinkhaus C. (2019). Piglet survival: benefits of the immunocompetence. Animal 13 (10), 2114–2124. doi: 10.1017/S1751731119000430
Holt M., Vangen O., Farstad W. (2005). Components of litter size in mice after 110 generations of selection. J. Anim. Breed. Genet. 122, 199–209. doi: 10.1530/rep.1.00118
Ibáñez-Escriche N., Varona L., Casellas J., Quintanilla R., Noguera J. L. (2009). Bayesian Threshold analysis of direct and maternal genetic parameters for piglet mortality at farrowing in Large white, landrace, and pietrain populations. J. Anim. Sci. 87, 80–87. doi: 10.2527/jas.2007-0670
Ibáñez-Escriche N., Varona L., Magallon E., Noguera J. L. (2014). “Crossbreeding effects and genetic parameters on piglet survival from three Iberian strains,” in 10th world congress on genetics applied to livestock production (Vancouver, Canada). Available at: http://www.wcgalp.org/system/files/proceedings/2014/crossbreeding-effects-and-genetic-parameters-piglet-survival-three-iberian-strains.pdf.
Jezierski W. (1977). Longevity and mortality rate in a population of wild boar. Acta Theriologica 22,24, 337–348. https://rcin.org.pl/Content/10471/PDF/BI002_2613_Cz-40-2_Acta-T22-nr24-337-348_o.pdf.
Kapell D. N. R. G., Ashworth C. J., Knap P. W., Roehe R. (2011). Genetic parameters for piglet survival, litter size and birth weight or its variation within litter in sire and dam lines using Bayesian analysis. Livestock Sci. 135, 215–224. doi: 10.1016/j.livsci.2010.07.005
Kerr J. C., Cameron N. D. (1995). Reproductive performance of pigs selected for components of efficient lean growth. Anim. Sci. 60, 281–290. doi: 10.1017/S1357729800008444
King R. L., Matheson S. M., Baxter E. M., Edwards S. A. (2020). Sow behaviour and piglet weight gain after late cross-fostering in farrowing crates and pens. Animal 14, 1923–1933. doi: 10.1017/S1751731120000580
Kirkden R. D., Broom D. M., Andersen I. L. (2013). Piglet mortality: management solutions. J. Anim. Sci. 91 (7), 3361–3389. doi: 10.2527/jas.2012-5637
Knap P. W. (2012). “Pig breeding for increased sustainability,” in Sustainable food production. Eds. Christou P., Savin R., Costa-Pierce B. A., Misztal I., Whitelaw C. B. A. (New York NY, USA: Springer Science+Business Media), 1256–1295. doi: 10.1007/978-1-4614-5797-8_342
Knap P. W. (2014). “Pig breeding goals in competitive markets,” in 10th world congress on genetics applied to livestock production (Vancouver, Canada). Available at: http://wcgalp.org/system/files/proceedings/2014/pig-breeding-goals-competitive-markets.pdf.
Knap P. W., Rauw W. M. (2009). “Selection for high productivity in pigs,” in Resource allocation theory applied to farm animal production. Ed. Rauw W. M. (Wallingford, UK: CABI), 288–301.
Knap P. W., Van Alst G. J. M., Versteeg J., Kanis E. (1993). Realized genetic improvement of litter size in Dutch pig herdbook breeding: 1984-1991. Pig News Inf. 14 (3), 119N–121N.
Knol E. F. (2001). Genetic aspects of piglet survival (Netherlands: Wageningen University). Available at: https://edepot.wur.nl/197617.
Knol E., Ducro B., Van Arendonk J., van der Lende T. (2002). Direct, maternal and nurse sow genetic effects on farrowing, pre-weaning and total piglet survival. Livestock Production Sci. 73, 153–164. doi: 10.1016/S0301-6226(01)00248-2
Knol E. F., Nielsen B., Knap P. W. (2016). Genomic selection in commercial pig breeding. Anim. Front. 6 (1), 15–11. doi: 10.2527/af.2016-0003
Knol E., van der Spek D., Zak L. (2022). Genetic aspects of piglet survival and related traits: a review. J. Anim. Sci. 100, 1–9. doi: 10.1093/jas/skac190
Krahn G. T. (2015). Comparison of piglet birth weight classes, parity of the dam, number born alive and the relationship with litter variation and piglet survival until weaning (USA: Iowa State University). Available at: https://tinyurl.com/bdhyycff.
Lay D. C., Matteri R. L., Carroll J. A., Fangman T. J., Safranski T. J. (2002). Preweaning survival in swine. J. Anim. Sci. 80 (E. Suppl. 1), E74–E86. doi: 10.2527/animalsci2002.0021881200800ES10011x
Le Dividich J., Martineau G. P., Madec F., Orgeur P. (2003). “Saving and rearing underprivileged and supernumerary piglets, and improving their health at weaning,” in Weaning the pig: concepts and consequences. Eds. Pluske J. R., Le Dividich J., Verstegen M. W. A. (Netherlands: Wageningen Academic Publishers). doi: 10.3920/978-90-8686-513-0
Leenhouwers J. I., Knol E. F., De Groot P. N., Vos H., van der Lende T. (2002). Fetal development in the pig in relation to genetic merit for piglet survival. J. Anim. Sci. 80, 1759–1770. doi: 10.2527/2002.8071759x
Lefort G., Servien R., Quesnel H., Billon Y., Canario L., Iannuccelli N., et al. (2020). The maturity in fetal pigs using a multi−fluid metabolomic approach. Sci. Rep. 10, 19912. doi: 10.1038/s41598-020-76709-8
Legault C. (1985). La mortalité des porcelets de la naissance au sevrage: aspects génétiques. Porc Magazine 174, 25–30.
Lundeheim N., Chalkias H., Rydhmer L. (2013). Genetic analysis of teat number and litter traits in pigs. Acta Agriculturae Scandinavica Section A Anim. Sci. 63, 121–125. doi: 10.1080/09064702.2013.841749
Marois D., Larochelle M. (2008). Genetic selection on number of teats (AGBU Pig Genetics Workshop). Available at: http://agbu.une.edu.au/pig_genetics/pdf/2008/Paper3_DM_Geneticselectiononnumberofteats.pdf.
Matheson S. M., Walling G. A., Edwards S. A. (2018). Genetic selection against intrauterine growth retardation in piglets: a problem at the piglet level with a solution at the sow level. Genet. Selection Evol. 50, 46. doi: 10.1186/s12711-018-0417-7
Miles J., Vallet J. L. (2021). Breed differences in placental development during late gestation between Chinese meishan and white crossbred gilts in response to intrauterine crowding. Anim. Reprod. Sci. 226, 106711. doi: 10.1016/j.anireprosci.2021.106711
Mrode R. A., Pocrnic I. (2023). Linear models for the prediction of the genetic merit of animals. 4th edition (Egham, UK: CABI).
Muñoz M., Rodríguez M. C., García-Cortes L. A., González A., García-Casco J. M., Silió L. (2017). Direct and maternal additive effects are not the main determinants of Iberian piglet perinatal mortality. J. Anim. Breed. Genet. 134 (6), 512–519. doi: 10.1111/jbg.12298
Náhlik A., Sándor G. (2003). Birth rate and offspring survival in a free-ranging wild boar sus scrofa population. Wildlife Biol. 9 (Suppl.1), 37–42. doi: 10.2981/wlb.2003.062
Nam N. H., Sukon P. (2022). Incidence of dystocia at piglet level in cloprostenol-induced farrowings and associated risk factors. Arch. Anim. Breed. 65, 97–103. doi: 10.5194/aab-65-97-2022
Neal S. M., Irvin K. M. (1991). The effects of crossfostering pigs on survival and growth. J. Anim. Sci. 69, 41–46. doi: 10.2527/1991.69141x
Neeteson-Van Nieuwenhoven A. M., Knap P. W., Avendaño S. (2013). The role of sustainable commercial pig and poultry breeding for food security. Anim. Front. 3 (1), 52–57. doi: 10.2527/af.2013-0008
Nielsen B., Henriksen T. M. (2004). “Avlsmål 2003/2004,” in Rapport 25, landsudvalget for svin (Copenhagen, Denmark). Available at: https://svineproduktion.dk/Publikationer/Kilder/lu_Rapporter/25.aspx.
Nieto R., García-Casco J., Lara L., Palma-Granados P., Izquierdo M., Hernandez F., et al. (2019). “Ibérico (Iberian) pig,” in European Local pig breeds - diversity and performance. Eds. Čandek-Potokar M., Nieto R. (London, UK: IntechOpen), 25–50. doi: 10.5772/intechopen.83765
Oliviero C. (2022). Offspring of hyperprolific sows: immunity, birthweight and heterogeneous litters. Mol. Reprod. Dev. 1-5. doi: 10.1002/mrd.23572
Olsson A. C., Botermans J., Englund J. E. (2018). Piglet mortality, a parallel comparison between loose-housed and temporarily confined farrowing sows in the same herd. Acta Agriculturae Scandinavica Section A Anim. Sci. 68, 52–62. doi: 10.1080/09064702.2018.1561934
Pandolfi F., Edwards S. A., Robert F., Kyriazakis I. (2017). Risk factors associated with the different categories of piglet perinatal mortality in French farms. Prev. Veterinary Med. 137, 1–12. doi: 10.1016/j.prevetmed.2016.12.005
Pluske J. R., Williams I. H., Aherne F. X. (1995). “Nutrition of the neonatal pig,” in The neonatal pig: development and survival. Ed. Varley M. A. (Wallingford, UK: CABI), 187–238.
Price E. O., Hutson G. D., Price M. I., Borgwardt R. (1994). Fostering in swine as affected by age of offspring. J. Anim. Sci. 72, 1697–1701. doi: 10.2527/1994.7271697x
Putman B., Hickman J., Bandekar P., Matlock M., Thoma G. (2018). A retrospective assessment of US pork productions: 1960 to 2015 (Fayetteville AR, USA: University of Arkansas Resiliency Center). Available at: https://scholarworks.uark.edu/rescentfs/2.
Quesnel H., Brossard L., Valancogne A., Quiniou N. (2008). Influence of some sow characteristics on within-litter variation of piglet birth weight. Animal 2, 1842–1849. doi: 10.1017/S175173110800308X
Quiniou N., Dagorn J., Gaudre D. (2002). Variation of piglets' birth weight and consequences on subsequent performance. Livestock Production Sci. 78, 63–70. doi: 10.1016/S0301-6226(02)00181-1
Ramsay T. G., Stoll M. J., Schreier L. L., Shannon A. E. (2018). Use of cross-fostering to enhance growth of pigs that are predicted to grow poorly based on plasma α-1 acid glycoprotein concentration. Open J. Anim. Sci. 8, 39–50. doi: 10.4236/ojas.2018.81004
Rauw W. M., Luiting P., Beilharz R. G., Verstegen M. W. A., Vangen O. (1999). Selection for litter size and its consequences for the allocation of feed resources: a concept and its implications illustrated by mice selection experiments. Livestock Production Sci. 60, 329–342. doi: 10.1016/S0301-6226(99)00104-9
Robert S., Martineau G. (2001). Effects of repeated cross-fosterings on preweaning behavior and growth performance of piglets and on maternal behavior of sows. J. Anim. Sci. 79, 88–93. doi: 10.2527/2001.79188x
Roehe R. (1999). Genetic determination of individual birth weight and its association with sow productivity traits using Bayesian analyses. J. Anim. Sci. 77, 330–343. doi: 10.2527/1999.772330x
Roehe R., Kalm E. (1997). Effiziente zucht auf fruchtbarkeit sollte geburtsgewichte einbeziehen. Schweinezucht und Schweinemast 45 (4), 38–40.
Roehe R., Kalm E. (2000). Estimation of genetic and environmental risk factors associated with preweaning mortality in piglets using generalized linear mixed models. Anim. Sci. 70, 227–240. doi: 10.1017/S1357729800054692
Roehe R., Shrestha N. P., Mekkawy W., Baxter E. M., Knap P. W., Smurthwaite K. M., et al. (2010). Genetic parameters of piglet survival and birth weight from a two-generation crossbreeding experiment under outdoor conditions designed to disentangle direct and maternal effects. J. Anim. Sci. 88, 1276–1285. doi: 10.2527/jas.2009-2287
Rutherford K. M. D., Baxter E. M., Ask B., Berg P., D'Eath R. B., Jarvis S., et al. (2011). “The ethical and welfare implications of large litter size in the domestic pig: challenges and solutions,” in Project report 17, Danish centre for bioethics and risk assessment (Denmark: University of Copenhagen). Available at: https://static-curis.ku.dk/portal/files/37642367/17_Ethics_welfare_pig_litter_size.pdf.
Sanjoaquin Romero L. (2019). “Management protocols in lactation piglets from hyperprolific sows,” in Nutrition of hyperprolific sows. Ed. Yagüe A. P. (Saint Charles MI, USA: Libro Novus), 255–270. Available at: https://www.novusint.com/pages/nutritionofhyperprolificsows.
Sell-Kubiak E., Wang S., Knol E. F., Mulder H. A. (2015). Genetic analysis of within-litter variation in piglets’ birth weight using genomic or pedigree relationship matrices. J. Anim. Sci. 93, 1471–1480. doi: 10.2527/jas2014-8674
Smit M. (2007). Study of fetal programming, sex ratio and maternal parity in pigs in a multigenerational field dataset (Netherlands: Wageningen, Wageningen University). Available at: https://tinyurl.com/34aecrme.
Straw B. E., Bürgi E. J., Dewey C. E., Duran C. O. (1998b). Effects of extensive crossfostering on performance of pigs on a farm. J. Am. Veterinary Med. Assoc. 212 (6), 855–856. Available at: https://pubmed.ncbi.nlm.nih.gov/9530427/.
Straw B. E., Dewey C. E., Bürgi E. J. (1998a). Patterns of crossfostering and piglet mortality on commercial U.S. and Canadian swine farms. Prev. Veterinary Med. 33, 83–89. doi: 10.1016/S0167-5877(97)00051-2
Threadgold T., Greenwood E. C., VanWettere W. (2021). Identifying suitable supplements to improve piglet survival during farrowing and lactation. Animals 11, 2912. doi: 10.3390/ani11102912
Tucker B. S., Craig J. R., Morrison R. S., Smits R. J., Kirkwood R. N. (2021). Piglet viability: a review of identification and pre-weaning management strategies. Animals 11, 2902. doi: 10.3390/ani11102902
Vanden Hole C., Aerts P., Prims S., Ayuso M., Van Cruchten S., Van Ginneken C., et al (2018). Does intrauterine crowding affect locomotor development? a comparative study of motor performance, neuromotor maturation and gait variability among piglets that differ in birth weight and vitality. PLoS ONE 13 (4), e0195961. doi: 10.1371/journal.pone.0195961
Vande Pol K. D., Bautista R. O., Harper H., Shull C. M., Brown C. B., Ellis M. (2021b). Effect of rearing cross-fostered piglets in litters of either uniform or mixed birth weights on preweaning growth and mortality. Trans. Anim. Sci. 5 (1), txab030. doi: 10.1093/tas/txab030
Vande Pol K. D., Tolosa A. F., Shull C. M., Brown C. B., Alencar S. A. S., Ellis M. (2021a). Effect of drying and warming piglets at birth on preweaning mortality. Trans. Anim. Sci. 5, 1–12. doi: 10.1093/tas/txab016
Van Son M., Ranberg I. A., Olsen D., Lien S., Hamland H., Grindflek E. (2015). “Genome wide association study for number of teats and inverted teats in norsvin landrace pigs,” in 66th EAAP Conference. S46–S45 (Poland: Warsaw). Available at: https://meetings.eaap.org/wp-content/uploads/2015/S46_05.pdf.
Varkens in Nood (2016). Zes miljoen varkens sterven nog voor de slacht (Amsterdam: Stichting Varkens in Nood). Available at: https://www.varkensinnood.nl/sites/default/files/2019-02/rapport-zes-miljoen-varkens-sterven-nog-voor-de-slacht-2016.pdf.
Varona L., Noguera J. L., Casellas J., Martín de Hijas M., Rosas J. P., Ibáñez−Escriche N. (2020). A cross−specific multiplicative binomial recursive model for the analysis of perinatal mortality in a diallel cross among three varieties of Iberian pig. Sci. Rep. 10, 21190. doi: 10.1038/s41598-020-78346-7
Via Ritzau (2010). 25.000 pattegrise destrueres hver dag (Copenhagen: DR Danmarks Radio). Available at: https://www.dr.dk/nyheder/penge/25000-pattegrise-destrueres-hver-dag.
Villanueva-García D., Mota-Rojas D., Martínez-Burnes J., Olmos-Hernández A., Mora-Medina P., Salmerón C., et al. (2021). Hypothermia in newly born piglets: mechanisms of thermoregulation and pathophysiology of death. J. Anim. Behav. Biometeorology 9, 2101. doi: 10.31893/jabb.21001
Visscher P. M., Hemani G., Vinkhuyzen A. A. E., Chen G. B., Lee S. H., Wray N. R., et al. (2014). Statistical power to detect genetic (co)variance of complex traits using SNP data in unrelated samples. PloS Genet. 10 (4), e1004269. doi: 10.1371/journal.pgen.1004269
Voillet V., SanCristobal M., Père M. C., Billon Y., Canario L., Liaubet L., et al. (2018). Integrated analysis of proteomic and transcriptomic data highlights late fetal muscle maturation process. Mol. Cell. Proteomics 17, 672–693. doi: 10.1074/mcp.M116.066357
Vonnahme K. A., Wilson M. E., Ford S. P. (2002). Conceptus competition for uterine space: different strategies exhibited by the meishan and Yorkshire pig. J. Anim. Sci. 80, 1311–1316. doi: 10.2527/2002.8051311x
Wahlgren P. (2018). Factors influencing variation in birth weight and its impact on later performance in Swedish Yorkshire pigs (Swedish University of Agricultural Sciences). Available at: https://stud.epsilon.slu.se/13201.
Wattanaphansak S., Luengyosluechakul S., Larriestra A., Deen J. (2002). The impact of cross-fostering on swine production. Thai J. Veterinary Med. 32 (Suppl), 101–106.
Yao Y., Voillet V., Jegou M., SanCristobal M., Dou S., Romé V., et al. (2017). Comparing the intestinal transcriptome of meishan and Large white piglets during late fetal development reveals genes involved in glucose and lipid metabolism and immunity as valuable clues of intestinal maturity. BMC Genomics 18, 647. doi: 10.1186/s12864-017-4001-2
Yuan T. L., Zhu Y. H., Shi M., Li T. T., Li N., Wu G. Y., et al. (2015). Within-litter variation in birth weight: impact of nutritional status in the sow. J. Zhejiang Univ. Sci. B 16 (6), 417–435. doi: 10.1631/jzus.B1500010
Zaalberg R. M., Villumsen T. M., Jensen J., Chu T. T. (2022). Effective selection for lower mortality in organic pigs through selection for total number born and number of dead piglets. Animals 12, 1796. doi: 10.3390/ani12141796
Keywords: pig, breeding, welfare, litter size, survival, mortality, birth weight
Citation: Knap PW, Knol EF, Sørensen AC, Huisman AE, van der Spek D, Zak LJ, Granados Chapatte A and Lewis CRG (2023) Genetic and phenotypic time trends of litter size, piglet mortality, and birth weight in pigs. Front. Anim. Sci. 4:1218175. doi: 10.3389/fanim.2023.1218175
Received: 06 May 2023; Accepted: 15 June 2023;
Published: 20 July 2023.
Edited by:
Luca Fontanesi, University of Bologna, ItalyReviewed by:
David Solà-Oriol, Autonomous University of Barcelona, SpainFrancesco Tiezzi, University of Florence, Italy
Copyright © 2023 Knap, Knol, Sørensen, Huisman, van der Spek, Zak, Granados Chapatte and Lewis. This is an open-access article distributed under the terms of the Creative Commons Attribution License (CC BY). The use, distribution or reproduction in other forums is permitted, provided the original author(s) and the copyright owner(s) are credited and that the original publication in this journal is cited, in accordance with accepted academic practice. No use, distribution or reproduction is permitted which does not comply with these terms.
*Correspondence: Pieter W. Knap, cGlldGVyLmtuYXBAZ2VudXNwbGMuY29t